- Center for Nuclear Engineering and Sciences, Paul Scherrer Institut, Villigen, Switzerland
During severe accidents in nuclear power plants, filtered containment venting system is foreseen to be employed once the containment pressure increases above a pre-set value called venting pressure. Ag-zeolite filters are applied in filtered containment venting systems to retain iodine and organic iodides in the gas phase. In this work, the applicability of Ag-zeolites to not only retain gas phase iodine species, but to also catalyze hydrogen recombination has been experimentally investigated under challenging high humidity conditions. Tests were performed in the medium-scale facility using two Ag-zeolites, one of them designed to both retain gas phase iodine species and recombine hydrogen, the other one designed to only retain gas phase iodine species. Experiments studied the effect of residence time and the carrier gas mixture (steam, N2 or air) on the retention of organic iodine, represented in the tests by CH3I, and hydrogen recombination rate with the two Ag-zeolites. The experiments were carried out under the conditions expected in the containment during severe accidents, however, considering practical limitations. The effects of pressure and the presence of contaminant gases (CO, N2O) were investigated in additional tests not included in this study. The steam fraction in the tests varied between 32% and 90%, air fraction was 0%, 5% or 19%, and hydrogen content either 2.5% or 5%. Nitrogen made up the balance for the gas atmosphere. Gas residence time in the zeolite bed was either 100 m or 200 m. Both zeolites showed high retention of CH3I under all the gas atmospheres as long as the residence time in the reaction chamber was 200 m. CH3I retention was lower when the residence time was reduced to 100 m. Hydrogen recombination was more dependent on the gas atmosphere, as expected. The effect of the gas atmosphere on the hydrogen recombination and retention is discussed.
1 Introduction
During a hypothetical nuclear reactor severe accident, fission products and other radionuclides are released from the fuel to the gas phase. In the gas, they are present either as gas phase compounds or as fine particles, i.e., aerosols, which are easily transported with the gas flow. To avoid release of radionuclides to the atmosphere, many nuclear reactors are equipped with a filtered containment venting system (FCVS). FCVS is foreseen to be employed during a severe accident to avoid over-pressure failure of the containment. FCVS is actuated once the containment pressure increases above a pre-set value called venting pressure (OECD/NEA, 2014).
At the time of FCVS actuation, the reactor has typically already suffered a large core damage. Hydrogen produced by oxidation of zirconium contained in the fuel cladding as well as volatile fission products have been released from the core into the containment. The major fraction of the fission products are in the form of aerosol particles when released to the containment. The aerosol concentration in the containment can be very high (Kissane, 2008; OECD/NEA, 2009).
The main radionuclides in the gas phase in the containment atmosphere are the noble gases. In addition, a fraction of iodine may be in the gas phase in the containment, the main compounds being elemental iodine (I2) and different organic iodides. The gas phase fraction of the iodine as well as the relative distribution between I2 and organic iodides varies widely depending on different parameters such as the material of the control rods, reactor type, temperature, composition of the atmosphere, sump pH, etc. (e.g., Wren et al., 2000; OECD/NEA, 2007; Simondi-Teisseire et al., 2013; Leroy and Bosland, 2023). As all these parameters vary dynamically during a severe accident, and the gas phase iodine concentration is a balance between its generation, decompositions and deposition, it is very difficult to determine the concentration of gas phase iodine in the containment. Thereby, in order to avoid release of iodine to the atmosphere, FCVS needs to be able to retain not only aerosols but also gas phase iodine compounds.
Most of the FCVS systems, both dry and wet, are efficient in capturing aerosol particles. Only a small fraction of the hard-to-collect particles in the diameter range of 0.1–1.0 µm may typically penetrate the FCVS. Gas phase compounds are more difficult to collect and may require dedicated filter materials specific to a particular gas phase compound. Elemental iodine can be rather efficiently trapped in wet scrubbers (e.g., Bal et al., 2018) but organic iodides have a very limited solubility in water and the retention in the wet scrubbers is low. To retain organic iodides, zeolites doped with silver, Ag-zeolites, have been applied as an additional filtering stage to FCVS (OECD/NEA, 2014).
Zeolites are crystalline microporous aluminosilicates (Pérez-Botella et al., 2022). They are versatile materials capable of being tailored for different purposes by modifying their structure, Si/Al ratio and by doping them with different elements. Zeolites are also found in nature. Ag-zeolites are commonly used to retain iodine compounds in several processes, e.g., nuclear fuel reprocessing (Soelberg et al., 2013). During severe accidents in nuclear reactors, these materials have been applied in FCVS to retain gas phase iodine, and especially organic iodides which are difficult to retain by other methods (e.g., Albiol et al., 2018). Typically, silver containing zeolites are implemented as a secondary filtration stage downstream of a wet scrubber or a dry filter to increase retention of organic iodides. Depending on the features of a specific Ag-zeolite, retention of iodine can be physical adsorption or chemical reaction with the Ag included in the zeolite structure (e.g., Chebbi et al., 2017). Physical adsorption is a reversible process whereas a chemical reaction would result in the formation of AgI. AgI can be decomposed to release iodine if exposed to very reducing conditions at a high temperature, however, it is stable under the foreseen conditions of the FCVS. Thereby, a chemical reaction between Ag+ in the zeolite and I- from iodine compounds is the most preferred way of iodine retention in most of the FCVS applications to avoid iodine re-release from the zeolite.
In addition to retaining iodine species, it has been shown that silver can also catalyze the reaction of hydrogen with oxygen (Choi et al., 2015). In this hydrogen recombination reaction, H2 reacts with O2 when they are absorbed on the surface of the catalyst, in this case silver. The catalyst lowers the activation energy needed for the reaction, thereby enabling reaction at lower temperature and/or at lower hydrogen and oxygen concentrations. The hydrogen recombination reaction is used in passive autocatalytic recombiners used to reduce the hydrogen risk in nuclear reactor containments during accidents (e.g., Gupta et al., 2021). The recombiners use mainly Pt or Pd as the catalytic material to promote hydrogen recombination, these materials being more efficient than silver as catalysts.
