- 1In-vivo Testing Laboratory, Molecular Bioprospection Department, CSIR-Central Institute of Medicinal and Aromatic Plants, Lucknow, India
- 2Academy of Scientific and Innovative Research (AcSIR), Ghaziabad, India
- 3Analytical Chemistry Department, CSIR-Central Institute of Medicinal and Aromatic Plants, Lucknow, India
Ellagic acid (EA), a fruit- and vegetable-derived flavonoid, has been reported for multiple pharmacological activities, which encouraged us to examine its useful effect in severe malaria pathogenesis, especially malaria-induced cytokine storms and oxidative stress linked to damage in major organs. Malaria was induced by injecting Plasmodium berghei–infected RBCs intraperitoneally into the mice. EA was given orally (5, 10, and 20 mg/kg) following Peter’s 4-day suppression test. EA exhibited the suppression of parasitemia, production of inflammatory cytokine storms and oxidative stress marker level quantified from vital organs significantly and an increase in hemoglobin, blood glucose, and mean survival time compared to the vehicle-treated infected group. EA administration also restored the blood–brain barrier integrity evidenced through Evans blue staining. Furthermore, we demonstrated the protecting effect of EA in LPS-induced inflammatory cytokine storms and oxidative stress in glial cells. The present study conclude that ellagic acid is able to alleviate severe malaria pathogenesis by reducing cytokine storms and oxidative stress–induced by malarial parasites. It also attributed promising antimalarial activity and afforded to improve the blood glucose and hemoglobin levels in treated mice. These research findings suggested the suitability of ellagic acid as a useful bioflavonoid for further study for the management of severe malaria pathogenesis.
Introduction
According to the WHO, the incidence and death rates due to malaria have dropped down worldwide over the past 16 years; in 2018, there were 228 million new cases and 405,000 deaths from malaria. Still malaria is an important public health problem in many developing countries of the tropical and subtropical regions of the world, with high mortality in children and pregnant women (World Health Organization, 2019). Malaria is caused by Plasmodium parasites which are transmitted to humans through the bite of female mosquitoes of Anopheles species (Snow et al., 2005). Vaccination is the most effective method of preventing infectious diseases; however, producing an effective vaccine remains a challenge in malarial infection (Stanisic and Good, 2015). Current findings established that free radicals and its connection with oxidative stress in severe malaria can be responsible for several additional complications (Pabon et al., 2003; Becker et al., 2004). Clinical indications in severe malaria were found to be linked with the blood stage of infection with an excessive release of inflammatory cytokines (TNF-α, IL-6, and INF-γ) which contribute to further severity of infection such as organ damage and severe anemia (Saxena et al., 2016). Treatment of malaria has been complicated by the development of resistance to combination therapies based on artemisinin (Kumar et al., 2015).
Ellagic acid (EA) is a thermostable dilactone of hexahydroxydiphenic acid [2,3,7,8-tetrahydroxy-chromeno (5,4,3-cde)chromene-5,10-dione] with a molecular mass of 338.2 g/mol. EA is an important bioflavonoid present in several fruits, berries, and vegetables. It is also present as a primary constituent of various tannin-bearing antimalarial plants which are found in Africa (Vattem et al., 2005). EA has received considerable biomedical research attention due to their potent antioxidant activity and noticeable pharmacological effects on the prevention of various chronic pathological conditions linked with oxidative stress (Shakeri et al., 2018). EA possesses multiple health benefits including anti-inflammatory, antiviral, anticancer, antibacterial, hepatoprotective, cardioprotective, neuroprotective, gastroprotective, and antihyperlipidemic effects (Evtyugin et al., 2020). Considering the pharmacological importance of ellagic acid, several research groups are working on nanotechnology-related experimental approaches based on innovative oral drug carriers to improve its bioavailability (Ceci et al., 2020). In an attempt to evaluate the novel pharmacological activity of ellagic acid, we have explored the beneficial effect of EA on oxidative stress and inflammation-linked severe malaria pathogenesis in mice. The research findings of this study suggested the suitability of ellagic acid as a useful bioflavonoid for further study for the management of severe malaria pathogenesis.
Materials and Methods
Chemicals
Ellagic acid (Figure 1), chloroquine diphosphate, sodium chloride (NaCl), potassium dihydrogen phosphate (KH2PO4), LPS (Escherichia coli 055:B5), disodium hydrogen phosphate (Na2HPO4), DMEM, FBS, streptomycin, penicillin, potassium chloride, 2′,7′-dichlorofluorescein diacetate (DCFH-DA), trichloroacetic acid, thiobarbituric acid, pyrogallol, TMB, Evans blue, and dimethylsulfoxide (DMSO) were procured from Sigma-Aldrich, United States. Mouse- and rat-specific ELISA kits were purchased from BD Biosciences.
In Vivo Study
Experimental Animals and Ethical Approval
Swiss albino (male, 18–22 g) mice were obtained from the institutional animal facility and acclimatized for 7 days under standard environmental conditions (12:12 dark-to-light cycle, 23 ± 2°C). After the termination of experiments, the experimental mice were euthanized by cervical dislocation after anesthetizing the animals using ketamine (80 mg/kg) and xylazine (10 mg/kg) as per the approved protocol (CIMAP/IAEC/2016-19/09). The vital organs of the experimental mice were immediately isolated for quantification of inflammation and oxidative stress markers. Animal experiments were performed as per the approved protocol by the Institutional Animal Ethics Committee (IAEC).
Infection and Drug Treatment
Malaria was induced by giving intraperitoneal injection of Plasmodium berghei K-173–infected RBC’s into the experimental mice. The antimalarial activity was assessed using the method described by Knight and Peters (Kalia et al., 2015). One hour after infection, the experimental mice were orally treated with EA (5, 10, and 20 mg/kg) for 4 days consecutively. Carboxymethylcellulose (CMC) was used as a vehicle. Thin smears were prepared from blood of the infected mice and stained with Giemsa stain every alternate day from 4th day up to 28th day to calculate parasitemia by microscopic examination. Parasitemia was counted based on parasitized RBCs counted per 100 normal RBCs. Suppression of parasitemia after treatment was calculated by using the formula [(A-B)/A]100, where A denotes the mean percent parasitemia of the vehicle-treated mice and B denotes the mean percent parasitemia of the treated group. Survival of the infected experimental mice was observed up to the 28th day to calculate the percent survival and mean survival time.
