- 1Department of Neurology, Binzhou Medical University Hospital, Binzhou, China
- 2Institute for Metabolic and Neuropsychiatric Disorders, Binzhou Medical University Hospital, Binzhou, China
The gut microbiota plays a key role in the function of the host immune system and neuroimmune diseases. Alterations in the composition of the gut microbiota can lead to pathology and altered formation of microbiota-derived components and metabolites. A series of neuroimmune diseases, such as myasthenia gravis (MG), multiple sclerosis (MS), neuromyelitis optica spectrum disorders (NMOSDs), Guillain–Barré syndrome (GBS), and autoimmune encephalitis (AIE), are associated with changes in the gut microbiota. Microecological therapy by improving the gut microbiota is expected to be an effective measure for treating and preventing some neuroimmune diseases. This article reviews the research progress related to the roles of gut microbiota and fecal microbiota transplantation (FMT) in neuroimmune diseases.
1. The gut microbiota
The gut is colonized by a multitude of microorganisms, commonly referred to as the gut microbiota. Although most of these organisms are bacteria, others include archaea, protists, fungi, and viruses. The gut microbiota comprises about 1,200 bacterial species. The dominant phyla in the intestinal tract belong mainly to Bacteroidetes, Firmicutes, Actinobacteria, and Proteobacteria (1, 2). The gut microbiota forms a long-term, dynamically balanced symbiotic relationship with the host that plays an important role in human immunity and metabolism. The gut microbiota affects the synthesis of polysaccharides, glycosides, vitamins, and essential amino acids. Additionally, the gut microbiota catalyzes the metabolism of drugs, carcinogens, and hormones and reduces the synthesis of histamine to play a detoxification role. Moreover, the gut microbiota performs protective functions by producing various antibacterial and bacteriostatic substances and degrading bacterial toxins (3, 4).
Gut microbiota dysbiosis can lead to pathology and altered formation of microbiota-derived components and metabolites, resulting in dysregulation of the immune system and metabolism (5).
2. Gut-brain axis (GBA)
The gut microbiota, as a well-known part of bidirectional neurohormonal communication system, affects gut pathophysiology and central nervous system (CNS) function through multiple bidirectional pathways of the GBA. The gut microbiota is transmitted to the brain via multiple afferent signaling pathways, including neural (vagal and spinal afferents), endocrine (cytokines, metabolites, and microbial signaling molecules), the hypothalamic–pituitary–adrenal (HPA) axis, and immune signaling (6, 7). Conversely, signals from CNS and neuroendocrine systems might monitor and regulate changes in the gut microbiota to adapt to environmental changes (8, 9).
2.1. Vagus nerve
The vagus nerve is the main conduit for communication between the brain and the gut. It connects the gastrointestinal tract with the nucleus tractus solitarius and the higher emotional regulation network (10). Numerous microbial-derived metabolites are able to impact vagal activation. Butyrate can directly activate vagal afferent terminals in the gut, and SCFA oleate activates the vagus nerve via the CCK-A receptor (11). In addition, toll-like receptor 4, a pattern recognition receptor for expression of vagus nerve fibers, can directly recognize bacterial products and thus activate the vagus nerve (12). Lactobacillus rhamnose-JB-1 alters the expression of γ-aminobutyric acid (GABA) receptors in the amygdala and hippocampus, and improves anxiety-like behaviors in mice (13). Most effects of L. rhamnosus JB-1 are lost in vagotomized mice, emphasize beneficial roles for the vagus nerve in microbiota-CNS communication (14). Futhermore, vagotomy can block central signaling of Lactobacillus and Bifidobacterium species, aggravate anxiety and depression related behavior in mice (13, 15). On the contrary, targeted vagal stimulation can suppress lipopolysaccharides (LPS)-induced proinflammatory cytokine production by microglia (16, 17). Interestingly, patients who underwent a full truncal vagotomy for treatment of peptic ulcer disease have a decreased risk of certain neurological diseases such as Parkinson's disease when they enter old age (18).
2.2. Microbe-derived metabolites (short-chain fatty acids, SCFAs)
Some studies have found that direct contact between microbiota-derived components and metabolites (vitamins, bile acids, LPS, SCFAs, derivatives of tryptophan metabolism, etc.) and host metabolite sensing receptors expressed in immune cells of the intestinal mucosa initiates the mucosal immune response and activates macrophages, dendritic cells (DCs), and T cells. The gut microbiota is indispensable for host immune functions and actively regulates immune homeostasis in the peripheral and CNS (19). SCFAs are the most widely studied metabolites involved in regulating inflammation and the immune response, which are produced in the process of microbial fermentation of indigestible dietary fibers or resistant starch (20, 21). The main SCFAs are acetate, propionate, and butyrate (Figure 1) (22). In the human gut, members of the Bacteroides phylum provide most of the acetate and propionate. Butyrate production is mainly by Firmicutes (23, 24). An increase in SCFA levels is commonly achieved through a high-fiber diet (25). SCFAs are recognized by receptors on intestinal endocrine cells and intestinal epithelial cells and have anti-inflammatory effects on the intestinal mucosa. SCFAs exert anti-inflammatory effects beyond the gut, which help increase the number of Tregs and inhibit the differentiation of Th17 cells (26, 27) (Figure 1). Tregs can suppress the function of antigen-presenting cells and other effector T cells and suppress immune responses (28). Therefore, Tregs play a significant role in maintaining immune homeostasis and immune tolerance (29). However, Th17 cells are critical for mediating chronic inflammation in autoimmune diseases, producing cytokines such as IL-17, IL-21, IL-22, with pro-inflammatory phenotype (30). It has been shown that Bacteroides, Enterobacteriaceae, and Sphingobacteriaceae are positively correlated with some autoimmune diseases, while SCFAs are generally negatively correlated with these diseases (31).
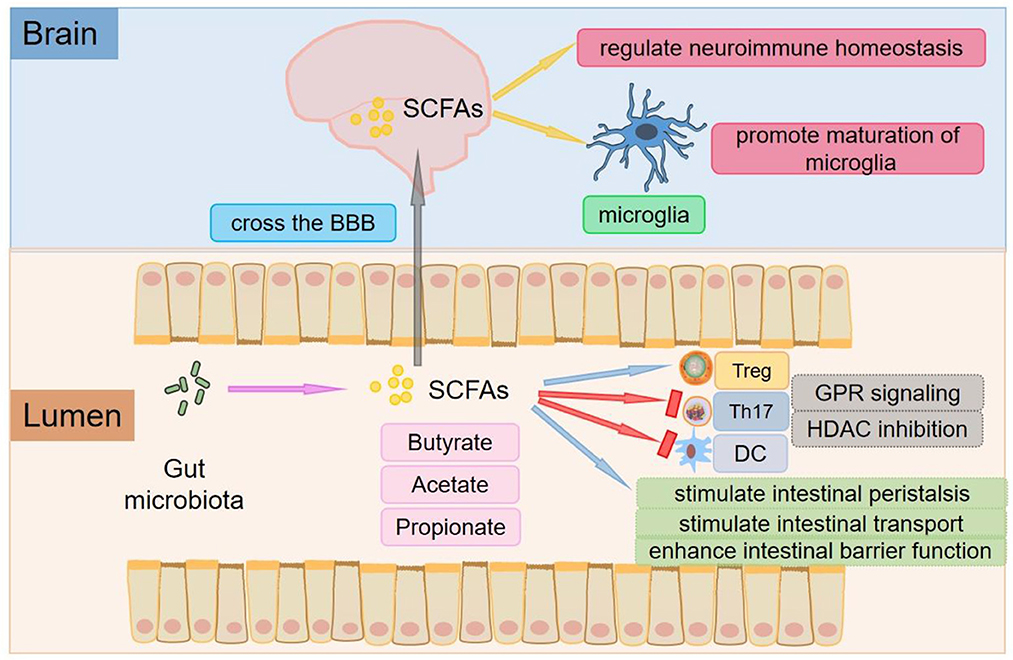
Figure 1. The roles of SCFAs. The main SCFAs are acetate, propionate, and butyrate, which are produced in the process of microbial fermentation of indigestible dietary fibers or resistant starch. SCFAs are key mediators of metabolism and immune cell function in the gut mucosa and can stimulate intestinal peristalsis and intestinal transport and affect the integrity of the intestinal barrier. SCFAs exert anti-inflammatory effects beyond the gut, which help increase the number of Tregs and inhibit the differentiation of Th17 cells. SCFAs can promote the differentiation of Tregs by inhibiting HDAC or activating GPR109A/HCA2. Tregs can inhibit the function of antigen-presenting cells and other effector T cells and suppress immune responses. SCFAs also inhibit DCs development and regulate antigen presentation of DCs by inhibiting HDAC and interacting with FFARs. SCFAs can not only cross the BBB and control neuroimmune homeostasis but also play an important role in the recovery and maturation of microglial function.
Through protein-coupled receptors [also known as free fatty acid receptors (FFARs)] (Figure 1), signals are transmitted to several non-intestinal cell types (32). One of the receptors, G protein-coupled receptor 109A/ hydroxy carboxylate 2 receptor (GPR109A/HCA2), is activated by butyric acid in the immune system (33). The interaction of butyrate with GPR109A/HCA2 is involved in the development of Tregs (34, 35), which is the basis for regulating homeostasis and maintaining pathogen immune balance and symbiotic bacterial immune tolerance. Depending on the different cytokine environments, the interaction between SCFAs and FFARs affects not only the differentiation of T cells into Tregs but also the differentiation of T cells into effector T cells. Butyric acid regulates gene expression by inhibiting histone deacetylase (HDAC) (Figure 1), especially HDAC1 and HDAC3 (36), which is another mechanism of immune regulation by SCFAs (37). In addition, propionic acid can be used as a weak inhibitor of HDAC (38). Recent studies have shown that inhibition of HDAC may promote the development and function of Tregs, one of the mechanisms by which the gut microbiota enhances intestinal Treg production (39). SCFAs such as butyric acid and propionic acid also inhibit DCs development and regulate antigen presentation by inhibiting HDAC and interacting with FFARs (40–42).
