Long non-coding RNAs in intracerebral hemorrhage
- 1Department of Pharmacy, West China Hospital, Sichuan University, Chengdu, China
- 2West China School of Pharmacy, Sichuan University, Chengdu, China
Intracerebral hemorrhage (ICH), a subtype of stroke, can lead to long-term disability and is one of the leading causes of death. Unfortunately, the effectiveness of pharmacological therapy for ICH is still uncertain. Long non-coding RNA (lncRNA) was defined as an RNA molecule that consists of more than 200 nt without translational activity. As a vital class of diverse molecules, lncRNAs are involved in developmental and pathological processes and have been attractive for decades. LncRNAs have also become potential targets for therapies, as they were massively identified and profiled. In particular, emerging evidence has revealed the critical role of lncRNAs in ICH while attempts were made to treat ICH via regulating lncRNAs. But the latest evidence remains to be summarized. Thus, in this review, we will summarize the recent advances in lncRNA in ICH, highlighting the regulatory role of lncRNAs and their potential as therapeutic targets.
1. Introduction
Intracerebral hemorrhage (ICH), a subtype of stroke, refers to the spontaneous rupture of injured small arteries or arterioles, leading to blood accumulation in cerebral parenchymal (Steiner et al., 2014; Gross et al., 2019). Although ICH counts for a smaller portion of all types of strokes (9–27%; Feigin et al., 2009; Sacco et al., 2009; Steiner et al., 2014), the global burden of ICH is higher than that of ischemic stroke (Krishnamurthi et al., 2013). A meta-analysis of nine studies showed that the mortality rate could be as high as 35.3% 3 months after ICH onset (Pinho et al., 2019).
Acute interventions for ICH, including medical therapies and minimally invasive surgery are likely to improve acute outcomes. Besides, sustained blood pressure control and optimized antithrombotic therapy are regarded as essential preventive strategies for improving longer-term outcomes in ICH (Hostettler et al., 2019). A better understanding of how the pathological mechanisms drive neurological injury in individuals is urgently required in order to develop therapies for acute and secondary progressive stages of ICH. Research on long non-coding RNA (lncRNA) has been attractive for decades. Up to now, a variety of lncRNAs have been proven to be involved in cerebral development and diseases, such as degeneration diseases of the central nerve system (Scheele et al., 2007; Chiba et al., 2009; Lagier-Tourenne et al., 2012; Ciarlo et al., 2013) and stroke (both ischemic and hemorrhagic stroke; Zhang and Wang, 2019). With the gain- and loss-of function methods, the functions of lncRNAs in ICH were further studied.
In this regard, it is crucial to reveal the current knowledge about the roles of lncRNAs in ICH. Therefore, we will briefly review the involvement of lncRNAs in the ICH-caused damages and their potential to be therapeutic targets and biomarkers will be discussed. The searching strategies used in this article are summarized in Supplementary material.
2. The pathogenesis of acute and secondary insults after ICH
Quickly after vessel rupture, blood accumulates in the cerebral parenchymal, causing intracranial pressure elevation, perilesional edema, and structural damage, which may lead to brain hernia and can be fatal (Wilkinson et al., 2018). Mostly, bleeding stops shortly after ICH, but 14–22% of ICH patients may experience hematoma expansion in 6–24 h after ICH onset, which causes more severe structural damage and neurological deterioration, and leads to worse outcomes (Kazui et al., 1996, 1997; Fujii et al., 1998).
After the primary mechanical injury, complicated pathological responses will be triggered. The over-activated microglia release several cytokines and contributes to inflammation, blood brain barrier (BBB) breakdown, and edema in turn (Taylor and Sansing, 2013). Components of the complement system can pass through the damaged BBB and form membrane attack complex, enhancing the BBB injury and neurological damage (Xi et al., 2006). Heme and iron will be released during the erythrocyte elimination process (Wagner et al., 2003). The debris of blood enhances the production of free radicals and contributes to neurological injury, inflammation, BBB damage, and edema (Garton et al., 2016; Yang et al., 2016). Also, excitotoxicity molecules, such as glutamate was shown to anticipate in brain injury after intracerebral hemorrhage (Sharp et al., 2008). Although lots of efforts were made, the full picture of the ICH damage mechanisms, especially of the secondary injury, remains to be further explored.
After the acute stage, microglia polarize toward M2-like microglial, contributing to hematoma clearance (Lan et al., 2017). The hematoma breaks down with the invasion of macrophages and microvessel formation. The hemosiderin-stained scar, a cavity containing blood surrounded by fibrous tissue, and eventually gliosis will ultimately form (Fewel et al., 2003). And neuronal plasticity allows the brain to cope better with the indirect effects of brain damage resulting from ICH (Keep et al., 2012).
3. Insights into biological roles of lncRNAs in ICH
3.1. Introduction to lncRNA
Long non-coding RNA is commonly defined as an RNA molecule that consists of more than 200 nt without translational activity (Ponting et al., 2009; Nagano and Fraser, 2011). There are at least 170,000 lncRNAs found in humans and more than 130,000 lncRNAs identified in mice (Zhao et al., 2021). In accordance with the relationships between lncRNAs and their regulated genes, lncRNAs were classified as sense lncRNA, antisense lncRNA, intronic lncRNA, intergenic lncRNA, enhancer lncRNA, and circular lncRNA (Uchida and Dimmeler, 2015).
Long non-coding RNAs were long regarded as transcriptional garbage or transcriptional noise. Studies on lncRNA H19 and lncRNA Xist have initially revealed the biological functions of lncRNAs (Brannan et al., 1990; Wilusz et al., 2009; Gendrel and Heard, 2014). Briefly, lncRNAs can interact with proteins, DNA and RNA transcripts to control alternative splicing, chromosome remodeling, nuclear import and mRNA decay, and lncRNAs participate in almost every aspect of gene expression programs (Schmitz et al., 2010; Grote et al., 2013; Khorkova et al., 2015; Isoda et al., 2017).
