- State Key Laboratory of Organ Failure Research, Guangdong Provincial Key Laboratory of Renal Failure Research, National Clinical Research Center for Kidney Disease, Guangdong Provincial Institute of Nephrology, Division of Nephrology, Nanfang Hospital, Southern Medical University, Guangzhou, China
The patients with kidney diseases are increasing rapidly all over the world. With the rich abundance of mitochondria, kidney is an organ with a high consumption of energy. Hence, renal failure is highly correlated with the breakup of mitochondrial homeostasis. However, the potential drugs targeting mitochondrial dysfunction are still in mystery. The natural products have the superiorities to explore the potential drugs regulating energy metabolism. However, their roles in targeting mitochondrial dysfunction in kidney diseases have not been extensively reviewed. Herein, we reviewed a series of natural products targeting mitochondrial oxidative stress, mitochondrial biogenesis, mitophagy, and mitochondrial dynamics. We found lots of them with great medicinal values in kidney disease. Our review provides a wide prospect for seeking the effective drugs targeting kidney diseases.
1 Introduction
Kidney diseases, including acute kidney injury (AKI) and chronic kidney diseases (CKD), are becoming a public health problem. The prevalence of kidney diseases is over 10% worldwide (Eckardt et al., 2013; Glassock et al., 2017; Huang et al., 2021). Notably, a large majority of CKD patients would inevitably progress into end-stage renal diseases (ESRD), a diseased state depending on dialysis or transplantation to survive. These treatments result into the heavy financial and medical burdens (Levey and Coresh, 2012). However, even on dialysis or transplantation, kidney diseases are still facing the high mortality rates (Vijayan, 2021).
With a massive mass of mitochondria (Forbes and Thorburn, 2018; Zhang et al., 2021a), the prominent organelle for adenosine triphosphate (ATP) production (Forbes and Thorburn, 2018), kidney is the second highest organ consuming oxygen at resting state. Tubular epithelial cells (TECs) are the main constituent in kidney parenchyma. They, on huge demand of ATP supply, undertake active reabsorption and secretion (Forbes and Thorburn, 2018). Other renal cells, like podocytes, also require a high supply of energy. In physiological condition in kidney, the most proportion of ATP is from oxidative phosphorylation (OXPHOS) in mitochondrial matrix. Mitochondrial homeostasis is vital to maintain normal kidney function (Forbes and Thorburn, 2018), as mitochondrial dysfunction not only contributes to energy deficiency, but also leads to disruption of cellular homeostasis and renal function (Bhargava and Schnellmann, 2017). The main phenomenon of mitochondrial dysfunction is the excessive generation of reactive oxygen species (ROS), which plays a key role in cell injury and kidney diseases (Linkermann et al., 2014; Dan Dunn et al., 2015; Kang et al., 2015; Pickles et al., 2018; Zhao et al., 2021b; Liang et al., 2021). As reported, mitochondrial dysfunction contributes to nearly all kinds of kidney diseases, such as diabetic kidney diseases (DKD) (Tan et al., 2021), inherited renal diseases (Emma et al., 2016), podocytopathy (Arif et al., 2019), IgA nephritis (Yu et al., 2019), hypertensive nephritis (Eirin et al., 2015) and so on.
Natural products are defined as the mixture and monomer from natural sources of animals, plants and microorganisms. They have exhibited a wide variety of biological activities for thousands of years, including targeting mitochondrial dysfunction (Katz and Baltz, 2016; Mu et al., 2020; Liang et al., 2021). Large reports have shown natural products have strong therapeutic values in promoting mitochondrial biogenetics and energetics, reducing mitochondrial ROS, enhancing mitophagy, and regulating mitochondrial dynamics (Mu et al., 2020; Liang et al., 2021). Therefore, natural products may exert beneficial effects for protecting kidney. However, their therapeutic values in kidney diseases have not been extensively reviewed. In our review, we introduced natural products targeting mitochondrial dysfunction in kidney diseases in detailed comments. Our review provides the important potential strategies for clinical treatments.
2 Mitochondrial dysfunctions in kidney diseases
2.1 Mitochondrial homeostasis imbalance
Mitochondrial quality control (MQC) is an elaborate mechanism to maintain homeostasis, ensuring the enough numbers of functional mitochondria (Pickles et al., 2018; Huang et al., 2022b). Involving mitochondrial biogenesis, dynamics, and mitophagy, mitochondrial homeostasis is intimately correlated with normal kidney function (Rahman et al., 2022) (Figure 1). Large reports have shown mitochondrial dysfunction is closely related to the progression of kidney diseases (Galvan et al., 2017). In both AKI and CKD models, mitochondrial biogenesis is greatly impaired, leading to the insufficient supply of ATP and cell injury in various cell types, particularly in TECs in kidney, (Galvan et al., 2017; Zhang et al., 2021a).
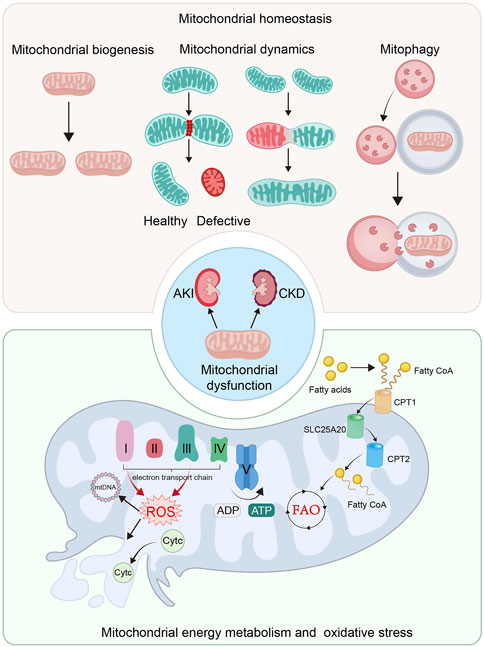
FIGURE 1. Mitochondrial dysfunction plays an important role in the pathological process of AKI and CKD. The imbalance of mitochondrial homeostasis, defective mitochondrial energy metabolism and mitochondrial oxidative stress are the crucial causes of mitochondrial dysfunction. The imbalance of mitochondrial homeostasis includes the impaired mitochondrial biogenesis, imbalance of mitochondrial dynamics and insufficient mitophgy. Mitochondria is the main organelle for energy production. Defective mitochondrial energy metabolism, including FAO and OXPHOS, plays a significant role in mitochondrial dysfunction. Mitochondrial energy metabolism not only induces ATP production insufficiency, but also in ROS production and mitochondrial impairment.
Mitochondrial structure and morphology plays a key role in energy production. They are tightly controlled by mitochondrial dynamics and mitophagy (Rahman et al., 2022). With a highly dynamic action, mitochondria frequently remodel themselves through fusion and fission cycles (Forbes and Thorburn, 2018; Mu et al., 2020; Liang et al., 2021). Mitochondrial fusion enables defective mitochondria mixing with healthy ones to repair (Forbes and Thorburn, 2018; Mu et al., 2020; Liang et al., 2021), while fission can remove their defective contents to keep healthy mitochondrial function (Aparicio-Trejo et al., 2018; Forbes and Thorburn, 2018; Mu et al., 2020; Liang et al., 2021). Furthermore, after losing membrane potentials, defective mitochondria would be degraded by mitophagy (Forbes and Thorburn, 2018; Jin et al., 2021). When this balance is disrupted, mitochondrial dysfunction occurs, which plays a significant role in the progression of kidney diseases (Galvan et al., 2017; Zhang et al., 2021a).
2.2 Defective energy metabolism and increased oxidative stress in mitochondria
To execute energy production and substance metabolism are the main functions of mitochondria (Spinelli and Haigis, 2018; Liang et al., 2021). The production of ATP mainly depends on aerobic respiration (Bhargava and Schnellmann, 2017). The pyruvate is derived from the process of glycolysis in cytoplasm, and then is converted into acetyl-coenzyme A (acetyl-CoA) to participate tricarboxylic acid (TCA) cycle in mitochondrial matrix. Furthermore, TCA cycle releases NAD+ to ultimately initiate OXPHOS. The OXPHOS system comprises complex I to V. Electrons are delivered by complex I and II in inner mitochondrial membrane (IMM), and then transmit to complex IV and are accepted by oxygen. When electrons travel through complex I, III, and IV, protons are actively flowing into intermembrane space. Complex V, the ATP synthase, generates ATP from ADP through the electrochemical gradient of protons. When this process is damaged, some electrons would leak and lead to ROS production (Bhargava and Schnellmann, 2017; Mani et al., 2020; Liang et al., 2021). Hence, mitochondria are also the main source of cellular ROS (Sinha et al., 2013). At physiological concentrations, ROS is important to cellular division, but excessive ROS leads to stressed damages to protein, DNA and lipid (Mani et al., 2020). Of note, mitochondria not only are the main generator of ROS, but always are the primary targets of ROS. ROS could impair mitochondrial DNA, ETC., and damage mitochondrial membrane, further leading to more production of ROS (Szczepanowska et al., 2012; Mani et al., 2020). Excessive ROS could lead to the depolarization of IMM, the opening of mitochondrial permeability transition pore (mPTP), and the leakage of cytochrome c (Cytc) into the cytoplasm. Furthermore, caspase signaling pathway would be activated to induce cell apoptosis and the development of kidney diseases (Sinha et al., 2013; Galvan et al., 2017; Mani et al., 2020; Zhang et al., 2021a; Rahman et al., 2022).
As a major cell type in kidney, proximal tubular cell produce ATP via fatty acid β-oxidation (FAO) in mitochondria (Bhargava and Schnellmann, 2017). The fatty acids are transferred from cytoplasm into mitochondrial matrix by carnitine O-palmitoyltransferase 1 (CPT1), carnitine–acylcarnitine translocase (SLC25A20) and carnitine O-palmitoyltransferase 2 (CPT2) to be metabolized for energy production (Houten and Wanders, 2010; Bhargava and Schnellmann, 2017; Spinelli and Haigis, 2018). Peroxisome proliferator–activated receptor α (PPARα), a crucial transcriptional factor, regulates FAO via controlling the transcription and translation of FAO-related enzymes (Chung et al., 2018; Barone et al., 2019). The impaired mitochondrial FAO leads to insufficient supply of ATP, but also results in lipotoxicity, which together contribute to renal fibrosis (Lv et al., 2019; Miguel et al., 2021). In both AKI and CKD models, the FAO failure and the following lipid accumulation commonly occur in renal TECs (Rong et al., 2021; Xu et al., 2022), which could further trigger the impairment of mitochondrial structure and function (Huang et al., 2022b).
3 Natural products for regulating mitochondrial function
3.1 Enhancing mitochondrial biogenesis and energetics
It is widely found that impaired mitochondrial biogenesis and energetics contribute to the pathogenesis of kidney diseases (Weinberg, 2011; Bhargava and Schnellmann, 2017). Mitochondrial biogenesis involves the growth and binary fission of pre-existing mitochondria (Jornayvaz and Shulman, 2010) to supply functional mitochondria for cellular requirement (Figure 2).
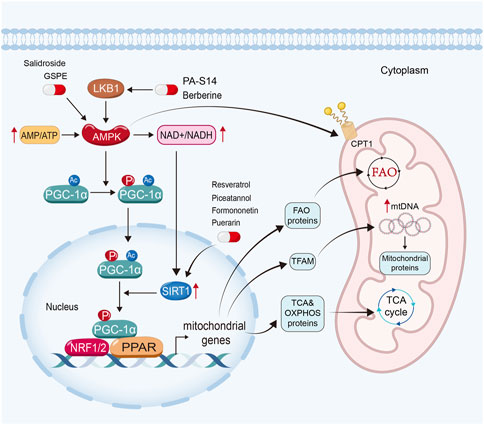
FIGURE 2. Enhancing mitochondrial biogenesis and energetics is a crucial therapeutic strategy for natural products to restore mitochondrial function in kidney diseases. PA-S14 and berberine promote mitochondrial biogenesis and energetics by activating LKB1, while salidroside and GSPE induce AMPK. Moreover, resveratrol, piceatannol, formononetin and puerarin exert their mitochondrial protective function via the activation of Sirtuin-1.
AMP-activated protein kinase (AMPK), Sirtuin-1, and peroxisome proliferator-activated receptor-γ (PPARγ) coactivator-1α (PGC-1α) in particular, play important roles in mitochondrial biogenesis (Jornayvaz and Shulman, 2010; Liang et al., 2021). In eukaryotic cells, when there is a deficiency of functional mitochondria, the AMP/ATP ratio increases to activate AMPK to induce the expression and phosphorylated activation of PGC-1α. Moreover, AMPK can enhance Sirtuin-1, the NAD+-dependent deacetylases family member owning deacetylating activity (Wang et al., 2019), through increasing cellular nicotinamide adenine dinucleotide (NAD+) levels. Sirtuin-1 is the main deacetylase of PGC-1α (Rodgers et al., 2005; Gerhart-Hines et al., 2007). After deacetylation and phosphorylation, PGC-1α translocates from the cytoplasm into the nucleus. As a co-activator, PGC-1α binds with several transcription factors (Galvan et al., 2017) and activates them. As reported, PGC-1α could activate nuclear respiratory factors (NRF) 1 and NRF2, estrogen-related receptors (ERRs), and PPARs (Jornayvaz and Shulman, 2010).
PGC-1α could dock on NRF 1 and 2 to together drive the transcription and translation of the genes involving TCA cycle and OXPHOS systems, and then induce mitochondrial biogenesis. Furthermore, it also induces the expression of mitochondrial transcription factor A (TFAM), a controller in replication and transcription of mitochondrial DNA, to further drive mitochondrial biogenesis (Jornayvaz and Shulman, 2010; Liang et al., 2021). PGC-1α also co-activates ERRs to enhance mitochondrial biogenesis and glucose utilization (Jornayvaz and Shulman, 2010; Deblois et al., 2013; Singh et al., 2018). Moreover, PGC-1α also interacts with PPARα, the master regulator of FAO to upregulate the expression of FAO-related genes, such as CPT1, SLC25A20 and CPT2 (Djouadi et al., 2005; Tachibana et al., 2009; Jornayvaz and Shulman, 2010; Galvan et al., 2017; Suzuki et al., 2021). Furthermore, AMPK can also promote mitochondrial FAO to enhance the activity of CPT1 by PGC-1α-independent pathway (Herzig and Shaw, 2018).
3.1.1 AMPK signaling pathway activators
3.1.1.1 Piericidin analogue S14 (PA-S14)
Many natural products can stimulate mitochondrial biogenesis and energetics via AMPK signaling pathway. PA-S14, a natural product extracted from the marine-derived Streptomyces reported by our group, is an analogue of Piericidin A, a mitochondrial complex I inhibitor. PA-S14 can activate liver kinase B1 (LKB1), the key upstream kinase for AMPK activation, with no inhibitory effects on mitochondrial complex I. We found that PA-S14 promotes mitochondrial biogenesis via LKB1-AMPK-PGC-1α signaling pathway, which protects against renal TECs cell senescence, renal fibrosis in various CKD models, such as unilateral ureteral obstruction (UUO), unilateral ischemia-reperfusion injury (UIRI), adriamycin nephropathy and 5/6 nephrectomized model (Liu et al., 2022a) (Table 1).
