- 1Department of Industrial and Manufacturing Systems Engineering, College of Engineering, Iowa State University, Ames, IA, United States
- 2Virtual Reality Applications Center, Iowa State University, Ames, IA, United States
- 3Human Development and Family Studies, Iowa State University, Ames, IA, United States
Virtual reality (VR) usage continues to grow, but visually induced motion sickness (VIMS) can decrease VR effectiveness for some users. This study seeks to compare methods of VIMS mitigation and explore sickness among gender and video game experience groups. Participant discomfort and early dropout are problems for studies that involve virtual environment (VE) exposure, but previous research has demonstrated that natural decay and physical, real-world hand–eye coordination tasks can serve as effective mitigation strategies. In this study, 57 participants wore a head-mounted display (HMD) and navigated a maze VE designed to induce cybersickness. Participants then experienced one of four mitigation techniques: real natural decay (HMD off), virtual natural decay (HMD on with idyllic VE and no locomotion), real hand–eye coordination task (HMD off), and virtual hand–eye coordination task (HMD on). Simulator Sickness Questionnaire (SSQ) measures were taken periodically throughout maze and mitigation tasks. Results demonstrated that peak sickness during the maze VE occurred after approximately 10 min. Analyses of mitigation techniques showed that real natural decay resulted in significantly more sickness recovery when compared with the virtual hand–eye coordination task for SSQ total score, nausea, and oculomotor constructs, but not disorientation. The real natural decay technique was the most effective at bringing participants' final sickness measure back to their initial baseline measure; however, other mitigation techniques yielded effectiveness, but at a lower rate. This study extends previous research about hand–eye mitigation approaches by demonstrating that natural decay and hand–eye tasks in a virtual and real-world setting were effective in reducing VIMS. Real-world natural decay was the most effective at mitigating VIMS, and the virtual hand–eye task was not as effective as the other three tasks. Women experienced more VIMS than men did but also recovered than men did during mitigation. Video gamers experienced less VIMS than non-gamers. These findings bolster extant knowledge about VIMS mitigation techniques and can inform future development of virtual mitigation techniques.
Introduction
Visually induced motion sickness (VIMS) is a subcategory of motion sickness that specifically relates to nausea, oculomotor strain, and disorientation from the perception of motion while remaining still (Kennedy et al., 2010). VIMS presents an obstacle to widespread adoption of virtual reality (VR) experiences because it can have devastating results on any study in which participants move within a virtual environment (VE). VIMS has the potential to compromise a study, but it can also pose a safety risk to participants if they become physically ill. The effects of these symptoms may make using VR, both recreationally and professionally, too uncomfortable in the short and long term for many users. As such, VIMS could render adoption and innovation around VR fruitless. In combination with improving the virtual experience to prevent sickness, it is also critical to provide solutions for users to readapt and reduce sickness after exposure.
The existence of VIMS within VR is well-known (Lo and So, 2001; Jerome et al., 2005; Kennedy et al., 2010; Mousavi et al., 2013; Davis et al., 2014), but less is understood about how to readapt from post-VE exposure symptoms (Champney et al., 2007), so it is unclear how best to mitigate VR-related sickness. If research can provide empirically substantiated solutions to VIMS, it may be possible to increase post-VR exposure adaption and thus reduce individual safety risk, improve experience, and bolster VR growth. The current study extends VIMS research to compare the effectiveness of four mitigation techniques, within and outside of VR, following exposure in order to better understand VIMS recovery.
VIMS recovery techniques vary from breathing exercises (Russell et al., 2014) to medication (Regan, 1995; Regan and Ramsey, 1996), to simply waiting for symptoms to attenuate (Kennedy and Fowlkes, 1992). The last technique, called natural decay, has successfully reduced VIMS following VE exposure but can take up to 24 h to fully eliminate symptoms (Baltzley et al., 1989). An alternative technique involving a hand–eye coordination task was developed in an attempt to more rapidly reduce VIMS by engaging ocular focus and proprioception (Champney et al., 2007). However, both of these techniques require a user to exit the VE, so they do not allow users experiencing VIMS to remain immersed in a VE while mitigating symptoms. Virtual mitigation techniques allow users to remain in the virtual environment, extending the possible exposure periods. A VR version of Champney's hand–eye coordination task was developed to reduce symptoms rapidly within exiting the VE. The virtual hand–eye task successfully reduced VIMS following VR exposure (Curtis et al., 2015) and may be an alternative for the real-world version of the technique. Curtis (2014) also investigated virtual natural decay, which did not perform significantly different from the real natural decay. While these mitigation techniques have shown evidence of VIMS mitigation, it is unclear how all four perform relative to one another. Also, previous analyses have not examined the impact of gender and video game experience on sickness and on mitigation. Further evidence behind successful VIMS mitigation techniques can help (1) develop understandings around VIMS recovery and (2) provide individuals (i.e., companies, app developers, etc.) with methods to reduce the safety risk associated with VEs.
Literature Review
For the purposes of this study, it is worth reviewing previous research on VIMS, individual differences in VIMS susceptibility, and methods of recovery from VIMS. The extant literature is reviewed in order to understand what contributes to sickness symptoms, as well as the effectiveness of current techniques for recovering from VIMS.
Visually Induced Motion Sickness
Motion sickness is a widespread human experience characterized by nausea, oculomotor issues, and disorientation (Kennedy et al., 2010). This discomfort occurs when someone is exposed to a motion stimulus that is sufficient to disrupt the function of their vestibular system (Golding, 2006). Stimuli that induce motion sickness include land movement (e.g., cars, trains), sea travel, air flight, and optokinetic exposure (i.e., slow- and fast-paced eye tracking movement), including virtual reality (VR) simulators (Golding, 2006). VR simulators are rising in prominence as less expensive, less risky alternatives to professional training, such as piloting or surgery. However, some users of these VR systems have reported excessive motion sickness discomfort following exposure to immersive VEs (Estrada et al., 2007), such as VR environments when using head-mounted displays (HMDs) (Boyd, 2014; Lewis, 2015), increasing the demand for methods to mitigate motion sickness resulting from simulators.
Simulator sickness is motion sickness caused by any simulator used for leisure or professional purposes (Buker et al., 2012). In order to better understand simulator sickness susceptibility and recovery, the Simulator Sickness Questionnaire (SSQ) was developed to capture an individual's nausea, oculomotor, and disorientation symptoms during exposure to flight and vehicle simulators (Kennedy et al., 1993). The SSQ has been demonstrated as a both reliable and valid assessment of symptoms (Kennedy et al., 1993). The SSQ remains the most commonly utilized measure for simulator sickness is the Simulator Sickness Questionnaire (SSQ) (Rebenitsch and Owen, 2016). Other subjective assessments for sickness caused by virtual reality have been developed, such as the Virtual Reality Sickness Questionnaire (Kim H. K. et al., 2018) and the Virtual Reality Symptom Questionnaire (Ames et al., 2005). However, such assessments have not been widely utilized in research, thus leaving the SSQ as the most robust choice for measuring simulator sickness symptoms.
Two methodological questions of interest have been explored in the literature when assessing simulator sickness: (1) whether measurements be conducted verbally and (2) whether measurements can be taken while participants are experiencing the VE. Although the SSQ is primarily used as a written response assessment, some studies have verbally administered it because of its concise format that can be used without disrupting visual exposure to a VE (Min et al., 2004; Moss and Muth, 2011; Duzmańska et al., 2018). For example, Moss and Muth (2011) administered a verbal recording of the SSQ participants in between 2-min VR exposures while their HMD was still worn, as well as twice before and after exposure. While they did not administer the SSQ during the VR task, some researchers have concluded that the ideal frequency of SSQ measurement during and after VR exposure remains undecided and should be further addressed (Duzmańska et al., 2018).
Keshavarz and Hecht (2011) were interested in understanding how sickness changed throughout virtual exposure, rather than just the final amount of sickness after exposure. They developed the Fast Motion Sickness Scale (FMS) as a method for verbally assessing motion sickness multiple times during exposure to a VE. In order to cross-validate the FMS with the most common sickness assessment, the SSQ, they administered the SSQ immediately post-exposure, but not throughout. Researchers found that the final FMS measurement was highly correlated with the SSQ total score (TS), as well as its subscales: Nausea (N), Oculomotor (O), and Disorientation (D). Further, the researchers plotted the FMS scores throughout the stimuli and overlaid the SSQ TS and SSQ N regression lines to reveal that the FMS scores and regression slope directly mirrored each other. The authors concluded that the FMS was cross-validated with the SSQ (Keshavarz and Hecht, 2011; Keshavarz et al., 2018), suggesting that verbal assessment of sickness during a virtual stimuli is possible and may result in a similar reported amount of final sickness to an assessment only given at the end of a stimuli, such as the SSQ. These findings could also be used to postulate that other sickness assessments, such as the SSQ, could be verbally administered throughout a virtual stimulus with little negative impact on sickness.