Colonization of the gut with SCFA-producing bacteria also decreases the permeability of the BBB, suggesting that SCFAs play an important role in the development and maintenance of the BBB (27). SCFAs can not only cross the BBB and control neuroimmune homeostasis but also exert a significant role in the recovery and maturation of microglial function (43) (Figure 1). Global defects in the maturation and function of microglial cells have both been found in mice deficient in SCFAs receptors and germ-free mice to display, resulting in the impaired cellular defense that could be rescued by treatment with SCFAs (44).
2.3. Immune cells
The gut microbiota and their metabolites influence the activation and differentiation of intestinal B and T cells. Subpopulations of gut T and B cells can circulate from the gut to the meninges, where they release cytokines (45, 46). Cytokines (e.g., IL-10) and antibodies (e.g., IgA) induced by the gut microbiota enter the systemic circulation and influence the local neuroimmune microenvironment through the blood-brain barrier (BBB). These cytokines act on central neurons and microglia to prevent meningeal infection (46). In addition, specific members of the microbiota can also induce defined T cell subsets. Segmented filamentous bacteria can induce the development of IL-17A-producing T helper 17 (Th17) cells in the mouse small intestine (47, 48). However, the human gut bacterium Bacteroides fragilis improves the development of IL-10-producing Tregs via capsular expression of polysaccharide A (PSA) in the mouse colon (49, 50). The gut microbiota also influences microglial maturation and function. Microglia are the most abundant resident immune cells in the brain and are considered to be endogenous macrophages of the CNS, which are essential for tissue homeostasis.
2.4. Microbe-associated molecular patterns (MAMPs)
MAMPs (LPS, flagellins, bacterial lipoproteins and unmethylated CpG motifs) recognized by Toll-like receptors (TLRs), and activate immune cells (51). TLRs are expressed by many immune cells, including T cells, B cells macrophages, DCs, and neutrophils, which exert a significant role in molecular communication between changes in the gut microbiota and homeostasis of immune system (52). Cytokine environment is significantly affected by the type and degree of TLRs activation. For example, activation of TLR2 may induce T cells to differentiate to Th2 phenotype through the production of IL-10 and IL-13. However, activation of TLR4 and TLR9 can induce the production of IL-12 through DCs to differentiate T cells to Th1 phenotype (53–55). TLR4-induced IL-23 also contributes to Th17 cells proliferation (56). Once TLRs are activated, pro-inflammatory cytokines IL-1α, I L - 1β, I L - 6, TNF α produced by gut-associated immune cells can cross the BBB to the brain via diffusion or cytokine transporters, where they act on receptors on microglia and stimulate further cytokine release from microglia (57).
2.5. Neurotransmitters
Levels of host neurotransmitters can be regulated by the gut microbiota, which have an extensive crosstalk with immune cells. Neurotransmitters are actively involved in various brain functions including movement, emotion, learning, and memory (58, 59). Neurotransmitters such as glutamate, acetylcholine (ACh), and dopamine have excitatory effects on neurons GABA, glycine, and serotonin have inhibitory effects on neuron (60, 61). Imbalances of these neurotransmitters can lead to neurological and psychological disorders such as Alzheimer's disease, Parkinson's disease, autism spectrum disorder, anxiety disorders, and depressive disorders (62).
2.5.1. Tryptophan and serotonin
Serotonin, which is also known as 5-hydroxytryptamine (5-HT). Certain resident bacteria, such as Streptococcus and Enterococcus, produce 5-HT directly (63). Bifidobacterium infantis has been demonstrated to affect central 5-HT by elevate plasma tryptophan levels, a precursor of 5-HT (64). 5-HT is involved in the regulation of numerous physiological processes, including respiration, gastrointestinal peristalsis and secretion, vasoconstriction, neurological function, and behavior. Several of the 5-HT receptors are associated with immune cells such as DCs, macrophages, monocytes, and lymphocytes (65). The expression of 5-HT receptors (5-HTR's) on T cells, specifically mRNA expression for 5-HTR1a and 5-HTR2 in naive CD4 T cells and Th1, Th17, and Tregs (66, 67). Indeed, 5-HT has been shown to activate T cells via 5-HTR3-mediated signaling, increase intracellular Na+ and facilitate T cells proliferation (68). These open up new avenues of understanding how 5-HT influences T cells function.
2.5.2. GABA
As well as producing precursors, many bacteria can synthesize and release neurotransmitters, several microorganisms such as Bacteroides, Parabacteroides, and Bifidobacterium Escherichia produce GABA (69). In addition, Lactobacillus rhamnosus produces GABA and regulates GABAAα2 and GABAB1b receptors in the brain, thereby attenuating anxiety-like and depression behavior in mice (13). In the immune system, the GABAergic signaling system plays a key role in response to various inflammatory disorders and influences various functional properties of the immune cells, such as LPS-induced cytokine release, antigen-induced T-cells proliferation, cytotoxicity and chemotaxis of effector T-cells activity (70). GABA transporters that critically implicated in GABA secretion have been identified in mononuclear macrophages and CD4 T cells (71). GABA transporter GAT-1 is expressed on activated T cells, and inhibits the proliferation of CD4 T cells (72). GABA acts as a negative regulator of macrophage and microglial production of inflammatory cytokines and T cell activation via regulating the function of antigen presenting cells (APC) (71, 73) and blocking calcium signaling and NF-κ activity (74).
2.5.3. Dopamine
Dopamine, a precursor for other catecholamines, like epinephrine (E) and norepinephrine (NE), associated with a variety of neurological processes, including motor control, cognition, learning and reward, which can be produced by Bacillus (75). Dopamine stimulates T cells activation resulting in TNFα and IL-10 cytokine production from naive T cells (76). By activating T cell D1 receptors, dopamine activates T cells via inhibiting function of Tregs (77). NE is known for its role in behavior and cognition, like memory, learning, and attention, which can be produced by Saccharomyces, Escheridia, and Bacillu s(78). By binding to adrenergic receptors, E and NE regulate immune activity (79). NE restricts the activation of microglia and diminishes pro-inflammatory mediators production including IL-1β, TNF-α (80).
2.5.4. Ach
ACh, synthesized and released by the parasympathetic nerves, directly affects immune cells via muscarinic Ach receptors and nicotinic Ach receptors, which can also be produced by Lactobacillus(81, 82). ACh exerts an anti-inflammatory effect on macrophages, mast cells, and basophils via α7-nicotinic acetylcholine receptors (α7nAChRs) (83), receptors on inflammatory macrophages. Activation of the cholinergic system by α7nAChR reduces inflammation levels in experimental autoimmune encephalomyelitis (EAE) (84). Also, ACh treatment may diminish the levels of pro-inflammatory cytokines in the blood such as IL-1β, IL-6, and TNF-α in mice (85). Furthermore, ACh implicates in the anti-inflammation via down-regulation inflammatory cytokine synthesis and inhibition of NF-κB nuclear translocation (86). Since acetylcholinesterase (AChE) inactivates acetylcholine, Li et al. reported that inhibition of AChE can play a positive role in the treatment of autoimmune diseases (87).
2.6. HPA axis
Neurotransmitters and cytokines coming from the above systems might influence the. Within the nervous system, stress can also activate the HPA axis response (88–90). Activation of HPA axis causes hypothalamic neurons to secrete corticotropin receptor hormone (CRH), which enters the brain or portal circulation, triggering the release of corticotropin (ACTH), which then initiates the synthesis and release of glucocorticoids (GCs). As a mediator between the nervous and immune systems, GCs has an inhibitory effect on almost all immune cells, including lymphocytes, macrophages, neutrophils, mast cells and so on. During acute stress, through the HPA axis, adrenal corticokinin concentration in blood can be increased and immune function can be inhibited, which is one of the main ways for stress to inhibit immune function (91, 92). They may also promote the development of pathogenic Th cells and cause tissue damage through neural and intestinal inflammation, in turn, affect intestinal barrier integrity (93).
In conclusion, the gut microbiota regulates brain development by the bidirectional pathway of the GBA; thus, it can change host behavior and affect the occurrence and development of diseases (94). Studying the roles and mechanisms of microorganisms associated with the GBA will provide new means for diagnosing and treating neuroimmune diseases.
3. Fecal microbiota transplantation (FMT)
FMT refers to transplanting the gut microbiota from a healthy donor's feces into a patient's gastrointestinal tract to rebuild intestinal microecology of the recipient (95). Recent evidence suggests that the gut microbiota probably plays a causal role in the pathophysiology and pathogenesis of some diseases. Therefore, changing the composition of gut bacteria may affect the course of some diseases. In most cases, the causal pathogen is unlikely to be a single microorganism. FMT can remedy an altered structure of gut microbiota in disease to a certain degree. Therefore, compared with probiotics, one superiority of FMT is the transplantation of intact and healthy gut microbiota. FMT has been successfully used in recurrent or refractory Clostridium infection. FMT is widely used because of its high safety and good efficacy and plays a crucial role in the treatment of autoimmune enteritis, hepatitis B, chronic nonalcoholic cirrhosis, epilepsy, and autism spectrum disorder (96). However, as a biological treatment, FMT also has side effects similar to all medicines and medical therapies. The most common side effects after FMT are often related to constipation, diarrhea, abdominal pain, and transient low-grade fevers, all of which may subside within a few days to weeks (97–99). These side effects are the natural response of the body after the introduction of live microbes and their metabolites. Although extremely rare, serious adverse events and mortality have been reported, but in many instances, have been found as a result of comorbidities or causes other than FMT.