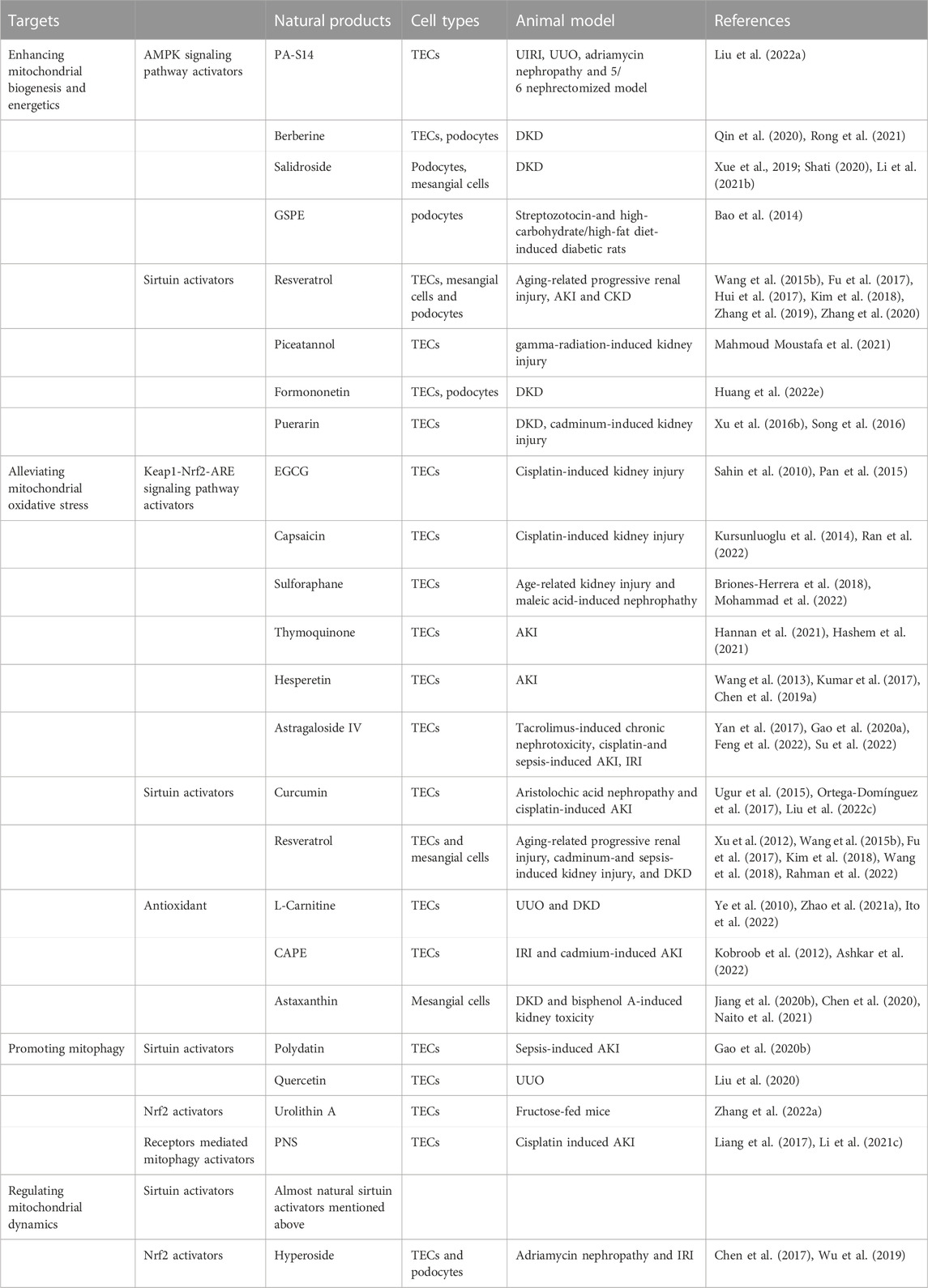
TABLE 1. Therapeutic mechanisms and targets of natural products targeting mitochondria in kidney diseases.
3.1.1.2 Berberine
Berberine, found in Coptidis rhizoma and Cortex Phellodendri, can activate AMPK via LKB1-dependent or independent pathway (Joshi et al., 2019). Berberine can alleviate mitochondrial dysfunction in DKD mice and palmitic acid-induced podocyte injury via increasing AMPK and PGC-1α (Qin et al., 2020; Li et al., 2021b). Moreover, berberine can also improve FAO and rescue mitochondrial function by inducing CPT1 and CPT2 in podocytes, which further attenuates lipid accumulation and ROS overproduction in DKD (Qin et al., 2020). Berberine treatment also decreases the excretion of urinary microalbumin (Qin et al., 2020). Besides podocytes, berberine can also upregulate the expression of CPT1 and PPARα in renal TECs, further improving mitochondrial FAO and reducing lipid accumulation in DKD mice (Rong et al., 2021). In one clinical trial, berberine decreases albumin-to-creatine ratio and improves renal hemodynamics in hypertensive patients with type 2 diabetes mellitus (Dai et al., 2015).
3.1.1.3 Salidroside
Salidroside, a phenolic glucoside found in Rhodiola rosea (Liang et al., 2021), exhibits versatile pharmacologic properties, such as anti-apoptosis, anti-inflammation, and improving mitochondrial function (Wang et al., 2022). Salidroside also shows great protective effects on kidney function maintaining (Huang et al., 2019; Fan et al., 2022). The underlying mechanism lies on its activity to stimulate AMPK-Sirtuin-1-PGC-1α signaling (Xue et al., 2019; Shati, 2020; Li et al., 2021b) and promotes mitochondrial biogenesis (Xue et al., 2019).
3.1.1.4 Grape seed proanthocyanidin extract (GSPE)
GSPE, a natural bioactive compound from plant, exhibits great therapeutic properties through inhibiting oxidative stress and promoting mitochondrial biogenesis (Ashkar et al., 2022). Researches show in streptozotocin-and high-carbohydrate/high-fat diet-induced diabetic rats, GSPE treatment is able to enhance mitochondrial biogenesis, and ameliorate podocyte injury and apoptosis via AMPK-Sirtuin-1-PGC-1α-TFAM signaling pathway (Bao et al., 2014).
3.1.2 Sirtuin activators
3.1.2.1 Resveratrol
Resveratrol, a natural compound extracted from the berries of Vaccinium species, can activate sirtuin-1, sirtuin-3, and sirtuin-5 (Dai et al., 2018). Clinical studies have shown that resveratrol exhibits therapeutic effects on several diseases, including cardiovascular disease and neurological diseases (Xia et al., 2017; Singh et al., 2019). In kidney diseases, resveratrol could safely improve peritoneal ultrafiltration activity and ameliorate renal dysfunction (Lin et al., 2016; Singh et al., 2019). The mechanisms are because resveratrol can activate Sirtuin-1 to further promote activation of PGC-1α and mitochondrial biogenesis. Resveratrol can also regulate mitochondrial biogenesis via Sirtuin-1-independent pathway, such as resveratrol can directly activate AMPK (Ungvari et al., 2011; Joshi et al., 2019). The protective effects of resveratrol are shown in various models of kidney diseases, including aging-related progressive renal injury, AKI and CKD (Wang et al., 2015b; Fu et al., 2017; Hui et al., 2017; Kim et al., 2018; Zhang et al., 2019; Zhang et al., 2020). To induce Sirtuin-1-PGC-1α-PPARα pathway, resveratrol can also enhance mitochondrial FAO function in renal cells (Kim et al., 2013).
3.1.2.2 Piceatannol
Piceatannol, a natural phytochemical from various fruits and vegetables, is an activator of sirtuin-1. Piceatannol exhibits versatile pharmacologic therapeutic potencies in cancer, cardiovascular diseases and atherosclerosis, as well as in kidney diseases (Tovar-Palacio et al., 2022). Piceatannol can ameliorate necrosis and apoptosis in renal TECs through activating Sirtuin-1-PGC-1α pathway (Mahmoud Moustafa et al., 2021).
3.1.2.3 Formononetin
Formononetin, the major phytochemical of the Astragalus membranaceus (Tovar-Palacio et al., 2022), can also upregulate and activate Sirtuin-1 (Rasbach and Schnellmann, 2008). Formononetin is famous for its therapeutic effects on cardiac diseases and hyperlipidemia (Tovar-Palacio et al., 2022). Reports have also demonstrated its protective effects in kidney. Formononetin can alleviate nephrotoxicity in methotrexate-, cisplatin-and gentamicin-induced kidney injury and DKD (Aladaileh et al., 2019; Oza and Kulkarni, 2019; Hao et al., 2021; Althunibat et al., 2022). Formononetin promotes mitochondrial biogenesis via Sirtuin-1-PGC-1α pathway, which plays an important role in kidney protection (Rasbach and Schnellmann, 2008; Huang et al., 2022e). Formononetin treatment could attenuate tubular cell apoptosis and podocyte injury, then further to decrease urinary albumin excretion and ameliorate the progression of DKD (Huang et al., 2022e).
3.1.2.4 Puerarin
Puerarin, the bioactive compound extracted from radix puerariae, exhibits beneficial therapeutic effects on cardiovascular diseases, cancer, diabetes and osteonecrosis. Puerarin has wide pharmacological properties, especially the activation of Sirtuin-1 (Zhou et al., 2014; Tovar-Palacio et al., 2022). Puerarin can ameliorate mitochondrial damage to inhibit cadminum-induced TEC apoptosis in kidney (Song et al., 2016). Moreover, puerarin upregulates Sirtuin-1 and PGC-1α expression in DKD, to further ameliorate matrix expansion, glomerular collapse and tubular dilatation, and rescue kidney function (Xu et al., 2016b). Puerarin can also activate LKB1 and AMPK to further promote mitochondrial biogenesis in kidney (Song et al., 2017; Li et al., 2020). In clinical trials, puerarin could also significantly decrease the urinary albumin excretion rate in patients with DKD (Wang et al., 2015a; Shao et al., 2021).
3.2 Alleviating mitochondrial oxidative stress
Mitochondrial respiration is the main source of cellular ATP. The impairment and uncoupling of ETC leads to the excessive generation of ROS, which further trigger a series of cellular injuries. As the primary source of cellular ROS (Sinha et al., 2013), mitochondria also own the adequate anti-oxidant system. Superoxide dismutase (SOD) plays a major role in eliminating mitochondrial ROS. SOD could convert ROS into hydrogen dioxide, which is then deactivated by catalase or glutathione (GSH). GSH could also be converted to oxidized GSH (GSSG) via various glutathione peroxidases (GPX) in this process (Balaban et al., 2005) (Figure 3).
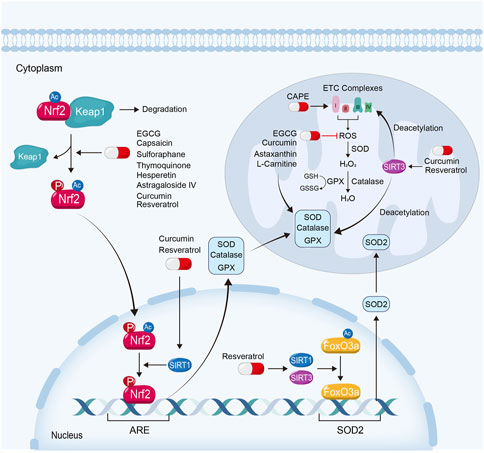
FIGURE 3. Attenuating mitochondrial oxidative stress is also an important way for natural products to ameliorate kidney injury. EGCG, capsaicin, sulforaphane, thymoquinone, hesperetin and astragaloside IV, exhibit their renoprotective effects via the activation of Keap1-Nrf2-ARE signaling pathway. Curcumin and resveratrol ameliorate mitochondrial dysfunction in kidney diseases not only depending on Keap1-Nrf2-ARE signaling pathway, but also on the activation of Sirtuin-1 and Sirtuin-3. Moreover, some natural products protect against mitochondrial oxidative stress by scavenging ROS, reducing mitochondrial ROS production or restoring anti-oxidative enzyme, such as L-Carnitine, CAPE, EGCG, curcumin and astaxanthin.
Nuclear factor-erythroid-derived 2-like 2 (NFE2L2, also named Nrf2) is a transcription factor which plays a crucial role in resistance to oxidative stress (Ma, 2013). Under basal conditions, Nrf2 is degraded through kelch-like erythroid cell-derived protein with CNC homology-associated protein 1 (Keap1)-dependent ubiquitination and proteasomal degradation. When the binding of Keap1 and Nrf2 is disrupted, Nrf2 translocates into the nucleus, where it binds with a common DNA sequence of antioxidant response element (ARE). ARE involves various genes of anti-oxidative stress function, such as SOD, GPX and catalase (Ma, 2013; Dinkova-Kostova and Abramov, 2015; Ryoo and Kwak, 2018; Wang et al., 2020a). Nrf2 can be phosphorylated by AMPK and deacetylated by Sirtuin-1, and reversely enhanced the transcriptional activity by them (Gureev et al., 2019; Xu et al., 2021). Of note, to reduce the generation of mitochondrial ROS or to increase the scavenge of ROS similarly attenuate mitochondrial oxidative stress and kidney injury (Li et al., 2021b).
3.2.1 Keap1-Nrf2-ARE signaling pathway activators
3.2.1.1 Epigallocatechin 3-Gallate (EGCG)
EGCG, a bioactive constituent extracted from green tea, is widely investigated in various diseases, such as cardiovascular diseases, diabetes and cancer. EGCG exhibits anti-oxidation properties and therapeutic effects against AKI, CKD, kidney stone diseases, glomerulonephritis, lupus nephritis, and renal cell carcinoma (Kanlaya and Thongboonkerd, 2019; Li et al., 2021b). In one clinical trial, EGCG is able to decrease albuminuria and ameliorate DKD (Avila-Carrasco et al., 2021). Studies have shown that EGCG inhibits mitochondrial oxidative stress and ameliorates kidney injury via inhibiting Keap1 and upregulating Nrf2-ARE signaling pathway (Wang et al., 2015c; Granata et al., 2015; Pan et al., 2015; Kanlaya et al., 2016; Sun et al., 2017). There are two mechanisms for EGCG in enhancing mitochondrial antioxidant defense. First, EGCG can reduce mitochondrial ROS, then to further prevent mitochondrial respiratory enzymes injury. Second, EGCG could upregulate Keap1-Nrf2-ARE pathway, to further induce the expression of SOD, GPX, GSH and catalase. Hence, EGCG protects mitochondrial respiratory enzymes and decreases mitochondrial ROS production in cisplatin-induced nephrotoxicity (Sahin et al., 2010; Pan et al., 2015). EGCG treatment also significantly reverses the increased levels of Scr and BUN, and alleviates tubular injury in cisplatin-induced kidney injury (Pan et al., 2015).
3.2.1.2 Capsaicin
Capsaicin, the primary bioactive compound from red chili peppers (Liu et al., 2022d), exhibits therapeutic effects on kidney in various animal models, such as UUO (Liu et al., 2022d), DKD (Ríos-Silva et al., 2018) and nephrotoxicity (Jung et al., 2014). Capsaicin can activate Nrf2-ARE signaling pathway and rescue kidney function in cisplatin-induced kidney injury. Capsaicin treatment enhances the activities of mitochondrial ROS scavenging enzymes and ameliorates mitochondrial swelling and fragmentation, and prevents TECs apoptosis (Kursunluoglu et al., 2014; Ran et al., 2022). In clinical trials, capsaicin also shows to alleviate uremic conditions in patients with CKD (Makhlough et al., 2010; Yeam et al., 2021).
3.2.1.3 Sulforaphane
Sulforaphane, extracted from Brassicae vegetables, can activate Nrf2 signaling pathway and protect against mitochondrial oxidative stress (Briones-Herrera et al., 2018). Sulforaphane shows great renoprotective effects via targeting mitochondrial dysfunction in various kidney diseases. A previous study showed that sulforaphane treatment could increase SOD to decrease mitochondrial damage and preserve mitochondrial microstructure after kidney transplantation (Cekauskas et al., 2013). Sulforaphane can also alleviate age-related mitochondrial dysfunction and kidney injury via inhibiting Keap1 and upregulating Nrf2 (Mohammad et al., 2022). In maleic acid-induced nephropathy, sulforaphane rescues mitochondrial function and alleviates kidney injury. Sulforaphane could also improve mitochondrial electron transport system to alleviate mitochondrial oxidative stress through promoting Nrf2-induced ROS scavenging (Briones-Herrera et al., 2018).