The use of silver-zeolites to simultaneously both retain iodine species and catalyze hydrogen recombination is a novel application of these materials (Wang et al., 2017). In this application, iodine species are retained by chemical reaction in the silver forming AgI, and additional silver catalyzes hydrogen recombination.
In this investigation, two Ag-zeolites are experimentally characterized. One of the zeolites has been designed to retain organic gas phase iodides and to simultaneously catalyze recombination of hydrogen, the other zeolite has been designed to retain gas phase organic iodides but not to catalyze hydrogen reaction. The capability of the two zeolites to retain organic iodides, represented by CH3I, is determined under challenging high humidity conditions in both oxidizing and reducing atmosphere. As the novelty in this investigation, the capability of the zeolites to catalyze recombination of hydrogen is assessed. As hydrogen and iodine are both present in the containment atmosphere at the same time, and thereby reducing their concentrations simultaneously can be beneficial. After characterization of the physical properties of the two investigated zeolites, they are exposed to gas phase organic iodide CH3I and hydrogen, and the retention of CH3I as well as reduction of hydrogen concentration are measured.
2 Methods
2.1 Test facility
The experimental work was carried out in the medium-scale HERZSAN test facility (HTF) at Paul Scherrer Institute. The test rig is dedicated to Ag-zeolite testing for hydrogen catalytic reactions in a gas stream composed of steam and nitrogen or air in the presence of gas phase elemental iodine (I2) and organic iodide (represented by CH3I). The facility consists of supply lines for steam, nitrogen/air, the safety vessel, exhaust line, pressure control valve, and measurement systems for iodine and organic iodide.
The main component of the test facility is a cylindrical safety vessel (pressure vessel), Figure 1, with an inner diameter of 0.273 m. The safety vessel holds the reactor vessel with an inner diameter of 0.109 m. The samples of two zeolites, AgZeo-1 and AgZeo-2, are placed in the reactor vessel, Figure 2 (Wang et al., 2017). Approximately, 1.4 kg of AgZeo-2 and 1.9 kg of AgZeo-1 were loaded into the reactor vessel for the experiments. The safety vessel is designed for a maximum pressure of 0.60 MPa and planned maximum operating pressures of 0.40–0.45 MPa for zeolite testing.
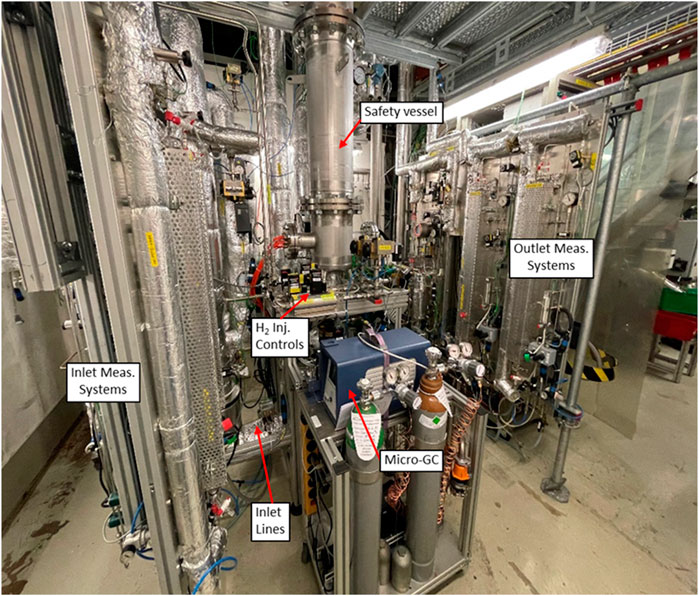
Figure 1. View to the HERZSAN test facility with inlet, safety vessel, and measurement systems for iodine and organic iodide.
For startup and shutdown of the test, the carrier gas flow is directed through a bypass line. The actual operating pressure during the tests inside the safety vessel and the reactor vessel assembly is controlled by dedicated control valve systems at the outlet line. As the reactor vessel assembly forms a liner inside the pressure vessel, there will be no appreciable pressure difference between the inner and the outer parts of the reactor assembly, which greatly simplifies the overall design.
The test section is equipped with several classical thermal-hydraulic instruments such as type K thermocouples for fluid and wall temperature measurements, as well as absolute and gauge pressure transmitters. All piping lines requiring temperature control are trace heated to 423–443 K and thermally insulated. The facility control is accomplished mainly by remote controlled valves, mass flow controllers and as well as by temperature and pressure controls. Pressurized air and nitrogen can be used as the main non-condensable carrier gas mixed with hydrogen. Steam is provided by a dedicated steam generator. All these gases in combination with steam can be mixed, heated, and fed to the test section.
2.2 Test matrix
Altogether more than 50 experiments were carried out in the HERZAN test facility to determine retention of gas phase iodine species I2 and CH3I as well as hydrogen recombination in the zeolite bed. In the current investigation, we report results of 13 tests which were focused on simultaneous retention of CH3I and hydrogen recombination using AgZeo-1 and AgZeo-2. The main test conditions are given in Table 1. The same test conditions were always used with both AgZeo-2 and AgZeo-1. Test 16 is a repetition of test 10, and therefore one more test was conducted with AgZeo-1 than with AgZeo-2.