4. The gut microbiota and FMT in neuroimmune diseases
Emerging data have suggested that gut microbiota dysbiosis may be related to the progress of neuroimmune diseases, namely, myasthenia gravis (MG), multiple sclerosis (MS), neuromyelitis optica spectrum disorders (NMOSDs), autoimmune encephalitis (AIE), and Guillain–Barré syndrome (GBS). The exact pathogenesis of these diseases is not completely clear. FMT, which is aimed at reconstructing the gut microbiota, has been proposed as a new therapy for neuroimmune disease treatment. In addition to the gut microbiota, bacterial metabolites (SCFAs) are associated with these diseases. The roles of the gut microbiota and the effects of FMT in neuroimmune diseases are summarized below.
4.1. Myasthenia gravis (MG)
MG is an autoimmune disease caused by autoantibodies that target the neuromuscular junction, resulting in muscle weakness and fatigue (100). Patients with MG whose weakness is confined to the extraocular muscles are referred to oculomotor myasthenia gravis (OMG). Ocular muscle weakness is the most common presenting symptom. Once fatigue spreads to the bulbar, limb, axial, and ventilator muscles, the disorder can progress to generalized myasthenia gravis (GMG) (101). The typical pathological change in MG patients is the formation of germinal centers in the thymus (102). Acetylcholine receptor (AChR) antibodies can be detected with routine assays in 70–80% of MG patients, and antibody titers tend to correlate with disease severity. The production of AChR antibodies is relevant to the disequilibrium of Th17 and Treg cells (103). Hence, rebuilding the Thl7/Treg balance has promising applications for the biological target therapy of MG.
Studies on the close relationship between severe MG and intestinal microbiota have found lower microbial diversity in MG patients than in HCs, suggesting an abnormal microbial status (104, 105). In particular, SCFAs in the feces of MG patients are significantly reduced compared with those in the feces of HCs. Moreover, phylum-level analyses have indicated the decreased abundance of the phylum Firmicutes along with an increased abundance of the phyla Bacteroidetes and Proteobacteria in MG patients' fecal samples. Hence, the ratio of Firmicutes/Bacteroidetes (F/B ratio) is obviously low in MG patients (106), which would reduce the production of SCFAs, resulting in modifications of immune homeostasis and intestinal permeability, thus leading to the exacerbation of autoimmune diseases (107). In another study, compared with the HCs, the MG patients were found to harbor significantly lower relative proportions of the families Bifidobacteriaceae and Verrucomicrobiaceae as well as Coriobacteriaceae, Flavobacteriaceae, and Leuconostocacceae. In contrast, the MG patients were shown to harbor higher proportions of Desulfovibrionaceae, Acidaminococcaceae, and Pasteurellaceae. At the genus level, the relative abundances of Clostridium and Eubacterium were sharply lower in the MG patients, while the proportions of Streptococcus and Parasutterella were enriched (108). Surana et al. found that the main characteristic of MG patients was a decrease in OTUs belonging to Lachnospiraceae (23 OTUs) and Ruminococcaceae (8 OTUs) (109) (Table 1). Lachnospira and Ruminococcaceae are two of the most abundant families in Clostridiales and have been associated with the maintenance of gut health. There is ample evidence demonstrating that the abundance of clostridia has profound effects on the production of SCFAs (110). These SCFAs could affect T cells by regulating their differentiation into Tregs. To directly examine the effect of Clostridium on the induction of colonic Tregs, Atarashi et al. used chloroform to treat the feces of conventionally reared mice. Then, they obtained 46 strains of clostridia and inoculated them into GF mice, inducing a robust accumulation of Tregs in the colons lamina propria (LP) of these mice (125). It provides new insights into the treatment of MG at the microbial level.
Experimental autoimmune myasthenia gravis (EAMG) in the susceptible Lewis rat is a well-accepted animal model elucidating the pathogenesis of the disease and developing new or improved MG therapies. Rinaldi et al. found a strong reduction in Lachnospiraceae abundance in chronic EAMG mice (Table 1) and an increase in the Ruminococcaceae/Lachnospiraceae (R/L) ratio (111). In a study, the total distance traveled by GF mice colonized with MG microbiota (MMb) was substantially decreased relative to the mice colonized with healthy microbiota (HMb) during the open-field test (OFT). However, this effect could be reversed after co-inoculation with both MMb and HMb, indicating that an intervention with the microbiota may be a potential therapeutic strategy for MG (31).
4.2. Multiple sclerosis (MS)
MS is an autoimmune disease characterized by progressive demyelination and deterioration of neurologic function (126, 127). There are four clinical forms of MS: primary progressive MS (PPMS), relapsing-remitting MS (RRMS), secondary progressive MS (SPMS), and progressive relapsing MS (PRMS) (128, 129). Among them, the most common phenotype of MS (80% of cases) is RRMS, characterized by clearly defined attacks or relapses, followed by a variable degree of recovery (130).
Recently, the role of gut microbiota in the development of multiple sclerosis has received increasing attention, as well as SCFAs. In a Chinese cohort study, fecal SCFAs were discovered to be reduced in MS compared to HCs (131).The majority of intestinal dysbiotic microbiota data for patients with MS are characterized by lower abundances of Butyricimonas, a butyrate-producing genus, in MS patients. These changes may increase the pro-inflammatory autoreactive T cells, i.e., Th17 and Th1 cells in peripheral blood. Increased IL-17 mRNA was first noted in the blood and CSF of MS patients (112). Subsequently, increased Th17 cells and IL-17 protein were found in the brains of MS patients (132). This leads to increased BBB permeability, which in turn leads to increased CNS inflammation (133). Altered intestinal microbiota communities are related to autoimmune responses and systemic inflammatory in the host (117). Indeed, concentrations of fecal SCFAs have been shown to be decreased in RRMS patients compared to HCs (134). Hence, inflammatory trigger could be mediate by a low SCFA-producing microbial community in MS patients (135). Butyrate, produced by Faecalibacterium, Lachnospiraceae, and Anaerostipes, inhibits to suppress CNS demyelination via G-protein-coupled receptor activation and histone deacetylase (113), the main pathological feature in MS. Butyrate can also enhance barrier function and anti-inflammatory activities (26, 136, 137). Longitudinal data for 97 patients with MS who underwent propionate supplementation for at least 1 year showed a reduced annual relapse rate, disability stabilization, and reduced brain atrophy (116, 138). Hence, SCFA supplementation is efficacious in reducing MS clinical severity and inflammation.
Compared with HCs, MS patients have been found to harbor significantly lower relative proportions of Bacteroides, Faecalibacterium, Prevotella, Butyricimonas, Paraprevotella, Haemophilus, Slackia, and Anaerostipes. In contrast, MS patients have been shown to harbor higher proportions of Bifidobacterium, Streptococcus, Methanobrevibacter, and Akkermansia (114, 115). A lower abundance of the phytoestrogen-metabolizing bacterium Prevotella, Parabacteroides, and Adlercreutzia were observed in RRMS patients than in HCs (116, 117) (Table 1). Treatment with estrogens can suppress MS patients' symptoms (139). In a patient with SPMS complicated with recurrent Clostridium difficile infection (CDI), FMT delayed the disease progression and alleviated the recurrent infection of MS, but the Expanded Disability Status Scale (EDSS) score of the patient was stable, and the symptoms did not improve. After FMT treatment for severe constipation, three wheelchair-bound MS patients could defecate normally, and they had a dramatic improvement in neurological symptoms and were able to walk unassisted. Therefore, although the therapeutic effect of FMT is limited, it appears to provide long-term benefits for patients with MS (140).
By using an EAE mouse model, which can best simulate the clinical manifestations and pathophysiological characteristics of MS. Wang et al. found that Adlercreutzia was the most abundant genus related to the differentially expressed genes (DEGs) of the spinal cord in EAE mice treated with FMT. However, the DEGs associated with inflammation were negatively correlated with the relative abundance of Adlercreutzia. These results indicate a potential Adlercreutzia-mediated immune regulation mechanism for FMT treatment of EAE (118). Compared with HCs, EAE mice exhibited significantly decreased relative abundances of total gut microbiota, Bacteroides, and Lactobacillus and considerably increased abundances of Streptococcus, Firmicutes, Tenericutes, and Cyanobacteria (119). Oral administration of the fecal microbiota of MS patients can aggravate the symptoms of EAE mice and reduce the level of the anti-inflammatory cytokine IL-10. FMT-treated EAE mice present changes in abundances of Verrucomicrobia and six intestinal bacterial phyla, Bacteroidetes, Firmicutes, Tenericutes, Cyanobacteria, Proteobacteria, and Actinobacteria, all of which were converted to normal control levels. In addition, FMT can reduce the abundance of Bacteroides and Actinobacteria (118) (Table 1). These results suggest that FMT can alter the structure of gut microbiota in EAE to a certain extent. Compared with those of EAE controls, FMT treatment not only alleviated clinical symptoms, but also significantly reduced clinical scores and cumulative disease scores in mice throughout the clinical course of EAE (141). Li et al. also found that FMT of healthy mice alleviates the symptoms of EAE mice by restoring the integrity of the BBB and axon myelination (142).
4.3. Neuromyelitis optica spectrum disorders (NMOSDs)
NMOSDs are disabling, sometimes fatal CNS inflammatory demyelinating diseases encompassing a brain syndrome, optic neuritis, and acute myelitis (143). Although traditionally considered a severe atypical form of MS, NMOSD is now recognized as a distinct clinical entity (144). Compared with HCs, patients with NMOSD have more aquaporin (AQP) autoantibodies against the optic nerve and spinal cord (145).
