- 1Hospital S. Maria del Carmine, Azienda Provinciale per i Servizi Sanitari, Rovereto, Italy
- 2Laboratory of Functional Neuroimaging, Center for Mind/Brain Sciences, University of Trento, Trento, Italy
- 3Faculty of Medicine, University of Southampton, Southampton, United Kingdom
The cerebral vasculature is made up of highly specialized structures that assure constant brain perfusion necessary to meet the very high demand for oxygen and glucose by neurons and glial cells. A dense, redundant network of arteries is spread over the entire pial surface from which penetrating arteries dive into the cortex to reach the neurovascular units. Besides providing blood to the brain parenchyma, cerebral arteries are key in the drainage of interstitial fluid (ISF) and solutes such as amyloid-beta. This occurs along the basement membranes surrounding vascular smooth muscle cells, toward leptomeningeal arteries and deep cervical lymph nodes. The dense microvasculature is made up of fine capillaries. Capillary walls contain pericytes that have contractile properties and are lined by a highly specialized blood–brain barrier that regulates the entry of solutes and ions and maintains the integrity of the composition of ISF. They are also important for the production of ISF. Capillaries drain into venules that course centrifugally toward the cortex to reach cortical veins and empty into dural venous sinuses. The walls of the venous sinuses are also home to meningeal lymphatic vessels that support the drainage of cerebrospinal fluid, although such pathways are still poorly understood. Damage to macro- and microvasculature will compromise cerebral perfusion, hamper the highly synchronized movement of neurofluids, and affect the drainage of waste products leading to neuronal and glial degeneration. This review will present vascular anatomy, their role in fluid dynamics, and a summary of how their dysfunction can lead to neurodegeneration.
Introduction
Damage to cerebral vasculature and reduction in cerebral perfusion initiate a cascade of events that rapidly leads to disturbed cellular homeostasis and death of neurons and glial cells (1). The cerebral arterial network of vessels is unique in its anatomy, and its flow dynamics is inextricably intertwined with those of other fluids such as venous blood, cerebrospinal fluid (CSF), and the interstitial fluid (ISF) (2, 3). Emerging evidence regarding the role of cerebral vasculature in the drainage of solutes and fluids adds to the complexity of the overall interaction with neurofluids.
The arteries of the brain have a dual function: to supply oxygenated blood to neurons and glia and to drain ISF. Neurons and glial cells are constantly “at work,” even during rest, and this very high demand for oxygen and glucose requires a steady supply of oxygenated blood. Histological and tracer studies reveal the intricate relationship of cortical arteries with meningeal sheaths and the constitution of the perivascular compartment and spaces that provide a pathway for inflow and outflow of ISF (4–6). Cerebral capillaries are considered important sites of CSF and ISF production and absorption. Capillaries drain into venules that are hierarchically organized and run centrifugally toward the cortex. All venous drainage occurs through dural venous sinuses that drain toward the neck veins. The walls of dural venous sinuses are also home to meningeal lymphatic vessels (7, 8), with a role in the drainage of CSF. In this review, a brief overview of the current evidence for the anatomy and function of vessels in the brain will be provided, followed by a summary of mechanisms of interaction of what we term “neurofluids”: blood, CSF, and ISF (2). A disruption of such mechanisms will trigger a series of pathological events such as microvascular injury, failure of ISF drainage, local deposition of amyloid-beta as cerebral amyloid angiopathy (CAA), focal ischemia, and demyelination.
Arterial and Capillary Systems
The brain parenchyma is supplied by two internal carotid arteries (ICAs) and two vertebral arteries. The ICA enters the skull-base through the carotid canal, located in the petrous portion of the temporal lobe. It pierces through the dura mater at the level of the cavernous sinus and bifurcates within the subarachnoid space (SAS) into middle cerebral arteries and anterior cerebral arteries. The ICA carries ~80% of the total blood to the brain. The vertebral arteries enter the vertebral foramina at the level of C6; they exit out of C1 foramina, loop around the posterior arch of the atlas as they enter the foramen magna, and lies on the ventral surface of the brain stem to form the basilar artery (BA). The BA terminates into two posterior cerebral arteries. The anterior (ICA and its branches) and the posterior circulation (vertebral arteries and its branches) arteries come together at the base of the skull to form the circle of Willis that lies in the cisternal space (9).
A rich, anastomotic network of leptomeningeal arteries spreads over the pial surface from which numerous branches (arterioles) sprout out and pierce the glia limitans to dive into the cortex at approximately right angles to it. From a structural point of view, both pial arteries and penetrating arterioles lack an external elastic lamina, but leptomeningeal arteries retain an internal elastic lamina (10). The gray matter (GM) has a larger number of arterioles with respect to those in the white matter (WM) with a ratio of 8:1, which is proportionate to the elevated energy demand of the more cellular GM (11, 12). Penetrating arterioles are completely encased by a sheet of pia mater, which reflects off the cortical surface, separating them from the surrounding SAS and the brain parenchyma (4) (Figure 1). However, around the perivascular compartment of the arterioles in the WM, there are two such sheaths, creating a potential space for the accumulation of edematous fluid (13). At the capillary level, direct observations under the electron microscope in a variety of species reveal that the basement membrane of the pial sheath and the basement membranes of the astrocytes (glia limitans) fuse together to create a perivascular compartment, or periarterial space, filled with an extracellular matrix (ECM), which is not continuous with the SAS (4, 14) and referred to as the “perivascular space” (PVS) (Figure 1). Indeed, PVSs, or more appropriately the periarterial spaces, are not visible within the cortical GM even under pathological conditions, whereas they are seen in the WM both in histological specimens and on neuroimaging (13, 15). Changes in the walls of capillaries and arterioles associated with aging, hypertension, or diabetes mellitus lead to small vessel disease (SVD) and vascular dementia (16, 17).
