Restoring Soil Functions and Agroecosystem Services Through Phytotechnologies
- Laval University, Soil and Agri-Food Engineering Department, Quebec, QC, Canada
Phytotechnology has traditionally been considered as a tool to remediate contaminated soils. While phytotechnology has been generally defined as the application of science and engineering to study problems and provide solutions involving plants, the practical applications go far beyond restoring contaminated land. This review aims to broaden the way we think about phytotechnologies while highlighting how these living technologies can restore, conserve and regenerate the multiple functions and ecosystem services provided by the soil, particularly in the context of agroecosystems. At first, the main problems of soil degradation in agroecosystems are shortly underlined. Subsequently, the importance of plants and their living roots as engines of restoration are reviewed. This paper demonstrates the importance of root traits and functions for soil restoration. It also demonstrates that plant and root diversity together with perenniality are key component of an efficient soil restoration process. Then, a phytotechnology toolbox which includes three pillars for agroecosystems restoration is presented. The three pillars are agricultural practices and land management (1), rhizosphere engineering (2) and ecological intensification (3). This paper also highlights the importance of developing targeted phytotechnology-based restoration strategies developed from root functions and knowledge of rhizosphere processes. More work is needed to evaluate the potential benefits of incorporating phytotechnology-based restoration strategies in the context of grain or vegetable crop productions as most of the studies for agroecosystem restoration strategies were intended to mimic natural prairies.
Introduction
The United Nations has declared 2021-2030 as the Decade on Ecosystem Restoration, a call to prevent, halt, and reverse the degradation of ecosystems. Agroecosystems are among those systems needing protection. This effort offers a unique opportunity for soil scientists and phytotechnologists to work together towards restoring sustainable terrestrial ecosystems.
Phytotechnology, a relatively new field, is defined as “the application of science and engineering to study problems and provide solutions involving plants” (1). Mangkoedihardjo (2007) (2) later incorporated the idea of sustainability into that definition: “Phytotechnology is a domain of applied science and engineering that focuses on the use of plants to provide cost-effective, sustainable solutions to environmental issues”. These rather broad definitions evolved from the more well-known concept of phytoremediation, which explains why the literature today still abounds with definitions confining the term phytotechnology to the remediation of contaminated land. A few examples of definitions are presented in Table 1.
Plants used in phytotechnology are numerous, and historically more attention have been focused on plant having abilities for contaminated soil remediation. For example, Populus sp., Salix sp. and Brassicaceae sp. have been used for phytoextraction of heavy metal (8). Salix sp. have also been used for phyto-treatment of landfill leachate (9) and phytoremediation of site with previous petrochemicals activities (10). As such, in a bibliometric analysis of phytotechnologies, Koelmel et al. (2015) (6) observed that while the proportion of articles including the term phytoremediation has increased steadily since 1999, a comparatively small percentage of articles mention the term phytotechnology. The concept of phytotechnology thus appears to be underexploited. This paper revisits the previous definitions from the UN Environmental Programme (UNEP, 2003) and Mangkoedihardjo (2007) “the application of science and engineering to study environmental problems and provide sustainable and cost-effective solutions involving plants and expands it with and their living roots”. This expanded definition opens up new perspectives for this green technology and underscores how plants and, in particular, plant roots and rhizosphere functions are essential contributors to the restoration, conservation and regeneration of the multiple functions of the soil in ecosystems.
This review highlights the main problems encountered in degraded agroecosystems and underlines the importance of root and rhizosphere functions in restoring degraded soils. Then, a phytotechnology toolbox is presented for application in agroecosystems restoration, and how integrating rhizosphere engineering and ecological intensification research to this toolbox may benefit agroecosystem management for a sustainable and resilient future.
The Loss of Soil Functions and Services in Degraded Agroecosystems
As described by Hatfield et al. (2017) (11), soil properties, functions and ecosystem services are interrelated. Soil properties represent the foundation on which soil functions and services are based. Soil degradation affects soil properties and, in turn, the associated functions and services provided by the soil. Modern agricultural practices have often been singled out as a cause of soil degradation (12, 13). Indeed, intensive agricultural practices involving synthetic fertilizers and pesticides, monocropping with little or no return of organic matter, soils left bare for prolonged periods, and over- and inappropriate use of heavy machinery have all disturbed, reduced or inhibited the natural balance of agroecosystems, sometimes to a nearly irreversible degree. Consequently, an estimated 12 million hectares of agricultural land are lost to soil degradation each year (14). Soil degradation leads to the loss of soil functions to varying extents. The main processes involved in the degradation of agroecosystems are physical, chemical and biological (Figure 1). In addition to these three degradation processes, Lal (2015) (12) includes ecological degradation in soil degradation processes, referring to the disruption of nutrient and hydrological cycles, the decline in net biome productivity, the loss of nutrients and carbon, the decline in input use efficiency, and impeded denaturing of pollutants. This reviews will focus on the physical, chemical and biological aspects of soil degradation.
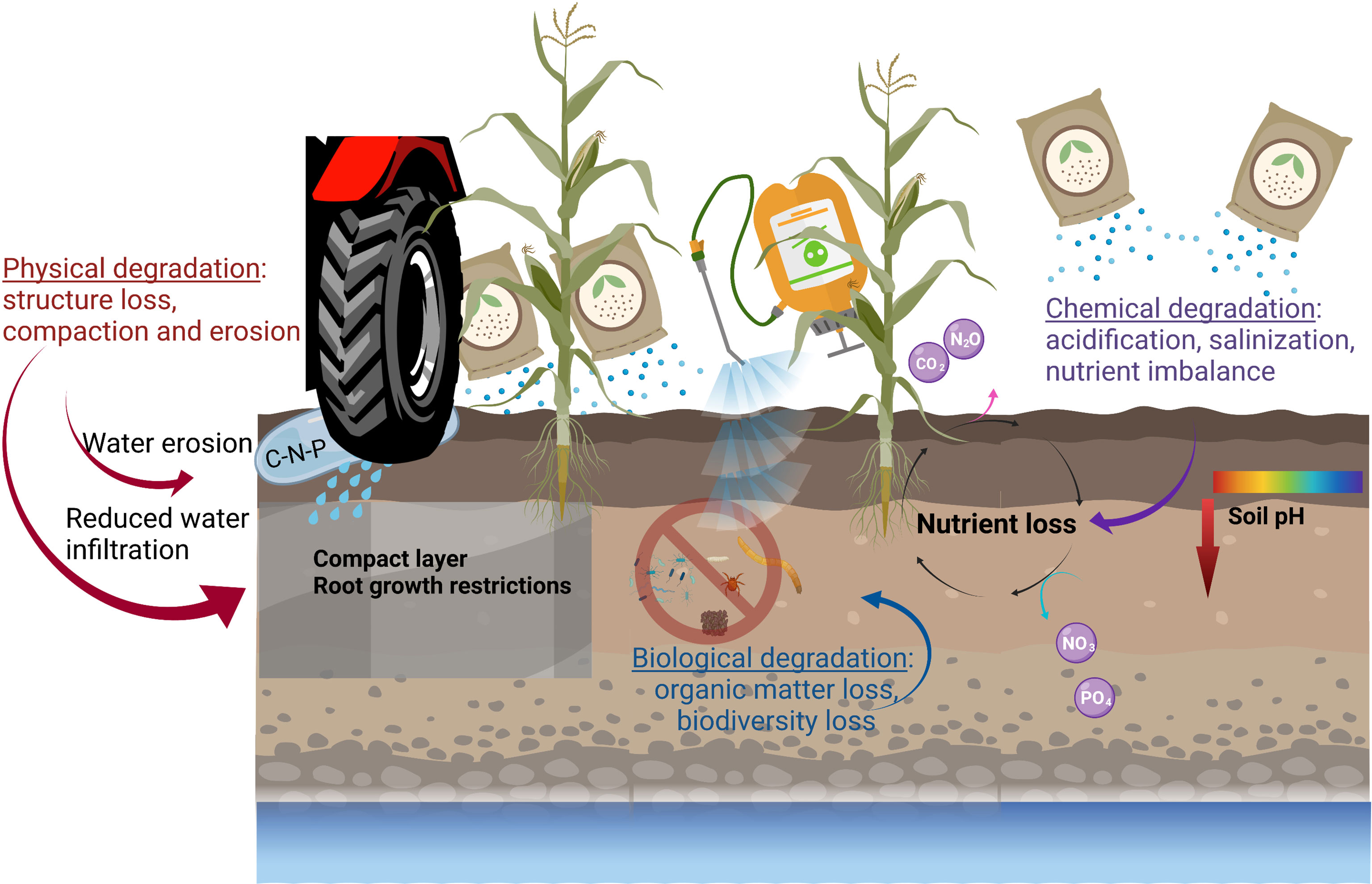
Figure 1 Soil degradation in an agroecosystem. Physical degradation includes structure loss, compaction and erosion. These problems come with crop growth restriction (compact layer, aeration restriction) and overuse of fertilizer to compensate. Biological degradation includes the loss of organic matter and biodiversity often related to tillage intensity and frequency, poor crop rotation and soil coverage and heavy use of pesticides. Chemical degradation includes acidification, salinization and nutrient imbalance often related to heavy use of fertilizer, poor irrigation water quality and intensity of cropping systems.
Physical degradation refers to the loss of the structural quality of the soil through compaction, erosion, and eventually desertification. A worldwide study of soil degradation conducted 30 years ago estimated that 68 million hectares of agricultural land were affected by soil compaction (15). Soil compaction increases soil density and decreases its porosity, mainly macro-porosity, impeding soil functions such as water infiltration, root penetration, and water and gas exchange. Crop productivity can be severely reduced by soil compaction as well (16). Soil erosion causes not only the loss of soil, but of carbon (C), nutrients, and pesticides as well. Globally, agricultural carbon erosion has been estimated at 0.5 ± 0.15 Pg C y–1, with water erosion delivering 0.08 ± 0.02 Pg C to river systems each year (17). This same study evaluated the loss of nitrogen by erosion at 23–42 Tg y-1, and the loss of organic phosphorus at 2.1–3.9 Tg y-1 and inorganic phosphorous at 12.5–22.5 Tg y-1 (17). Pesticide loss through soil erosion depends on the molecular characteristics of the pesticides, among other factors. Wind erosion, for example, has been estimated to remove from 1941 mg ha-1 y− 1 to 3000 mg ha-1 y-1 of the well-known herbicide glyphosate and its degradation product aminomethylphosphonic acid (AMPA), depending on the initial load in the soil (18). Water erosion could lead to higher losses, from 9753 mg ha-1 y-1 to 47 667 mg ha-1 y-1 (18). All these losses caused by soil degradation processes have substantial environmental, economical, and societal consequences (12, 14, 19, 20).
