- 1Eye Clinic, Department of Surgical Sciences, University of Torino, Torino, Italy
- 2Department of Medical Sciences, University of Torino, Torino, Italy
Glaucoma is a multifactorial optic neuropathy characterized by the continuous loss of retinal ganglion cells, leading to progressive and irreversible visual impairment. In this minireview, we report the results of the most recent experimental studies concerning cells, molecular mechanisms, genes, and microbiome involved in neuroinflammation processes correlated to glaucoma neurodegeneration. The identification of cellular mechanisms and molecular pathways related to retinal ganglion cell death is the first step toward the discovery of new therapeutic strategies. Recent experimental studies identified the following possible targets: adenosine A2A receptor, sterile alpha and TIR motif containing 1 (neurofilament light chain), toll-like receptors (TLRs) 2 and 4, phosphodiesterase type 4 (PDE4), and FasL-Fas signaling (in particular ONL1204, a small peptide antagonist of Fas receptors), and therapies directed against them. The continuous progress in knowledge provides interesting data, although the total lack of human studies remains an important limitation. Further research is required to better define the role of neuroinflammation in the neurodegeneration processes that occur in glaucomatous disease and to discover neuroprotective treatments amenable to clinical trials. The hereinafter reviewed studies are reported and evaluated according to their translational relevance.
Introduction
Glaucoma is a chronic and progressive optic neuropathy characterized by death of retinal ganglion cells (RGCs) with consequent localized or diffuse thinning of the nerve fiber layer and increased cupping of the optic nerve head (ONH) (1). It is a social disease; its prevalence is believed to grow strongly, and about 80 million glaucomatous patients are expected in 2020 and 112 million in 2040 (2, 3). More than 7 million people worldwide are blind due to glaucoma, and the prevalence of bilateral blindness caused by this pathology varies from 6 to 16% in western countries (4).
The precise mechanisms leading to RGCs loss are not fully understood. Several studies have suggested that glaucoma has important analogies with other neurodegenerative pathologies correlated to inflammatory responses like amyotrophic lateral sclerosis, Alzheimer's disease, Parkinson's disease, Huntington's disease, and frontotemporal dementia (5–8).
The purpose of this minireview is to discuss the consolidated knowledge of neuroinflammation in glaucoma and to focus on new experimental evidences about possible therapeutic targets and latest treatment proposals.
Main Actors Of Neuroinflammation in Glaucoma
Microglia and Astroglia
Microglia and astroglia are the cell types involved in inflammatory responses within the retina (9); they consist of Müller cells and astrocytes and provide metabolic support of neurons, neurological regulation of ionic concentrations, and neuroprotective activities (8, 10–12). Microglial cells originate from blood monocytes migrating to the central nervous system (CNS) and present cellular antigens like CD11b/c and chemokine fractalkine receptor (CX3CR1) (13). They start from primitive erythromyeloid progenitors and mature in microglia (14) differentiating through different pathways dependent on colony-stimulating factor 1 receptor (CSF-1R) (15), interleukin-34 (IL-34) (16), and transforming growth factor-β (TGF-β) (17). After becoming mature, they take part in the inflammation process, which is activated by damage-associated molecular patterns (DAMPs) released by neural cells and also by astroglia and microglia (18–20). Among DAMPs, heat shock proteins (HSPs) are produced by RGCs when intraocular pressure (IOP) is elevated (21), while Tenascin-C is upregulated in astrocytes and induces toll-like receptor (TLR) activation (22). In response to the neuroinflammatory process, microglia release cytokines and chemokines (22–24) such as complement factors, tumor necrosis factor-alpha (TNF-α), and interleukin-6 (IL-6) that amplify the response and contribute to promote morphological changes of microglia into macrophages (25). M1 and M2 are two phenotypes of activated macrophages. M1 is proinflammatory and produces IL-1β, IL-12, and TNF-α (26); on the other hand, M2 synthetizes anti-inflammatory intermediaries such as IL-10, TGF-β, and neurotrophic factor insulin-like growth factor (IGF-1) (27–32).
Recently, in a study in DBA/2J mice with experimental glaucoma, the triggering receptor expressed on myeloid cells/TYROsine kinase binding protein (TREM/TYROBP) signaling network has been identified as the principal regulator mechanism of microglial responses to elevated IOP; infiltrating monocyte-like cells are likely responsible for the early proinflammatory signals (33).
Microglial cells communicate with astroglia via signaling proteins. Depending on this interaction, astroglia are differentiated into two types of reactive astrocytes, A1 and A2 (34–36). A1 has a detrimental effect (37); on the other hand, A2 has a neuroprotective function (38). Indeed, microglia and astroglia collaborate to regulate the inflammation process.
Different genes are implicated in the inflammatory pathways and are upregulated in the retina and ONH (39, 40). The first to be upregulated are TLR signaling pathways: for example, HSPs increase the expression of major histocompatibility complex (MHC) II and cytokine production (21). The second pathway is represented by nuclear factor-kappa B (NF-κB), which causes an increased expression of IL-1 cytokine family that promotes the cascade of inflammatory cytokines (TNF-α and IL-6). TNF-α is found in the optic nerve in glaucoma patients (41–43) and also Fas ligand (FasL), a proapoptotic protein, both being implicated in glaucoma pathogenesis (44). Recently, Oikawa et al. (45) demonstrated in feline's early glaucoma the upregulation of genes related to cell proliferation and immune responses (linked with the TLR and NF-κB signaling pathway), and they observed that proliferating cell types are different in ONH sub-regions. Microglia–macrophages were found in the prelaminar region and in the lamina cribrosa, while oligodendrocytes were more numerous in the retrolaminar region.
Molecular Mechanisms
After reporting the fundamental concepts related to the neuroinflammation cells and the genes involved, we now refer to the latest studies on the main molecular mechanisms selected according to their presence in neurodegenerative diseases and their translational relevance.