It is unclear how the verbal administration of the FMS (or the SSQ) impacts presence in VR, or the extent to which it interrupts the virtual experience. That answer likely depends on the context, i.e., the authenticity (Gilbert, 2016) or coherence of the task (Skarbez et al., 2018). In a recent review of 20 articles examining the connection between presence and sickness during VR exposure, authors found mixed results (Weech et al., 2019). Among the articles reviewed, 11 reported a negative correlation between presence and sickness, while 9 reported a null or positive correlation between presence and sickness. Although the review postulated that presence and cybersickness most commonly have an inverse relationship, there are enough mixed findings to warrant further investigation. As such, it is possible that the SSQ could be verbally administered throughout a virtual experience and possibly have a null impact on presence, and visually induced motion sickness.
Visually induced motion sickness (VIMS) is another term developed as an additional subcategory to motion sickness that is similar to simulator sickness, referring specifically to symptoms caused by the perception of motion when using contemporary interactive technologies while sitting still (Kennedy et al., 2010). VIMS differs from simulator sickness in that it broadly applies to any VE that causes feelings of sickness while the user does not move, whereas simulator sickness may refer more specifically to flight and vehicle scenarios. The additional term cybersickness is a more popular term for the clinical label VIMS, and the term cybersickness tends to connote sickness related to a digitally enhanced reality, e.g., virtual reality (VR), augmented reality (AR), or mixed reality (MR), all of which can be encompassed with the more general term extended reality (XR).
It is clear that VIMS and other types of motion sickness (e.g., simulator sickness, car sickness) occur, but the reason is not clear. The sensory conflict theory has been used to speculate why sickness occurs (Reason, 1978). It posits that a movement-related visual stimulus causes a neural mismatch wherein the visual input does not match stored neural patterns of movement, resulting in sensory disturbances and thus sickness. An alternative theory, postural instability (Riccio and Stoffregen, 1991; Walter et al., 2019), suggests that sickness occurs when people are in situations in which they are uncertain or unable to maintain postural stability for prolonged periods of time. While these theories are helpful in illuminating how VIMS occurs, the reason for individual differences in susceptibility to VIMS is less known.
Susceptibility to Visually Induced Motion Sickness
Likeliness to get motion sickness varies notably among people in the general population; some people are approximately 10,000 more susceptible to become sick than others (Lackner, 2014). Susceptibility involves individual stimulation sensitivity, stimulation adaptation, and stimulation adaptation rate (Golding, 2006; Lackner, 2014). It is possible that individual differences in susceptibility are due to physiological differences related to the vestibular and somatosensory systems (Golding, 2006). For instance, there is some evidence that motion sickness is less frequent in individuals with bilateral loss of labyrinthine function, when occurs when the deficient vestibulo-oculor reflex of the inner ear, and the retina cannot reconcile visual stimulus (Golding, 2006). Additionally, some individuals may be naturally less reliant on vestibular and ocular inputs, increasing somatosensory dependence for maintaining balance, resulting in increased susceptibility to motion sickness (Nachum et al., 2004). Recent research observed that greater susceptibility was predicted by increased visual sensitivity to sensory cues when viewing motion parallax (Fulvio et al., 2020). Because sensory conflict theory focuses the cause of sickness more on the stimuli than the person, it has more difficulty explaining individual differences in susceptibility. Postural instability theory, on the other hand, focuses more of the cause on the individual's ability to stabilize.
Gender has been discussed as a possible individual difference contributing to VIMS variability. Females generally report higher levels of sickness than males (Koslucher et al., 2016; Munafo et al., 2017). When exposed to linear oscillating visual motion stimuli, women experienced VIMS four times as often as men (Koslucher et al., 2015). Women were also found to be more susceptible to VIMS than men when playing games using an Oculus Rift (Munafo et al., 2017). Contradictory evidence, however, showed no gender differences in VIMS (e.g., Klosterhalfen et al., 2006), neither in severity nor on incidence (Curry et al., 2020). Some research has further suggested that gender differences may stem from male–female differences related to video game experience (Shafer et al., 2017). Other research suggests that differences may result in part from male HMD configurations (Fulvio et al., 2020), potentially confounding gender-related VIMS findings, indicating that there is not yet a full consensus on gender-based susceptibility differences.
Video game play experience has also been examined as an individual difference contributing to variability in VIMS. Prior virtual environment experiences, including video game play, may reduce individual susceptibility to cybersickness (Knight and Arns, 2006). However, other research has found limited to no support for the relationship between video game play experience and VIMS (Gamito et al., 2008, 2010). Given these limited and conflicting results, further investigation of prior VE experience via video game play is warranted. The entire set of factors that contribute to VIMS susceptibility is not yet fully understood.
Recovery From Visually Induced Motion Sickness
While some research has focused on individual susceptibility to VIMS, less is known about recovery from VIMS (Champney et al., 2007). For general motion sickness, individuals may utilize anti-cholinergic medications or wrist acupressure bands to reduce sickness symptoms (Miller and Muth, 2004; Estrada et al., 2007). Hyoscine hydrobromide, an anti-motion sickness anti-cholinergic drug, can be used to inhibit nausea caused by motion sickness. This drug has also successfully reduced VIMS from VR after 20 min of exposure (Regan, 1995; Regan and Ramsey, 1996). However, medications can come with undesirable side effects, such as drowsiness, blurred vision, impaired psychomotor function, and slower information processing (Estrada et al., 2007). Thus, recovery methods that do not rely on chemical intervention have been developed as potentially safer alternatives.
Non-invasive methods for reducing VIMS during exposure have been explored. Some evidence has suggested that paced diaphragmatic breathing during VR exposure results in lower sickness than a control condition, but it is unclear whether this method reduces symptoms post-exposure (Russell et al., 2014). Pilot research successfully implemented air cushions on seats during VR video game play to reduce symptoms of dizziness, headaches, stomach awareness, sweating, and fatigue (Onuki et al., 2017). Due to the approach of reporting results, it is unclear which analyses were utilized and to what extent VIMS were reduced. Regardless, these preliminary findings suggest that the use of air cushions could be beneficial in reducing VIMS from VR exposure. Research relating to adjusting visual settings, such as through dynamic non-salient area blurring (Nie et al., 2019) and restricted field of view (Kim S. et al., 2018), have also mitigated VIMS. There has additionally been evidence that distracting participants from their symptoms through tactile stimulation may be yet another method for reducing VIMS (Gálvez-García et al., 2017). While these methods are promising, there has been little research on mitigation tasks that could be performed within a VE.
Real Natural Decay
A common non-invasive VIMS mitigation technique is natural decay, wherein an individual sits calmly with their eyes open or shut for a given extended period of time (Kennedy and Fowlkes, 1992). It has been suggested that the amount of natural decay recovery time is similar to the amount of virtual time (Baltzley et al., 1989). At least one study has shown that VIMS from 15 min of VR exposure was significantly reduced after 15 min of natural decay (Curtis et al., 2015); however, symptoms of VIMS have been observed up to 24 h after exposure (Baltzley et al., 1989), and the decay time can vary among individuals with a factor of 100 to 1 (Lackner, 2014). The potentially large amount of time required for natural decay to eliminate VIMS symptoms suggests that additional mitigation techniques are needed to expedite recovery.
Real Hand–Eye Coordination Task
Champney et al. (2007) investigated alternative strategies for re-adapting virtual reality users to the real world with a hand–eye coordination task that recalibrates the sensory systems. The task involved a peg-in-hole task wherein participants used a 25-hole pegboard and had to accurately insert a longer wooden peg into and out of the holes, one at a time. After 1 h in a virtual environment, participants who completed the hand–eye coordination task had a significant reduction in VIMS. A more recent study also found that the real hand–eye task significantly mitigated VIMS (Curtis, 2014). It is possible that hand–eye coordination tasks require ocular focus and proprioception to accomplish their respective tasks, thus reconciling sensory systems and reestablishing depth perception. Both natural decay and hand–eye coordination tasks are effective for users who exit VR to re-adapt their senses; however, they do not provide VIMS relief for users need to remain exposed to VR stimuli for extended periods of time without exiting.
Virtual Mitigation
VIMS mitigation within VR could allow users to remain fully immersed in a virtual environment without debilitating sickness symptoms. Curtis et al. (2015) expanded on Champney's work by designing a virtual version of the peg-in-hole hand–eye coordination task. Participants were presented with an identical virtual pegboard that included 25 pegs (five rows, five columns) with different peg colors in each row. The participants were required to use a Logitech gamepad controller to place the pegs. This task was performed for up to 15 min or until the task was completed. In a comparison of VIMS mitigation between real natural decay and the virtual hand–eye task, both conditions significantly reduced symptoms and there were no significant task group differences. However, the real hand–eye task resulted in lower VIMS than the virtual hand–eye task. It is also worth noting that Curtis (2014) did not find significant VIMS mitigation differences between the real natural decay and the virtual natural decay conditions, but no other research could be found regarding virtual natural decay. These findings suggest that a virtual hand–eye coordination task could be a potential solution for reducing VIMS symptoms while remaining in a virtual environment. Given the limited research in the area, additional exploration is needed to better understand how it compares to the real hand–eye coordination task, real natural decay, and virtual natural decay.
