- 1Institute of Feed Research, Chinese Academy of Agricultural Sciences, Beijing, China
- 2Key Laboratory of Feed Biotechnology, Ministry of Agriculture and Rural Affairs, Beijing, China
- 3State Key Laboratory of Pathogen and Biosecurity, Beijing Institute of Biotechnology, Beijing, China
With the rapid advancement of genetic and protein engineering, proteins and peptides have emerged as promising drug molecules for therapeutic applications. Consequently, there has been a growing interest in the field of chemical modification technology to address challenges associated with their clinical use, including rapid clearance from circulation, immunogenicity, physical and chemical instabilities (such as aggregation, adsorption, deamination, clipping, oxidation, etc.), and enzymatic degradation. Polyethylene glycol (PEG) modification offers an effective solution to these issues due to its favorable properties. This review presents recent progress in the development and application of PEGylated therapeutic proteins and peptides (TPPs). For this purpose, firstly, the physical and chemical properties as well as classification of PEG and its derivatives are described. Subsequently, a detailed summary is provided on the main sites of PEGylated TPPs and the factors that influence their PEGylation. Furthermore, notable instances of PEG-modified TPPs (including antimicrobial peptides (AMPs), interferon, asparaginase and antibodies) are highlighted. Finally, we propose the chemical modification of TPPs with PEG, followed by an analysis of the current development status and future prospects of PEGylated TPPs. This work provides a comprehensive literature review in this promising field while facilitating researchers in utilizing PEG polymers to modify TPPs for disease treatment.
1 Introduction
With the development of gene recombinant technology, therapeutic proteins and peptides (TPPs) currently account for 10% of the global pharmaceutical market and are projected to exceed US$70 billion annually due to their distinctive attributes, including high specificity, potent bioactivity, and minimal side effects (Wang et al., 2002; Leader et al., 2008; Manning et al., 2010; Du et al., 2015). The Food and Drug Administration (FDA) has granted clinical approval for more than 239 medicinal TPPs. However, despite their increasing utilization in clinical practice, most TPPs suffer from several drawbacks such as low solubility, poor stability, short half-life, and high immunogenicity that compromise their efficacy and restrict their therapeutic applications (Veronese and Pasut, 2005). Chemical modification emerges as a robust strategy to enhance the stability, solubility, and reduce the immunogenicity profile of TPPs (Braman, 2002; Sung et al., 2003; Qin et al., 2005; Veronese and Mero, 2008). Notably, significant attention has been directed towards developing novel polymers aimed at improving the properties of TPPs.
The linear polymer polyethylene glycol (PEG) is composed of repeated ethylene glycol units [-(O-CH2-CH2)n] and has a molecular weight (MW) range of 0.4–150 kDa. It has been extensively used in the pharmaceutical industry for several decades due to its favorable properties, such as high hydrophilicity, low viscosity, non-immunogenicity, and excellent biocompatibility (Guichard et al., 2017; Zuma et al., 2022a). The covalent conjugation of PEG to TPPs plays a crucial role in regulating and determining the structure, function, activity, immunogenicity, and pharmacokinetic profiles of drug molecules. In the late 1970s, PEG was initially employed to modify bovine serum albumin (BSA), resulting in improved immunological and soluble properties compared to its unmodified form (Abuchowski et al., 1977). In the subsequent two or three decades, PEG modification technology has undergone rapid development to prolong the biological half-life of TPPs, diminish their immunogenicity, and promote their stability, therapeutic efficacy, as well as accumulation in target organs or cells through improved permeability and retention effects (Knauf et al., 1988; Nakaoka et al., 1997; Chapman, 2002; Basu et al., 2006; Treetharnmathurot et al., 2008; Nojima et al., 2009b; Park et al., 2010; Erak et al., 2018; Guichard et al., 2018). It has been demonstrated that PEGylated interferon λ (PEG-IFN-λ) exhibits no inflammatory side effects or broad-spectrum antiviral activity both in vitro or in vivo, including against hepatitis and symptomatic coronavirus disease 2019 (COVID-19) (Leader et al., 2008; Manning et al., 2010; Du et al., 2015). Reis et al. evaluated the effectiveness of PEG-IFN-λ in preventing COVID-19 and reported a 41% reduction in time to COVID-19-related deaths or hospitalizations following administration of a single subcutaneous injection containing 180 μg of PEG-IFN-λ (Manning et al., 2010). Currently, PEG-IFN-λ1 is the sole available IFN-λ therapeutic agent. The use of PEGylated IFN-λ significantly decreases viral loads among patients with acute COVID-19, and it may serve as an effective therapeutic agent against this disease (Reis et al., 2023). Moreover, pegilodecakin (PEGylated interleukin-10) has been demonstrated to play a pivotal role in the inhibition of tumor growth and metastasis (Autio and Oft, 2019; Tannir et al., 2021). Recently, several PEGylated antimicrobial peptides (AMPs), such as OM19r-8, N6, Onc72, SAAP-148, etc., have shown potential in enhancing resistance against proteolytic enzymes, promoting antibacterial/immunomodulatory activities, and prolonging in vivo half-time (Cui et al., 2021; Li et al., 2022; Mohammed et al., 2023; Van Gent et al., 2023). In general, PEGylation of TPPs offers various advantages for overall efficacy. These include improving solubility, stability, permeability, and pharmacokinetic properties, while reducing glomerular filtration clearance rate, immunogenicity, and toxicity. Additionally, it extends drug circulation time (Figure 1) (Knauf et al., 1988; Nakaoka et al., 1997; Chapman, 2002; Basu et al., 2006; Nojima et al., 2009b; Park et al., 2010; Li et al., 2022). However, the widespread application of PEGylated TPPs also presents certain potential drawbacks, such as diminished bioactivity, poor bioavailability and limited biodegradability due to waxy behavior or the use of unnatural PEG polymers (Figure 1) (Witt et al., 2001; Imura et al., 2007a; Armstrong et al., 2007; Schlapschy et al., 2013; Ko and Maynard, 2018; Cao et al., 2021; Li et al., 2022).
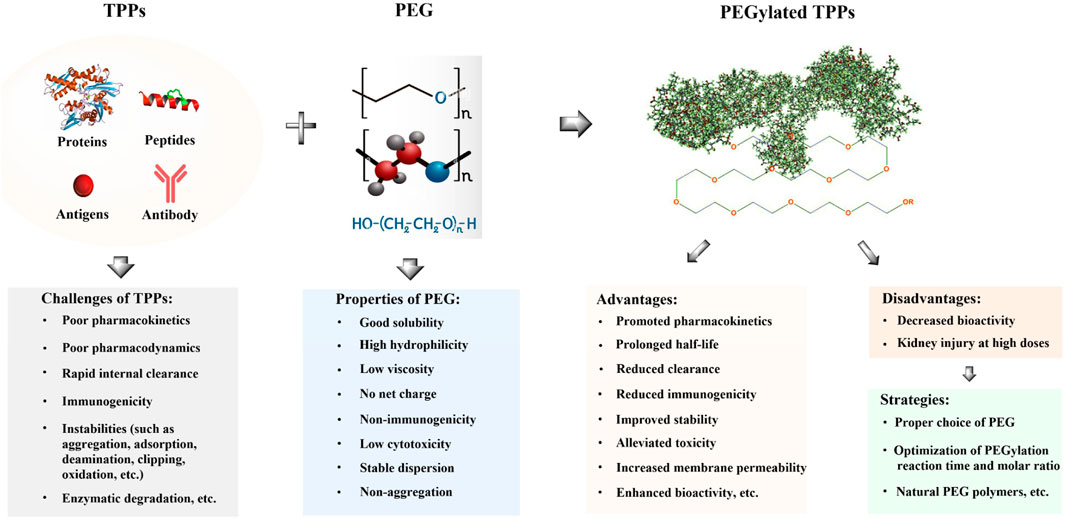
FIGURE 1. Some advantages and disadvantages of PEGylated TPPs. Challenges in the clinical application of TPPs include their short half-life, poor pharmacokinetics, instabilities, and immunogenicity, which limit their therapeutic effectiveness. PEGylation can enhance the solubility and membrane permeability of TPPs through the hydrophilicity of PEG molecules and improve their pharmacokinetics and efficacy by increasing accumulation in target organs or sites (Levy et al., 1988; Casey et al., 2000; Guiotto et al., 2003; Chae et al., 2009; Singh et al., 2014; Cui et al., 2021; Li et al., 2022). Additionally, PEG modification can reduce clearance by the reticuloendothelial system and immunogenicity by shielding from proteolytic enzymes while decreasing cytotoxicity (Vugmeyster et al., 2012; Benincasa et al., 2015). Moreover, PEGylation of TPPs may promote membrane permeability (Tan et al., 2019; Li et al., 2022). However, a major disadvantage of TPPs is the potential loss of bioactivity or function following PEGylation due to significant conformational changes involved. Subcutaneous administration of PEGylated TPPs may result in low bioavailability due to their waxy nature, and unnatural PEG polymers used can contribute to poor biodegradability. To address these issues, several solutions exist including careful selection appropriate PEG molecules, optimization of reaction conditions for PEGylation (including temperature, time, pH, molar ratio, etc.), and utilization of natural PEG polymers (Witt et al., 2001; Armstrong et al., 2007; Imura et al., 2007b; Schlapschy et al., 2013; Cao et al., 2021; Li et al., 2022).
Several studies have comprehensively reviewed various aspects of PEGylation, focusing on the technology and modification of non-TPP drugs. In this study, we conducted a comprehensive survey on PEGylated TPPs, providing detailed insights into the physicochemical properties and classification of PEG and its derivatives, sites modified by PEGylation in TPPs, as well as factors influencing the PEG modification process. Furthermore, we highlighted typical cases and current developments in the field of PEGylated TPPs, followed by an analysis of the potential application of this technology in TPPs.
2 Physicochemical properties and classification of PEG and its derivatives
2.1 Chemical structure of PEG and its derivatives
PEG is a linear polymer with hydroxyl groups at both ends, which is formed by gradually adding ethylene oxide to water or ethylene glycol. It consists of repeated oxyethylene units. The simplest structure of PEG is a straight-chain hydroxyl-terminated polyether represented by the following structural formula: HO-(CH2CH2O)n-CH2CH2-OH (Roberts et al., 2002) (Figure 2). The terminal hydroxyl group of PEG serves as the functional group in chemical modification reactions, but its reactivity is low; therefore, it needs to be activated for modifying TPPs. Monomethoxy PEG (mPEG) is commonly used for TPP modification, and its general structure can be depicted as CH3O–(CH2CH2O)n-CH2CH2-OH (Figure 2). mPEG is obtained by blocking one end hydroxyl group of PEG with a methyl group to prevent crosslinking and agglomeration with TPPs during the modification process (Zhang et al., 2007).
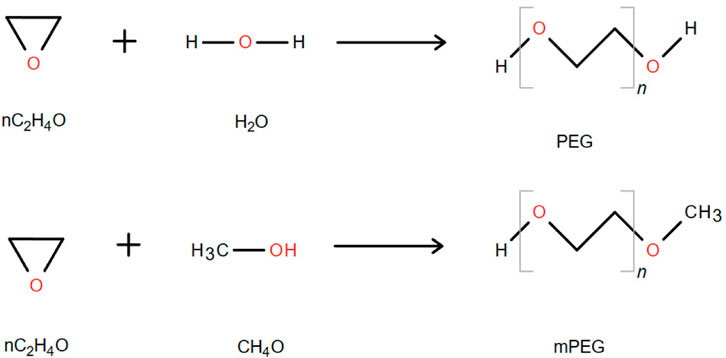
FIGURE 2. Synthetic chemical formula of PEG and monomethoxy PEG. “n” represents the average number of repeating oxyethylene groups (Zhang et al., 2007).
2.2 Biological properties of PEG and its derivatives
The MWs of linear and branched chain PEGs used in TPPs typically range from 3 to 60 kDa, which is larger compared to nanomedicines like Doxil and mRNA-based COVID-19 vaccines that have a size of 2 kDa (Na et al., 2008; Shi et al., 2022). PEG exhibits hydration in aqueous solution, with each ethane oxide unit (-CH2-CH2-O-) binding 2–3 water molecules. Consequently, the apparent MW of PEG in solution is significantly higher than that of proteins or peptides with the same MW (Antonsen and Hoffman, 1992). Youn et al. (2008) used PEGylated salmon calcitonin (sCT) with a relative MW of 5 kDa and observed that the MW of sCT modified with PEG5000 reached as high as 259 kDa, for exceeding its actual MW of 84 kDa. This property not only prolongs the circulating half-life of PEGylated protein drugs but also enhances their stability in solution. Moreover, PEG demonstrates good solubility in water and most organic solvents while being insoluble in ether and aliphatic hydrocarbons, making it an amphiphilic molecule (Henning, 2002). Interestingly, the immunogenicity of PEGylated TPPs may be reduced due to steric hindrance caused by PEG, which can impede immune recognition (Harris et al., 2001; Shi et al., 2022). For instance, PEGylation of asparaginase could eliminate its antigenicity (Abuchowski et al., 1977; Duval et al., 2002; Molineux, 2003; Wang et al., 2012).