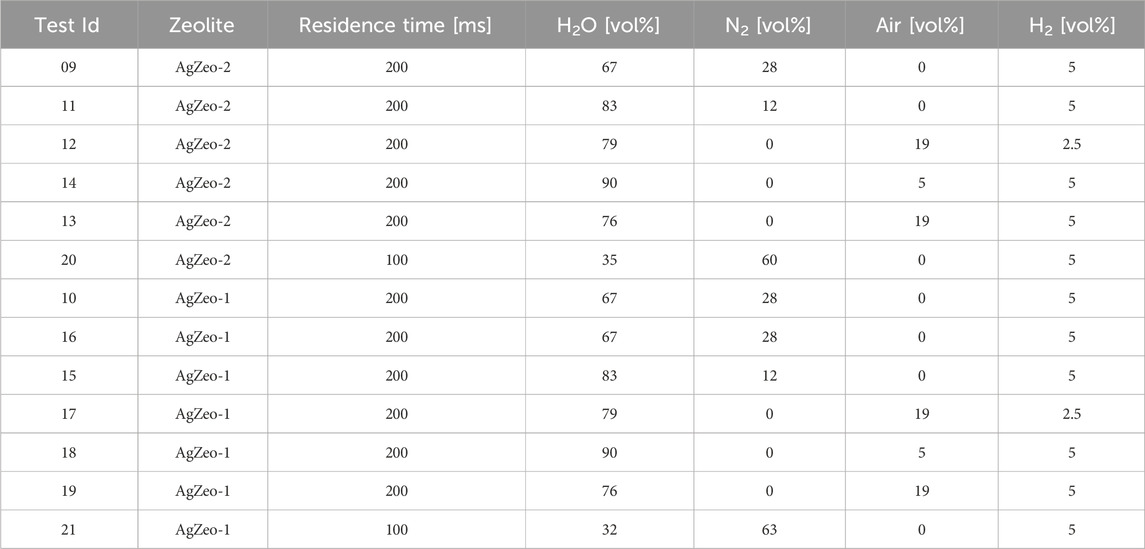
Table 1. The test matrix for experiments to determine simultaneous retention of CH3I and hydrogen recombination.
The main aim of the tests was to determine CH3I retention under challenging test conditions, i.e., high steam content and not only oxidizing but also reducing conditions. The gas residence time was 200 m in most of the tests, two tests (20 and 21) were conducted with a shorter residence time of 100 m to determine the effect of residence time on both CH3I retention and H2 recombination. Hydrogen content was either 5 or 2.5 vol%.
CH3I concentration at the test section inlet was in the range of 100–280 ppm which is very high compared to the concentration expected in the nuclear reactor containment or FCVS during a severe accident. The reason for the high CH3I concentration was purely practical: in order to be able to accurately determine the outlet concentration, the inlet concentration needed to be relatively high. The high CH3I concentration in the inlet gas is not expected to affect the measured retention in the beginning of the test, however, with the high CH3I concentration the zeolite may lose its efficiency faster than with a low CH3I loading as the Ag sites in the zeolite will get saturated with iodine.
An effective test duration of 20 min was used in the tests. This means that after the start-up of the facility with pre-heating and stabilization of the gas flows, the measurements were started and conducted for 20 min. During this time, the gas flows were kept constant, and the temperatures at different locations of the facility and the test section as well as the gas composition were measured. During this time, CH3I concentration was determined at the test section inlet and outlet as described below. The total CH3I retention was determined during this 20 min test time.
2.3 Organic iodide
2.3.1 Organic iodide feeding
Organic iodide was fed to the facility from a borosilicate glass bottle filled with about 0.20 L of liquid CH3I (Sigma-Aldrich Chemie GmbH, liquid >99.0%). A nitrogen gas flow with a temperature of about 300 K was passed downward towards the surface of the liquid to transfer the CH3I vapor. The nitrogen-CH3I gas mixture left the glass bottle at the top through a 0.002 m inner diameter stainless steel tube. The nitrogen gas flow rate through the bottle was controlled by a thermal mass flow controller Brooks Model 5850 S. The flow rate was adjusted to achieve the desired CH3I concentration in the facility. The outgoing gas mixture from the bottle was heated by electrical heating tape to 433 K before being added to the main feed line of the facility. To ensure that the correct amount of CH3I was present in the gas mixture, an ionization detector (PID) and a gas mass spectrometer were used.
2.3.2 Organic iodide measurement system
Gas sampling systems were installed at the test facility inlet and outlet lines to determine the concentration of CH3I. The gas sampled from the facility lines was directed by a pneumatically actuated valve to a 0.004 m heated stainless-steel pipe to the sample gas conditioning system. Firstly, nitrogen was added to the gas stream corresponding to the same volumetric fraction as steam present in the sample. Next, the sampled gas stream was cooled down using a Dimroth spiral glass condensing heat exchanger with the length of 2 × 0.30 m, and any steam condensed was trapped in a separation funnel. The secondary side coolant water of the heat exchanger was operated at a temperature of 290 K. Then the gas stream leaving the cooler was heated up to about 313 K to reduce the relative humidity.
CH3I concentration at the facility inlet and outlet sampling system was determined by two methods. The online measurement of the CH3I concentration was performed by Photo Ionization Detector (PID; RaeSystems (Honeywell), Model RAEGuard 2). The PID was mainly used to continuously monitor the stability of the organic iodide feed and concentration. The PID measures volatile organic gases by ionizing the gas by means of an ultraviolet (UV) light source (10.6 eV). Two systems with different measurement ranges (0.01–100 ppm and 0.1–1,000 ppm) were used at the facility outlet and inlet, respectively. The resolution was 10 ppb with an accuracy related to the calibration point of ±2%.
For determination of CH3I retention in the zeolite, a mass spectrometer (Pfeiffer Vacuum, Omnistar GSD300, 1–200 amu) using a Faraday detector was connected to the test facility at the inlet and the outlet lines, alternating between measurement of inlet and outlet concentration. The mass spectrometer was attached to a heated gas inlet distribution system to which two heated capillary stainless-steel sampling lines of 0.0015 m inner diameter were connected from the test facility. The gas was continuously sampled to the gas inlet distribution system through electrically actuated 3-way valve. Nitrogen purge gas was used to clean the inlet lines to the mass spectrometer when switching between the two sampling lines in order to purge any residual gas from the previous measurement. The mass spectrometer provided concentration measurements of the CH3I gas in a semi-online mode, one scan of the sampled gas taking typically around 1–2 s per data point. Before each test, the mass spectrometer was calibrated against certified calibration gases.