Study Motivation
VIMS symptoms of nausea, oculomotor strain, and disorientation pose a potential barrier to an optimal VR experience. While it is clear that VIMS occurs during and after VR exposure, much less is known about how to effectively and efficiently mitigate sickness (Champney et al., 2007). Sickness due to VR exposure can include nausea, oculomotor strain, and disorientation. These side effects may make VR too uncomfortable for many users and ultimately limit the widespread adoption and growth of VR. Limited research has examined VIMS mitigation techniques within and outside of a virtual environment, including natural decay and a hand–eye coordination tasks (e.g., Champney et al., 2007; Curtis et al., 2015). Some research has suggested these methods could be effective, but it remains unclear how virtual and real-world natural decay or hand–eye coordination tasks compare to one another. Thus, the purpose of the current research is to directly compare the effectiveness of a real-world hand–eye coordination task, real-world natural decay, virtual hand–eye coordination task, and virtual natural decay in mitigating VIMS. Understanding the relative effectiveness of various mitigation techniques will bolster recovery and readaptation knowledge and inform the development of future mitigation tasks, in turn reducing the risk posed by VIMS following VR exposure.
Materials and Methods
Objective
While author Curtis completed a master's thesis (2014) based on a subset of the data analyzed for this paper, that work has not been published in the academic literature. The current paper analyzes those unpublished data using new methods to explore the effectiveness of four mitigation methods and the impact of individual differences on susceptibility and recovery.
Participants
The sample included 57 participants (21 females, 36 males) ranging in age from 18 to 38 (M = 21.75 years old). Participants were recruited from Iowa State University and were compensated with $20 at the completion of the study. Potential participants were screened for and excluded based on a history of seizures or for having taken any motion sickness medication in the prior 24 h. Most respondents reported never or seldom having car sickness (78.3%), plane sickness (84%), sea sickness (83.1%), and train sickness (86.8%). Table 1 summarizes the participant demographic descriptive statistics. This study was approved by the Iowa State University Institutional Review Board. Participants provided their written informed consent to participate in this study.
Experimental Design Overview
Maze
The experiment was divided into two phases: (1) maze run to induce VIMS and (2) mitigation. In the maze phase, participants navigated the “Corn Maze” virtual environment (VE), which was designed to cause virtually induced motion sickness (VIMS). There were two independent variables in the experiment: Mitigation (4 levels) and Movement Control (2 levels). The subsequent mitigation phase tested mitigation techniques (described in the section Independent Variables) to assess their efficacy.
In the Maze phase, participants navigated the maze for up to 15 min (or until they felt too sick to continue), then completed one of four possible VIMS mitigation tasks for 15 min. The design of the primary sections of the virtual environment was based on tasks from the Virtual Environment Performance Assessment Battery known to induce VIMS (VEPAB; Lampton et al., 1994). One of these tasks, called “Turns,” consisted of a total of 44 left and right 90° turns while the user briefly lost control of their movement (Curtis et al., 2015). To ensure a consistent VE path for all participants, it included no decision-making points (i.e., no forking paths). Trampolines and spinning rooms were added to serve as rotational and translational scene oscillations (O'Hanlon and McCauley, 1974; Lo and So, 2001). Spiral slides and non-descript ramps were also included to reduce the number of visual cues the participants could use to determine motion. In addition, the forward movement speed was changed during the virtual environment without indication, reducing the participants' feeling of control. An area in which participants had no control at all and moved at a very rapid pace was also included to induce sickness (Dong et al., 2011). The maze was navigated using the Logitech Dual Action gamepad; pushing the joystick forward led to forward movement. The maze took approximately 7 min to complete, and participants were tasked with completing the virtual environment twice, for a total stimulus exposure of about 15 min. The Corn Maze was designed and run using the Unity 3D game engine (Figure 1). The corn maze code can be found at https://github.com/isuvrac/CyberSickness-Cornmaze.
Headset and Controller
Participants were seated while wearing a HMD and given a gamepad. Participants were able to control movement with the left thumb stick and jump by using their right thumb, which was consistent with typical first-person game controls. The participant's view, or camera in the virtual world, was controlled by the participant's head movement. The head movement was tracked by the Oculus DK1, absent any hardware or software motion sickness mitigation. A typical user of the DK1 would have been able to adjust their FOV via the lenses supplied with the headset. Participants were limited to a single lens setup for 20/20 or corrected vision, which was asked prior to the start of the session. If the participant was in a “no control” condition, the participant could not navigate via the left thumb stick, normally used for motion, nor jump by pressing the bottom button.
Independent Variables
There were two independent variables: Movement control in the Maze phase (two levels) and migration technique in the Mitigation phase (four levels).
In the maze phase, participants were assigned to a no movement control or movement control group. Those in the no movement control group were not able to manually control progress through the maze, but rather automatically traveled through it (i.e., “on-rails”). The movement control group was able to manually progress through the maze using the joystick. The Maze design also included one short segment in which all users lost control once per lap for approximately 7 s. The Control independent variable, however, was not found to impact results. ANOVAs were completed to determine whether participants with movement control and no movement control throughout the entire maze differed in Maze Sickness or Mitigation Recovery (Table 2). The movement control group did not have any significant impact on Maze Sickness {[F(1, 55)MSTS = 0.65, p = 0.424]; [F(1, 55)MSN = 2.30, p = 0. 135]; [F(1, 55)MSO = 0.21, p = 0.646]; [F(1, 55)MSD = 0.16, p = 0.693]} or Mitigation Recovery {[F(1, 53)MRTS = 0.18, p = 0.674]; [F(1, 53)MRN = 0.53, p = 0.471]; [F(1, 53)MRO = 0.83, p = 0.366]; [F(1, 55)MRD = 1.00, p = 0.321]}. Based on these results, the control and no control groups were collapsed for further analysis.
In the mitigation phase, each participant was randomly assigned to one of four mitigation experimental task groups: real natural decay (RND), real hand–eye coordination (RHE), virtual natural decay (VND), or virtual hand–eye coordination (VHE). The RND required participants to sit quietly with their eyes open or closed for 15 min while not receiving any virtual or real stimuli. In the RHE (Figure 2, left), participants were instructed to place a peg into straw-like holes from back to front (Champney et al., 2007; Stone et al., 2012). The pegboard included 25 pegs (five rows, five columns), and each row had different peg colors. This task was performed until participants completed the pegboard or until 15 min elapsed (whichever came first). The VHE (Figure 2, right) was the virtual reality equivalent of the real hand–eye coordination task. Using a Razer Hydra with handheld magnetic tracking controllers, participants were required to guide a virtual peg into a virtual pegboard for 15 min or until the task was completed. In the VND (Figure 3), participants sat in a calm VE wherein they could look around at fields and mountains. There was no locomotion within the VE. This was completed for 15 min.
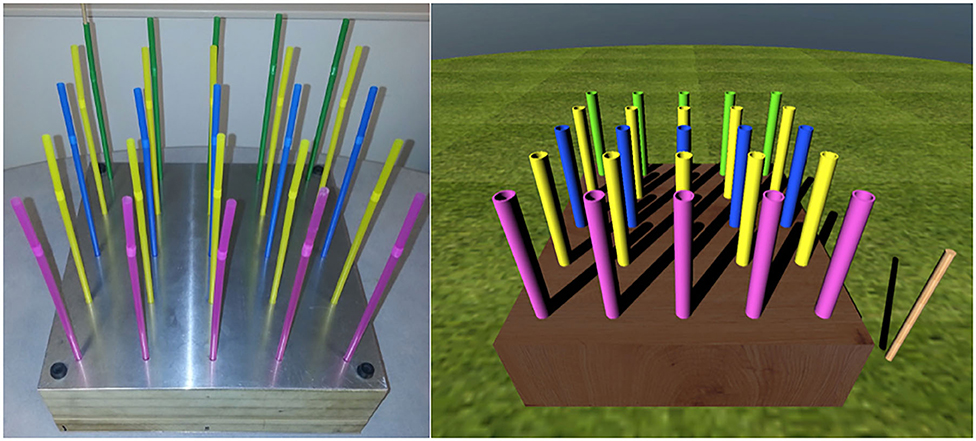
Figure 2. The real hand–eye coordination task (left) (Stone et al., 2012) and the virtual hand–eye coordination task (right).
Measures
Demographics and Background
Demographic information (Table 1) was gathered on age and gender (0 = female, 1 = male). Background information was gathered on whether or not the participant played video games (0 = no, 1 = yes), average weekly video game experience (0 = less than 1 h per week, 1 = 1–5 h per week, 2 = 6–10 h per week, 3 = 10+ h per week, prior experiences with VR (0 = no, 1 = yes), amount of sleep (0 = less than normal, 1 = normal, 2 = more than normal), and if they had eaten the day of the study (0 = no, 1 = yes).