2.3 Classification of PEG derivatives
With the development of biotechnology, PEG derivatives have been categorized into three distinct generations based on their MWs (Table 1; Figure 3). The first-generation of low MW PEG derivatives primarily involved coupling with the amino group of TPPs (Miron and Wilchek, 1993). Notable examples from this first generation include PEG succinimide carbonate (PEG-SC), PEG benzotriazole carbonate (PEG-BTC), PEG dichlorotriazine, PEG tresylate, PEG p-nitrophenyl carbonate, and PEG trichlorophen. Among these, both PEG-SC (Zalipsky et al., 1992) and PEG-BTC are extensively employed in TPPs (Dolence et al., 1997) as they selectively react with lysine residues within protein molecules to form carbamates. Additionally, Adagen (PEG-ademase bovine), OncoSpar (PEG-aspargase), and PEG-Intron (PEG-interferon α-2b) were also modified using linear PEG from the first generation (Veronese and Pasut, 2005). However, first-generation PEG derivatives often exhibit weak interactions upon conjugation with proteins and undergo side reactions with protein drugs. Consequently, numerous issues arise, including the formation of multiple side reaction products (Abuchowski et al., 1977) and degradation products (Gais and Ruppert, 1995), as well as difficulties in separating modified products (Zalipsky et al., 1992). Furthermore, these derivatives display poor stability, high toxicity, and inadequate homogeneity. In contrast, second-generation PEG derivatives employ more efficient functional groups such as aldehydes, esters, and amides for specific and functional chemical modifications (Wang et al., 2011). For instance, Kinstler et al. (1996) initially discovered PEG-aldehyde derivatives to achieve site-specific modification of the N-terminal amino group of polypeptides. Second-generation PEG derivatives can also accomplish targeted modifications of sulfhydryl groups (Brocchini et al., 2008); commonly used sulfhydryl-modified PEG derivatives include PEG-maleimide (MAL-PEG), PEG-vinyl sulfone (VS-PEG), etc. (Pasut and Veronese, 2012). As the field of PEGylation chemistry advances further, there is a growing demand for heterobifunctional PEG derivatives. Yoo et al. (2012) conjugated folate (FA) to one end of PEG and superparamagnetic iron oxide nanoparticles (SPIONs) to the other end, resulting in the formation of FA-PEG-SPIONs. The introduction of biocompatible PEG into the drug mixture conferred excellent biocompatibility on FA-PEG-SPIONs, including low cytotoxicity, stable dispersion, non-aggregation, and strong optical imaging ability in a mouse model of lung cancer. The third generation of PEG derivatives was developed as branched PEG derivatives, such as tree-type PEG, Y-type PEG, and comb-type PEG, etc.) (Figure 3) (Zhang et al., 2012). Vugmeyster et al. (2012) performed site-specific modification of the antitumor necrosis factor alpha (TNF-α) nano antibody using linear, Y-shaped, and tree-shaped PEG, respectively. Among them, the two-arms branching structure exhibited the longest half-life in rats.
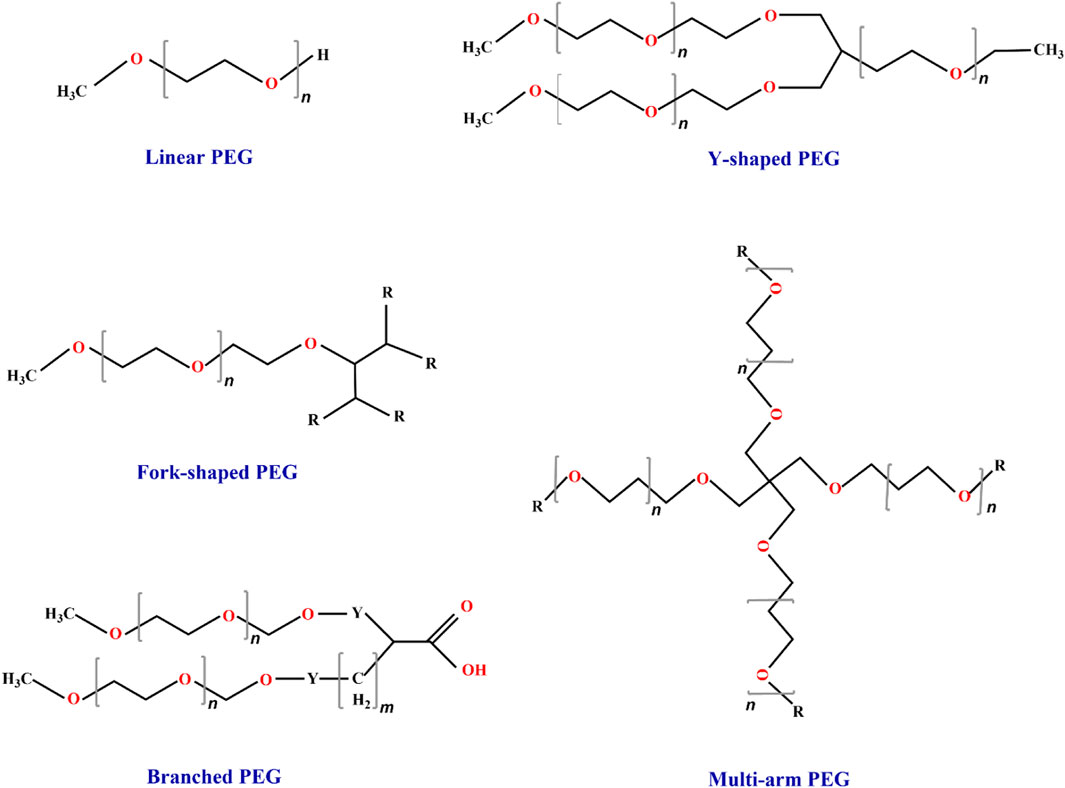
FIGURE 3. Classification of PEG and its derivatives. The market currently utilizes five types of PEG materials, namely, linear, Y-shaped, multiarm, fork-shaped, and branched materials. Among these options, linear PEG is commonly employed for the PEGylation of TPPs (Kolate et al., 2014; Lu and Zhang, 2018). “n” represents the average number of repeating oxyethylene groups.
3 PEG-modified sites on TPPs
PEG can be covalently conjugated to specific sites in protein drugs, and the common modification sites of TPPs are illustrated in Figure 4 (Pasut and Veronese, 2012). Different activated PEGs were chosen for various modified groups. The modification reactions involving PEG and TPPs exhibit distinct properties, including acylation, alkylation, redox, and aromatic ring substitution. PEG chemically modifies side chain groups such as amino, sulfhydryl and carboxyl groups of TPPs (Herman et al., 1995; Veronese, 2001). This review discusses the methodologies employed for amino, sulfhydryl, and carboxyl group modifications in PEG-conjugated TPPs along with their advantages and disadvantages (Table 2).
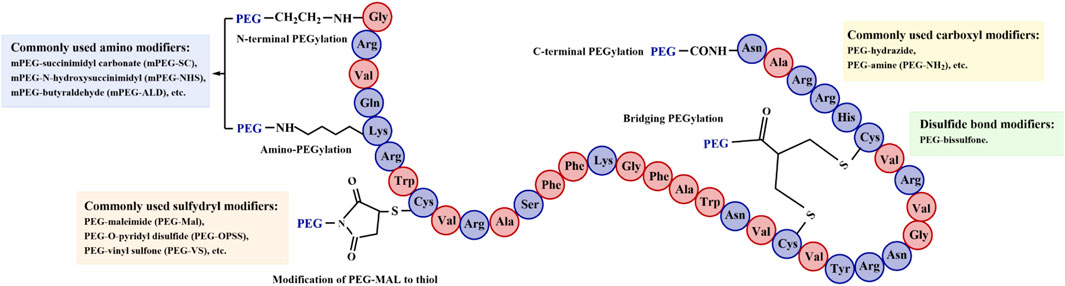
FIGURE 4. PEG-modified sites in TPPs. The modification reactions can be categorized into acylation, alkylation, redox, and aromatic ring substitution. Theses reactions involve N-terminal PEGylation, C-terminal PEGylation, amino PEGylation, sulfhydryl PEGylation, and bridging PEGylation (Herman et al., 1995; Veronese, 2001; Pasut and Veronese, 2012).
3.1 Site-modified amino
The groups involved in the PEGylation of TPPs typically consist of α- and ε-amino groups. Therefore, the PEGylation of amino groups in TPPs can be categorized into N-terminal α-NH2 modification and lysine ε-NH2 modification. Lysine is one of the most prevalent amino acids in TPPs, usually located on the surface of protein molecules’ three-dimensional structure, making it easily accessible for conjugation with PEG modifiers. A unique two-step site-specific PEGylation method was employed to produce Lys21-amine PEGylated growth hormone-releasing factor (GRF) (1–29) through a newly devised site-specific PEGylation process using a 9-fluorenylmethoxycarbonyl (FMOC)-protection/deprotection method at N-α Tyr1 and Lys12 (Youn and Lee, 2007). The His7-(N-terminus), Lys26-or Lys34-amine specific PEGylation of glucagon-like peptide-1 (GLP-1) was prepared by a site-specific PEGylation process using a maleic anhydride (MA)-protection/deprotection method (Youn et al., 2007a). Similarly, Lys18-, Cys1-or Lys11-amine mono-PEGylated salmon calcitonin (sCT) was generated using an FMOC protection/deprotection technique (Youn et al., 2007b). This strategy resulted in higher production yield, longer half-lives, and improved biological stability compared to conventional non-specific PEGylation (Youn et al., 2007a; Youn et al., 2007b; Youn and Lee, 2007). Regarding the N-terminal α-NH2 modification, the pKa of the protein’s N-terminal α-NH2 is typically around 7.6 to 8.0, while the pKa of lysine ε-NH2 is generally in the range of 10.0–10.2. Therefore, precise modification of the N-terminal α-NH2 can be achieved by controlling pH under acidic conditions (Kinstler et al., 2002). Yamamoto et al. (2003) and Yoshioka et al. (2004), Yoshioka et al. (2011) proposed a novel method for site-specific PEGylation at fixed points on TPPs that lack lysine residues entirely, such as TNF-α. These Lys-deficient TPPs were mono-PEGylated exclusively at their N termini and exhibited enhanced bioactivity in vitro as well as superior antitumor therapeutic efficacy compared to randomly mono-PEGylated wild-type counterparts. Additionally, when a peptide or protein has serine or threonine at its N-terminus, periodic acid can be used to oxidize the functional group into an aldehyde group, enabling subsequent site-directed modification with PEG-hydrazide (Gaertner and Offord, 1996; Zhou et al., 2014).
3.2 Site-modified thiol
Modification of the cysteine-free sulfhydryl group or disulfide bond on the protein surface is a method employed to achieve targeted modifications. Thiol modification of cysteine refers to the introduction of PEG fixed-point modification on proteins containing a single cysteine residue. Examples of site-directed PEG modifications include Cys34 in human serum albumin (HSA) and Cys17 in granulocyte colony-stimulating factor (G-CSF) (Hao et al., 2006; Zhao et al., 2012). For peptides or proteins lacking sulfhydryl groups, site-specific modifications can be achieved through genetic engineering by introducing sulfhydryl groups at appropriate locations. Chi et al. (2008) employed a microwave-assisted solid-phase synthesis technique to synthesize GLP-1 (7–36), wherein Gly and Cys were substituted for Ala at positions 8 and 30, respectively, followed by PEG-modification of Cys at position 30. In the context of disulfide bond modification, the presence of a disulfide bond in a peptide or protein plays a crucial role in stabilizing its secondary and tertiary structure; however, modifying this bond may potentially lead to loss of activity. Hence, the development of highly efficient PEG modifiers and feasible strategies for site-specific disulfide bond modification has become pivotal. Brocchini et al. (2006) devised a dialkylated PEG reagent capable of alkylating both thiols within the reduced disulfide bond to form a three-carbon bridge. By employing such a three-carbon bridge, PEG can be covalently attached to proteins while preserving their biological activity of TPPs (Balan et al., 2007).
3.3 Site-modified carboxyl groups
The side chains of TPPs contain a significant number of carboxyl groups, with modification sites including glutamic acid, aspartic acid, and carboxyl groups at the end. In recent years, mPEG-hydrazine has emerged as a specific modifier capable of binding to carboxyl groups. In the presence of 1-ethyl-3-dimethylaminopropylcarbodiimide hydrochloride (EDC) or dicyclohexylcarbodiimide (DCCI), the carboxyl group can form an amide bond with the amino group of mPEG-NH2 (Gault et al., 2008). Additionally, in acidic conditions (pH 4.5–5.0) and in the presence of EDC, protonation occurs without any cross-linking reactions involving the amino group in proteins or peptides (Veronese, 2001).
3.4 Site-specific modifications of the other groups
Other groups on TPPs can also be utilized as potential sites for PEGylation, such as amino acid residues located at the protein or peptide’s C-terminus (Cazalis et al., 2004), glycosylation sites consisting of serine and threonine residues (Giorgi et al., 2014), and histidine residue (Wylie et al., 2001; Zuma et al., 2022b; Zuma et al., 2022a; Khataminezhad et al., 2023). Both IFN α-2b and IFN β-1b were fused to the Mycobacterium xenopi GyrA intein and expressed in Escherichia coli. They were subsequently cleaved by hydrazine to generate the corresponding IFN α-2b C-terminal hydrazide, followed by site-specific modification with a pyruvyl derivative of PEG with a MW of 10 kDa (Thom et al., 2011). The PEGylated forms of IFN α-2b and IFN β-1b exhibited excellent antiviral activity, indicating that intein technology is compatible with TPPs containing disulfide bonds and can be used for C-terminal PEGylation of other TPPs, including antibody fragments, using PEGs with different MWs. Both domain antibody (dAb) and IFN α-2a tagged with His tags at the C-terminus and the N-terminus underwent site-specific PEGylation at their histidine tags, resulting in extended circulation half-lives (Cong et al., 2012).