2.4 Hydrogen measurement
The hydrogen content of the gas at the test section outlet was measured using a Micro-Gas Chromatography (micro-GC; INFICON, Micro-GC Fusion). In addition to hydrogen, the micro-GC was also capable of measuring a range of other compounds, such as inhibiting gases (e.g., CO, N2O) and hydrocarbons (e.g., CH2H6, C2H6O, CH3OH). For the measurement of the hydrogen concentration, the micro-GC system was integrated into an automated gas distribution system using a 10-port valve switching device providing the possibility to switch between the sample gas, calibration and purge gas during the test. The micro-GC was calibrated prior to the use and on regular intervals depending on the expected concentration range and species.
2.5 Materials
In this work, two different Ag-zeolites were investigated, AgZeo-1 and AgZeo-2. AgZeo-2 has been designed to retain gas phase iodine in the conditions corresponding to the nuclear reactor containment as well as FCVS. AgZeo-1 has been designed, in addition to iodine retention, also to be effective in catalyzing hydrogen recombination thereby reducing hydrogen risk in the containment and in the FCVS.
Several applications are proposed to AgZeo-1 and AgZeo-2 in nuclear power industry. The aim of this work is to assess their suitability for two different applications:
1. As a secondary filter downstream of a wet scrubber. In this application, the main function of the Ag-zeolite is to retain gas phase, mainly organic, iodine species in the FCVS. In addition, it can decrease the risk of hydrogen combustion in the stack by reducing the hydrogen content in the vent gas by catalyzing the recombination of hydrogen.
2. In the containment during an accident in which hydrogen and/or iodine are released to the containment. Here Ag-zeolites can be used to reduce the content of both hydrogen and gas phase iodine in the containment atmosphere, thereby reducing the risk of hydrogen combustion in the containment and reducing the iodine load to the FCVS in case containment venting is needed. This part of the work is highly exploratory as the practical application of the zeolites in the containment has so far not been employed.
The conditions under which AgZeo-1 and AgZeo-2 are expected to operate are highly dynamic with a wide range of temperatures, pressures, gas compositions and other parameters. In addition, the conditions for the two applications are different. Thereby the tests in this investigation covered a wide range of conditions from high steam content to low steam content, as well as highly oxidiging and highly reducing atmospheres.
The material properties of AgZeo-1 and AgZeo-2 are summarized in Table 2. The apparent bulk density was determined experimentally by using a measuring cylinder, which was filled up to 0.10 L of AgZeo-1 and AgZeo-2 zeolite, respectively. A balance recorded the weight. BET (Brunauer–Emmett–Teller) area was measured by N2 physisorption. AgZeo-1 showed considerably larger BET area than AgZeo-2. This is a significant difference as the specific surface area of a material has a decisive effect on surface reactions. Based on higher surface area, it can be expected that AgZeo-1 would have a better accessibility for the reactive gases then AgZeo-2. Images taken by an optical microscope reveal a distinctly different structure of the two zeolites, Figure 3.
3 Results
3.1 Catalyst structure and elemental composition
Fresh AgZeo-1 samples as well as samples taken from two experiments were analyzed with Scanning Electron Microscopy and Energy Dispersive X-Ray spectroscopy (EDX). The samples were taken from tests in which the capability of AgZeo-1 to catalyze hydrogen recombination was tested, i.e., no iodine species were used. In test A, 2% hydrogen was used in a small-scale facility (Espegren et al., 2022) in air atmosphere; in test B, 6% hydrogen was used under otherwise similar conditions. It should be noted that the maximum temperature of the zeolite reached approximately 470K and 820 K in the two tests, respectively. The inlet gas temperature was 415 K.
The comparison of the fresh AgZeo-1 with that from tests A and B shows only very small differences, Figures 4, 5. This indicates that even at a relatively high catalyst temperature of more than 800 K in test A, the catalyst structure retained its original shape.
Qualitative elemental mapping of Si, Ag, Al, C, and O using EDX reveals that all these elements are uniformly distributed in the fresh AgZeo-1, Figure 6. It should be noted that no calibration standards were used for the analysis and that the concentration of carbon is affected by the coating applied in sample preparation. Thereby the results should be considered of qualitative nature. The sample taken after test A, Figure 7, shows that the distributions of Si and Ag remain homogeneous even when the catalyst was exposed to hydrogen and a high temperature above 800 K. Even when the temperature reached more than to 800K, AgZeo-1 maintained its structural integrity and did not break. This indicates that the distribution of Ag in the zeolite was not affected by exposure to high temperature, and that retention capacity for CH3I can be expected to remain high even at high temperature exposure of the AgZeo-1 zeolite.