Dependent Variables
The dependent variables were calculated using the Simulator Sickness Questionnaire (SSQ) (Kennedy et al., 1993). Responses to each item were scored on a four-point scale from “None” (0), “Slight” (1), “Moderate” (2), or “Severe” (3). The questionnaire is composed of three subscales: nausea, oculomotor, and disorientation. To calculate the score for each subcategory, one must add together all the relevant symptom responses and multiply by the subcategory's multiplier. Likewise, the total severity (TS) score is a sum of the symptom responses given by the participant multiplied by the TS multiplier. The relationship between the subcategory scores and TS scores is not simply additive. The minimum value for each score is 0, signifying no motion sickness symptoms. Higher scores signify more severe symptoms. The maximum value for each score is 200.34 for nausea (N), 159.18 for oculomotor (O), 292.32 for disorientation (D), and 235.62 for TS (Kennedy et al., 1993). Over a large sample of aircraft pilots experiencing aircraft flight simulators, Kennedy et al. (1993) observed an average total score of 9.8 (SD = 15.0).
Procedure and Timeline for Measuring Dependent Variables
The study timeline began with a baseline measurement, followed by maze and mitigation phases, ending with debriefing Figure 4. At baseline, participants completed the informed consent and demographic questionnaire. The SSQ was verbally measured eight times throughout the experiment: once at baseline (SSQ-base-1), three times during the maze [SSQ-maze-2 (5 min into maze), SSQ-maze-3 (10 min into maze), SSQ-maze-4 (15 min into maze)], three times during the mitigation phase [SSQ-mit-5 (0 min into mitigation), SSQ-mit-6 (5 min into mitigation), SSQ-mit-7 (10 min into mitigation)], and once after debriefing (SSQ-debr-8). Participants were allowed to exit the maze phase of the study at any point that they felt too uncomfortable to continue; not everyone completed all three of the maze SSQ measurements. It should be noted that the SSQ numbering was determined by a specific timepoint, and not based on the number of surveys a participant took. If a participant was not able to complete a specific timepoint, that data is considered missing or incomplete. The SSQ was used to create three dependent variables (DVs): Final Maze SSQ, Maze Sickness, and Mitigation Recovery (Table 2).
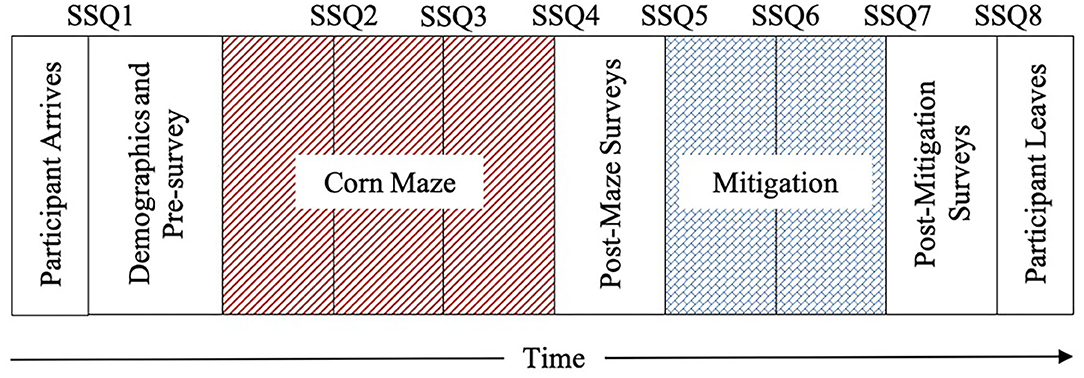
Figure 4. Experimental timeline adapted from Curtis (2014).
Final Maze SSQ
The SSQ was taken three times during the maze portion of the experiment at 5 min (SSQ-maze-2), 10 min (SSQ-maze-3), and 15 min (SSQ-maze-4) into the maze. Because participants were allowed to exit the maze phase of the study at any point that they felt too uncomfortable to continue, not everyone completed all three of the maze SSQ measurements. Therefore, the Final Maze SSQ reflects the final SSQ measurement each participant completed before exiting the maze. The Final Maze SSQ was calculated for the SSQ TS and subscales N, O, and D, and those were used to create the Maze Sickness and Mitigation Recovery dependent variables.
Maze Sickness
Maze Sickness was calculated by subtracting the baseline SSQ (SSQ-base-1 taken prior to entering the maze) from the Final Maze SSQ (Table 2). The Maze Sickness variable therefore reflects each participant's amount of sickness increase before VR exposure to the mitigation technique. The Maze Sickness variable relates to susceptibility prior to mitigation, so group differences in this could only be examined for gender and video game play experience. Maze Sickness was calculated for the SSQ TS (MSTS), N (MSN), O (MSO), and D (MSD).
Mitigation Recovery
The SSQ was taken three times during the mitigation portion of the experiment: At the beginning of mitigation (0 min into mitigation, SSQ-mit-5), 5 min into mitigation (SSQ-mit-6), and 10 min into mitigation (SSQ-mit-7). Mitigation Recovery was calculated by subtracting the final SSQ measurement during mitigation (SSQ-mit-7) from the Final Maze SSQ to reflect each participant's amount of recovery during their respective mitigation task (Table 2). The Mitigation Recovery variable relates to adaptation from sickness from mitigation tasks. Mitigation Recovery was calculated for the SSQ TS (MRTS) and subscales N (MRN), O (MRO), and D (MRD).
Apparatus
An Oculus Rift DK1 HMD was used to display graphics and track user movement for an immersive experience. The Oculus Rift weighed 0.38 kg and had a 110-degree field of view with a total resolution of 1,280 × 800 pixels. It was configured in stereo mode throughout the duration of the experiment. The maze was navigated using the Logitech Dual Action gamepad. For those completing the VHE, directions were given on how to navigate the peg through the peg-in-hole scene using a Razer Hydra handheld controller.
Data Analysis Approach
First, descriptive statistics were assessed for each for the experimental mitigation groups (RND, RHE, VND, and VHE) and the dependent variables: Final Maze SSQ, Maze Sickness, and Mitigation Recovery (Section Descriptive Statistics). Second, group differences during the maze were examined using one-way ANOVAs and Scheffe post-hoc analyses (when there were more than two groups. i.e., there were four mitigation groups) in three sections: Experimental Mitigation Group Differences in Maze Sickness; Gender Group Differences in Maze Sickness; and Video Game Play Group Differences in Maze Sickness. Third, group differences during mitigation were examined using one-way ANOVAs and Scheffe post-hoc analyses in three sections: Experimental Mitigation Group Differences in Mitigation Recovery; Gender Group Differences in Mitigation Recovery; and Video Game Play Group Differences in Mitigation Recovery.
Interactions between gender and videogame play on Maze Sickness and Mitigation Recovery could not be assessed because the group sizes were too disproportional (i.e., Female-Video Game = 4, Female-No Video Game = 17, Male-Video Game = 34, Male-No Video Game Play = 2). Based on the sample, the majority of females were non-game players and the majority of males were video game players. More data is needed to tease apart the effects of gender and video game play.
All analyses were completed in SPSS version 26. Eta-squared was used to measure effect size, where 0.02 is considered a small effect, 0.13 a medium effect, and 0.26 a large effect (Cohen, 1988). The assumptions for all ANOVAs were met.
Results
Descriptive Statistics
Over 70% of participants remained in the Corn Maze through SSQ-maze-4 (n = 40). On average, participants reached their highest level of sickness during SSQ-maze-3. One participant did not complete SSQ-maze-3 and 17 participants did not complete SSQ-maze-4. Additionally, two participants have incomplete data for SSQ-mit-7 and SSQ-debrief-8.
The SSQ TS, N, O, and D raw mean scores at each SSQ measurement point for the entire sample are presented in Table 3. During mitigation, there is a slight increase in overall average SSQ scores for all subscales. Paired sample t-tests reveal that the change in SSQ score between SSQ-mit-5 and SSQ-mit-7 was insignificant for TS [t(54) = −1.627, p = 0.110], N [t(54) = −1.626, p = 0.110], O [t(54) = −1.707, p = 0.094], and D [t(54) = −1.135, p = 0.262], indicating that the increase in scores was minimal and non-impactful. Final Maze SSQ scores for TS ranged from 0 to 175.78 (m = 77.36, SD = 49.74); N ranged from 0 to 162.18 (m = 64.44, SD = 41.75); O ranged from 0 to 136.44 (m = 55.32, SD = 35.67); and D ranged from 0 to 250.56 (m = 92.31, SD = 70.67), indicating a broad range of differences between individuals. Table 4 provides the descriptive statistics for Maze Sickness and Mitigation Recovery for each SSQ subscale. Maze Sickness VIMS variables (i.e., MSTS, MSN, MSO, and MSD) ranged from as low as −15.16 up to 236.64. Comparatively, Mitigation Recovery VIMS variables (i.e., MRTS, MRN, MRO, and MRD) ranged from −111.36 to 180.96. These ranges suggest a substantial amount of individual variability in sickness susceptibility and recovery. Finally, in regard to the experimental mitigation groups, the RND experimental group had n = 16, the RHE experimental group had n = 15, the VND experimental group had n = 14, and the VHE experimental group had n = 12.