3.2.1.4 Thymoquinone
Thymoquinone, the main bioactive compound from Nigella sativa seed and its oil, exhibits protective effects in various organs. Clinical trials have shown that N. sativa oil can reduce Scr levels and delay the progression of CKD. Moreover, it can also decrease the size of kidney stones (Hannan et al., 2021). Thymoquinone protects against drug-induced kidney injury, ischemia-reperfusion injury (IRI) and CKD via its anti-oxidative, anti-inflammatory and anti-fibrotic properties (Hannan et al., 2021). Thymoquinone can increase the expression of mitochondrial ROS scavenging enzymes and antioxidant molecules, such as GPX, catalase, SOD and GSH, and restore renal mitochondrial viability via Nrf2 in various kidney diseases models (Hannan et al., 2021; Hashem et al., 2021). Thymoquinone is regarded as a mitochondria-targeted antioxidant (Granata et al., 2015). Hilal Alkis et al. found that thymoquinone can alleviate radiation-induced oxidative stress in kidney tissue in rats (Alkis et al., 2021). Another study has shown that thymoquinone attenuates doxorubicin-induced redox imbalance in renal tissue via the upregulation and activation of Nrf2 (Elsherbiny and El-Sherbiny, 2014). Other studies also found that thymoquinone upregulates mitochondrial anti-oxidative ability, rescues mitochondrial function and ameliorates kidney injury through Nrf2 (Dera et al., 2020; Hashem et al., 2021).
3.2.1.5 Hesperetin
Hesperetin, a natural bioactive phytochemical from citrus plants, exhibits wide pharmacological therapeutic effects on cancer, cardiovascular diseases and diabetes (Chen et al., 2019b; Ashkar et al., 2022). Hesperetin also shows renal protective effects by its anti-oxidative properties (Ashkar et al., 2022). Hesperetin can activate Nrf2-ARE signaling pathway via increasing Nrf2 and p-Nrf2 levels in kidney tissue (Chen et al., 2019b). Hesperetin treatment can also significantly rescue kidney function and inhibit tubular cell apoptosis in various AKI models, through increasing mitochondrial ROS scavenging enzymes via Nrf2-ARE signaling pathway (Wang et al., 2013; Kumar et al., 2017; Chen et al., 2019a).
3.2.1.6 Astragaloside IV
A. membranaceus, is a traditional Chinese herbs used in diverse kidney diseases. Astragaloside IV, one of the main bioactive ingredients isolated from A. membranaceus, exhibits significant protective effects in kidney (Gao et al., 2020a). Mitochondrial oxidative defense is one of the main mechanisms of astragaloside IV to restore kidney function. It lies in its effects on increasing Nrf2 protein levels and promoting Nrf2 transcriptional activity (Gao et al., 2020a). Studies have found that astragaloside IV can also induce the expression of GPX, SOD, as well as catalase, and ameliorate mitochondrial oxidative stress via Keap1-Nrf2-ARE signaling pathway in AKI and CKD models (Yan et al., 2017; Gao et al., 2020a; Feng et al., 2022; Su et al., 2022).
3.2.2 Sirtuin activators
Sirtuin activators not only play important roles in mitochondrial biogenesis, but also in mitochondrial oxidation defense. Sirtuin-1 can promote Nrf2 deacetylation and enhance its transcriptional activity, further protecting against mitochondrial oxidative stress (Xu et al., 2021). Sirtuin-3, a nuclear protein translocated to the mitochondrial matrix upon oxidative stress (Klotz et al., 2015), plays an important role in mitochondrial bioenergetics and defense to oxidative stress. On one hand, Sirtuin-3 is able to deacetylate the complexes of the ETC and increase their activities to further reduce the production of ROS. On the other hand, Sirtuin-3 can deacetylate mitochondrial proteins involved in the elimination of ROS to reduce mitochondrial ROS levels (Clark and Parikh, 2021). Moreover, both Sirtuin-3 and Sirtuin-1 can deacetylate and activate forkhead box class O 3a (FoxO3a), the ubiquitously expressed transcription factors, and then upregulating the genes involved in mitochondrial oxidative defense, such as SOD2 (Jacobs et al., 2008; Tseng et al., 2013; Klotz et al., 2015; Liu et al., 2018; Xie et al., 2020; Li et al., 2021a; Ni et al., 2021; Rahman et al., 2022). Sirtuin-3 can also rescue kidney function by relieving mitochondrial oxidative stress (Fu et al., 2017).
3.2.2.1 Curcumin
Curcumin, the bioactive compound extracted from turmeric, shows the potent therapeutic effects in various diseases including renal IRI, shock and myocardial ischemia with its antioxidant properties (Trujillo et al., 2013). Curcumin is a powerful bifunctional antioxidant. On one hand, it exhibits renoprotective effects and improves mitochondrial function through scavenging mitochondrial ROS directly (Trujillo et al., 2013). On the other hand, it can alleviate mitochondrial oxidative stress via the activation of Nrf2, Sirtuin-1 and Sirtuin-3 (Molina-Jijón et al., 2011; Tapia et al., 2012; Martínez-Morúa et al., 2013; Trujillo et al., 2013; Huang et al., 2022a; Liu et al., 2022c). Curcumin can activate Nrf2 signaling pathway via inhibiting Keap1, upregulating Nrf2 and inducing the nuclear translocation of Nrf2 (Ashrafizadeh et al., 2020). Moreover, a study also has shown that curcumin alleviates aristolochic acid-induced nephropathy and tubular cell apoptosis via Sirtuin-1-Nrf2-ARE signaling pathway (Liu et al., 2022c). Curcumin can also upregulate the expression of SOD, GPX and catalase via Nrf2 to ameliorate mitochondrial oxidative stress, and further restore the activities of renal mitochondrial respiratory enzyme in various kidney diseases (Trujillo et al., 2013; Liu et al., 2022c). Curcumin also induces Sirtuin-3 for renoprotective effects in cisplatin-induced kidney injury (Ugur et al., 2015; Ortega-Domínguez et al., 2017). Clinical trials showed that curcumin can attenuate proteinuria in patients with DKD and lupus nephritis (Khajehdehi et al., 2011; Khajehdehi et al., 2012). In patients with end stage renal disease, curcumin can also alleviate uremic condition (Pakfetrat et al., 2014).
3.2.2.2 Resveratrol
Besides promoting mitochondrial biogenesis, resveratrol also attenuates mitochondrial dysfunction and restores renal function via other multiple mechanisms. Resveratrol is also a natural product with anti-oxidative properties. Resveratrol can promote the nuclear translocation of Nrf2 by inhibiting Keap1 expression (Farkhondeh et al., 2020). Moreover, resveratrol activates sirtuin-1 to activate Nrf2 pathway and protect against oxidative stress (Zhang et al., 2022b). Furthermore, it exhibits anti-oxidation properties via the activation of Sirtuin-3. On one hand, Sirtuin-3 can regulate SOD2 expression via Sirtuin-3-Foxo3a-SOD2 pathway. On the other hand, Sirtuin-3 can also deacetylate and activate mitochondrial proteins involved in the elimination of ROS (Xu et al., 2016a; Clark and Parikh, 2021). Studies have found that resveratrol can upregulate the expression of mitochondrial ROS scavenging enzymes, and attenuate mitochondrial oxidative stress via the activation of Nrf2 pathway and Sirtuin-3-FoxO3a pathway in AKI and CKD models (Xu et al., 2012; Wang et al., 2015b; Fu et al., 2017; Kim et al., 2018; Wang et al., 2018; Rahman et al., 2022).
3.2.3 Antioxidant
3.2.3.1 L-carnitine
L-carnitine mainly derives from daily diet (Granata et al., 2015). Abundant studies have demonstrated that L-carnitine exhibits protective effects for its antioxidant properties through targeting mitochondria (Zhao et al., 2021a). L-carnitine can improve mitochondrial respiratory chain activity and decrease mitochondrial ROS production via promoting acetyl-CoA production (Zhao et al., 2021a). Moreover, L-carnitine can also directly scavenge ROS and protect against the ETC complex impairment. Furthermore, L-carnitine can also restore anti-oxidative molecules and enzymes, such as GSH, SOD, catalase and GPX, in kidney diseases (Ye et al., 2010; Granata et al., 2015). Studies also showed L-carnitine treatment reduces mitochondrial ROS, ameliorates mitochondrial damage in renal TECs, alleviates renal tubulointerstitial fibrosis in UUO, and reduces urinary albumin excretion in DKD (Ye et al., 2010; Zhao et al., 2021a; Ito et al., 2022).
3.2.3.2 Caffeic acid phenethyl ester (CAPE)
CAPE, a natural antioxidant, possesses beneficial roles in cadioprotective, neuroprotective and renoprotective effects (Kamarauskaite et al., 2021). Mitochondrial respiratory chain complex I and III are considered to be the main producers of ROS, the impairment of which contribute to mitochondrial ROS formation (Ashkar et al., 2022). CAPE exhibits a strong antioxidant potential on scavenging mitochondrial ROS (Ashkar et al., 2022). First, CAPE pretreatment protects complex I activity and inhibits ROS generation in complex II in renal IRI model. It can protect against outer mitochondrial membrane (OMM) damage and the release of Cytc from mitochondria. CAPE pretreatment also protects against necrotic cell death and cell apoptosis after renal IRI (Trumbeckaite et al., 2017; Ashkar et al., 2022). Second, CAPE treatment can restore the activity of mitochondrial antioxidant enzymes and increase GSH after kidney injury (Kobroob et al., 2012; Ashkar et al., 2022).
3.2.3.3 Astaxanthin
Astaxanthin, a natural non-toxic product in most of marine organisms, exhibits wide biologic properties, such as strong antioxidant, anti-apoptotic and anti-inflammatory activities, for its unique structure (Chen et al., 2020; Giannaccare et al., 2020). Accumulated studies have demonstrated that astaxanthin exerts protective effects against oxidative stress-associated mitochondrial dysfunction (Kim and Kim, 2018). Study has shown that the antioxidant ability of astaxanthin is 100 times stronger than GSH, an important mitochondrial anti-oxidative molecule (Chen et al., 2020). Astaxanthin can easily span through the cell membrane, and then exhibits its antioxidant activities by scavenging ROS and enhancing the activities of antioxidant enzymes (Kim and Kim, 2018). Studies have demonstrated that astaxanthin treatment can alleviate mitochondrial dysfunction and rescue kidney function via its antioxidant activities in several kidney diseases, such as DKD (Chen et al., 2020; Naito et al., 2021) and bisphenol A-induced kidney toxicity (Jiang et al., 2020b).
3.3 Promoting mitophagy
Mitophagy is a specialized form of autophagy. When damaged mitochondria is unable to maintain their membrane potential, it needs to be degraded through mitophagy (Forbes and Thorburn, 2018; Jin et al., 2021). The defection of mitophagy will lead to the accumulation of dysfunctional mitochondria, resulting in the leakage of Cytc into the cytoplasm to induce the activation of the caspase signaling pathway, and contribute to cell death (Sinha et al., 2013; Mani et al., 2020; Rahman et al., 2022).
Mitophagy mainly consists of PTEN-induced kinase 1 (PINK1)/parkin RBR E3 ubiquitin-protein ligase (Parkin)-mediated and receptor-mediated mitophagy (Dagar et al., 2022). PINK1 and Parkin play important roles in the formation of autophagosome (Forbes and Thorburn, 2018). Under normal condition, PINK1 is transported from cytosol to mitochondrial matrix, where it is cleaved. When mitochondria lose their membrane potential, mitochondrial protein input is impaired, and PINK1 accumulates on OMM. And then it phosphorylates the pre-existing ubiquitin (Ub) on OMM, which recruits Parkin into OMM. The recruited Parkin on OMM is also phosphorylated by PINK1. Phosphorylated Parkin is able to ubiquitinate various proteins on OMM surface, which of them further are phosphorylated by PINK1. And then, Parkin is recruited and phosphorylated. It turns to be a feedforward cycle (Pickles et al., 2018; Jin et al., 2021). Then Ubs on OMM recruits ubiquitin-bond autophagy receptors like p62, which interacts with LC3 to form autophagosome (Kerr et al., 2017; Sun et al., 2021). These receptors, including B-cell lymphoma 2/adenovirus E1B 19-kDa-interacting protein 3 (BNIP3), BNIP3-like (BNIP3L), FUN14 domain-containing protein 1 (FUNDC1) and the activating molecule in BECN1-regulated autophagy protein 1 (AMBRA1), would bind with LC3 to form autophagosome (Dagar et al., 2022). Then the autophagosome containing injured mitochondria is transported to lysosome for degradation (Jin et al., 2021). It has been reported that enhanced mitophagy can relieve mitochondrial dysfunction and attenuate kidney injury (Xiao et al., 2017; Wang et al., 2020b; Zhang et al., 2021b) (Figure 4).
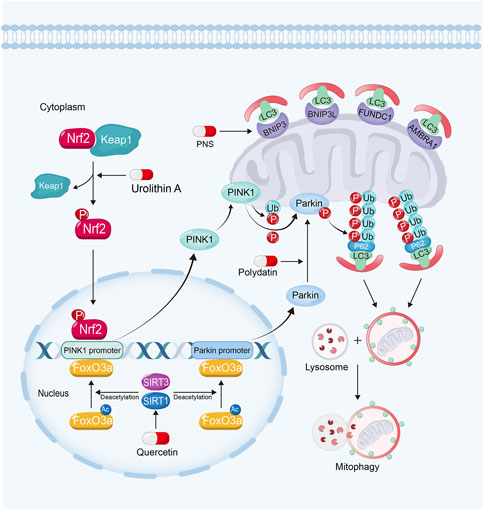
FIGURE 4. Polydatin promotes mitophagy by driving Parkin translocation from cytosol into OMM in kidney diseases. Quercetin can upregulate PINK1 and Parkin expression via sirtuin-1-FoxO3a-PINK1/Parkin signaling pathway. Urolithin A enhances mitophagy in kidney diseases by Nrf2-PINK1 pathway and PNS enhances mitophagy via the upregulation of BNIP3.
3.3.1 Sirtuin activators
Sirtuins also play important roles in regulating mitophagy for their deacetylation activity (Zhao N. et al., 2021). Sirtuin-1 and Sirtuin-3 can deacetylate and activate FoxO3a. Furthermore, FoxO3a can bind to PINK1 and Parkin gene promoter and upregulate their expression to enhance mitophagy (Zheng et al., 2019; Zhao et al., 2021c; Ding et al., 2021; Guo et al., 2021; Liu et al., 2022b; Huang et al., 2022d; Gupta et al., 2022; Yao et al., 2022). Moreover, the activation of Sirtuin-1 can promote Parkin to translocate from cytosol into OMM, while inhibition of Sirtuin-1 reverses these effects (Gao et al., 2020b). However, the mechanism of this remains unknown.
3.3.1.1 Polydatin
Polydatin, a resveratrol glycoside isolated from traditional Chinese herb, Polygonum cuspidatum (Gao et al., 2015), exhibits renoprotective effects via the activation of Sirtuin-1. For example, it has been found that polydatin promotes mitochondrial biogenesis and protects against mitochondrial oxidative stress via the activation of Sirtuin-1-PGC-1α pathway and Sirtuin-1-Nrf2-ARE pathway in kidney diseases (Huang et al., 2015; Zeng et al., 2016; Chen and Lan, 2017; Gao et al., 2020b). Polydatin can trigger the translocation of Parkin from cytosol into OMM, resulting in the enhanced mitophagy. Polydatin treatment alleviates tubular cell apoptosis and rescues renal function in sepsis-induced AKI (Gao et al., 2020b).
3.3.1.2 Quercetin
Quercetin, a Sirtuin-1 and Sirtuin-3 activator, can be widely obtained from many fruits and vegetables, and exhibits wide biological effects in resisting inflammation, diabetic and microbial activities (Chen et al., 2021; Zymone et al., 2022). Quercetin also shows renoprotective effects against nephrotoxicity, AKI and CKD (Lu et al., 2021; Chen et al., 2022). It has been found quercetin can upregulate PINK1 and Parkin expression via Sirtuin-1 activation. Quercetin treatment also attenuates renal TEC senescence by promoting mitophagy (Liu et al., 2020).