Additionally, genetic engineering and other techniques have been employed to introduce specific groups at the C-terminus or N-terminus of proteins or peptides for site-mediated PEGylation using histidine tags (Thom et al., 2011; Cong et al., 2012). Moreover, noncanonical amino acids have also been incorporated into recombinant TPPs for targeted PEGylation (Nischan and Hackenberger, 2014).
4 Factors affecting PEGylation of TPPs
The reaction of PEGylated TPPs can be influenced by various factors, including the selection of an appropriate PEG modifier, the molar ratio between PEG and protein drugs, the optimization of reaction conditions (such as temperature, pH, and time), the MW distribution of PEG, and the structural characteristics of its molecular chains.
4.1 Selection of PEG modifiers
The selection of PEG modifiers should take into account the relative molecular mass (Mn), MW distribution coefficient (PDI), modification sites, functional groups, and molecular chain structure. Previous studies have demonstrated that the reaction time of modified protein drugs is directly proportional to the amount of PEG and Mn conjugated, while the biological activity is inversely proportional to this amount (Chiu et al., 2010; Ma et al., 2013; Morgenstern et al., 2017). However, excessive Mn in PEG-modified protein drugs may lead to a decrease in their overall biological activity (Weinberg et al., 2018). Moreover, it is preferable for PEGylated agents to have a small PDI and a narrower MW distribution as this facilitates separation and purification of PEG-modified TPPs (Chen et al., 2018). When selecting the modification site, it is important to consider the structure‒activity relationship analysis of TPPs. Preferably, residues on the protein surface that do not bind to receptors should be chosen as modification sites for modified TPPs to retain their high biological activity (Dozier and Distefano, 2015).
For the specificity of the modification reaction, it is crucial to select and utilize PEG modifiers with appropriate functional groups, such as cross-functionalized mono-sulfone PEG, in TPPs (Pasut and Veronese, 2012). It should be noted that PEGylated molecules with distinct chain structures of linear and branched chains have various biological characteristics that can affect numerous pharmacokinetic parameters of TPPs (Vugmeyster et al., 2012; Zhang et al., 2012). Branched PEG modifiers exhibit reduced accessibility to hidden sites and enhanced stability against proteolysis compared to linear PEG modifiers (Wan et al., 2017; Sun et al., 2023).
4.2 Molar ratio of PEG to TPPs
Generally, PEG molecules with lower MWs and degrees of PEGylation may lead to higher residual activities of TPPs, while higher PEG MWs and degrees of PEGylation can enhance the conformational and colloidal stability of TPPs (Morgenstern et al., 2017). The increase in the molar ratio of PEG to TPPs, results in elevated relative MWs of the PEG-modified TPPs and modification rate, which affect the biological activity of the TPPs. Behi et al. (2018) conducted modifications on rhG-CSF using different molar ratios of methoxy PEG propionaldehyde (mPEG-ALD) and recombinant human granulocyte colony stimulating factor (rhG-CSF), finding that a molar ratio of 5:1 for mPEG-ALD to protein yielded optimal single-PEGylated rhG-CSF. Chinol et al. (1998) modified avidin (AV) with monomethoxy PEG (mPEG) at various molar ratios, observing a gradual decrease in AV-biotin binding rate as more mPEG was attached to AV. BSA modified with PEG800 exhibited maximum conformational stability at a BSA:PEG molar ratio of 1:0.75 due to surface residue protection and buried hydrophobic residue shielding by PEG (Rawat et al., 2020). Therefore, it is crucial to control the appropriate proportion between PEG and TPPs during modification processes (de Lencastre Novaes et al., 2010).
4.3 Reaction pH and time and TPPs’ concentration
The pH is a critical factor that can influence the modification of PEG-modified TPPs. By controlling the appropriate pH, specific modifications can be made to the amino acid residues in the protein, thereby enhancing modification specificity and reducing separation difficulties. Lactoferrin was modified with N-hydroxysuccinimide-activated PEG (PEG-NHS) to enhance its pharmacokinetic properties (Nojima et al., 2009a). The results demonstrated that pH played a crucial role in achieving optimal conditions for PEG-NHS-modified bovine lactoferrin. While increasing reaction time led to higher modification rates of PEGylated products, it also resulted in increased heterogeneity of the modified products. Laccase was subjected to monomethoxy PEG (20, 30, 40 kDa and 40 kDa-branched) modification durations of 4 h and 17 h, respectively (Mayolo-Deloisa et al., 2015). The optimal reaction time for laccase PEGylation was found to be 4 h at a PEG:protein molar ratio of 4:1; furthermore, laccases modified with 30 kDa linear PEG exhibited greater activity compared to other types of PEGs (Mayolo-Deloisa et al., 2015).
Additionally, the concentration of TPPs plays a crucial role in PEGylation reactions. Koussoroplis et al. (2013) reported that higher concentrations of recombinant human deoxyribonuclease I (rhDNase) result in more efficient PEGylation reactions. When the concentration of rhDNase was 1 mg/mL, and the molar ratio of PEG to protein was 16:1, the reaction time lasted for 96 h (Guichard et al., 2017). However, increasing the concentration of rhDNase to 10 mg/mL resulted in a reduced molar ratio of PEG to protein to 4:1 and a shortened reaction time with overnight incubation (Mahri et al., 2021).
4.4 MWs and molecular chain structures of PEG
Increasing the MW of PEG can extend the half-life of a drug, and branched PEG offers more advantages compared to linear PEG. Higher MWs of branched PEG exhibit a stronger steric hindrance effect, thereby reducing the likelihood of accessing the active site. Additionally, binding of branched PEG to the surface of TPPs effectively screens for surface antigens and enzymatic hydrolysis sites while minimizing immunogenicity (Pasut and Veronese, 2007). Yoshioka et al. (2004) utilized linear PEG with MWs of 5 and 20 kD as well as branched PEG with MWs of 10 and 40 kDa to modify lysine-deficient TNF-α. The in vitro activity retention rates were found to be 82%, 58%, 93%, and 65% respectively. Lysine-deficient TNF-α modified with linear PEG at a MW of 20 kDa and branched PEG at a MW of 10 kDa exhibited higher antitumor activity in mice compared to linear PEG at a MW of 5 kDa; however, modification with branched PEG at a MW of 40 kDa resulted in loss of activity (Yoshioka et al., 2004). Furthermore, increasing the MW of PEG leads to enhanced half-life for modified TPPs in vivo when compared to unmodified TPPs. rhDNase was conjugated with linear 20 kDa, linear 30 kDa or 2-armed 40 kDa PEG. While PEG20-rhDNase and PEG30-rhDNase progressively lost their activity over time in the lungs of mice, PEG40-rhDNase remained active. Notably, PEG20-rhDNase lost most of its activity. Additionally, compared to PEG20-rhDNase and PEG30-rhDNase, PEG40-rhDNase had lower thermodynamic stability (Guichard et al., 2017; Mahri et al., 2023). Similar destabilization upon PEGylation was also observed in cytochrome C modified with a 5 kDa PEG and recombinant human interleukin-1 receptor antagonist (rhIL-1ra) modified with a 20 kDa PEG, where TPPs’ thermodynamic stability decreased due to the modification by the polymer (García-Arellano et al., 2002; Sorret et al., 2019). The thermal stability of PEGylated green fluorescent protein (GFP) was assessed by de Lencastre Novaes et al. (2010) at temperatures ranging from 70°C to 95°C, considering different molar masses and concentrations of PEG. It was found that only PEG with molar masses of 600 and 4,000 g/mol provided protection to GFP molecules at 75°C, while the stabilization effect was not observed for GFP when using PEG with a molar mass of 10,000 g/mol between temperatures of 75°C and 95°C.
5 Typical cases of PEGylated TPPs
Among PEG-modified TPPs, several promising PEG-modified TPPs are highlighted in this study.
5.1 PEGylated AMPs
AMPs, also known as host defense peptides (HDPs), play a crucial role in innate immunity in multicellular organisms (Yang et al., 2017). Due to their broad antimicrobial spectrum and low toxicity, AMPs have emerged as potential candidates for novel therapeutic agents (Zasloff, 2002; Wang et al., 2020). Over the last three decades of AMP research, these peptide molecules have been proven to possess multiple biological functions including antibacterial, antifungal, antiviral, antiparasitic, anticancer and immunomodulatory activities (Mahlapuu et al., 2016; Mazurkiewicz-Pisarek et al., 2023). However, the clinical application of many AMPs is limited to topical medications only due to issues such as instability, potential antigenicity, rapid renal clearance, short circulation half-life and low therapeutic indices in vivo (Darveau et al., 1991; Steinberg et al., 1997; Zasloff, 2002; Harris and Chess, 2003; Chan et al., 2006; Hancock and Sahl, 2006; Răileanu et al., 2023). Furthermore, some AMPs exhibit reduced activity when exposed to serum (Johansson et al., 1998; Yeaman et al., 2002), plasma (Yeaman et al., 2002) or divalent cations (Matsuzaki et al., 1997; Johansson et al., 1998).
To address the limitations of AMPs, researchers have employed various strategies. These include targeted mutation techniques (Braman, 2002), fusion expression with proteins like albumin and immunoglobulin that possess longer half-lives (Sung et al., 2003; Qin et al., 2005), and chemical modifications to alter the structure of AMPs (Veronese and Mero, 2008). Among these approaches, PEG-modified AMPs have garnered significant attention due to the favorable biocompatibility of PEG. For instance, PEGylation substantially enhanced the enzymatic resistance of nisin A (34 aa) (Guiotto et al., 2003). Singh et al. (2014) demonstrated that PEGylation effectively reduced toxicity, increased selectivity, maintained anti-inflammatory effects, and decreased serum protein clearance of KYE28 peptide. Similarly, other AMPs such as M33, MA, and SAAP-148 exhibited improved stability and selectivity after being modified with different MWs of PEG. Additionally, they showed reduced scavenging by serum proteins while retaining their anti-inflammatory activity and promoting antimicrobial activity following PEGylation (Table 3) (Zhang et al., 2008; Falciani et al., 2014; Van Gent et al., 2023). Compared to the unmodified peptide, C-terminal PEGylated N6 displayed broader biodistribution in mice along with slower renal clearance and prolonged in vivo half-life (Li et al., 2022).
5.2 PEGylated interferon
In clinical application, interferon α-2b exhibits a short circulating half-life, limited immunogenicity and antigenicity, as well as rapid clearance by the circulatory system, resulting in significant inconvenience for patients' daily life (Bell et al., 2008). Furthermore, high-dose regimens of interferon α-2b are associated with considerable toxicity (Kirkwood et al., 2002). Conjugation of PEG to therapeutically valuable proteins represents an important and effective strategy to address these challenges and has been extensively employed in TPPs to reduce elimination rate while enhancing systemic exposure without compromising biological activity (Roberts et al., 2002). Several studies have demonstrated that peginterferon α-2b is more efficacious than non-PEGylated interferon in the treatment of hepatitis (Carrat et al., 2004; Khalili et al., 2005), which can be attributed to its altered pharmacokinetic (PK) profile leading to prolonged drug exposure (Zeuzem et al., 2000; Lindsay et al., 2001). Clinical evidence indicates that high-dose PEGylated interferon α-2b significantly reduces disease recurrence in resected stage III melanoma patients compared to unmodified interferon α-2b (Daud et al., 2010). Additionally, PEGylated interferon α-2b has shown effectiveness in treating hepatitis C virus (HCV) among children with end-stage renal disease (ESRD) (Mogahed et al., 2016). Moreover, PEGylation of interferon β-1a has been found to improve its pharmacokinetic and pharmacodynamic properties (Pepinsky et al., 2001; Cocco and Marrosu, 2015). These findings collectively demonstrate that optimal PEG modification enhances the bioavailability of interferons.
5.3 PEGylated asparaginase
The bacterial enzyme L-asparaginase, found in Gram-negative bacteria, can inhibit normal protein synthesis in tumor cells by degrading L-asparagine, leading to cell death (Narta et al., 2007). However, due to short half-life and antigenicity, L-asparaginase induces severe allergic reactions (Chung, 2010). PEGylated L-asparaginase has the ability to shield antigenic epitopes, reduce immunogenicity, prolong plasma retention time, and decrease proteolysis and renal excretion (Duval et al., 2002; Wang et al., 2012; Molineux, 2023). Clinical trials of PEGylated L-asparaginase began in 1984 and it was found to be safe for patients who had previously experienced allergic reactions to E. coli or Erwinia L-asparaginase (Graham, 2003). FDA approval for PEGylated asparaginase (Rhone-Poulenc Rorer as Oncaspar®) was granted in 1994 for the treatment of acute lymphoblastic leukemia (ALL) patients who are hypersensitive to the two native isoforms of the enzyme (Pasut and Veronese, 2009) (Table 3). Currently, improved PEGylated asparaginase with reduced hyposensitivity and a longer half-life is widely used in pediatric ALL patients (Avramis and Tiwari, 2006; Zhang et al., 2020; Riley et al., 2021). These findings demonstrate that PEGylated asparaginase can effectively reduce immunogenicity while extending its half-life for improved clinical applications.