Group Differences During the Maze
Experimental Mitigation Group Differences in Maze Sickness
There were no significant mitigation group differences in MSTS, MSN, MSO, or MSD {[F(3, 53)MSTS = 0.60, p = 0.621]; [F(3, 53)MSN = 0.99, p = 0.406]; [F(3, 53)MSO = 0.57, p = 0.636]; [F(3, 53)MSD = 0.28, p = 0.842]} (Table 5). This indicates that none of the mitigation groups were predisposed to more VIMS due to sampling bias.
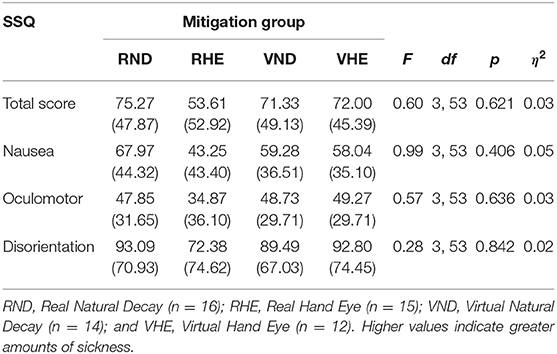
Table 5. Maze sickness mean scores, standard deviations, and experimental mitigation group differences using ANOVAs.
Gender Group Differences in Maze Sickness
Women had significantly more VIMS than men for MSTS, MSN, MSO, and MSD {[F(1, 55)MSTS = 8.39, p = 0.005]; [F(1, 55)MSN = 9.84, p = 0.003]; [F(1, 55)MSO = 4.92, p = 0.031]; [F(1, 55)MSD = 7.68, p = 0.008]} (Table 6). This indicates that women experienced more sickness from the corn maze than the men did.
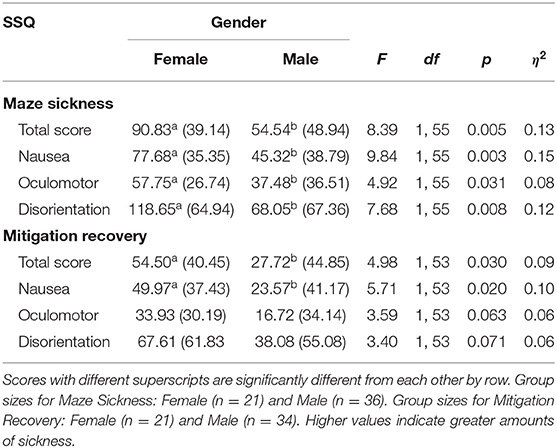
Table 6. Maze sickness and mitigation recovery mean scores, standard deviations, and gender group differences using ANOVAs.
Video Game Play Group Differences in Maze Sickness
Those who play video games had significantly less VIMS than those who did not play video games for MSTS, MSN, MSO, and MSD [(F(1, 55)MSTS = 8.74, p = 0.005]; [F(1, 55)MSN = 7.90, p = 0.007]; [F(1, 55)MSO = 4.82, p = 0.032)]; [F(1, 55)MSD = 10.40, p = 0.002)] (Table 7).
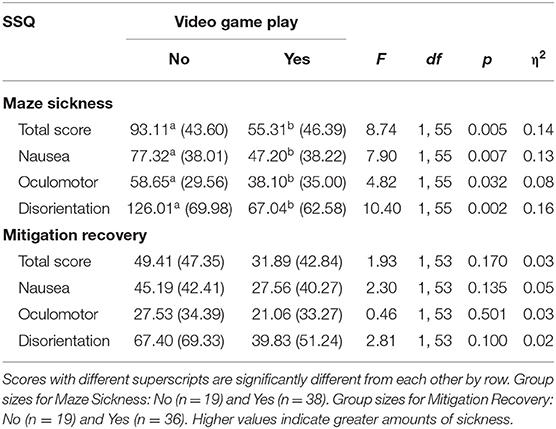
Table 7. Maze sickness and mitigation recovery mean scores, standard deviations, and video game play group differences using ANOVAs.
Group Differences During Mitigation
Experimental Mitigation Group Differences in Mitigation Recovery
There were significant mitigation group differences in MRTS, MRN, and MRO {[F(1, 53)MRTS = 4.98, p = 0.030]; [F(1, 53)MRN = 5.71, p = 0.020]; [F(1, 53)MRO = 5.13, p = 0.004]} (Table 8, Figure 5). There were no significant group differences in MRD [F(1, 53)MRD = 2.34, p = 0.084]. Specifically, the RND group experienced significantly more recovery than the VHE group for MSTS, MRN, and MRO [(RND-VHEMRTS = 56.72, 95% CI [10.18,103.25], p = 0.011); (RND-VHEMRN = 50.84, 95% CI [7.46,94.22], p =.015); (RND-VHEMRO = 42.85, 95% CI [8.66,77.05], p =.008)].
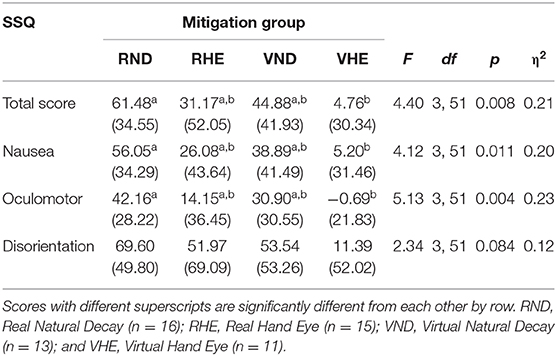
Table 8. Mitigation recovery mean scores, standard deviations, and experimental mitigation group differences using ANOVAs.
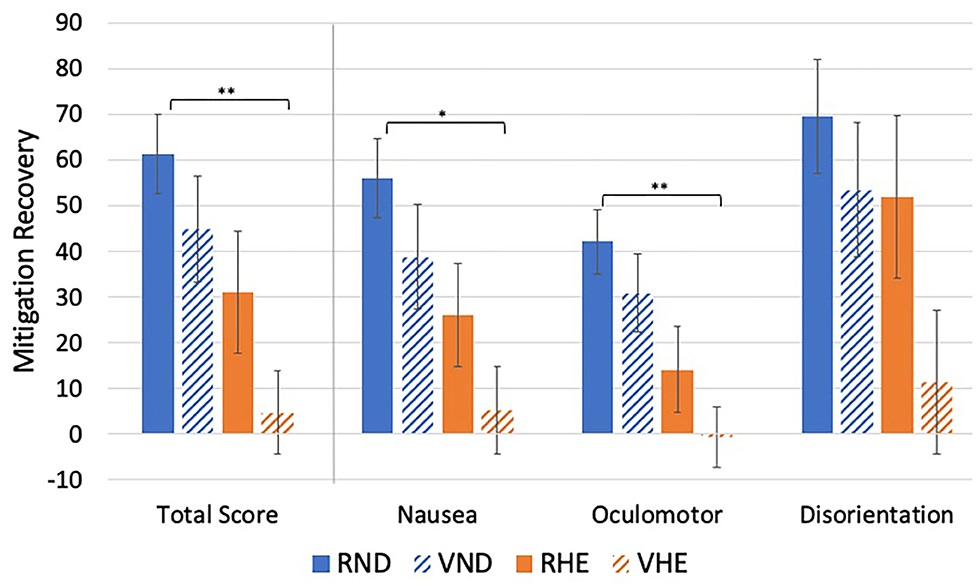
Figure 5. Experimental mitigation group mean differences in mitigation recovery. *p < 0.05, **p < 0.01. Higher values indicate greater amounts of recovery.
Gender Group Differences in Mitigation Recovery
Women had significantly more VIMS recovery than men for MSTS and MSN {[F(1, 53)MRTS = 4.98, p = 0.030]; [F(1, 53)MRN = 5.71, p = 0.020]} (Table 6, Figure 6). Comparatively, there were no significant gender differences in MRO or MRD, although they approached significance {[F(1, 53)MRO = 3.59, p = 0.063]; [F(1, 53)MRD = 3.40, p = 0.071]}.
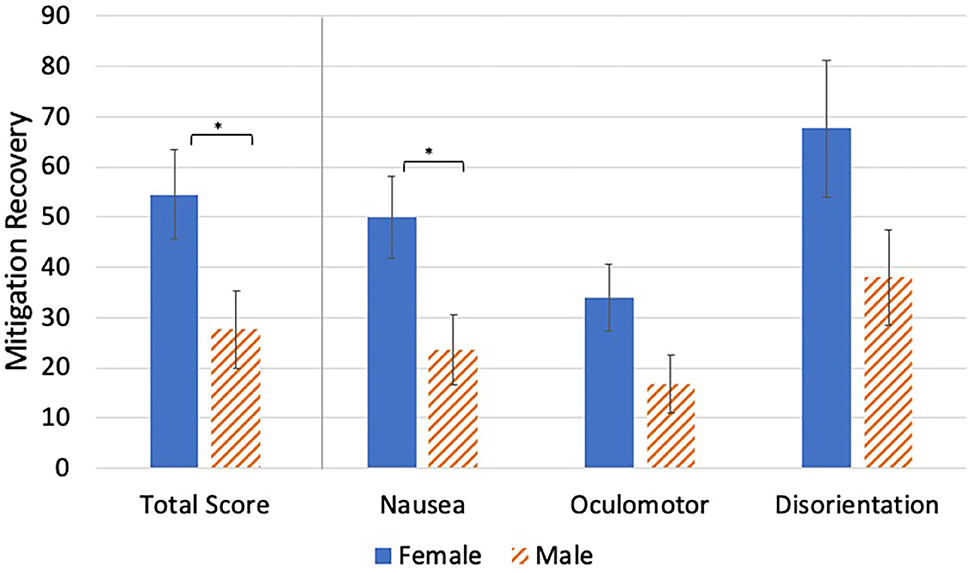
Figure 6. Video game play group mean differences in mitigation recovery. *p < 0.05. Higher values indicate greater amounts of recovery.