3.3.2 Nrf2 activators
The activation of Nrf2-ARE signaling pathway also plays an important role in protecting against mitochondrial dysfunction through promoting mitophagy (Ran et al., 2022). Nrf2 can bind to AREs on PINK1 promoter, and then upregulate PINK1 expression and promote mitophagy (Murata et al., 2015; Xiao et al., 2017; Gumeni et al., 2021; Chen, 2022).
3.3.2.1 Urolithin A
Urolithin A, a natural gut metabolite of ellagic acid and ellagitannins, rich in nuts, is a safe mitophagy activator (Andreux et al., 2019; Jin et al., 2021). Accumulated evidences have shown that urolithin A exhibits multiple protective effects inducing mitophagy in heart (Huang et al., 2022c), muscle (Luan et al., 2021) and brain (Jayatunga et al., 2021). As a Nrf2 activator, urolithin A not only protects kidney against oxidative stress via promoting Nrf2 translocating into nucleus (Zhang et al., 2022c), but also ameliorates hyperuricemic nephropathy via enhancing PINK1-associated mitophagy in renal TECs (Zhang et al., 2022a). A study showed that urolithin A treatment restores renal function, and alleviates tubular cell dilation and collagen deposition in glomerular basement membrane (Zhang et al., 2022a).
3.3.3 Receptors mediated mitophagy activators
Panax notoginseng saponins (PNS), the primary ingredient from Panax notoginseng, exhibits extensive therapeutic effects in cardiovascular diseases (Liu et al., 2014), lung cancer (Yang et al., 2016) and diabetes (Uzayisenga et al., 2014). Studies have demonstrated that PNS also exhibits powerful protective effects against DKD and AKI (Li et al., 2021c; He et al., 2022). It has been found that PNS is able to alleviate cisplatin-induced nephrotoxicity by inducing BNIP3-mediated mitophagy via hypoxia-inducible factor-1α (HIF-1α). PNS treatment restores renal function via attenuating the accumulation of injury mitochondria and cell apoptosis in TECs (Liang et al., 2017; Li et al., 2021c). In one clinical trial, PNS shows renoprotective effects on reducing albuminuria, proteinuria and Scr in patients with DKD (Tang et al., 2020).
3.4 Regulating mitochondrial dynamics
As a dynamic organelle, mitochondria frequently change their shape to satisfy the variations in metabolic demands (Qin et al., 2019). The accumulation of dysfunctional mitochondria with abnormal morphology contributes to the progression of kidney diseases (Galvan et al., 2017). Mitochondrial dynamics plays important roles in maintaining mitochondrial structure and morphology (Rahman et al., 2022). The maintenance of mitochondrial dynamics involves the balance between mitochondrial fission and fusion. In kidney diseases, extensive mitochondrial fission leads to mitochondrial fragmentation and the insufficient function (Galvan et al., 2017) (Figure 5).
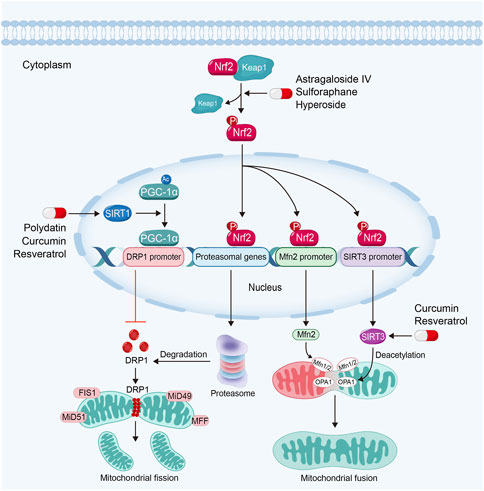
FIGURE 5. Sirtuin activators such as polydatin, resveratrol and curcumin regulate mitochondrial dynamics via sirtuin-1-PGC-1α-DRP1 pathway and the activation of OPA1 by sirtuin-3 in kidney diseases. Nrf2 activators inhibit mitochondrial fission and promote mitochondrial fusion in kidney diseases by Nrf2-proteasomal genes/Mfn2/sirtuin-3 signaling pathway.
Dynamin related protein 1 (DRP1), the main mediator for mitochondrial fission, is recruited into OMM by its receptors, such as mitochondrial fission 1 protein (FIS1), mitochondrial fission factor (MFF) and mitochondrial dynamics proteins of 49 (MiD49) and 51. Then DRP1 constricts mitochondrial membrane and drives mitochondrial fission (Galvan et al., 2017). Upregulated DRP1 in kidney diseases can promote the permeability of OMM via the interaction with Bcl-2-associated X protein (BAX), a crucial executor of mitochondria-regulated apoptosis (Spitz and Gavathiotis, 2022) to trigger cell apoptosis (Qin et al., 2019). By contrast, the interaction of mitofusin 1 (Mfn1) and 2 mediates OMM fusion, while optic atrophy 1 (OPA1) regulates IMM fusion (Galvan et al., 2017). Studies have found that the downregulation of OPA1 will induce mitochondrial fragmentation and cellular apoptosis (Ni et al., 2017). It also has been found that extensive mitochondrial fission and defective mitochondrial fusion contribute to kidney diseases. Maintaining the balance of mitochondrial fission and fusion can restore mitochondrial function and subsequently protect renal function (Jiang et al., 2020a; Tang et al., 2021).
3.4.1 Sirtuin activators
Sirtuins, due to its deacetylating activity, also play significant roles in the regulation of mitochondrial dynamics. Sirtuin-1 can deacetylate PGC-1α. PGC-1α regulates DRP1 expression by enriching in the promoter region to downregulate DRP1 expression and further inhibits mitochondrial fission (Ding et al., 2018; Dong et al., 2022). Study also has found that Sirtuin-3 can promote mitochondrial fusion via deacetylating and activating OPA1 (Samant et al., 2014).
Almost natural Sirtuin activators mentioned above are able to regulate mitochondrial dynamics in kidney diseases. For instance, polydatin is able to stabilize mitochondrial morphology and alleviate podocyte apoptosis via suppressing the expression and activation of DRP1 in DKD (Ni et al., 2017). Moreover, polydatin treatment attenuates sepsis-induced irregular or swollen mitochondria in renal TECs (Gao et al., 2015). Resveratrol can alleviate kidney injury via regulating mitochondrial dynamics. Hui et al. found that resveratrol alleviates excessive mitochondrial fission and promotes mitochondrial fusion in TGF-β1-treated mesangial cells (Li et al., 2021b). Curcumin can also reverse mitochondrial fragmentation in renal TECs via inhibiting mitochondrial fission and promoting mitochondrial fusion in cisplatin-induced kidney injury (Ortega-Domínguez et al., 2017; Cai et al., 2022).
3.4.2 Nrf2 activators
Studies have demonstrated that Nrf2-ARE signaling pathway also plays a core role in the regulation of mitochondrial dynamics. Nrf2 can bind to the AREs in proteasomal gene promoter, which plays important roles in the degradation of DRP1. Moreover, activated Nrf2 can also dock on the AREs in Mfn2 gene promoter to promote mitochondrial fusion (Chen, 2022). Furthermore, Nrf2 can also upregulate Sirtuin-3 expression through binding to AREs in Sirtuin-3 promoter (Hu et al., 2021). Consequently, Sirtuin-3 can promote mitochondrial fusion via activating OPA1 (Samant et al., 2014).
A majority of Nrf2 activators can also regulate mitochondrial dynamics via the inhibition of mitochondrial fission and promotion of mitochondrial fusion in kidney diseases. For example, sulforaphane regulates mitochondrial dynamics and stabilizes mitochondrial morphology via downregulation of DRP1 in maleic acid-induced nephropathy and Fanconi syndrome (Briones-Herrera et al., 2018; Briones-Herrera et al., 2020). Astragaloside IV can protect against mitochondrial dysfunction and delay the progression of DKD via downregulating the expression of DRP1. In addition, Astragaloside IV treatment also ameliorates urinary albumin excretion and kidney injury in db/db mice (Liu et al., 2017).
Hyperoside, a Nrf2 nuclear translocation activator extracted from the flowers of Abelmoschus manihot (L.) Medic, shows a great potency in anti-inflammatory, anti-oxidation and anti-apoptosis effects (Chen et al., 2017). Hyperoside can alleviate excessive mitochondrial fission and promotes mitochondrial fusion via the inhibition of DRP1 and upregulation of Mfn (Chen et al., 2017). Furthermore, hyperoside is able to promote mitochondrial fusion and stabilize mitochondrial morphology via OPA1 in renal TECs (Wu et al., 2019).
4 Conclusion and perspectives
Although human kidney diseases arise from complicated pathological mechanisms and different etiologies, mitochondrial dysfunction is no doubt plays an important role in the pathogenesis. As a result, to maintain mitochondrial function has already shown to be a prospect strategy for therapeutic value in various kidney diseases. Hence, more and more studies in recent years have focused on the strategy targeting mitochondria in kidney diseases (Granata et al., 2015; Rahman et al., 2022; Tovar-Palacio et al., 2022).
Natural products, the mixture or monomer from animals, plants and microorganisms, with various bioactive ingredients, are huge valuable sources for drug candidates development (Katz and Baltz, 2016; Mu et al., 2020; Liang et al., 2021). In this review, we generally summarized the feasible strategies for attenuating mitochondrial dysfunction and restoring their function, including promoting mitochondrial biogenetics and energetics, reducing mitochondrial ROS, enhancing mitophagy and regulating mitochondrial dynamics. We focused on various kinds of natural products regulating mitochondrial function, such as AMPK activators, Sirtuin activators, Nrf2 activators and other antioxidants. This review may provide a practical guidance for clinic to choose appropriate natural products, from a new prospect, to restore mitochondrial function.
Author contributions
Wrote the paper: JH and YL. Language modification and guidance: LZ. All authors have read and approved the final manuscript.
Funding
National Natural Science Foundation of China Grant 82225010, 82070707; and Outstanding youth cultivation program in Nanfang Hospital (2021J001) and the Presidential Foundation of Nanfang Hospital (Grant No. 2019Z006), and Guangdong Provincial Clinical Research Center for Kidney Disease (No. 2020B1111170013).
Conflict of interest
The authors declare that the research was conducted in the absence of any commercial or financial relationships that could be construed as a potential conflict of interest.
Publisher’s note
All claims expressed in this article are solely those of the authors and do not necessarily represent those of their affiliated organizations, or those of the publisher, the editors and the reviewers. Any product that may be evaluated in this article, or claim that may be made by its manufacturer, is not guaranteed or endorsed by the publisher.
References
Aladaileh, S. H., Hussein, O. E., Abukhalil, M. H., Saghir, S. a. M., Bin-Jumah, M., Alfwuaires, M. A., et al. (2019). Formononetin upregulates Nrf2/HO-1 signaling and prevents oxidative stress, inflammation, and kidney injury in methotrexate-induced rats. Antioxidants (Basel) 8, 430. doi:10.3390/antiox8100430
Alkis, H., Demir, E., Taysi, M. R., Sagir, S., and Taysi, S. (2021). Effects of Nigella sativa oil and thymoquinone on radiation-induced oxidative stress in kidney tissue of rats. Biomed. Pharmacother. 139, 111540. doi:10.1016/j.biopha.2021.111540
Althunibat, O. Y., Abukhalil, M. H., Aladaileh, S. H., Qaralleh, H., Al-Amarat, W., Alfwuaires, M. A., et al. (2022). Formononetin ameliorates renal dysfunction, oxidative stress, inflammation, and apoptosis and upregulates Nrf2/HO-1 signaling in a rat model of gentamicin-induced nephrotoxicity. Front. Pharmacol. 13, 916732. doi:10.3389/fphar.2022.916732
Andreux, P. A., Blanco-Bose, W., Ryu, D., Burdet, F., Ibberson, M., Aebischer, P., et al. (2019). The mitophagy activator urolithin A is safe and induces a molecular signature of improved mitochondrial and cellular health in humans. Nat. Metab. 1, 595–603. doi:10.1038/s42255-019-0073-4
Aparicio-Trejo, O. E., Tapia, E., Sánchez-Lozada, L. G., and Pedraza-Chaverri, J. (2018). Mitochondrial bioenergetics, redox state, dynamics and turnover alterations in renal mass reduction models of chronic kidney diseases and their possible implications in the progression of this illness. Pharmacol. Res. 135, 1–11. doi:10.1016/j.phrs.2018.07.015
Arif, E., Solanki, A. K., Srivastava, P., Rahman, B., Fitzgibbon, W. R., Deng, P., et al. (2019). Mitochondrial biogenesis induced by the β2-adrenergic receptor agonist formoterol accelerates podocyte recovery from glomerular injury. Kidney Int. 96, 656–673. doi:10.1016/j.kint.2019.03.023
Ashkar, F., Bhullar, K. S., and Wu, J. (2022). The effect of polyphenols on kidney disease: Targeting mitochondria. Nutrients 14, 3115. doi:10.3390/nu14153115
Ashrafizadeh, M., Ahmadi, Z., Mohammadinejad, R., Farkhondeh, T., and Samarghandian, S. (2020). Curcumin activates the Nrf2 pathway and induces cellular protection against oxidative injury. Curr. Mol. Med. 20, 116–133. doi:10.2174/1566524019666191016150757
Avila-Carrasco, L., García-Mayorga, E. A., Díaz-Avila, D. L., Garza-Veloz, I., Martinez-Fierro, M. L., and González-Mateo, G. T. (2021). Potential therapeutic effects of natural plant compounds in kidney disease. Molecules 26, 6096. doi:10.3390/molecules26206096
Balaban, R. S., Nemoto, S., and Finkel, T. (2005). Mitochondria, oxidants, and aging. Cell 120, 483–495. doi:10.1016/j.cell.2005.02.001
Bao, L., Cai, X., Dai, X., Ding, Y., Jiang, Y., Li, Y., et al. (2014). Grape seed proanthocyanidin extracts ameliorate podocyte injury by activating peroxisome proliferator-activated receptor-γ coactivator 1α in low-dose streptozotocin-and high-carbohydrate/high-fat diet-induced diabetic rats. Food Funct. 5, 1872–1880. doi:10.1039/c4fo00340c