Chemical degradation is characterized by acidification, salinization, nutrient imbalance, reduced cation exchange capacity (CEC), increased metal toxicities, and leaching of NO3-N and other essential plant nutrients. Of the land area affected by salinization worldwide, 17 per cent of the soil and 24 per cent of the irrigated land was found to be affected by human-induced salinization (21). Crop productivity is directly impacted by soil salinity which leads to losses of close to US$ 30 billion in crop production worldwide each year (22). Another example is the contamination of agroecosystems with heavy metals contained in fertilizers, pesticides, biosolids, manure, and waste water, in addition to atmospheric deposits (23). Soil contamination with heavy metals can reduce soil fertility, modify microbial processes such as carbon mineralization and nitrogen transformation, and reduce crop production (23). Soil fertility is a critical component of food and water safety, and the ever-growing pressure to increase food, fiber, and fuel production to meet worldwide demand and achieve zero hunger has put severe pressure on soil resources (11, 24). Losses of carbon and essential nutrients from the agricultural system have resulted in nutrient deficient food for human consumption. These losses will only increase as crop management is intensified to replace the lost fertility, exacerbating the problem (25). Intensified crop management include also an increase in the use of pesticides which have been related to a decrease in nitrifying bacteria, N2-fixing bacteria and nitrogenase activity (26) which may have a significant and negative impact on soil fertility.
Biological degradation refers mainly to processes leading to the loss of organic matter and soil biodiversity. Soil organic matter (SOM) and carbon (SOC) are central to many soil functions and ecosystem services. Such losses therefore severely impact the performance of agroecosystems in terms of crop productivity, carbon sequestration, nutrient and water availability, and biological diversity. Changes in land use and land cover worldwide have resulted in substantial losses of carbon from soils (27). In their epic publication, Sanderman et al. (2017) (27) estimated that agricultural land use has resulted in a cumulative loss of ∼133 Pg C from the earth. Authors Guo and Gifford (2002) (28) observed in a meta-analysis that changes in land use from natural pasture or forest to cropping systems have decreased the soil organic carbon stock by 40% to 60%. These losses are the direct consequence of agricultural activities such as tillage, fertilization, and biomass alteration, which have modified the carbon (C), nitrogen (N), and phosphorus (P) pools and fluxes that originally existed in native ecosystems (29). Likewise, the loss of soil biodiversity has been closely linked to soil organic matter loss and other soil health related problems (30). The widespread use of agrochemicals has also resulted in reduced biodiversity (31). Likewise, soil biological degradation has disrupted the food web, in turn affecting nutrient and carbon cycling and the control of pathogens (32, 33). In addition, degraded agroecosystems have been found to have a negative impact on arbuscular mycorrhizae, earthworms, and microarthropods (34). As examples, accumulation of pesticides in agroecosystems have been related to a reduction in the microbial functional diversity (35), and the use of pesticides, particularly organophosphate, have been shown to negatively impact the total earthworms community biomass and density (36).
Restoration of Soil Functions and Services in Agroecosystems: A Plant and Rhizosphere Perspective
Soil restoration has been the subject of many literature reviews. When related to agroecosystems, these reviews often focus on soil management practices, including reducing tillage, incorporating organic amendments, and returning crop straw (37, 38). Other reviews include a wider range of practices, such as green manure, crop rotation, and agroforestry (39, 40). Very few, however, have examined soil restoration from a plant and rhizosphere perspective. Although the distinction between soil restoration and soil conservation is not always well-defined for agroecosystems, this paper focuses on soil restoration, aimed at recovering and sustaining optimal soil functioning, rather than on soil conservation, which by definition is a combination of management and land use methods (mainly reducing tillage, covering the soil, and diversifying crop rotations) that safeguard the soil against depletion or deterioration by natural or human-induced factors (41). Soil restoration, which implies recovery or an approximate return to a natural state, should ideally be inspired by nature itself. Agroecosystems were once natural prairies, forests, or peatlands characterized by a state of dynamic equilibrium within a community of organisms including among others, plant diversity, root diversity, an associated rhizosphere food web.The restoration practices implemented in degraded agroecosystems must therefore take inspiration from this original state of dynamic equilibrium.
The Power of Roots
Roots are central to maintaining and restoring physical, chemical and biological conditions in the soil. They also drive the functioning of the rhizosphere, the microenvironment found at the interface between roots, soil and soil biota. Roots explore the soil and exploit its resources. Roots of higher plants shape-up the belowground diversity of terrestrial ecosystems and provide much of the carbon to power the soil ecosystem (42). The next sections illustrate how roots are powerful drivers of soil restoration.
Roots and Soil Physical Restoration
Structure and Compaction
Plant roots have a major impact on the physical properties of soil (43, 44). Some morphology traits (e.g., root length, specific root length and root diameter) have also been linked to soil stability (45, 46). Although soil structure can influence root distribution in the soil, plant roots are capable of modifying soil structure to accommodate their growth (47) and can thus play a significant role in restoring physically degraded soil. Indeed, plant roots affect soil physical properties through a variety of mechanisms, including direct penetration and anchorage (48), modifying water availability (49), reorganizing the soil pore structure (50), releasing exudates (51), and altering the soil structure through their association with mycorrhizae (52). In addition, plant species adopt different strategies to adapt for soil compaction, such as changing root length and diameter (53–57). Integrating perennial and deep-rooting plants that tolerate high soil penetration resistance and low concentrations of oxygen in the rotation sequence can help repair soil structure by improving soil penetrability and aeration (58–61). Increasing the variety of plant species has been shown to enhance soil aggregate stability (62). By improving soil physical conditions, this strategy may also contributes to higher productivity in subsequent crops (63, 64).
Erosion
The protection afforded by plant roots against soil erosion is well known (65–69). Gyssels et al. (2005) (65) showed that the decrease in water erosion rates with increasing root mass is exponential and highlighted the importance of roots in controlling this degradation process. Zuazo and Pleguezuelo (2008) (67) also stressed the role of roots in controlling the erosion process, highlighting how they contribute to improving soil properties, such as soil aggregation, structural stability, and cohesion between soil particles. In a review paper, Ola et al. (2015) (68) demonstrated the importance of root system architecture in controlling soil erosion and illustrated the key mechanisms by which plant roots influence soil physical properties: increasing shear strength, physically retaining soil particles, increasing aggregation, supplying additional organic matter, and altering pore size distribution. In a study on purple alfalfa in a Loess soil, Li et al. (2017) (66) found that the physical intertwining of roots was the main factor involved in reducing soil erosion and that root surface area density could be regarded as the key indicator of changes in soil erosion resistance in loam soils. Finally, Hao et al. (2020) (69) showed that vegetation restoration improves many of the soil physical properties (soil noncapillary porosity, aggregate stability, and shear strength) that are key to mitigating erosion, and that the functional traits of roots, in particular, fine roots, play a substantial role in this relationship. The authors propose that a restoration approach using species with desired root traits could be a promising tool to control soil erosion.
Roots and Soil Chemical Restoration
Salinity
In arid and semi-arid regions, precipitation may not be adequate to leach out salts from the rhizosphere, leading to toxic conditions for most cultivated plants. Halophytes are native flora that can tolerate or exploit the high sodium chloride concentrations in the soil water of saline soils (70). Halophytes have been the topic of numerous studies aimed at restoring saline soils (71) and have attracted attention around the world as a potential source of human food, forage, oilseeds, protein, and energy (72). These plants can decrease exchangeable sodium and soluble salt concentrations in soils through absorption by roots and accumulation in the biomass. Root associated processes in the restoration of salinity-affected soils have been related so far to root exudates, production of extracellular enzyme and alteration in root architecture (73). In a review paper, Panta et al. (2014) (72) reported that some species of plants removed between 0.66 and 6.35 t ha-1 a-1 of NaCl. Halophytes have also been used as intercrops with watermelon (74), cotton (75), maize (76), and many other crops, as reviewed by (77). Until now, however, most of the studies on intercropping with halophytes have been done under greenhouse conditions, with both successful and unsuccessful responses (77). Further study under field conditions would thus be required. Nonetheless, intercropping cotton with halophytes has been shown to decrease soil salinity and bulk density while increasing soil porosity, soil organic carbon, root growth, total aboveground biomass, and cotton yield, in comparison with a conventional monoculture system (75, 78). The results are thus promising in terms of cash crop yield and quality, owing mainly to the effect of the halophytes in restoring adequate soil conditions.
Fertility
Numerous studies have shown that changes in land use from natural grassland and forest to intensive annual cropping systems alter soil fertility by lowering carbon, nitrogen and phosphorus stocks (79–81). There is growing evidence, however, that degraded lands can be restored while remaining productive (82). Central to this restoration is the incorporation of perennial crops and forages in long rotations (24, 83). Perennial plants that have a higher root-to-shoot ratio (24), higher root biomass production (84–86), and higher root-associated C input through exudation and turnover (87, 88) can improve soil properties that are closely linked to soil fertility, such as soil aggregation, water-holding capacity, and microbial biomass and diversity. In addition, perennial plants are known to make better use of nitrogen, as their deeper and more extensive root systems optimize inorganic N and P retention in the soil, thus reducing leaching of these elements (81, 89–92). In addition, some species contribute to N retention by translocating N in their belowground biomass, such as in rhizomes at the end of the growing season (24). Further to this perenniality concept in restoring soil fertility is the diversification concept. In a 23-year survey of plant biodiversity and soil fertility, Furey and Tilman (2021) (93) observed that, relative to monocultures of these same species, plots containing 16 perennial grassland plant species had about 30% to 90% more nitrogen, potassium, calcium, magnesium and carbon in the soil, in addition to a greater cation exchange capacity. They also reported that increasing plant functional biodiversity may help restore soil fertility. As biodiversity performs key ecological services, an approach appropriately implemented in time and space can contribute to re-establishing agroecosystems capable of maintaining soil fertility, crop health and productivity (94). In addition, selecting species that develop root hair may help the restoration process as these plant are particularly effective in acquiring immobile or sparingly soluble nutrient in addition to being performant in dry soil conditions (95). Finally, plant root exudates play a key role in shaping the rhizosphere and regulating nutrient cycling which are important for restoring soil fertility. For example, Yuan et al. (2022) (96) observed in an incubation study using low fertility soils that the type of root exudates (organic acids or carbohydrates) affect differently the N mineralization and N fixation rates. Organic acids addition was related to higher plant-available N compared to carbohydrates additions (96). More studies related to the root exudation in relation to soil fertility restoration are needed as both plant and soil conditions will influence the dominant exudates released and therefore its impact on nutrient availability.