Video Game Play Group Differences in Mitigation Recovery
There were no significant video game play group differences in VIMS recovery {[F(1, 53)MRTS = 1.93, p = 0.170]; [F(1, 53)MSN = 2.30, p = 135]; [F(1, 53)MSO = 0.46, p = 0.501]; [F(1, 53)MSD = 2.81, p = 0.100]} (Table 7, Figure 7).
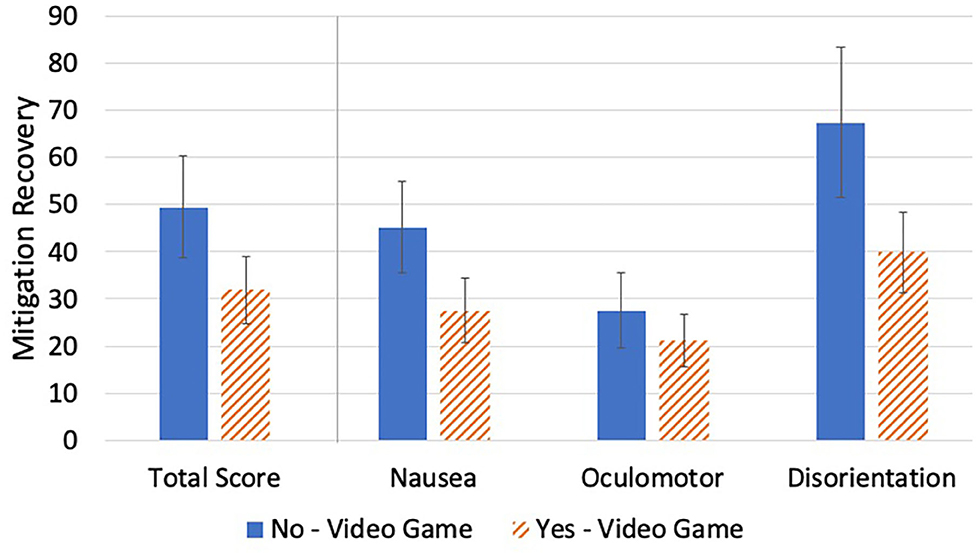
Figure 7. Video game play group mean differences in mitigation recovery. No significant differences in Mitigation Recovery were found.
Discussion
The purpose of this study was to explore the effectiveness of four VIMS mitigation methods (i.e., RND, VND, RHE, VHE) using the Corn Maze designed to induce sickness symptoms measured by Kennedy's SSQ. All mitigation methods reduced VIMS to a certain extent. However, RND resulted in greater Mitigation Recovery, followed by VND, the RHE, and VHE. The largest amount of Mitigation Recovery was seen within the disorientation subscale, followed by the nausea and oculomotor subscales. This is consistent with the VR exposure profile seen in other SSQ research: disorientation is greater than nausea, which is greater than oculomotor strain (Stanney et al., 1997).
Mitigation Techniques
Consistent with previous work (Curtis et al., 2015), RND was the most effective VIMS mitigation technique. However, there were no significant differences in Mitigation Recovery between the RND and VND groups. This suggests that a VR scene could possibly aid in VIMS recovery without removing the user from the VE. What remains unclear for both the RND and VND is how much time beyond exposure is needed for users to completely recover from VIMS, or if that is always possible within VND. The prolonged measurement period needed to measure complete recovery was beyond the scope of the current study. Future research should consider measuring VIMS periodically after exposure (Baltzley et al., 1989), such as once every 15 min up to 1 h after exposure (e.g., Champney et al., 2007) or even longer to better illuminate the amount of time natural decay requires.
These results suggest that mitigation within a VE is possible but may require certain alterations to perform as well as RND. For instance, the VND environment allowed participants to look around a scene with a grass, mountains, and clouds. Because of this rotational visual stimulus within the VND environment, it is possible that ocular focus and proprioception were still engaged, perhaps impeding the reconciliation of the sensory systems (Champney et al., 2007). In RND, participants sat quietly in a room with their eyes open or shut, so there may have been fewer visual stimuli to focus on compared to the VND environment. Because there is extremely limited research on VND (e.g., Curtis, 2014), these findings bolster credibility for the benefits of VND. It remains unclear if virtual natural decay requires prolonged recovery periods, like the real-world version, in order to be fully effective. Future research would benefit from implementing this mitigation technique and measuring VIMS for at least an hour after exposure. These results were not expected. Effectiveness of virtual mitigation tasks could be improved as equipment advances, such as with higher resolution, improved latency, and lower weight. In addition to measuring VIMS for longer post-exposure and with advanced hardware, future research should explore physiological measurements of sickness, such as electrodermal activity or heart rate, to help validate subjective self-assessments of sickness.
Both natural decay mitigation techniques resulted in slightly more, but not significantly more, Mitigation Recovery than the hand–eye coordination techniques. Previous research has suggested that a hand–eye task is more effective for VIMS recovery than natural decay because it recalibrates the sensory systems (Champney et al., 2007), an effect that is not supported by the current findings. These differences may be due in part to the hardware and controller used. For the current RHE, the pegboard was larger than in the Champney et al. study, requiring movement of the entire arm, thus not requiring the same fine motor skills, perhaps impacting effectiveness.
The VHE task was by far the least effective mitigation technique. The VHE group had significantly less Mitigation Recovery than the RND. The motivation behind utilizing a VHE task was that it could potentially provide a task that engages ocular focus and proprioception, which may reduce sickness symptoms per Champney et al. (2007), while remaining immersed in a virtual environment. There are several possible ergonomic explanations for the gap between VHE and RHE scores. Because of the Razer Hydra interface, which felt somewhat like controlling the peg by moving a television remote-sized object through space, the VHE task did not have haptic or force feedback to indicate to the user than the peg and a straw collided. This issue was reinforced by participants mentioning how difficult it was to determine the point of contact. Second, the Razer Hydra was heavier than what participants experienced in the real-world version of the task, lifting the controller rather than a lightweight peg. Third, the real task required the index finger, middle finger, and thumb to pick up the virtual peg and mostly lower arm movement to place the peg. This is an example of finer motor control, closer to a third-class ergonomic motion vs. the less fine motor task of moving the controller with primarily whole arm and wrist (a fourth-class motion) (Freivalds and Niebel, 2013). Finally, participants had a fixed point of view (POV) when completing the VHE task. Not having headtracking of the headset relative to the peg board could yield an awkward positioning above the board. These differences limited the physical affordances of the hand–eye task and potentially altered the experience that would recalibrate one's system to mitigate VIMS. Future iterations of VHE should consider implementing more natural interfaces, perhaps using a controller such as the Phantom or Tap Strap, which could provide haptic feedback for finger-level motion. Further, it could be beneficial to bolster the virtual experience by including 3D sound and/or head-tracking during the peg-in-hole task to increase its similarity to the real peg-in-hole task. These additional affordances may provide the user with a more realistic virtual peg-in-hole task, refined motor control, and more realistic visual orientation, perhaps improving its ability to mitigate sickness. A broader review of the fidelity of the virtual hand–eye coordination task using the lens of authenticity (Gilbert, 2016) or coherence (Skarbez et al., 2018) might be valuable to ensure that the types of fidelity required by the task match the fidelity of the system.
It is interesting, yet unclear, why VIMS slightly increased after the beginning of mitigation. While there was a slight increase and plateau in SSQ TS, N, O, and D across SSQ-mit-5, SSQ-mit-6, and SSQ-mit-7, paired sample t-tests revealed that there were not statistically significant changes in these measurement points. Regardless, future research should closely monitor changes in VIMS during mitigation tasks, use additional measures, such as physiological indicators of sickness, and employ state-of-the-art HMD hardware to cross-validate VIMS experiences.
Within the present study, RND was the most effective mitigation technique; however, all of the mitigation tasks did reduce VIMS. It was somewhat disappointing that RND remained the most impactful mitigation technique, as the other three offered promising potential alternatives. We believe that future research should consider improving the fidelity within the VEs, utilize the most up-to-date hardware, and refine the dexterity capabilities for virtual tasks, like the VHE. It is possible that certain mitigation techniques are more effective based on individual differences, so additional research with larger sample sizes are needed to examine interactions and predictive relationships among individual characteristics and mitigation techniques.