Barone, R., Rizzo, R., Tabbì, G., Malaguarnera, M., Frye, R. E., and Bastin, J. (2019). Nuclear peroxisome proliferator-activated receptors (PPARs) as therapeutic targets of resveratrol for autism spectrum disorder. Int. J. Mol. Sci. 20, 1878. doi:10.3390/ijms20081878
Bhargava, P., and Schnellmann, R. G. (2017). Mitochondrial energetics in the kidney. Nat. Rev. Nephrol. 13, 629–646. doi:10.1038/nrneph.2017.107
Briones-Herrera, A., Avila-Rojas, S. H., Aparicio-Trejo, O. E., Cristóbal, M., León-Contreras, J. C., Hernández-Pando, R., et al. (2018). Sulforaphane prevents maleic acid-induced nephropathy by modulating renal hemodynamics, mitochondrial bioenergetics and oxidative stress. Food Chem. Toxicol. 115, 185–197. doi:10.1016/j.fct.2018.03.016
Briones-Herrera, A., Ramírez-Camacho, I., Zazueta, C., Tapia, E., and Pedraza-Chaverri, J. (2020). Altered proximal tubule fatty acid utilization, mitophagy, fission and supercomplexes arrangement in experimental Fanconi syndrome are ameliorated by sulforaphane-induced mitochondrial biogenesis. Free Radic. Biol. Med. 153, 54–70. doi:10.1016/j.freeradbiomed.2020.04.010
Cai, Y., Huang, C., Zhou, M., Xu, S., Xie, Y., Gao, S., et al. (2022). Role of curcumin in the treatment of acute kidney injury: Research challenges and opportunities. Phytomedicine 104, 154306. doi:10.1016/j.phymed.2022.154306
Cekauskas, A., Bruns, H., Manikas, M., Herr, I., Gross, M. L., Zorn, M., et al. (2013). Sulforaphane decreases kidney injury after transplantation in rats: Role of mitochondrial damage. Ann. Transpl. 18, 488–496. doi:10.12659/aot.884013
Chen, L., and Lan, Z. (2017). Polydatin attenuates potassium oxonate-induced hyperuricemia and kidney inflammation by inhibiting NF-κB/NLRP3 inflammasome activation via the AMPK/SIRT1 pathway. Food Funct. 8, 1785–1792. doi:10.1039/c6fo01561a
Chen, Q. M. (2022). Nrf2 for protection against oxidant generation and mitochondrial damage in cardiac injury. Free Radic. Biol. Med. 179, 133–143. doi:10.1016/j.freeradbiomed.2021.12.001
Chen, W. J., Cheng, Y., Li, W., Dong, X. K., Wei, J. L., Yang, C. H., et al. (2021). Quercetin attenuates cardiac hypertrophy by inhibiting mitochondrial dysfunction through SIRT3/PARP-1 pathway. Front. Pharmacol. 12, 739615. doi:10.3389/fphar.2021.739615
Chen, X., Wei, W., Li, Y., Huang, J., and Ci, X. (2019a). Hesperetin relieves cisplatin-induced acute kidney injury by mitigating oxidative stress, inflammation and apoptosis. Chem. Biol. Interact. 308, 269–278. doi:10.1016/j.cbi.2019.05.040
Chen, Y. J., Kong, L., Tang, Z. Z., Zhang, Y. M., Liu, Y., Wang, T. Y., et al. (2019b). Hesperetin ameliorates diabetic nephropathy in rats by activating Nrf2/ARE/glyoxalase 1 pathway. Biomed. Pharmacother. 111, 1166–1175. doi:10.1016/j.biopha.2019.01.030
Chen, Y. Q., Chen, H. Y., Tang, Q. Q., Li, Y. F., Liu, X. S., Lu, F. H., et al. (2022). Protective effect of quercetin on kidney diseases: From chemistry to herbal medicines. Front. Pharmacol. 13, 968226. doi:10.3389/fphar.2022.968226
Chen, Z., An, X., Liu, X., Qi, J., Ding, D., Zhao, M., et al. (2017). Hyperoside alleviates adriamycin-induced podocyte injury via inhibiting mitochondrial fission. Oncotarget 8, 88792–88803. doi:10.18632/oncotarget.21287
Chen, Z., Li, W., Shi, L., Jiang, L., Li, M., Zhang, C., et al. (2020). Kidney-targeted astaxanthin natural antioxidant nanosystem for diabetic nephropathy therapy. Eur. J. Pharm. Biopharm. 156, 143–154. doi:10.1016/j.ejpb.2020.09.005
Chung, K. W., Lee, E. K., Lee, M. K., Oh, G. T., Yu, B. P., and Chung, H. Y. (2018). Impairment of PPARα and the fatty acid oxidation pathway aggravates renal fibrosis during aging. J. Am. Soc. Nephrol. 29, 1223–1237. doi:10.1681/asn.2017070802
Clark, A. J., and Parikh, S. M. (2021). Targeting energy pathways in kidney disease: The roles of sirtuins, AMPK, and PGC1α. Kidney Int. 99, 828–840. doi:10.1016/j.kint.2020.09.037
Dagar, N., Kale, A., Steiger, S., Anders, H. J., and Gaikwad, A. B. (2022). Receptor-mediated mitophagy: An emerging therapeutic target in acute kidney injury. Mitochondrion 66, 82–91. doi:10.1016/j.mito.2022.08.004
Dai, H., Sinclair, D. A., Ellis, J. L., and Steegborn, C. (2018). Sirtuin activators and inhibitors: Promises, achievements, and challenges. Pharmacol. Ther. 188, 140–154. doi:10.1016/j.pharmthera.2018.03.004
Dai, P., Wang, J., Lin, L., Zhang, Y., and Wang, Z. (2015). Renoprotective effects of berberine as adjuvant therapy for hypertensive patients with type 2 diabetes mellitus: Evaluation via biochemical markers and color Doppler ultrasonography. Exp. Ther. Med. 10, 869–876. doi:10.3892/etm.2015.2585
Dan Dunn, J., Alvarez, L. A., Zhang, X., and Soldati, T. (2015). Reactive oxygen species and mitochondria: A nexus of cellular homeostasis. Redox Biol. 6, 472–485. doi:10.1016/j.redox.2015.09.005
Deblois, G., St-Pierre, J., and Giguère, V. (2013). The PGC-1/ERR signaling axis in cancer. Oncogene 32, 3483–3490. doi:10.1038/onc.2012.529
Dera, A. A., Rajagopalan, P., Alfhili, M. A., Ahmed, I., and Chandramoorthy, H. C. (2020). Thymoquinone attenuates oxidative stress of kidney mitochondria and exerts nephroprotective effects in oxonic acid-induced hyperuricemia rats. Biofactors 46, 292–300. doi:10.1002/biof.1590
Ding, D., Ao, X., Li, M., Miao, S., Liu, Y., Lin, Z., et al. (2021). FOXO3a-dependent Parkin regulates the development of gastric cancer by targeting ATP-binding cassette transporter E1. J. Cell Physiol. 236, 2740–2755. doi:10.1002/jcp.30040
Ding, M., Feng, N., Tang, D., Feng, J., Li, Z., Jia, M., et al. (2018). Melatonin prevents Drp1-mediated mitochondrial fission in diabetic hearts through SIRT1-PGC1α pathway. J. Pineal Res. 65, e12491. doi:10.1111/jpi.12491
Dinkova-Kostova, A. T., and Abramov, A. Y. (2015). The emerging role of Nrf2 in mitochondrial function. Free Radic. Biol. Med. 88, 179–188. doi:10.1016/j.freeradbiomed.2015.04.036
Djouadi, F., Aubey, F., Schlemmer, D., and Bastin, J. (2005). Peroxisome proliferator activated receptor delta (PPARdelta) agonist but not PPARalpha corrects carnitine palmitoyl transferase 2 deficiency in human muscle cells. J. Clin. Endocrinol. Metab. 90, 1791–1797. doi:10.1210/jc.2004-1936
Dong, W., Yan, L., Tan, Y., Chen, S., Zhang, K., Gong, Z., et al. (2022). Melatonin improves mitochondrial function by preventing mitochondrial fission in cadmium-induced rat proximal tubular cell injury via SIRT1-PGC-1α pathway activation. Ecotoxicol. Environ. Saf. 242, 113879. doi:10.1016/j.ecoenv.2022.113879
Eckardt, K. U., Coresh, J., Devuyst, O., Johnson, R. J., Köttgen, A., Levey, A. S., et al. (2013). Evolving importance of kidney disease: From subspecialty to global health burden. Lancet 382, 158–169. doi:10.1016/s0140-6736(13)60439-0
Eirin, A., Lerman, A., and Lerman, L. O. (2015). Mitochondria: A pathogenic paradigm in hypertensive renal disease. Hypertension 65, 264–270. doi:10.1161/hypertensionaha.114.04598
Elsherbiny, N. M., and El-Sherbiny, M. (2014). Thymoquinone attenuates doxorubicin-induced nephrotoxicity in rats: Role of Nrf2 and NOX4. Chem. Biol. Interact. 223, 102–108. doi:10.1016/j.cbi.2014.09.015
Emma, F., Montini, G., Parikh, S. M., and Salviati, L. (2016). Mitochondrial dysfunction in inherited renal disease and acute kidney injury. Nat. Rev. Nephrol. 12, 267–280. doi:10.1038/nrneph.2015.214
Fan, H., Su, B. J., Le, J. W., and Zhu, J. H. (2022). Salidroside protects acute kidney injury in septic rats by inhibiting inflammation and apoptosis. Drug Des. Devel Ther. 16, 899–907. doi:10.2147/dddt.s361972
Farkhondeh, T., Folgado, S. L., Pourbagher-Shahri, A. M., Ashrafizadeh, M., and Samarghandian, S. (2020). The therapeutic effect of resveratrol: Focusing on the Nrf2 signaling pathway. Biomed. Pharmacother. 127, 110234. doi:10.1016/j.biopha.2020.110234
Feng, M., Lv, J., Zhang, C., Chen, D., Guo, H., Tu, Y., et al. (2022). Astragaloside IV protects sepsis-induced acute kidney injury by attenuating mitochondrial dysfunction and apoptosis in renal tubular epithelial cells. Curr. Pharm. Des. 28, 2825–2834. doi:10.2174/1381612828666220902123755
Forbes, J. M., and Thorburn, D. R. (2018). Mitochondrial dysfunction in diabetic kidney disease. Nat. Rev. Nephrol. 14, 291–312. doi:10.1038/nrneph.2018.9
Fu, B., Zhao, J., Peng, W., Wu, H., and Zhang, Y. (2017). Resveratrol rescues cadmium-induced mitochondrial injury by enhancing transcriptional regulation of PGC-1α and SOD2 via the Sirt3/FoxO3a pathway in TCMK-1 cells. Biochem Biophys. Res. Commun. 486, 198–204. doi:10.1016/j.bbrc.2017.03.027
Galvan, D. L., Green, N. H., and Danesh, F. R. (2017). The hallmarks of mitochondrial dysfunction in chronic kidney disease. Kidney Int. 92, 1051–1057. doi:10.1016/j.kint.2017.05.034
Gao, P., Du, X., Liu, L., Xu, H., Liu, M., Guan, X., et al. (2020a). Astragaloside IV alleviates tacrolimus-induced chronic nephrotoxicity via p62-keap1-nrf2 pathway. Front. Pharmacol. 11, 610102. doi:10.3389/fphar.2020.610102
Gao, Y., Dai, X., Li, Y., Li, G., Lin, X., Ai, C., et al. (2020b). Role of Parkin-mediated mitophagy in the protective effect of polydatin in sepsis-induced acute kidney injury. J. Transl. Med. 18, 114. doi:10.1186/s12967-020-02283-2
Gao, Y., Zeng, Z., Li, T., Xu, S., Wang, X., Chen, Z., et al. (2015). Polydatin inhibits mitochondrial dysfunction in the renal tubular epithelial cells of a rat model of sepsis-induced acute kidney injury. Anesth. Analg. 121, 1251–1260. doi:10.1213/ane.0000000000000977
Gerhart-Hines, Z., Rodgers, J. T., Bare, O., Lerin, C., Kim, S. H., Mostoslavsky, R., et al. (2007). Metabolic control of muscle mitochondrial function and fatty acid oxidation through SIRT1/PGC-1alpha. Embo J. 26, 1913–1923. doi:10.1038/sj.emboj.7601633
Giannaccare, G., Pellegrini, M., Senni, C., Bernabei, F., Scorcia, V., and Cicero, A. F. G. (2020). Clinical applications of astaxanthin in the treatment of ocular diseases: Emerging insights. Mar. Drugs 18, 239. doi:10.3390/md18050239
Glassock, R. J., Warnock, D. G., and Delanaye, P. (2017). The global burden of chronic kidney disease: Estimates, variability and pitfalls. Nat. Rev. Nephrol. 13, 104–114. doi:10.1038/nrneph.2016.163
Granata, S., Dalla Gassa, A., Tomei, P., Lupo, A., and Zaza, G. (2015). Mitochondria: A new therapeutic target in chronic kidney disease. Nutr. Metab. (Lond) 12, 49. doi:10.1186/s12986-015-0044-z
Gumeni, S., Papanagnou, E. D., Manola, M. S., and Trougakos, I. P. (2021). Nrf2 activation induces mitophagy and reverses Parkin/Pink1 knock down-mediated neuronal and muscle degeneration phenotypes. Cell Death Dis. 12, 671. doi:10.1038/s41419-021-03952-w
Guo, J., Wang, R., and Liu, D. (2021). Bone marrow-derived mesenchymal stem cells ameliorate sepsis-induced acute kidney injury by promoting mitophagy of renal tubular epithelial cells via the SIRT1/parkin Axis. Front. Endocrinol. (Lausanne) 12, 639165. doi:10.3389/fendo.2021.639165
Gupta, P., Sharma, G., Lahiri, A., and Barthwal, M. K. (2022). FOXO3a acetylation regulates PINK1, mitophagy, inflammasome activation in murine palmitate-conditioned and diabetic macrophages. J. Leukoc. Biol. 111, 611–627. doi:10.1002/jlb.3a0620-348rr
Gureev, A. P., Shaforostova, E. A., and Popov, V. N. (2019). Regulation of mitochondrial biogenesis as a way for active longevity: Interaction between the Nrf2 and PGC-1α signaling pathways. Front. Genet. 10, 435. doi:10.3389/fgene.2019.00435
Hannan, M. A., Zahan, M. S., Sarker, P. P., Moni, A., Ha, H., and Uddin, M. J. (2021). Protective effects of black cumin (Nigella sativa) and its bioactive constituent, thymoquinone against kidney injury: An aspect on pharmacological insights. Int. J. Mol. Sci. 22, 9078. doi:10.3390/ijms22169078
Hao, Y., Miao, J., Liu, W., Peng, L., Chen, Y., and Zhong, Q. (2021). Formononetin protects against cisplatin-induced acute kidney injury through activation of the PPARα/Nrf2/HO-1/NQO1 pathway. Int. J. Mol. Med. 47, 511–522. doi:10.3892/ijmm.2020.4805
Hashem, K. S., Abdelazem, A. Z., Mohammed, M. A., Nagi, A. M., Aboulhoda, B. E., Mohammed, E. T., et al. (2021). Thymoquinone alleviates mitochondrial viability and apoptosis in diclofenac-induced acute kidney injury (AKI) via regulating Mfn2 and miR-34a mRNA expressions. Environ. Sci. Pollut. Res. Int. 28, 10100–10113. doi:10.1007/s11356-020-11313-x
He, J. Y., Hong, Q., Chen, B. X., Cui, S. Y., Liu, R., Cai, G. Y., et al. (2022). Ginsenoside Rb1 alleviates diabetic kidney podocyte injury by inhibiting aldose reductase activity. Acta Pharmacol. Sin. 43, 342–353. doi:10.1038/s41401-021-00788-0
Herzig, S., and Shaw, R. J. (2018). Ampk: Guardian of metabolism and mitochondrial homeostasis. Nat. Rev. Mol. Cell Biol. 19, 121–135. doi:10.1038/nrm.2017.95
Houten, S. M., and Wanders, R. J. (2010). A general introduction to the biochemistry of mitochondrial fatty acid β-oxidation. J. Inherit. Metab. Dis. 33, 469–477. doi:10.1007/s10545-010-9061-2
Hu, S., Zhang, C., Qian, T., Bai, Y., Chen, L., Chen, J., et al. (2021). Promoting nrf2/sirt3-dependent mitophagy suppresses apoptosis in nucleus pulposus cells and protects against intervertebral disc degeneration. Oxid. Med. Cell Longev. 2021, 6694964. doi:10.1155/2021/6694964
Huang, C., Jiang, S., Gao, S., Wang, Y., Cai, X., Fang, J., et al. (2022a). Sirtuins: Research advances on the therapeutic role in acute kidney injury. Phytomedicine 101, 154122. doi:10.1016/j.phymed.2022.154122