Roots and Soil Biological Restoration
Soil Organic Matter
In a highly recognized study, Rasse et al. (2005) (97) asked the simple question: Is soil carbon mostly root carbon? In doing so, they initiated discussions and interest about the importance of root structures and functions in controlling the soil C cycle. Plant roots indeed contribute substantially to the formation of stable SOM and, to a varying extent depending on their traits, to C sequestration in soil (98). Indeed, it has been shown that root exudation adds OC to the soil which will be partly consume by microorganisms and partly stabilized to mineral-associated SOC (99). In addition, the role of root hairs in this process has been highlighted by Zhang et al. (2020) (100). Agricultural practices are responsible for dramatic losses of SOM (27). Two of the primary reasons why native terrestrial ecosystems contain more SOM than agroecosystems are that a greater percentage of native ecosystem productivity is generated below ground in perennial roots and that the soils in these systems remain relatively undisturbed (101, 102). Crews and Rumsey (2017) (101) reviewed approaches to rebuilding SOM in croplands and stated that shifting from annual to perennial crops is central, as “perennials address the root of the problem “. Shifting from annual to perennial grain crops may be the most promising way for agriculture to approach the SOM levels that accumulate in native ecosystems, and Crews and Rumsey (2017) (101) estimated that between 0.13 and 1.70 t ha-1 y-1 of SOC could be accumulated in fields converted to perennial grains. Indeed, studies have shown that higher belowground allocation of carbon resources and reduced soil disturbance promote the accumulation of SOM in perennial-based ecosystems (103, 104). As was advocated for fertility restoration, biodiversity is also fundamental to improving SOM (105–107). Yang et al. (2019) (106) concluded from a long-term field experiment that restoring late-successional grassland plant diversity accelerates annual carbon storage, noting that higher soil carbon storage rates are linked to greater root biomass and aboveground production. In another long-term field experiment exploring grassland diversity, Lange et al. (2015) (107) reported that soil carbon storage is governed by the metabolic activity of soil microbes, which is stimulated by plant diversity that ensures greater root input through root exudation. In their experiment, however, legumes were found to negatively affect soil carbon concentrations. In a chronosequence experiment, Liu et al. (2019) (105) observed that greater plant species diversity increases community productivity and enhances root biomass and rhizosphere carbon inputs in the soil, increasing soil carbon storage and in turn improving other soil properties, such as soil infiltration capacity, by influencing soil aggregate stability and porosity.
Biological Diversity
Plant diversity is an important determinant of soil biodiversity (108). Enhancing crop diversity in space (e.g., intercropping) or time (e.g., crop rotation or cover crops) has been proven to have beneficial effects on ecosystem processes. Greater attention has thus been focused on the impact of plant diversity on soil biodiversity. Venter et al. (2016) (109) observed in a meta-analysis that soils under a higher diversity of crops in rotation produced higher microbial richness (+15.11%, n = 26) and diversity (+3.36%, n = 43) scores. In a long-term experiment, Scherber et al. (2010) (110) studied how plant diversity influences the richness and abundance of above- and belowground organisms. They revealed that, in both cases, herbivores responded more strongly to changes in plant diversity than did carnivores or omnivores. The density and richness of carnivorous taxa were independent of the vegetation structure, but increased plant diversity decreased biological invasion, pathogen infestation and hyperparasitism. Eisenhaeur et al. (2013) (111) observed that greater plant diversity increased soil pH, soil N concentration, soil water content, and plant root biomass, which increased soil microbial biomass and the density of micro- and mesofauna detritivores. Moreover, plant species richness may have fueled the soil decomposer food web by increasing levels of rhizodeposition, although this specific parameter was not directly measured in their study. A meta-analysis conducted by Zhang et al. (2022) (112) showed that fauna (p=0.009) and diversity (p=0.076) increased with plant species richness, with a particular effect on herbivores. Their study also highlighted the time factor in the response of soil fauna to plant diversity and underlined the importance of soil fauna in ecosystem functions such as decomposition and soil formation. In a study using the dilution-to-extinction approach, Yang et al. (2021) (113) observed that resiliency against environmental variability is greater when both soil biodiversity and plant diversity are high and that reduced biodiversity strongly impacts the stability of biomass production. Re-establishing biodiversity at a multitrophic level (plant and soil) is therefore crucial to the restoration of ecosystem functions. The changes in biodiversity often observed under high plant diversity have been linked to higher microbial biomass, fungi, respiration and gross N mineralisation, all of which contribute to improving ecosystem productivity (114). Although plant species richness has mostly been studied in terms of the number of plants, many questions remain as to what extent interactions between the different species drive the positive effects observed (115). To that end, Bakker et al. (2019) (115) studied how root traits (specific root length, root length density, root tissue density, and the deep root fraction) explain temporal changes in biodiversity in grassland mixtures. They observed that increased positive effects of species richness on community biomass in a dry year were mainly the result of increased dominance of deep-rooting species, supporting the hypothesis of the protective effect of biodiversity. The findings on root traits are also echoed in soil food web studies (116–119), where plant diversity and root traits have been shown to have an impact beyond productivity and thus have an important role to play in any soil restoration process.
From Roots to Phytotechnology: Presentation of a phytotechnology toolbox for Agroecosystem Restoration
The sections above demonstrated the importance of root traits and functions for soil restoration. It also demonstrated that plant and root diversity together with perenniality are key component of an efficient soil restoration process. Improving soil function and agroecosystem services can be achieved with a set of tools based on the ingenious use of plants. The following sections present a phytotechnology toolbox for agroecosystem restoration, health and sustainability which is based on three main pillars being agricultural practices and land management, rhizosphere engineering and ecological intensification (Figure 2).
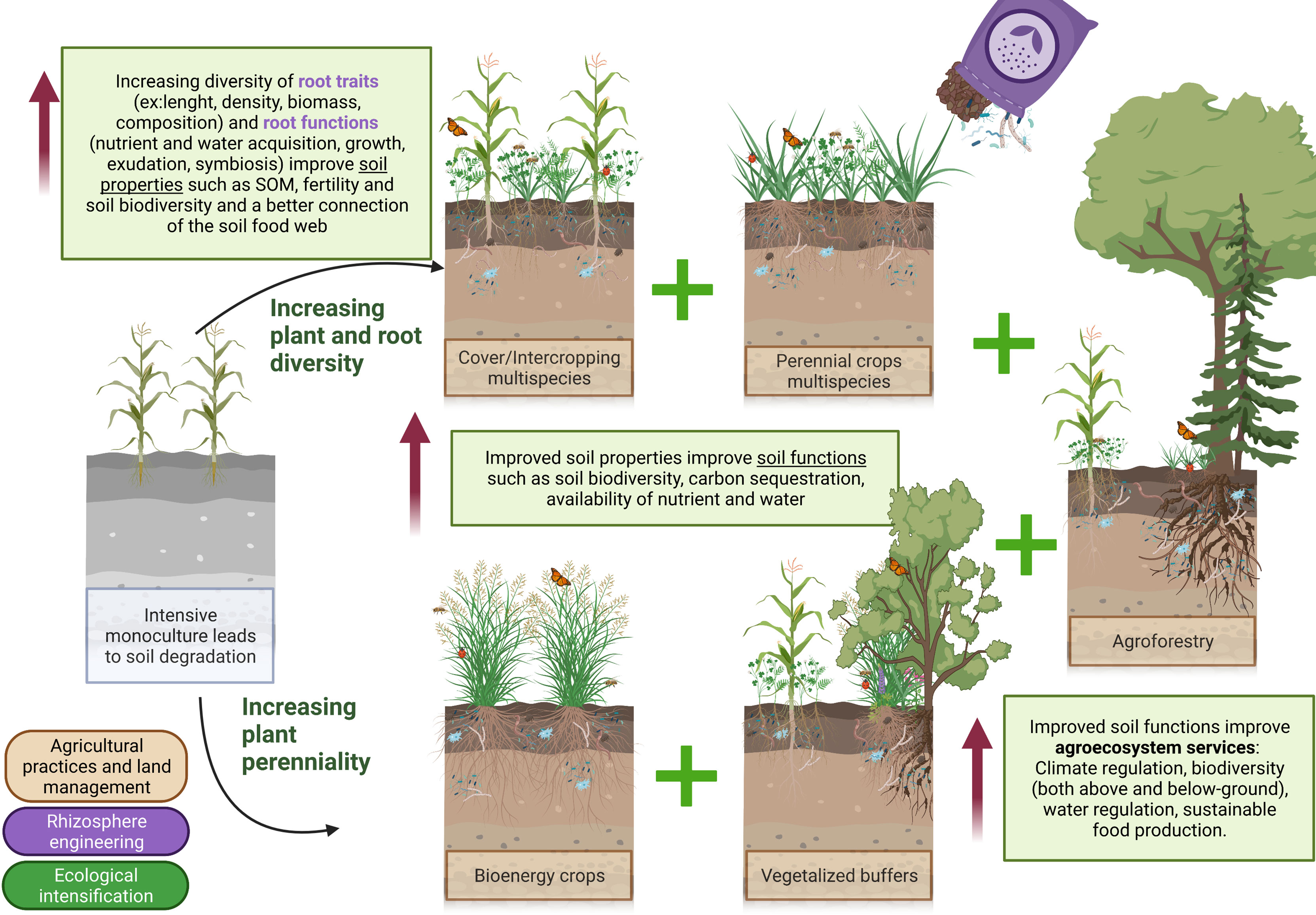
Figure 2 The phytotechnology toolbox: From root and rhizosphere functions to agroecosystem services. Intensive monoculture leads to soil degradation. Increasing plant and root diversity and plant perenniality at the farm and agroecosystem scales improves soil properties, soil functions and therefore related agroecosystem services. The phytotechnology toolbox is based on three pillars. 1) Agricultural practices and land management: cover crops and intercrops in multispecies, perennials crops in the rotations including bioenergy crops, agroforestry and vegetalized buffers. 2) Rhizosphere engineering: organic amendment, inoculation and selection of plant species based on root trait and functions to achieve a specific goal (improving soil fertility?, soil biology?, soil physical conditions?). 3) Ecological intensification: the more the better e.g., to multiply at the farm scale the beneficial agricultural practices. Increasing at the farm and agroecosystems scales the integration of the three pillars included in the phytotechnology toolbox will benefits degraded soil and improves the sustainability of food production.