Quantification of Blood Glucose and Hemoglobin
On the peak day of parasitemia, blood glucose and hemoglobin were quantified to evaluate the possible effect of EA in malarial infection. Hemoglobin was quantified using Drabkin’s cyanmethemoglobin procedure and blood glucose using a glucometer.
Quantification of Inflammatory Mediators
Blood was collected from each mouse for serum, and whole brain was isolated on the peak day of infection. Serum and brain homogenate were used for the quantification of pro-inflammatory cytokine production by using ELISA reagents.
Quantification of Oxidative Stress Markers
To determine the effect of EA on organ damage caused by oxidative stress due to malaria infection in mice, a separate set of experiment was performed. The vital organs were harvested from the infected mice for oxidative stress marker quantification. In total, 10% (w/v) tissue homogenates were prepared and centrifuged at 5000 g for 10 min, and the collected supernatant was stored at -80°C immediately. The activity of superoxide dismutase was evaluated for its ability to inhibit pyrogallol autoxidation (Del Maestro and McDonald, 1985). The production of reactive oxygen species (ROS) was quantified using DCF-DA, a non-fluorescent cell-permeating compound, as described previously (Driver et al., 2000). Myeloperoxidase activity was quantified in tissue homogenates using TMB (3,3′5,5′ tetramethylbenzidine), as previously described (Suzuki et al., 1983). Lipid peroxidation was quantified by measuring the content of thiobarbituric acid reactive substances (TBARS) spectrophotometrically (Draper and Hadley, 1990).
Evans Blue Extravasation Assay
For Evans blue assay, 0.2 ml of 2% Evans blue solution in PBS was injected intraperitoneally into the infected mice to assess the blood–brain barrier permeability on the peak day of parasitemia. After 2 h, brain tissues were removed for dye extraction in 100% formamide. After 48 h, absorbance was measured at 620 nm and presented as micrograms of Evans blue stain per gram of brain tissue (Baptista et al., 2010).
In Vitro Study
Quantification of ROS Generation and Pro-Inflammatory Mediators in C6 Glial Cells
C6 glial cells, procured from the National Centre for Cell Science (NCCS), Pune, India, were cultured using Ham’s F-10 media containing 10% FBS and placed in an incubator (5% CO2, 37°C). The cytotoxicity effect of EA was assessed using MTT assay on C6 cells, as described previously (Sharma et al., 2012). Intracellular ROS generation induced by H2O2 was calculated using DCFH-DA. The fluorescence intensity was measured with a spectrofluorometer (FLUO Star Omega, BMG Labtech), with an excitation wavelength of 485 nm and an emission wavelength of 520 nm. The intracellular reactive oxygen species production was also measured by flow cytometry (Vinoth et al., 2015). LPS-induced production of pro-inflammatory cytokines was quantified in the cell culture supernatant using rat-specific EIA reagents (Gupta et al., 2018).
Statistical Analysis
Experimental data were shown as means ± SEM. The statistical significance of vehicle vs treatment groups was calculated using ANOVA, followed by Tukey’s multiple comparison tests. p < 0.05 was considered significant.
Results
Effect of EA on Parasitemia and Mortality in Malaria Pathogenesis
Oral treatment with EA at a dose of 5, 10, and 20 mg/kg showed a significant inhibition of parasitemia (p < 0.05) in comparison to the vehicle-treated infected mice in a dose-dependent manner (Figure 2A). Mean survival time was also significantly improved in the EA-treated group compared to the vehicle-treated infected group (Figure 2B).
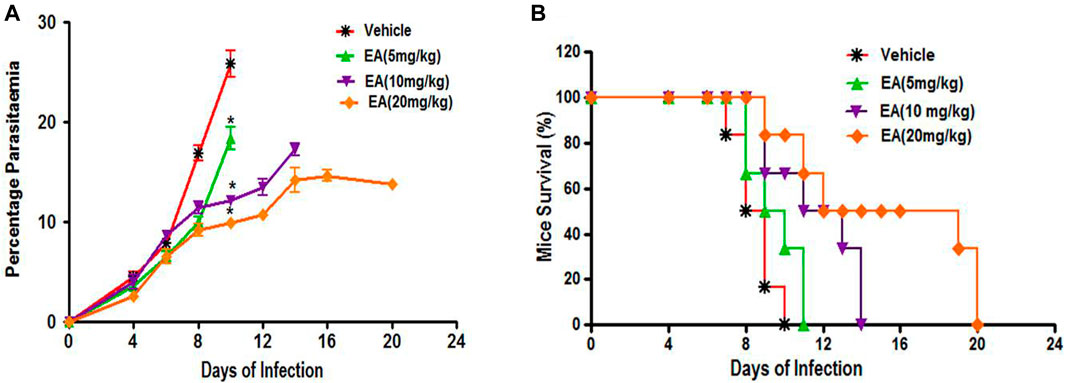
FIGURE 2. Effect of EA on antimalarial activity in P. berghei included malaria in mice (A) Percent Parasiteman (B) Percent Survival. Data are expressed as Mean ± SEM. *P < 0.05; *Vehicle vs Treatment; n = 6.
Effect of EA on Blood Glucose and Hemoglobin in Malaria Pathogenesis
Blood glucose and hemoglobin levels were found to be decreased significantly on the peak day of parasitemia in the vehicle-treated infected mice in compared to uninfected normal. Treatment with EA improved the blood glucose and hemoglobin in a dose-dependent manner (Figure 3).