In recent years, lncRNAs have been characterized are implicated in diverse diseases, including cardiovascular diseases (Uchida and Dimmeler, 2015), neurodegeneration diseases, ischemic stroke, and traumatic brain injury (Riva et al., 2016; Zhang and Wang, 2019; Ren et al., 2020).
3.2. LncRNAs play versatile roles in pathological processes underlying ICH
Several studies using high-throughput RNA-seq technique were conducted on mice or rat ICH model, and human samples. In a collagenase-induced mice ICH model, 31 lncRNAs were found to differentially express 24 h after modeling (Hanjin et al., 2018). And another study carried out on a similar model showed 625 dysregulated lncRNAs 21 days after ICH onset (Cao et al., 2020). A similar dynamic change of lncRNAs was observed in rats. Kim et al. (2019) found there were 83, 289, and 401 lncRNAs significantly upregulated and 52, 459, and 786 lncRNAs significantly downregulated 1, 3, and 7 days after collagenase-induced ICH modeling, respectively. These studies revealed the extensive involvement and the dynamic changes of lncRNAs in ICH.
RNA-sequencing data from GSE24265 (containing four human patients’ RNA-seq data) were re-analyzed by Liu et al. (2021) and Yang et al. (2022). Liu et al. (2021) predicted that nine lncRNAs were associated with MAPK1 and may contribute to the progression of ferroptosis after ICH. Yang et al. (2022) found six hub lncRNAs and constructed the potential ceRNA network. In the peripheral blood of ICH patients, 211 lncRNAs dysregulated and were classified into16 lncRNA modules by weighted gene co-expression network analysis and some immune-related lncRNAs were also identified by using ceRNA network (Hao et al., 2022).
These studies supported the advantages of high-throughput techniques in the discovery, functional prediction, and key regulator identification of lncRNAs. However, the exact functions of dysregulated lncRNAs detected by RNA-seq remain to be explored and validated. And the different characteristics of lncRNA dysregulation between species, modeling methods and the less conserved characters of lncRNAs (Sharma and Carninci, 2020) require further study and more careful interpretation from preclinical study results.
Long non-coding RNA H19 (also known as H19 imprinted maternally expressed transcript) was one of the earliest identified lncRNAs (Brannan et al., 1990). H19 was observed to be one of the most stable lncRNAs in the gray matter of human brain and was extensively studied in the development and diseases of the central nerve system, including ischemic stroke, glioma, pituitary adenoma, neuroblastoma, degeneration, and trauma (Zhong et al., 2021). Recently, the role of H19 in ICH was extensively studied. In Kim’s study, lncRNA H19 was the most upregulated lncRNA from day 1 through day 7 after ICH both in the ICH model induced by collagenase or autologous blood (Kim et al., 2019). Further bioinformatic analysis predicted that H19 was associated with type I interferon signaling pathway. Following this study, Chen and colleagues further studied the roles of H19 in ICH. After confirming the high expression level of H19 in the ICH cell model, Chen B. et al. (2021) demonstrated that H19 targeted miR-106b-5p and thus regulated ACSL4, enhancing ferroptosis in brain microvascular endothelial cells (BMVECs) under oxygen and glucose deprivation hemin-treated (OGD/H-treated) condition, as validated by RNA pull-down and luciferase reporter gene assays. In ICH rat model induced by type IV collagenase, NF-κBp65 and IKKβ expression were significantly lower and IκBα was significantly higher in the sh-H19 group when compared with ICH model group, indicating that H19 may be associated with NF-κB pathway. Mao et al. (2022) also found that elevated level of H19 expression was associated with the levels of TNF-α, IL-6, IL-1β, ROS, and MDA, showing that H19 was also associated with inflammation and oxidative stress. Also, H19 was found to be associated with the risk of symptomatic ICH in ischemic stroke patients after recombinant tissue plasminogen activator treatment (Han et al., 2022). Collectively, H19 could interact with miRNA and was demonstrated to be associated with NF-κB pathway, inflammation, free radical production and cell death after ICH.
The lncRNA FOXF1 adjacent non-coding developmental regulatory RNA (FENDRR) increased in C57BL/6 mice with hypertensive ICH (Dong et al., 2018) and was demonstrated to target miR-126 by RNA immunoprecipitation and RNA pull-down. Via targeting miR-126, FENDRR regulated VEGFA and thus contributed to the apoptosis of human brain microvascular endothelial cells. Importantly, it was demonstrated that VEGFA was important in the activation of phosphatidylinositol 3-kinase (PI3K)/protein kinase B (AKT; Ruan and Kazlauskas, 2012).
Xie et al. found that MEG3 elevated in collagenase-induced ICH rat brain tissues. The interaction of MEG3 and miR-181b was validated by Starbase analysis and dual-luciferase reporter assay. And they proved the interaction of MEG3 and miR-181b was associated with the activity of PI3K/AKT pathway. In this study, upregulation of MEG3 was associated with the release of inflammatory cytokines and oxidative stress, contributing to brain edema, neuronal apoptosis, and increased caspase3 activity, which can be reversed by miR-181b inhibition (Xie B. et al., 2021). More importantly, the relationship of MEG3 and miR-181b was confirmed in patients with severe ICH. The upregulation of MEG3 and the downregulation of miR-181b were also observed in serum from ICH patients (Wang H. et al., 2022).
Similarly, lncRNA FGD5-AS1 was also found to regulate PI3K/AKT pathway in collagenase-induced C57BL/6 mice ICH model, although via targeting miR6838-5p/VEGFA axis, which was validated by luciferase reporter gene and pull-down assays. The interaction between FGD5-AS1 and miR6836-5p led to inhibited cell proliferation, increased pro-inflammatory factors, and injured BBB. The proinflammatory effects of FGD5-AS1 were also verified in BMVECs. This study also detected the upregulation of FGD5-AS1 in the serum of ICH patients (Jiang et al., 2022).