5.4 PEGylated antibodies
Over the past decade, PEGylated antibodies have been extensively reported in the field of tumor immunotherapy (Table 3). PEGylation of antibodies prolonged circulation half-life and reduced immunogenicity when introduced into xenograft models (Casey et al., 2000; Chapman, 2002). Kitamura et al. (1990) evaluated the efficacy of murine monoclonal antibodies A7 (MAb A7) and F(ab′)2 fragments modified with PEG (MW of 5,000 Da) both in vitro and in vivo; their findings demonstrated that PEGylated MAb A7 and F(ab′)2 exhibited prolonged half-lives and enhanced accumulation within tumors compared to their unmodified counterparts. Furthermore, it has been shown that PEGylation of F(ab′)2 fragment and Fab' fragments derived from the A5B7 antibody targeting carcinoembryonic antigen (CEA), significantly enhances antibody accumulation within tumors while prolonging circulating half-life and reducing immunogenicity. However, no significant advantage was observed for PEGylated immunoglobulin G (IgG) over unmodified forms, indicating that PEGylated antibody fragments may possess an advantage over intact IgG modified with PEG for tumor targeting due to improved tumor penetration capabilities (Pedley et al., 1994). The findings suggest that PEGylated antibody fragments hold promise as effective drug carriers for targeted cancer chemotherapy. Several studies have demonstrated altered biodistribution of antibodies or antibody fragments, such as the Fab' fragment (F9) of A5B7 and mCC49 Fab' after PEG modification, resulting in increased tumor accumulation and reduced levels in normal tissues (Table 3) (Delgado et al., 1996; Chapman, 2002; Ding et al., 2013). Moreover, PEGylation significantly prolongs the local residence time of antibody fragments like anti-interleukin-17A (IL-17A) F(ab′)2 and anti-IL-13 Fab’ greatly in the lungs of rats, mice, and rabbits without causing significant pulmonary toxicity. In contrast, unconjugated IL-17A is cleared from the lungs within 24 h (Koussoroplis et al., 2014; Freches et al., 2017; Freches et al., 2019). Overall, PEGylated antibodies have great potential to revolutionize immunotherapy for chronic diseases.
6 Prospects of PEG-modified TPPs
Since Davis’s pioneering research on PEGylated protein drugs in the 1970s, the field of long-acting protein drugs has increasingly focused on PEGylation of TPPs (Davis et al., 1979; Davis, 2002). In 1981, Davis and Abuchowski established Enzon, the first company dedicated to PEGylation, which gained FDA approval in March 1990 for their groundbreaking PEGylated protein drug (Adagen) (Lee et al., 1991). Subsequently, numerous PEGylated TPPs have emerged in research; however, many are still undergoing clinical trials or under development. As research on PEG derivatives and modification technology intensifies, the limitations of non-specific site modification technology used to generate PEGylated TPPs have gradually come to light. Consequently, targeted modifications of PEGylated protein drugs have entered clinical trials. In 2002, Amgen’s pegfilgrastim (trade name: Neulasta®), a recombinant human granulocyte colony stimulating factor modified with site-directed PEGylation technology, became one of the most successful and also the first FDA-approved PEGylated protein drug (de Graaf et al., 2009). Pegfilgrastim is a mPEG covalently linked to the N-terminal amino group of rhG-CSF, resulting in an approximately 10-fold increase in its in vivo half-life compared to the unmodified form (Kinstler, et al., 1996; Bowen et al., 1999). Cetuzumab (Cimzia®) is a protein drug that has been modified with PEG and was introduced into the market in 2008. It represents the first PEGylated anti-TNF antibody, where a 40 kDa PEG moiety was specifically attached to the free cysteine residue at the C-terminus of the Fab' fragment of this humanized monoclonal antibody against TNF-α (Pasut, 2014).
Pegcetacoplan (APL-2/Empaveli) is a PEGylated cyclic peptide that functions as a complement C3 inhibitor. It received FDA approval in 2019 for the treatment of ocular diseases, including age-related macular degeneration (AMD) and paroxysmal nocturnal hemoglobinuria (PNH), based on successful clinical trials (Turecek et al., 2016; Hoy, 2021; Ji et al., 2021; Weitz, 2023). Other PEGylated TPPs, such as α1-antitrypsin, IFN α, and Fab fragments, have demonstrated increased residence time in the lungs and improved stability within the airways (Cantin et al., 2002; Koussoroplis et al., 2014; McLeod et al., 2015; Freches et al., 2017; Patil et al., 2018). These modifications enhance the ocular and pulmonary penetration and retention capabilities of TPPs, resulting in prolonged duration of action and reduced dosage frequency. This approach holds promise for improving patient compliance while minimizing systemic side effects associated with systemic administration of PEGylated TPPs for retinal disorder treatment.
With the advancement of science and technology, progressively more sophisticated PEG-modified TPPs currently in experimental or theoretical stage will gradually transit into clinical trials, thereby expanding the scope of applications and enhancing the development prospects for PEG-modified TPPs. Simultaneously, as the human genome project (HGP) undergoes comprehensive exploration, an increasing number of bioactive TPPs will be unearthed, further establishing PEG modification as a pivotal approach to maximize the efficacy of TPPs. Table 4.
7 Conclusion
The therapeutic potential of TPPs is widely recognized; however, their short circulating half-life, poor pharmacokinetics, rapid internal clearance, and high immunogenicity present significant challenges. PEG modification has emerged as a crucial approach to address these clinical limitations of TPPs and has garnered considerable attention in the biotechnology and biomedicine field. In this review, we provide a comprehensive overview of PEG properties, modification sites, factors influencing PEGylation response, typical cases of PEG-modified TPPs, and the future prospects of PEGylated TPPs. Furthermore, we emphasize that PEGylation offers promising benefits such as prolonged half-life, enhanced tumor accumulation, improved efficacy profiles, etc. Nevertheless, there remains ample room for further exploration and innovation in this field:
i) Future research aims to further investigate the mechanism of PEGylation and its impact on the structural and functional aspects of TPPs. Researchers should strive to elucidate the underlying principles governing PEG-TPP conjugation, ensuring optimal bioactivity and stability of resulting drugs.
ii) The variability in sizes and structures of PEG poses a significant challenge. Exploring different MWs and structures of PEG can provide valuable insights into the effects of these variables on drug delivery, biodistribution, and pharmacokinetics. By investigating these factors, researchers can optimize the PEGylation process to maximize therapeutic potential while minimizing side effects.
iii) Another area of exploration should involve the development of innovative PEGylation strategies. Current methods primarily rely on the conjugation of PEG to the TPP molecule through reactive chemistry. While effective, this approach may encounter specific limitations such as restricted site specificity and potential modification of drug functionality. Therefore, researchers may focus on exploring alternative PEGylation techniques, including enzymatic or chemoenzymatic approaches, to overcome these limitations and enhance drug efficacy. Additionally, researchers are investigating the utilization of other polymer systems, such as polypropylene glycol, poly(ethylene oxide-co-propylene oxide) or carboxybetaine, as substitutes for PEG.
iv) It is crucial to comprehend the impact of PEGylation on immune response. While PEGylation of TPPs generally enhances drug circulation time and reduces immunogenicity, certain studies have reported potential immunotoxicity associated with PEG-modified drugs. Therefore, it is imperative for researchers to delve deeper into the immunological aspects, investigating the mechanisms underlying the observed immune responses and striving towards strategies that mitigate any undesirable reactions.
By focusing on these key areas, researchers can advance the field of TPPs, fostering the development of more efficient and safer PEG-modified options.
Author contributions
CL: Conceptualization, Investigation, Writing–original draft. TL: Writing–original draft, Conceptualization, Investigation, Software. XT: Writing–original draft, Investigation, Resources. WA: Software, Visualization, Writing–review and editing. ZW: Writing–original draft. BH: Investigation, Writing–review and editing. HT: Methodology, Writing–review and editing. JW: Resources, Writing–review and editing. XW: Conceptualization, Funding acquisition, Investigation, Supervision, Visualization, Writing–original draft.
Funding
The author(s) declare that financial support was received for the research, authorship, and/or publication of this article. This work was supported by the National Natural Science Foundation of China (Grant No. 32072770).
Acknowledgments
We want to thank all the scientists, without their dedicated work, this review would not have been possible. We would also like to thank the editor and the reviewers for their critical reading, thoughtful comments, and constructive suggestions.
Conflict of interest
The authors declare that the research was conducted in the absence of any commercial or financial relationships that could be construed as a potential conflict of interest.
Publisher’s note
All claims expressed in this article are solely those of the authors and do not necessarily represent those of their affiliated organizations, or those of the publisher, the editors and the reviewers. Any product that may be evaluated in this article, or claim that may be made by its manufacturer, is not guaranteed or endorsed by the publisher.
References
Aapro, M., Boccia, R., Leonard, R., Camps, C., Campone, M., Choquet, S., et al. (2017). Refining the role of pegfilgrastim (a long-acting G-CSF) for prevention of chemotherapy-induced febrile neutropenia: consensus guidance recommendations. Support. Care Cancer 25 (11), 3295–3304. doi:10.1007/s00520-017-3842-1
Abuchowski, A., van Es, T., Palczuk, N. C., and Davis, F. F. (1977). Alteration of immunological properties of bovine serum albumin by covalent attachment of polyethylene glycol. J. Biol. Chem. 252 (11), 3578–3581. doi:10.1016/s0021-9258(17)40291-2
Antonsen, K. P., and Hoffman, A. S. (1992). “Water structure of PEG solutions by differential scanning calorimetry measurements,” in Topics in applied chemistry. poly (ethylene glycol) chemistry: biotechnical and biomedical applications (New York: Plenum Press), 15–28.
Armstrong, J. K., Hempel, G., Koling, S., Chan, L. S., Fisher, T., Meiselman, H. J., et al. (2007). Antibody against poly(ethylene glycol) adversely affects PEG-asparaginase therapy in acute lymphoblastic leukemia patients. Cancer 110, 103–111. doi:10.1002/cncr.22739
Autio, K., and Oft, M. (2019). Pegylated interleukin-10: clinical development of an immunoregulatory cytokine for use in cancer therapeutics. Curr. Oncol. Rep. 21 (2), 19. doi:10.1007/s11912-019-0760-z
Avramis, V. I., and Tiwari, P. N. (2006). Asparaginase (native ASNase or pegylated ASNase) in the treatment of acute lymphoblastic leukemia. Int. J. Nanomedicine 1 (3), 241–254. doi:10.2147/DIJN.1.S623
Balan, S., Choi, J. W., Godwin, A., Teo, I., Laborde, C. M., Heidelberger, S., et al. (2007). Site-specific PEGylation of protein disulfide bonds using a three-carbon bridge. Bioconjug. Chem. 18 (1), 61–76. doi:10.1021/bc0601471
Basu, A., Yang, K., Wang, M., Liu, S., Chintala, R., Palm, T., et al. (2006). Structure‒function engineering of interferon-beta-1b for improving stability, solubility, potency, immunogenicity, and pharmacokinetic properties by site-selective mono-PEGylation. Bioconjugate Chem. 17, 618–630. doi:10.1021/bc050322y
Behi, J., Hassiki, R., Said, N. B., Bouhaouala-Zahar, B., and Benkhoud, M. L. (2018). Optimization of PEGylation reaction time and molar ratio of rhG-CSF toward increasing bioactive potency of monoPEGylated protein. Int. J. Biol. Macromol. 109, 888–895. doi:10.1016/j.ijbiomac.2017.11.070
Bell, S. J., Fam, C. M., Chlipala, E. A., Carlson, S. J., Lee, J. I., Rosendahl, M. S., et al. (2008). Enhanced circulating half-life and antitumor activity of a site-specific pegylated interferon-alpha protein therapeutic. Bioconjug. Chem. 19 (1), 299–305. doi:10.1021/bc070131q
Benincasa, M., Zahariev, S., Pelillo, C., Milan, A., Gennaro, R., and Scocchi, M. (2015). PEGylation of the peptide Bac7(1-35) reduces renal clearance while retaining antibacterial activity and bacterial cell penetration capacity. Y. Eur. J. Med. Chem. 95, 210–219. doi:10.1016/j.ejmech.2015.03.028
Bhowmik, S., Bhowmick, S., Maiti, K., Chakra, A., Jain, D., Rajamannar, T., et al. (2018). Two multicenter Phase I randomized trials to compare the bioequivalence and safety of a generic doxorubicin hydrochloride liposome injection with Doxil® or Caelyx® in advanced ovarian cancer. Cancer Chemother. Pharmacol. 82 (3), 521–532. doi:10.1007/s00280-018-3643-3
Booth, C., and Gaspar, H. B. (2009). Pegademase bovine (PEG-ADA) for the treatment of infants and children with severe combined immunodeficiency (SCID). Biologics 3, 349–358. doi:10.2147/btt.2009.3286
Bowen, S., Tare, N., Inoue, T., Yamasaki, M., Okabe, M., Horii, I., et al. (1999). Relationship between molecular mass and duration of activity of polyethylene glycol conjugated granulocyte colony-stimulating factor mutein. Exp. Hematol. 27 (3), 425–432. doi:10.1016/s0301-472x(98)00051-4
Bradford, K. L., Moretti, F. A., Carbonaro-Sarracino, D. A., Gaspar, H. B., and Kohn, D. B. (2017). Adenosine deaminase (ADA)-deficient severe combined immune deficiency (SCID): molecular pathogenesis and clinical manifestations. J. Clin. Immunol. 37 (7), 626–637. doi:10.1007/s10875-017-0433-3
J. Braman (2002). In vitro mutagenesis protocols, methods in molecular. Biology (Totowa, NJ: Humana Press), 182.
Brocchini, S., Balan, S., Godwin, A., Choi, J. W., Zloh, M., and Shaunak, S. (2006). PEGylation of native disulfide bonds in proteins. Nat. Protoc. 1 (5), 2241–2252. doi:10.1038/nprot.2006.346
Brocchini, S., Godwin, A., Balan, S., Choi, J. W., Zloh, M., and Shaunak, S. (2008). Disulfide bridge based PEGylation of proteins. Adv. Drug Deliv. Rev. 60 (1), 3–12. doi:10.1016/j.addr.2007.06.014
Brokx, S., Scrocchi, L., Shah, N., and Dowd, J. (2017). A demonstration of analytical similarity comparing a proposed biosimilar pegfilgrastim and reference pegfilgrastim. Biologicals 48, 28–38. doi:10.1016/j.biologicals.2017.06.001
Cantin, A. M., Woods, D. E., Cloutier, D., Dufour, E. K., and Leduc, R. (2002). Polyethylene glycol conjugation at Cys232 prolongs the half-life of alpha1 proteinase inhibitor. Am. J. Respir. Cell Mol. Biol. 27, 659–665. doi:10.1165/rcmb.4866
Cao, S. J., Lv, Z. Q., Guo, S., Jiang, G. P., and Liu, H. L. (2021). An update - prolonging the action of protein and peptide drugs. J. Drug Deliv. Sci. Technol. 61, 102124. doi:10.1016/j.jddst.2020.102124
Carrat, F., Bani-Sadr, F., Pol, S., Rosenthal, E., Lunel-Fabiani, F., Benzekri, A., et al. (2004). Pegylated interferon alfa-2b vs standard interferon alfa-2b, plus ribavirin, for chronic hepatitis C in HIV-infected patients: a randomized controlled trial. JAMA 292, 2839–2848. doi:10.1001/jama.292.23.2839
Casey, J. L., Pedley, R. B., King, D. J., Boden, R., Chapman, A. P., Yarranton, G. T., et al. (2000). Improved tumor targeting of di-Fab’ fragments modified with polyethylene glycol. Tumor Target 4, 235–244.