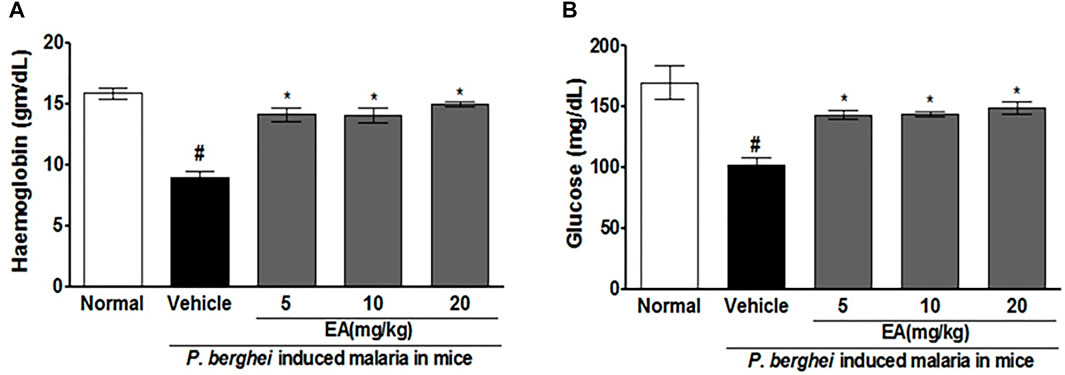
FIGURE 3. Effect of EA on haemoglobin and blood glucose level on peak day of parasitaemia in mice. (A) Haemoglobin (B) Blood glucose. Data are expressed as Mean ± SEM: *P < 0.05; #Normal vs vehicle treated; *Vehicle vs Treatment; n = 3.
Effect of EA on Oxidative and Antioxidative Markers in Malaria Pathogenesis
The MDA level, MPO activity, and ROS production were increased significantly (p˂0.05) in the brain, liver, and spleen of the vehicle-treated infected group in comparison to the uninfected normal group. EA treatment reduced the level of these oxidative stress markers compared with the vehicle-treated infected group. The SOD level was found to be significantly (p˂0.05) decreased in the vehicle-treated infected group compared to that in normal uninfected group, whereas EA-treated groups significantly increased the SOD level in comparison to the vehicle-treated infected group. The effect of EA treatment on the oxidative stress marker level in tissue homogenate is illustrated in Table 1.
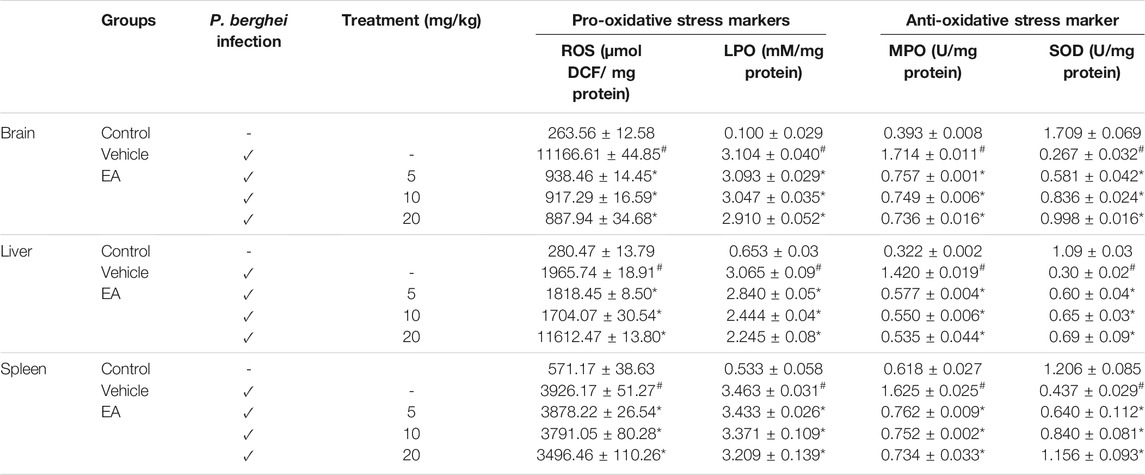
TABLE 1. Effect of EA on oxidative and antioxidant stress marker levels in major organs affected by malaria infection. Data are expressed as mean±SEM; P < 0.05 considered statistically significant; *vehicle vs treatment group; # control vs vehicle group; n = 4.
Effect of EA on Pro-Inflammatory Markers in Malaria Pathogenesis
The production of pro-inflammatory cytokines (TNF-α, IL-1β, IL-6, and IFN-γ) in serum and brain homogenate of the vehicle-treated infected mice was significantly increased compared to the normal group on the peak day of parasitemia. EA treatment showed a significant inhibition of pro-inflammatory cytokine production dose-dependently in malaria pathogenesis than in the vehicle-treated infected mice (Figures 4, 5).