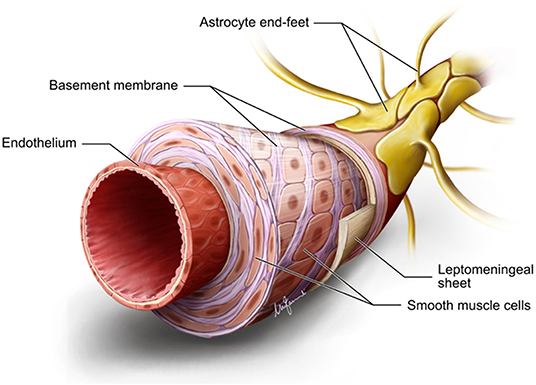
Figure 1. Diagrammatic summary of the structure of an arteriole in the gray matter. Endothelium hosts the blood–brain barrier. There are several layers of smooth muscle cells separated by basement membranes. Adventitial leptomeningeal sheath has its own basement membrane that fuses with the basement membrane of astrocyte end feet to form a perivascular compartment or perivascular space. Diagram drawn by Marco Fanuli.
Pial surface arterial networks are richly innervated by sympathetic nerves from the superior cervical ganglion, sphenopalatine, otic, and trigeminal ganglia that release several neurotransmitters and neuromodulators such as a vasoactive intestinal peptide, nitric oxide synthase, acetylcholine, norepinephrine, and substance P (18). This innervation, also termed “extrinsic” innervation, ends in the precapillary segment and, more precisely, where the PVS terminates. The extrinsic innervation is primarily responsible for a prompt myogenic response to temporary pressure differences. According to Poiseuille's law, a change in radius directly affects resistance to flow to the fourth power, thereby modulating blood flow instantly and efficaciously (19). Intraparenchymal arterioles are innervated by nerves arising from the nuclei of basal forebrain such as the locus coeruleus, nucleus basalis of Meynert, and raphe nuclei in the brain stem that release norepinephrine, acetylcholine, and 5-hydroxythyrosine as well as other neuropeptides either directly to the walls of capillaries or indirectly via local interneurons and astrocytes (18, 20). Such nerve endings are likely to control the intrinsic spontaneous contractile activity of vascular smooth muscle cells (VSMCs) in the tunica media, also termed “vasomotion.” Vasomotor oscillations form the basis of ultra-slow 0.1-Hz wave activity in the microvasculature independent of neuronal activity (21, 22).
A dense capillary anastomotic network characterizes the GM and varies with its depth (23). Approximately 50–60% of total blood volume is within the capillaries (23). Capillary walls are made up of a single layer of endothelial cells, pericytes, and a basal lamina made up of collagen type IV, heparan sulfate proteoglycans, laminin, fibronectin, and other ECM proteins, in various proportions and with different isoforms depending on the type of vessel (24–26). The endothelial cells are bound together by tight junction proteins such as claudins and occludins, creating a highly regulated blood–brain barrier (BBB) that restricts transcellular flux of ions and hydrophilic solutes, shielding the internal parenchymal milieu from even the slightest fluctuations in the osmolarity of surrounding tissues and blood plasma (27, 28). The endothelium contains a spectrum of receptors essential for the entry and efflux of peptides, such as low-density lipoprotein related protein-1 or adenosine triphosphate-binding cassette transporters, which are essential for the efflux of soluble amyloid-beta from the brain parenchyma (29). The abluminal surface of the capillaries is continuous with astrocytic end feet (or glia limitans), containing aquaporin-4 (AQP-4) water channels.
Venous System
The parenchymal microvasculature drains deoxygenated blood centrifugally from the ventricular ependymal surface toward the cortical pial surface, via medullary venules and veins arranged hierarchically and centrifugally from the ventricular ependymal wall toward the cortex (30). Large cortical bridging veins, such as the vein of Labbè and the Trolard vein, empty into the superficial dural venous sinuses (31). The superior sagittal sinus subdivides into right and left transverse sinus and continues directly via sigmoid sinuses into the internal jugular veins, extracranial neck vessels, and the intra- and extra-spinal venous plexi, conveying deoxygenated blood to the right atrium (32, 33). Deep internal veins form the inferior sagittal sinus, the vein of Galen, and the straight sinus to drain into the superior sagittal sinus posteriorly. Anterior venous drainage occurs through the cavernous sinus, sphenopetrosal sinuses, and sigmoid sinuses. Several anatomical variations exist, and veins can vary in number, size, symmetry across hemispheres, and their extracranial venous drainage patterns, adding to the complexity of the cerebral venous system. It is important to note that dural venous sinuses are valveless, making cephalad retrograde flow possible in cases of obstruction to downward flow (34).
Surrounding each parenchymal arteriole are eight venules (5). Venules typically have a larger lumen area and a thinner vessel wall with respect to arterioles (35). Exiting venules in the cortex are surrounded by an incomplete layer of pia mater (4). Paravenous spaces communicate with the SAS directly. The first reports of the presence of lymphatic vessels in the dura mater were reported in 1787, whereas histologic evidence of their existence was provided much later (36). More recently, lymphatic channels were described lining the dural venous sinuses that appear to be additional routes for the drainage of fluids and cells toward the deep cervical lymph nodes (7, 8). Lymphatic channels are also found in the cribriform plate that drains fluids, cells, and solutes via nasal lymphatics toward the superficial cervical lymph nodes (37).
Production and Drainage of Cerebrospinal Fluid and Interstitial Fluid
Our classic understanding of CSF fluid production and absorption is being challenged, as new evidence suggests that CSF production also occurs at other sites such as the capillary endothelial surface, as formulated by the Bulat–Klarica–Orešković hypothesis (38). Almost 80% of CSF is secreted by fenestrated capillaries in the choroid plexi at a rate of ~0.3–0.4 ml/min for a total production of 430–580 ml daily. CSF secretion across the blood–CSF barrier depends on hydrostatic and osmolarity gradients that exist between the plasma and the intraventricular CSF fluids. CSF comprises 99% water, some ions, and negligible quantities of proteins and glucose. Arachnoid granulations found in the dural venous sinuses are traditionally recognized to play a prime role in CSF reabsorption. However, the contemporary presence of the meninx primitiva and the lack of arachnoid granulations in the fetus suggests that there must be alternative routes for its absorption (37, 39).