A striking depletion of fecal SCFAs with a significantly negative correlation with disease severity has been observed in NMOSD patients (146), showing that the significant reduction in fecal SCFA levels may become another important feature of NMOSD patients. Correlation analysis shows significant reductions in fecal butyrate levels in NMOSD patients. SCFAs play a critical regulatory role in host physiology and immunity. SCFAs have anti-inflammatory effects that are not limited to the intestinal tract, which help increase Treg and suppress the differentiation of Th17 cells. Increased Th17 cells and IL-17 have been noted in patients with NMOSD (147). Therefore, the lack of protection from anti-inflammatory metabolites of beneficial bacteria is also involved in the pathogenesis of NMOSD (146).
The gut microbial composition of NMOSD patients is distinguished from that of HCs. Generally speaking, the gut microbiota of NMOSD patients has a high abundance of Streptococcus, Alistipes, Haemophilus, Veillonella, Butyricimonas, and Rothia. However, the abundance of Clostridium, Parabacteroides, Oxalobacter, and Burkholderia abundances is low (120) (Table 1). Streptococcus, which is significantly increased in NMOSD patients, is positively correlated with disease severity. The use of immunosuppressants results in a decrease in Streptococcus, suggesting that Streptococcus might exert a significant role in the pathogenesis of NMOSD. Remarkably, of bacteria identified at the species level, C. perfringens was the species most significantly enriched in patients with NMOSD compared with HCs (121).
4.4. Autoimmune encephalitis (AIE)
AIE is a neurological disorder caused by inflammation of the brain parenchyma. Its most common cause is an underlying viral infection, but autoimmune factors are increasingly being considered the cause of encephalitis (148, 149). Different antibodies directed mainly toward synaptic receptors, including the N-methyl-D-aspartate receptor (NMDAR), the α-amino-3-hydroxy-5-methyl-4-isoxazole-propionic acid receptor (AMPAR), and the GABA B-receptor (GABABR), contribute to the development of AIE. It has been reported that anti-NMDAR encephalitis is the most prevalent and severe autoimmune encephalitis type (150).
Relative to HCs, anti-NMDAR encephalitis patients have a decreased microbiome alpha-diversity index and marked disturbances in the gut microbial composition. Chen et al. observed a decrease in various SCFA-producing bacteria such as Faecalibacterium, Roseburia, Lachnospira, Ruminococcus, Coprococcus, and Collinsella and an increase in Bacteroides, Enterococcus, Escherichia, Veillonella, Streptococcus, Dorea, Scardovia and Clostridium in anti-NMDAR encephalitis patients relative to HCs (122) (Table 1). The reduction in SCFA production by the microbiome increases the destruction of the intestinal barrier (151). In verifying intestinal mucosal damage, they found that patients with anti-NMDAR encephalitis had significantly elevated serum levels of two chemical markers, D-LAC and DAO, which are usually at higher levels during intestinal barrier disruption (152). Notably, Streptococcus, the tax on most closely associated with NMOSDs, has also been shown to be associated with anti-NMDAR encephalitis and D-LAC, suggesting that it is involved in intestinal mucosal damage (146). Therefore, a lack of protection from anti-inflammatory metabolites produced by beneficial bacteria and abnormal intestinal permeability also take part in the pathogenesis of anti-NMDAR encephalitis. Related to HMb mice, microbiota-depleted mice receiving FMT from anti-NMDAR encephalitis patients had cognitive impairment and hypersensitivity. Furthermore, via FMT, transplantation of anti-NMDAR encephalitis patients into specific pathogen-free (SPF) mice can induce Th17 response and abnormal behaviors. These results suggest the potentially important involvement of the gut microbiota in anti-NMDAR encephalitis (122).
4.5. Guillain–Barré syndrome (GBS)
GBS is a paralytic neuropathy and is considered to be predominantly preceded by an autoimmune response after gastrointestinal infection with Campylobacter jejuni (Table 1) or other immune stimulation. GBS is frequently characterized by the rapid progression of bilateral weakness, combined with autonomic or additional neurologic symptoms. The majority of patients improve, however, permanent disability can occur in some patients (153). The innate immune response to campylobacteriosis is manifested by the accumulation of neutrophils and macrophages, inflammatory mucosal damage, intestinal barrier defects, malabsorption, and ultimately bloody diarrhea (154).
Mice inoculated with Campylobacter jejuni from GBS patients show increased levels of autoantibodies and peripheral nerve injury (123, 124), suggesting that intestinal microbiome dysregulation is closely related to the pathogenesis of GBS. The cross-reaction between LPS on the surface of Campylobacter jejuni and autoimmune anti-ganglioside antibody resulted in complement-mediated nerve damage (155). In addition, Brooks et al. found that Th2 and autoimmune responses in mice infected with Campylobacter jejuni increased after treatment with human FMT. The combination of FMT and antibiotics obviously accelerated the clearance of Campylobacter jejuni in infected mice (156).
5. Conclusion
The gut microbiota regulates the function of the brain by altering the microbiota composition, diversity, and metabolites through the bidirectional pathways between the gut and brain; thus, it can alter host behavior and affect the progression of neuroimmune diseases. FMT positively impacts the composition and function of microbiota by increasing the level of SCFAs. Hence, it is theoretically feasible to apply FMT to diseases with Th17/Treg cell imbalances. Therefore, FMT may be an effective therapy for treating human neuroimmune diseases. However, the evidence level of current clinical research is not sufficient. To date, studies on FMT for many neuroimmune diseases have been limited to animal models. Notably, whether the results obtained in animal experiments can be directly applied to human patients is unclear. At present, further experimental and clinical studies are needed to clarify the mechanism of the gut microbiota and its metabolites in neuroimmune diseases. Nevertheless, transplantations of anti-inflammatory strains and SCFAs seem to be helpful in the treatment of neuroimmune diseases and will provide more methods for treating neuroimmune diseases.
Author contributions
All authors listed have made a substantial, direct, and intellectual contribution to the work and approved it for publication.
Funding
This work was supported by the following grants: National Natural Science Foundation of China (Grant numbers: 81701192 and 81901380), Shandong Provincial Natural Science Foundation, China (Grant numbers: ZR2017BH078 and ZR2017BC047), and Scientific Research Foundation of Binzhou Medical University (Grant numbers: BY2017KYQD15 and BY2016KYQD21).
Conflict of interest
The authors declare that the research was conducted in the absence of any commercial or financial relationships that could be construed as a potential conflict of interest.
Publisher's note
All claims expressed in this article are solely those of the authors and do not necessarily represent those of their affiliated organizations, or those of the publisher, the editors and the reviewers. Any product that may be evaluated in this article, or claim that may be made by its manufacturer, is not guaranteed or endorsed by the publisher.
References
1. Martinez-Guryn K, Leone V, Chang EB. Regional Diversity of the Gastrointestinal Microbiome. Cell Host Microbe. (2019) 26:314–24. doi: 10.1016/j.chom.2019.08.011
2. Vaga S, Lee S, Ji B, Andreasson A, Talley NJ, Agréus L, et al. Compositional and functional differences of the mucosal microbiota along the intestine of healthy individuals. Sci Rep. (2020) 10:14977. doi: 10.1038/s41598-020-71939-2
3. Jandhyala SM, Talukdar R, Subramaniyam C, Vuyyuru H, Sasikala M, Reddy D. Role of the normal gut microbiota. World J Gastroenterol. (2015) 21:8787–803. doi: 10.3748/wjg.v21.i29.8787
4. O'Hara AM Shanahan F The The gut flora as a forgotten organ. EMBO Rep. (2006) 7:688–93. doi: 10.1038/sj.embor.7400731
5. Ciccia F, Gandolfo S Will fecal microbiota transplantation eventually be an effective therapeutic strategy for systemic lupus erythematosus? Clin Immunol. (2022) 242:109096. doi: 10.1016/j.clim.2022.109096
6. Naveed M, Zhou Q, Xu C, Taleb A, Meng F, Ahmed B, et al. Gut-brain axis: A matter of concern in neuropsychiatric disorders! Prog Neuropsychopharmacol Biol Psychiatry. (2021) 104:110051. doi: 10.1016/j.pnpbp.2020.110051
7. Mayer EA, Tillisch K, Gupta A. Gut/brain axis and the microbiota. J Clin Invest. (2015) 125:926–38. doi: 10.1172/JCI76304
8. Mestre L, Carrillo-Salinas FJ, Mecha M, Feliú A, Guaza C. Gut microbiota, cannabinoid system and neuroimmune interactions: New perspectives in multiple sclerosis. Biochem Pharmacol. (2018) 157:51–66. doi: 10.1016/j.bcp.2018.08.037