Cover Crops and Intercropping
Cover crops, intercropping and living mulch are well-known and well-studied agricultural practices (120, 121) (Figure 3). Through the mechanisms of the plant root and rhizosphere functions, these practices have been observed to improve soil structure (122, 123), carbon sequestration (124, 125), N and P fertility (126, 127), and soil biodiversity (128, 129), in addition to reducing erosion (130, 131). For example, specific cover crops have been used to enhance soil biota to increase the yield of the subsequent crop (132). Living mulch has often been studied in the context of vegetable crop production, where it has been shown to provide multiple benefits (133–135). In the phytotechnology toolbox, both cover crops and intercropping/living mulch can improve the diversity and the perenniality of agroecosystems. Multispecies cover crops or intercrops are increasingly being studied in a context of sustainable agricultural practices (136–138). Although some studies found no improvement with multispecies as compared to monoculture cover crops (139), Finney and Kaye (2017) (137), in a 2-year study of 18 cover crop treatments ranging in diversity from 1 to 8 species, reported an increase in N retention and weed suppression and concluded that using multispecies cover crops that maximize functional diversity could increase the multifunctionality of agroecosystems. Saleem et al. (2020) (136) described how cover crop diversity improves root architectural traits and root coverage in addition to soil properties, such as the composition of soil aggregate-size classes, nutrients, as well as SOM and SOC contents across the soil depth. They concluded that competition among plant roots in species-rich communities may improve rhizosphere soil carbon storage, the composition of soil aggregate-size classes, and nutrients. Intercropping with perennial crops has also been shown to benefit soil functions, such as nutrient acquisition, as well as microbial abundance and diversity (140–142).
Agroforestry
The association of woody species with agricultural production has long been recognized in many civilizations as an essential component of sustainable agriculture (143). When well adapted to the crops and under favorable environmental conditions, an agroforestry system, with its supply of humus and litter and the root system of trees, can improve the edaphic conditions for the root system of the crops (144). Typically, there is a noticeable increase in organic matter and nitrogen, a biological rise of nutrients from deep soil horizons, and an increased mycorrhizal presence. Other notable benefits are a decrease in water and wind erosion, improved soil structure, greater porosity, reduced acidity and salinity, and enhanced soil biological activity. An agroforestry farming system generally exhibits greater microbial biomass and earthworm populations, due to inputs of organic matter from trees (145). The tree component of an agroforestry system is known to provide nutritional benefits through various mechanisms: 1) reducing nutrient losses through a trapping system (or safety net) (146, 147); 2) adding “new” nutrients through N2 fixation and increasing deep soil nutrients (148); and 3) inducing morphological and chemical changes at the rhizosphere level through root activity and plasticity (149). A review written by Udawatta et al. (2019) (150) indicated that flora, fauna and soil microbial diversity were significantly higher in agroforestry systems compared to monoculture systems. Among soil organisms, arbuscular mycorrhizae, bacteria and enzyme levels were significantly higher in the agroforestry systems. Agroforestry also creates high-density biodiversity (including the different trophic levels of the rhizosphere) concentrated in proximity to trees due to the favorable soil-plant-water-microclimate conditions there. As the system matures, the evenly distributed leaves, litter, roots, and dead/living biological material and the microclimate improve the soil and microclimate in adjacent crop and pasture areas. Finally, in agroforestry systems, the potential of the root system to contribute to sequestering and transferring carbon to the soil is very high. With some tree root systems extending to a depth of 60 m, the contribution to soil carbon can be substantial. Compared to the roots of agricultural plants, tree roots are coarse and lignified, and so decompose much more slowly. The dynamics of tree root growth, renewal and decomposition are still poorly understood today, however, and most studies on carbon in these systems focus on carbon fluxes in the top 40 cm rather than on sequestration by the entire root system. Nonetheless, a recent meta-analysis on carbon sequestration in temperate agroforestry systems showed that carbon stocks are higher in areas under agroforestry systems compared to conventional systems, that forest hedgerows are more efficient than alley cropping and silvopastoral systems, and that broadleaved trees perform better than conifers in increasing soil carbon stocks. Again here, however, the study only focused on carbon fluxes and did not take into account the whole root system (151).
Vegetative Buffers
Buffer zones are interfaces between natural areas and agroecosystems that include a mixture of herbaceous, floral and ligneous vegetation (152). Although not considered as a soil restoration practice per se, buffer zones are included in the phytotechnology toolbox as they can benefit agroecosystems by enhancing biodiversity, removing contaminants, and acting as carbon sinks. Studies on vegetative buffer zones (also known as riparian zones) have shown that increased plant diversity can improve the nematode food web structure, increase total microbial biomass, and reduce N et P loading in the soil (153, 154). Nitrogen removal by vegetative buffers was also observed by Mayer et al. (2007) (155), who pointed out that buffer width, soil type, subsurface hydrology (e.g., soil saturation, groundwater flow paths), and subsurface biogeochemistry (organic carbon supply, nitrate inputs) are all important considerations for nitrogen management in watersheds. Pesticide removal has also been observed in riparian buffer zones adjacent to no-till crops (156). Finally, carbon sequestration was observed under restored rangeland riparian (157). Other authors compared woody to herbaceous buffers and concluded that woodlot and poplar buffers have much higher potential for increasing C stock (158, 159). Hence, depending on the characteristics of both the vegetative buffer and the site, this phytotechnology strategy can provide a multitude of services to the ecosystem.
Perennial Bioenergy Crop
Bioenergy crop production on degraded or marginal land has been the topic of numerous studies (160–162). Bioenergy crops have been found to be good candidates for soil restoration (163), improving soil health and contributing to carbon sequestration (164) thanks to minimal soil disturbance. Moreover, they generally have low external input requirements (165) and can provide continuous carbon input from root and leaf litter (166). In fact, experimental evidence suggests that SOM accumulation in perennial bioenergy systems may be more important than aboveground biomass production in offsetting fossil fuel use (167). Plant root traits such as high exudation and deep rooting also increase the potential for soil C sequestration (168). While most of the work on bioenergy crops has focused on monoculture, which could have a negative impact on biodiversity (169), although abandoned and degraded soils can become breeding grounds for invasive plant species (170), there is an emerging interest in intercropping with bioenergy crops (171, 172) (Figure 4). Despite the obvious importance of root and microbiome traits in determining the sustainable productivity of bioenergy cropping systems, little attention has been focused on belowground traits (173). Bioenergy crops have many outlets, such as mulch and bedding for farms (174), amendments (175), biochar (176), and bioplastics (177), which are used in the circular economy of sustainable production. Integrating bioenergy crops with cover crops on farms is considered to be a realistic strategy to restore and conserve cultivated organic soils (178) (Figure 5).
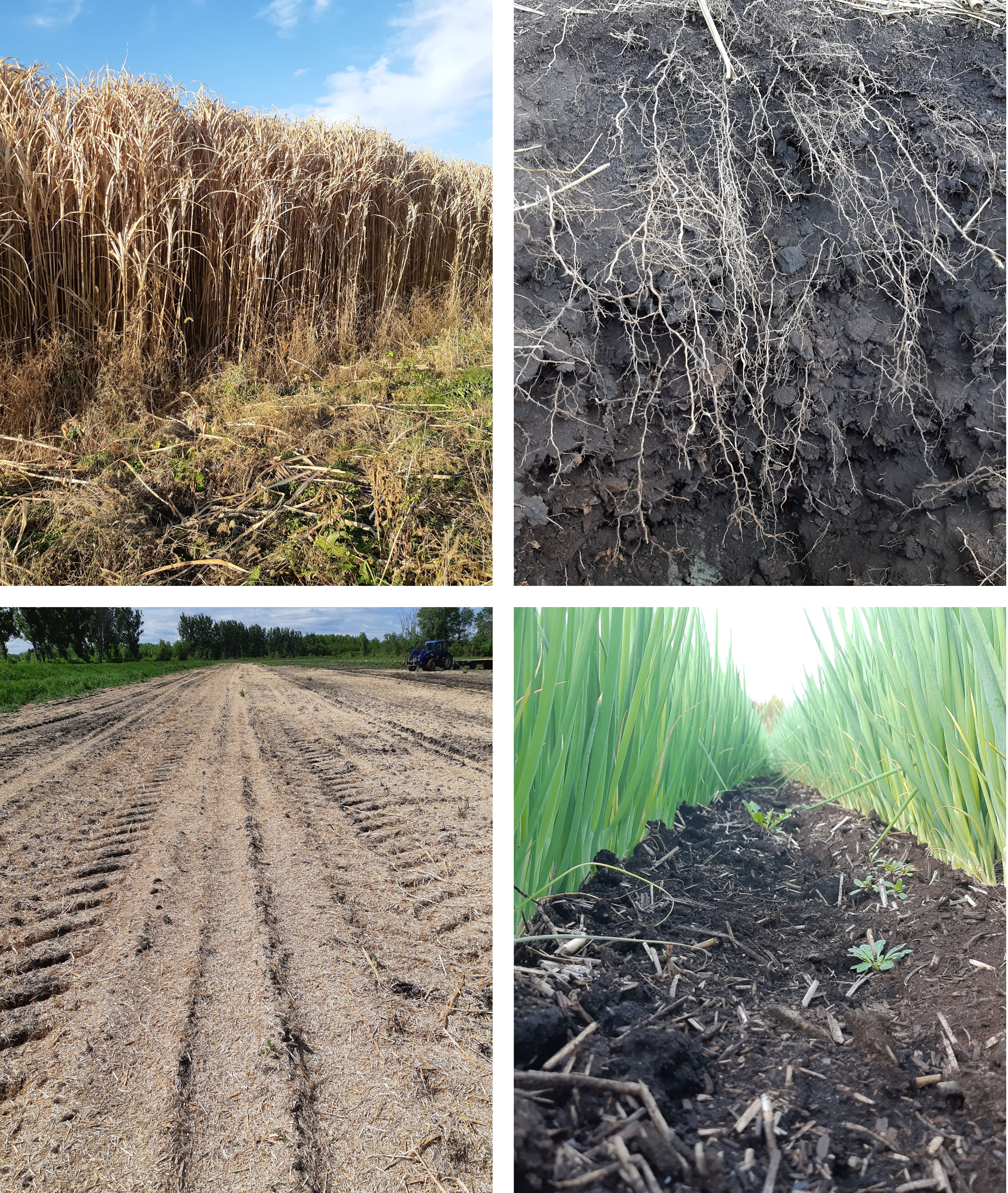
Figure 5 Miscanthus production on degraded organic soils. Upper left: Miscanthus field; upper right: miscanthus root profile; bottom left: field application of chopped miscanthus; bottom right: growth of green onion on miscanthus amended organic soil: Photo credit: Jacynthe Dessureault-Rompré.
Rhizosphere Engineering and Ecological Intensification for Soil Restoration
In addition to agricultural practices and land management, both rhizosphere engineering and ecological intensification offer green and accessible means for restoring degraded agroecosystems in which root and rhizosphere functions play a central role. Both concepts can be integrated into the phytotechnology toolbox (Figure 2).