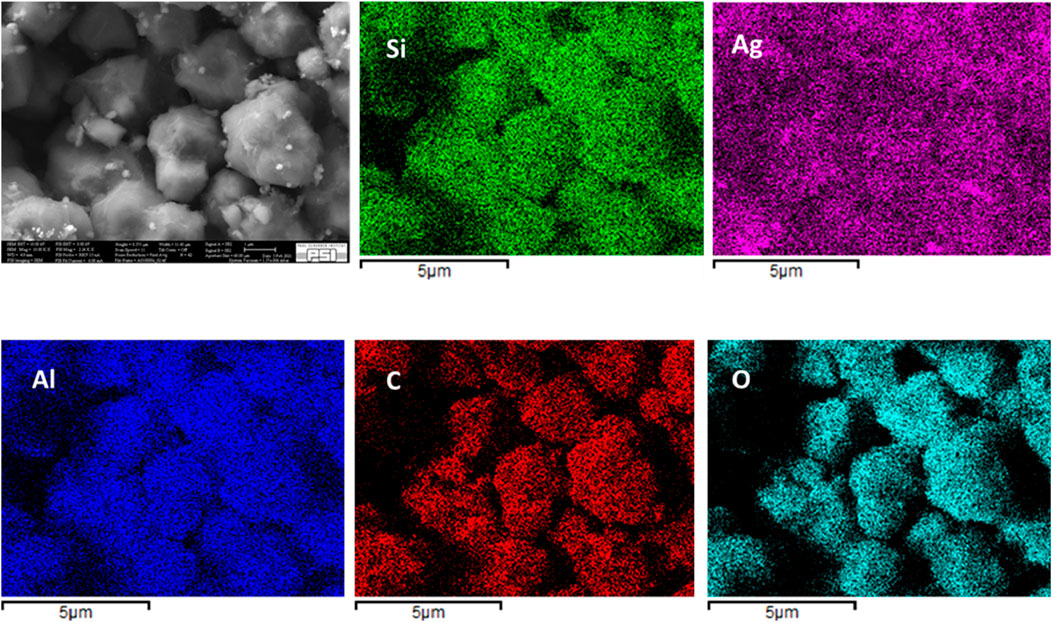
Figure 6. Elemental mapping of fresh AgZeo-1: The area used in the EDX analysis (upper left), distribution of Si (upper middle), Ag (upper right), Al (lower left), C (lower middle) and O (lower right) in the fresh AgZeo-1.
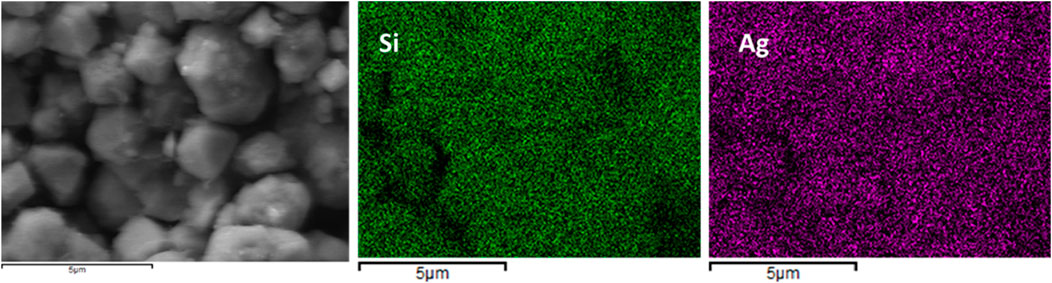
Figure 7. Elemental mapping of AgZeo-1 from test A: Distribution of Si (middle) and Ag (right) in the AgZeo-1 after test A. The area used in the analysis if shown on the left.
3.2 CH3I retention
CH3I retention during the 20 min test time was high in all the tests, Table 3. Retention was approximately 99% or higher in all the tests with the residence time of 200 m. It is notable that even in the tests 14 and 18 with the steam content of 90% and air content of 5%, CH3I retention was 99.9%. Also, the gas atmosphere, i.e., reducing or oxidizing, did not affect CH3I retention. The hydrogen content in the carrier gas did not affect CH3I retention efficiency, as demonstrated by comparing tests 12 and 14, as well as 17 and 19, respectively. In these tests, other test conditions remained the same with 19% air, but the former tests were conducted with 2.5% hydrogen and the latter ones with 5% hydrogen. CH3I retention efficiency remained high in all the tests regardless of the hydrogen concentration.
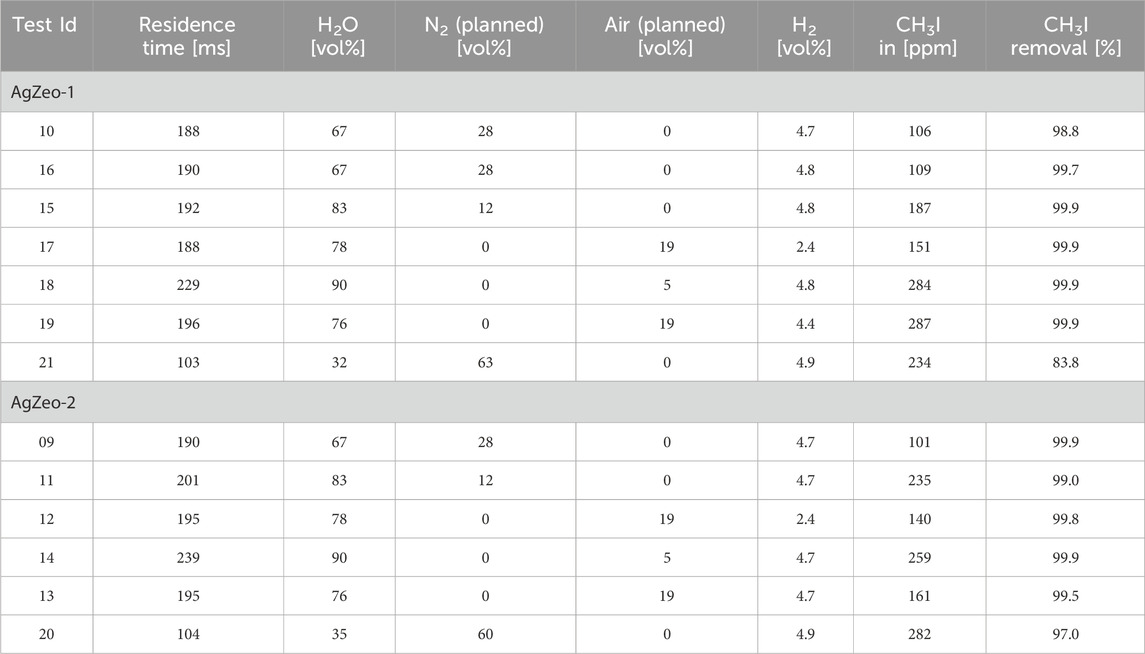
Table 3. The test matrix for experiments to determine simultaneous retention of CH3I and hydrogen recombination; results for CH3I retention.