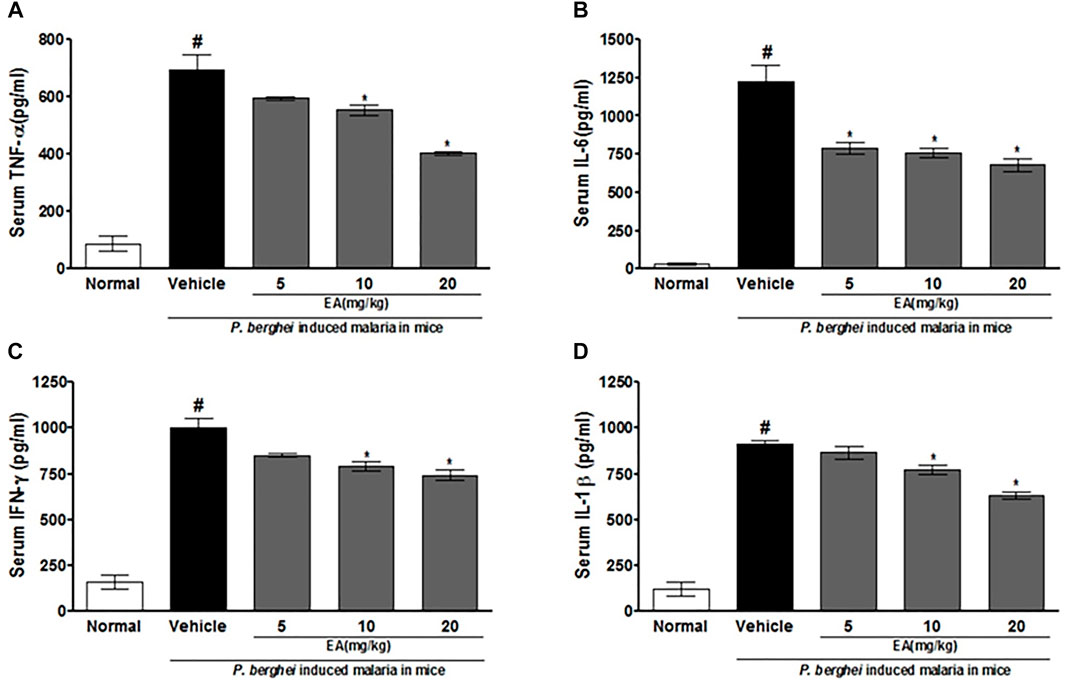
FIGURE 4. Effect of EA on production of pro-inflammatory cytokines TNF--α, IL-6, IFN-λ and IL-1β on peak day of parasitaemia in serum in P. berghei infected mice. Date are expressed as Mean ± SEM: P < 0.05; #Normal vs vehicle treated; *Vehicle vs treatment; n = 3.
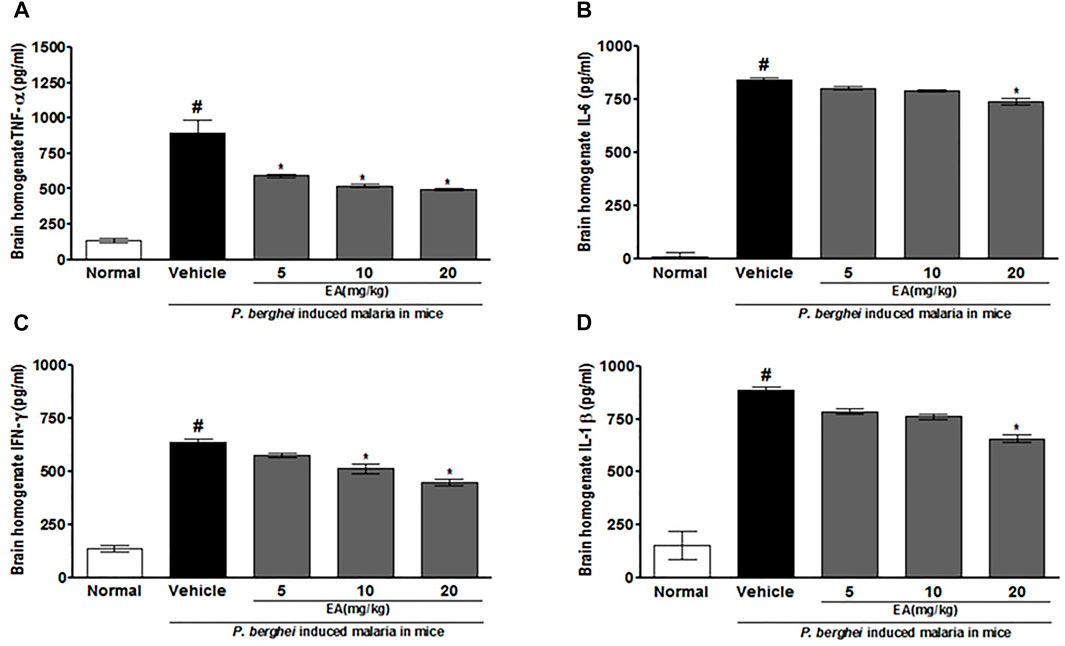
FIGURE 5. Effect of EA on production of pro-inflammatory cytokines TNF-α, IL-6, IFN-λ and IL-1β on peak day of parasitaemia in brain homogenate in P. berghei infected mice. Data are expressed as Mean ± SEM: P < 0.05; #Normal vs vehicle treated; *Vehicle vs treatment; n = 3. #Normal vs vehicle treated; *p < 0.05; *Vehicle vs treatment; n = 3.
Effect of EA on Blood–Brain Barrier Permeability in Malaria Pathogenesis
An increase in the blood–brain barrier (BBB) permeability is a characteristic feature of severe malaria pathogenesis. Evans blue leakage assay showed that the normal mice have no disruption of BBB, whereas the brain isolated from the vehicle-treated infected mice has shown prominent dye accumulation, indicating the breakdown of the BBB during malaria pathogenesis. The brain isolated from the EA-treated mice has shown the significant (p < 0.05) lower staining of dye than that from the vehicle-treated infected mice (Figure 6).
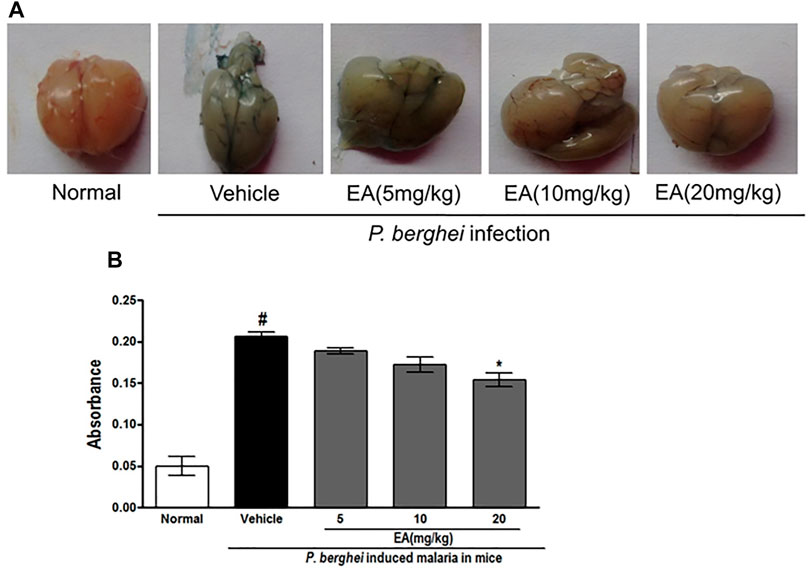
FIGURE 6. Vascular permeability of brains was assessed by Evans Blue injection on peak day of parasitaemia (A) Vascular leakage was assessed by the blue discoloration of the brain tissue of the various treated groups (B) Quantification of Evans Blue extravasation in formamide, absorbance was measured at 620 nm; n = 3. P < 0.05; #Normal vs vehicle treated; *Vehicle vs treatment.