Huang, J., Kong, Y., Xie, C., and Zhou, L. (2021). Stem/progenitor cell in kidney: Characteristics, homing, coordination, and maintenance. Stem Cell Res. Ther. 12, 197. doi:10.1186/s13287-021-02266-0
Huang, J., Meng, P., Wang, C., Zhang, Y., and Zhou, L. (2022b). The relevance of organelle interactions in cellular senescence. Theranostics 12, 2445–2464. doi:10.7150/thno.70588
Huang, J. R., Zhang, M. H., Chen, Y. J., Sun, Y. L., Gao, Z. M., Li, Z. J., et al. (2022c). Urolithin A ameliorates obesity-induced metabolic cardiomyopathy in mice via mitophagy activation. Acta Pharmacol. Sin. 44, 321–331. doi:10.1038/s41401-022-00919-1
Huang, K., Chen, C., Hao, J., Huang, J., Wang, S., Liu, P., et al. (2015). Polydatin promotes Nrf2-ARE anti-oxidative pathway through activating Sirt1 to resist AGEs-induced upregulation of fibronetin and transforming growth factor-β1 in rat glomerular messangial cells. Mol. Cell Endocrinol. 399, 178–189. doi:10.1016/j.mce.2014.08.014
Huang, L., Yao, T., Chen, J., Zhang, Z., Yang, W., Gao, X., et al. (2022d). Effect of Sirt3 on retinal pigment epithelial cells in high glucose through Foxo3a/PINK1-Parkin pathway mediated mitophagy. Exp. Eye Res. 218, 109015. doi:10.1016/j.exer.2022.109015
Huang, Q., Chen, H., Yin, K., Shen, Y., Lin, K., Guo, X., et al. (2022e). Formononetin attenuates renal tubular injury and mitochondrial damage in diabetic nephropathy partly via regulating sirt1/PGC-1α pathway. Front. Pharmacol. 13, 901234. doi:10.3389/fphar.2022.901234
Huang, X., Xue, H., Ma, J., Zhang, Y., Zhang, J., Liu, Y., et al. (2019). Salidroside ameliorates Adriamycin nephropathy in mice by inhibiting β-catenin activity. J. Cell Mol. Med. 23, 4443–4453. doi:10.1111/jcmm.14340
Hui, Y., Lu, M., Han, Y., Zhou, H., Liu, W., Li, L., et al. (2017). Resveratrol improves mitochondrial function in the remnant kidney from 5/6 nephrectomized rats. Acta histochem. 119, 392–399. doi:10.1016/j.acthis.2017.04.002
Ito, S., Nakashima, M., Ishikiriyama, T., Nakashima, H., Yamagata, A., Imakiire, T., et al. (2022). Effects of L-carnitine treatment on kidney mitochondria and macrophages in mice with diabetic nephropathy. Kidney Blood Press Res. 47, 277–290. doi:10.1159/000522013
Jacobs, K. M., Pennington, J. D., Bisht, K. S., Aykin-Burns, N., Kim, H. S., Mishra, M., et al. (2008). SIRT3 interacts with the daf-16 homolog FOXO3a in the mitochondria, as well as increases FOXO3a dependent gene expression. Int. J. Biol. Sci. 4, 291–299. doi:10.7150/ijbs.4.291
Jayatunga, D. P. W., Hone, E., Khaira, H., Lunelli, T., Singh, H., Guillemin, G. J., et al. (2021). Therapeutic potential of mitophagy-inducing microflora metabolite, urolithin A for alzheimer's disease. Nutrients 13, 3744. doi:10.3390/nu13113744
Jiang, N., Zhao, H., Han, Y., Li, L., Xiong, S., Zeng, L., et al. (2020a). HIF-1α ameliorates tubular injury in diabetic nephropathy via HO-1-mediated control of mitochondrial dynamics. Cell Prolif. 53, e12909. doi:10.1111/cpr.12909
Jiang, W., Zhao, H., Zhang, L., Wu, B., and Zha, Z. (2020b). Maintenance of mitochondrial function by astaxanthin protects against bisphenol A-induced kidney toxicity in rats. Biomed. Pharmacother. 121, 109629. doi:10.1016/j.biopha.2019.109629
Jin, X., Guo, J. L., Wang, L., Zhong, X., Yao, W. F., Gao, H., et al. (2021). Natural products as pharmacological modulators of mitochondrial dysfunctions for the treatments of alzheimer's disease: A comprehensive review. Eur. J. Med. Chem. 218, 113401. doi:10.1016/j.ejmech.2021.113401
Jornayvaz, F. R., and Shulman, G. I. (2010). Regulation of mitochondrial biogenesis. Essays Biochem 47, 69–84. doi:10.1042/bse0470069
Joshi, T., Singh, A. K., Haratipour, P., Sah, A. N., Pandey, A. K., Naseri, R., et al. (2019). Targeting AMPK signaling pathway by natural products for treatment of diabetes mellitus and its complications. J. Cell Physiol. 234, 17212–17231. doi:10.1002/jcp.28528
Jung, S. H., Kim, H. J., Oh, G. S., Shen, A., Lee, S., Choe, S. K., et al. (2014). Capsaicin ameliorates cisplatin-induced renal injury through induction of heme oxygenase-1. Mol. Cells 37, 234–240. doi:10.14348/molcells.2014.2322
Kamarauskaite, J., Baniene, R., Trumbeckas, D., Strazdauskas, A., and Trumbeckaite, S. (2021). Caffeic acid phenethyl ester protects kidney mitochondria against ischemia/reperfusion induced injury in an in vivo rat model. Antioxidants (Basel) 10, 747. doi:10.3390/antiox10050747
Kang, H. M., Ahn, S. H., Choi, P., Ko, Y. A., Han, S. H., Chinga, F., et al. (2015). Defective fatty acid oxidation in renal tubular epithelial cells has a key role in kidney fibrosis development. Nat. Med. 21, 37–46. doi:10.1038/nm.3762
Kanlaya, R., Khamchun, S., Kapincharanon, C., and Thongboonkerd, V. (2016). Protective effect of epigallocatechin-3-gallate (EGCG) via Nrf2 pathway against oxalate-induced epithelial mesenchymal transition (EMT) of renal tubular cells. Sci. Rep. 6, 30233. doi:10.1038/srep30233
Kanlaya, R., and Thongboonkerd, V. (2019). Protective effects of epigallocatechin-3-gallate from green tea in various kidney diseases. Adv. Nutr. 10, 112–121. doi:10.1093/advances/nmy077
Katz, L., and Baltz, R. H. (2016). Natural product discovery: Past, present, and future. J. Ind. Microbiol. Biotechnol. 43, 155–176. doi:10.1007/s10295-015-1723-5
Kerr, J. S., Adriaanse, B. A., Greig, N. H., Mattson, M. P., Cader, M. Z., Bohr, V. A., et al. (2017). Mitophagy and alzheimer's disease: Cellular and molecular mechanisms. Trends Neurosci. 40, 151–166. doi:10.1016/j.tins.2017.01.002
Khajehdehi, P., Pakfetrat, M., Javidnia, K., Azad, F., Malekmakan, L., Nasab, M. H., et al. (2011). Oral supplementation of turmeric attenuates proteinuria, transforming growth factor-β and interleukin-8 levels in patients with overt type 2 diabetic nephropathy: A randomized, double-blind and placebo-controlled study. Scand. J. Urol. Nephrol. 45, 365–370. doi:10.3109/00365599.2011.585622
Khajehdehi, P., Zanjaninejad, B., Aflaki, E., Nazarinia, M., Azad, F., Malekmakan, L., et al. (2012). Oral supplementation of turmeric decreases proteinuria, hematuria, and systolic blood pressure in patients suffering from relapsing or refractory lupus nephritis: A randomized and placebo-controlled study. J. Ren. Nutr. 22, 50–57. doi:10.1053/j.jrn.2011.03.002
Kim, E. N., Lim, J. H., Kim, M. Y., Ban, T. H., Jang, I. A., Yoon, H. E., et al. (2018). Resveratrol, an Nrf2 activator, ameliorates aging-related progressive renal injury. Aging (Albany NY) 10, 83–99. doi:10.18632/aging.101361
Kim, M. Y., Lim, J. H., Youn, H. H., Hong, Y. A., Yang, K. S., Park, H. S., et al. (2013). Resveratrol prevents renal lipotoxicity and inhibits mesangial cell glucotoxicity in a manner dependent on the AMPK-SIRT1-PGC1α axis in db/db mice. Diabetologia 56, 204–217. doi:10.1007/s00125-012-2747-2
Kim, S. H., and Kim, H. (2018). Inhibitory effect of astaxanthin on oxidative stress-induced mitochondrial dysfunction-A mini-review. Nutrients 10, 1137. doi:10.3390/nu10091137
Klotz, L. O., Sánchez-Ramos, C., Prieto-Arroyo, I., Urbánek, P., Steinbrenner, H., and Monsalve, M. (2015). Redox regulation of FoxO transcription factors. Redox Biol. 6, 51–72. doi:10.1016/j.redox.2015.06.019
Kobroob, A., Chattipakorn, N., and Wongmekiat, O. (2012). Caffeic acid phenethyl ester ameliorates cadmium-induced kidney mitochondrial injury. Chem. Biol. Interact. 200, 21–27. doi:10.1016/j.cbi.2012.08.026
Kumar, M., Dahiya, V., Kasala, E. R., Bodduluru, L. N., and Lahkar, M. (2017). The renoprotective activity of hesperetin in cisplatin induced nephrotoxicity in rats: Molecular and biochemical evidence. Biomed. Pharmacother. 89, 1207–1215. doi:10.1016/j.biopha.2017.03.008
Kursunluoglu, G., Kayali, H. A., and Taskiran, D. (2014). The effect of cisplatin toxicity and capsaicin on electron transport chain in liver and kidney of sprague dawley rats. Cell Biochem Biophys. 69, 707–716. doi:10.1007/s12013-014-9857-z
Levey, A. S., and Coresh, J. (2012). Chronic kidney disease. Lancet 379, 165–180. doi:10.1016/s0140-6736(11)60178-5
Li, C., Miao, X., Wang, S., Liu, Y., Sun, J., Liu, Q., et al. (2021a). Elabela may regulate SIRT3-mediated inhibition of oxidative stress through Foxo3a deacetylation preventing diabetic-induced myocardial injury. J. Cell Mol. Med. 25, 323–332. doi:10.1111/jcmm.16052
Li, Q., Xing, C., and Yuan, Y. (2021b). Mitochondrial targeting of herbal medicine in chronic kidney disease. Front. Pharmacol. 12, 632388. doi:10.3389/fphar.2021.632388
Li, Q., Zhang, Y., Yang, Y., Huang, S., Zou, X., Wei, C., et al. (2021c). Panax notoginseng saponins reduces the cisplatin-induced acute renal injury by increasing HIF-1α/BNIP3 to inhibit mitochondrial apoptosis pathway. Biomed. Pharmacother. 142, 111965. doi:10.1016/j.biopha.2021.111965
Li, X., Zhu, Q., Zheng, R., Yan, J., Wei, M., Fan, Y., et al. (2020). Puerarin attenuates diabetic nephropathy by promoting autophagy in podocytes. Front. Physiol. 11, 73. doi:10.3389/fphys.2020.00073
Liang, X., Yang, Y., Huang, Z., Zhou, J., Li, Y., and Zhong, X. (2017). Panax notoginseng saponins mitigate cisplatin induced nephrotoxicity by inducing mitophagy via HIF-1α. Oncotarget 8, 102989–103003. doi:10.18632/oncotarget.19900
Liang, Z., Currais, A., Soriano-Castell, D., Schubert, D., and Maher, P. (2021). Natural products targeting mitochondria: Emerging therapeutics for age-associated neurological disorders. Pharmacol. Ther. 221, 107749. doi:10.1016/j.pharmthera.2020.107749
Lin, C. T., Sun, X. Y., and Lin, A. X. (2016). Supplementation with high-dose trans-resveratrol improves ultrafiltration in peritoneal dialysis patients: A prospective, randomized, double-blind study. Ren. Fail 38, 214–221. doi:10.3109/0886022x.2015.1128236
Linkermann, A., Chen, G., Dong, G., Kunzendorf, U., Krautwald, S., and Dong, Z. (2014). Regulated cell death in AKI. J. Am. Soc. Nephrol. 25, 2689–2701. doi:10.1681/asn.2014030262
Liu, C., Wang, X., Wang, X., Zhang, Y., Min, W., Yu, P., et al. (2022a). A new LKB1 activator, piericidin analogue S14, retards renal fibrosis through promoting autophagy and mitochondrial homeostasis in renal tubular epithelial cells. Theranostics 12, 7158–7179. doi:10.7150/thno.78376
Liu, J., Wang, Y., Qiu, L., Yu, Y., and Wang, C. (2014). Saponins of panax notoginseng: Chemistry, cellular targets and therapeutic opportunities in cardiovascular diseases. Expert Opin. Investig. Drugs 23, 523–539. doi:10.1517/13543784.2014.892582
Liu, T., Yang, Q., Zhang, X., Qin, R., Shan, W., Zhang, H., et al. (2020). Quercetin alleviates kidney fibrosis by reducing renal tubular epithelial cell senescence through the SIRT1/PINK1/mitophagy axis. Life Sci. 257, 118116. doi:10.1016/j.lfs.2020.118116
Liu, W., Yan, F., Xu, Z., Chen, Q., Ren, J., Wang, Q., et al. (2022b). Urolithin A protects human dermal fibroblasts from UVA-induced photoaging through NRF2 activation and mitophagy. J. Photochem Photobiol. B 232, 112462. doi:10.1016/j.jphotobiol.2022.112462
Liu, X., Wang, W., Song, G., Wei, X., Zeng, Y., Han, P., et al. (2017). Astragaloside IV ameliorates diabetic nephropathy by modulating the mitochondrial quality control network. PLoS One 12, e0182558. doi:10.1371/journal.pone.0182558
Liu, Y., Ao, X., Ding, W., Ponnusamy, M., Wu, W., Hao, X., et al. (2018). Critical role of FOXO3a in carcinogenesis. Mol. Cancer 17, 104. doi:10.1186/s12943-018-0856-3
Liu, Z., Shi, B., Wang, Y., Xu, Q., Gao, H., Ma, J., et al. (2022c). Curcumin alleviates aristolochic acid nephropathy based on SIRT1/Nrf2/HO-1 signaling pathway. Toxicology 479, 153297. doi:10.1016/j.tox.2022.153297
Liu, Z., Wang, W., Li, X., Tang, S., Meng, D., Xia, W., et al. (2022d). Capsaicin ameliorates renal fibrosis by inhibiting TGF-β1-Smad2/3 signaling. Phytomedicine 100, 154067. doi:10.1016/j.phymed.2022.154067
Lu, S., Zhou, S., Chen, J., Zheng, J., Ren, J., Qi, P., et al. (2021). Quercetin nanoparticle ameliorates lipopolysaccharide-triggered renal inflammatory impairment by regulation of Sirt1/NF-KB pathway. J. Biomed. Nanotechnol. 17, 230–241. doi:10.1166/jbn.2021.3031
Luan, P., D'amico, D., Andreux, P. A., Laurila, P. P., Wohlwend, M., Li, H., et al. (2021). Urolithin A improves muscle function by inducing mitophagy in muscular dystrophy. Sci. Transl. Med. 13, eabb0319. doi:10.1126/scitranslmed.abb0319
Lv, T., Hu, Y., Ma, Y., Zhen, J., Xin, W., and Wan, Q. (2019). GCN5L1 controls renal lipotoxicity through regulating acetylation of fatty acid oxidation enzymes. J. Physiol. Biochem 75, 597–606. doi:10.1007/s13105-019-00711-6
Ma, Q. (2013). Role of nrf2 in oxidative stress and toxicity. Annu Rev. Pharmacol. Toxicol. 53, 401–426. doi:10.1146/annurev-pharmtox-011112-140320
Mahmoud Moustafa, E., Rashed, E. R., Rashed, R. R., and Omar, N. N. (2021). Piceatannol promotes hepatic and renal AMPK/SIRT1/PGC-1α mitochondrial pathway in rats exposed to reserpine or gamma-radiation. Int. J. Immunopathol. Pharmacol. 35, 20587384211016194. doi:10.1177/20587384211016194
Makhlough, A., Ala, S., Haj-Heydari, Z., Kashi, Z., and Bari, A. (2010). Topical capsaicin therapy for uremic pruritus in patients on hemodialysis. Iran. J. Kidney Dis. 4, 137–140.