GAS5, an extensively studied lncRNA in ischemic stroke, also played important roles in ICH. Wang and colleagues found that FoxO1 could enhance the expression of GAS5 by binding to its promoter. GAS5 bond miR-378a-5p and upregulated the expression of Hspa5, which caused a significant elevation of pro-inflammatory factors, brain edema and neurological injury (Wang B. et al., 2022). And FoxO1 was demonstrated to be a major PI3K-AKT downstream effector (Xing et al., 2018).
Chen and colleagues established the ICH model in mice by collagenase injection and in the cellular inflammation model by treating microglia with lipopolysaccharide. After sequencing RNAs obtained from mice, they found the expression of NONMMUT023599.2 was significantly upregulated. Thus, NONMMUT023599.2 knocking down was conducted in both ICH mice and cellular inflammation model, which strongly downregulated the expression of TRIF, p65 phosphorylation and the secretion of TNF-α and IL-1β, indicating the regulatory potential of NONMMUT023599.2 in NF-κB pathway. The elevation of miR-709, which was previously predicted to be the target of NONMMUT023599.2, was also observed. Authors concluded that elevated levels of NONMMUT023599.2 targeted miR-709, regulated NF-κB pathway, enhanced inflammatory cytokine secretion (such as TNF-α and IL-1β) and thus worsened the outcome after ICH in mice (Chen et al., 2020).
Nuclear factor-k-gene binding interacting lncRNA (NKILA) was first identified in breast cancer. NKILA binds to NF-κB/IκB, masks the phosphorylation motifs of IκB, and thereby inhibits IKK-induced IκB phosphorylation and NF-κB activation. The interaction of NKILA and NF-κB pathway signaling molecules prevented the over-activation of NF-κB pathway (Liu et al., 2015). Zhang et al. also proved that NKILA got involved in the pathological changes after ICH on a collagenase-induced rat model (Jia et al., 2018). NKILA was downregulated in the collagenase-induced rat ICH model. Interestingly, further inhibition of NKILA by siRNA activated NF-κB pathway, reduced endoplasmic reticulum stress, neuron autophagy and neurological deficits, but exacerbated brain edema, neuronal cell apoptosis, BBB breakdown, and promoted inflammatory cytokines release.
The c-Jun N-terminal kinase (JNK) signaling pathway also regulates both physiological and pathological processes, such as neurodegenerative diseases, and inflammatory diseases. Dual specificity phosphatases (DUSPs) were found to regulate the JNK pathway by dephosphorylating their substrates, e.g., DUSP6 binds to JNK2/3 and thus inhibits JNK phosphorylation (Ha et al., 2019). LncRNA TCONS_00145741 could disrupt the interaction between DUSP6 and JNK and stabilize JNK phosphorylation, which suppressed M2 differentiation of microglia after ICH (Wu et al., 2021).
Other studies also pointed out the important roles of some lncRNAs in ICH, although, lack specific targets. Zhang et al. found that SNHG3 expression was significantly induced both in OGD/H-treated BMVECs and in the collagenase-induced rat ICH model. The overexpression of SNHG3 upregulated TWEAK and its receptor Fn14, activating the downstream neuroinflammatory pathway STAT3 and enhancing the expression of matrix metallopeptidase 2/9, causing dysfunction of cerebral microvascular cells after ICH (Zhang et al., 2019). In the autologous blood injection ICH mice model, BLNC1 was upregulated in perihematomal edema, hematoma and microvessel. The elevation of BLNC1 enhanced the apoptosis of BMVECs, potentially by activating PPAR-γ/SIRT6/FoxO3 pathway (Xie L. et al., 2021).
The current knowledge about lncRNAs in ICH-induced secondary injury was summarized in Figure 1. The studies included in this section and the locations of the corresponding lncRNAs are summarized in Table 1. Although many of the lncRNAs were studied in mice or rats, most of them were named in their original articles with capital letters, so we named them with capital letters.