Cytotoxicity Profile of EA in Glial Cells
The effect of EA on cytotoxicity in C6 glial cells was studied using MTT assay. After EA treatment at 10, 30, and 100 μg/ml, percent live cell population was not significantly (p < 0.05) changed compared with normal untreated cells.
Effect of EA on ROS Generation and Neuroinflammation in C6 Glial Cells
To investigate the effect of EA on ROS generation, the spectrofluorometric and flow cytometric analyses were performed. The absorbance recorded spectrophotometrically showed that H2O2 alone significantly (p < 0.05) generated ROS in C6 glial cells after 1.5 h of incubation when compared with unstimulated cells. EA treatment (1, 10, 30 μg/ml) significantly attenuated H2O2-induced ROS generation in a dose-dependent manner when compared to H2O2-treated cells (Figure 7A). In agreement with the spectrophotometric observation, in the flow cytometric analysis, DCF-positive cells were found to decrease dose-dependently when treated with EA (1, 10, 30 μg/ml) (Figure 7B). Also, H2O2-induced cells exhibited higher DCF fluorescence intensities than the unstimulated cells. ROS generation was significantly (p < 0.05) decreased when the H2O2-induced cells were treated with EA (1, 10, 30 μg/ml) dose-dependently (Figure 7C). Experiments were performed on C6 glial cells stimulated with LPS to induce the production of pro-inflammatory cytokines. The production of pro-inflammatory cytokines (TNF-α and IL-6) in the cell culture supernatant was increased significantly in the LPS-stimulated cells compared with that in the normal cells. EA treatment (1, 10 and 30 μg/ml) exhibited a significant (p < 0.05) inhibition of pro-inflammatory cytokine production dose-dependently compared with the LPS-stimulated cells (Figure 8).
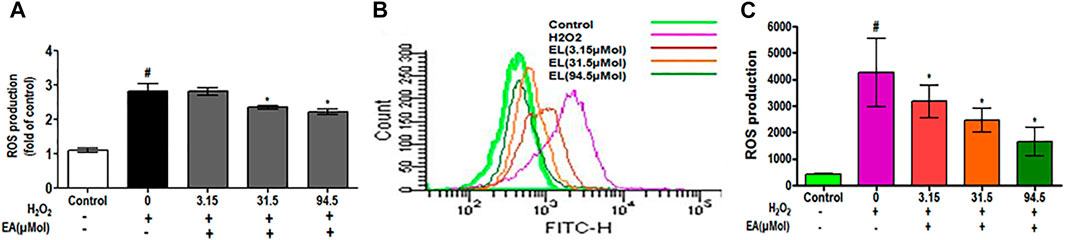
FIGURE 7. Effect of EA on H2O2-simulated ROS production using (A) spectrofluorometer and (B, C) flowcytometric analysis in C6 microglial cells. Data are expressed as Mean ± SEM; *p < 0.05 *treatment vs H2O2 treated cells. #p < 0.05 #control vs H2O2 treated cells; n = 3.
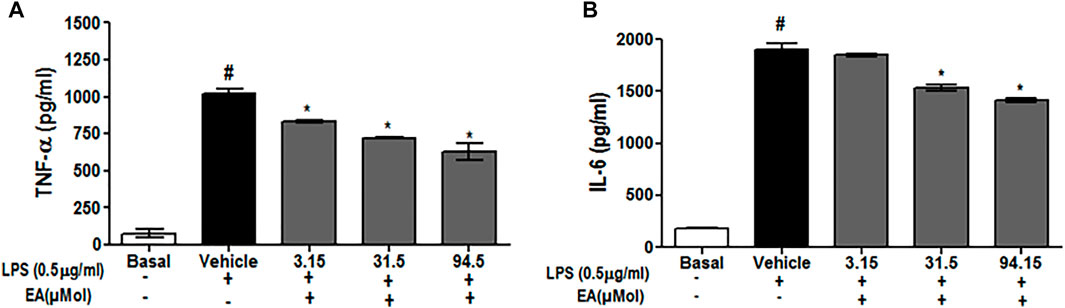
FIGURE 8. Effect of EA on production of pro-inflammatory cytokine (A) TNF-α and (B) IL-6 in C6 microglial cells. Data are expressed as Mean ± SEM; P < 0.05; #Normal vs Vehicle treated; *Vehicle vs treatment; n = 3.