Mani, S., Swargiary, G., and Singh, K. K. (2020). Natural agents targeting mitochondria in cancer. Int. J. Mol. Sci. 21, 6992. doi:10.3390/ijms21196992
Martínez-Morúa, A., Soto-Urquieta, M. G., Franco-Robles, E., Zúñiga-Trujillo, I., Campos-Cervantes, A., Pérez-Vázquez, V., et al. (2013). Curcumin decreases oxidative stress in mitochondria isolated from liver and kidneys of high-fat diet-induced obese mice. J. Asian Nat. Prod. Res. 15, 905–915. doi:10.1080/10286020.2013.802687
Miguel, V., Tituaña, J., Herrero, J. I., Herrero, L., Serra, D., Cuevas, P., et al. (2021). Renal tubule Cpt1a overexpression protects from kidney fibrosis by restoring mitochondrial homeostasis. J. Clin. Invest 131, e140695. doi:10.1172/jci140695
Mohammad, R. S., Lokhandwala, M. F., and Banday, A. A. (2022). Age-related mitochondrial impairment and renal injury is ameliorated by sulforaphane via activation of transcription factor NRF2. Antioxidants (Basel) 11, 156. doi:10.3390/antiox11010156
Molina-Jijón, E., Tapia, E., Zazueta, C., El Hafidi, M., Zatarain-Barrón, Z. L., Hernández-Pando, R., et al. (2011). Curcumin prevents Cr(VI)-induced renal oxidant damage by a mitochondrial pathway. Free Radic. Biol. Med. 51, 1543–1557. doi:10.1016/j.freeradbiomed.2011.07.018
Mu, J. K., Li, Y. Q., Shi, T. T., Yu, L. P., Yang, Y. Q., Gu, W., et al. (2020). Remedying the mitochondria to cure human diseases by natural products. Oxid. Med. Cell Longev. 2020, 5232614. doi:10.1155/2020/5232614
Murata, H., Takamatsu, H., Liu, S., Kataoka, K., Huh, N. H., and Sakaguchi, M. (2015). NRF2 regulates PINK1 expression under oxidative stress conditions. PLoS One 10, e0142438. doi:10.1371/journal.pone.0142438
Naito, Y., Uchiyama, K., Handa, O., and Aoi, W. (2021). Therapeutic potential of astaxanthin in diabetic kidney disease. Adv. Exp. Med. Biol. 1261, 239–248. doi:10.1007/978-981-15-7360-6_22
Ni, Y., Deng, J., Liu, X., Li, Q., Zhang, J., Bai, H., et al. (2021). Echinacoside reverses myocardial remodeling and improves heart function via regulating SIRT1/FOXO3a/MnSOD axis in HF rats induced by isoproterenol. J. Cell Mol. Med. 25, 203–216. doi:10.1111/jcmm.15904
Ni, Z., Tao, L., Xiaohui, X., Zelin, Z., Jiangang, L., Zhao, S., et al. (2017). Polydatin impairs mitochondria fitness and ameliorates podocyte injury by suppressing Drp1 expression. J. Cell Physiol. 232, 2776–2787. doi:10.1002/jcp.25943
Ortega-Domínguez, B., Aparicio-Trejo, O. E., García-Arroyo, F. E., León-Contreras, J. C., Tapia, E., Molina-Jijón, E., et al. (2017). Curcumin prevents cisplatin-induced renal alterations in mitochondrial bioenergetics and dynamic. Food Chem. Toxicol. 107, 373–385. doi:10.1016/j.fct.2017.07.018
Oza, M. J., and Kulkarni, Y. A. (2019). Formononetin attenuates kidney damage in type 2 diabetic rats. Life Sci. 219, 109–121. doi:10.1016/j.lfs.2019.01.013
Pakfetrat, M., Basiri, F., Malekmakan, L., and Roozbeh, J. (2014). Effects of turmeric on uremic pruritus in end stage renal disease patients: A double-blind randomized clinical trial. J. Nephrol. 27, 203–207. doi:10.1007/s40620-014-0039-2
Pan, H., Chen, J., Shen, K., Wang, X., Wang, P., Fu, G., et al. (2015). Mitochondrial modulation by Epigallocatechin 3-Gallate ameliorates cisplatin induced renal injury through decreasing oxidative/nitrative stress, inflammation and NF-kB in mice. PLoS One 10, e0124775. doi:10.1371/journal.pone.0124775
Pickles, S., Vigié, P., and Youle, R. J. (2018). Mitophagy and quality control mechanisms in mitochondrial maintenance. Curr. Biol. 28, R170–R185. doi:10.1016/j.cub.2018.01.004
Qin, X., Jiang, M., Zhao, Y., Gong, J., Su, H., Yuan, F., et al. (2020). Berberine protects against diabetic kidney disease via promoting PGC-1α-regulated mitochondrial energy homeostasis. Br. J. Pharmacol. 177, 3646–3661. doi:10.1111/bph.14935
Qin, X., Zhao, Y., Gong, J., Huang, W., Su, H., Yuan, F., et al. (2019). Berberine protects glomerular podocytes via inhibiting drp1-mediated mitochondrial fission and dysfunction. Theranostics 9, 1698–1713. doi:10.7150/thno.30640
Rahman, M. A., Akter, S., Dorotea, D., Mazumder, A., Uddin, M. N., Hannan, M. A., et al. (2022). Renoprotective potentials of small molecule natural products targeting mitochondrial dysfunction. Front. Pharmacol. 13, 925993. doi:10.3389/fphar.2022.925993
Ran, F., Yang, Y., Yang, L., Chen, S., He, P., Liu, Q., et al. (2022). Capsaicin prevents contrast-associated acute kidney injury through activation of Nrf2 in mice. Oxid. Med. Cell Longev. 2022, 1763922. doi:10.1155/2022/1763922
Rasbach, K. A., and Schnellmann, R. G. (2008). Isoflavones promote mitochondrial biogenesis. J. Pharmacol. Exp. Ther. 325, 536–543. doi:10.1124/jpet.107.134882
Ríos-Silva, M., Santos- lvarez, R., Trujillo, X., Cárdenas-María, R. Y., López-Zamudio, M., Bricio-Barrios, J. A., et al. (2018). Effects of chronic administration of capsaicin on biomarkers of kidney injury in male wistar rats with experimental diabetes. Molecules 24, 36. doi:10.3390/molecules24010036
Rodgers, J. T., Lerin, C., Haas, W., Gygi, S. P., Spiegelman, B. M., and Puigserver, P. (2005). Nutrient control of glucose homeostasis through a complex of PGC-1alpha and SIRT1. Nature 434, 113–118. doi:10.1038/nature03354
Rong, Q., Han, B., Li, Y., Yin, H., Li, J., and Hou, Y. (2021). Berberine reduces lipid accumulation by promoting fatty acid oxidation in renal tubular epithelial cells of the diabetic kidney. Front. Pharmacol. 12, 729384. doi:10.3389/fphar.2021.729384
Ryoo, I. G., and Kwak, M. K. (2018). Regulatory crosstalk between the oxidative stress-related transcription factor Nfe2l2/Nrf2 and mitochondria. Toxicol. Appl. Pharmacol. 359, 24–33. doi:10.1016/j.taap.2018.09.014
Sahin, K., Tuzcu, M., Gencoglu, H., Dogukan, A., Timurkan, M., Sahin, N., et al. (2010). Epigallocatechin-3-gallate activates Nrf2/HO-1 signaling pathway in cisplatin-induced nephrotoxicity in rats. Life Sci. 87, 240–245. doi:10.1016/j.lfs.2010.06.014
Samant, S. A., Zhang, H. J., Hong, Z., Pillai, V. B., Sundaresan, N. R., Wolfgeher, D., et al. (2014). SIRT3 deacetylates and activates OPA1 to regulate mitochondrial dynamics during stress. Mol. Cell Biol. 34, 807–819. doi:10.1128/mcb.01483-13
Shao, M., Ye, C., Bayliss, G., and Zhuang, S. (2021). New insights into the effects of individual Chinese herbal medicines on chronic kidney disease. Front. Pharmacol. 12, 774414. doi:10.3389/fphar.2021.774414
Shati, A. A. (2020). Salidroside ameliorates diabetic nephropathy in rats by activating renal AMPK/SIRT1 signaling pathway. J. Food Biochem 44, e13158. doi:10.1111/jfbc.13158
Singh, A. P., Singh, R., Verma, S. S., Rai, V., Kaschula, C. H., Maiti, P., et al. (2019). Health benefits of resveratrol: Evidence from clinical studies. Med. Res. Rev. 39, 1851–1891. doi:10.1002/med.21565
Singh, B. K., Sinha, R. A., Tripathi, M., Mendoza, A., Ohba, K., Sy, J. a. C., et al. (2018). Thyroid hormone receptor and ERRα coordinately regulate mitochondrial fission, mitophagy, biogenesis, and function. Sci. Signal 11, eaam5855. doi:10.1126/scisignal.aam5855
Sinha, K., Das, J., Pal, P. B., and Sil, P. C. (2013). Oxidative stress: The mitochondria-dependent and mitochondria-independent pathways of apoptosis. Arch. Toxicol. 87, 1157–1180. doi:10.1007/s00204-013-1034-4
Song, X. B., Liu, G., Wang, Z. Y., and Wang, L. (2016). Puerarin protects against cadmium-induced proximal tubular cell apoptosis by restoring mitochondrial function. Chem. Biol. Interact. 260, 219–231. doi:10.1016/j.cbi.2016.10.006
Song, X., Li, Z., Liu, F., Wang, Z., and Wang, L. (2017). Restoration of autophagy by puerarin in lead-exposed primary rat proximal tubular cells via regulating AMPK-mTOR signaling. J. Biochem Mol. Toxicol. 31, e21869. doi:10.1002/jbt.21869
Spinelli, J. B., and Haigis, M. C. (2018). The multifaceted contributions of mitochondria to cellular metabolism. Nat. Cell Biol. 20, 745–754. doi:10.1038/s41556-018-0124-1
Spitz, A. Z., and Gavathiotis, E. (2022). Physiological and pharmacological modulation of BAX. Trends Pharmacol. Sci. 43, 206–220. doi:10.1016/j.tips.2021.11.001
Su, Y., Xu, J., Chen, S., Feng, J., Li, J., Lei, Z., et al. (2022). Astragaloside IV protects against ischemia/reperfusion (I/R)-induced kidney injury based on the Keap1-Nrf2/ARE signaling pathway. Transl. Androl. Urol. 11, 1177–1188. doi:10.21037/tau-22-505
Sun, K., Jing, X., Guo, J., Yao, X., and Guo, F. (2021). Mitophagy in degenerative joint diseases. Autophagy 17, 2082–2092. doi:10.1080/15548627.2020.1822097
Sun, W., Liu, X., Zhang, H., Song, Y., Li, T., Liu, X., et al. (2017). Epigallocatechin gallate upregulates NRF2 to prevent diabetic nephropathy via disabling KEAP1. Free Radic. Biol. Med. 108, 840–857. doi:10.1016/j.freeradbiomed.2017.04.365
Suzuki, K., Suda, G., Yamamoto, Y., Furuya, K., Baba, M., Nakamura, A., et al. (2021). Tenofovir-disoproxil-fumarate modulates lipid metabolism via hepatic CD36/PPAR-alpha activation in Hepatitis B virus infection. J. Gastroenterol. 56, 168–180. doi:10.1007/s00535-020-01750-3
Szczepanowska, J., Malinska, D., Wieckowski, M. R., and Duszynski, J. (2012). Effect of mtDNA point mutations on cellular bioenergetics. Biochim. Biophys. Acta 1817, 1740–1746. doi:10.1016/j.bbabio.2012.02.028
Tachibana, K., Takeuchi, K., Inada, H., Yamasaki, D., Ishimoto, K., Tanaka, T., et al. (2009). Regulation of the human SLC25A20 expression by peroxisome proliferator-activated receptor alpha in human hepatoblastoma cells. Biochem Biophys. Res. Commun. 389, 501–505. doi:10.1016/j.bbrc.2009.09.018
Tan, S. M., Lindblom, R. S. J., Ziemann, M., Laskowski, A., Granata, C., Snelson, M., et al. (2021). Targeting methylglyoxal in diabetic kidney disease using the mitochondria-targeted compound MitoGamide. Nutrients 13, 1457. doi:10.3390/nu13051457
Tang, C., Cai, J., Yin, X. M., Weinberg, J. M., Venkatachalam, M. A., and Dong, Z. (2021). Mitochondrial quality control in kidney injury and repair. Nat. Rev. Nephrol. 17, 299–318. doi:10.1038/s41581-020-00369-0
Tang, X., Huang, M., Jiang, J., Liang, X., Li, X., Meng, R., et al. (2020). Panax notoginseng preparations as adjuvant therapy for diabetic kidney disease: A systematic review and meta-analysis. Pharm. Biol. 58, 138–145. doi:10.1080/13880209.2020.1711782
Tapia, E., Soto, V., Ortiz-Vega, K. M., Zarco-Márquez, G., Molina-Jijón, E., Cristóbal-García, M., et al. (2012). Curcumin induces Nrf2 nuclear translocation and prevents glomerular hypertension, hyperfiltration, oxidant stress, and the decrease in antioxidant enzymes in 5/6 nephrectomized rats. Oxid. Med. Cell Longev. 2012, 269039. doi:10.1155/2012/269039
Tovar-Palacio, C., Noriega, L. G., and Mercado, A. (2022). Potential of polyphenols to restore SIRT1 and NAD+ metabolism in renal disease. Nutrients 14, 653. doi:10.3390/nu14030653
Trujillo, J., Chirino, Y. I., Molina-Jijón, E., Andérica-Romero, A. C., Tapia, E., and Pedraza-Chaverrí, J. (2013). Renoprotective effect of the antioxidant curcumin: Recent findings. Redox Biol. 1, 448–456. doi:10.1016/j.redox.2013.09.003
Trumbeckaite, S., Pauziene, N., Trumbeckas, D., Jievaltas, M., and Baniene, R. (2017). Caffeic acid phenethyl ester reduces ischemia-induced kidney mitochondrial injury in rats. Oxid. Med. Cell Longev. 2017, 1697018. doi:10.1155/2017/1697018
Tseng, A. H., Shieh, S. S., and Wang, D. L. (2013). SIRT3 deacetylates FOXO3 to protect mitochondria against oxidative damage. Free Radic. Biol. Med. 63, 222–234. doi:10.1016/j.freeradbiomed.2013.05.002
Ugur, S., Ulu, R., Dogukan, A., Gurel, A., Yigit, I. P., Gozel, N., et al. (2015). The renoprotective effect of curcumin in cisplatin-induced nephrotoxicity. Ren. Fail 37, 332–336. doi:10.3109/0886022x.2014.986005
Ungvari, Z., Sonntag, W. E., De Cabo, R., Baur, J. A., and Csiszar, A. (2011). Mitochondrial protection by resveratrol. Exerc Sport Sci. Rev. 39, 128–132. doi:10.1097/JES.0b013e3182141f80
Uzayisenga, R., Ayeka, P. A., and Wang, Y. (2014). Anti-diabetic potential of panax notoginseng saponins (PNS): A review. Phytother. Res. 28, 510–516. doi:10.1002/ptr.5026
Vijayan, A. (2021). Tackling AKI: Prevention, timing of dialysis and follow-up. Nat. Rev. Nephrol. 17, 87–88. doi:10.1038/s41581-020-00390-3
Wang, B., Chen, S., Yan, X., Li, M., Li, D., Lv, P., et al. (2015a). The therapeutic effect and possible harm of puerarin for treatment of stage III diabetic nephropathy: A meta-analysis. Altern. Ther. Health Med. 21, 36–44.