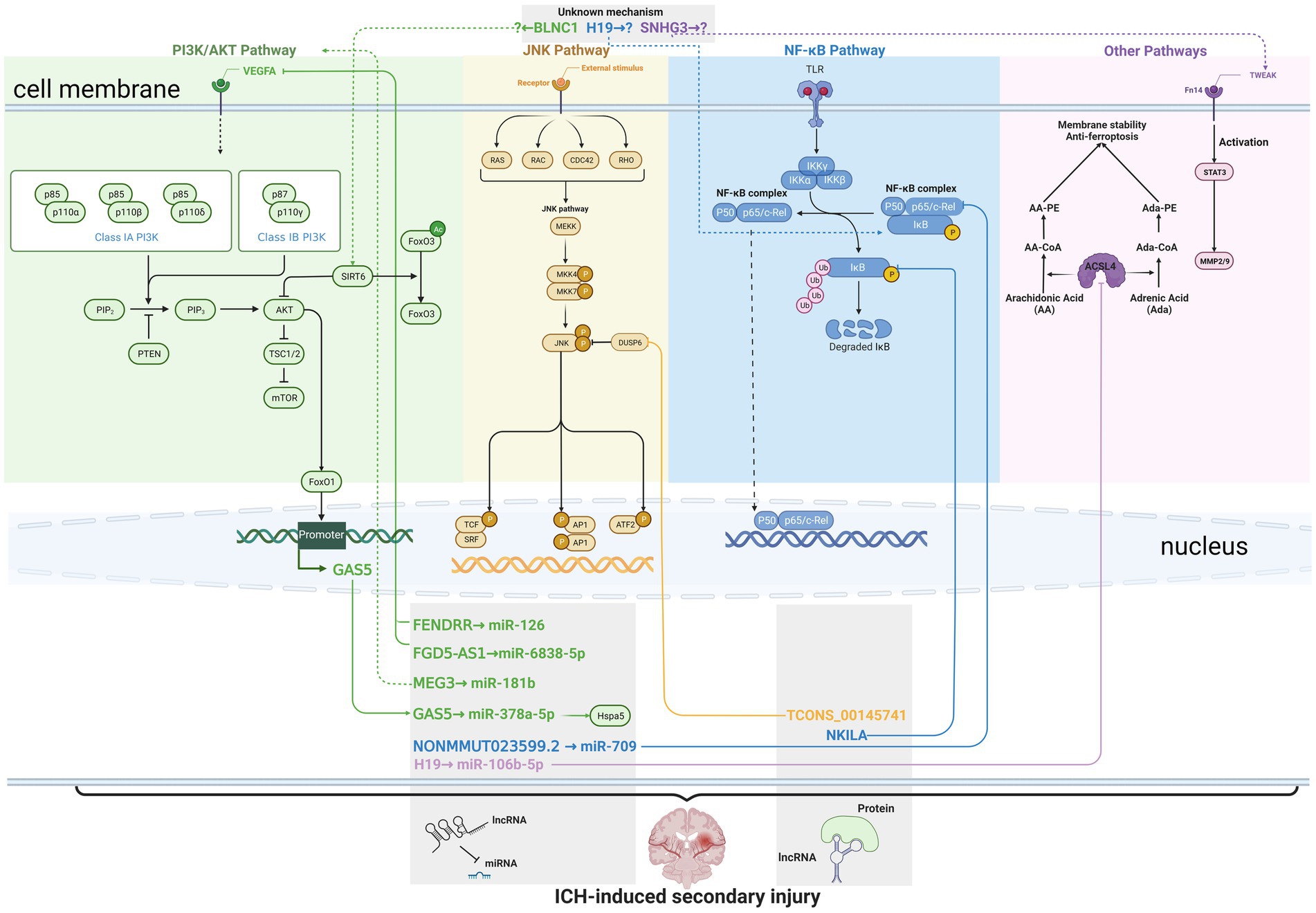
Figure 1. Current knowledge about long non-coding RNAs (lncRNAs) in intracerebral hemorrhage (ICH)-induced secondary injury. Solid lines indicate direct interaction, while dashed lines indicate unknown or indirect regulation. The lncRNAs are marked in green, orange, blue, and purple to indicate their regulation of (or modulation by) the PI3L/AKT pathway, JNK pathway, NF-κB pathway, and other pathways, respectively. The lncRNAs in the same gray box share the similar action mechanisms, such as interacting with miRNAs or proteins. FENDRR, FGD5-AS1, GAS5, NONMMUT023599.2, and H19 were reported to interact with miRNAs, while TCONS_00145741 and NKILA were reported to interact with proteins that contributed to pathological changes after the onset of ICH.
3.3. LncRNAs are implicated as diagnose markers and therapeutic targets for ICH
The pathological process of ICH is complicated, thus if the treatment targets versatile regulators, the outcomes of ICH might be improved. Therefore, lncRNAs were capable to be the targets. This assumption was proven right, as knockdown of H19 in ICH rats significantly reduced inflammatory response, oxidative injury, and improved neurological function (Mao et al., 2022). Several drugs were proved to alleviate ICH-induced injury via regulating lncRNAs. After treating ICH rats with Buyang Huanwu decoction, the traditional Chinese formula, Cui et al. found 18 lncRNAs related to ICH were regulated (Cui et al., 2018). Paeonol, a natural product derived from Paeonia Suffruticosa Andr., was found to inhibit ferroptosis by regulating lncRNA HOTAIR/UPFI/ACSL4 axis and thus inhibit the progression of ICH (Jin et al., 2021). These two studies showed that lncRNAs involved in ICH could be regulated by drugs, and the regulation of lncRNAs ameliorated ICH-induced damage. More importantly, the results of Cui’s study indicated that numerous lncRNAs can be regulated simultaneously and contributed to better outcomes.
Although we now know that lncRNAs may control the secondary injury in ICH, the specific targets were not extensively studied. lncRNAs can interact with several types of macromolecules, but only limited miRNAs and signaling molecules as targets were further analyzed and validated, while some studies even lacked the validation of targets and numerous lncRNAs were not studied. Lacking specific targets impede drug R&D, even when the transcriptomics data provide hints (Dugger et al., 2018). Identifying the targets and validating the exact functions of lncRNAs in ICH remain attractive and important for drug R&D. Also, efforts were made to use CRISPR/Cas9, siRNAs, etc. to treat diseases by targeting lncRNAs. Viral, liposomes and exosomes might be used to deliver lncRNAs to peri-hematoma tissue (Chen Y. et al., 2021). However, stable targeting delivery systems are still lacking. Although most pharmaceutical research is still far from clinical translation, these studies are creative, attractive and have the potential to be beneficial for ICH treatment.
As mentioned above, after ICH onset, lncRNAs dysregulated not only in brain tissue, but also in the circulation system, such as MEG3 and FGD5-AS1 (Hao et al., 2022; Jiang et al., 2022; Wang H. et al., 2022). Also, the association between H19 and the risk of symptomatic ICH in ischemic stroke patients treated with recombinant tissue plasminogen activator was established (Han et al., 2022). The presence of dysregulated lncRNAs in serum, which were also associated with ICH, provided an easier sampling routine and opportunities to use lncRNAs as biomarkers in diagnosing ICH.
On the other hand, the opposite treatments between ischemic stroke and ICH and the nature of rapid pathological changes of both ischemic stroke and ICH require accurate and quick differential diagnosis between these two diseases. With technique development, RNA identification and quantification can be much faster (Carter et al., 2021), which aids the diagnosis and saves time when deciding on proper treatments, especially in rural hospitals (Kamtchum-Tatuene and Jickling, 2019).