There are multiple sources of ISF production, such as filtration across the capillaries via the development of hydrostatic and osmotic pressures across the endothelium, secretion through choroid plexi, and cellular metabolism (40, 41). ISF fills the extracellular space (ECS) or interstitial space. This space contains an ECM made up of glycosaminoglycans, glycoproteins (e.g., laminins, collagen, chondroitin, fibronectin) and proteoglycans (e.g., hyaluronic acid, heparan sulfate). Such an environment determines a negatively charged ambient necessary for cellular communication, volume transmission, immunosurveillance, and a binding capacity for solutes to be transported around brain areas. ECS occupies ~15–20% of the total brain volume, and this volume can change in physiologic and pathologic conditions such as sleep, under anesthesia, and stroke (42–45). ISF is also the primary fluid medium for waste removal; however, the presence of BBB notably restricts the movement of proteins across the capillaries, which suggested that there must be alternative pathways. Bulk flow of ISF through the brain parenchyma was proposed as a route to flush out waste products and fluids toward the ventricular ependymal walls (46). In the past decade, multiple waste clearance pathways have been characterized in the brain: the glymphatic pathway, intramural periarterial drainage pathway (IPAD), flow along cranial nerves, and meningeal lymphatics along the dural venous sinuses (6, 39, 47), still extensively debated (48, 49). The glymphatic system proposes that CSF from the SAS recycles along the para-arterial spaces and enters the brain tissue via astrocytic AQP-4 water channels. CSF intermingles with ISF, which flows toward paravenous spaces via bulk flow, thus flushing out fluids and solutes from the brain (50, 51). However, diffusion rather than bulk flow may be the likely principal mechanism for flow with an unclear role for AQP-4 channels (40, 52–55). Also, the mechanism of unidirectional CSF flow along intraparenchymal para-arterial spaces remains debatable, as arterial pulsations alone do not determine such flow (56). Furthermore, the glymphatic hypothesis does not explain why in CAA, the deposition of proteins occurs in the tunica media of arterioles and arteries, spreading to occupy the whole of the arterial wall and rarely involves veins (57–59).
On the other hand, tracer injection studies in animal brains have unequivocally demonstrated that one important route for ISF and solute removal is the IPAD. For decades, perivascular compartments have been considered to play a fundamental role in the removal of waste products (36, 60, 61). According to this mechanism, fluids and waste products flow within the basement membranes of arterioles and arteries in the opposite direction to arterial blood flow within their lumen and is primarily driven by vasomotion (62–65). The ultraslow frequency oscillation (<0.1 Hz) appears to be critical to the clearance of solutes. Electrophysiologically observed slow-wave oscillations characteristic of sleep are intricately associated with large CSF flow oscillations suggestive of vasomotion driven clearance of CSF and thereby of solutes and supportive of IPAD pathways of clearance (66).
Neurofluid Physiology
To understand the interaction between the several space-competing compartments within the cranium, we must remind ourselves of the Monro–Kellie hypothesis, which remains a cardinal principle in the understanding of fluid movements (67). This hypothesis maintains that because the brain contents are enclosed in a non-expandable bony skull, the total brain volume must remain constant at all times to avoid a dangerous increase in ICP (68). However, with the recent discoveries of meningeal lymphatics and the understanding of mechanisms for brain waste clearance mechanisms, it has become necessary to revisit the original Monro–Kellie doctrine (69). With every systole, an increase in arterial pressure pumps ~700 ml of oxygenated blood, causing inflation of arteries, arterioles, and the microvascular bed (70). This expansion of vessels will squeeze ISF and CSF from the interstitium and promote flow. The creation of a pressure gradient between the cranial SAS and the spinal SAS will cause displacement of CSF toward the spinal SAS and facilitate venous outflow toward the extracranial neck vessels (3, 71). During diastole, as the elastic vessels relax, CSF flows back with little net forward displacement. Such pulsatile forces will also create a variable magnitude of brain tissue deformation, generating additional forces affecting blood flow, production, and absorption of ISF and CSF. The intrinsic viscoelasticity of the brain, or brain compliance, is the capacity of brain tissue to deform in conditions of intracranial pressure changes. Such mechanical and viscoelastic properties vary in different brain regions and depend on cellular morphology, capillary distribution, the compactness of white matter axons, their orientation, and ECM composition (72). These properties are different both at a macroscale (WM is stiffer than GM) and at a microscale (cortical GM is stiffer than hippocampal GM; WM in the corpus callosum is stiffer than WM in the corona radiata) (72). WM is three times stiffer than GM, accounting for differential response to compression load (73). Physiologic rheological properties of the brain can be measured in vivo by magnetic resonance elastography (74, 75). Thus, in one magnetic resonance elastography study, the compression of internal jugular veins in the neck was shown to increase CSF pulsatility in the brain and increase stiffness within the brain parenchyma in accordance with the Monro–Kellie doctrine (75).
Cerebrovascular Damage and Neurodegeneration
Our attention is drawn to the intricate coupling of arterial, venous, CSF, and brain parenchymal dynamics; damage to any one of them can initiate a cascade of events affecting clearance of waste products in the brain and lead thereby to neurodegeneration. Reduced cerebral perfusion is considered a potential link between vascular risk factors and the development of SVD, vascular dementia, and Alzheimer's disease (AD) (76). The most important risk factors are advancing age and hypertension, both of which will hamper cerebral blood flow by directly damaging arterial walls and the microvasculature. Patients with SVD and AD often present with increased arterial stiffness, altered BBB permeability, VSMC loss, multiple fenestrations in the internal elastic lamina, remodeled arterial wall basement membranes, pericyte degeneration, increased intercapillary distance, reduced capillary density, increased arteriolar tortuosity, and swelling of astrocyte end feet, ultimately reducing the capacity for an optimal exchange of substances across the capillary endothelium (77–80). The inefficient transfer of pulsatile energy from the arterial bed toward the capillaries and the venous walls will disrupt hydrostatic forces. Arterial vasomotion will also be affected in several ways: direct arterial wall damage, deposition of amyloid-beta, and loss of cholinergic innervation of VSMCs. The geometry of ECS changes with age and disease, as free water within the parenchyma increases and toxic solutes such as amyloid-beta deposit within the extracellular space (81). In this scenario, the glymphatic/convective influx as well as IPAD will be hampered.