Individual Differences
Gender differences in VIMS were consistent with previous literature that found women to be more susceptible to sickness than men (Koslucher et al., 2015, 2016; Munafo et al., 2017). There has been less research on gender differences in mitigation, however, and this study offers the interesting result that women experienced more recovery than men during mitigation, pointing to a future area of research. Some theorize that gender differences in VIMS could be due to hardware differences (Fulvio et al., 2020). For example, default HMD settings are generally sized to fit the interpupillary distance of men, rather than women (Fulvio et al., 2020). When the interpupillary distance is not calibrated to women, it is possible that they will experience more VIMS. It is unclear why there were no video game play group differences in Mitigation Recovery; however, it is possible that video game players are more de-sensitized to the visual effects of virtual worlds, thus more resistant to VIMS.
Limitations
A limitation of the current sample is that it primarily consisted of men who play video games and women who did not play video games (Table 3). Due to this confound in sampling, the comparison between men and women and the comparison between video game players and non-video game players yielded similar results. As such, more data is needed to tease apart the effects of gender and video game play. Future work exploring the independent effects of gender and video game experience on VIMS would help contribute to the broader understanding of cybersickness. It is also possible that the verbal administration of the SSQ during both the maze and the mitigation tasks could have interrupted user attention, potentially affecting presence and increasing sickness levels. Further investigations would benefit from comparing sickness between participants when the SSQ is administered throughout tasks vs. only at the end of tasks. The RHE did not result in more Mitigation Recovery than RND, which may suggest that the experimental equipment was insufficient to realize the benefits, and should thus be reconceptualized in future research with more state-of-the-art hardware and particular attention to the experimental setup. Ongoing work should apply higher resolution HMDs with head tracking to reduce sickness during mitigation and properly highlight the effectiveness of mitigation techniques, as the Oculus Rift DK1 was earlier hardware.
Data Availability Statement
The raw data supporting the conclusions of this article will be made available by the authors, without undue reservation.
Ethics Statement
The studies involving human participants were reviewed and approved by Institutional Review Board, Iowa State University. The patients/participants provided their written informed consent to participate in this study.
Author's Note
The present research extends VIMS research to compare the effectiveness of four mitigation methods, within and outside of VR, following exposure in order to better understand VIMS recovery. These findings extend current knowledge by providing empirical evidence for the effectiveness of not only RND, but also VND, RHE, and, to a lesser extent, VHE. Although VHE was the least effective of all mitigation techniques, modifications to the hardware and controller interface provide promising future steps for the development of VIMS mitigation techniques during VE immersion.
Author Contributions
AJ: Primary authorship throughout document, data analysis, figures/tables creation. NC: Secondary authorship throughout document, data analysis, figure/tables creation. CM and MC: Study design, data collection, text revisions. MD: Authorship throughout, figures/tables revisions, study design. SG: Authorship throughout, figures/tables revisions, reference list. All authors contributed to the article and approved the submitted version.
Conflict of Interest
The authors declare that the research was conducted in the absence of any commercial or financial relationships that could be construed as a potential conflict of interest.
Acknowledgments
Thanks to Kayla Dawson, Kelli Jackson, and Liat Litwin for the design of the original Corn Maze; to Xin Wang for aiding in data collection; and to professors Jonathan Kelly and Richard Stone for consultation during this process. This material supported in part by the National Science Foundation Grant No. 1156841.
References
Ames, S. L., Wolffsohn, J. S., and Mcbrien, N. A. (2005). The development of a symptom questionnaire for assessing virtual reality viewing using a head-mounted display. Optom. Vis. Sci. 82, 168–176. doi: 10.1097/01.OPX.0000156307.95086.6
Baltzley, D. R., Kennedy, R. S., Berbaum, K. S., Lilienthal, M. G., and Gower, D. W. (1989). The time course of postflight simulator sickness symptoms. Aviat. Space. Environ. Med. 60, 1043–1048.
Boyd, D. (2014). Is the Oculus Rift sexist? Quartz Daily Brief. Available online at: https://qz.com/192874/is-the-oculus-rift-designed-to-be-sexist/ (accessed August 20, 2020).
Buker, T. J., Vincenzi, D. A., and Deaton, J. E. (2012). The effect of apparent latency on simulator sickness while using a see-through helmet-mounted display: reducing apparent latency with predictive compensation. Hum. Factors 54, 235–249. doi: 10.1177/0018720811428734
Champney, R. K., Stanney, K. M., Hash, P. A. K., Malone, L. C., Kennedy, R. S., and Compton, D. E. (2007). Recovery From virtual environment exposure: expected time course of symptoms and potential readaptation strategies. Hum. Factors 49, 491–506. doi: 10.1518/001872007X200120
Cohen, J. (1988). Statistical Power Analysis for the Behavioral Sciences, 2nd Edn. Mahwah: Lawrence Erlbaum Associates.
Curry, C., Li, R., Peterson, N., and Stoffregen, T. A. (2020). Cybersickness in virtual reality head-mounted displays: examining the influence of sex differences and vehicle control. Int. J. Hum. Comput. Interact. 36, 1161–1167. doi: 10.1080/10447318.2020.1726108
Curtis, M. K. (2014). Investigation of visually induced motion sickness: a comparison of mitigation techniques in real and virtual environments (Publication Number 14094) (Masters Thesis). Graduate Theses and Dissertations, Iowa State University, Ames, IA, United States. Available online at: https://lib.dr.iastate.edu/etd/14094 (accessed July 1, 2020).
Curtis, M. K., Dawson, K., Jackson, K., Litwin, L., Meusel, C., Dorneich, M. C., et al. (2015). Mitigating visually induced motion sickness a virtual hand-eye coordination task. Proc. Hum. Factors Ergon. Soc. Annu. Meet. 59, 1839–1843. doi: 10.1177/1541931215591397
Davis, S., Nesbitt, K., and Nalivaiko, E. (2014). “A systematic review of cybersickness,” in Proceedings of the 2014 Conference on Interactive Entertainment (Newcastle, NSW). doi: 10.1145/2677758.2677780
Dong, X., Yoshida, K., and Stoffregen, T. A. (2011). Control of a virtual vehicle influences postural activity and motion sickness. J. Exp. Psychol. 17, 128–138. doi: 10.1037/a0024097
Duzmańska, N., Strojny, P., and Strojny, A. (2018). Can simulator sickness be avoided? A review on temporal aspects of simulator sickness. Front. Psychol. 9:2132. doi: 10.3389/fpsyg.2018.02132
Estrada, A., LeDuc, P. A., Curry, I. P., Phelps, S. E., and Fuller, D. R. (2007). Airsickness prevention in helicopter passengers. Aviat. Space. Environ. Med. 78, 408–413.
Freivalds, A., and Niebel, B. (2013). Niebel's Methods, Standards, and Work Design. New York, NY: Mcgraw-Hill Higher Education.
Fulvio, J. M., Ji, M., and Rokers, B. (2020). Variation in visual sensitivity predicts motion sickness in virtual reality. bioRxiv. doi: 10.1101/488817
Gálvez-García, G., Albayay, J., Rehbein, L., and Tornay, F. (2017). Mitigating simulator adaptation syndrome by means of tactile stimulation. Appl. Ergon. 58, 13–17. doi: 10.1016/j.apergo.2016.05.004
Gamito, P., Oliveira, J., Morais, D., Baptista, A., Santos, N., Soares, F., et al. (2010). Training presence: the importance of virtual reality experience on the “sense of being there”. Annu. Rev. Cybertherapy Telemed. 2010, 128–133.
Gamito, P., Oliveira, J., Santos, P., Morais, D., Saraiva, T., Pombal, M., et al. (2008). Presence, immersion and cybersickness assessment through a test anxiety virtual environment. Annu. Rev. Cyber Ther. Telemed. 6, 83–90.