Although previous studies demonstrated that mRNAs may have the potential to be biomarkers for the diagnosis and the differentially diagnose of ICH (Stamova et al., 2019), we still lack knowledge about the sensitivity, specificity, and the value of lncRNA for ICH therapy. Opportunities lie ahead, but studies are still needed.
4. Conclusion
Previous studies profiled the multi-functional roles of lncRNAs in ICH. lncRNAs are involved in ICH-induced secondary injury, such as inflammatory response, oxidative stress and cell death, etc., via targeting miRNAs or signaling molecules. We are at the infant stage and this field remains attractive. More studies could be conducted to validate the targets of lncRNAs, evaluate the value of lncRNAs as risk prediction for ICH, and develop drugs or special delivery systems to treat ICH by targeting lncRNAs.
Author contributions
CZ and YZ wrote the manuscript. QW and ZF drew the figure. MZ and XX conducted literature search. TX reviewed and edited the manuscript. All authors contributed to the article and approved the submitted version.
Funding
This work was supported by the National Key R&D Program of China (2020YFC2008302), the National Natural Science Foundation of China (81673631) and Excellence Development 1·3·5 project of West China Hospital of Sichuan University (ZYJC18028).
Acknowledgments
This research was supported by National Key Clinical Specialties Construction Program.
Conflict of interest
The authors declare that the research was conducted in the absence of any commercial or financial relationships that could be construed as a potential conflict of interest.
Publisher’s note
All claims expressed in this article are solely those of the authors and do not necessarily represent those of their affiliated organizations, or those of the publisher, the editors and the reviewers. Any product that may be evaluated in this article, or claim that may be made by its manufacturer, is not guaranteed or endorsed by the publisher.
Supplementary material
The Supplementary material for this article can be found online at: https://www.frontiersin.org/articles/10.3389/fnmol.2023.1119275/full#supplementary-material
References
Brannan, C. I., Dees, E. C., Ingram, R. S., and Tilghman, S. M. (1990). The product of the H19 gene may function as an RNA. Mol. Cell. Biol. 10, 28–36. doi: 10.1128/mcb.10.1.28
Cao, F., Guo, Y., Zhang, Q., Fan, Y., Liu, Q., Song, J., et al. (2020). Integration of transcriptome resequencing and quantitative proteomics analyses of collagenase VII-induced intracerebral hemorrhage in mice. Front. Genet. 11:551065. doi: 10.3389/fgene.2020.551065
Carter, J. G., Orueta Iturbe, L., Duprey, J. H. A., Carter, I. R., Southern, C. D., Rana, M., et al. (2021). Ultrarapid detection of SARS-CoV-2 RNA using a reverse transcription-free exponential amplification reaction, RTF-EXPAR. Proc. Natl. Acad. Sci. U. S. A. 118. doi: 10.1073/pnas.2100347118
Chen, Y., Li, Z., Chen, X., and Zhang, S. (2021). Long non-coding RNAs: from disease code to drug role. Acta Pharm. Sin. B 11, 340–354. doi: 10.1016/j.apsb.2020.10.001
Chen, B., Wang, H., Lv, C., Mao, C., and Cui, Y. (2021). Long non-coding RNA H19 protects against intracerebral hemorrhage injuries via regulating microRNA-106b-5p/acyl-CoA synthetase long chain family member 4 axis. Bioengineered 12, 4004–4015. doi: 10.1080/21655979.2021.1951070
Chen, J. X., Wang, Y. P., Zhang, X., Li, G. X., Zheng, K., and Duan, C. Z. (2020). lncRNA Mtss1 promotes inflammatory responses and secondary brain injury after intracerebral hemorrhage by targeting miR-709 in mice. Brain Res. Bull. 162, 20–29. doi: 10.1016/j.brainresbull.2020.04.017
Chiba, M., Kiyosawa, H., Hiraiwa, N., Ohkohchi, N., and Yasue, H. (2009). Existence of Pink1 antisense RNAs in mouse and their localization. Cytogenet. Genome Res. 126, 259–270. doi: 10.1159/000251963
Ciarlo, E., Massone, S., Penna, I., Nizzari, M., Gigoni, A., Dieci, G., et al. (2013). An intronic ncRNA-dependent regulation of SORL1 expression affecting Abeta formation is upregulated in post-mortem Alzheimer's disease brain samples. Dis. Model. Mech. 6, 424–433. doi: 10.1242/dmm.009761
Cui, H., Liu, T., Li, P., Yang, A., Zhou, H., Luo, J., et al. (2018). An intersectional study of LncRNAs and mRNAs reveals the potential therapeutic targets of Buyang Huanwu decoction in experimental intracerebral hemorrhage. Cell. Physiol. Biochem. 46, 2173–2186. doi: 10.1159/000489547
Dong, B., Zhou, B., Sun, Z., Huang, S., Han, L., Nie, H., et al. (2018). LncRNA-FENDRR mediates VEGFA to promote the apoptosis of brain microvascular endothelial cells via regulating miR-126 in mice with hypertensive intracerebral hemorrhage. Microcirculation 25:e12499. doi: 10.1111/micc.12499
Dugger, S. A., Platt, A., and Goldstein, D. B. (2018). Drug development in the era of precision medicine. Nat. Rev. Drug Discov. 17, 183–196. doi: 10.1038/nrd.2017.226