Rhizosphere engineering, is the manipulation of one or more of the three axes of the rhizosphere, the soil, the biota, and the plant roots, in order to emulate the mutually beneficial interactions that have evolved in nature between soils, plants and soil organisms (179). Much work has been done on rhizosphere engineering, and many studies have focused on soil amendment, inoculation, and plant metabolic engineering, in order to improve crop productivity and crop resilience against abiotic or biotic stresses (179–182). For examples compost and biochar have been shown multiple times to improve soil physical, chemical and biological conditions which have positive impact on crop productivity (183, 184). Inoculation with mycorrhizae, plant-growth promoting bacteria have been related to better biological properties of the rhizosphere under drought stress in horticultural crops (185, 186) and have been related to improved growth condition in marginal lands (187). The engineering of plant has received considerable attention over the past 30 years, especially with the development of genetic engineering techniques which have lead to genetically modified plants resistant to some insects or tolerant to one or more herbicide families or tolerant to high metal concentration (179). Looking back to crop wild relative and especially to the roots of these wild relatives is increasingly recognized has a way to sustain food production in a changing world (188, 189). More simply, rhizosphere can be efficiently engineered by selecting a plant or a group of plants for a specific purpose, for example, including a leguminous crop in a rotation to improve N fertility or selecting grass plant for their high root biomass production to improve carbon sequestration into the soil. Rhizosphere engineering applications for soil restoration purpose are still in their infancy and therefore a world of scientific opportunity awaits unfolding.
Ecological intensification has been recently proposed as an approach to integrate ecological processes into land-management strategies to enhance ecosystem service delivery and reduce anthropogenic input (190). Ecological intensification aims to combine nature and agriculture to design multifunctional agroecosystems that are both “sustained by nature and sustainable in their nature” (34, 191). With the objective of soil restoration, the integration of the principles of ecological intensification makes it possible to multiply the opportunities to increase biodiversity at the scale of a farm or an agroecosystem. These increases in biodiversity will go through an increase in agricultural practices and land management which are presented in Figure 2, on these same scales. Designing a sustainable agroecosystem with phytotechnologies implies taking into account the three pillars discussed above and presented in Figure 2.
Designing Sustainable Agroecosystem Landscapes With Phytotechnologies
Agroecosystem restoration should be considered for purposes beyond food production (192). The phytotechnology toolbox discussed in this paper includes a variety of management options for restoring soil functions and services provided by agroecosystems. Management strategies can be implemented at the field level, but the potential benefits would be enhanced by integrating the different phytotechnology tools on a farm and landscape scale. As stated by Asbjornsen et al. (2014) (82), over the past century, agricultural landscapes worldwide have increasingly been managed for the primary purpose of producing food. However, agroecosystems should be designed to incorporate additional services. Increasing biodiversity in time and space and incorporating areas of perennial vegetation in strategic locations (as discussed above: cover crops and intercropping, vegetative buffers, perennial bioenergy crops, and agroforestry) within agricultural landscapes could represent an opportunity to delivery a wider range of goods and services to society, including water purification and hydrologic regulation, pollination, pest and pathogen control, diverse food and fuel products, optimized nutrient cycling, carbon sequestration, and greater resilience to climate change and extreme disturbances (Figure 4). Ferrarini et al. (2017) (193) conducted a thorough review of multiple ecosystem services provision and biomass logistics management in bioenergy buffers, in which they presented a land-use scenario for bioenergy crops on a farm and landscape scale. Their scenario proposed the conversion of marginal land within the landscape to either biomass production or natural area. The phytotechnology toolbox presented in this paper allows to go even further in our way of designing sustainable agroecosystems.
The present paper proposed a phytotechnology toolbox to restore degraded soils and design sustainable agroecosystems, starting with root trait and functions and rhizosphere engineering then integrating agricultural practices and land management together with ecological intensification. By increasing plant diversity and perenniality, and by diversifying root traits and rhizosphere functions, soils can be restored to support sustainable agroecosystems (Figure 2).
Conclusion
Plants, their root systems and associated rhizosphere functions are the foundation of a phytotechnology-based restoration system. Covering physical, chemical and biological soil degradation, this review showed that plant diversification, in time and space, and perenniality together with rhizosphere engineering and ecological intensification strategies should play a fundamental role in any restoration plan. Implementing strategies aimed at diversification and perenniality at the farm and agroecosystem level could lead to the recovery of optimal soil function and agroecosystem services. Many studies on phytotechnology-based agroecosystem restoration strategies were intended to mimic natural prairies and although the long-term studies presented in this review have provided solid and thought-provoking information, more work is needed to evaluate the potential benefits of incorporating phytotechnology-based restoration strategies in the context of grain or vegetable crop productions. In addition, despite growing interest worldwide for ecosystem restoration, there is an unfortunate lack of interest in restoring degraded agroecosystems for sustainable development. Moreover, it is imperative to go beyond generic plant use and focus further research on developing targeted phytotechnology-based restoration strategies based on root functions and knowledge of rhizosphere processes, with the ultimate goal of restoring soil function and agroecosystem services to ensure sustainable and resilient agriculture.
Author contributions
The author confirms being the sole contributor of this work and has approved it for publication.
Conflict of Interest
The author declare that the research was conducted in the absence of any commercial or financial relationships that could be construed as a potential conflict of interest.
Publisher’s Note
All claims expressed in this article are solely those of the authors and do not necessarily represent those of their affiliated organizations, or those of the publisher, the editors and the reviewers. Any product that may be evaluated in this article, or claim that may be made by its manufacturer, is not guaranteed or endorsed by the publisher.
References
1. UNEP. Freshwater Management Series No. 7 Phytotechnologies: A Technical Approach in Environmental Management. (France: United Nations Environment Programme) (2003).
2. Mangkoedihardjo S. Phytotechnology Integrity in Environmental Sanitation for Sustainable Development. J Appl Sci Res Washington (2007) 3(10):1037–44.
3. ITRC. Phytotechnology Technical and Regulatory Guidance Document. Interstate Technology and Regulatory Cooperation Costa Rica (2001) 78.
4. Tsao DT. Overview of Phytotechnologies. Phytoremediation (2003), 1–50. doi: 10.1007/3-540-45991-X_1
5. Technology I-I, Council R. Phytotechnology Technical and Regulatory Guidance and Decision Trees, Revised. Phyto-3. Washington: Interstate Technology & Regulatory Council, Phytotechnologies Team, Tech Reg (2009).
6. Koelmel J, Prasad M, Pershell K. Bibliometric Analysis of Phytotechnologies for Remediation: Global Scenario of Research and Applications. Int J Phytoremediation (2015) 17(2):145–53. doi: 10.1080/15226514.2013.862207
7. Pidlisnyuk V, Hettiarachchi GM, Zgorelec Z, Prelac M, Bilandžija N, Davis LC, et al. Phytotechnologies for Site Remediation. Phytotechnology With Biomass Production. Boca Raton: CRC Press (2021) 5–36.
8. Tangahu BV, Sheikh Abdullah SR, Basri H, Idris M, Anuar N, Mukhlisin M. A Review on Heavy Metals (As, Pb, and Hg) Uptake by Plants Through Phytoremediation. Int J Chem Eng (2011) 2011:31. doi: 10.1155/2011/939161
9. Nissim WG, Palm E, Pandolfi C, Mancuso S, Azzarello E. Willow and Poplar for the Phyto-Treatment of Landfill Leachate in Mediterranean Climate. J Environ Management (2021) 277:111454. doi: 10.1016/j.jenvman.2020.111454
10. Fortin Faubert M, Desjardins D, Hijri M, Labrecque M. Willows Used for Phytoremediation Increased Organic Contaminant Concentrations in Soil Surface. Appl Sci (2021) 11(7):2979. doi: 10.3390/app11072979
11. Hatfield JL, Sauer TJ, Cruse RM. Soil: The Forgotten Piece of the Water, Food, Energy Nexus. Adv Agronomy (2017) 143:1–46. doi: 10.1016/bs.agron.2017.02.001
12. Karlen DL, Rice CW. Soil Degradation: Will Humankind Ever Learn? Sustainability (2015) 7(5):12490–501. doi: 10.3390/su70912490
13. Karlen DL, Rice CW. Soil Degradation: Will Humankind Ever Learn? Multidiscip Digital Publishing Inst (2015), 12490–501. doi: 10.3390/su70912490
14. Rickson R, Deeks L, Graves A, Harris J, Kibblewhite M, Sakrabani R. Input Constraints to Food Production: The Impact of Soil Degradation. Food Security (2015) 7(2):351–64. doi: 10.1007/s12571-015-0437-x
15. Oldeman LR. Global Extent of Soil Degradation. Bi-Annual Rep 1991-1992/ISRIC: ISRIC; (1992), 19–36.
16. Shaheb MR, Venkatesh R, Shearer SA. A Review on the Effect of Soil Compaction and Its Management for Sustainable Crop Production. J Biosyst Eng (2021) 46:1–23. doi: 10.1007/s42853-021-00117-7
17. Quinton JN, Govers G, Van Oost K, Bardgett RD. The Impact of Agricultural Soil Erosion on Biogeochemical Cycling. Nat Geosci (2010) 3(5):311–4. doi: 10.1038/ngeo838
18. Silva V, Montanarella L, Jones A, Fernández-Ugalde O, Mol HG, Ritsema CJ, et al. Distribution of Glyphosate and Aminomethylphosphonic Acid (AMPA) in Agricultural Topsoils of the European Union. Sci Total Environ (2018) 621:1352–9. doi: 10.1016/j.scitotenv.2017.10.093
19. Gomiero T. Soil Degradation, Land Scarcity and Food Security: Reviewing a Complex Challenge. Sustainability (2016) 8(3):281. doi: 10.3390/su8030281
21. Shahid SA, Zaman M, Heng L. Soil Salinity: Historical Perspectives and a World Overview of the Problem. Guideline Salinity Sssessment Mitigation Adapt Using Nucl Related Techniques: Springer (2018) p:43–53. doi: 10.1007/978-3-319-96190-3_2
22. Qadir M, Quillérou E, Nangia V, Murtaza G, Singh M, Thomas RJ, et al. Economics of Salt-Induced Land Degradation and Restoration. In: Natural Resources Forum. Wiley Online Library (2014).
23. Srivastava V, Sarkar A, Singh S, Singh P, De Araujo AS, Singh RP. Agroecological Responses of Heavy Metal Pollution With Special Emphasis on Soil Health and Plant Performances. Front Environ Sci (2017) 5:64. doi: 10.3389/fenvs.2017.00064
24. Mosier S, Córdova S, Robertson GP. Restoring Soil Fertility on Degraded Lands to Meet Food, Fuel, and Climate Security Needs via Perennialization. Front Sustain Food Syst (2021) 5. doi: 10.3389/fsufs.2021.706142
25. FAO. Climate-Smart Agriculture and the Sustainable Development Goals: Mapping Interlinkages, Synergies and Trade-Offs and Guidelines for Integrated Implementation. Rome, Italy: FAO (2019).