Discussion
Malaria is a major public health problem in endemic countries with greater mortality in pregnant women and children, and severe malaria pathogenesis involves the series of host immune responses which eventually gets amplified, leading to severe organ injury and dysfunction (World Health Organization, 2019). Several research studies reported that malaria is a highly inflammatory condition characterized by acute periods of fever, headache, and nausea caused by Plasmodium infection of host red blood cells (Hunt, and Grau 2003; Togbe et al., 2007). Malaria-induced inflammatory cytokine storm circulating in the bloodstream of patients (Clark et al., 2008) is a critical pathological process which leads to severities like cerebral malaria, hypoglycemia, hyper-lactatemia, and acidosis (Schofield and Grau 2005) in malarial pathogenesis. Results of this study demonstrated that EA treatment is able to reduce parasitemia and increase the mean survival time in a dose-dependent manner in experimental malaria induced by P. berghei–infected RBCs in mice. Several findings suggest that plant-derived molecules exhibit antimalarial activity (Mohanty et al., 2013; Saxena et al., 2018; Gupta et al., 2018). Flavonoids are the natural molecules abundantly present in fruits and vegetables, showing beneficial effects against malarial pathogenesis (Puttappa et al., 2017; Amiri et al., 2018). Anemia and hypoglycemia are associated with severe malaria pathogenesis, which leads to mortality, especially in children and pregnant women (Boeuf et al., 2012). Oral treatment of EA is also able to restore hemoglobin and blood glucose in experimental malaria toward normal. Earlier published reports demonstrated that plant-derived natural molecules are able to restore hemoglobin and glucose levels in experimentally infected animals (Mohanty et al., 2015; Saxena et al., 2016). Oxidative stress due to malaria parasites if not checked by the host antioxidant mechanism can lead to oxidative damage in host tissues, which contributes to severe malarial pathogenesis (Becker et al., 2004). This study also revealed that EA treatment is able to protect from oxidative stress in vital organs during malarial pathogenesis. These findings are in agreement with the findings of recent reports that ellagic acid alleviates clozapine-induced oxidative stress and mitochondrial dysfunction in cardiomyocytes (Ahangari et al., 2020) and that plant-derived molecules are able to reduce the level of oxidative stress and improve the level of antioxidant enzymes in P. berghei–infected mice (Singh et al., 2017; Gupta et al., 2018). Results of this study reported a very high level of pro-inflammatory cytokine production (cytokine storms) in serum and the brain homogenate of P. berghei vehicle-treated infected mice on the peak day of parasitemia. EA treatment significantly inhibited the production of pro-inflammatory cytokines in serum and brain homogenates of malaria-infected mice in a dose-dependent manner when compared to the vehicle-treated infected mice. Cerebral malaria is the most severe form of infection with Plasmodium falciparum characterized by a highly inflammatory response (IFN-γ, IL-1β, TNF-α, iNOS, and IL-6), which contributes to severity of the disease (Angulo and Fresno, 2002). There are several reports concluding that standard antimalarial drugs (chloroquine and artemisinin) and other plant-derived molecules exert antimalarial activity by reducing parasitemia and also modulating the pro-inflammatory cytokines (Mohanty et al., 2015; Gupta et al., 2018). Severe acidosis, anemia, hypoxia, and renal and hepatic insufficiencies are associated with BBB dysfunction in malarial pathogenesis (Clark et al., 2004). In this study, the BBB integrity was restored by EA-treated mice as evident by reduced Evans blue extravasation. To further substantiate results related to the beneficial effect of EA treatment in malarial pathogenesis by promoting antioxidant and anti-inflammatory effects, we performed the additional experiments using C6 glial cells. EA significantly reduced the H2O2-induced ROS generation in C6 cells and LPS-induced inflammatory mediators (TNF-α and IL-6) in a dose-dependent manner without any cytotoxic effect. This finding also supports the previous finding which indicated that the neuroprotective role of ellagic acid is due to its antioxidant and anti-inflammatory effects on brain tissue (Wang et al., 2020).
Conclusion
Taken together, the results of this study indicate that ellagic acid is able to alleviate severe malaria pathogenesis by reducing cytokine storms and oxidative stress induced by malaria parasites. It also attributed promising antimalarial activity and afforded to improve the blood glucose and hemoglobin levels in treated mice. These research findings suggested the suitability of ellagic acid as a useful bioflavonoid for further study for the management of severe malaria pathogenesis.
Data Availability Statement
The original contributions presented in the study are included in the article/Supplementary Material, and further inquiries can be directed to the corresponding author.
Ethics Statement
The animal study was reviewed and approved, and the animal experiments were carried out as per the approved protocol by the Institutional Animal Ethics Committee (IAEC), followed by the Committee for the Purpose of Control and Supervision of Experimental Animals (CPCSEA), government of India (Registration No: 400/01/AB/ CPCSEA).
Author Contributions
The authors listed in this article have contributed to perform the antimalarial, oxidative stress-, inflammation-related parameters of tissue and serum from the experimental mice, as well as to the analysis of data and preparation of the manuscript.
Funding
The study was financially supported by the Council of Scientific and Industrial Research (CSIR), New Delhi, under project HCP-0019. AG, an author is grateful to the University Grants Commission (UGC), New Delhi, India, for providing the fellowship. The authors are grateful to the director, CSIR-Central Institute of Medicinal and Aromatic Plants, Lucknow, India, for providing essential research facilities and support.
Conflict of Interest
The authors declare that the research was conducted in the absence of any commercial or financial relationships that could be construed as a potential conflict of interest.
Publisher’s Note
All claims expressed in this article are solely those of the authors and do not necessarily represent those of their affiliated organizations, or those of the publisher, the editors, and the reviewers. Any product that may be evaluated in this article, or claim that may be made by its manufacturer, is not guaranteed or endorsed by the publisher.
Supplementary Material
The Supplementary Material for this article can be found online at: https://www.frontiersin.org/articles/10.3389/fphar.2021.777400/full#supplementary-material
Abbreviations
BSA, bovine serum albumin; CMC, carboxymethylcellulose; DMEM, Dulbecco’s modified Eagle medium; DMSO, dimethylsulfoxide; DCFH-DA, 2′,7′-dichlorofluorescein diacetate; DCF, dichlorofluorescein; EA, ellagic acid; ELISA, enzyme-linked immunosorbent assay; FBS, fetal bovine serum; H2O2, hydrogen peroxide; LPS, lipopolysaccharide; MTT, (3-(4,5-dimethylthiazol-2-yl)-2,5-diphenyltetrazolium); MPO, myeloperoxidase; MDA, malondialdehyde; PBS, phosphate-buffered saline; ROS, reactive oxygen species; SOD, superoxide dismutase; TBARS, thiobarbituric acid reactive substances; TBA, thiobarbituric acid; TMB, tetramethylbenzidine.