Feigin, V. L., Lawes, C. M., Bennett, D. A., Barker-Collo, S. L., and Parag, V. (2009). Worldwide stroke incidence and early case fatality reported in 56 population-based studies: a systematic review. Lancet Neurol. 8, 355–369. doi: 10.1016/S1474-4422(09)70025-0
Fewel, M. E., Thompson, B. G. Jr., and Hoff, J. T. (2003). Spontaneous intracerebral hemorrhage: a review. Neurosurg. Focus. 15, E1–E16. doi: 10.3171/foc.2003.15.4.0
Fujii, Y., Takeuchi, S., Sasaki, O., Minakawa, T., and Tanaka, R. (1998). Multivariate analysis of predictors of hematoma enlargement in spontaneous intracerebral hemorrhage. Stroke 29, 1160–1166. doi: 10.1161/01.str.29.6.1160
Garton, T., Keep, R. F., Hua, Y., and Xi, G. (2016). Brain iron overload following intracranial haemorrhage. Stroke Vasc. Neurol. 1, 172–184. doi: 10.1136/svn-2016-000042
Gendrel, A. V., and Heard, E. (2014). Noncoding RNAs and epigenetic mechanisms during X-chromosome inactivation. Annu. Rev. Cell Dev. Biol. 30, 561–580. doi: 10.1146/annurev-cellbio-101512-122415
Gross, B. A., Jankowitz, B. T., and Friedlander, R. M. (2019). Cerebral Intraparenchymal hemorrhage: a review. JAMA 321, 1295–1303. doi: 10.1001/jama.2019.2413
Grote, P., Wittler, L., Hendrix, D., Koch, F., Währisch, S., Beisaw, A., et al. (2013). The tissue-specific lncRNA Fendrr is an essential regulator of heart and body wall development in the mouse. Dev. Cell 24, 206–214. doi: 10.1016/j.devcel.2012.12.012
Ha, J., Kang, E., Seo, J., and Cho, S. (2019). Phosphorylation dynamics of JNK signaling: effects of dual-specificity phosphatases (DUSPs) on the JNK pathway. Int. J. Mol. Sci. 20, 6157–6175. doi: 10.3390/ijms20246157
Han, Z., Li, L., Tao, Z., Wang, R., Zhao, H., Zheng, Y., et al. (2022). Neutrophilic noncoding RNAs predict outcomes of acute ischemic stroke patients treated with recombinant tissue plasminogen activator. Front. Pharmacol. 13:1003806. doi: 10.3389/fphar.2022.1003806
Hanjin, C., Tao, L., Pengfei, L., Ali, Y., Huajun, Z., Jiekun, L., et al. (2018). Altered long noncoding RNA and messenger RNA expression in experimental intracerebral hemorrhage—a preliminary study. Cell. Physiol. Biochem. 45, 1284–1301. doi: 10.1159/000487464
Hao, Y., Xu, X., Wang, Y., Jin, F., Tang, L., Zheng, W., et al. (2022). Comprehensive analysis of immune-related biomarkers and pathways in intracerebral hemorrhage using weighted gene co-expression network analysis and competing endogenous ribonucleic acid. Front. Mol. Neurosci. 15:955818. doi: 10.3389/fnmol.2022.955818
Hostettler, I. C., Seiffge, D. J., and Werring, D. J. (2019). Intracerebral hemorrhage: an update on diagnosis and treatment. Expert. Rev. Neurother. 19, 679–694. doi: 10.1080/14737175.2019.1623671
Isoda, T., Moore, A. J., He, Z., Chandra, V., Aida, M., Denholtz, M., et al. (2017). Non-coding transcription instructs chromatin folding and compartmentalization to dictate enhancer-promoter communication and T cell fate. Cells 171, 103–119.e18. doi: 10.1016/j.cell.2017.09.001
Jia, J., Zhang, M., Li, Q., Zhou, Q., and Jiang, Y. (2018). Long noncoding ribonucleic acid NKILA induces the endoplasmic reticulum stress/autophagy pathway and inhibits the nuclear factor-k-gene binding pathway in rats after intracerebral hemorrhage. J. Cell. Physiol. 233, 8839–8849. doi: 10.1002/jcp.26798
Jiang, F., Liu, X., Wang, X., Hu, J., Chang, S., and Cui, X. (2022). LncRNA FGD5-AS1 accelerates intracerebral hemorrhage injury in mice by adsorbing miR-6838-5p to target VEGFA. Brain Res. 1776:147751. doi: 10.1016/j.brainres.2021.147751
Jin, Z. L., Gao, W. Y., Liao, S. J., Yu, T., Shi, Q., Yu, S. Z., et al. (2021). Paeonol inhibits the progression of intracerebral haemorrhage by mediating the HOTAIR/UPF1/ACSL4 axis. ASN Neuro 13:17590914211010647. doi: 10.1177/17590914211010647
Kamtchum-Tatuene, J., and Jickling, G. C. (2019). Blood biomarkers for stroke diagnosis and management. NeuroMolecular Med. 21, 344–368. doi: 10.1007/s12017-019-08530-0
Kazui, S., Minematsu, K., Yamamoto, H., Sawada, T., and Yamaguchi, T. (1997). Predisposing factors to enlargement of spontaneous intracerebral hematoma. Stroke 28, 2370–2375. doi: 10.1161/01.str.28.12.2370
Kazui, S., Naritomi, H., Yamamoto, H., Sawada, T., and Yamaguchi, T. (1996). Enlargement of spontaneous intracerebral hemorrhage. Incidence and time course. Stroke 27, 1783–1787. doi: 10.1161/01.str.27.10.1783
Keep, R. F., Hua, Y., and Xi, G. (2012). Intracerebral haemorrhage: mechanisms of injury and therapeutic targets. Lancet Neurol. 11, 720–731. doi: 10.1016/s1474-4422(12)70104-7
Khorkova, O., Hsiao, J., and Wahlestedt, C. (2015). Basic biology and therapeutic implications of lncRNA. Adv. Drug Deliv. Rev. 87, 15–24. doi: 10.1016/j.addr.2015.05.012
Kim, J. M., Moon, J., Yu, J. S., Park, D. K., Lee, S. T., Jung, K. H., et al. (2019). Altered long noncoding RNA profile after intracerebral hemorrhage. Ann. Clin. Transl. Neurol. 6, 2014–2025. doi: 10.1002/acn3.50894