The exosomes have a demonstrated role in neurodegenerative diseases. In an experimental glaucoma model (46), the exosomes, produced by naive microglia (BV-2 cells) in a condition of elevated hydrostatic pressure (BV-Exo-EHP, e.g., high IOP), induced the activation of retina microglia, production of cytokines, hypermotility, proliferation, and increase of phagocytosis. They promoted increase of reactive oxygen species and cell death, causing a reduction of retina ganglion cell number. The translation relevance of this study is that the exosomes own an autocrine function in the neuroinflammation pathway correlated to neurodegeneration.
Necroptosis, a recently discovered genetic form of cell death, has a fundamental role in neurodegenerative diseases (47). It is similar to necrosis with cell swelling, granular cytoplasm, chromatin fragmentation, and cellular lysis. Necroptosis differs from apoptosis because the cell content moves into the extracellular matrix in a passive way through the altered cell membrane. Necroptosis is induced by TNF-α and also by Fas and TNF-related apoptosis-inducing ligand (TRAIL), interferons (IFNs), TLR signaling, and viral infection via DAI (DNA sensor DNA-dependent activator of IFN regulatory factor). Ko et al. (47) in a neuroinflammation model of experimental glaucoma proved that the axon degeneration is sterile alpha and TIR motif1 (SARM1)-dependent and is induced by TNF-α with a consequent oligodendrocyte loss and RGC death. The necroptosis perpetrator is mixed lineage kinase domain-like pseudokinase (MLKL) through the reduction of the axon survival factors nicotinamide mononucleotide adenylyltransferase 2 (NMNAT2) and stathmin 2 (STMN2) that inhibit SARM1 NADase action. TNF-α also activates SARM1-dependent axon degeneration in sensory nerve cells through a different necroptotic signaling mechanism.
Several recent studies have dealt with the topic of SARM1 axon degeneration pathway. The axon health is preserved by the balance between the pro-survival NMNAT2 and STMN2 and pro-degenerative molecules dual leucine zipper kinase (DLK) and SARM1 (48). Activated DLK reduces the concentration of protective factors (NMNAT2 and SCG10) and exposes axons to traumatic and metabolic damages; this event is also related to mitochondrial dysfunction that in an independent way reduces the NMNAT2 and SCG10 concentrations in the axons (49). The equilibrium between axon survival and self-destruction is strictly related to axonal NAD+ metabolism. Sasaki et al. (50) studied in cell cultures and in vivo the biomarker cADPR, which controls NAD+ levels via SARM1 and mobilizes calcium. SARM1 has a basal activity in normal conditions. After injury, the axon degeneration is preceded by SARM1-dependent rise in the amount of axonal cADPR, but the contribution of cADPR in degenerative mechanisms has not been proven. The mitochondrial dysfunction can produce an incomplete activation of SARM1 and could predispose the axons to neurodegeneration.
In the context of neuroinflammation, it is mandatory to report the interesting developments that have taken place in recent years regarding the relationship between microbiome and glaucoma. Chen et al. conducted a study in germ-free mice, demonstrating that the absence of gastrointestinal (GI) bacteria abolished the development of glaucoma (51). The immune mechanism involved in the pathogenesis of the neural damage is related to CD4+ T-lymphocytes that recognize HSPs. Bacterial HSPs cross-reacted with both mouse and human HSPs. High IOP values induced the passage of CD4+ T-cells in the retina, and these T-cells were to blame for the extended phase of neurodegeneration in glaucomatous disease. They recognized specifically both bacterial and human HSPs. The induction of neurodegeneration in glaucoma requires pre-exposure to microbial flora, either the GI and oral one (51). In the past century, the presence of elevated titers of antibodies directed against small HSPs and cross-reacting with human HSPs was demonstrated in glaucoma patients (52). It was reported that these antibodies exert cytotoxicity when directly applied to human retina (53). It was proposed that immunomodulation should become the basis of glaucoma therapy. However, the immunosuppressive therapy could be dangerous and sometimes burdened by serious side effects. One solution could be the identification of antigens triggering or enhancing the autoimmune mechanism(s) and their elimination, aiming at reducing the active aggression by autoimmune T-cells. Since the development of glaucoma was shown to be linked to the microbiome, some of its components are potentially curable, such as Helicobacter pylori, a Gram-negative flagellar bacterium present worldwide, in 20–90% of individuals. Only active H. pylori infection induces cellular immune responses against the nervous system, due to molecular mimicry and cross-reactivity with components of host nerves. A large meta-analysis was recently published by Doulberis et al. (54). When the diagnosis of H. pylori infection was performed by gastric biopsy, the odds ratio (OR) for an association was very high (5.4), with confidence interval of 3.17–9.2 (highly significant); the strong association diminished to an OR of 2.08 when only serum antibodies were measured. Similarly, also in glaucoma, the antigens recognized by the immune system were reported in quite a large number. Geyer and Levo (55) suggested that the therapy should be directed not only against the IOP but also against autoreactive lymphocytes, on the basis of the lack of neurodegeneration in experimental mice models after depletion of either B-cells and in particular T-cells directed against HSP-derived peptides (55). However, this might not be sufficient. Beutgen et al. in a recent review reported elevated levels of a variety of autoantibodies (autoAbs) both in systemic circulation and in aqueous humor of glaucoma patients (56): not only anti-HSPs, but also against myelin basic protein and glial fibrillary acid protein. Such autoantibodies are typically involved in diseases of the nervous system.
New Possible Targets and Proposals on Pharmacological Therapies
Hereinafter, the possible new therapeutic targets to reduce or block the neuroinflammation, which are more likely to be used in future clinical practice, are outlined. They are listed in alphabetical order. In Table 1, we provided more detail on new targets, their neuroinflammatory and neurodegenerative effects, and, where provided, the therapeutic options.