9. Cryan JF O'Mahony SM The The microbiome-gut-brain axis: from bowel to behavior. Neurogastroenterol Motil. (2011) 23:187–92. doi: 10.1111/j.1365-2982.2010.01664.x
10. Han W, Tellez LA, Perkins MH, Perez IO, Qu T, Ferreira J, et al. A neural circuit for gut-induced reward. Cell. (2018). 175:665–678.e23. doi: 10.1016/j.cell.2018.08.049
11. Lal S, Kirkup AJ, Brunsden AM, Thompson DG, Grundy D. Vagal afferent responses to fatty acids of different chain length in the rat. Am J Physiol Gastrointest Liver Physiol. (2001) 281:G907–15. doi: 10.1152/ajpgi.2001.281.4.G907
12. Goehler LE, Gaykema RP, Nguyen KT, Lee JE, Tilders FJ, Maier SF, et al. Interleukin-1beta in immune cells of the abdominal vagus nerve: a link between the immune and nervous systems? J Neurosci. (1999) 19:2799–806. doi: 10.1523/JNEUROSCI.19-07-02799.1999
13. Bravo JA, Forsythe P, Chew MV, Escaravage E, Savignac HM, Dinan TG, et al. Ingestion of Lactobacillus strain regulates emotional behavior and central GABA receptor expression in a mouse via the vagus nerve. Proc Natl Acad Sci USA. (2011) 108:16050–5. doi: 10.1073/pnas.1102999108
14. Sgritta M, Dooling SW, Buffington SA, Momin EN, Francis MB, Britton RA, et al. Mechanisms underlying microbial-mediated changes in social behavior in mouse models of autism spectrum disorder. Neuron. (2019) 101:246–259.e6. doi: 10.1016/j.neuron.2018.11.018
15. Buffington SA, Di Prisco GV, Auchtung TA, Ajami NJ, Petrosino JF, Costa-Mattioli M. Microbial reconstitution reverses maternal diet-induced social and synaptic deficits in offspring. Cell. (2016) 165:1762–75. doi: 10.1016/j.cell.2016.06.001
16. Meneses G, Bautista M, Florentino A, Díaz G, Acero G, Besedovsky H, et al. Electric stimulation of the vagus nerve reduced mouse neuroinflammation induced by lipopolysaccharide. J Inflamm (Lond). (2016) 13:33. doi: 10.1186/s12950-016-0140-5
17. Zhang J, Ma L, Chang L, Pu Y, Ou Y, Hashmoto K. A key role of the subdiaphragmatic vagus nerve in the depression-like phenotype and abnormal composition of gut microbiota in mice after lipopolysaccharide administration. Transl Psychiatry. (2020) 10:186. doi: 10.1038/s41398-020-00878-3
18. Svensson E, Horváth-Puhó E, Thomsen RW, Djurhuus JC, Pedersen L, Borghammer P, et al. Vagotomy and subsequent risk of Parkinson's disease. Ann Neurol. (2015) 78:522–9. doi: 10.1002/ana.24448
19. Anthony WE, Burnham C-AD, Dantas G, Kwon JH. The gut microbiome as a reservoir for antimicrobial resistance. J Infect Dis. (2021) 223:S209–S213. doi: 10.1093/infdis/jiaa497
20. Cox LM, Weiner HL. Microbiota signaling pathways that influence neurologic disease. Neurotherapeutics. (2018) 15:135–45. doi: 10.1007/s13311-017-0598-8
21. Silva YP, Bernardi A, Frozza RL. The role of short-chain fatty acids from gut microbiota in gut-brain communication. Front Endocrinol (Lausanne). (2020) 11:25. doi: 10.3389/fendo.2020.00025
22. Canfora EE, Jocken JW, Blaak EE. Short-chain fatty acids in control of body weight and insulin sensitivity. Nat Rev Endocrinol. (2015) 11:577–91. doi: 10.1038/nrendo.2015.128
23. Louis P. and Flint HJ, Formation of propionate and butyrate by the human colonic microbiota. Environ Microbiol. (2017) 19:29–41. doi: 10.1111/1462-2920.13589
24. Flint HJ, Duncan SY, Scott KP, Louis P. Links between diet, gut microbiota composition and gut metabolism. Proc Nutr Soc. (2015) 74:13–22. doi: 10.1017/S0029665114001463
25. Wong JM, Souza R, Kendell C, Eman A, Jenkins D. Colonic health: fermentation and short chain fatty acids. J Clin Gastroenterol. (2006) 40:235–43. doi: 10.1097/00004836-200603000-00015
26. Furusawa Y, Obata Y, Fukuda S, Endo TA, Nakato G, Takahashi D, et al. Commensal microbe-derived butyrate induces the differentiation of colonic regulatory T cells. Nature. (2013) 504:446–50. doi: 10.1038/nature12721
27. Brown AJ, Goldsworthy SM, Barnes AA, Eilert MM, Tcheang L, Daniels D, et al. The Orphan G protein-coupled receptors GPR41 and GPR43 are activated by propionate and other short chain carboxylic acids. J Biol Chem. (2003) 278:11312–9. doi: 10.1074/jbc.M211609200
28. Rodriguez-Perea AL, Arcia ED, Rueda CM, Velila PA. Phenotypical characterization of regulatory T cells in humans and rodents. Clin Exp Immunol. (2016) 185:281–91. doi: 10.1111/cei.12804
29. Scott DW. Driving CARs to BARs: the winding road to specific regulatory T cells for tolerance. Front Immunol. (2021) 12:742719. doi: 10.3389/fimmu.2021.742719
30. Diller ML, Kudchadkar RR, Delman KA, Lawson DH, Ford ML. Balancing inflammation: the link between Th17 and regulatory T cells. Mediators Inflamm. (2016) 2016:6309219. doi: 10.1155/2016/6309219
31. Tan X, Huang Y, Chai T, Zhao X, Li Y, Wu J, et al. Differential gut microbiota and fecal metabolites related with the clinical subtypes of myasthenia gravis. Front Microbiol. (2020) 11:564579. doi: 10.3389/fmicb.2020.564579
32. Poul EL, Loison C, Struyf S, Springael J-Y, Lannoy V, Decobecq M-E, et al. Functional characterization of human receptors for short chain fatty acids and their role in polymorphonuclear cell activation. J Biol Chem. (2003) 278:25481–9. doi: 10.1074/jbc.M301403200
33. Thangaraju M, Cresci GA, Liu K, Ananth S, Gnanaprakasam JP, Browning DD, et al. GPR109A is a G-protein-coupled receptor for the bacterial fermentation product butyrate and functions as a tumor suppressor in colon. Cancer Res. (2009) 69:2826–32. doi: 10.1158/0008-5472.CAN-08-4466
34. Singh N, Gurav A, Sivaprakasam S, Brady E, Padia R, Shi H, et al. Activation of Gpr109a, receptor for niacin and the commensal metabolite butyrate, suppresses colonic inflammation and carcinogenesis. Immunity. (2014) 40:128–39. doi: 10.1016/j.immuni.2013.12.007
35. Arpaia N, Campbell C, Fan X, Dikiy S, van der Veekan J, deRoos P, et al. Metabolites produced by commensal bacteria promote peripheral regulatory T-cell generation. Nature. (2013) 504:451–5. doi: 10.1038/nature12726
36. Thangaraju M, Carswell KN, Prasad PD, Ganapathy V. Colon cancer cells maintain low levels of pyruvate to avoid cell death caused by inhibition of HDAC1/HDAC3. Biochem J. (2009) 417:379–89. doi: 10.1042/BJ20081132
37. Davie JR. Inhibition of histone deacetylase activity by butyrate. J Nutr. (2003) 133:2485S−93S. doi: 10.1093/jn/133.7.2485S
38. Sanford JA, Zhang L-J, Williams MR, Gangoiti JA, Huang C-M, Gallo RL. Inhibition of HDAC8 and HDAC9 by microbial short-chain fatty acids breaks immune tolerance of the epidermis to TLR ligands. Sci Immunol. (2016) 1:4. doi: 10.1126/sciimmunol.aah4609
39. Huang J, Wang L, Dahyia S, Beier UH, Han R, Samanta A, et al. Histone/protein deacetylase 11 targeting promotes Foxp3+ Treg function. Sci Rep. (2017) 7:8626. doi: 10.1038/s41598-017-09211-3
40. Liu L, Li L, Min J, Wang J, Wu H, Zeng Y, et al. Butyrate interferes with the differentiation and function of human monocyte-derived dendritic cells. Cell Immunol. (2012) 277:66–73. doi: 10.1016/j.cellimm.2012.05.011
41. Millard AL, Mertes PM, Ittelet D, Villard F, Jeannesson P, Bernard J. Butyrate affects differentiation, maturation and function of human monocyte-derived dendritic cells and macrophages. Clin Exp Immunol. (2002) 130:245–55. doi: 10.1046/j.0009-9104.2002.01977.x
42. Singh N, Thangaraju M, Prasad PD, Martin PM, Lambert NA, Boettger T, et al. Blockade of dendritic cell development by bacterial fermentation products butyrate and propionate through a transporter (Slc5a8)-dependent inhibition of histone deacetylases. J Biol Chem. (2010) 285:27601–8. doi: 10.1074/jbc.M110.102947
43. Corrêa-Oliveira R, Fachi JL, Vieira A, Sato FT, Vinolo MAR. Regulation of immune cell function by short-chain fatty acids. Clin Transl Immunology. (2016) 5:e73. doi: 10.1038/cti.2016.17
44. Erny D, de Angelis ALH, Jaitin D, Wieghofer P, Staszewski O, David E, et al. Host microbiota constantly control maturation and function of microglia in the CNS. Nat Neurosci. (2015) 18:965–77. doi: 10.1038/nn.4030
45. Fung TC, Olson CA, Hsiao EY. Interactions between the microbiota, immune and nervous systems in health and disease. Nat Neurosci. (2017) 20:145–55. doi: 10.1038/nn.4476
46. Nguyen M. and Palm NW, Gut instincts in neuroimmunity from the eighteenth to twenty-first centuries. Semin Immunopathol. (2022) 44:569–79. doi: 10.1007/s00281-022-00948-2
47. Gaboriau-Routhiau V, Rakotobe S, Lécuyer E, Mulder I, Lan A, Bridonneau C, et al. The key role of segmented filamentous bacteria in the coordinated maturation of gut helper T cell responses. Immunity. (2009) 31:677–89. doi: 10.1016/j.immuni.2009.08.020
48. Singhal G, Fisher FM, Chee MJ, Tan TG, Ouaamari AE, Adams AC, et al. Fibroblast growth factor 21 (FGF21) protects against high fat diet induced inflammation and islet hyperplasia in pancreas. PLoS ONE. (2016) 11:e0148252. doi: 10.1371/journal.pone.0148252
49. Round JL, Lee SM, Li J, Tran G, Jabri B, Chatila TA, et al. The Toll-like receptor 2 pathway establishes colonization by a commensal of the human microbiota. Science. (2011) 332:974–7. doi: 10.1126/science.1206095