Krishnamurthi, R. V., Feigin, V. L., Forouzanfar, M. H., Mensah, G. A., Connor, M., Bennett, D. A., et al. (2013). Global and regional burden of first-ever ischaemic and haemorrhagic stroke during 1990-2010: findings from the global burden of disease study 2010. Lancet Glob. Health 1, e259–e281. doi: 10.1016/S2214-109X(13)70089-5
Lagier-Tourenne, C., Polymenidou, M., Hutt, K. R., Vu, A. Q., Baughn, M., Huelga, S. C., et al. (2012). Divergent roles of ALS-linked proteins FUS/TLS and TDP-43 intersect in processing long pre-mRNAs. Nat. Neurosci. 15, 1488–1497. doi: 10.1038/nn.3230
Lan, X., Han, X., Li, Q., Yang, Q. W., and Wang, J. (2017). Modulators of microglial activation and polarization after intracerebral haemorrhage. Nat. Rev. Neurol. 13, 420–433. doi: 10.1038/nrneurol.2017.69
Liu, T., Li, X., Cui, Y., Meng, P., Zeng, G., Wang, Y., et al. (2021). Bioinformatics analysis identifies potential Ferroptosis key genes in the pathogenesis of intracerebral hemorrhage. Front. Neurosci. 15:661663. doi: 10.3389/fnins.2021.661663
Liu, B., Sun, L., Liu, Q., Gong, C., Yao, Y., Lv, X., et al. (2015). A cytoplasmic NF-κB interacting long noncoding RNA blocks IκB phosphorylation and suppresses breast cancer metastasis. Cancer Cell 27, 370–381. doi: 10.1016/j.ccell.2015.02.004
Mao, S., Huang, H., and Chen, X. (2022). lncRNA H19 aggravates brain injury in rats following experimental intracerebral hemorrhage via NF-kappaB pathway. Comput. Math. Methods Med. 2022, 1–7. doi: 10.1155/2022/3017312
Nagano, T., and Fraser, P. (2011). No-nonsense functions for long noncoding RNAs. Cells 145, 178–181. doi: 10.1016/j.cell.2011.03.014
Pinho, J., Costa, A. S., Araujo, J. M., Amorim, J. M., and Ferreira, C. (2019). Intracerebral hemorrhage outcome: a comprehensive update. J. Neurol. Sci. 398, 54–66. doi: 10.1016/j.jns.2019.01.013
Ponting, C. P., Oliver, P. L., and Reik, W. (2009). Evolution and functions of long noncoding RNAs. Cells 136, 629–641. doi: 10.1016/j.cell.2009.02.006
Ren, D., Chen, W., Cao, K., Wang, Z., and Zheng, P. (2020). Expression profiles of long non-coding RNA and messenger RNA in human traumatic brain injury. Mol. Ther. Nucleic Acids 22, 99–113. doi: 10.1016/j.omtn.2020.08.012
Riva, P., Ratti, A., and Venturin, M. (2016). The long non-coding RNAs in neurodegenerative diseases: novel mechanisms of pathogenesis. Curr. Alzheimer Res. 13, 1219–1231. doi: 10.2174/1567205013666160622112234
Ruan, G. X., and Kazlauskas, A. (2012). Axl is essential for VEGF-A-dependent activation of PI3K/Akt. EMBO J. 31, 1692–1703. doi: 10.1038/emboj.2012.21
Sacco, S., Marini, C., Toni, D., Olivieri, L., and Carolei, A. (2009). Incidence and 10-year survival of intracerebral hemorrhage in a population-based registry. Stroke 40, 394–399. doi: 10.1161/STROKEAHA.108.523209
Scheele, C., Petrovic, N., Faghihi, M. A., Lassmann, T., Fredriksson, K., Rooyackers, O., et al. (2007). The human PINK1 locus is regulated in vivo by a non-coding natural antisense RNA during modulation of mitochondrial function. BMC Genomics 8. doi: 10.1186/1471-2164-8-74
Schmitz, K. M., Mayer, C., Postepska, A., and Grummt, I. (2010). Interaction of noncoding RNA with the rDNA promoter mediates recruitment of DNMT3b and silencing of rRNA genes. Genes Dev. 24, 2264–2269. doi: 10.1101/gad.590910
Sharma, H., and Carninci, P. (2020). The secret life of lncRNAs: conserved, yet not conserved. Cells 181, 512–514. doi: 10.1016/j.cell.2020.04.012
Sharp, F., Liu, D. Z., Zhan, X., and Ander, B. P. (2008). Intracerebral hemorrhage injury mechanisms: glutamate neurotoxicity, thrombin, and Src. Acta Neurochir. Suppl. 105, 43–46. doi: 10.1007/978-3-211-09469-3_9
Stamova, B., Ander, B. P., Jickling, G., Hamade, F., Durocher, M., Zhan, X., et al. (2019). The intracerebral hemorrhage blood transcriptome in humans differs from the ischemic stroke and vascular risk factor control blood transcriptomes. J. Cereb. Blood Flow Metab. 39, 1818–1835. doi: 10.1177/0271678X18769513
Steiner, T., al-Shahi Salman, R., Beer, R., Christensen, H., Cordonnier, C., Csiba, L., et al. (2014). European stroke organisation (ESO) guidelines for the management of spontaneous intracerebral hemorrhage. Int. J. Stroke 9, 840–855. doi: 10.1111/ijs.12309
Taylor, R. A., and Sansing, L. H. (2013). Microglial responses after ischemic stroke and intracerebral hemorrhage. Clin. Dev. Immunol. 2013:746068. doi: 10.1155/2013/746068
Uchida, S., and Dimmeler, S. (2015). Long noncoding RNAs in cardiovascular diseases. Circ. Res. 116, 737–750. doi: 10.1161/CIRCRESAHA.116.302521
Wagner, K. R., Sharp, F. R., Ardizzone, T. D., Lu, A., and Clark, J. F. (2003). Heme and iron metabolism: role in cerebral hemorrhage. J. Cereb. Blood Flow Metab. 23, 629–652. doi: 10.1097/01.WCB.0000073905.87928.6D
Wang, H., Wang, L., and Shi, Q. (2022). Changes in serum LncRNA MEG3/miR-181b and UCH-L1 levels in patients with moderate and severe intracerebral hemorrhage. Turkish neurosurgery. Advance online publication.