Gilbert, S. (2016). Perceived realism of virtual environments depends on authenticity. Presence 25, 322–324. doi: 10.1162/PRES_a_00276
Golding, J. F. (2006). Motion sickness susceptibility. Auton. Neurosci. 129, 67–76. doi: 10.1016/j.autneu.2006.07.019
Jerome, C., Darnell, R., Oakley, B., and Pepe, A. (2005). The effects of presence and time of exposure on simulator sickness. Proc. Hum. Factors Ergon. Soc. Annu. Meeting 49, 2258–2262. doi: 10.1177/154193120504902609
Kennedy, R. S., Drexler, J., and Kennedy, R. C. (2010). Research in visually induced motion sickness. Appl. Ergon. 41, 494–503. doi: 10.1016/j.apergo.2009.11.006
Kennedy, R. S., and Fowlkes, J. E. (1992). Simulator sickness is polygenic and polysymptomatic: implications for research. Int. J. Aviat. Psychol. 2, 23–38. doi: 10.1207/s15327108ijap0201_2
Kennedy, R. S., Lane, N. E., Berbaum, K. S., and Lilienthal, M. G. (1993). Simulator sickness questionnaire: an enhanced method for quantifying simulator sickness. Int. J. Aviat. Psychol. 3, 203–220. doi: 10.1207/s15327108ijap0303_3
Keshavarz, B., and Hecht, H. (2011). Validating an efficient method to quantify motion sickness. Hum. Factors 53, 415–426. doi: 10.1177/0018720811403736
Keshavarz, B., Ramkhalawansingh, R., Haycock, B., Shahab, S., and Campos, J. (2018). Comparing simulator sickness in younger and older adults during simulated driving under different multisensory conditions. Transp. Res. Part F 54, 47–62. doi: 10.1016/j.trf.2018.01.007
Kim, H. K., Park, J., Choi, Y., and Choe, M. (2018). Virtual reality sickness questionnaire (VRSQ): motion sickness measurement index in a virtual reality environment. Appl. Ergon. 69, 66–73. doi: 10.1016/j.apergo.2017.12.016
Kim, S., Lee, S., Kala, N., Lee, J., and Choe, W. (2018). An effective FoV restriction approach to mitigate VR sickness on mobile devices. J. Soc. Inf. Display 26, 376–384. doi: 10.1002/jsid.669
Klosterhalfen, S., Pan, F., Kellermann, S., and Enck, P. (2006). Gender and race as determinants of nausea induced by circular vection. Gend. Med. 3, 236–242. doi: 10.1016/S1550-8579(06)80211-1
Knight, M. M., and Arns, L. L. (2006). “The relationship among age and other factors on incidence of cybersickness in immersive environment users,” in Proceedings of the 3rd symposium on Applied perception in graphics and visualization (Boston, MA). doi: 10.1145/1140491.1140539
Koslucher, F., Haaland, E., Malsch, A., Webeler, J., and Stoffregen, T. A. (2015). Sex differences in the incidence of motion sickness induced by linear visual oscillation. Aerosp. Med. Hum. Perform. 86, 787–793. doi: 10.3357/AMHP.4243.2015
Koslucher, F., Munafo, J., and Stoffregen, T. A. (2016). Postural sway in men and women during nauseogenic motion of the illuminated environment. Exp. Brain Res. 234, 2709–2720. doi: 10.1007/s00221-016-4675-8
Lackner, J. R. (2014). Motion sickness: more than nausea and vomiting. Exp. Brain Res. 232, 2493–2510. doi: 10.1007/s00221-014-4008-8
Lampton, D. R., Knerr, B. W., Goldberg, S. L., Bliss, J. P., Moshell, J. M., and Blau, B. S. (1994). The virtual environment performance assessment battery (VEPAB): development and evaluation. Presence 3, 145–157. doi: 10.1162/pres.1994.3.2.145
Lewis, T. (2015). When will virtual-reality headsets stop making people sick? Live Science. Available online at: https://www.livescience.com/50129-virtual-reality-nausea-sickness.html (accessed June 28, 2020).
Lo, W. T., and So, R. H. Y. (2001). Cybersickness in the presence of scene rotational movements along different axes. Appl. Ergon. 32, 1–14. doi: 10.1016/S0003-6870(00)00059-4
Miller, K. E., and Muth, E. R. (2004). Efficacy of acupressure and acustimulation bands for the prevention of motion sickness. Aviat. Space. Environ. Med. 75, 227–234.
Min, B.-C., Chung, S.-C., Min, Y.-K., and Sakamoto, K. (2004). Psychophysiological evaluation of simulator sickness evoked by a graphic simulator. Appl. Ergon. 35, 549–556. doi: 10.1016/j.apergo.2004.06.002
Moss, J. D., and Muth, E. R. (2011). Characteristics of head-mounted displays and their effects on simulator sickness. Hum. Factors 53, 308–319. doi: 10.1177/0018720811405196
Mousavi, M., Jen, Y. H., and Musa, S. N. B. (2013). A review on cybersickness and usability in virtual environments. Adv. Eng. Forum 10, 34–39. doi: 10.4028/www.scientific.net/AEF.10.34
Munafo, J., Diedrick, M., and Stoffregen, T. A. (2017). The virtual reality head-mounted display Oculus Rift induces motion sickness and is sexist in its effects. Exp. Brain Res. 235, 889–901. doi: 10.1007/s00221-016-4846-7
Nachum, Z., Shupak, A., Letichevsky, V., Ben-David, J., Tal, D., Tamir, A., et al. (2004). Mal de debarquement and posture: reduced reliance on vestibular and visual cues. Laryngoscope 114, 581–586. doi: 10.1097/00005537-200403000-00036
Nie, G.-Y., Duh, H. B.-L., Liu, Y., and Wang, Y. (2019). Analysis on mitigation of visually induced motion sickness by applying dynamical blurring on a user's retina. IEEE Trans. Vis. Comput. Graph. 26, 2535–2545. doi: 10.1109/TVCG.2019.2893668
O'Hanlon, J. F., and McCauley, M. E. (1974). Motion sickness incidence as a function of the frequency and acceleration of a vertical sinusoidal motion. Aerosp. Med. 45, 366–369. doi: 10.21236/AD0768215
Onuki, Y., Ono, S., and Kumazawa, I. (2017). “Air cushion: a pilot study of the passive technique to mitigate simulator sickness by responding to vection,” in 2017 IEEE Virtual Reality (VR) (Los Angeles, CA), 323–324. doi: 10.1109/VR.2017.7892307
Reason, J. T. (1978). Motion sickness adaptation: a neural mismatch model. J. R. Soc. Med. 71, 819–829. doi: 10.1177/014107687807101109
Rebenitsch, L., and Owen, C. (2016). Review on cybersickness in applications and visual displays. Virtual Real. 20, 101–125. doi: 10.1007/s10055-016-0285-9
Regan, C. (1995). An investigation into nausea and other side-effects of head-coupled immersive virtual reality. Virtual Real. 1, 17–31. doi: 10.1007/BF02009710
Regan, E. C., and Ramsey, A. D. (1996). The efficacy of hyoscine hydrobromide in reducing side-effects induced during immersion in virtual reality. Aviat. Space. Environ. Med. 67, 222–226.
Riccio, G. E., and Stoffregen, T. A. (1991). An ecological theory of motion sickness and postural instability. Ecol. Psychol. 3, 195–240. doi: 10.1207/s15326969eco0303_2
Russell, M. E. B., Hoffman, B., Stromberg, S., and Carlson, C. R. (2014). Use of controlled diaphragmatic breathing for the management of motion sickness in a virtual reality environment. App. Psychophysiol. Biofeedback 39, 269–277. doi: 10.1007/s10484-014-9265-6
Shafer, D. M., Carbonara, C. P., and Korpi, M. F. (2017). Modern virtual reality technology: cybersickness, sense of presence, and gender. Media Psychol. Rev. 11, 1–13.
Skarbez, R., Brooks, F. P., and Whitton, M. C. (2018). “Immersion and coherence in a stressful virtual environment,” in Proceedings of the 24th ACM Symposium on Virtual Reality Software and Technology (Tokyo). doi: 10.1145/3281505.3281530
Stanney, K. M., Kennedy, R. S., and Drexler, J. M. (1997). Cybersickness is not simulator sickness. Proc. Hum. Factors Ergon. Soc. Annu. Meet. 41, 1138–1142. doi: 10.1177/107118139704100292
Stone, R. T., Watts, K. P., and Rosenquist, B. E. (2012). Evaluation of 3D Television: Impact on Depth Perception. Proc. Hum. Factors Ergon. Soc. Annu. Meet. 56, 733–737. doi: 10.1177/1071181312561153
Walter, H. J., Li, R., Munafo, J., Curry, C., Peterson, N., and Stoffregen, T. A. (2019). Unstable coupling of body sway with imposed motion precedes visually induced motion sickness. Hum. Mov. Sci. 64, 389–397. doi: 10.1016/j.humov.2019.03.006
Keywords: virtual environment (VE), mitigation, virtual reality (VR), cybersickness, visually induced motion sickness (VIMS)
Citation: Jasper A, Cone N, Meusel C, Curtis M, Dorneich MC and Gilbert SB (2020) Visually Induced Motion Sickness Susceptibility and Recovery Based on Four Mitigation Techniques. Front. Virtual Real. 1:582108. doi: 10.3389/frvir.2020.582108
Received: 10 July 2020; Accepted: 24 September 2020;
Published: 29 October 2020.
Edited by:
Charles McMaster Oman, Massachusetts Institute of Technology, United StatesReviewed by:
Pierre Bourdin, Open University of Catalonia, SpainAnderson Maciel, Federal University of Rio Grande do Sul, Brazil
Copyright © 2020 Jasper, Cone, Meusel, Curtis, Dorneich and Gilbert. This is an open-access article distributed under the terms of the Creative Commons Attribution License (CC BY). The use, distribution or reproduction in other forums is permitted, provided the original author(s) and the copyright owner(s) are credited and that the original publication in this journal is cited, in accordance with accepted academic practice. No use, distribution or reproduction is permitted which does not comply with these terms.
*Correspondence: Angelica Jasper, YW1qYXNwZXJAaWFzdGF0ZS5lZHU=