50. Round JL. and Mazmanian SK, Inducible Foxp3+ regulatory T-cell development by a commensal bacterium of the intestinal microbiota. Proc Natl Acad Sci U S A. (2010) 107:12204–9. doi: 10.1073/pnas.0909122107
51. Rogier R, Koenders MI, Abdollahi-Roodsaz S. Toll-like receptor mediated modulation of T cell response by commensal intestinal microbiota as a trigger for autoimmune arthritis. J Immunol Res. (2015) 2015:527696. doi: 10.1155/2015/527696
52. Hopkins PA, Sriskandan S. Mammalian toll-like receptors: to immunity and beyond. Clin Exp Immunol. (2005) 140:395–407. doi: 10.1111/j.1365-2249.2005.02801.x
53. Krieg AM. CpG motifs in bacterial DNA and their immune effects. Annu Rev Immunol. (2002) 20:709–60. doi: 10.1146/annurev.immunol.20.100301.064842
54. Trinchieri G. Interleukin-12 and the regulation of innate resistance and adaptive immunity. Nat Rev Immunol. (2003) 3:133–46. doi: 10.1038/nri1001
55. Agrawal S, Agrawal A, Doughty B, Gerwitz A, Blenis J, Van Dyke T, et al. Cutting edge: different Toll-like receptor agonists instruct dendritic cells to induce distinct Th responses via differential modulation of extracellular signal-regulated kinase-mitogen-activated protein kinase and c-Fos. J Immunol. (2003) 171:4984–9. doi: 10.4049/jimmunol.171.10.4984
56. Kattah MG, Wong MT, Yocum MD, Utz PJ. Cytokines secreted in response to Toll-like receptor ligand stimulation modulate differentiation of human Th17 cells. Arthritis Rheum. (2008) 58:1619–29. doi: 10.1002/art.23497
57. Needham BD, Adame MD, Serena G, Rose DR, Preston GM, Conrad MC, et al. Plasma and fecal metabolite profiles in autism spectrum disorder. Biol Psychiatry. (2021) 89:451–62. doi: 10.1016/j.biopsych.2020.09.025
58. Jameson KG, Olson CA, Kazmi SA, Hsiao EY. Toward understanding microbiome-neuronal signaling. Mol Cell. (2020) 78:577–83. doi: 10.1016/j.molcel.2020.03.006
59. Kaelberer MM, Rupprecht LE, Liu WW, Weng P, Bohórquez DV. Neuropod cells: the emerging biology of gut-brain sensory transduction. Annu Rev Neurosci. (2020) 43:337–53. doi: 10.1146/annurev-neuro-091619-022657
60. Sarkar A, Lehto SM, Harty S, Dinan TG, Cryan JF, Burnet PWJ. Psychobiotics and the manipulation of bacteria-gut-brain signals. Trends Neurosci. (2016) 39:763–81. doi: 10.1016/j.tins.2016.09.002
61. Strandwitz P. Neurotransmitter modulation by the gut microbiota. Brain Res. (2018) 1693:128–133. doi: 10.1016/j.brainres.2018.03.015
62. Chen Y, Xu J, Chen Y. Regulation of neurotransmitters by the gut microbiota and effects on cognition in neurological disorders. Nutrients. (2021) 13:6. doi: 10.3390/nu13062099
63. Mishima Y, Ishihara S. Enteric microbiota-mediated serotonergic signaling in pathogenesis of irritable bowel syndrome. Int J Mol Sci, (2021) 22:19. doi: 10.3390/ijms221910235
64. Desbonnet L, Garrett L, Clarke G, Kiely B, Cryan JF, Dinan TG. Effects of the probiotic Bifidobacterium infantis in the maternal separation model of depression. Neuroscience. (2010) 170:1179–88. doi: 10.1016/j.neuroscience.2010.08.005
65. Cloez-Tayarani I. and Changeux JP, Nicotine and serotonin in immune regulation and inflammatory processes: a perspective. J Leukoc Biol. (2007) 81:599–606. doi: 10.1189/jlb.0906544
66. Regmi SC, Park S-Y, Ku SK, Kim J-A. Serotonin regulates innate immune responses of colon epithelial cells through Nox2-derived reactive oxygen species. Free Radic Biol Med. (2014) 69:377–89. doi: 10.1016/j.freeradbiomed.2014.02.003
67. Wu H, Herr D, Maclver NJ, Rathwell JC, Gerriets VA. CD4 T cells differentially express cellular machinery for serotonin signaling, synthesis, and metabolism. Int Immunopharmacol. (2020) 88:106922. doi: 10.1016/j.intimp.2020.106922
68. Karmakar S Lal G Role Role of serotonin receptor signaling in cancer cells and anti-tumor immunity. Theranostics. (2021) 11:5296–312. doi: 10.7150/thno.55986
69. Strandwitz P, Kim KH, Terekhova D, Liu JK, Sharma A, Levering J, et al. GABA-modulating bacteria of the human gut microbiota. Nat Microbiol. (2019) 4:396–403. doi: 10.1038/s41564-018-0307-3
70. Paschoalin T, Carmona AK, Rodrigues EG, Oliveira V, Monteiro HP, Juliano MA, et al. Characterization of thimet oligopeptidase and neurolysin activities in B16F10-Nex2 tumor cells and their involvement in angiogenesis and tumor growth. Mol Cancer. (2007) 6:44. doi: 10.1186/1476-4598-6-44
71. Bhat R, Axtell R, Mitra A, Miranda M, Lock C, Tsien RW, et al. Inhibitory role for GABA in autoimmune inflammation. Proc Natl Acad Sci U S A. (2010) 107:2580–5. doi: 10.1073/pnas.0915139107
72. Redecker P. Immunoreactivity for multiple GABA transporters (GAT-1, GAT-2, GAT-3) in the gerbil pineal gland. Neurosci Lett. (1999) 266:117–20. doi: 10.1016/S0304-3940(99)00268-2
73. Barragan A, Weidner JM, Jin Z, Korpi ER, Birnir B. GABAergic signalling in the immune system. Acta Physiol (Oxf). (2015) 213:819–27. doi: 10.1111/apha.12467
74. Prud'Homme GJ, Glinka Y, Wang Q. Immunological GABAergic interactions and therapeutic applications in autoimmune diseases. Autoimmun Rev. (2015) 14:1048–56. doi: 10.1016/j.autrev.2015.07.011
75. Matt S.M, Gaskill PJ Where is dopamine and how do immune cells see it?: dopamine-mediated immune cell function in health and disease. J Neuroimmune Pharmacol, (2020) 15:114–64. doi: 10.1007/s11481-019-09851-4
76. Besser MJ, Ganor Y, Levite M. Dopamine by itself activates either D2, D3 or D1/D5 dopaminergic receptors in normal human T-cells and triggers the selective secretion of either IL-10, TNFalpha or both. J Neuroimmunol. (2005) 169:161–71. doi: 10.1016/j.jneuroim.2005.07.013
77. Cosentino M, Fietta AM, Ferrari M, Rasini E, Bombelli R, Carcano E, et al. Human CD4+CD25+ regulatory T cells selectively express tyrosine hydroxylase and contain endogenous catecholamines subserving an autocrine/paracrine inhibitory functional loop. Blood. (2007) 109:632–42. doi: 10.1182/blood-2006-01-028423
78. Borodovitsyna O, Flamini M, Chandler D. Noradrenergic modulation of cognition in health and disease. Neural Plast. (2017) 2017:6031478. doi: 10.1155/2017/6031478
79. Sneader W. The discovery and synthesis of epinephrine. Drug News Perspect. (2001) 14:491–4. doi: 10.1358/dnp.2001.14.8.858417
80. O'Neill E Harkin A Targeting the noradrenergic system for anti-inflammatory and neuroprotective effects: implications for Parkinson's disease. Neural Regen Res. (2018) 13:1332–7. doi: 10.4103/1673-5374.235219
81. Lyte M. Microbial endocrinology in the microbiome-gut-brain axis: how bacterial production and utilization of neurochemicals influence behavior. PLoS Pathog. (2013) 9:e1003726. doi: 10.1371/journal.ppat.1003726
82. Lyte M. Microbial endocrinology and the microbiota-gut-brain axis. Adv Exp Med Biol. (2014) 817:3–24. doi: 10.1007/978-1-4939-0897-4_1
83. Bosmans G, Bassi GS, Florens M, Gonzalez-Dominguez E, Matteoli G, Boekxstaens GE. Cholinergic modulation of type 2 immune responses. Front Immunol. (2017) 8:1873. doi: 10.3389/fimmu.2017.01873
84. Kempuraj D, Thangavel R, Kempuraj DD, Ahmed ME, Selvakumar GP, Raikwar SP, et al. Neuroprotective effects of flavone luteolin in neuroinflammation and neurotrauma. Biofactors. (2021) 47:190–7. doi: 10.1002/biof.1687
85. Zabrodskii PF. Effect of acetylcholine on mortality of mice from sepsis and proinflammatory cytokine production. Bull Exp Biol Med. (2011) 150:340–2. doi: 10.1007/s10517-011-1137-y
86. Baez-Pagan CA, Delgado-Velez M, Lasalde-Dominicci JA. Activation of the macrophage alpha7 nicotinic acetylcholine receptor and control of inflammation. J Neuroimmune Pharmacol. (2015) 10:468–76. doi: 10.1007/s11481-015-9601-5
87. Kaushik V, Smith ST, Mikobi E, Raji MA. Acetylcholinesterase inhibitors: beneficial effects on comorbidities in patients with Alzheimer's disease. Am J Alzheimers Dis Other Demen. (2018) 33:73–85. doi: 10.1177/1533317517734352
88. Bellavance MA, Rivest S. The HPA - immune axis and the immunomodulatory actions of glucocorticoids in the brain. Front Immunol. (2014) 5:136. doi: 10.3389/fimmu.2014.00136
89. Hueston CM, Deak T. The inflamed axis: the interaction between stress, hormones, and the expression of inflammatory-related genes within key structures comprising the hypothalamic-pituitary-adrenal axis. Physiol Behav. (2014) 124:77–91. doi: 10.1016/j.physbeh.2013.10.035
90. Forsythe P, Kunze W, Bienenstock J. Moody microbes or fecal phrenology: what do we know about the microbiota-gut-brain axis? BMC Med. (2016) 14:58. doi: 10.1186/s12916-016-0604-8
91. Morais LH, Schreiber HT, Mazmanian SK. The gut microbiota-brain axis in behaviour and brain disorders. Nat Rev Microbiol. (2021) 19:241–55. doi: 10.1038/s41579-020-00460-0
92. Hodo TW, de Aquino MTP, Shimamoto A, Shanker A. Critical neurotransmitters in the neuroimmune network. Front Immunol. (2020) 11:1869. doi: 10.3389/fimmu.2020.01869
93. Shimba A, Ikuta K. Control of immunity by glucocorticoids in health and disease. Semin Immunopathol. (2020) 42:669–80. doi: 10.1007/s00281-020-00827-8
94. Jangi S, Gandhi R, Cox LM, Li N, Glehn F, Yan R, et al. Alterations of the human gut microbiome in multiple sclerosis. Nat Commun. (2016) 7:12015.