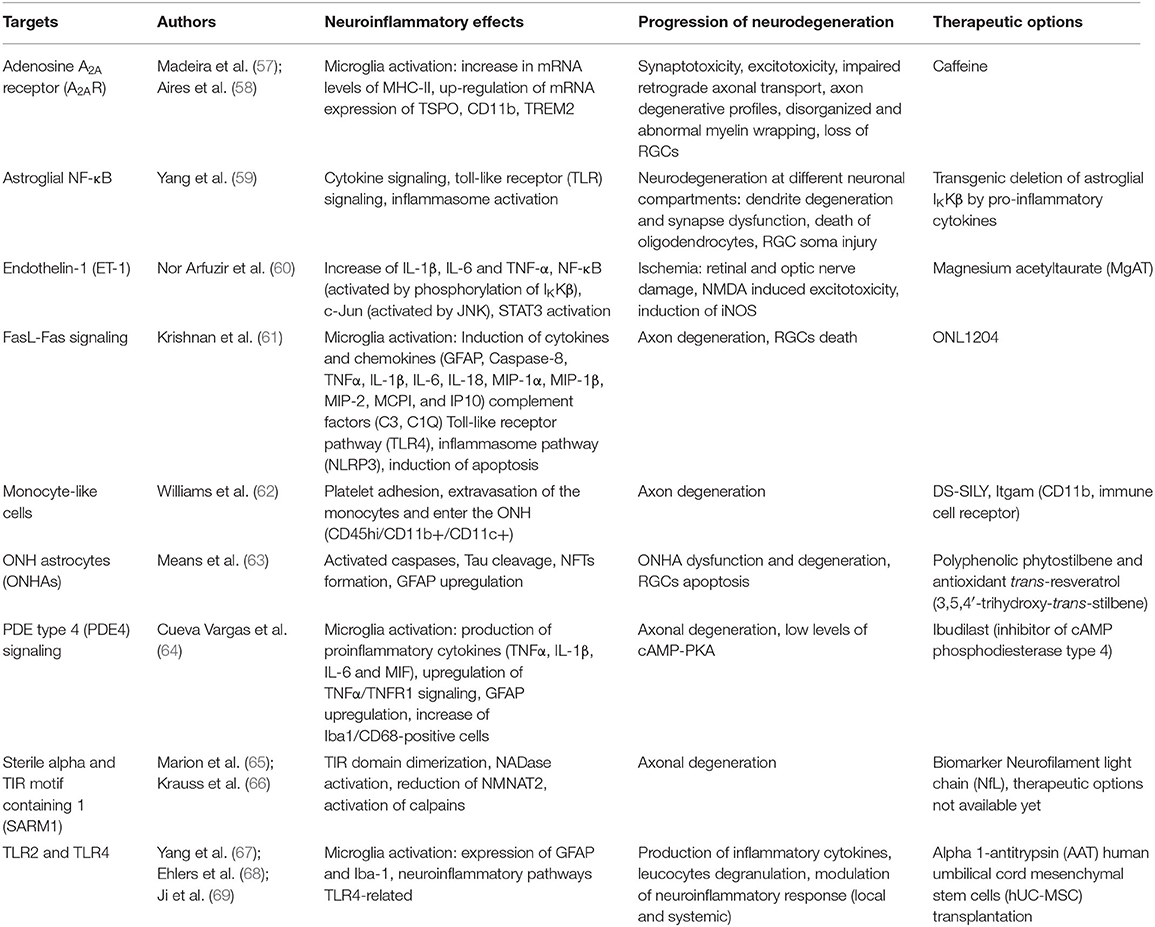
Table 1. New targets, their neuroinflammatory effects, impact of neurodegeneration and therapeutic options.
Adenosine A2A Receptor (A2AR)
Madeira et al. (57, 70) demonstrated in a Sprague Dawley rats model of ocular hypertension (OHT) that caffeine (antagonist of adenosine receptors) administration prevents OHT-induced microglial activation and causes the modulation of retinal neuroinflammation and prevention of the RGCs loss. A recent study outlined the protective effect of microglial adenosine A2A receptor (A2AR) blockade that can prevent, in human retina, microglial cell response to elevated IOP (58).
Astroglial Nuclear Factor-Kappa B
The astroglial NF-κB was evaluated as a possible treatment target for the modulation of immune response in an experimental transgenic glaucoma mouse with deletion of IkappaB kinase beta (IκKβ) in astroglial cells (59). The study showed a reduction of the increase in proinflammatory cytokines (in particular TNF-α) with consequent protection of axon from degeneration and RGCs from apoptosis, as demonstrated also by pattern electroretinogram (PERG) data.
Endothelin-1
Since 2012 (71), it was clarified that the increment of endothelin-1 (ET-1) in glaucomatous patients is linked to sub-clinical inflammation. Nor Arfuzir et al. (60), using Sprague Dawley rats that underwent intravitreal injection of ET-1, proved that the pre-treatment with magnesium acetyltaurate (MgAT) was protective against the increase induced by ET-1 in the retinal expression of IL-1β, IL-6, and TNF-α and against the activation of NF-κB and c-JUN induced by ET-1. MgAT promoted a higher RGC survival.
FasL-Fas Signaling
FasL, a type II transmembrane protein of the TNF group, promotes apoptosis after binding to the Fas receptor and is involved in the pathogenesis of glaucoma also through inflammatory pathways. Krishnan et al. (61) demonstrated in microbead-injected wild-type (WT) mice that the treatment with ONL1204, a small peptide antagonist of Fas receptors, significantly reduced RGC death and loss of axons. The authors proved that ONL1204 blocks microglial activation and inhibits the induction of multiple genes involved in glaucomatous disease, as cytokines and chemokines (GFAP, caspase-8, TNFα, IL-1β, IL-6, IL-18, MIP-1α, MIP-1β, MIP-2, MCPI, and IP10), elements of the complement (C3 and C1Q), inflammasome pathway (NLRP3), and TLR4.