Cazalis, C. S., Haller, C. A., Sease-Cargo, L., and Chaikof, E. L. (2004). C-terminal site-specific PEGylation of a truncated thrombomodulin mutant with retention of full bioactivity. Bioconjug. Chem. 15 (5), 1005–1009. doi:10.1021/bc049903y
Chae, S. Y., Chun, Y. G., Lee, S., Jin, C. H., Lee, E. S., Lee, K. C., et al. (2009). Pharmacokinetic and pharmacodynamic evaluation of site-specific PEGylated glucagon-like peptide-1 analogs as flexible postprandial-glucose controllers. J. Pharm. Sci. 98 (4), 1556–1567. doi:10.1002/jps.21532
Chan, D. I., Prenner, E. J., and Vogel, H. J. (2006). Tryptophan- and arginine-rich antimicrobial peptides: structures and mechanisms of action. Biochim. Biophys. Acta 1758 (9), 1184–1202. doi:10.1016/j.bbamem.2006.04.006
Chapman, A. P. (2002). PEGylated antibodies and antibody fragments for improved therapy: a review. Adv. Drug Deliv. Rev. 54, 531–545. doi:10.1016/s0169-409x(02)00026-1
Chen, Z., Shengyu, D., and Changle, C. (2018). Ethylene polymerization and copolymerization using nickel 2-iminopyridine-N-oxide catalysts: modulation of polymer molecular weights and molecular-weight distributions. Macromolecules 51 (1), 49–56. doi:10.1021/acs.macromol.7b02156
Cheng, J., Wang, Y., Hou, J., Luo, D., Xie, Q., Ning, Q., et al. (2014). Peginterferon alfa-2b in the treatment of Chinese patients with HBeAg-positive chronic hepatitis B: a randomized trial. J. Clin. Virol. 61 (4), 509–516. doi:10.1016/j.jcv.2014.08.008
Chi, Y., Zhang, H., Huang, W., Zhou, J., Zhou, Y., Qian, H., et al. (2008). Microwave-assisted solid phase synthesis, PEGylation, and biological activity studies of glucagon-like peptide-1(7-36)amide. Bioorg. Med. Chem. 16 (16), 7607–7614. doi:10.1016/j.bmc.2008.07.019
Chinol, M., Casalini, P., Maggiolo, M., Canevari, S., Omodeo, E. S., Caliceti, P., et al. (1998). Biochemical modifications of avidin improve pharmacokinetics and biodistribution, and reduce immunogenicity. Brit. J. Cancer 78 (2), 189–197. doi:10.1038/bjc.1998.463
Chiu, K., Agoubi, L. L., Lee, I., Limpar, M. T., Lowe, J. W., and Joh, S. L. (2010). Effects of polymer molecular weight on the size, activity, and stability of PEG-functionalized trypsin. Biomacromolecules 11, 3688–3692. doi:10.1021/bm1006954
Chung, H. S. (2010). PTD-mediated red blood cell encapsulation of L-asparaginase for potential treatment of acute lymphoblastic leukemia. Ph.D. and Master's. Michigan: The University of Michigan.
Cocco, E., and Marrosu, M. G. (2015). Profile of PEGylated interferon beta in the treatment of relapsing-remitting multiple sclerosis. Ther. Clin. Risk Manag. 11, 759–766. doi:10.2147/TCRM.S69123
Cong, Y., Pawlisz, E., Bryant, P., Balan, S., Laurine, E., Tommasi, R., et al. (2012). Site-specific PEGylation at histidine tags. Bioconjug. Chem. 23 (2), 248–263. doi:10.1021/bc200530x
Cooksley, W. G. (2005). Peginterferon-alpha 2a for the treatment of hepatitis B infection. Expert Opin. Pharmacother. 6 (8), 1373–1380. doi:10.1517/14656566.6.8.1373
Cui, Q., Xu, Q. J., Liu, L., Guan, L. L., Jiang, X. Y., Inam, M., et al. (2021). Preparation, characterization and Pharmacokinetic study of N-terminal PEGylated D-form antimicrobial peptide OM19r-8. J. Pharm. Sci. 110 (3), 1111–1119. doi:10.1016/j.xphs.2020.10.048
Darveau, R. P., Cunningham, M. D., Seachord, C. L., Cassiano-Clough, L., Cosand, W. L., Blake, J., et al. (1991). Beta-lactam antibiotics potentiate magainin 2 antimicrobial activity in vitro and in vivo. Antimicrob. Agents Chemother. 35 (6), 1153–1159. doi:10.1128/aac.35.6.1153
Daud, A. I., Xu, C., Hwu, W. J., Urbas, P., Andrews, S., Papadopoulos, N. E., et al. (2010). Pharmacokinetic/pharmacodynamic analysis of adjuvant pegylated interferon α-2b in patients with resected high-risk melanoma. Cancer Chemother. Pharmacol. 67 (3), 657–666. doi:10.1007/s00280-010-1326-9
Davis, F. F. (2002). The origin of pegnology. Adv. Drug Deliv. Rev. 54 (4), 457–458. doi:10.1016/s0169-409x(02)00021-2
Davis, F. F., Van Es, T., and Palczuk, N. C. (1979). Nonimmunogenic polypeptides: US 4179337 a. Washington, DC: U.S. Patent and Trademark Office.
de Graaf, A. J., Kooijman, M., Hennink, W. E., and Mastrobattista, E. (2009). Nonnatural amino acids for site-specific protein conjugation. Bioconjug. Chem. 20 (7), 1281–1295. doi:10.1021/bc800294a
de Lencastre Novaes, L. C., Mazzola, P. G., Pessoa, A., and Penna, T. C. (2010). Effect of polyethylene glycol on the thermal stability of green fluorescent protein. Biotechnol. Prog. 26 (1), 252–256. doi:10.1002/btpr.296
Delgado, C., Pedley, R. B., Herraez, A., Boden, R., Boden, J. A., Keep, P. A., et al. (1996). Enhanced tumour specificity of an anti-carcinoembrionic antigen Fab' fragment by poly(ethylene glycol) (PEG) modification. Br. J. Cancer 73 (2), 175–182. doi:10.1038/bjc.1996.32
Ding, H. M., Carlton, M. M., Povoski, S. P., Milum, K., Kumar, K., Kothandaraman, S., et al. (2013). Site specific discrete PEGylation of (124)I-labeled mCC49 Fab' fragments improves tumor MicroPET/CT imaging in mice. Bioconjug. Chem. 24 (11), 1945–1954. doi:10.1021/bc400375f
Dolence, E. K., Hu, C. Z., Tsang, R., Sanders, C. G., and Osaki, S. (1997). Electrophilic polyethylene oxides for the modification of polysaccharides, polypeptides(proteins)and surfaces. Washington, DC: U.S. Patent and Trademark Office.
Dozier, J. K., and Distefano, M. D. (2015). Site-specific PEGylation of therapeutic proteins. Int. J. Mol. Sci. 16 (10), 25831–25864. doi:10.3390/ijms161025831
Du, Q. S., Xie, N. Z., and Huang, R. B. (2015). Recent development of peptide drugs and advance on theory and methodology of peptide inhibitor design. Med. Chem. 11 (3), 235–247. doi:10.2174/1573406411666141229163355
Duval, M., Suciu, S., Ferster, A., Rialland, X., Nelken, B., Lutz, P., et al. (2002). Comparison of Escherichia coli-asparaginase with Erwinia-asparaginase in the treatment of childhood lymphoid malignancies: results of a randomized European Organization for Research and Treatment of Cancer-Children's Leukemia Group phase 3 trial. Blood 99 (8), 2734–2739. doi:10.1182/blood.v99.8.2734
Erak, M., Bellmann-Sickert, K., Els-Heindl, S., and Beck-Sickinger, A. G. (2018). Peptide Chemistry toolbox - transforming natural peptides into peptide therapeutics. Bioorg. Med. Chem. 26 (10), 2759–2765. doi:10.1016/j.bmc.2018.01.012
Falciani, C., Lozzi, L., Scali, S., Brunetti, J., Bracci, L., and Pini, A. (2014). Site-specific pegylation of an antimicrobial peptide increases resistance to Pseudomonas aeruginosaelastase. Amino Acids 46 (5), 1403–1407. doi:10.1007/s00726-014-1686-2
Fishbane, S., Schiller, B., Locatelli, F., Covic, A. C., Provenzano, R., Wiecek, A., et al. (2013). Peginesatide in patients with anemia undergoing hemodialysis. N. Engl. J. Med. 368 (4), 307–319. doi:10.1056/NEJMoa1203165
Floettmann, E., Bui, K., Sostek, M., Payza, K., and Eldon, M. (2017). Pharmacologic profile of naloxegol, a peripherally acting µ-opioid receptor antagonist, for the treatment of opioid-induced constipation. J. Pharmacol. Exp. Ther. 361 (2), 280–291. doi:10.1124/jpet.116.239061
Freches, D., Patil, H. P., Franco, M. M., Uyttenhove, C., Heywood, S., and Vanbever, R. (2017). PEGylation prolongs the pulmonary retention of an anti-IL-17A Fab' antibody fragment after pulmonary delivery in three different species. Int. J. Pharm. 521 (1-2), 120–129. doi:10.1016/j.ijpharm.2017.02.021
Freches, D., Rocks, N., H Patil, H. P., Perin, F., Snick, J. V., Vanbever, R., et al. (2019). Preclinical evaluation of topically-administered PEGylated Fab' lung toxicity. Int. J. Pharm. X 1, 100019. doi:10.1016/j.ijpx.2019.100019
Gaertner, H. F., and Offord, R. E. (1996). Site-specific attachment of functionalized poly(ethylene glycol) to the amino terminus of proteins. Bioconjug. Chem. 7 (1), 38–44. doi:10.1021/bc950074d
Gais, H. J., and Ruppert, S. (1995). Modification and immobilization of proteins with polyethylene glycol tresylates and polysaccharide tresylates: evidence suggesting a revision of the coupling mechanism and the structure of the polymer-polymer linkage. Tetrahedron Lett. 36 (22), 3837–3838. doi:10.1016/0040-4039(95)00633-n
García-Arellano, H., Valderrama, B., Saab-Rincón, G., and Vazquez-Duhalt, R. (2002). High temperature biocatalysis by chemically modified cytochrome C. Bioconjug. Chem. 13 (6), 1336–1344. doi:10.1021/bc025561p
Gault, V. A., Kerr, B. D., Irwin, N., and Flatt, P. R. (2008). C-terminal mini-PEGylation of glucose-dependent insulinotropic polypeptide exhibits metabolic stability and improved glucose homeostasis in dietary-induced diabetes. Biochem. Pharmacol. 75 (12), 2325–2333. doi:10.1016/j.bcp.2008.03.011
Giorgi, M. E., Agusti, R., and de Lederkremer, R. M. (2014). Carbohydrate PEGylation, an approach to improve pharmacological potency. Beilstein J. Org. Chem. 10, 1433–1444. doi:10.3762/bjoc.10.147
Gogia, P., Tarantino, M., Schramm, W., and Aledort, L. (2023). New directions to develop therapies for people with hemophilia. Expert Rev. Hematol. 16 (6), 417–433. doi:10.1080/17474086.2023.2184341
Graham, M. L. (2003). Pegaspargase: a review of clinical studies. Adv. Drug Deliv. Rev. 55, 1293–1302. doi:10.1016/s0169-409x(03)00110-8
Guichard, M. J., Kinoo, D., Aubriot, A. S., Bauwens, N., Gougué, J., Vermeulen, F., et al. (2018). Impact of PEGylation on the mucolytic activity of recombinant human deoxyribonuclease I in cystic fibrosis sputum. Clin. Sci. 132 (13), 1439–1452. doi:10.1042/CS20180315
Guichard, M. J., Leal, T., and Vanbever, R. (2017). PEGylation, an approach for improving the pulmonary delivery of biopharmaceuticals. Curr. Opin. Colloid Int. Sci. 31, 43–50. doi:10.1016/j.cocis.2017.08.001
Guiotto, A., Pozzobon, M., Canevari, M., Manganelli, R., Scarin, M., and Veronese, F. M. (2003). PEGylation of the antimicrobial peptide nisin A: problems and perspectives. Farmaco 58 (1), 45–50. doi:10.1016/S0014-827X(02)01301-0
Hancock, R. E., and Sahl, H. G. (2006). Antimicrobial and host-defense peptides as new anti-infective therapeutic strategies. Nat. Biotechnol. 24 (12), 1551–1557. doi:10.1038/nbt1267
Hao, Y., Chen, J., Wang, X., Zhu, H., and Rong, Z. (2006). Effects of site-specific polyethylene glycol modification of recombinant human granulocyte colony-stimulating factor on its biologic activities. Biodrugs 20 (6), 357–362. doi:10.2165/00063030-200620060-00006
Harris, J. M., and Chess, R. B. (2003). Effect of pegylation on pharmaceuticals. Nat. Rev. Drug Discov. 2 (3), 214–221. doi:10.1038/nrd1033
Harris, J. M., Martin, N. E., and Modi, M. (2001). Pegylation: a novel process for modifying pharmacokinetics. Clin. Pharmacokinet. 40 (7), 539–551. doi:10.2165/00003088-200140070-00005
Henning, T. P. (2002). Polyethylene glycols (PEGs) and the pharmaceutical industry. Pharma. Chem. 1, 57–59.