95. Antushevich H. Fecal microbiota transplantation in disease therapy. Clinica Chimica Acta. (2020) 503:90–8. doi: 10.1016/j.cca.2019.12.010
96. Kang DW, Adams JB, Gregory AC, Borody T, Chittick L, Fasano A, et al. Microbiota Transfer Therapy alters gut ecosystem and improves gastrointestinal and autism symptoms: an open-label study. Microbiome. (2017) 5:10. doi: 10.1186/s40168-016-0225-7
97. Baxter M, Colville A. Adverse events in faecal microbiota transplant: a review of the literature. J Hosp Infect. (2016) 92:117–27. doi: 10.1016/j.jhin.2015.10.024
98. Wang JW, Kuo C, Kuo F, Wang Y, Hsu W, Yu F, et al. Fecal microbiota transplantation: review and update. J Formos Med Assoc. (2019) 118:S23–31. doi: 10.1016/j.jfma.2018.08.011
99. Dailey FE, Turse EP, Daglilar E, Tahan V. The dirty aspects of fecal microbiota transplantation: a review of its adverse effects and complications. Curr Opin Pharmacol. (2019) 49:29–33. doi: 10.1016/j.coph.2019.04.008
100. Gilhus NE, Skeie GO, Romi F, Lazaridis K, Zisimopoulou P, Tzartos S. Myasthenia gravis - autoantibody characteristics and their implications for therapy. Nat Rev Neurol. (2016) 12:259–68. doi: 10.1038/nrneurol.2016.44
101. Gilhus NE, Verschuuren JJ. Myasthenia gravis: subgroup classification and therapeutic strategies. Lancet Neurol. (2015) 14:1023–36. doi: 10.1016/S1474-4422(15)00145-3
102. Le Panse R, Cizeron-Clairac G, Cuvelier M, Truffault F, Bismuth J, Nancy P, et al. Regulatory and pathogenic mechanisms in human autoimmune myasthenia gravis. Ann N Y Acad Sci. (2008) 1132:135–42. doi: 10.1196/annals.1405.019
103. Yi JS, Guptill JT, Stathopoulos P, Nowak R, O'Connor K. B cells in the pathophysiology of myasthenia gravis. Muscle Nerve. (2018) 57:172–84. doi: 10.1002/mus.25973
104. Human Microbiome Project Consortium. Structure, function and diversity of the healthy human microbiome. Nature. (2012) 486:207–14. doi: 10.1038/nature11234
105. Stoffel MA, Acevedo-Whitehouse K, Morales-Durán N, Grosser S, Chakarov N, Krüger O, et al. Early sexual dimorphism in the developing gut microbiome of northern elephant seals. Mol Ecol. (2020) 29:2109–22. doi: 10.1111/mec.15385
106. Stojanov S, Berlec A, Strukelj B. The influence of probiotics on the firmicutes/bacteroidetes ratio in the treatment of obesity and inflammatory bowel disease. Microorganisms. (2020) 8:11. doi: 10.3390/microorganisms8111715
107. Correale J. Immunosuppressive amino-acid catabolizing enzymes in multiple sclerosis. Front Immunol. (2020) 11:600428. doi: 10.3389/fimmu.2020.600428
108. Qiu D, Xia Z, Jiao X, Deng J, Zhang L, Li J. Altered gut microbiota in myasthenia gravis. Front Microbiol. (2018) 9:2627. doi: 10.3389/fmicb.2018.02627
109. Surana NK, Kasper DL. Moving beyond microbiome-wide associations to causal microbe identification. Nature. (2017) 552:244–7. doi: 10.1038/nature25019
110. Kuhn KA, Stappenbeck TS. Peripheral education of the immune system by the colonic microbiota. Semin Immunol. (2013) 25:364–9. doi: 10.1016/j.smim.2013.10.002
111. Rinaldi E, Consonni A, Cordiglieri C, Sacco G, Crasà C, Fontana A, et al. Therapeutic effect of bifidobacterium administration on experimental autoimmune myasthenia gravis in lewis rats. Front Immunol. (2019) 10:2949. doi: 10.3389/fimmu.2019.02949
112. Matusevicius D, Kivisäkk P, He B, Kostulas N, Ozenci V, Fredrikson S, et al. Interleukin-17 mRNA expression in blood and CSF mononuclear cells is augmented in multiple sclerosis. Mult Scler. (1999) 5:101–4. doi: 10.1177/135245859900500206
113. Chen T, Noto D, Hoshino Y, Mizuno M, Miyake S. Butyrate suppresses demyelination and enhances remyelination. J Neuroinflammation. (2019) 16:165. doi: 10.1186/s12974-019-1552-y
114. Miyake S, Kim S, Suda W, Oshima K, Nakamura M, Matsuoka T, et al. Dysbiosis in the gut microbiota of patients with multiple sclerosis, with a striking depletion of species belonging to clostridia XIVa and IV clusters. PLoS ONE. (2015) 10:e0137429. doi: 10.1371/journal.pone.0137429
115. Berer K, Gerdes LA, Cekanaviciute E, Jia X, Xiao L, Xia Z, et al. Gut microbiota from multiple sclerosis patients enables spontaneous autoimmune encephalomyelitis in mice. Proc Natl Acad Sci U S A. (2017) 114:10719–24. doi: 10.1073/pnas.1711233114
116. Chen J, Chia N, Kalari KR, Yao JZ, Novotna M, Soldan MMP, et al. Multiple sclerosis patients have a distinct gut microbiota compared to healthy controls. Sci Rep. (2016) 6:28484. doi: 10.1038/srep28484
117. Cekanaviciute E, Yoo BB, Runia TF, Debelius JW, Singh S, Nelson CA, et al. Gut bacteria from multiple sclerosis patients modulate human T cells and exacerbate symptoms in mouse models. Proc Natl Acad Sci U S A. (2017) 114:10713–8. doi: 10.1073/pnas.1711235114
118. Wang S, Chen H, Wen X, Mu J, Sun M, Song X, et al. The efficacy of fecal microbiota transplantation in experimental autoimmune encephalomyelitis: transcriptome and gut microbiota profiling. J Immunol Res. (2021) 2021:4400428. doi: 10.1155/2021/4400428
119. Farshbafnadi M, Agah E, Rezaei N. The second brain: The connection between gut microbiota composition and multiple sclerosis. J Neuroimmunol. (2021) 360:577700. doi: 10.1016/j.jneuroim.2021.577700
120. Cui C, Tan S, Tao L, Gong J, Chang Y, Wang Y, et al. Intestinal barrier breakdown and mucosal microbiota disturbance in neuromyelitis optical spectrum disorders. Front Immunol. (2020) 11:2101. doi: 10.3389/fimmu.2020.02101
121. Zamvil SS, Spencer CM, Baranzini SE, Cree BAC. The gut microbiome in neuromyelitis. Optica Neurotherapeutics. (2018) 15:92–101. doi: 10.1007/s13311-017-0594-z
122. Chen H, Chen Z, Shen L, Wu X, Ma X, Lin D, et al. Fecal microbiota transplantation from patients with autoimmune encephalitis modulates Th17 response and relevant behaviors in mice. Cell Death Discov. (2020) 6:75. doi: 10.1038/s41420-020-00309-8
123. Malik A, Sharma D, Charles JS, Dybas LA, Mansfield LS. Contrasting immune responses mediate Campylobacter jejuni-induced colitis and autoimmunity. Mucosal Immunol. (2014) 7:802–17. doi: 10.1038/mi.2013.97
124. Charles JLS, Bell JA, Gadsden BJ, Malik A, Cooke H, Van de Grift LK, et al. Guillain Barre Syndrome is induced in Non-Obese Diabetic (NOD) mice following Campylobacter jejuni infection and is exacerbated by antibiotics. J Autoimmun. (2017) 77:11–38. doi: 10.1016/j.jaut.2016.09.003
125. Atarashi K, Tanoue T, Shima T, Imaoka A, Kuwahara T, Momose Y, et al. Induction of colonic regulatory T cells by indigenous Clostridium species. Science. (2011) 331:337–41. doi: 10.1126/science.1198469
126. Hemmer B, Kerschensteiner M, Korn T. Role of the innate and adaptive immune responses in the course of multiple sclerosis. Lancet Neurol. (2015) 14:406–19. doi: 10.1016/S1474-4422(14)70305-9
127. Mahad DH, Trapp BD, Lassmann H. Pathological mechanisms in progressive multiple sclerosis. Lancet Neurol. (2015) 14:183–93. doi: 10.1016/S1474-4422(14)70256-X
128. Lassmann H, Bruck W, Lucchinetti CF. The immunopathology of multiple sclerosis: an overview. Brain Pathol. (2007) 17:210–8. doi: 10.1111/j.1750-3639.2007.00064.x
129. Lassmann H. Pathogenic mechanisms associated with different clinical courses of multiple sclerosis. Front Immunol. (2018) 9:3116. doi: 10.3389/fimmu.2018.03116