The only tests in which CH3I retention was less than approximately 99% were those with a reduced residence time of 100 m. With 100 m residence time, CH3I retention in AgZeo-2 was 97.0% and in AgZeo-1 83.8%. These two tests were conducted in reducing atmosphere with no air, 5% hydrogen, 60% nitrogen and 32%–35% steam in the carrier gas.
It should be noted that the determination of the CH3I retention was limited by the accuracy of approximately 1 ppm of the CH3I measurements at the test section outlet. Thereby in the tests, CH3I retention might have been higher than the one determined using the current instrumentation. The experimental uncertainty is given in the Supplementary Material.
3.3 Hydrogen recombination
One of the investigated zeolites, AgZeo-1, was designed to catalyze recombination of hydrogen in addition to retaining CH3I under severe accident conditions. In AgZeo-2, the capability for catalyzing recombination is not promoted. This behavior was clearly visible when comparing tests under oxidizing conditions with 19% air in the carrier gas in the tests 12 and 13 for AgZeo-2 and 17 and 19 for AgZeo-1, Table 4 and Figure 8. Whereas hydrogen removal in these tests with AgZeo-2 was rather low at 4% and 43%, respectively, with 2.5% and 5% hydrogen, it was clearly higher with AgZeo-1 at 83% and 100%, again with 2.5% and 5% hydrogen, respectively. These results show also that hydrogen retention by recombination was, as expected, higher at higher hydrogen content in the gas, as visible in results with both zeolites. Notable is that the recombination reaction was started already at a low concentration of 2.5% hydrogen.
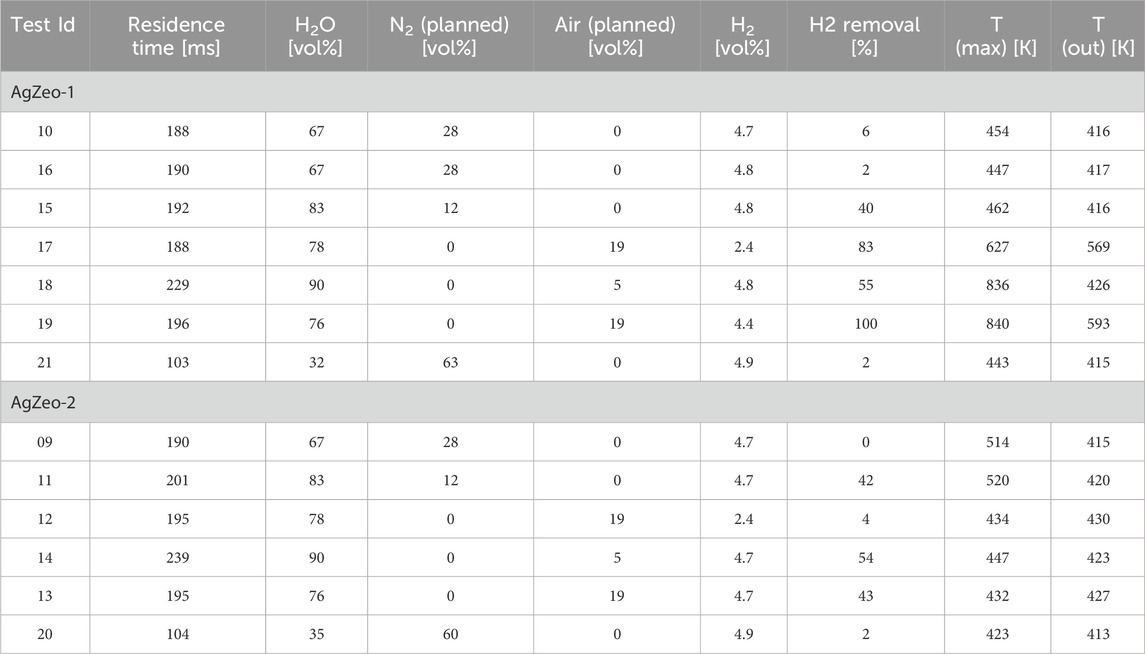
Table 4. The test matrix for experiments to determine simultaneous retention of CH3I and hydrogen recombination; results for hydrogen removal.
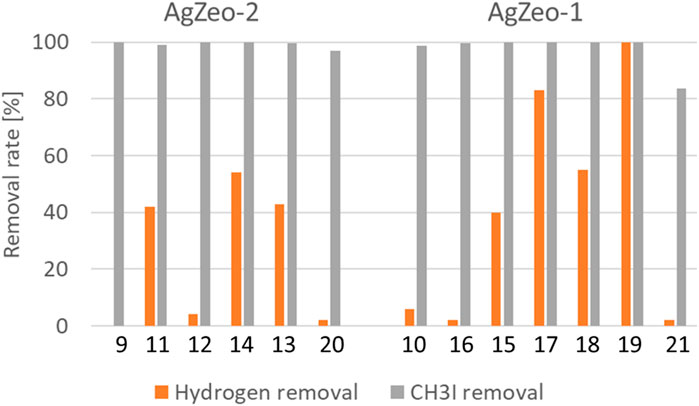
Figure 8. Hydrogen and CH3I removal in the tests with AgZeo-2 and AgZeo-1. For the test numbers, see Table 3.