Herman, S., Hooftman, G., and Schacht, E. (1995). Poly(ethylene glycol) with reactive endgroups: I. modification of proteins. J. Bioact. Compat. Pol. 10, 145–187. doi:10.1177/088391159501000205
Hoy, S. M. (2015). Peginterferon beta-1a: a review of its use in patients with relapsing-remitting multiple sclerosis. CNS Drugs 29 (2), 171–179. doi:10.1007/s40263-015-0227-1
Hoy, S. M. (2021). Pegcetacoplan: first approval. Drugs 81 (12), 1423–1430. doi:10.1007/s40265-021-01560-8
Kitamura, K., Takahashi, T., Takashina, K., Yamaguchi, T., Noguchi, A., Tsurumi, H., et al. (1990). Polyethylene glycol modification of the monoclonal antibody A7 enhances its tumor localization. Biochem. Biophys. Res. Commun. 171 (3), 13871394. doi:10.1016/0006-291x(90)90839-f
Imura, Y., Nishida, M., and Matsuzaki, K. (2007a). Action mechanism of PEGylated magainin 2 analogue peptide. Biochim. Biophys. Acta 1768 (10), 2578–2585. doi:10.1016/j.bbamem.2007.06.013
Imura, Y., Nishida, M., Ogawa, Y., Takakura, Y., and Matsuzaki, K. (2007b). Action mechanism of tachyplesin I and effects of PEGylation. Biochim. Biophys. Acta 1768 (5), 1160–1169. doi:10.1016/j.bbamem.2007.01.005
Ji, L., Dong, X., Li, Y., Li, Y., Lim, S., Liu, M., et al. (2021). Efficacy and safety of once-weekly semaglutide versus once-daily sitagliptin as add-on to metformin in patients with type 2 diabetes in SUSTAIN China: a 30-week, double-blind, phase 3a, randomized trial. Diabetes Obes. Metab. 23, 404–414. doi:10.1111/dom.14232
Johansson, J., Gudmundsson, G. H., Rottenberg, M. E., Berndt, K. D., and Agerberth, B. (1998). Conformation-dependent antibacterial activity of the naturally occurring human peptide LL-37. J. Biol. Chem. 273 (6), 3718–3724. doi:10.1074/jbc.273.6.3718
Khalili, M., Bernstein, D., Lentz, E., Barylski, C., and Hoffman-Terry, M. (2005). Pegylated interferon -2a with or without ribavirin in HCV/HIV coinfection: partially blinded, randomized multicenter trial. Dig. Dis. Sci. 50, 1148–1155. doi:10.1007/s10620-005-2723-5
Khataminezhad, E. S., Hajihassan, Z., and Astaraei, F. R. (2023). Magnetically purification/immobilization of poly histidine-tagged proteins by PEGylated magnetic graphene oxide nanocomposites. Protein Expr. Purif. 207, 106264. doi:10.1016/j.pep.2023.106264
Kinstler, O., Molineux, G., Treuheit, M., Ladd, D., and Gegg, C. (2002). Mono-N-terminal poly(ethylene glycol)-protein conjugates. Adv. Drug Deliv. Rev. 54, 477–485. doi:10.1016/s0169-409x(02)00023-6
Kinstler, O. B., Brems, D. N., Lauren, S. L., Paige, A. G., Hamburger, J. B., and Treuheit, M. J. (1996). Characterization and stability of N-terminally PEGylated rhG-CSF. Pharm. Res. 13 (7), 996–1002. doi:10.1023/a:1016042220817
Kirkwood, J. M., Bender, C., Agarwala, S., Tarhini, A., Shipe-Spotloe, J., Smelko, B., et al. (2002). Mechanisms and management of toxicities associated with high-dose interferon alfa-2b therapy. J. Clin. Oncol. 20, 3703–3718. doi:10.1200/JCO.2002.03.052
Kizilel, S., Scavone, A., Liu, X., Nothias, J. M., Ostrega, D., Witkowski, P., et al. (2010). Encapsulation of pancreatic islets within nano-thin functional polyethylene glycol coatings for enhanced insulin secretion. Tissue Eng. Part A 16 (7), 2217–2228. doi:10.1089/ten.TEA.2009.0640
Knauf, M. J., Bell, D. P., Hirtzer, P., Luo, Z. P., Young, J. D., and Katre, N. V. (1988). Relationship of effective molecular size to systemic clearance in rats of recombinant interjeukin-2 chemically modified with water soluble polymers. J. Biol. Chem. 263, 1064–1070. doi:10.1016/S0021-9258(18)68146-3
Ko, J. H., and Maynard, H. D. (2018). A guide to maximizing the therapeutic potential of protein–polymer conjugates by rational design. Chem. Soc. Rev. 47, 8998–9014. doi:10.1039/c8cs00606g
Kolate, A., Baradia, D., Patil, S., Vhora, I., Kore, G., and Misra, A. (2014). PEG - a versatile conjugating ligand for drugs and drug delivery systems. J. Control. Release 192, 67–81. doi:10.1016/j.jconrel.2014.06.046
Koussoroplis, S.-J., Heywood, S., Uyttenhove, C., Barilly, C., Van Snick, J., and Vanbever, R. (2013). Production, purification and biological characterization of monoPEGylated anti-IL-17A antibody fragments. Int. J. Pharm. 454, 107–115. doi:10.1016/j.ijpharm.2013.06.077
Koussoroplis, S. J., Paulissen, G., Tyteca, D., Goldansaz, H., Todoroff, J., Barilly, C., et al. (2014). PEGylation of antibody fragments greatly increases their local residence time following delivery to the respiratory tract. J. Control Release 187, 91–100. doi:10.1016/j.jconrel.2014.05.021
Kumar, P., Pletzer, D., Haney, E. F., Rahanjam, N., Cheng, J. T. J., Yue, M., et al. (2019). Aurein-derived antimicrobial peptides formulated with pegylated phospholipid micelles to target methicillin-resistant Staphylococcus aureus skin infections. ACS Infect. Dis. 5 (3), 443–453. doi:10.1021/acsinfecdis.8b00319
Lamb, Y. N. (2022). Lonapegsomatropin: pediatric first approval. Paediatr. Drugs 24 (1), 83–90. doi:10.1007/s40272-021-00478-8
Leader, B., Baca, Q. J., and Golan, D. E. (2008). Protein therapeutics: a summary and pharmacological classification. Nat. Rev. Drug Discov. 7 (1), 21–39. doi:10.1038/nrd2399
Lee, C. R., McKenzie, C. A., Webster, K. D., and Whaley, R. (1991). Pegademase bovine: replacement therapy for severe combined immunodeficiency disease. DICP 25 (10), 1092–1095. doi:10.1177/106002809102501014
Levy, Y., Hershfield, M. S., Fernandez-Mejia, C., Polmar, S. H., Scudiery, D., Berger, M., et al. (1988). Adenosine deaminase deficiency with late onset of recurrent infections: response to treatment with polyethylene glycol-modified adenosine deaminase. J. Pediatr. 113, 312–317. doi:10.1016/s0022-3476(88)80271-3
Li, T., Yang, N., Teng, D., Mao, R. Y., Hao, Y., Wang, X. M., et al. (2022). C-terminal mini-PEGylation of a marine peptide N6 had potent antibacterial and anti-inflammatory properties against Escherichia coli and Salmonella strains in vitro and in vivo. BMC Microbiol. 22, 128. doi:10.1186/s12866-022-02534-w
Lindsay, K. L., Trepo, C., Heintges, T., Shiffman, M. L., Gordon, S. C., Hoefs, J. C., et al. (2001). A randomized, double-blind trial comparing pegylated interferon alfa-2b to interferon alfa-2b as initial treatment for chronic hepatitis C. Hepatology 34, 395–403. doi:10.1053/jhep.2001.26371
Longo, N., Dimmock, D., Levy, H., Viau, K., Bausell, H., Bilder, D. A., et al. (2019). Evidence- and consensus-based recommendations for the use of pegvaliase in adults with phenylketonuria. Genet. Med. 21 (8), 1851–1867. doi:10.1038/s41436-018-0403-z
Lu, X. G., and Zhang, K. (2018). PEGylation of therapeutic oligonucletides: from linear to highly branched PEG architectures. Nano Res. 11 (10), 5519–5534. doi:10.1007/s12274-018-2131-8
Ma, Q. M., Hu, T., and Yu, J. K. (2013). Molecular insight into the steric shielding effect of PEG on the conjugated staphylokinase: biochemical characterization and molecular dynamics simulation. PLoS One 8 (7), e68559. doi:10.1371/journal.pone.0068559
Mahlapuu, M., Håkansson, J., Ringstad, L., and Björn, C. (2016). Antimicrobial peptides: an emerging category of therapeutic agents. Front. Cell Infect. Microbiol. 6, 194. doi:10.3389/fcimb.2016.00194
Mahri, S., Rondon, A., Wilms, T., Bosquillon, C., and Vanbever, R. (2021). Biodistribution and elimination pathways of PEGylated recombinant human deoxyribonuclease I after pulmonary delivery in mice. J. Control Release 329, 1054–1065. doi:10.1016/j.jconrel.2020.10.034
Mahri, S., Wilms, T., Hagedorm, P., Guichard, M. J., Vanvarenberg, K., Dumoulin, M., et al. (2023). Nebulization of PEGylated recombinant human deoxyribonuclease I using vibrating membrane nebulizers: a technical feasibility study. Eur. J. Pharm. Sci. 189, 106522. doi:10.1016/j.ejps.2023.106522
Manning, M. C., Chou, D. K., Murphy, B. M., Payne, R. W., and Katayama, D. S. (2010). Stability of protein pharmaceuticals: an update. Pharm. Res. 27 (4), 544–575. doi:10.1007/s11095-009-0045-6
Matsuzaki, K., Sugishita, K., Harada, M., Fujii, N., and Miyajima, K. (1997). Interactions of an antimicrobial peptide, magainin 2, with outer and inner membranes of gram-negative bacteria. Biochim. Biophys. Acta 1327 (1), 119–130. doi:10.1016/s0005-2736(97)00051-5
Mayolo-Deloisa, K., González-González, M., Simental-Martínez, J., and Rito-Palomares, M. (2015). Aldehyde PEGylation of laccase from trametes versicolor in route to increase its stability: effect on enzymatic activity. J. Mol. Recognit. 28 (3), 173–179. doi:10.1002/jmr.2405
Mazurkiewicz-Pisarek, A., Baran, J., and Ciach, T. (2023). Antimicrobial peptides: challenging journey to the pharmaceutical, biomedical, and cosmeceutical use. Int. J. Mol. Sci. 24 (10), 9031. doi:10.3390/ijms24109031
McLeod, V. M., Chan, L. J., Ryan, G. M., Porter, C. J., and Kaminskas, L. M. (2015). Optimal PEGylation can improve the exposure of interferon in the lungs following pulmonary administration. J. Pharm. Sci. 104, 1421–1430. doi:10.1002/jps.24353
Miron, T., and Wilchek, M. (1993). A simplified method for the preparation of succinimidyl carbonate polyethylene glycol for coupling to proteins. Bioconjug. Chem. 4 (6), 568–569. doi:10.1021/bc00024a022
Mogahed, E. A., Abdelaziz, H., Helmy, H., Ghita, H., Abdel Mawla, M. A., Hassanin, F., et al. (2016). Safety and efficacy of pegylated interferon alpha-2b monotherapy in hepatitis C virus-infected children with end-stage renal disease on hemodialysis. J. Interf. Cytok. Res. 36 (12), 681–688. doi:10.1089/jir.2016.0019
Mohammed, G. K., Böttger, R., Krizsan, A., Volke, D., Mötzing, M., Li, S. D., et al. (2023). In vitro properties and pharmacokinetics of temporarily PEGylated Onc72 prodrugs. Adv. Healthc. Mat. 12 (11), e2202368. doi:10.1002/adhm.202202368
Molineux, G. (2003). Pegylation: engineering improved biopharmaceuticals for oncology. Pharmacotherapy 23 (8 Pt 2), 3S–8S. doi:10.1592/phco.23.9.3s.32886
Morgenstern, J., Baumann, P., Brunner, C., and Hubbuch, J. (2017). Effect of PEG molecular weight and PEGylation degree on the physical stability of PEGylated lysozyme. Int. J. Pharm. 519 (1-2), 408–417. doi:10.1016/j.ijpharm.2017.01.040
Morris, C. J., Beck, K., Fox, M. A., Ulaeto, D., Clark, G. C., and Gumbleton, M. (2012). Pegylation of antimicrobial peptides maintains the active peptide conformation, model membrane interactions, and antimicrobial activity while improving lung tissue biocompatibility following airway delivery. Antimicrob. Agents Chemother. 56 (6), 3298–3308. doi:10.1128/AAC.06335-11
Na, D. H., Park, E. J., Jo, Y. W., and Lee, K. C. (2008). Capillary electrophoretic separation of high-molecular-weight poly(ethylene glycol)-modified proteins. Anal. Biochem. 373 (2), 207–212. doi:10.1016/j.ab.2007.08.013