References
Ahangari, R., Khezri, S., Jahedsani, A., Bakhshii, S., and Salimi, A. (2020). Ellagic Acid Alleviates Clozapine-induced O-xidative S-tress and M-itochondrial D-ysfunction in C-ardiomyocytes. Drug Chem. Toxicol., 1–9. doi:10.1080/01480545.2020.1850758
Amiri, M., Nourian, A., Khoshkam, M., and Ramazani, A. (2018). Apigenin Inhibits Growth of the Plasmodium Berghei and Disrupts Some Metabolic Pathways in Mice. Phytother Res. 32, 1795–1802. doi:10.1002/ptr.6113
Angulo, I., and Fresno, M. (2002). Cytokines in the Pathogenesis of and protection against Malaria. Clin. Diagn. Lab. Immunol. 9, 1145–1152. doi:10.1128/cdli.9.6.1145-1152.2002
Baptista, F. G., Pamplona, A., Pena, A. C., Mota, M. M., Pied, S., and Vigário, A. M. (2010). Accumulation of Plasmodium Berghei-Infected Red Blood Cells in the Brain Is Crucial for the Development of Cerebral Malaria in Mice. Infect. Immun. 78, 4033–4039. doi:10.1128/IAI.00079-10
Becker, K., Tilley, L., Vennerstrom, J. L., Roberts, D., Rogerson, S., and Ginsburg, H. (2004). Oxidative Stress in Malaria Parasite-Infected Erythrocytes: Host-Parasite Interactions. Int. J. Parasitol. 34, 163–189. doi:10.1016/j.ijpara.2003.09.011
Boeuf, P. S., Loizon, S., Awandare, G. A., Tetteh, J. K., Addae, M. M., Adjei, G. O., et al. (2012). Insights into Deregulated TNF and IL-10 Production in Malaria: Implications for Understanding Severe Malarial Anaemia. Malar. J. 11, 253. doi:10.1186/1475-2875-11-253
Ceci, C., Graziani, G., Faraoni, I., and Cacciotti, I. (2020). Strategies to Improve Ellagic Acid Bioavailability: From Natural or Semisynthetic Derivatives to Nanotechnological Approaches Based on Innovative Carriers. Nanotechnology 31, 382001. doi:10.1088/1361-6528/ab912c
Clark, I. A., Alleva, L. M., Budd, A. C., and Cowden, W. B. (2008). Understanding the Role of Inflammatory Cytokines in Malaria and Related Diseases. Trav. Med Infect Dis 6 (1-2), 67–81. doi:10.1016/j.tmaid.2007.07.002
Clark, I. A., Alleva, L. M., Mills, A. C., and Cowden, W. B. (2004). Pathogenesis of Malaria and Clinically Similar Conditions. Clin. Microbiol. Rev. 17, 509–contents. doi:10.1128/CMR.17.3.509-539.2004
Del Maestro, R., and McDonald, W. (1985), Oxidative Enzymes in Tissue Homogenates. Handbook of Methods for Oxygen Radical Research, 291–296.
Draper, H. H., and Hadley, M. (1990). Malondialdehyde Determination as index of Lipid Peroxidation. Methods Enzymol. 186, 421–431. Elsevier. doi:10.1016/0076-6879(90)86135-i
Driver, A. S., Kodavanti, P. R., and Mundy, W. R. (2000). Age-related Changes in Reactive Oxygen Species Production in Rat Brain Homogenates. Neurotoxicol Teratol 22, 175–181. doi:10.1016/s0892-0362(99)00069-0
Evtyugin, D. D., Magina, S., and Evtuguin, D. V. (2020). Recent Advances in the Production and Applications of Ellagic Acid and its Derivatives. A Review. A ReviewMolecules 25, 2745. doi:10.3390/molecules25122745
Gupta, A. C., Mohanty, S., Saxena, A., Maurya, A. K., and Bawankule, D. U. (2018). Plumbagin, a Vitamin K3 Analogue Ameliorate Malaria Pathogenesis by Inhibiting Oxidative Stress and Inflammation. Inflammopharmacology 26, 983–991. doi:10.1007/s10787-018-0465-1
Hunt, N. H., and Grau, G. E. (2003). Cytokines: Accelerators and Brakes in the Pathogenesis of Cerebral Malaria. Trends Immunol. 24 (9), 491–499. doi:10.1016/s1471-4906(03)00229-1
Kalia, S., Walter, N. S., and Bagai, U. (2015). Antimalarial Efficacy of Albizia Lebbeck (Leguminosae) against Plasmodium Falciparum In Vitro & P. Berghei In Vivo. Indian J. Med. Res. 142 Suppl, S101–S107. doi:10.4103/0971-5916.176635
Kumar, S., Kumari, R., and Pandey, R. (2015). New Insight-Guided Approaches to Detect, Cure, Prevent and Eliminate Malaria. Protoplasma 252, 717–753. doi:10.1007/s00709-014-0697-x
Mohanty, S., Maurya, A. K., Jyotshna, A., Saxena, A., Shanker, K., Pal, A., et al. (2015). Flavonoids Rich Fraction of Citrus Limetta Fruit Peels Reduces Proinflammatory Cytokine Production and Attenuates Malaria Pathogenesis. Curr. Pharm. Biotechnol. 16, 544–552. doi:10.2174/138920101606150407114023
Mohanty, S., Srivastava, P., Maurya, A. K., Cheema, H. S., Shanker, K., Dhawan, S., et al. (2013). Antimalarial and Safety Evaluation of Pluchea Lanceolata (DC.) Oliv. & Hiern: In-Vitro and In-Vivo Study. J. Ethnopharmacol 149, 797–802. doi:10.1016/j.jep.2013.08.003
Pabón, A., Carmona, J., Burgos, L. C., and Blair, S. (2003). Oxidative Stress in Patients with Non-complicated Malaria. Clin. Biochem. 36, 71–78. doi:10.1016/s0009-9120(02)00423-x