As the density of capillaries is lower in the white matter than in the gray matter and capillary basement membranes are the entry portals for IPAD by which ISF and solutes drain from brain tissue, the shortage of capillaries in the white matter may be a factor in a reduced capacity for IPAD in the white matter (82). Obstruction of CSF drainage from the cerebral ventricles results in dilatation of the ventricular system and the accumulation of fluid in the periventricular white matter in the acute stages of hydrocephalus with the slowly progressive destruction of white matter fibers and gliosis, suggesting that the capacity for IPAD is lower in the white matter compared with the gray matter (83).
Damage to veins, venules, and capillaries can also characterize other subtypes of SVD, such as perivenous collagenosis (84). This is characterized by concentric thickening of venular walls and pathological deposition of collagen resulting in leukoaraiosis or white matter hyperintensities on magnetic resonance imaging. Occlusion of venules and veins causes hypoperfusion and ischemia and affects the drainage of CSF via meningeal lymphatics (85).
There are several, albeit nonspecific, magnetic resonance imaging biomarkers such as dilated PVS, white matter hyperintensity, cerebral microbleeds, and superficial siderosis that characterize SVD, AD, and CAA that are an expression of impaired clearance of proteins and fluids, focal ischemia, and deposition of amyloid-beta within the walls of capillaries and neurodegeneration (82, 86–89). Neural tissue can become stiffer via several processes such as Wallerian degeneration, axonal atrophy, loss of oligodendroglial cells, microglial activation, neuroinflammation, and microvascular damage, resulting in a range of microstructural changes from increased tissue water content to progressive gliosis and loss of volume.
There is substantial evidence that fluid movements in the brain are related such that damage to one compartment can lead to several events leading to neuroglial vascular compromise (Figure 2). In particular, the morphological damage to macro/microvasculature or their dysfunction will most likely compromise the movement of fluids, with impact on the perfusion of the brain and the drainage of CSF, ISF, altering the homeostasis of the brain, which in turn leads to neuronal cell loss and dementia.
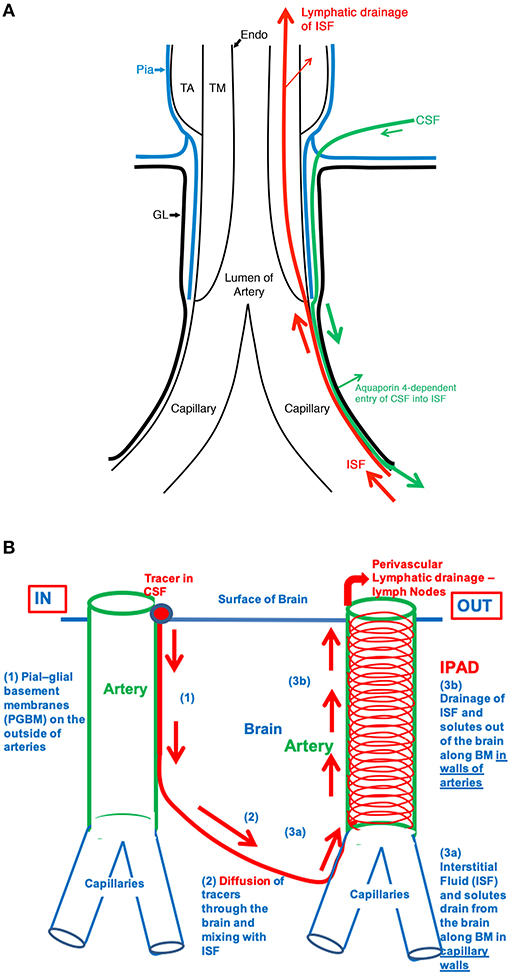
Figure 2. (A) The fine anatomy of the cerebral arterial wall. An artery is lined by endothelium (Endo) and coated by the tunica media (TM) composed of smooth muscle cells and by the outermost tunica adventitia (TA) composed of connective tissue. As it enters the brain, the artery loses the tunica adventitia but is still coated by a layer of pia-arachnoid (Pia) that intervenes between the artery and the glia limitans (GL) of the brain. As the arteriole divides into capillaries, the tunica media, and the layer of pia mater are lost. Thus, at the level of the capillary, the GL is in direct contact with the wall of the capillary. (B) Schematic representation of the IPAD and convective influx/glymphatic systems of the brain. On the left-hand side of the diagram, an artery enters the brain from the SAS, and an arteriole divides into capillaries. Tracers in the CSF enter the brain along the pial-glial basement membrane (1) between the pia mater and the GL (indicated by a green arrow) and enter the brain parenchyma and interstitial fluid by an aquaporin four-dependent mechanism, which is the glymphatic pathway (2). On the right-hand side of the diagram, the red arrows indicate the intramural perivascular lymphatic drainage pathway by which interstitial fluid (ISF) and solutes pass out of the brain along basement membranes in the walls of capillaries (3a) and along basement membranes surrounding smooth muscle cells in the tunica media of arterioles and arteries (3b). Reproduced with permission from Morris et al. (90)
Author Contributions
NA wrote the manuscript. RC edited the manuscript. All authors contributed to the article and approved the submitted version.
Conflict of Interest
The authors declare that the research was conducted in the absence of any commercial or financial relationships that could be construed as a potential conflict of interest.
Acknowledgments
The authors thank the Stroke Association of the United Kingdom for financial support.