Monocyte-Like Cells
Recently, in a mouse model of ocular hypertension (DBA/2J eyes), it was proved that monocyte-like cells enter the ONH and that monocyte–platelet interactions occur in glaucomatous tissue (62). Using these monocyte-like cells as therapeutic target, the authors demonstrated that the treatment with DS-SILY, a peptidoglycan that hinders the platelet adhesion to the vessel endothelium and to monocytes, and the treatment with genetic targeting of Itgam (CD11b), an immune cell receptor that blocks effusion of the monocytes from the vessels, can both be neuroprotective by reducing neuroinflammation.
Optic Nerve Head Astrocytes
Means et al. (63) demonstrated that pretreatment with polyphenolic phytostilbene and antioxidant trans-resveratrol (3,5,4′-trihydroxy-trans-stilbene) on ONHs underwent oxidative stress with tert-butyl hydroperoxide (tBHP) and induced a significant reduction in activated caspases, neurofibrillary tangle (NFT) formation, and cleaved Tau. These findings outlined that resveratrol can have protective properties to prevent ONH astrocyte (ONHA) dysfunction and degeneration.
Phosphodiesterase Type 4 Signaling
Cueva Vargas et al. (64) evaluated the anti-neuroinflammatory activity of ibudilast, a clinically approved cAMP phosphodiesterase (PDE) inhibitor with special affinity for PDE type 4 (PDE4). In an OHT rat model, the intraocular administration of ibudilast decreased the production of proinflammatory cytokines and reduced macroglia and microglial reactivity in the retina and optic nerve. Ibudilast had a positive effect on RGC soma survival, avoided axonal degeneration, and enhanced anterograde axonal transport in POAG eyes through activation of the cAMP/PKA pathway.
Sterile Alpha and TIR Motif Containing 1
Since SARM1 induces Wallerian degeneration, this pathway could be a therapeutic target (65). The SARM1 inhibition prevents axonal degeneration in traumatic injuries and neurodegenerative disorders. As reported before, SARM1 is involved in innate immune response, and it is possible that the relationship between immune regulation and neurodegenerative disorders will play an important role for new therapeutic possibilities. The discovery of a reliable biomarker of axonal damage, i.e., the neurofilament light chain (NfL) and the possibility of testing NfL in plasma or serum, could be an important step to develop SARM1 inhibitors that protect axons from degeneration (66).
Toll-Like Receptors 2 and 4
As reported, TLR signaling is involved in homeostasis and in pathology of CNS. TLR2 and TLR4 expressed by microglia take part in the glial response and in the neuroinflammation. Yang et al. (67) demonstrated that in C57BL/6J mice, the alpha 1-antitrypsin (AAT), a serine protease inhibitor, blocks microglial activation in chronic ocular hypertension model. It was shown that AAT inhibits leukocyte migration and has antithrombotic, antiapoptotic, and anti-inflammatory properties (68). In OHT rats, Ji et al. (69) demonstrated that the human umbilical cord mesenchymal stem cell (hUC-MSC) transplantation blocks TLR4-related microglial activation and neuroinflammatory pathways.
New Proposal Holistic Approaches
In this section, we reported studies proposing holistic medicinal treatments to modulate/reduce the neuroinflammation in an experimental model of glaucoma. Table 2 illustrates new therapies with details on modulations of inflammatory mechanisms.
Antioxidants
Oxidative stress can alter the immune function of the glia and promotes the neuroinflammation in glaucoma. The effects of the antioxidant Tempol were tested on ocular hypertensive retina and optic nerve samples and on NF-κB, a redox-sensitive transcriptional regulator of neuroinflammation. The analysis of markers of oxidative stress (proinflammatory cytokines, including IL-1, IL-2, IFN-γ, and TNF-α) demonstrated that the treatment was able to decrease the neuroinflammation markers (72).
Ketogenic Diet
A recent research study (73) evidenced that the ketogenic diet reduces inflammation through inhibition of AMPK activation and HCAR1-mediated inhibition of the NLRP3 inflammasome. The way by which ketogenic diet works in neuroprotection is not completely known up to now. Researches supposed that ketogenic diet reduces oxidative stress, inhibits class I histone deacetylases, promotes Nrf2 activation to upregulate antioxidants, and inhibits NF-κB to reduce inflammation (74, 75).
Coriolus and Hericium Nutritional Mushrooms
Trovato Salinaro et al. (76) reported the potential effect of Coriolus and Hericium, nutritional mushrooms, in the therapy of neurodegenerative diseases including Alzheimer's disease and glaucoma; they act as modulators of mechanisms of cellular protection (antioxidant properties) from mitochondrial dysfunction and neuroinflammation.
Hydrophilic Saffron Extract
Studies conducted (77) in a mouse model of laser-induced unilateral OHT on a hydrophilic saffron extract (crocin 3%) demonstrated the reduction of morphological features of microglial activation in OHT and in contralateral eyes. The treatment with saffron extract in part inverted the downregulation of P2RY12. The saffron extract administrated orally protected RGCs from death by reducing neuroinflammation correlated with increased IOP.
Discussion
The co-participation of the innate immune response and the inflammation in the pathogenesis of optic nerve degeneration in glaucomatous disease is now an established and proven event (78–82). The purpose of this minireview was to outline potential targets for future therapies, some of which are already being studied, and to describe possible treatments related to alternative medicine. These recent studies performed on animal models have improved our knowledge on cellular mechanisms, partly already known, but mainly on the signaling pathways of neuroinflammation. The studies analyzed openly the way to new possibilities of modulation of neuroinflammation (such as antagonist of A2AR, stem cells, MgAT, resveratrol, and alpha 1-antitrypsin) with a view to achieve effective neuroprotective treatments for glaucomatous disease. An important limitation is the almost total lack of researches in human. On the basis of the data reported, the possible ways of developing future research are illustrated in Figure 1. Further studies are needed to transfer the current experimental evidence into clinical research in order to slow down or to prevent the progressive glaucoma neurodegeneration.