Puttappa, N., Kumar, R. S., and Yamjala, K. (2017). Artesunate-quercetin/luteolin Dual Drug Nanofacilitated Synergistic Treatment for Malaria: A Plausible Approach to Overcome Artemisinin Combination Therapy Resistance. Med. Hypotheses 109, 176–180. doi:10.1016/j.mehy.2017.10.016
Saxena, A., Yadav, D., Mohanty, S., Cheema, H. S., Gupta, M. M., Darokar, M. P., et al. (2016). Diarylheptanoids Rich Fraction of Alnus Nepalensis Attenuates Malaria Pathogenesis: In-Vitro and In-Vivo Study. Phytother Res. 30, 940–948. doi:10.1002/ptr.5596
Saxena, A., Upadhyay, H. C., Cheema, H. S., Srivastava, S. K., Darokar, M. P., and Bawankule, D. U. (2018). Antimalarial Activity of Phytol Derivatives: In Vitro and In Vivo Study. Med. Chem. Res. 27, 1345–1354. doi:10.1007/s00044-017-2132-2
Schofield, L., and Grau, G. E. (2005). Immunological Processes in Malaria Pathogenesis. Nat. Rev. Immunol. 5 (9), 722–735. doi:10.1038/nri1686
Shakeri, A., Zirak, M. R., and Sahebkar, A. (2018). Ellagic Acid: A Logical lead for Drug Development? Curr. Pharm. Des. 24, 106–122. doi:10.2174/1381612823666171115094557
Sharma, S., Chattopadhyay, S. K., Yadav, D. K., Khan, F., Mohanty, S., Maurya, A., et al. (2012). QSAR, Docking and In Vitro Studies for Anti-inflammatory Activity of Cleomiscosin A Methyl Ether Derivatives. Eur. J. Pharm. Sci. 47, 952–964. doi:10.1016/j.ejps.2012.09.008
Singh, D. K., Cheema, H. S., Saxena, A., Jyotshana, S., Singh, S., Darokar, M. P., et al. (2017). Fraxetin and Ethyl Acetate Extract from Lawsonia Inermis L. Ameliorate Oxidative Stress in P. Berghei Infected Mice by Augmenting Antioxidant Defence System. Phytomedicine 36, 262–272. doi:10.1016/j.phymed.2017.09.012
Snow, R. W., Guerra, C. A., Noor, A. M., Myint, H. Y., and Hay, S. I. (2005). The Global Distribution of Clinical Episodes of Plasmodium Falciparum Malaria. Nature 434, 214–217. doi:10.1038/nature03342
Stanisic, D. I., and Good, M. F. (2015). Whole Organism Blood Stage Vaccines against Malaria. Vaccine 33, 7469–7475. doi:10.1016/j.vaccine.2015.09.057
Suzuki, K. T., Tanaka, Y., and Kawamura, R. (1983). Properties of Metallothionein Induced by Zinc, Copper and Cadmium in the Frog, Xenopus laevis. Comp. Biochem. Physiol. C Comp. Pharmacol. Toxicol. 75, 33–37. doi:10.1016/0742-8413(83)90007-5
Togbe, D., Schofield, L., Grau, G. E., Schnyder, B., Boissay, V., Charron, S., et al. (2007). Murine Cerebral Malaria Development Is Independent of Toll-like Receptor Signaling. Am. J. Pathol. 170 (5), 1640–1648. doi:10.2353/ajpath.2007.060889
Vattem, D. A., Ghaedian, R., and Shetty, K. (2005). Enhancing Health Benefits of Berries through Phenolic Antioxidant Enrichment: Focus on cranberry. Asia Pac. J. Clin. Nutr. 14, 120–130.
Vinoth, K. J., Manikandan, J., Sethu, S., Balakrishnan, L., Heng, A., Lu, K., et al. (2015). Differential Resistance of Human Embryonic Stem Cells and Somatic Cell Types to Hydrogen Peroxide-Induced Genotoxicity May Be Dependent on Innate Basal Intracellular ROS Levels. Folia Histochem. Cytobiol 53, 169–174. doi:10.5603/FHC.a2015.0016
Wang, W., Yang, L., Liu, T., Wang, J., Wen, A., and Ding, Y. (2020). Ellagic Acid Protects Mice against Sleep Deprivation-Induced Memory Impairment and Anxiety by Inhibiting TLR4 and Activating Nrf2. Aging (Albany NY) 12, 10457–10472. doi:10.18632/aging.103270
Keywords: ellagic acid, malaria, inflammation, cytokine, storms, oxidative stress, mice
Citation: Mohanty S, Gupta AC, Maurya AK, Shanker K, Pal A and Bawankule DU (2021) Ameliorative Effects of Dietary Ellagic Acid Against Severe Malaria Pathogenesis by Reducing Cytokine Storms and Oxidative Stress. Front. Pharmacol. 12:777400. doi: 10.3389/fphar.2021.777400
Received: 15 September 2021; Accepted: 11 November 2021;
Published: 09 December 2021.
Edited by:
Massimo Lucarini, Council for Agricultural Research and Economics, ItalyReviewed by:
Mavondo Greanious Alfred, National University of Science and Technology, ZimbabweEdson Roberto Silva, University of São Paulo, Brazil
Copyright © 2021 Mohanty, Gupta, Maurya, Shanker, Pal and Bawankule. This is an open-access article distributed under the terms of the Creative Commons Attribution License (CC BY). The use, distribution or reproduction in other forums is permitted, provided the original author(s) and the copyright owner(s) are credited and that the original publication in this journal is cited, in accordance with accepted academic practice. No use, distribution or reproduction is permitted which does not comply with these terms.
*Correspondence: Dnyaneshwar Umrao Bawankule, du.bawankule@cimap.res.in
†These authors have contributed equally to this work