References
1. Iadecola C, Nedergaard M. Glial regulation of the cerebral microvasculature. Nat Neurosci. (2007) 10:1369–76. doi: 10.1038/nn2003
2. Agarwal N, Contarino C, Toro EF. Neurofluids: a holistic approach to their physiology, interactive dynamics and clinical implications for neurological diseases. Veins Lymphat. (2019) 8:49–58. doi: 10.4081/vl.2019.8470
3. Beggs CB. Cerebral venous outflow and cerebrospinal fluid dynamics. Veins Lymphat. (2014) 3:1–8. doi: 10.4081/vl.2014.1867
4. Zhang ET, Inman CBE, Weller RO. Interrelationships of the pia mater and the perivascular (Virchow-Robin) spaces in the human cerebrum. J Anat. (1990) 170:111–23.
5. Marín-Padilla M. The human brain intracerebral microvascular system: development and structure. Neuroanat. (2012) 6:38. doi: 10.3389/fnana.2012.00038
6. Carare RO, Bernardes-Silva M, Newman TA, Page AM, Nicoll JAR, Perry VH, et al. Solutes, but not cells, drain from the brain parenchyma along basement membranes of capillaries and arteries: significance for cerebral amyloid angiopathy and neuroimmunology. Neuropathol Appl Neurobiol. (2008) 34:131–44. doi: 10.1111/j.1365-2990.2007.00926.x
7. Louveau A, Smirnov I, Keyes TJ, Eccles JD, Rouhani SJ, Peske JD, et al. Structural and functional features of central nervous system lymphatic vessels. Nature. (2015) 523:337–41. doi: 10.1038/nature14432
8. Aspelund A, Antila S, Proulx ST, Karlsen TV, Karaman S, Detmar M, et al. A dural lymphatic vascular system that drains brain interstitial fluid and macromolecules. J Exp Med. (2015) 212:991–9. doi: 10.1084/jem.20142290
9. Agarwal N, Port JD. Neuroimaging: Anatomy Meets Function. Switzerland: Springer International (2017).
10. Hill MA, Nourian Z, Ho IL, Clifford PS, Martinez-Lemus L, Meininger GA. Small artery elastin distribution and architecture—focus on three dimensional organization. Microcirculation. (2016) 23:614–20. doi: 10.1111/micc.12294
11. Blinder P, Tsai PS, Kaufhold JP, Knutsen PM, Suhl H, Kleinfeld D. The cortical angiome: an interconnected vascular network with noncolumnar patterns of blood flow. Nat Neurosci. (2013) 16:889–97. doi: 10.1038/nn.3426
12. Tsai PS, Kaufhold JP, Blinder P, Friedman B, Drew PJ, Karten HJ, et al. Correlations of neuronal and microvascular densities in murine cortex revealed by direct counting and colocalization of nuclei and vessels. J Neurosci. (2009) 29:14553–70. doi: 10.1523/JNEUROSCI.3287-09.2009
13. MacGregor Sharp M, Bulters D, Brandner S, Holton J, Verma A, Werring DJ, et al. The fine anatomy of the perivascular compartment in the human brain: relevance to dilated perivascular spaces in cerebral amyloid angiopathy. Neuropathol Appl Neurobiol. (2018) 45:305–8. doi: 10.1111/nan.12480
14. Hutchings M, Weller RO. Anatomical relationships of the pia mater to cerebral blood vessels in man. J Neurosurg. (1986) 65:316–25. doi: 10.3171/jns.1986.65.3.0316
15. Wardlaw JM, Smith EE, Biessels GJ, Cordonnier C, Fazekas F, Frayne R, et al. Neuroimaging standards for research into small vessel disease and its contribution to ageing and neurodegeneration. Lancet Neurol. (2013) 12:822–38. doi: 10.1016/S1474-4422(13)70124-8
16. Hase Y, Polvikoski TM, Firbank MJ, Craggs LJL, Hawthorne E, Platten C, et al. Small vessel disease pathological changes in neurodegenerative and vascular dementias concomitant with autonomic dysfunction. Brain Pathol. (2020) 30:191–202. doi: 10.1111/bpa.12769
17. Horsburgh K, Wardlaw JM, Van Agtmael T, Allan SM, Ashford MLJ, Bath PM, et al. Small vessels, dementia and chronic diseases - molecular mechanisms and pathophysiology. Clin Sci. (2018) 132:851–68. doi: 10.1042/CS20171620
18. Hamel E. Perivascular nerves and the regulation of cerebrovascular tone. J Appl Physiol. (2006) 100:1059–64. doi: 10.1152/japplphysiol.00954.2005
19. Fantini S, Sassaroli A, Tgavalekos KT, Kornbluth J. Cerebral blood flow and autoregulation: current measurement techniques and prospects for noninvasive optical methods. Neurophotonics. (2016) 3:031411. doi: 10.1117/1.NPh.3.3.031411
20. Toussay X, Basu K, Lacoste B, Hamel E. Locus coeruleus stimulation recruits a broad cortical neuronal network and increases cortical perfusion. J Neurosci. (2013) 33:3390–401. doi: 10.1523/JNEUROSCI.3346-12.2013
21. Rayshubskiy A, Wojtasiewicz TJ, Mikell CB, Bouchard MB, Timerman D, Youngerman BE, et al. Direct, intraoperative observation of ~0.1Hz hemodynamic oscillations in awake human cortex: implications for fMRI. Neuroimage. (2014) 87:323–31. doi: 10.1016/j.neuroimage.2013.10.044