Author Contributions
All authors listed have made a substantial, direct and intellectual contribution to the work, and approved it for publication.
Conflict of Interest
The authors declare that the research was conducted in the absence of any commercial or financial relationships that could be construed as a potential conflict of interest.
Acknowledgments
Carla Malinverni contributed to correcting the English version.
References
1. European Glaucoma Society. Terminology and Guidelines for Glaucoma. 4th ed. Zug: European Glaucoma Society (2014).
2. Cook C, Foster P. Epidemiology of glaucoma: what's new? Can J Ophthalmol. (2012) 47:223–6. doi: 10.1016/j.jcjo.2012.02.003
3. Tham YC, Li X, Wong TY, Quigley HA, Aung T, Cheng CY. Global prevalence of glaucoma and projections of glaucoma burden through 2040: a systematic review and meta-analysis. Ophthalmology. (2014) 121:2081–90. doi: 10.1016/j.ophtha.2014.05.013
4. Rossetti L, Digiuni M, Montesano G, Centofanti M, Fea AM, Iester M, et al. Blindness and glaucoma: a multicenter data review from 7 academic eye clinics. PLoS ONE. (2015) 10:e0136632. doi: 10.1371/journal.pone.0136632
5. Toth RP, Atkin JD. Dysfunction of optineurin in amyotrophic lateral sclerosis and glaucoma. Front Immunol. (2018) 9:1017. doi: 10.3389/fimmu.2018.01017
6. Wiggs JL, Pasquale LR. Genetics of glaucoma. Hum Mol Genet. (2017) 26(R1):R21–7. doi: 10.1093/hmg/ddx184
7. Fingert JH, Robin AL, Scheetz TE, Kwon YH, Liebmann JM, Ritch R, et al. Tank-Binding kinase 1 (TBK1) gene and open-angle glaucomas (An American Ophthalmological Society Thesis). Trans Am Ophthalmol Soc. (2016) 114:T6
8. Ramirez AI, de Hoz R, Salobrar-Garcia E, Salazar JJ, Rojas B, Ajoy D, et al. The role of microglia in retinal neurodegeneration: Alzheimer's Disease, Parkinson, and Glaucoma. Front Aging Neurosci. (2017) 9:214. doi: 10.3389/fnagi.2017.00214
9. Soto I, Howell GR. The complex role of neuroinflammation in glaucoma. Cold Spring Harb Perspect Med. (2014) 4:a017269. doi: 10.1101/cshperspect.a017269
10. Iadecola C, Nedergaard M. Glial regulation of the cerebral microvasculature. Nature neuroscience. (2007) 10:1369–76. doi: 10.1038/nn2003
11. Rouach N, Koulakoff A, Abudara V, Willecke K, Giaume C. Astroglial metabolic networks sustain hippocampal synaptic transmission. Science. (2008) 322:1551–5. doi: 10.1126/science.1164022
12. Ullian EM, Sapperstein SK, Christopherson KS, Barres BA. Control of synapse number by glia. Science. (2001) 291:657–61. doi: 10.1126/science.291.5504.657
13. Dudvarski Stankovic N, Teodorczyk M, Ploen R, Zipp F, Schmidt MH. Microglia-blood vessel interactions: a double-edged sword in brain pathologies. Acta Neuropathol. (2016) 131:347–63. doi: 10.1007/s00401-015-1524-y
14. Ginhoux F, Greter M, Leboeuf M, Nandi S, See P, Gokhan S, et al. Fate mapping analysis reveals that adult microglia derive from primitive macrophages. Science. (2010) 330:841–5. doi: 10.1126/science.1194637
15. Erblich B, Zhu L, Etgen AM, Dobrenis K, Pollard JW. Absence of colony stimulation factor-1 receptor results in loss of microglia, disrupted brain development and olfactory deficits. PLoS ONE. (2011) 6:e26317. doi: 10.1371/journal.pone.0026317
16. Greter M, Lelios I, Pelczar P, Hoeffel G, Price J, Leboeuf M, et al. Stroma-derived interleukin-34 controls the development and maintenance of langerhans cells and the maintenance of microglia. Immunity. (2012) 37:1050–60. doi: 10.1016/j.immuni.2012.11.001
17. Butovsky O, Jedrychowski MP, Moore CS, Cialic R, Lanser AJ, Gabriely G, et al. Identification of a unique TGF-beta-dependent molecular and functional signature in microglia. Nat Neurosci. (2014) 17:131–43. doi: 10.1038/nn.3599
18. Rifkin IR, Leadbetter EA, Busconi L, Viglianti G, Marshak-Rothstein A. Toll-like receptors, endogenous ligands, and systemic autoimmune disease. Immunol Rev. (2005) 204:27–42. doi: 10.1111/j.0105-2896.2005.00239.x
19. Yu L, Wang L, Chen S. Endogenous toll-like receptor ligands and their biological significance. J Cell Mol Med. (2010) 14:2592–603. doi: 10.1111/j.1582-4934.2010.01127.x
20. Zhu H, Wang L, Ruan Y, Zhou L, Zhang D, Min Z, et al. An efficient delivery of DAMPs on the cell surface by the unconventional secretion pathway. Biochem Bioph Res Co. (2011) 404:790–5. doi: 10.1016/j.bbrc.2010.12.061
21. Luo C, Yang X, Kain AD, Powell DW, Kuehn MH, Tezel G. Glaucomatous tissue stress and the regulation of immune response through glial Toll-like receptor signaling. Invest Ophthalmol Vis Sci. (2010) 51:5697–707. doi: 10.1167/iovs.10-5407