Wang, B., Zhao, X., Xiao, L., and Chen, Y. (2022). FoxO1 silencing facilitates neurological function recovery in intracerebral hemorrhage mice via the lncRNA GAS5/miR-378a-5p/Hspa5 Axis. J. Stroke Cerebrovasc. Dis. 31:106443. doi: 10.1016/j.jstrokecerebrovasdis.2022.106443
Wilkinson, D. A., Pandey, A. S., Thompson, B. G., Keep, R. F., Hua, Y., and Xi, G. (2018). Injury mechanisms in acute intracerebral hemorrhage. Neuropharmacology 134, 240–248. doi: 10.1016/j.neuropharm.2017.09.033
Wilusz, J. E., Sunwoo, H., and Spector, D. L. (2009). Long noncoding RNAs: functional surprises from the RNA world. Genes Dev. 23, 1494–1504. doi: 10.1101/gad.1800909
Wu, L., Zhan, Q., Liu, P., Zheng, H., Liu, M., Min, J., et al. (2021). LncRNA TCONS_00145741 knockdown prevents thrombin-induced M1 differentiation of microglia in intracerebral hemorrhage by enhancing the interaction between DUSP6 and JNK. Front. Cell Dev. Biol. 9:684842. doi: 10.3389/fcell.2021.684842
Xi, G., Keep, R. F., and Hoff, J. T. (2006). Mechanisms of brain injury after intracerebral haemorrhage. Lancet Neurol. 5, 53–63. doi: 10.1016/S1474-4422(05)70283-0
Xie, B., Qiao, M., and Xuan, J. (2021). lncRNA MEG3 downregulation relieves intracerebral hemorrhage by inhibiting oxidative stress and inflammation in an miR-181b-dependent manner. Med. Sci. Monit. 27:e929435. doi: 10.12659/MSM.929435
Xie, L., Wang, Y., and Chen, Z. (2021). LncRNA Blnc1 mediates the permeability and inflammatory response of cerebral hemorrhage by regulating the PPAR-gamma/SIRT6/FoxO3 pathway. Life Sci. 267:118942. doi: 10.1016/j.lfs.2020.118942
Xing, Y. Q., Li, A., Yang, Y., Li, X. X., Zhang, L. N., and Guo, H. C. (2018). The regulation of FOXO1 and its role in disease progression. Life Sci. 193, 124–131. doi: 10.1016/j.lfs.2017.11.030
Yang, G., Hu, R., Zhang, C., Qian, C., Luo, Q. Q., Yung, W. H., et al. (2016). A combination of serum iron, ferritin and transferrin predicts outcome in patients with intracerebral hemorrhage. Sci. Rep. 6:21970. doi: 10.1038/srep21970
Yang, C., Wu, J., Lu, X., Xiong, S., and Xu, X. (2022). Identification of novel biomarkers for intracerebral hemorrhage via long noncoding RNA-associated competing endogenous RNA network. Mol Omics 18, 71–82. doi: 10.1039/d1mo00298h
Zhang, J., Dong, B., Hao, J., Yi, S., Cai, W., and Luo, Z. (2019). LncRNA Snhg3 contributes to dysfunction of cerebral microvascular cells in intracerebral hemorrhage rats by activating the TWEAK/Fn14/STAT3 pathway. Life Sci. 237:116929. doi: 10.1016/j.lfs.2019.116929
Zhang, L., and Wang, H. (2019). Long non-coding RNA in CNS injuries: a new target for therapeutic intervention. Mol. Ther. Nucleic Acids 17, 754–766. doi: 10.1016/j.omtn.2019.07.013
Zhao, L., Wang, J., Li, Y., Song, T., Wu, Y., Fang, S., et al. (2021). NONCODEV6: an updated database dedicated to long non-coding RNA annotation in both animals and plants. Nucleic Acids Res. 49, D165–D171. doi: 10.1093/nar/gkaa1046
Keywords: intracerebral hemorrhage, long non-coding RNA, pathology, therapeutic target, hemorrhagic stroke
Citation: Zhang C, Zhang Y, Wang Q, Fang Z, Xu X, Zhao M and Xu T (2023) Long non-coding RNAs in intracerebral hemorrhage. Front. Mol. Neurosci. 16:1119275. doi: 10.3389/fnmol.2023.1119275
Edited by:
Gang-Min Hur, Chungnam National University, Republic of KoreaReviewed by:
Qing Cai, Fourth Military Medical University, ChinaDing Xu, Zhejiang University, China
Copyright © 2023 Zhang, Zhang, Wang, Fang, Xu, Zhao and Xu. This is an open-access article distributed under the terms of the Creative Commons Attribution License (CC BY). The use, distribution or reproduction in other forums is permitted, provided the original author(s) and the copyright owner(s) are credited and that the original publication in this journal is cited, in accordance with accepted academic practice. No use, distribution or reproduction is permitted which does not comply with these terms.
*Correspondence: Ting Xu, tingx2009@163.com
†These authors share first authorship