22. Zanatta P, Toffolo GM, Sartori E, Bet A, Baldanzi F, Agarwal N, et al. The human brain pacemaker: synchronized infra-slow neurovascular coupling in patients undergoing non-pulsatile cardiopulmonary bypass. Neuroimage. (2013) 72:10–9. doi: 10.1016/j.neuroimage.2013.01.033
23. Gould IG, Tsai P, Kleinfeld D, Linninger A. The capillary bed offers the largest hemodynamic resistance to the cortical blood supply. J Cereb Blood Flow Metab. (2016) 37:52–68. doi: 10.1177/0271678X16671146
24. Di Russo J, Hannocks MJ, Luik AL, Song J, Zhang X, Yousif L, et al. Vascular laminins in physiology and pathology. Matrix Biol. (2017) 57–8:140–8. doi: 10.1016/j.matbio.2016.06.008
25. Yousif LF, Di Russo J, Sorokin L. Laminin isoforms in endothelial and perivascular basement membranes. Cell Adhes Migr. (2013) 7:101–10. doi: 10.4161/cam.22680
26. Abbott NJ, Rönnbäck L, Hansson E. Astrocyte-endothelial interactions at the blood-brain barrier. Nat Rev Neurosci. (2006) 7:41–53. doi: 10.1038/nrn1824
27. Searson PC. The blood-brain barrier: an engineering perspective. Neuroeng. (2013) 6:7. doi: 10.3389/fneng.2013.00007
28. Hannocks MJ, Pizzo ME, Huppert J, Deshpande T, Abbott NJ, Thorne RG, et al. Molecular characterization of perivascular drainage pathways in the murine brain. J Cereb Blood Flow Metab. (2018) 38:669–86. doi: 10.1177/0271678X17749689
29. Bell RD, Sagare AP, Friedman AE, Bedi GS, Holtzman DM, Deane R, et al. Transport pathways for clearance of human alzheimer's amyloid β-peptide and apolipoproteins E and J in the mouse central nervous system. J Cereb Blood Flow Metab. (2007) 27:909–18. doi: 10.1038/sj.jcbfm.9600419
30. Taoka T, Fukusumi A, Miyasaka T, Kawai H, Nakane T, Kichikawa K, et al. Structure of the medullary veins of the cerebral hemisphere and related disorders. Radiographics. (2017) 37:281–97. doi: 10.1148/rg.2017160061
31. Schmidek H, Auer LM, Kapp JP. The cerebral venous system. Neurosurgery. (1985) 17:663–78. doi: 10.1227/00006123-198510000-00024
32. Batson O. The function of the vertebral veins and their role in the spread of metastases. Clin Orthop Rel Res. (1995) 112:138–49. doi: 10.1097/00000658-194007000-00016
34. Kiliç T, Akakin A. Anatomy of cerebral veins and sinuses. Front Neurol Neurosci. (2008) 23:4–15. doi: 10.1159/000111256
35. MacGregor Sharp M, Criswell TP, Dobson H, Finucane C, Verma A, Carare RO. Solving an old dogma: is it an arteriole or a venule? Front Aging Neurosci. (2019) 11:289. doi: 10.3389/fnagi.2019.00289
36. Földi M, Gellért A, Kozma M, Poberai M, Zoltán OT, Csanda E. New contributions to the anatomical connections of the brain and the lymphatic system. Acta Anat. (1966) 64:498–505. doi: 10.1159/000142849
37. Weller RO, Sharp MM, Christodoulides M, Carare RO, Møllgård K. The meninges as barriers and facilitators for the movement of fluid, cells and pathogens related to the rodent and human CNS. Acta Neuropathol. (2018) 135:363–85. doi: 10.1007/s00401-018-1809-z
38. Orešković D, Radoš M, Klarica M. New concepts of cerebrospinal fluid physiology and development of hydrocephalus. Pediatr Neurosurg. (2016) 52:417–25. doi: 10.1159/000452169
39. Ahn JH, Cho H, Kim J-H, Kim SH, Ham J-S, Park I, et al. Meningeal lymphatic vessels at the skull base drain cerebrospinal fluid. Nature. (2019) 572:1–29. doi: 10.1038/s41586-019-1419-5
40. Abbott NJ. Evidence for bulk flow of brain interstitial fluid: significance for physiology and pathology. Neurochem Int. (2004) 45:545–52. doi: 10.1016/j.neuint.2003.11.006
41. Brinker T, Stopa E, Morrison J, Klinge P. A new look at cerebrospinal fluid circulation. Fluids Barriers CNS. (2014) 11:10. doi: 10.1186/2045-8118-11-10
42. Syková E, Nicholson C. Diffusion in brain extracellular space. Physiol Rev. (2008) 88:1277–340. doi: 10.1152/physrev.00027.2007
43. Hablitz LM, Vinitsky HS, Sun Q, Stæger FF, Sigurdsson B, Mortensen KN, et al. Increased glymphatic influx is correlated with high EEG delta power and low heart rate in mice under anesthesia. Sci Adv. (2019) 5:eaav5447. doi: 10.1126/sciadv.aav5447
44. Hauglund NL, Pavan C, Nedergaard M. Cleaning the sleeping brain – the potential restorative function of the glymphatic system. Curr Opin Physiol. (2020) 15:1–6. doi: 10.1016/j.cophys.2019.10.020
45. Xie L, Kang H, Xu Q, Chen MJ, Liao Y, Thiyagarajan M, et al. Sleep drives metabolite clearance from the adult brain. Science (80-). (2013) 342:373–7. doi: 10.1126/science.1241224
46. Cserr HF. Role of secretion and bulk flow of brain interstitial fluid in brain volume regulation. Ann N Y Acad Sci. (1988) 529:9–20. doi: 10.1111/j.1749-6632.1988.tb51415.x