22. Howell GR, Soto I, Zhu X, Ryan M, Macalinao DG, Sousa GL, et al. Radiation treatment inhibits monocyte entry into the optic nerve head and prevents neuronal damage in a mouse model of glaucoma. J Clin Invest. (2012) 122:1246–61. doi: 10.1172/JCI61135
23. Bosco A, Crish SD, Steele MR, Romero CO, Inman DM, Horner PJ, et al. Early reduction of microglia activation by irradiation in a model of chronic glaucoma. PLoS ONE. (2012) 7:e43602. doi: 10.1371/journal.pone.0043602
24. Ebneter A, Casson RJ, Wood JP, Chidlow G. Microglial activation in the visual pathway in experimental glaucoma: spatiotemporal characterization and correlation with axonal injury. Invest Ophthalmol Vis Sci. (2010) 51:6448–60. doi: 10.1167/iovs.10-5284
25. Callahan MK, Ransohoff RM. Analysis of leukocyte extravasation across the blood-brain barrier: conceptual and technical aspects. Cur Allergy Asthma Rep. (2004) 4:65–73. doi: 10.1007/s11882-004-0046-9
26. Varnum MM, Ikezu T. The classification of microglial activation phenotypes on neurodegeneration and regeneration in Alzheimer's disease brain. Arch Immunol Ther Exp. (2012) 60:251–66. doi: 10.1007/s00005-012-0181-2
27. Suh HS, Zhao ML, Derico L, Choi N, Lee SC. Insulin-like growth factor 1 and 2 (IGF1, IGF2) expression in human microglia: differential regulation by inflammatory mediators. J Neuroinflamm. (2013) 10:37. doi: 10.1186/1742-2094-10-37
28. Tang Y, Le W. Differential roles of M1 and M2 microglia in neurodegenerative diseases. Mol Neurobiol. (2016) 53:1181–94. doi: 10.1007/s12035-014-9070-5
29. Burguillos MA, Deierborg T, Kavanagh E, Persson A, Hajji N, Garcia-Quintanilla A, et al. Caspase signalling controls microglia activation and neurotoxicity. Nature. (2011) 472:319–24. doi: 10.1038/nature09788
30. Glezer I, Simard AR, Rivest S. Neuroprotective role of the innate immune system by microglia. Neuroscience. (2007) 147:867–83. doi: 10.1016/j.neuroscience.2007.02.055
31. Gonzalez H, Elgueta D, Montoya A, Pacheco R. Neuroimmune regulation of microglial activity involved in neuroinflammation and neurodegenerative diseases. J Neuroimmunol. (2014) 274:1–13. doi: 10.1016/j.jneuroim.2014.07.012
32. Gordon R, Anantharam V, Kanthasamy AG, Kanthasamy A. Proteolytic activation of proapoptotic kinase protein kinase Cdelta by tumor necrosis factor alpha death receptor signaling in dopaminergic neurons during neuroinflammation. J Neuroinflamm. (2012) 9:82. doi: 10.1186/1742-2094-9-82
33. Tribble JR, Jeffrey M, Harder JM, Williams PA, John SWM. Ocular hypertension suppresses homeostatic gene expression in optic nerve head microglia of DBA/2J mice. Mol Brain. (2020) 13:81. doi: 10.1186/s13041-020-00603-7
34. Heppner FL, Ransohoff RM, Becher B. Immune attack: the role of inflammation in Alzheimer disease. Nat Rev Neurosci. (2015) 16:358–72. doi: 10.1038/nrn3880
35. Liddelow SA, Guttenplan KA, Clarke LE, Bennett FC, Bohlen CJ, Schirmer L, et al. Neurotoxic reactive astrocytes are induced by activated microglia. Nature. (2017) 541:481–87. doi: 10.1038/nature21029
36. Martinez FO, Gordon S. The M1 and M2 paradigm of macrophage activation: time for reassessment. F1000prime Rep. (2014) 6:13. doi: 10.12703/P6-13
37. Sofroniew MV, Vinters HV. Astrocytes: biology and pathology. Acta Neuropathol. (2010) 119:7–35. doi: 10.1007/s00401-009-0619-8
38. Zador Z, Stiver S, Wang V, Manley GT. Role of aquaporin-4 in cerebral edema and stroke. Handb Exp Pharmacol. (2009) 190:159–70. doi: 10.1007/978-3-540-79885-9_7
39. Ahmed F, Brown KM, Stephan DA, Morrison JC, Johnson EC, Tomarev SI. Microarray analysis of changes in mRNA levels in the rat retina after experimental elevation of intraocular pressure. Invest Ophthalmol Vis Sci. (2004) 45:1247–58. doi: 10.1167/iovs.03-1123
40. Yang Z, Quigley HA, Pease ME, Yang Y, Qian J, Valenta D, et al. Changes in gene expression in experimental glaucoma and optic nerve transection: the equilibrium between protective and detrimental mechanisms. Invest Ophthalmol Vis Sci. (2007) 48:5539–48. doi: 10.1167/iovs.07-0542
41. Yan X, Tezel G, Wax MB, Edward DP. Matrix metalloproteinases and tumor necrosis factor alpha in glaucomatous optic nerve head. Arch Ophthalmol. (2000) 118:666–73. doi: 10.1001/archopht.118.5.666
42. Sawada H, Fukuchi T, Tanaka T, Abe H. Tumor necrosis factor-alpha concentrations in the aqueous humor of patients with glaucoma. Invest Ophthalmol Vis Sci. (2010) 51:903–6. doi: 10.1167/iovs.09-4247
43. Tezel G, Li LY, Patil RV, Wax MB. TNF-alpha and TNF-alpha receptor-1 in the retina of normal and glaucomatous eyes. Invest Ophthalmol Vis Sci. (2001) 42:1787–94.