Nakaoka, R., Tabata, Y., Yamaoka, T., and Ikada, Y. (1997). Prolongation of the serum half-life period of superoxide dismutase by poly(ethylene glycol) modification. J. Control. Release 46, 253–261. doi:10.1016/s0168-3659(96)01605-7
Narta, U. K., Kanwar, S. S., and Azmi, W. (2007). Pharmacological and clinical evaluation of l-asparaginase in the treatment of leukemia. Crit. Rev. Oncol. Hematol. 61 (3), 208–221. doi:10.1016/j.critrevonc.2006.07.009
Ng, E. W., Shima, D. T., Calias, P., Cunningham, E. T., Guyer, D. R., and and& Adamis, A. P. (2006). Pegaptanib, a targeted anti-VEGF aptamer for ocular vascular disease. Nat. Rev. Drug Discov. 5, 123–132. doi:10.1038/nrd1955
Nischan, N., and Hackenberger, C. P. (2014). Site-specific PEGylation of proteins: recent developments. J. Org. Chem. 79 (22), 10727–10733. doi:10.1021/jo502136n
Nojima, Y., Iguchi, K., Suzuki, Y., and Sato, A. (2009a). The pH-dependent formation of PEGylated bovine lactoferrin by branched polyethylene glycol (PEG)-N-hydroxysuccinimide (NHS) active esters. Biol. Pharm. Bull. 32 (3), 523–526. doi:10.1248/bpb.32.523
Nojima, Y., Suzuki, Y., Yoshida, K., Abe, F., Shiga, T., Takeuchi, T., et al. (2009b). Lactoferrin conjugated with 40-kDa branched poly (ethylene glycol) has an improved circulating half-life. Pharm. Res. 26, 2125–2132. doi:10.1007/s11095-009-9925-z
Park, J. B., Kwon, Y. M., Lee, T. Y., Brim, R., Ko, M. C., Sunahara, R. K., et al. (2010). PEGylation of bacterial cocaine esterase for protection against protease digestion and immunogenicity. J. Control. Release 142, 174–179. doi:10.1016/j.jconrel.2009.10.015
Pasut, G. (2014). Pegylation of biological molecules and potential benefits: pharmacological properties of certolizumab pegol. BioDrugs 28 (Suppl. 1), 15–23. doi:10.1007/s40259-013-0064-z
Pasut, G., and Veronese, F. M. (2007). Polymer-drug conjugation, recent achievements and general strategies. Prog. Polym. Sci. 32 (8-9), 933–961. doi:10.1016/j.progpolymsci.2007.05.008
Pasut, G., and Veronese, F. M. (2009). PEG conjugates in clinical development or use as anticancer agents: an overview. Adv. Drug Del. Rev. 61 (13), 1177–1188. doi:10.1016/j.addr.2009.02.010
Pasut, G., and Veronese, F. M. (2012). State of the art in PEGylation: the great versatility achieved after forty years of research. J. Control. Release 161 (2), 461–472. doi:10.1016/j.jconrel.2011.10.037
Patil, H. P., Freches, D., Karmani, L., Duncan, G. A., Ucakar, B., Suk, J. S., et al. (2018). Fate of PEGylated antibody fragments following delivery to the lungs: influence of delivery site, PEG size and lung inflammation. J. Control Release 272, 62–71. doi:10.1016/j.jconrel.2017.12.009
Pedley, R. B., Boden, J. A., Boden, R., Begent, R. H. J., Turner, A., Haines, A. M. R., et al. (1994). The potential for enhanced tumour localisation by poly(ethylene glycol) modification of anti-CEA antibody. Br. J. Cancer 70, 1126–1130. doi:10.1038/bjc.1994.459
Pepinsky, R. B., LePage, D. J., Gill, A., Chakraborty, A., Vaidyanathan, S., Green, M., et al. (2001). Improved pharmacokinetic properties of a polyethylene glycol-modified form of interferon-beta-1a with preserved in vitro bioactivity. J. Pharmacol. Exp. Ther. 297 (3), 1059–1066.
Punzi, L., Lapadula, G., and Mathieu, A. (2014). Efficacy and safety of certolizumab pegol in rheumatoid arthritis: meeting rheumatologists' requirements in routine clinical practice. BioDrugs 28 (Suppl. 1), 25–37. doi:10.1007/s40259-013-0065-y
Qin, Y., Wang, X. H., Cui, H. L., Cheung, Y. K., Hu, M. H., Zhu, S. G., et al. (2005). Human papillomavirus type 16 E7 peptide(38-61) linked with an immunoglobulin G fragment provides protective immunity in mice. Gynecol. Oncol. 96 (2), 475–483. doi:10.1016/j.ygyno.2004.10.028
Rabe, E., Breu, F. X., Flessenkämper, I., Gerlach, H., Guggenbichler, S., Kahle, B., et al. (2021). Sclerotherapy in the treatment of varicose veins: S2k guideline of the Deutsche Gesellschaft für Phlebologie (DGP) in cooperation with the following societies: DDG, DGA, DGG, BVP. Hautarzt 72 (Suppl. 2), 23–36. doi:10.1007/s00105-020-04705-0
Răileanu, M., Borlan, R., Campu, A., Janosi, L., Turcu, I., Focsan, M., et al. (2023). No country for old antibiotics! Antimicrobial peptides (AMPs) as next-generation treatment for skin and soft tissue infection. Int. J. Pharm. 642, 123169. doi:10.1016/j.ijpharm.2023.123169
Rajender Reddy, K., Modi, M. W., and Pedder, S. (2002). Use of peginterferon alfa-2a (40 KD) (Pegasys) for the treatment of hepatitis C. Adv. Drug Deliv. Rev. 54, 571–586. doi:10.1016/s0169-409x(02)00028-5
Rawat, S., Suri, C. R., and Sahoo, D. K. (2020). Molecular mechanism of polyethylene glycol mediated stabilization of protein. Biochem. Biophys. Res. Commun. 392 (4), 561–566. doi:10.1016/j.bbrc.2010.01.067
Reis, G., Silva, E. A. S. M., Silva, D. C. M., Thabane, L., Campos, V. H. S., Ferreira, T. S., et al. (2023). Early treatment with pegylated interferon lambda for Covid-19. N. Engl. J. Med. 388 (6), 518–528. doi:10.1056/NEJMoa2209760
Riley, D. O., Schlefman, J. M., Vitzthum Von Eckstaedt, V. H. C., Morris, A. L., Keng, M. K., and El Chaer, F. (2021). Pegaspargase in practice: minimizing toxicity, maximizing benefit. Curr. Hematol. Malig. Rep. 16 (3), 314–324. doi:10.1007/s11899-021-00638-0
Roberts, M. J., Bentley, M. D., and Harris, J. M. (2002). Chemistry for peptide and protein PEGylation. Adv. Drug Deliv. Rev. 54 (4), 459–476. doi:10.1016/s0169-409x(02)00022-4
Schlapschy, M., Binder, U., Borger, C., Wachinger, K., Kisling, S., Haller, D., et al. (2013). PASylation: a biological alternative to PEGylation for extending the plasma half-life of pharmaceutically active proteins. Protein Eng. Des. Sel. 26, 489–501. doi:10.1093/protein/gzt023
Schlesinger, N., and Lipsky, P. E. (2020). Pegloticase treatment of chronic refractory gout: update on efficacy and safety. Semin. Arthritis Rheu 50 (3S), S31–S38. doi:10.1016/j.semarthrit.2020.04.011
Seo, Y., Park, J., Lee, H. J., Kim, M., Kang, I., Son, J., et al. (2023). Development and validation of a method for analyzing the sialylated glycopeptides of recombinant erythropoietin in urine using LC–HRMS. Sci. Rep. 13 (1), 3860. doi:10.1038/s41598-023-31030-y
Shaunak, S., Godwin, A., Choi, J. W., Balan, S., Pedone, E., Vijayarangam, D., et al. (2006). Site-specific PEGylation of native disulfide bonds in therapeutic proteins. Nat. Chem. Biol. 2 (6), 312–313. doi:10.1038/nchembio786
Sherman, M. R., Saifer, M. G., and Perez-Ruiz, F. (2008). PEG-uricase in the management of treatment-resistant gout and hyperuricemia. Adv. Drug Deliv. Rev. 60, 59–68. doi:10.1016/j.addr.2007.06.011
Shi, D., Beasock, D., Fessler, A., Szebeni, J., Ljubimova, J. Y., Afonin, K. A., et al. (2022). To PEGylate or not to PEGylate: immunological properties of nanomedicine’s most popular component, poly(ethylene) glycol and its alternatives. Adv. Drug Deliv. Rev. 180, 114079. doi:10.1016/j.addr.2021.114079
Singh, S., Papareddy, P., Mörgelin, M., Schmidtchen, A., and Malmsten, M. (2014). Effects of pegylation on membrane and lipopolysaccharide interactions of host defense peptides. Biomacromolecules 15 (4), 1337–1345. doi:10.1021/bm401884e
Sorret, L. L., Monticello, C. R., DeWinter, M. A., Schwartz, D. K., and Randolph, T. W. (2019). Steric repulsion forces contributed by PEGylation of interleukin-1 receptor antagonist reduce gelation and aggregation at the silicone oil-water interface. J. Pharm. Sci. 108 (1), 162–172. doi:10.1016/j.xphs.2018.10.045
Steinberg, D. A., Hurst, M. A., Fujii, C. A., Kung, A. H., Ho, J. F., Cheng, F. C., et al. (1997). Protegrin-1: a broad-spectrum, rapidly microbicidal peptide with in vivo activity. Antimicrob. Agents Chemother. 41 (8), 1738–1742. doi:10.1128/AAC.41.8.1738
Sun, J. L., Chen, J. Y., Sun, Y. M., Hou, Y. Q., Liu, Z. B., and Lu, H. (2023). On the origin of the low immunogenicity and biosafety of a neutral α-helical polypeptide as an alternative to polyethylene glycol. Bioact. Mat. 32, 333–343. doi:10.1016/j.bioactmat.2023.10.011
Sung, C., Nardelli, B., LaFleur, D. W., Blatter, E., Corcoran, M., Olsen, H. S., et al. (2003). An IFN-beta-albumin fusion protein that displays improved pharmacokinetic and pharmacodynamic properties in nonhuman primates. J. Int. Cyt. Res. 23, 25–36. doi:10.1089/10799900360520423
Syed, Y. Y. (2017). Nonacog beta pegol: a review in haemophilia B. Drugs 77 (18), 2003–2012. doi:10.1007/s40265-017-0836-8
Takedani, H., and Hirose, J. (2015). Turoctocog alfa: an evidence-based review of its potential in the treatment of hemophilia A. Drug Des. devel. Ther. 9, 1767–1772. doi:10.2147/DDDT.S57967
Tan, X. Y., Zhang, Y., Wang, Q., Ren, T. Y., Gou, J. X., Guo, W., et al. (2019). Cell-penetrating peptide together with PEG-modified mesostructured silica nanoparticles promotes mucous permeation and oral delivery of therapeutic proteins and peptides. Biomater. Sci. 7, 2934–2950. doi:10.1039/c9bm00274j
Tannir, N. M., Papadopoulos, K. P., Wong, D. J., Aljumaily, R., Hung, A., Afable, M., et al. (2021). Pegilodecakin as monotherapy or in combination with anti-PD-1 or tyrosine kinase inhibitor in heavily pretreated patients with advanced renal cell carcinoma: final results of cohorts A, G, H and I of IVY phase I study. Int. J. Cancer 149 (2), 403–408. doi:10.1002/ijc.33556
Thom, J., Anderson, D., McGregor, J., and Cotton, G. (2011). Recombinant protein hydrazides: application to site-specific protein PEGylation. Bioconjug. Chem. 22 (6), 1017–1020. doi:10.1021/bc2001374
Trainer, P. J., Drake, W. M., Katznelson, L., Freda, P. U., Herman-Bonert, V., van der Lely, A. J., et al. (2000). Treatment of acromegaly with the growth hormone–receptor antagonist pegvisomant. N. Engl. J. Med. 342 (16), 1171–1177. doi:10.1056/NEJM200004203421604
Treetharnmathurot, B., Ovartlarnporn, C., Wungsintaweekul, J., Duncan, R., and Wiwattanapatapee, R. (2008). Effect of PEG molecular weight and linking chemistry on the biological activity and thermal stability of PEGylated trypsin. Int. J. Pharm. 357 (1-2), 252–259. doi:10.1016/j.ijpharm.2008.01.016
Turecek, P. L., Bossard, M. J., Schoetens, F., and Ivens, I. A. (2016). PEGylation of biopharmaceuticals: a review of chemistry and nonclinical safety information of approved drugs. J. Pharm. Sci. 105, 460–475. doi:10.1016/j.xphs.2015.11.015
Van Gent, M. E., Schonkeren-Ravensbergen, B., Achkif, A., Beentjes, D., Dolezal, N., van Meijgaarden, K. E., et al. (2023). C-terminal PEGylation improves SAAP-148 peptide's immunomodulatory activities. J. Innate Immun. 15 (1), 724–738. doi:10.1159/000534068