47. Iliff JJ, Wang M, Liao Y, Plogg BA, Peng W, Gundersen GA, et al. A paravascular pathway facilitates CSF flow through the brain parenchyma and the clearance of interstitial solutes, including amyloid β. Sci Transl Med. (2012) 4:147ra111. doi: 10.1126/scitranslmed.3003748
48. Hladky SB, Barrand MA. Elimination of substances from the brain parenchyma: efflux via perivascular pathways and via the blood–brain barrier. Fluids Barriers CNS. (2018) 15:1–73. doi: 10.1186/s12987-018-0113-6
49. Tarasoff-Conway JM, Carare RO, Osorio RS, Glodzik L, Butler T, Fieremans E, et al. Clearance systems in the brain—implications for alzheimer disease. Nat Rev Neurol. (2015) 11:457–70. doi: 10.1038/nrneurol.2015.119
50. Iliff JJ, Wang M, Zeppenfeld DM, Venkataraman A, Plog BA, Liao Y, et al. Cerebral arterial pulsation drives paravascular CSF-interstitial fluid exchange in the murine brain. J Neurosci. (2013) 33:18190–9. doi: 10.1523/JNEUROSCI.1592-13.2013
51. Benveniste H, Lee H, Volkow ND. The glymphatic pathway: waste removal from the CNS via cerebrospinal fluid transport. Neurosci. (2016) 23:454–65. doi: 10.1177/1073858417691030
52. Asgari M, De Zélicourt D, Kurtcuoglu V. Glymphatic solute transport does not require bulk flow. Sci Rep. (2016) 6:38635. doi: 10.1038/srep38635
53. Ray LA, Heys JJ. Fluid flow and mass transport in brain tissue. Fluids. (2019) 4:133–96. doi: 10.3390/fluids4040196
54. Martinac AD, Bilston LE. Computational modelling of fluid and solute transport in the brain. Biomech Model Mechanobiol. (2020) 19:781–800. doi: 10.1007/s10237-019-01253-y
55. Faghih MM, Sharp MK. Is bulk flow plausible in perivascular, paravascular and paravenous channels? Fluids Barriers CNS. (2018) 15:1–10. doi: 10.1186/s12987-018-0103-8
56. Jin BJ, Smith AJ, Verkman AS. Spatial model of convective solute transport in brain extracellular space does not support a “glymphatic” mechanism. J Gen Physiol. (2016) 148:489–501. doi: 10.1085/jgp.201611684
57. Attems J, Jellinger K, Thal DR, Van Nostrand W. Review: sporadic cerebral amyloid angiopathy. Neuropathol Appl Neurobiol. (2011) 37:75–93. doi: 10.1111/j.1365-2990.2010.01137.x
58. Carare RO, Hawkes CA, Jeffrey M, Kalaria RN, Weller RO. Review: cerebral amyloid angiopathy, prion angiopathy, CADASIL and the spectrum of protein elimination failure angiopathies (PEFA) in neurodegenerative disease with a focus on therapy. Neuropathol Appl Neurobiol. (2013) 39:593–611. doi: 10.1111/nan.12042
59. Keable A, Fenna K, Yuen HM, Johnston DA, Smyth NR, Smith C, et al. Deposition of amyloid β in the walls of human leptomeningeal arteries in relation to perivascular drainage pathways in cerebral amyloid angiopathy. Biochim Biophys Acta. (2016) 1862:1037–46. doi: 10.1016/j.bbadis.2015.08.024
60. Casley Smith JR, Foldi Borcsok E, Foldi M. The prelymphatic pathways of the brain as revealed by cervical lymphatic obstruction and the passage of particles. Br J Exp Pathol. (1976) 57:179–88.
61. Weller RO, Kida S, Zhang ET. Pathways of fluid drainage from the brain–morphological aspects and immunological significance in rat and man. Brain Pathol. (1992) 2:277–84. doi: 10.1111/j.1750-3639.1992.tb00704.x
62. Diem AK, Tan M, Bressloff NW, Hawkes C, Morris AWJ, Weller RO, et al. A simulation model of periarterial clearance of amyloid-β from the brain. Front Aging Neurosci. (2016) 8:18. doi: 10.3389/fnagi.2016.00018
63. Sharp MK, Diem AK, Weller RO, Carare RO. Peristalsis with oscillating flow resistance: a mechanism for periarterial clearance of amyloid beta from the brain. Ann Biomed Eng. (2015) 44:1–13. doi: 10.1007/s10439-015-1457-6
64. Carare RO, Aldea R, Bulters D, Alzetani A, Birch AA, Richardson G, et al. Vasomotion drives periarterial drainage of Aβ from the brain. Neuron. (2020) 105:400–1. doi: 10.1016/j.neuron.2020.01.011
65. van Veluw SJ, Hou SS, Calvo-Rodriguez M, Arbel-Ornath M, Snyder AC, Frosch MP, et al. Vasomotion as a driving force for paravascular clearance in the awake mouse brain. Neuron. (2020) 105:549–61.e5. doi: 10.1016/j.neuron.2019.10.033
66. Fultz NE, Bonmassar G, Setsompop K, Stickgold RA, Rosen BR, Polimeni JR, et al. Coupled electrophysiological, hemodynamic, and cerebrospinal fluid oscillations in human sleep. Science (80-). (2019) 366:628–31. doi: 10.1126/science.aax5440
67. Kellie G. An account of the appearances observed in the dissection of two of three individuals presumed to have perished in the storm of the 3d, and whose bodies were discovered in the vicinity of Leith on the morning of the 4th, November 1821 : with some reflectio. Trans Med Chir Soc Edinb. (1824) 1:84–122.