44. Gregory MS, Hackett CG, Abernathy EF, Lee KS, Saff RR, Hohlbaum AM, et al. Opposing roles for membrane bound and soluble Fas ligand in glaucoma-associated retinal ganglion cell death. PLoS ONE. (2011) 6:e17659. doi: 10.1371/journal.pone.0017659
45. Oikawa K, Ver Hoeve JN, Teixeira LBC, Snyder KC, Kiland JA, Ellinwood NM, et al. Sub-region-specific optic nerve head glial activation in glaucoma. Mol Neurobiol. (2020) 57:2620–38. doi: 10.1007/s12035-020-01910-9
46. Aires ID, Ribeiro-Rodrigues T, Boia R, Catarino S, Girão H, Ambrósio AF, et al. Exosomes derived from microglia exposed to elevated pressure amplify the neuroinflammatory response in retinal cells. Glia. (2020) 68:2705–24. doi: 10.1002/glia.23880
47. Ko KW, Milbrandt J, DiAntonio A. SARM1 acts downstream of neuroinflammatory and necroptotic signaling to induce axon degeneration. J Cell Biol. (2020) 219:e201912047. doi: 10.1083/jcb.201912047
48. Figley MD, DiAntonio A. The SARM1 axon degeneration pathway: control of the NAD+ metabolome regulates axon survival in health and disease. Curr Opin Neurobiol. (2020) 63:59–66. doi: 10.1016/j.conb.2020.02.012
49. Summers DW, Frey E, Walker LJ, Milbrandt J, DiAntonio A. DLK activation synergizes with mitochondrial dysfunction to downregulate axon survival factors and promote SARM1-dependent axon degeneration. Mol Neurobiol. (2020) 57:1146–58. doi: 10.1007/s12035-019-01796-2
50. Sasaki Y, Engber TM, Hughes RO, Figley MD, Wu T, Bosanac T, et al. cADPR is a gene dosage-sensitive biomarker of SARM1 activity in healthy, compromised, and degenerating axons. Exp Neurol. (2020) 329:113252. doi: 10.1016/j.expneurol.2020.113252
51. Chen H, Cho KS, Vu THK, Shen C-H, Kaur M, Chen G, et al. Commensal microflora-induced T cell responses mediate progressive neurodegeneration in glaucoma. Nat Commun. (2018) 9:3209. doi: 10.1038/s41467-018-05681-9
52. Tezel G, Seigel GM, Wax MB. Autoantibodies to small heat shock proteins in glaucoma. Invest Ophthalmol Vis Sci. (1998) 39:2277–87.
53. Tezel G, Yang J, Wax MB. Heat shock proteins, immunity and glaucoma. Brain Res Bull. (2004) 62:473–4. doi: 10.1016/S0361-9230(03)00074-1
54. Doulberis M, Papaefthymiou A, Polyzos SA, Bargiotas P, Liatsos C, Srivastava DS„, et al. Association between active Helicobacter pylori infection and glaucoma: a systematic review and meta-analysis. Microorganisms. (2020) 8:894. doi: 10.3390/microorganisms8060894
55. Geyer O, Levo Y. Glaucoma is an autoimmune disease. Autoimmun Rev. (2020) 19:102535. doi: 10.1016/j.autrev.2020.102535
56. Beutgen VM, Perumal N, Pfeiffer N, Grus FH. Autoantibody biomarker discovery in primary open angle glaucoma using serological proteome analysis (SERPA). Front Immunol. (2019) 10:381. doi: 10.3389/fimmu.2019.00381
57. Madeira MH, Ortin-Martinez A, Nadal-Nícolas F, Ambrósio AF, Vidal-Sanz M, Agudo-Barriuso M, et al. Caffeine administration prevents retinal neuroinflammation and loss of retinal ganglion cells in an animal model of glaucoma. Sci Rep. (2016) 6:27532; doi: 10.1038/srep27532
58. Aires ID, Boia R, Rodrigues-Neves AC, Madeira MH, Marques C, Ambrósio AF, et al. Blockade of microglial adenosine A2A receptor suppresses elevated pressure-induced inflammation, oxidative stress, and cell death in retinal cells. Glia. (2019) 67:896–914. doi: 10.1002/glia.23579
59. Yang X, Zeng O, Baris M, Tezel G. Transgenic inhibition of astroglial NκB restrains the neuroinflammatory and neurodegenerative outcomes of experimental mouse glaucoma. J Neuroinflamm. (2020) 17:252. doi: 10.1186/s12974-020-01930-1
60. Nor Arfuzir NN, Agarwal R, Iezhitsa I, Agarwal P, Ismail NM. Magnesium acetyltaurate protects against endothelin-1 induced RGC loss by reducing neuroinflammation in Sprague dawley rats. Exp Eye Res. (2020) 194:107996. doi: 10.1016/j.exer.2020.107996
61. Krishnan A, Kocab AJ, Zacs DN, Marshak-Rothstein A, Gregory-Ksander M. A small peptide antagonist of the Fas receptor inhibits neuroinflammation and prevents axon degeneration and retinal ganglion cell death in an inducible mouse model of glaucoma. J Neuroinflamm. (2019) 16:184. doi: 10.1186/s12974-019-1576-3
62. Williams PA, Braine CE, Kizhatil K, Foxworth NE, Tolman NG, Harder JM, et al. Inhibition of monocyte-like cell extravasation protects from neurodegeneration in DBA/2J glaucoma. Mol Neurodegener. (2019) 14:6. doi: 10.1186/s13024-018-0303-3
63. Means JC, Lopez AA, Koulen P. Resveratrol protects optic nerve head astrocytes from oxidative stress-induced cell death by preventing caspase-3 activation, tau dephosphorylation at Ser422 and formation of misfolded protein aggregates. Cell Mol Neurobiol. (2020) 40:911–26. doi: 10.1007/s10571-019-00781-6
64. Cueva Vargas JL, Belforte N, Di Polo A. The glial cell modulator ibudilast attenuates neuroinflammation and enhances retinal ganglion cell viability in glaucoma through protein kinase A signaling. Neurobiol Dis. (2016) 93:156–71. doi: 10.1016/j.nbd.2016.05.002