Veronese, F. M. (2001). Peptide and protein PEGylation: a review of problems and solutions. Biomaterials 22, 405–417. doi:10.1016/s0142-9612(00)00193-9
Veronese, F. M., and Mero, A. (2008). The impact of PEGylation on biological therapies. Biodrugs 22 (5), 315–329. doi:10.2165/00063030-200822050-00004
Veronese, F. M., and Pasut, G. (2005). PEGylation, successful approach to drug delivery. Drug Discov. Today 10 (21), 1451–1458. doi:10.1016/S1359-6446(05)03575-0
Verstovsek, S., Komatsu, N., Gill, H., Jin, J., Lee, S. E., Hou, H. A., et al. (2022). SURPASS-ET: phase III study of ropeginterferon alfa-2b versus anagrelide as second-line therapy in essential thrombocythemia. Future Oncol. 18 (27), 2999–3009. doi:10.2217/fon-2022-0596
Vimal, A., and Kumar, A. (2017). Biotechnological production and practical application of L-asparaginase enzyme. Biotechnol. Genet. Eng. Rev. 33 (1), 40–61. doi:10.1080/02648725.2017.1357294
Vrooman, L. M., Blonquist, T. M., Stevenson, K. E., Supko, J. G., Hunt, S. K., Cronholm, S. M., et al. (2021). Efficacy and toxicity of pegaspargase and calaspargase pegol in childhood acute lymphoblastic leukemia: results of DFCI 11-001. J. Clin. Oncol. 39 (31), 3496–3505. doi:10.1200/JCO.20.03692
Vugmeyster, Y., Entrican, C. A., Joyce, A. P., Lawrence-Henderson, R. F., Leary, B. A., Mahoney, C. S., et al. (2012). Pharmacokinetic, biodistribution, and biophysical profiles of TNF nanobodies conjugated to linear or branched poly(ethylene glycol). Bioconjug. Chem. 23 (7), 1452–1462. doi:10.1021/bc300066a
Walsh, G., and Walsh, E. (2022). Biopharmaceutical benchmarks 2022. Nat. Biotechnol. 40 (12), 1722–1760. doi:10.1038/s41587-022-01582-x
Wan, X., Zhang, J. K., Yu, W. L., Shen, L. J., Ji, S. Y., and Hu, T. (2017). Effect of protein immunogenicity and PEG size and branching on the anti-PEG immune response to PEGylated proteins. Process Biochem. 52, 183–191. doi:10.1016/j.procbio.2016.09.029
Wang, B., Cao, Y., Chi, S., and Lou, D. (2012). A PEGylation technology of L-asparaginase with monomethoxy polyethylene glycol-propionaldehyde. Z. Naturforsch. C J. Biosci. 67 (5-6), 312–318. doi:10.1515/znc-2012-5-611
Wang, J., Hu, T., Liu, Y., Zhang, G., Ma, G., and Su, Z. (2011). Kinetic and stoichiometric analysis of the modification process for N-terminal PEGylation of staphylokinase. Anal. Biochem. 412 (1), 114–116. doi:10.1016/j.ab.2010.12.030
Wang, Y. S., Youngster, S., Grace, M., Bausch, J., Bordens, R., and Wyss, D. F. (2002). Structural and biological characterization of pegylated recombinant interferon alpha-2b and its therapeutic implications. Adv. Drug Deliv. Rev. 54, 547–570. doi:10.1016/s0169-409x(02)00027-3
Wang, Z., Liu, X., Teng, D., Mao, R., Hao, Y., Yang, N., et al. (2020). Development of chimeric peptides to facilitate the neutralization of lipopolysaccharides during bactericidal targeting of multidrug-resistant Escherichia coli. Commun. Biol. 3 (1), 41. doi:10.1038/s42003-020-0761-3
Weinberg, J., Zhang, S., Crews, G., Carta, G., and Przybycien, T. (2018). Chemical modification of protein A chromatography ligands with polyethylene glycol. I: effects on IgG adsorption equilibrium, kinetics, and transport. J. Chromatogr. A 1546, 77–88. doi:10.1016/j.chroma.2018.03.011
Weitz, I. C. (2023). Pegcetacoplan: a new opportunity for complement inhibition in PNH. J. Blood Med. 14, 239–245. doi:10.2147/JBM.S362220
Witt, K. A., Gillespie, T. J., Huber, J. D., Egleton, R. D., and Davis, T. P. (2001). Peptide drug modifications to enhance bioavailability and blood-brain barrier permeability. Peptides 22 (12), 2329–2343. doi:10.1016/s0196-9781(01)00537-x
Wylie, D. C., Voloch, M., Lee, S., Liu, Y. H., Cannon-Carlson, S., Cutler, C., et al. (2001). Carboxyalkylated histidine is a pH-dependent product of pegylation with SC-PEG. Pharm. Res. 18 (9), 1354–1360. doi:10.1023/a:1013006515587
Yamamoto, Y., Tsutsumi, Y., Yoshioka, Y., Nishibata, T., Kobayashi, K., Okamoto, T., et al. (2003). Site-specific PEGylation of a lysine-deficient TNF-alpha with full bioactivity. Nat. Biotechnol. 21 (5), 546–552. doi:10.1038/nbt812
Yang, B. B., and Kido, A. (2011). Pharmacokinetics and pharmacodynamics of pegfilgrastim. Clin. Pharmacokinet. 50 (5), 295–306. doi:10.2165/11586040-000000000-00000
Yang, N., Liu, X., Teng, D., Li, Z., Wang, X., Mao, R., et al. (2017). Antibacterial and detoxifying activity of NZ17074 analogs with multilayers of selective antimicrobial actions against Escherichia coli and Salmonella enteritidis. Sci. Rep. 7 (1), 3392. doi:10.1038/s41598-017-03664-2
Yeaman, M. R., Gank, K. D., Bayer, A. S., and Brass, E. P. (2002). Synthetic peptides that exert antimicrobial activities in whole blood and blood-derived matrices. Antimicrob. Agents Chemother. 46 (12), 3883–3891. doi:10.1128/aac.46.12.3883-3891.2002
Yoo, M. K., Park, I. K., Lim, H. T., Lee, S. J., Jiang, H. L., Kim, Y. K., et al. (2012). Folate-PEG-superparamagnetic iron oxide nanoparticles for lung cancer imaging. Acta Biomater. 8 (8), 3005–3013. doi:10.1016/j.actbio.2012.04.029
Yoshioka, Y., Tsunoda, S., and Tsutsumi, Y. (2011). Development of a novel DDS for site-specific PEGylated proteins. Chem. Cent. J. 5, 25. doi:10.1186/1752-153X-5-25
Yoshioka, Y., Tsutsumi, Y., Ikemizu, S., Yamamoto, Y., Shibata, H., Nishibata, T., et al. (2004). Optimal site-specific PEGylation of mutant TNF-alpha improves its antitumor potency. Biochem. Biophys. Res. Commun. 315 (4), 808–814. doi:10.1016/j.bbrc.2004.01.125
Youn, Y. S., Chae, S. Y., Lee, S., Jeon, J. E., Shin, H. G., and Lee, K. C. (2007a). Evaluation of therapeutic potentials of site-specific PEGylated glucagon-like peptide-1 isomers as a type 2 anti-diabetic treatment: insulinotropic activity, glucose-stabilizing capability, and proteolytic stability. Biochem. Pharmacol. 73 (1), 84–93. doi:10.1016/j.bcp.2006.09.013
Youn, Y. S., Kwon, M. J., Na, D. H., Chae, S. Y., Lee, S., and Lee, K. C. (2008). Improved intrapulmonary delivery of site-specific PEGylated salmon calcitonin: optimization by PEG size selection. J. Control. Release 125 (1), 68–75. doi:10.1016/j.jconrel.2007.10.008
Youn, Y. S., and Lee, K. C. (2007). Site-specific PEGylation for high-yield preparation of Lys(21)-amine PEGylated growth hormone-releasing factor (GRF) (1-29) using a GRF(1-29) derivative FMOC-protected at Tyr(1) and Lys(12). Bioconjug. Chem. 18 (2), 500–506. doi:10.1021/bc060173z
Youn, Y. S., Na, D. H., and Lee, K. C. (2007b). High-yield production of biologically active mono-PEGylated salmon calcitonin by site-specific PEGylation. J. Control. Release 117 (3), 371–379. doi:10.1016/j.jconrel.2006.11.013
Zalipsky, S., and Lee, C. (1992). “Use of functionalized poly(ethylene glycol)s for modification of polypeptides,” in Poly(Ethylene glycol) chemistry (Berlin, Germany: Springer US), 1–15.
Zalipsky, S., Seltzer, R., and Menon-Rudolph, S. (1992). Evaluation of a new reagent for covalent attachment of polyethylene glycol to proteins. Biotechnol. Appl. Biochem. 15 (1), 100–114. doi:10.1111/j.1470-8744.1992.tb00198.x
Zasloff, M. (2002). Antimicrobial peptides of multicellular organisms. Nature 415 (6870), 389–395. doi:10.1038/415389a
Zeuzem, S., Feinman, S. V., Rasenack, J., Heathcote, E. J., Lai, M. Y., Gane, E., et al. (2000). Peginterferon alfa-2a in patients with chronic hepatitis C. N. Engl. J. Med. 343, 1666–1672. doi:10.1056/NEJM200012073432301
Zhang, C., Yang, X. L., Yuan, Y. H., Pu, J., and Liao, F. (2012). Site-specific PEGylation of therapeutic proteins via optimization of both accessible reactive amino acid residues and PEG derivatives. BioDrugs 26 (4), 209–215. doi:10.1007/BF03261880
Zhang, G. H., Han, B. Z., Lin, X. Y., Wu, X., and Yan, H. S. (2008). Modification of antimicrobial peptide with low molar mass poly(ethylene glycol). J. Biochem. 144 (6), 781–788. doi:10.1093/jb/mvn134
Zhang, J., Zhao, Y. J., Su, Z. G., and Ma, G. H. (2007). Synthesis of monomethoxy poly(ethylene glycol) without diol poly(ethylene glycol). J. Appl. Polym. Sci. 105 (6), 3782–3786. doi:10.1002/app.26457
Zhang, Y. Y., Yang, Q. S., Qing, X., Li, B. R., Qian, J., Wang, Y., et al. (2020). Peg-asparaginase-associated pancreatitis in chemotherapy-treated pediatric patients: a 5-year retrospective study. Front. Oncol. 10, 538779. doi:10.3389/fonc.2020.538779
Zhao, T., Cheng, Y. N., Tan, H. N., Liu, J. F., Xu, H. L., Pang, G. L., et al. (2012). Site-specific chemical modification of human serum albumin with polyethylene glycol prolongs half-life and improves intravascular retention in mice. Biol. Pharm. Bull. 35 (3), 280–288. doi:10.1248/bpb.35.280
Zhou, Z., Zhang, J., Sun, L., Ma, G., and Su, Z. (2014). Comparison of site-specific PEGylations of the N-terminus of interferon beta-1b: selectivity, efficiency, and in vivo/vitro activity. Bioconjug. Chem. 25 (1), 138–146. doi:10.1021/bc400435u
Zloh, M., Shaunak, S., Balan, S., and Brocchini, S. (2007). Identification and insertion of 3-carbon bridges in protein disulfide bonds: a computational approach. Nat. Protoc. 2 (5), 1070–1083. doi:10.1038/nprot.2007.119
Zuma, L. K., Gasa, N. L., Makhoba, X. H., and Pooe, O. J. (2022a). Protein PEGylation: navigating recombinant protein stability, aggregation, and bioactivity. Biomed. Res. Int. 2022, 8929715. doi:10.1155/2022/8929715
Keywords: PEGylation, therapeutic protein, antimicrobial peptides, antibodies, modification
Citation: Li C, Li T, Tian X, An W, Wang Z, Han B, Tao H, Wang J and Wang X (2024) Research progress on the PEGylation of therapeutic proteins and peptides (TPPs). Front. Pharmacol. 15:1353626. doi: 10.3389/fphar.2024.1353626
Received: 11 December 2023; Accepted: 22 February 2024;
Published: 08 March 2024.
Edited by:
Xavier Vila Farres, Contrafect, United StatesReviewed by:
Rita Vanbever, Université Catholique de Louvain, BelgiumOfentse Jacob Pooe, University of KwaZulu-Natal, South Africa
Copyright © 2024 Li, Li, Tian, An, Wang, Han, Tao, Wang and Wang. This is an open-access article distributed under the terms of the Creative Commons Attribution License (CC BY). The use, distribution or reproduction in other forums is permitted, provided the original author(s) and the copyright owner(s) are credited and that the original publication in this journal is cited, in accordance with accepted academic practice. No use, distribution or reproduction is permitted which does not comply with these terms.
*Correspondence: Zhenlong Wang, wangzhenlong02@caas.cn; Xiumin Wang, wangxiumin@caas.cn
†These authors have contributed equally to this work