It is noted that when the hydrogen removal rate was high with AgZeo-1, the maximum temperature measured inside the zeolite bed was significantly increased from the inlet temperature of 415–420 K. In test 19 with high hydrogen removal rate in the presence of 19% air, the maximum zeolite temperature was more than 800 K. As stated earlier, the zeolite structure maintained its structural integrity also at these high temperatures. The temperature increase is assumed to be the result of hydrogen recombination with oxygen. Thereby in the tests in oxidizing atmosphere, it is concluded that hydrogen recombination was responsible for hydrogen removal from the gas. As described earlier, SEM analysis showed that despite the high maximum temperature of the zeolite, Ag concentration remained homogeneous, and the microstructure of the zeolite did not show any significant changes after the tests.
An interesting result is shown in the absence of air, with 12% nitrogen and 5% hydrogen added to steam. In these tests 11 and 15, both zeolites retained approximately 40% of the hydrogen. The atmosphere in these tests was highly reducing, thereby hydrogen recombination by oxygen should be excluded. One possible explanation to this behavior is physical adsorption of hydrogen on the zeolite surface under the test conditions. If adsorption indeed was the reason for hydrogen removal, it can be presumed that the removal would be reversible. A comparison of the test results of tests 11 and 15 with the tests carried out at similar conditions in a smaller catalyst test facility (Espegren et al., 2022) reveals that the tests in the smaller test facility showed no removal of hydrogen. Thereby it seems that the removal of hydrogen under reducing conditions is related to the facility scale, possibly due to uneven temperature distribution in the larger test facility or other facility dependent factors. Further analysis is needed to determine if such adsorption would be expected in a large-scale application of zeolites. It is to be noted that removal of hydrogen under reducing conditions did not adversely affect CH3I removal, i.e., retention of hydrogen did not reduce retention efficiency of CH3I.
The tests in the highest nitrogen concentrations in steam atmosphere with 28% and 60% nitrogen showed no retention of hydrogen with either of the zeolites. This means that under these conditions, hydrogen was not recombined as there was not free oxygen available and it was not absorbed in the zeolite either.
5% air and 5% hydrogen were added to steam in tests 14 and 18. In these tests, oxygen concentration should be sufficient to oxidize approximately 40% of hydrogen. With hydrogen removal of 54% and 55%, the removal is close to that possible by hydrogen recombination, and it is not possible to conclude if the removal of hydrogen is due to recombination or adsorption in the zeolite. However, given that in the absence of air in tests with only nitrogen and hydrogen, up to 40% of hydrogen was removed by both zeolites, it is plausible that at least a fraction of the hydrogen retention in the 5% air tests is due to physical adsorption in the zeolites, and not only due to hydrogen recombination with oxygen. Further tests would be needed to investigate the effect of reactor size on the possible adsorption of hydrogen in Ag-zeolite, and if the adsorption would be reversible, i.e., physical adsorption, or irreversible. This additional investigation was beyond the scope of current work.
4 Conclusion
The characteristics and performance of two Ag-zeolites was experimentally investigated in a medium-scale laboratory facility under challenging, high humidity conditions in both oxidizing and reducing atmospheres. One of the zeolites, AgZeo-1, was designed to simultaneously retain gas phase organic iodides, in this investigation represented by CH3I, and recombine hydrogen thereby reducing hydrogen risk in the nuclear reactor containment during an accident. The other zeolite, AgZeo-2, was designed to retain organic iodides but not to recombine hydrogen.
Characterization of the zeolites showed a distinctly different structure of the materials as imaged with an optical microscope and demonstrated by a much larger surface area of AgZeo-1 as measured with BET measurement. AgZeo-1 was further analyzed after being exposed to high temperatures during a test showing hydrogen recombination. It was shown that after being exposed to temperatures above 800 K, the bulk volume of AgZeo-1 had been slightly reduced but the structural integrity was maintained, as shown by SEM analysis. The silver distribution was as homogeneous as with the fresh zeolite indicating a solid performance even at high temperatures. The performance for organic iodide retention and hydrogen recombination was indeed not affected by exposure of the zeolite to high temperatures.
CH3I retention was shown to be high in all the tests in which the residence time in the reaction chamber was 200 m, regardless of the gas composition. When the gas residence time in the reaction chamber was reduced to 100 m, CH3I retention was reduced to 97% and 84% with AgZeo-2 and AgZeo-1, respectively. In these tests, the gas atmosphere was reducing composed of about 5% hydrogen, 65% nitrogen and steam making up the balance, i.e., no air was added in the reaction gas. It should be noted that in the other tests carried out in reducing gas atmospheres, CH3I retention remained high as long as the gas residence time in the reaction chamber was 200 m.
Both zeolites were found to retain hydrogen to some extent. In oxidizing atmosphere, AgZeo-1 which has been designed to catalyze hydrogen recombination was much more efficient in reducing hydrogen concentration than AgZeo-2. Under reducing conditions the performance of both zeolites was comparatively similar. An interesting observation was done when 5% air and 5% hydrogen was added in steam. Under these conditions, hydrogen concentration was significantly reduced using both zeolites, presumably due to absorption in the zeolite. Similar behavior was not observed in small-scale laboratory tests under similar conditions but without organic iodide in the gas. It is noted that in the tests with air in the carrier gas, the temperature of AgZeo-1 increased significantly even in the presence of high steam fraction. The temperature increase if believed to be a result of the recombination reaction between hydrogen and oxygen. Tests at higher hydrogen and oxygen concentrations would be recommended to address this issue.
Data availability statement
The raw data supporting the conclusions of this article will be made available by the authors, without undue reservation.
Author contributions
TL: Conceptualization, Funding acquisition, Supervision, Visualization, Writing–original draft. FE: Data curation, Formal Analysis, Investigation, Writing–review and editing. DS: Investigation, Methodology, Project administration, Resources, Writing–review and editing.
Funding
The author(s) declare that financial support was received for the research and/or publication of this article. The funding to this work has been provided by Japanese METI and Rasa Industries. Open access funding by PSI - Paul Scherrer Institute
Acknowledgments
The authors would like to thank Rasa Industries and Morimura brothers, especially K. Endo, Y. Ishikawa, and S. Kobayashi, for collaboration, materials, and for funding this work. The work of the laboratory personnel at PSI and at Rasa Industries is gratefully acknowledged.