65. Marion CM, McDaniel DP, Armstrong RC. Sarm1 deletion reduces axon damage, demyelination, and white matter atrophy after experimental traumatic brain injury. Exp Neurol. (2019) 321:113040 doi: 10.1016/j.expneurol.2019.113040
66. Krauss R, Bosanac T, Devraj R, Engber T, Hughes RO. Axons matter: the promise of treating neurodegenerative disorders by targeting SARM1-mediated axonal degeneration. Trends Pharmacol Sci. (2020) 41:281–93. doi: 10.1016/j.tips.2020.01.006
67. Yang S, Xian B, Li K, Luo Z, Liu Y, Hu D, et al. Alpha 1-antitrypsin inhibits microglia activation and facilitates the survival of iPSC grafts in hypertension mouse model. Cell Immunol. (2018) 328:49–57. doi: 10.1016/j.cellimm.2018.03.006
68. Ehlers MR. Immune-modulating effects of alpha-1 antitrypsin. Biol Chem. (2014) 395:1187–93. doi: 10.1515/hsz-2014-0161
69. Ji S, Xiao J, Liu J, Tang S. Human umbilical cord mesenchymal stem cells attenuate ocular hypertension-induced retinal neuroinflammation via toll-like receptor 4 pathway. Stem Cells Int. (2019) 2019:17. doi: 10.1155/2019/9274585
70. Madeira MH, Elvas F, Boia R, Gonçalves FQ, Cunha RA, Ambrósio AF, et al. Adenosine A2R blockade prevents neuroinflammation-induced death of retinal ganglion cells caused by elevated pressure. J Neuroinflamm. (2015) 12:115. doi: 10.1186/s12974-015-0333-5
71. Cellini M, Strobe E, Gizzi C, Balducci N, Toschi PG, Campos EC. Endothelin-1 plasma levels and vascular endothelial dysfunction in primary open angle glaucoma. Life Sci. (2012) 91:699–702. doi: 10.1016/j.lfs.2012.02.013
72. Yang X, Hondur G, Tezel G. Antioxidant treatment limits neuroinflammation in experimental glaucoma. Invest Ophthalmol Vis Sci. (2016) 57:2344–54. doi: 10.1167/iovs.16-19153
73. Harun-Or-Rashid M, Inman DM. Reduced AMPK activation and increased HCAR activation drive anti-inflammatory response and neuroprotection in glaucoma. J Neuroinflamm. (2018) 15:313. doi: 10.1186/s12974-018-1346-7
74. Lu Y, Yang YY, Zhou MW, Liu N, Xing HY, Liu XX, et al. Ketogenic diet attenuates oxidative stress and inflammation after spinal cord injury by activating Nrf2 and suppressing the NF-kappaB signaling pathways. Neurosci Lett. (2018) 683:13–8. doi: 10.1016/j.neulet.2018.06.016
75. Shimazu T, Hirschey MD, Newman J, He W, Shirakawa K, Le Moan N, et al. Suppression of oxidative stress by beta-hydroxybutyrate, an endogenous histone deacetylase inhibitor. Science. (2013) 339:211–4. doi: 10.1126/science.1227166
76. Trovato Salinaro A, Pennisi M, Di Paola R, Scuto M, Crupi R, Cambria MT, et al. Neuroinflammation and neurohormesis in the pathogenesis of Alzheimer's disease and Alzheimer-linked pathologies: modulation by nutritional mushrooms. Immun Ageing. (2018) 15:8. doi: 10.1186/s12979-017-0108-1
77. Fernández-Albarral JA, Ramírez AI, de Hoz R, López-Villarín N, Salobrar-García E, López-Cuenca I, et al. Neuroprotective and anti-inflammatory effects of a hydrophilic saffron extract in a model of glaucoma. Int J Mol Sci. (2019) 20:4110. doi: 10.3390/ijms20174110
78. Russo R, Varano GP, Adornetto AG, Nucci C, Corasaniti MT, Bagetta G, et al. Retinal ganglion cell death in glaucoma: exploring the role of neuroinflammation. Eur J Pharmacol. (2016) 787:134–42. doi: 10.1016/j.ejphar.2016.03.064
79. Williams P, Marsh-Armstrong N, Howell GR. Neuroinflammation in glaucoma: a new opportunity. Exp Eye Res. (2017) 157:20–7. doi: 10.1016/j.exer.2017.02.014
80. Adornetto AG, Russo R, Parisi V. Neuroinflammation as a target for glaucoma therapy. Neural Regen Res. (2019) 14:391–4. doi: 10.4103/1673-5374.245465
81. Wei X, Cho KS, Thee EF, Jager MJ, Chen DF. Neuroimmflammation and microglia in glaucoma – time for a paradigm shift. J Neurosci Res. (2019) 97:70–6. doi: 10.1002/jnr.24256
Keywords: glaucoma, neuroinflammation, microglia, astrocytes, target, therapy, microbiome
Citation: Rolle T, Ponzetto A and Malinverni L (2021) The Role of Neuroinflammation in Glaucoma: An Update on Molecular Mechanisms and New Therapeutic Options. Front. Neurol. 11:612422. doi: 10.3389/fneur.2020.612422
Received: 30 September 2020; Accepted: 21 December 2020;
Published: 04 February 2021.
Edited by:
Ahmed Toosy, University College London, United KingdomReviewed by:
Alessio Martucci, University of Rome Tor Vergata, ItalyRebecca M. Sappington, Wake Forest School of Medicine, United States
Copyright © 2021 Rolle, Ponzetto and Malinverni. This is an open-access article distributed under the terms of the Creative Commons Attribution License (CC BY). The use, distribution or reproduction in other forums is permitted, provided the original author(s) and the copyright owner(s) are credited and that the original publication in this journal is cited, in accordance with accepted academic practice. No use, distribution or reproduction is permitted which does not comply with these terms.
*Correspondence: Teresa Rolle, dGVyZXNhLnJvbGxlQHVuaXRvLml0