Wang, H., Guan, Y., Karamercan, M. A., Ye, L., Bhatti, T., Becker, L. B., et al. (2015b). Resveratrol rescues kidney mitochondrial function following hemorrhagic shock. Shock 44, 173–180. doi:10.1097/shk.0000000000000390
Wang, J., Zhu, H., Yang, Z., and Liu, Z. (2013). Antioxidative effects of hesperetin against lead acetate-induced oxidative stress in rats. Indian J. Pharmacol. 45, 395–398. doi:10.4103/0253-7613.115015
Wang, T., Jian, Z., Baskys, A., Yang, J., Li, J., Guo, H., et al. (2020a). MSC-derived exosomes protect against oxidative stress-induced skin injury via adaptive regulation of the NRF2 defense system. Biomaterials 257, 120264. doi:10.1016/j.biomaterials.2020.120264
Wang, W., Sun, W., Cheng, Y., Xu, Z., and Cai, L. (2019). Role of sirtuin-1 in diabetic nephropathy. J. Mol. Med. Berl. 97, 291–309. doi:10.1007/s00109-019-01743-7
Wang, X., Tang, Y., Xie, N., Bai, J., Jiang, S., Zhang, Y., et al. (2022). Salidroside, a phenyl ethanol glycoside from Rhodiola crenulata, orchestrates hypoxic mitochondrial dynamics homeostasis by stimulating Sirt1/p53/Drp1 signaling. J. Ethnopharmacol. 293, 115278. doi:10.1016/j.jep.2022.115278
Wang, Y., Cai, J., Tang, C., and Dong, Z. (2020b). Mitophagy in acute kidney injury and kidney repair. Cells 9, 338. doi:10.3390/cells9020338
Wang, Y., Feng, F., Liu, M., Xue, J., and Huang, H. (2018). Resveratrol ameliorates sepsis-induced acute kidney injury in a pediatric rat model via Nrf2 signaling pathway. Exp. Ther. Med. 16, 3233–3240. doi:10.3892/etm.2018.6533
Wang, Y., Wang, B., Du, F., Su, X., Sun, G., Zhou, G., et al. (2015c). Epigallocatechin-3-Gallate attenuates oxidative stress and inflammation in obstructive nephropathy via NF-κB and Nrf2/HO-1 signalling pathway regulation. Basic Clin. Pharmacol. Toxicol. 117, 164–172. doi:10.1111/bcpt.12383
Weinberg, J. M. (2011). Mitochondrial biogenesis in kidney disease. J. Am. Soc. Nephrol. 22, 431–436. doi:10.1681/asn.2010060643
Wu, L., Li, Q., Liu, S., An, X., Huang, Z., Zhang, B., et al. (2019). Protective effect of hyperoside against renal ischemia-reperfusion injury via modulating mitochondrial fission, oxidative stress, and apoptosis. Free Radic. Res. 53, 727–736. doi:10.1080/10715762.2019.1623883
Xia, N., Daiber, A., Förstermann, U., and Li, H. (2017). Antioxidant effects of resveratrol in the cardiovascular system. Br. J. Pharmacol. 174, 1633–1646. doi:10.1111/bph.13492
Xiao, L., Xu, X., Zhang, F., Wang, M., Xu, Y., Tang, D., et al. (2017). The mitochondria-targeted antioxidant MitoQ ameliorated tubular injury mediated by mitophagy in diabetic kidney disease via Nrf2/PINK1. Redox Biol. 11, 297–311. doi:10.1016/j.redox.2016.12.022
Xie, W., Zhu, T., Zhou, P., Xu, H., Meng, X., Ding, T., et al. (2020). Notoginseng leaf triterpenes ameliorates OGD/R-Induced neuronal injury via SIRT1/2/3-foxo3a-MnSOD/PGC-1α signaling pathways mediated by the NAMPT-NAD pathway. Oxid. Med. Cell Longev. 2020, 7308386. doi:10.1155/2020/7308386
Xu, J. J., Cui, J., Lin, Q., Chen, X. Y., Zhang, J., Gao, E. H., et al. (2021). Protection of the enhanced Nrf2 deacetylation and its downstream transcriptional activity by SIRT1 in myocardial ischemia/reperfusion injury. Int. J. Cardiol. 342, 82–93. doi:10.1016/j.ijcard.2021.08.007
Xu, S., Gao, Y., Zhang, Q., Wei, S., Chen, Z., Dai, X., et al. (2016a). SIRT1/3 activation by resveratrol attenuates acute kidney injury in a septic rat model. Oxid. Med. Cell Longev. 2016, 7296092. doi:10.1155/2016/7296092
Xu, S., Jia, P., Fang, Y., Jin, J., Sun, Z., Zhou, W., et al. (2022). Nuclear farnesoid X receptor attenuates acute kidney injury through fatty acid oxidation. Kidney Int. 101, 987–1002. doi:10.1016/j.kint.2022.01.029
Xu, X., Zheng, N., Chen, Z., Huang, W., Liang, T., and Kuang, H. (2016b). Puerarin, isolated from Pueraria lobata (Willd.), protects against diabetic nephropathy by attenuating oxidative stress. Gene 591, 411–416. doi:10.1016/j.gene.2016.06.032
Xu, Y., Nie, L., Yin, Y. G., Tang, J. L., Zhou, J. Y., Li, D. D., et al. (2012). Resveratrol protects against hyperglycemia-induced oxidative damage to mitochondria by activating SIRT1 in rat mesangial cells. Toxicol. Appl. Pharmacol. 259, 395–401. doi:10.1016/j.taap.2011.09.028
Xue, H., Li, P., Luo, Y., Wu, C., Liu, Y., Qin, X., et al. (2019). Salidroside stimulates the Sirt1/PGC-1α axis and ameliorates diabetic nephropathy in mice. Phytomedicine 54, 240–247. doi:10.1016/j.phymed.2018.10.031
Yan, W., Xu, Y., Yuan, Y., Tian, L., Wang, Q., Xie, Y., et al. (2017). Renoprotective mechanisms of Astragaloside IV in cisplatin-induced acute kidney injury. Free Radic. Res. 51, 669–683. doi:10.1080/10715762.2017.1361532
Yang, Q., Wang, P., Cui, J., Wang, W., Chen, Y., and Zhang, T. (2016). Panax notoginseng saponins attenuate lung cancer growth in part through modulating the level of Met/miR-222 axis. J. Ethnopharmacol. 193, 255–265. doi:10.1016/j.jep.2016.08.040
Yao, J., Wang, J., Xu, Y., Guo, Q., Sun, Y., Liu, J., et al. (2022). CDK9 inhibition blocks the initiation of PINK1-PRKN-mediated mitophagy by regulating the SIRT1-FOXO3-BNIP3 axis and enhances the therapeutic effects involving mitochondrial dysfunction in hepatocellular carcinoma. Autophagy 18, 1879–1897. doi:10.1080/15548627.2021.2007027
Ye, J., Li, J., Yu, Y., Wei, Q., Deng, W., and Yu, L. (2010). L-carnitine attenuates oxidant injury in HK-2 cells via ROS-mitochondria pathway. Regul. Pept. 161, 58–66. doi:10.1016/j.regpep.2009.12.024
Yeam, C. T., Yo, T. E., Tan, Y. L. C., Liew, A., and Seng, J. J. B. (2021). Complementary and alternative medicine therapies for uremic pruritus - a systematic review of randomized controlled trials. Complement. Ther. Med. 56, 102609. doi:10.1016/j.ctim.2020.102609
Yu, B. C., Cho, N. J., Park, S., Kim, H., Choi, S. J., Kim, J. K., et al. (2019). IgA nephropathy is associated with elevated urinary mitochondrial DNA copy numbers. Sci. Rep. 9, 16068. doi:10.1038/s41598-019-52535-5
Zeng, Z., Chen, Z., Xu, S., Zhang, Q., Wang, X., Gao, Y., et al. (2016). Polydatin protecting kidneys against hemorrhagic shock-induced mitochondrial dysfunction via SIRT1 activation and p53 deacetylation. Oxid. Med. Cell Longev. 2016, 1737185. doi:10.1155/2016/1737185
Zhang, C., Song, Y., Chen, L., Chen, P., Yuan, M., Meng, Y., et al. (2022a). Urolithin A attenuates hyperuricemic nephropathy in fructose-fed mice by impairing STING-NLRP3 axis-mediated inflammatory response via restoration of parkin-dependent mitophagy. Front. Pharmacol. 13, 907209. doi:10.3389/fphar.2022.907209
Zhang, J., Li, R., Man, K., and Yang, X. B. (2022b). Enhancing osteogenic potential of hDPSCs by resveratrol through reducing oxidative stress via the Sirt1/Nrf2 pathway. Pharm. Biol. 60, 501–508. doi:10.1080/13880209.2022.2037664
Zhang, Q., Zhang, C., Ge, J., Lv, M. W., Talukder, M., Guo, K., et al. (2020). Ameliorative effects of resveratrol against cadmium-induced nephrotoxicity via modulating nuclear xenobiotic receptor response and PINK1/Parkin-mediated Mitophagy. Food Funct. 11, 1856–1868. doi:10.1039/c9fo02287b
Zhang, T., Chi, Y., Ren, Y., Du, C., Shi, Y., and Li, Y. (2019). Resveratrol reduces oxidative stress and apoptosis in podocytes via sir2-related enzymes, Sirtuins1 (SIRT1)/Peroxisome proliferator-activated receptor γ Co-activator 1α (PGC-1α) Axis. Med. Sci. Monit. 25, 1220–1231. doi:10.12659/msm.911714
Zhang, X., Agborbesong, E., and Li, X. (2021a). The role of mitochondria in acute kidney injury and chronic kidney disease and its therapeutic potential. Int. J. Mol. Sci. 22, 11253. doi:10.3390/ijms222011253
Zhang, X., Feng, J., Li, X., Wu, D., Wang, Q., Li, S., et al. (2021b). Mitophagy in diabetic kidney disease. Front. Cell Dev. Biol. 9, 778011. doi:10.3389/fcell.2021.778011
Zhang, Y., Liu, M., Zhang, Y., Tian, M., Chen, P., Lan, Y., et al. (2022c). Urolithin A alleviates acute kidney injury induced by renal ischemia reperfusion through the p62-Keap1-Nrf2 signaling pathway. Phytother. Res. 36, 984–995. doi:10.1002/ptr.7370
Zhao, H. Y., Li, H. Y., Jin, J., Jin, J. Z., Zhang, L. Y., Xuan, M. Y., et al. (2021a). L-carnitine treatment attenuates renal tubulointerstitial fibrosis induced by unilateral ureteral obstruction. Korean J. Intern Med. 36, S180–s195. doi:10.3904/kjim.2019.413
Zhao, M., Wang, Y., Li, L., Liu, S., Wang, C., Yuan, Y., et al. (2021b). Mitochondrial ROS promote mitochondrial dysfunction and inflammation in ischemic acute kidney injury by disrupting TFAM-mediated mtDNA maintenance. Theranostics 11, 1845–1863. doi:10.7150/thno.50905
Zhao, N., Xia, J., and Xu, B. (2021c). Physical exercise may exert its therapeutic influence on Alzheimer's disease through the reversal of mitochondrial dysfunction via SIRT1-FOXO1/3-PINK1-Parkin-mediated mitophagy. J. Sport Health Sci. 10, 1–3. doi:10.1016/j.jshs.2020.08.009
Zheng, Y., Shi, B., Ma, M., Wu, X., and Lin, X. (2019). The novel relationship between Sirt3 and autophagy in myocardial ischemia-reperfusion. J. Cell Physiol. 234, 5488–5495. doi:10.1002/jcp.27329
Zhou, Y. X., Zhang, H., and Peng, C. (2014). Puerarin: A review of pharmacological effects. Phytother. Res. 28, 961–975. doi:10.1002/ptr.5083
Zymone, K., Benetis, R., Trumbeckas, D., Baseviciene, I., and Trumbeckaite, S. (2022). Different effects of quercetin glycosides and quercetin on kidney mitochondrial function-uncoupling, cytochrome C reducing and antioxidant activity. Molecules 27, 6377. doi:10.3390/molecules27196377
Glossary
Ac acetyl
acetyl-CoA acetyl-coenzyme A
AKI acute kidney injury
AMBRA1 activating molecule in BECN1-regulated autophagy protein 1
AMPK AMP-activated protein kinase
ARE antioxidant response element
ATP adenosine triphosphate
BAX Bcl-2-associated X protein
BINP3 B-cell lymphoma 2/adenovirus E1B 19-kDa-interacting protein 3
BINP3L BINP3-like
BUN blood urea nitrogen
CAPE caffeic acid phenethyl ester
CKD chronic kidney diseases
CPT1 carnitine O-palmitoyltransferase 1
CPT2 carnitine O-palmitoyltransferase 2
Cytc cytochrome c
DKD diabetic kidney disease
DRP1 dynamin related protein 1
EGCG epigallocatechin 3-gallate
ERRs estrogen-related receptors
ESRD end-stage renal diseases
ETC electron transport chain
FAO fatty acid β-oxidation
FIS1 fission 1 protein
FoxO3a forkhead box class O 3a
FUNDC1 FUN14 domain-containing protein 1
GSSG oxidized GSSH
GSH glutathione
GSPE grape seed proanthocyanidin extract
GPX glutathione peroxidases
HIF-1α hypoxia-inducible factor-1α
IMM inner mitochondrial membrane
IRI ischemia-reperfusion injury
Keap1 kelch-like erythroid cell-derived protein with CNC homology-associated protein 1
LKB1 liver kinase B1
MiD49 mitochondrial dynamics proteins of 49
MFF mitochondrial fission factor
Mfn1 mitofusin 1
mPTP mitochondrial permeability transition pore
MQC Mitochondrial quality control
NAD+ nicotinamide adenine dinucleotide
Nrf2 nuclear factor-erythroid-derived 2-like 2
NRF nuclear respiratory factors
OMM outer mitochondrial membrane
OPA1 optic atrophy 1
OXPHOS oxidative phosphorylation
Parkin parkin RBR E3 ubiquitin-protein ligase
PA-S14 piericidin analogue S14
PINK1 PTEN-induced kinase 1
PGC-1α PPARγ coactivator-1α
PPARα peroxisome proliferator–activated receptor α
PNS panax notoginseng saponins
PPARγ peroxisome proliferator-activated receptor-γ
ROS reactive oxygen species
SIRT1 sirtuin-1
SIRT3 sirtuin-3
SLC25A20 carnitine–acylcarnitine translocase
Scr serum creatinine
SOD superoxide dismutase
TCA tricarboxylic acid
TECs tubular epithelial cells
TFAM mitochondrial transcription factor A
Ub ubiquitin
UIRI unilateral ischemia-reperfusion injury
UUO unilateral ureteral obstruction.
Keywords: natural products, mitochondrial dysfunction, energy metabolism, kidney diseases, mitochondrial homeostasis
Citation: Huang J, Liang Y and Zhou L (2023) Natural products for kidney disease treatment: Focus on targeting mitochondrial dysfunction. Front. Pharmacol. 14:1142001. doi: 10.3389/fphar.2023.1142001
Received: 11 January 2023; Accepted: 06 March 2023;
Published: 15 March 2023.
Edited by:
Xiaoxin Wang, Georgetown University Medical Center, United StatesReviewed by:
Anil Bhanudas Gaikwad, Birla Institute of Technology and Science, IndiaKomuraiah Myakala, Georgetown University, United States
Copyright © 2023 Huang, Liang and Zhou. This is an open-access article distributed under the terms of the Creative Commons Attribution License (CC BY). The use, distribution or reproduction in other forums is permitted, provided the original author(s) and the copyright owner(s) are credited and that the original publication in this journal is cited, in accordance with accepted academic practice. No use, distribution or reproduction is permitted which does not comply with these terms.
*Correspondence: Lili Zhou, jinli730@smu.edu.cn
†These authors have contributed equally to this work