Conflict of interest
The authors declare that the research was conducted in the absence of any commercial or financial relationships that could be construed as a potential conflict of interest.
Generative AI statement
The author(s) declare that no Generative AI was used in the creation of this manuscript.
Publisher’s note
All claims expressed in this article are solely those of the authors and do not necessarily represent those of their affiliated organizations, or those of the publisher, the editors and the reviewers. Any product that may be evaluated in this article, or claim that may be made by its manufacturer, is not guaranteed or endorsed by the publisher.
Supplementary material
The Supplementary Material for this article can be found online at: https://www.frontiersin.org/articles/10.3389/fnuen.2025.1567507/full#supplementary-material
References
Albiol, T., Herranz, L., Riera, E., Dalibart, C., Lind, T., Del Corno, A., et al. (2018). Main results of the European PASSAM project on severe accident source term mitigation. Ann. Nucler Energy 116, 42–56. doi:10.1016/j.anucene.2018.02.024
Bal, M., Reddy, T. T., and Meikap, B. C. (2018). Performance evaluation of venturi scrubber for the removal of iodine in filtered containment venting system. Chem. Eng. Res. Des. 138, 158–167. doi:10.1016/j.cherd.2018.08.019
Chebbi, M., Azambre, B., Cantrel, L., Huvé, M., and Albiol, T. (2017). Influence of structural, textural and chemical parameters of silver zeolites on the retention of methyl iodide. Microporous Mesoporous Mater. 244, 137–150. doi:10.1016/j.micromeso.2017.02.056
Choi, Y., Kim, H., Moon, G., Jo, S., and Choi, W. (2015). Boosting up the low catalytic activity of silver for H 2 production on Ag/TiO 2 photocatalyst: thiocyanate as a selective modifier. ACS Catal. 6, 821–828. doi:10.1021/acscatal.5b02376
Espegren, F., Suckow, D., Lind, T., Mantzaras, J., Theile, J., Ishikawa, Y., et al. (2022). “Effectiveness of hydrogen recombination by AgZeo-1 and AgZeo-2 zeolites,” in ERMSAR2022 (10th European Review Meeting on Severe Accident Research), Karlsruhe, Germany, May 16-19, 2022. KITopen-ID: 1000151444.
Gupta, S., Freitag, M., and Poss, G. (2021). Thai experimental research on hydrogen risk and source term related safety systems. Front. Energy 15, 887–915. doi:10.1007/s11708-021-0789-1
Kissane, M. (2008). On the nature of aerosols produced during a severe accident of a water-cooled nuclear reactor. Nucl. Eng. Des. 238, 2792–2800. doi:10.1016/j.nucengdes.2008.06.003
Leroy, O., and Bosland, L. (2023). Study of the stability of iodine oxides (IxOy) aerosols in severe accident conditions. Ann. Nucl. Energy 181, 109526. doi:10.1016/j.anucene.2022.109526
OECD/NEA (2007). “State of the art report on iodine chemistry,”. Nuclear Energy Agency of the OECD. OECD/NEA Report NEA/CSNI/R(2007)1.
OECD/NEA (2009). State-of-the-Art report on nuclear aerosol. Nuclear Energy Agency of the OECD. OECD/NEA Report NEA/CSNI/R(2009)5.
OECD/NEA (2014). Status report on filtered containment venting. Nuclear Energy Agency of the OECD. OECD/NEA Report NEA/CSNI/R(2014)7.
Pérez-Botella, E., Valencia, S., and Rey, F. (2022). Zeolites in adsorption processes: state of the art and future prospects. Chem. Rev. 122, 17647–17695. doi:10.1021/acs.chemrev.2c00140
Simondi-Teisseire, B., Girault, N., Payot, F., and Clément, B. (2013). Iodine behaviour in the containment in Phébus FP tests. Ann. Nucl. Energy 61, 157–169. doi:10.1016/j.anucene.2013.02.039
Soelberg, N. R., Garn, T. G., Greenhalgh, M. R., Law, J. D., Jubin, R., Strachan, D. M., et al. (2013). Radioactive iodine and krypton control for nuclear fuel reprocessing facilities. Sci. Technol. Nucl. Facil. 2013, 1–12. doi:10.1155/2013/702496
Wang, J. F., Kobayashi, T., Uzuyama, Y., and Endo, K. (2017). “Development of organic iodine adsorbents,” in Proceedings of TopSafe 2017, Vienna, Austria, February 12-16, 2017.
Keywords: iodine, severe accident, hydrogen, zeolite, fcvs
Citation: Lind T, Espegren F and Suckow D (2025) An experimental study of iodine retention in Ag-zeolites at high humidity conditions. Front. Nucl. Eng. 4:1567507. doi: 10.3389/fnuen.2025.1567507
Received: 27 January 2025; Accepted: 17 March 2025;
Published: 28 April 2025.
Edited by:
Sanjeev Gupta, Becker Technologies, GermanyReviewed by:
Željko Grahek, Rudjer Boskovic Institute, CroatiaTeemu Kärkelä, VTT Technical Research Centre of Finland Ltd., Finland
Copyright © 2025 Lind, Espegren and Suckow. This is an open-access article distributed under the terms of the Creative Commons Attribution License (CC BY). The use, distribution or reproduction in other forums is permitted, provided the original author(s) and the copyright owner(s) are credited and that the original publication in this journal is cited, in accordance with accepted academic practice. No use, distribution or reproduction is permitted which does not comply with these terms.
*Correspondence: Terttaliisa Lind, dGVydHRhbGlpc2EubGluZEBwc2kuY2g=
‡Retired
†Present address: Fredrik Espegren, Direktoratet for strålevern og atomsikkerhet (DSA), Oslo, Norway