26. Prashar P, Shah S. Impact of Fertilizers and Pesticides on Soil Microflora in Agriculture. In: Sustain Agric Rev Lichtfouse E (eds) Cham: Springer (2016) 19:331–61. doi: 10.1007/978-3-319-26777-7_8
27. Sanderman J, Hengl T, Fiske GJ. Soil Carbon Debt of 12,000 Years of Human Land Use. Proc Natl Acad Sci (2017) 114(36):9575–80. doi: 10.1073/pnas.1706103114
28. Guo LB, Gifford RM. Soil Carbon Stocks and Land Use Change: A Meta Analysis. Global Change Biol (2002) 8(4):345–60. doi: 10.1046/j.1354-1013.2002.00486.x
29. McLauchlan K. The Nature and Longevity of Agricultural Impacts on Soil Carbon and Nutrients: A Review. Ecosystems (2006) 9(8):1364–82. doi: 10.1007/s10021-005-0135-1
30. Tibbett M, Fraser TD, Duddigan S. Identifying Potential Threats to Soil Biodiversity. PeerJ (2020) 8:e9271. doi: 10.7717/peerj.9271
31. Cumming GS, Buerkert A, Hoffmann EM, Schlecht E, von Cramon-Taubadel S, Tscharntke T. Implications of Agricultural Transitions and Urbanization for Ecosystem Services. Nature (2014) 515(7525):50–7. doi: 10.1038/nature13945
32. Zhang X, Ferris H, Mitchell J, Liang W. Ecosystem Services of the Soil Food Web After Long-Term Application of Agricultural Management Practices. Soil Biol Biochem (2017) 111:36–43. doi: 10.1016/j.soilbio.2017.03.017
33. Lehman RM, Cambardella CA, Stott DE, Acosta-Martinez V, Manter DK, Buyer JS, et al. Understanding and Enhancing Soil Biological Health: The Solution for Reversing Soil Degradation. Sustainability (2015) 7(1):988–1027. doi: 10.3390/su7010988
34. Bender SF, Wagg C, van der Heijden MG. An Underground Revolution: Biodiversity and Soil Ecological Engineering for Agricultural Sustainability. Trends Ecol Evol (2016) 31(6):440–52. doi: 10.1016/j.tree.2016.02.016
35. Lupwayi N, Harker K, Clayton G, O’Donovan J, Blackshaw R. Soil Microbial Response to Herbicides Applied to Glyphosate-Resistant Canola. Agricult Ecosyst Environ (2009) 129(1-3):171–6. doi: 10.1016/j.agee.2008.08.007
36. Uwizeyimana H, Wang M, Chen W, Khan K. The Eco-Toxic Effects of Pesticide and Heavy Metal Mixtures Towards Earthworms in Soil. Environ Toxicol Pharmacol (2017) 55:20–9. doi: 10.1016/j.etap.2017.08.001
37. Xu X, Pei J, Xu Y, Wang J. Soil Organic Carbon Depletion in Global Mollisols Regions and Restoration by Management Practices: A Review. J Soils Sediments (2020) 20(3):1173–81. doi: 10.1007/s11368-019-02557-3
38. Garcia C, Hernandez T, Coll MD, Ondoño S. Organic Amendments for Soil Restoration in Arid and Semiarid Areas: A Review. AIMS Environ Sci (2017) 4(5):640–76. doi: 10.3934/environsci.2017.5.640
39. Saturday A. Restoration of Degraded Agricultural Land: A Review. J Environ Health Sci (2018) 4(2):44–51.
40. Lal R. Soils and Food Sufficiency: A Review. In: Lichtfouse E, Navarrete M, Debaeke P, Véronique S, Alberola C. (eds) Sustain Agric Dordrecht: Springer (2009), 25–49. doi: 10.1007/978-90-481-2666-8_4
41. Acton D, Coote D, Eilers W. Soil Conservation. The Canadian Encyclopedia. (2015). Available at: https://www.thecanadianencyclopedia.ca/en/article/soil-conservation
42. Hinsinger P, Bengough AG, Vetterlein D, Young IM. Rhizosphere: Biophysics, Biogeochemistry and Ecological Relevance. Plant Soil (2009) 321(1):117–52. doi: 10.1007/s11104-008-9885-9
43. Gould IJ, Quinton JN, Weigelt A, De Deyn GB, Bardgett RD. Plant Diversity and Root Traits Benefit Physical Properties Key to Soil Function in Grasslands. Ecol Lett (2016) 19(9):1140–9. doi: 10.1111/ele.12652
44. Erktan A, Cecillon L, Graf F, Roumet C, Legout C, Rey F. Increase in Soil Aggregate Stability Along a Mediterranean Successional Gradient in Severely Eroded Gully Bed Ecosystems: Combined Effects of Soil, Root Traits and Plant Community Characteristics. Plant Soil (2016) 398(1-2):121–37. doi: 10.1007/s11104-015-2647-6
45. Wang B, Zhang GH. Quantifying the Binding and Bonding Effects of Plant Roots on Soil Detachment by Overland Flow in 10 Typical Grasslands on the Loess Plateau. Soil Sci Soc America J (2017) 81(6):1567–76. doi: 10.2136/sssaj2017.07.0249
46. Ali HE, Reineking B, Munkemuller T. Effects of Plant Functional Traits on Soil Stability: Intraspecific Variability Matters. Plant Soil (2017) 411(1-2):359–75. doi: 10.1007/s11104-016-3036-5
47. Jin K, White PJ, Whalley WR, Shen J, Shi L. Shaping an Optimal Soil by Root–Soil Interaction. Trends Plant Sci (2017) 22(10):823–9. doi: 10.1016/j.tplants.2017.07.008
48. Bengough AG, Loades K, McKenzie BM. Root Hairs Aid Soil Penetration by Anchoring the Root Surface to Pore Walls. J Exp Botany (2016) 67(4):1071–8. doi: 10.1093/jxb/erv560
49. Carminati A, Moradi AB, Vetterlein D, Vontobel P, Lehmann E, Weller U, et al. Dynamics of Soil Water Content in the Rhizosphere. Plant Soil (2010) 332(1/2):163–76. doi: 10.1007/s11104-010-0283-8
50. Burr-Hersey JE, Ritz K, Bengough GA, Mooney SJ. Reorganisation of Rhizosphere Soil Pore Structure by Wild Plant Species in Compacted Soils. J Exp Botany (2020) 71(19):6107–15. doi: 10.1093/jxb/eraa323
51. Oleghe E, Naveed M, Baggs EM, Hallett PD. Plant Exudates Improve the Mechanical Conditions for Root Penetration Through Compacted Soils. Plant Soil (2017) 421(1/2):19–30. doi: 10.1007/s11104-017-3424-5
52. Rillig MC, Mummey DL. Mycorrhizas and Soil Structure. New Phytol (2006) 171(1):41–. doi: 10.1111/j.1469-8137.2006.01750.x
53. Azam G, Murray RS, Grant CD, Nuberg IK. Tolerance of Young Seedlings of Different Tree Species and a Cereal to Poor Soil Aeration. Soil Res (2014) 52(8):751–9. doi: 10.1071/SR13219
54. Fukao T, Bailey-Serres J. Plant Responses to Hypoxia–Is Survival a Balancing Act? Trends Plant Sci (2004) 9(9):449–56. doi: 10.1016/j.tplants.2004.07.005
55. Grzesiak MT, Ostrowska A, Hura K, Rut G, Janowiak F, Rzepka A, et al. Interspecific Differences in Root Architecture Among Maize and Triticale Genotypes Grown Under Drought, Waterlogging and Soil Compaction. Acta Physiol Plantarum (2014) 36(12):3249–61. doi: 10.1007/s11738-014-1691-9
56. Correa J, Postma JA, Watt M, Wojciechowski T. Soil Compaction and the Architectural Plasticity of Root Systems. J Exp Botany (2019) 70(21):6019–34. doi: 10.1093/jxb/erz383
57. Correa J, Postma JA, Wojciechowski T. Phenotypic Response to Soil Compaction Varies Among Genotypes and Correlates With Plant Size in Sorghum. Plant Soil (2022) 742(1):59–76. doi: 10.1007/s11104-021-05160-z
58. Chen G, Weil RR, Hill RL. Effects of Compaction and Cover Crops on Soil Least Limiting Water Range and Air Permeability. Soil Tillage Res (2014) 136:61–9. doi: 10.1016/j.still.2013.09.004
59. Chen G. Alleviation of Soil Compaction by Brassica Cover Crops. College Park: University of Maryland, College Park (2009).