130. Steinman L. Immunology of relapse and remission in multiple sclerosis. Annu Rev Immunol. (2014) 32:257–81. doi: 10.1146/annurev-immunol-032713-120227
131. Saresella M, Marventano I, Barone M, Rosa FL, Piancone F, Mendozzi L, et al. Alterations in circulating fatty acid are associated with gut microbiota dysbiosis and inflammation in multiple sclerosis. Front Immunol. (2020) 11:1390. doi: 10.3389/fimmu.2020.01390
132. Tzartos JS, Friese MA, Craner MJ, Palace J, Newcombe J, Esiri M, et al. Interleukin-17 production in central nervous system-infiltrating T cells and glial cells is associated with active disease in multiple sclerosis. Am J Pathol. (2008) 172:146–55. doi: 10.2353/ajpath.2008.070690
133. Dendrou CA, Fugger L, Friese MA. Immunopathology of multiple sclerosis. Nat Rev Immunol. (2015) 15:545–58. doi: 10.1038/nri3871
134. Zeng Q, Gong J, Liu X, Chen C, Sun X, Li H, et al. Gut dysbiosis and lack of short chain fatty acids in a Chinese cohort of patients with multiple sclerosis. Neurochem Int. (2019) 129:104468. doi: 10.1016/j.neuint.2019.104468
135. Engen PA, et al. Single-arm, non-randomized, time series, single-subject study of fecal microbiota transplantation in multiple sclerosis. Front Neurol. (2020) 11:978. doi: 10.3389/fneur.2020.00978
136. Wang H-B, Wang P-Y, Wang X, Wan Y-L, Liu Y-C. Butyrate enhances intestinal epithelial barrier function via up-regulation of tight junction protein Claudin-1 transcription. Dig Dis Sci. (2012) 57:3126–35. doi: 10.1007/s10620-012-2259-4
137. Baxter NT, Schmidt AW, Venkataraman A, Kim KS, Waldron C, Schmidt TM. Dynamics of human gut microbiota and short-chain fatty acids in response to dietary interventions with three fermentable fibers. mBio, (2019) 10:1. doi: 10.1128/mBio.02566-18
138. Duscha A, Gisevius B, Hirschberg S, Yissachar N, Stangl GI, Eilers E, et al. Propionic acid shapes the multiple sclerosis disease course by an immunomodulatory mechanism. Cell. (2020) 180:1067–1080.e16. doi: 10.1016/j.cell.2020.02.035
139. Moore SM, Khalaj AJ, Kumar S, Winchester Z, Yoon J, Yoo T, et al. Multiple functional therapeutic effects of the estrogen receptor beta agonist indazole-Cl in a mouse model of multiple sclerosis. Proc Natl Acad Sci U S A. (2014) 111:18061–6. doi: 10.1073/pnas.1411294111
140. Makkawi S, Camara-Lemarroy C, Metz L. Fecal microbiota transplantation associated with 10 years of stability in a patient with SPMS. Neurol Neuroimmunol Neuroinflamm. (2018) 5:e459. doi: 10.1212/NXI.0000000000000459
141. Li K, Wei S, Hu L, Yin X, Mai Y, Jiang C, et al. Protection of fecal microbiota transplantation in a mouse model of multiple sclerosis. Mediators Inflamm. (2020) 2020:2058272. doi: 10.1155/2020/2058272
142. Chang C-W, Lee H-C, Li L-H, Chiau J-SC, Wang T-E, Chuang W-H, et al. Fecal microbiota transplantation prevents intestinal injury, upregulation of toll-like receptors, and 5-fluorouracil/oxaliplatin-induced toxicity in colorectal cancer. Int J Mol Sci, (2020) 21:2. doi: 10.3390/ijms21020386
143. Wingerchuk DM, Lennon VA, Lucchinetti CF, Pittock SJ, Weinshenker B. The spectrum of neuromyelitis optica. Lancet Neurol. (2007) 6:805–15. doi: 10.1016/S1474-4422(07)70216-8
144. Lennon VA, Wingerchuk DM, Kryzer TJ, Pittock SJ, Lucchinetti CF, Fujihara K, et al. A serum autoantibody marker of neuromyelitis optica: distinction from multiple sclerosis. Lancet. (2004) 364:2106–12. doi: 10.1016/S0140-6736(04)17551-X
145. Carnero CE Correale J Neuromyelitis optica spectrum disorders: from pathophysiology to therapeutic strategies. J Neuroinflammation. (2021) 18:208. doi: 10.1186/s12974-021-02249-1
146. Gong J, Qiu W, Zeng Q, Liu X, Sun X, Li H, et al. Lack of short-chain fatty acids and overgrowth of opportunistic pathogens define dysbiosis of neuromyelitis optica spectrum disorders: a Chinese pilot study. Multiple Sclerosis J. (2019) 25:1316–25. doi: 10.1177/1352458518790396
147. Padilla-Docal B, Iglesias-González I, Bu-Coifiu-Fanego R, Socarrás-Hernández CA, Dorta-Contreras AJ. Intrathecal activation as a typical immune response within the central nervous system in angiostrongyliasis. Am J Trop Med Hyg. (2013) 88:230–5. doi: 10.4269/ajtmh.12-0151
148. Singh TD, Fugate JE, Rabinstein AA. The spectrum of acute encephalitis: causes, management, and predictors of outcome. Neurology. (2015) 84:359–66. doi: 10.1212/WNL.0000000000001190
149. Leypoldt F, Armangue T, Dalmau J. Autoimmune encephalopathies. Ann N Y Acad Sci. (2015) 1338:94–114. doi: 10.1111/nyas.12553
150. Tremlett H, Bauer K, Appel S, Finlay B, Waubaut E. The gut microbiome in human neurological disease: A review. Ann Neurol. (2017) 81:369–82. doi: 10.1002/ana.24901
151. Kelly CJ, Zheng L, Campbell EL, Saeedi B, Scholz CC, Bayless AJ, et al. Crosstalk between microbiota-derived short-chain fatty acids and intestinal epithelial hif augments tissue barrier function. Cell Host Microbe. (2015) 17:662–71. doi: 10.1016/j.chom.2015.03.005
152. Ji J, Gu Z, Li H, Su L, Liu Z. Cryptdin-2 predicts intestinal injury during heatstroke in mice. Int J Mol Med. (2018) 41:137–46.
153. Willison HJ, Jacobs BC, van Doorn PA. Guillain-Barre syndrome. Lancet. (2016) 388:717–27. doi: 10.1016/S0140-6736(16)00339-1
154. Mousavi S, Bereswill S, Heimesaat MM. Novel clinical campylobacter jejuni infection models based on sensitization of mice to lipooligosaccharide, a major bacterial factor triggering innate immune responses in human campylobacteriosis. Microorganisms. (2020) 8:4. doi: 10.3390/microorganisms8040482
155. Ang CW, Laman JD, Willison HJ, Wagner ER, Endtz HP, De Klerk MA, et al. Structure of Campylobacter jejuni lipopolysaccharides determines antiganglioside specificity and clinical features of Guillain-Barre and Miller Fisher patients. Infect Immun. (2002) 70:1202–8. doi: 10.1128/IAI.70.3.1202-1208.2002
Keywords: gut microbiota, fecal microbiota transplantation (FMT), neuroimmune diseases, myasthenia gravis (MG), multiple sclerosis (MS), neuromyelitis optica spectrum disorders (NMOSDs), autoimmune encephalitis (AIE), Guillain–Barré syndrome (GBS)
Citation: Wu N, Li X, Ma H, Zhang X, Liu B, Wang Y, Zheng Q and Fan X (2023) The role of the gut microbiota and fecal microbiota transplantation in neuroimmune diseases. Front. Neurol. 14:1108738. doi: 10.3389/fneur.2023.1108738
Received: 26 November 2022; Accepted: 16 January 2023;
Published: 01 February 2023.
Edited by:
Marija Mostarica-Stojkovic, University of Belgrade, SerbiaReviewed by:
Stanisavljević Suzana, University of Belgrade, SerbiaJavier Ochoa-Repáraz, Boise State University, United States
Copyright © 2023 Wu, Li, Ma, Zhang, Liu, Wang, Zheng and Fan. This is an open-access article distributed under the terms of the Creative Commons Attribution License (CC BY). The use, distribution or reproduction in other forums is permitted, provided the original author(s) and the copyright owner(s) are credited and that the original publication in this journal is cited, in accordance with accepted academic practice. No use, distribution or reproduction is permitted which does not comply with these terms.
*Correspondence: Yuan Wang, a2VsZWppYWZlaW1hb0AxMjYuY29t; Qi Zheng,
ZHJfemhlbmdAMTYzLmNvbQ==; Xueli Fan,
eHVlbGlmYW5AeWVhaC5uZXQ=
†These authors have contributed equally to this work