68. Mokri B. The monro-kellie hypothesis: applications in CSF volume depletion. Neurology. (2001) 56:1746–8. doi: 10.1212/WNL.56.12.1746
69. Wilson MH. Monro-Kellie 2.0: the dynamic vascular and venous pathophysiological components of intracranial pressure. J Cereb Blood Flow Metab. (2016) 36:1338–50. doi: 10.1177/0271678X16648711
70. Linninger AA, Tangen K, Hsu C-Y, Frim D. Cerebrospinal fluid mechanics and its coupling to cerebrovascular dynamics. Annu Rev Fluid Mech. (2016) 48:219–57. doi: 10.1146/annurev-fluid-122414-034321
71. Greitz D, Wirestam R, Franck A, Nordell B, Thomsen C, Ståhlberg F. Pulsatile brain movement and associated hydrodynamics studied by magnetic resonance phase imaging. The monro-kellie doctrine revisited. Neuroradiology. (1992) 34:370–80. doi: 10.1007/BF00596493
72. Lee SJ, King MA, Sun J, Xie HK, Subhash G, Sarntinoranont M. Measurement of viscoelastic properties in multiple anatomical regions of acute rat brain tissue slices. J Mech Behav Biomed Mater. (2014) 29:213–24. doi: 10.1016/j.jmbbm.2013.08.026
73. Budday S, Nay R, De Rooij R, Steinmann P, Wyrobek T, Ovaert TC, et al. Mechanical properties of gray and white matter brain tissue by indentation. J Mech Behav Biomed Mater. (2015) 46:318–30. doi: 10.1016/j.jmbbm.2015.02.024
74. Kruse SA, Rose GH, Glaser KJ, Manduca A, Felmlee JP, Jack CR, et al. Magnetic resonance elastography of the brain. Neuroimage. (2008) 39:231–7. doi: 10.1016/j.neuroimage.2007.08.030
75. Hatt A, Cheng S, Tan K, Sinkus R, Bilston LE. MR elastography can be used to measure brain stiffness changes as a result of altered cranial venous drainage during jugular compression. AJNR Am J Neuroradiol. (2015) 36:1971–7. doi: 10.3174/ajnr.A4361
76. Sweeney MD, Kisler K, Montagne A, Toga AW, Zlokovic BV. The role of brain vasculature in neurodegenerative disorders. Nat Neurosci. (2018) 21:1318–31. doi: 10.1038/s41593-018-0234-x
77. Kalaria RN, Pax AB. Increased collagen content of cerebral microvessels in Alzheimer's disease. Brain Res. (1995) 705:349–52. doi: 10.1016/0006-8993(95)01250-8
78. Vanherle L, Matuskova H, Don-Doncow N, Uhl FE, Meissner A. Improving cerebrovascular function to increase neuronal recovery in neurodegeneration associated to cardiovascular disease. Front Cell Dev Biol. (2020) 8:1–8. doi: 10.3389/fcell.2020.00053
79. Wolters FJ, Zonneveld HI, Hofman A, Van Der Lugt A, Koudstaal PJ, Vernooij MW, et al. Cerebral perfusion and the risk of dementia: a population-based study. Circulation. (2017) 136:719–28. doi: 10.1161/CIRCULATIONAHA.117.027448
80. Montagne A, Barnes SR, Sweeney MD, Halliday MR, Sagare AP, Zhao Z, et al. Blood-brain barrier breakdown in the aging human hippocampus. Neuron. (2015) 85:296–302. doi: 10.1016/j.neuron.2014.12.032
81. Dumont M, Roy M, Jodoin PM, Morency FC, Houde JC, Xie Z, et al. Free water in white matter differentiates MCI and AD from control subjects. Front Aging Neurosci. (2019) 11:270. doi: 10.3389/fnagi.2019.00270
82. Sharp MM, Saito S, Keable A, Gatherer M, Aldea R, Agarwal N, et al. Demonstrating a reduced capacity for removal of fluid from cerebral white matter and hypoxia in areas of white matter hyperintensity associated with age and dementia. Acta Neuropathol Commun. (2020) 1:1–14. doi: 10.1186/s40478-020-01009-1
83. Weller RO, Wisniewski H, Shulman K, Terry RD. Experimental hydrocephalus in young dogs: histological and ultrastructural study of the brain tissue damage. J Neuropathol Exp Neurol. (1971) 30:613–26. doi: 10.1097/00005072-197110000-00006
84. Moody DM, Brown WR, Challa VR, Anderson RL. Periventricular venous collagenosis: association with leukoaraiosis. Radiology. (1995) 194:469–76. doi: 10.1148/radiology.194.2.7824728
85. Nan D, Cheng Y, Feng L, Zhao M, Ma D, Feng J. Potential mechanism of venous system for leukoaraiosis: from post-mortem to in vivo research. Neurodegener Dis. (2020) 130021:101–8. doi: 10.1159/000505157
86. Banerjee G, Kim HJ, Fox Z, Jäger HR, Wilson D, Charidimou A, et al. MRI-visible perivascular space location is associated with alzheimer's disease independently of amyloid burden. Brain. (2017) 140:1107–16. doi: 10.1093/brain/awx003
87. Hurford R, Charidimou A, Fox Z, Cipolotti L, Jager R, Werring DJ. MRI-visible perivascular spaces: relationship to cognition and small vessel disease MRI markers in ischaemic stroke and TIA. J Neurol Neurosurg Psychiatry. (2014) 85:522–5. doi: 10.1136/jnnp-2013-305815
88. Brown R, Benveniste H, Black SE, Charpak S, Dichgans M, Joutel A, et al. Understanding the role of the perivascular space in cerebral small vessel disease. Cardiovasc Res. (2018) 114:1462–73. doi: 10.1093/cvr/cvy113
89. Francis F, Ballerini L, Wardlaw JM. Perivascular spaces and their associations with risk factors, clinical disorders and neuroimaging features: a systematic review and meta-analysis. Int J Stroke. (2019) 14:359–71. doi: 10.1177/1747493019830321
Keywords: cerebral vessel, glymphatic, intramural periarterial drainage, small vessel disease, neurodegeneration, perivascular space
Citation: Agarwal N and Carare RO (2021) Cerebral Vessels: An Overview of Anatomy, Physiology, and Role in the Drainage of Fluids and Solutes. Front. Neurol. 11:611485. doi: 10.3389/fneur.2020.611485
Received: 29 September 2020; Accepted: 30 November 2020;
Published: 13 January 2021.
Edited by:
Yulin Ge, New York University, United StatesReviewed by:
Mariel Kozberg, Massachusetts General Hospital and Harvard Medical School, United StatesMaxime Gauberti, INSERM U1237 Physiopathologie et imagerie des troubles Neurologiques (PhIND), France
Copyright © 2021 Agarwal and Carare. This is an open-access article distributed under the terms of the Creative Commons Attribution License (CC BY). The use, distribution or reproduction in other forums is permitted, provided the original author(s) and the copyright owner(s) are credited and that the original publication in this journal is cited, in accordance with accepted academic practice. No use, distribution or reproduction is permitted which does not comply with these terms.
*Correspondence: Nivedita Agarwal, bml2ZWRpdGEuYWdhcndhbEBhcHNzLnRuLml0