60. Wick A, Berti M, Lawley Y, Liebig M. Integration of Annual and Perennial Cover Crops for Improving Soil Health. In: Al-Kaisi MM, Lowery B. Editors. Soil Health Intensification Agroecosytems Academic Press (2017), 127–50. doi: 10.1016/B978-0-12-805317-1.00006-3
61. Williams SM, Weil RR. Crop Cover Root Channels may Alleviate Soil Compaction Effects on Soybean Crop. Soil Sci Soc America J (2004) 68(4):1403–9. doi: 10.2136/sssaj2004.1403
62. Peres G, Cluzeau D, Menasseri S, Soussana J, Bessler H, Engels C, et al. Mechanisms Linking Plant Community Properties to Soil Aggregate Stability in an Experimental Grassland Plant Diversity Gradient. Plant Soil (2013) 373(1-2):285–99. doi: 10.1007/s11104-013-1791-0
63. Colombi T, Keller T. Developing Strategies to Recover Crop Productivity After Soil Compaction—A Plant Eco-Physiological Perspective. Soil Tillage Res (2019) 191:156–61. doi: 10.1016/j.still.2019.04.008
64. Rosolem C, Pivetta L. Mechanical and Biological Approaches to Alleviate Soil Compaction in Tropical Soils: Assessed by Root Growth and Activity (Rb Uptake) of Soybean and Maize Grown in Rotation With Cover Crops. Soil Use Management (2017) 33(1):141–52. doi: 10.1111/sum.12313
65. Gyssels G, Poesen J, Bochet E, Li Y. Impact of Plant Roots on the Resistance of Soils to Erosion by Water: A Review. Prog Phys Geograp (2005) 29(2):189–217. doi: 10.1191/0309133305pp443ra
66. Li Q, Liu G, Zhang Z, Tuo D, Bai R, Qiao F. Relative Contribution of Root Physical Enlacing and Biochemistrical Exudates to Soil Erosion Resistance in the Loess Soil. Catena (2017) 153:61–5. doi: 10.1016/j.catena.2017.01.037
67. Zuazo VHD, Pleguezuelo CRR. Soil-Erosion and Runoff Prevention by Plant Covers. A Rev Agron Sustain Dev (2008) 28(1):65–86. doi: 10.1051/agro:2007062
68. Ola A, Dodd IC, Quinton JN. Can We Manipulate Root System Architecture to Control Soil Erosion? Soil (2015) 1(2):603–12. doi: 10.5194/soil-1-603-2015
69. Hao HX, Di HY, Jiao X, Wang JG, Guo ZL, Shi ZH. Fine Roots Benefit Soil Physical Properties Key to Mitigate Soil Detachment Capacity Following the Restoration of Eroded Land. Plant Soil (2020) 446(1-2):487–501. doi: 10.1007/s11104-019-04353-x
70. Zhao KF, Song J, Feng G, Zhao M, Liu JP. Species, Types, Distribution, and Economic Potential of Halophytes in China. Plant Soil (2011) 342(1-2):495–509. doi: 10.1007/s11104-010-0470-7
71. Nouri H, Borujeni SC, Nirola R, Hassanli A, Beecham S, Alaghmand S, et al. Application of Green Remediation on Soil Salinity Treatment: A Review on Halophytoremediation. Process Saf Environ Protect (2017) 107:94–107. doi: 10.1016/j.psep.2017.01.021
72. Panta S, Flowers T, Lane P, Doyle R, Haros G, Shabala S. Halophyte Agriculture: Success Stories. Environ Exp Botany (2014) 107:71–83. doi: 10.1016/j.envexpbot.2014.05.006
73. Wang S, Zhao Z, Ge S, Peng B, Zhang K, Hu M, et al. Root Morphology and Rhizosphere Characteristics Are Related to Salt Tolerance of Suaeda Salsa and Beta Vulgaris L. Front Plant Sci (2021) 12:1193. doi: 10.3389/fpls.2021.677767
74. Simpson CR, Franco JG, King SR, Volder A. Intercropping Halophytes to Mitigate Salinity Stress in Watermelon. Sustainability (2018) 10(3):681. doi: 10.3390/su10030681
75. Liang J, Shi W. Cotton/halophytes Intercropping Decreases Salt Accumulation and Improves Soil Physicochemical Properties and Crop Productivity in Saline-Alkali Soils Under Mulched Drip Irrigation: A Three-Year Field Experiment. Field Crops Res (2021) 262:108027. doi: 10.1016/j.fcr.2020.108027
76. Wang S, Zhao Z, Ge S, Zhang K, Tian C, Mai W. The Effects of Suaeda Salsa/Zea Mays L. Intercropping on Plant Growth and Soil Chemical Characteristics in Saline Soil. Agriculture (2022) 12(1):107. doi: 10.3390/agriculture12010107
77. Hamed KB, Castagna A, Ranieri A, García-Caparrós P, Santin M, Hernandez JA, et al. Halophyte Based Mediterranean Agriculture in the Contexts of Food Insecurity and Global Climate Change. Environ Exp Botany (2021) 191:104601. doi: 10.1016/j.envexpbot.2021.104601
78. Guo J, Shi W, Li J. Effects of Intercropping Different Halophytes in Bare Strips on Soil Water Content, Salt Accumulation, and Cotton (Gossypium Hirsutum) Yields in Mulched Drip Irrigation. Appl Ecol Environ Res (2020) 18(4):5923–37. doi: 10.15666/aeer/1804_59235937
79. Li XG, Li YK, Li FM, Ma Q, Zhang PL, Yin P. Changes in Soil Organic Carbon, Nutrients and Aggregation After Conversion of Native Desert Soil Into Irrigated Arable Land. Soil Tillage Res (2009) 104(2):263–9. doi: 10.1016/j.still.2009.03.002
80. Wei X, Shao M, Fu X, Horton R, Li Y, Zhang X. Distribution of Soil Organic C, N and P in Three Adjacent Land Use Patterns in the Northern Loess Plateau, China. Biogeochemistry (2009) 96(1):149–62. doi: 10.1007/s10533-009-9350-8
81. Breuer L, Huisman J, Keller T, Frede H. Impact of a Conversion From Cropland to Grassland on C and N Storage and Related Soil Properties: Analysis of a 60-Year Chronosequence. Geoderma (2006) 133(1-2):6–18. doi: 10.1016/j.geoderma.2006.03.033
82. Asbjornsen H, Hernandez-Santana V, Liebman M, Bayala J, Chen J, Helmers M, et al. Targeting Perennial Vegetation in Agricultural Landscapes for Enhancing Ecosystem Services. Renewable Agric Food Syst (2014) 29(2):101–25. doi: 10.1017/S1742170512000385
83. Zhang Q, Shao Ma. Soil Fertility Increases Rapidly During the 6–10 Yr Following Conversion of Cropland to Grassland in China’s Loess Plateau Region. Can J Soil Sci (2018) 98(3):531–41. doi: 10.1139/cjss-2017-0107
84. Ferchaud F, Vitte G, Mary B. Changes in Soil Carbon Stocks Under Perennial and Annual Bioenergy Crops. Gcb Bioenerg (2016) 8(2):290–306. doi: 10.1111/gcbb.12249
85. DuPont S, Beniston J, Glover J, Hodson A, Culman S, Lal R, et al. Root Traits and Soil Properties in Harvested Perennial Grassland, Annual Wheat, and Never-Tilled Annual Wheat. Plant Soil (2014) 381(1):405–20. doi: 10.1007/s11104-014-2145-2
86. DuPont ST, Culman SW, Ferris H, Buckley DH, Glover JD. No-Tillage Conversion of Harvested Perennial Grassland to Annual Cropland Reduces Root Biomass, Decreases Active Carbon Stocks, and Impacts Soil Biota. Agricult Ecosyst Environ (2010) 137(1-2):25–32. doi: 10.1016/j.agee.2009.12.021
87. Anderson-Teixeira KJ, Masters MD, Black CK, Zeri M, Hussain MZ, Bernacchi CJ, et al. Altered Belowground Carbon Cycling Following Land-Use Change to Perennial Bioenergy Crops. Ecosystems (2013) 16(3):508–20. doi: 10.1007/s10021-012-9628-x
88. Beniston JW, DuPont ST, Glover JD, Lal R, Dungait JA. Soil Organic Carbon Dynamics 75 Years After Land-Use Change in Perennial Grassland and Annual Wheat Agricultural Systems. Biogeochemistry (2014) 120(1):37–49. doi: 10.1007/s10533-014-9980-3
89. Fu X, Shao M, Wei X, Horton R. Soil Organic Carbon and Total Nitrogen as Affected by Vegetation Types in Northern Loess Plateau of China. Geoderma (2010) 155(1-2):31–5. doi: 10.1016/j.geoderma.2009.11.020
90. Jungers JM, DeHaan LH, Mulla DJ, Sheaffer CC, Wyse DL. Reduced Nitrate Leaching in a Perennial Grain Crop Compared to Maize in the Upper Midwest, USA. Agricult Ecosyst Environ (2019) 272:63–73. doi: 10.1016/j.agee.2018.11.007
91. Liu K, Elliott JA, Lobb DA, Flaten DN, Yarotski J. Nutrient and Sediment Losses in Snowmelt Runoff From Perennial Forage and Annual Cropland in the Canadian Prairies. J Environ Quality (2014) 43(5):1644–55. doi: 10.2134/jeq2014.01.0040
92. Hussain MZ, Hamilton SK, Robertson GP, Basso B. Phosphorus Availability and Leaching Losses in Annual and Perennial Cropping Systems in an Upper US Midwest Landscape. Sci Rep (2021) 11(1):1–12. doi: 10.1038/s41598-021-99877-7
93. Furey GN, Tilman D. Plant Biodiversity and the Regeneration of Soil Fertility. Proc Natl Acad Sci (2021) 118(49). doi: 10.1073/pnas.2111321118
94. Gangatharan R, Neri D. Can Biodiversity Improve Soil Fertility Resilience in Agroecosystems. N Medit (2012) 11(4):11–8.
95. Kohli PS, Maurya K, Thakur JK, Bhosale R, Giri J. Significance of Root Hairs in Developing Stress-Resilient Plants for Sustainable Crop Production. Plant Cell Environ (2022) 45(3):677–94. doi: 10.1111/pce.14237
96. Liu Y, Evans SE, Friesen ML, Tiemann LK. Root Exudates Shift How N Mineralization and N Fixation Contribute to the Plant-Available N Supply in Low Fertility Soils. Soil Biol Biochem (2022) 165:108541. doi: 10.1016/j.soilbio.2021.108541
97. Rasse DP, Rumpel C, Dignac M-F. Is Soil Carbon Mostly Root Carbon? Mechanisms for a Specific Stabilisation. Plant Soil (2005) 269(1):341–56. doi: 10.1007/s11104-004-0907-y
98. Poirier V, Roumet C, Munson AD. The Root of the Matter: Linking Root Traits and Soil Organic Matter Stabilization Processes. Soil Biol Biochem (2018) 120:246–59. doi: 10.1016/j.soilbio.2018.02.016
99. Panchal P, Preece C, Peñuelas J, Giri J. Soil Carbon Sequestration by Root Exudates. Trends Plant Sci (2022). doi: 10.1016/j.tplants.2022.04.009
100. Zhang X, Kuzyakov Y, Zang H, Dippold MA, Shi L, Spielvogel S, et al. Rhizosphere Hotspots: Root Hairs and Warming Control Microbial Efficiency, Carbon Utilization and Energy Production. Soil Biol Biochem (2020) 148:107872. doi: 10.1016/j.soilbio.2020.107872
101. Crews TE, Rumsey BE. What Agriculture can Learn From Native Ecosystems in Building Soil Organic Matter: A Review. Sustainability (2017) 9(4):578. doi: 10.3390/su9040578
102. Crews TE, Blesh J, Culman SW, Hayes RC, Jensen ES, Mack MC, et al. Going Where No Grains Have Gone Before: From Early to Mid-Succession. Agricult Ecosyst Environ (2016) 223:223–38. doi: 10.1016/j.agee.2016.03.012
103. Lorenz K, Lal R. Cropland Soil Carbon Dynamics. In: Lal R, Lorenz K, Hüttl RF, Schneider BU, von Braun J (Eds.) Recarbonization Biosphere Springer (2012), 303–46. doi: 10.1007/978-94-007-4159-1_14
104. Pausch J, Kuzyakov Y. Carbon Input by Roots Into the Soil: Quantification of Rhizodeposition From Root to Ecosystem Scale. Global Change Biol (2018) 24(1):1–12. doi: 10.1111/gcb.13850
105. Liu Y, Miao HT, Chang X, Wu GL. Higher Species Diversity Improves Soil Water Infiltration Capacity by Increasing Soil Organic Matter Content in Semiarid Grasslands. Land Degradation Dev (2019) 30(13):1599–606. doi: 10.1002/ldr.3349
106. Yang Y, Tilman D, Furey G, Lehman C. Soil Carbon Sequestration Accelerated by Restoration of Grassland Biodiversity. Nat Commun (2019) 10(1):1–7. doi: 10.1038/s41467-019-08636-w
107. Lange M, Eisenhauer N, Sierra CA, Bessler H, Engels C, Griffiths RI, et al. Plant Diversity Increases Soil Microbial Activity and Soil Carbon Storage. Nat Commun (2015) 6(1):1–8. doi: 10.1038/ncomms7707
108. Hooper DU, Bignell DE, Brown VK, Brussard L, Dangerfield JM, Wall DH, et al. Interactions Between Aboveground and Belowground Biodiversity in Terrestrial Ecosystems: Patterns, Mechanisms, and Feedbacks: We Assess the Evidence for Correlation Between Aboveground and Belowground Diversity and Conclude That a Variety of Mechanisms Could Lead to Positive, Negative, or No Relationship—Depending on the Strength and Type of Interactions Among Species. Bioscience (2000) 50(12):1049–61. doi: 10.1641/0006-3568(2000)050[1049:IBAABB]2.0.CO;2
109. Venter ZS, Jacobs K, Hawkins H-J. The Impact of Crop Rotation on Soil Microbial Diversity: A Meta-Analysis. Pedobiologia (2016) 59(4):215–23. doi: 10.1016/j.pedobi.2016.04.001
110. Scherber C, Eisenhauer N, Weisser WW, Schmid B, Voigt W, Fischer M, et al. Bottom-Up Effects of Plant Diversity on Multitrophic Interactions in a Biodiversity Experiment. Nature (2010) 468(7323):553–6. doi: 10.1038/nature09492
111. Eisenhauer N, Dobies T, Cesarz S, Hobbie SE, Meyer RJ, Worm K, et al. Plant Diversity Effects on Soil Food Webs are Stronger Than Those of Elevated CO2 and N Deposition in a Long-Term Grassland Experiment. Proc Natl Acad Sci (2013) 110(17):6889–94. doi: 10.1073/pnas.1217382110
112. Zhang Y, Peng S, Chen X, Chen HY. Plant Diversity Increases the Abundance and Diversity of Soil Fauna: A Meta-Analysis. Geoderma (2022) 411:115694. doi: 10.1016/j.geoderma.2022.115694
113. Yang G, Ryo M, Roy J, Hempel S, Rillig MC. Plant and Soil Biodiversity Have non-Substitutable Stabilising Effects on Biomass Production. Ecol Lett (2021) 24(8):1582–93. doi: 10.1111/ele.13769
114. Zak DR, Holmes WE, White DC, Peacock AD, Tilman D. Plant Diversity, Soil Microbial Communities, and Ecosystem Function: Are There Any Links? Ecology (2003) 84(8):2042–50. doi: 10.1890/02-0433
115. Bakker LM, Mommer L, van Ruijven J. Using Root Traits to Understand Temporal Changes in Biodiversity Effects in Grassland Mixtures. Oikos (2019) 128(2):208–20. doi: 10.1111/oik.05612
116. Thakur MP, Eisenhauer N. Plant Community Composition Determines the Strength of Top-Down Control in a Soil Food Web Motif. Sci Rep (2015) 5(1):1–6. doi: 10.1038/srep09134
117. Zhang C, Wang J, Ren Z, Hu Z, Tian S, Fan W, et al. Root Traits Mediate Functional Guilds of Soil Nematodes in an Ex-Arable Field. Soil Biol Biochem (2020) 151:108038. doi: 10.1016/j.soilbio.2020.108038
118. Otfinowski R, Coffey V. Can Root Traits Predict Communities of Soil Nematodes in Restored Northern Prairies? Plant Soil (2020) 453(1):459–71. doi: 10.1007/s11104-020-04624-y
119. Wardle DA, Yeates GW, Williamson W, Bonner KI. The Response of a Three Trophic Level Soil Food Web to the Identity and Diversity of Plant Species and Functional Groups. Oikos (2003) 102(1):45–56. doi: 10.1034/j.1600-0706.2003.12481.x
120. Bybee-Finley K, Ryan MR. Advancing Intercropping Research and Practices in Industrialized Agricultural Landscapes. Agriculture (2018) 8(6):80. doi: 10.3390/agriculture8060080
121. Ogilvie CM, Ashiq W, Vasava HB, Biswas A. Quantifying Root-Soil Interactions in Cover Crop Systems: A Review. Agriculture (2021) 11(3):218. doi: 10.3390/agriculture11030218
122. Bacq-Labreuil A, Crawford J, Mooney SJ, Neal AL, Ritz K. Cover Crop Species Have Contrasting Influence Upon Soil Structural Genesis and Microbial Community Phenotype. Sci Rep (2019) 9(1):1–9. doi: 10.1038/s41598-019-43937-6
123. N’Dayegamiye A, Whalen JK, Tremblay G, Nyiraneza J, Grenier M, Drapeau A, et al. The Benefits of Legume Crops on Corn and Wheat Yield, Nitrogen Nutrition, and Soil Properties Improvement. Agron J (2015) 107(5):1653–65. doi: 10.2134/agronj14.0416
124. Poeplau C, Don A. Carbon Sequestration in Agricultural Soils via Cultivation of Cover Crops–A Meta-Analysis. Agricult Ecosyst Environ (2015) 200:33–41. doi: 10.1016/j.agee.2014.10.024
125. Chapagain T, Riseman A. Barley–pea Intercropping: Effects on Land Productivity, Carbon and Nitrogen Transformations. Field Crops Res (2014) 166:18–25. doi: 10.1016/j.fcr.2014.06.014
126. Büchi L, Wendling M, Amossé C, Necpalova M, Charles R. Importance of Cover Crops in Alleviating Negative Effects of Reduced Soil Tillage and Promoting Soil Fertility in a Winter Wheat Cropping System. Agricult Ecosyst Environ (2018) 256:92–104. doi: 10.1016/j.agee.2018.01.005
127. Cong WF, Hoffland E, Li L, Six J, Sun JH, Bao XG, et al. Intercropping Enhances Soil Carbon and Nitrogen. Global Change Biol (2015) 21(4):1715–26. doi: 10.1111/gcb.12738
128. Kim N, Zabaloy MC, Guan K, Villamil MB. Do Cover Crops Benefit Soil Microbiome? A Meta-Analysis of Current Research. Soil Biol Biochem (2020) 142:107701. doi: 10.1016/j.soilbio.2019.107701
129. Wang G, Bei S, Li J, Bao X, Zhang J, Schultz PA, et al. Soil Microbial Legacy Drives Crop Diversity Advantage: Linking Ecological Plant–Soil Feedback With Agricultural Intercropping. J Appl Ecol (2021) 58(3):496–506. doi: 10.1111/1365-2664.13802
130. De Baets S, Poesen J, Meersmans J, Serlet L. Cover Crops and Their Erosion-Reducing Effects During Concentrated Flow Erosion. Catena (2011) 85(3):237–44. doi: 10.1016/j.catena.2011.01.009
131. Nyawade SO, Gachene CK, Karanja NN, Gitari HI, Schulte-Geldermann E, Parker ML. Controlling Soil Erosion in Smallholder Potato Farming Systems Using Legume Intercrops. Geoderma Regional (2019) 17:e00225. doi: 10.1016/j.geodrs.2019.e00225
132. Deguchi S, Shimazaki Y, Uozumi S, Tawaraya K, Kawamoto H, Tanaka O. White Clover Living Mulch Increases the Yield of Silage Corn via Arbuscular Mycorrhizal Fungus Colonization. Plant Soil (2007) 291(1):291–9. doi: 10.1007/s11104-007-9194-8
133. Pieper JR, Brown RN, Amador JA. Effects of Three Conservation Tillage Strategies on Yields and Soil Health in a Mixed Vegetable Production System. HortScience (2015) 50(12):1770–6. doi: 10.21273/HORTSCI.50.12.1770
134. Hill NS, Levi M, Basinger N, Thompson A, Cabrera M, Wallace J, et al. White Clover Living Mulch Enhances Soil Health vs. Annual Cover Crops. Agron J (2021) 113(4):3697–707. doi: 10.1002/agj2.20768
135. Yadav GS, Babu S, Das A, Datta M, Mohapatra K, Singh R, et al. Productivity, Soil Health, and Carbon Management Index of Indian Himalayan Intensified Maize-Based Cropping Systems Under Live Mulch Based Conservation Tillage Practices. Field Crops Res (2021) 264:108080. doi: 10.1016/j.fcr.2021.108080
136. Saleem M, Pervaiz ZH, Contreras J, Lindenberger JH, Hupp BM, Chen D, et al. Cover Crop Diversity Improves Multiple Soil Properties via Altering Root Architectural Traits. Rhizosphere (2020) 16:100248. doi: 10.1016/j.rhisph.2020.100248
137. Finney DM, Kaye JP. Functional Diversity in Cover Crop Polycultures Increases Multifunctionality of an Agricultural System. J Appl Ecol (2017) 54(2):509–17. doi: 10.1111/1365-2664.12765
138. Chapagain T, Lee EA, Raizada MN. The Potential of Multi-Species Mixtures to Diversify Cover Crop Benefits. Sustainability (2020) 12(5):2058. doi: 10.3390/su12052058
139. Florence A, McGuire A. Do Diverse Cover Crop Mixtures Perform Better Than Monocultures? A Systematic Review. Agron J (2020) 112(5):3513–34. doi: 10.1002/agj2.20340
140. Jez JM, Topp CN, Schlautman B, Bartel C, Diaz-Garcia L, Fei S, et al. Perennial Groundcovers: An Emerging Technology for Soil Conservation and the Sustainable Intensification of Agriculture. Emerging Topics Life Sci (2021) 5(2):337–47. doi: 10.1042/ETLS20200318
141. Han E, Czaban W, Dresbøll DB, Thorup-Kristensen K. Exploitation of Neighbouring Subsoil for Nutrient Acquisition Under Annual-Perennial Strip Intercropping Systems. bioRxiv (2022). doi: 10.1101/2022.01.09.475506
142. Duchene O, Vian J-F, Celette F. Intercropping With Legume for Agroecological Cropping Systems: Complementarity and Facilitation Processes and the Importance of Soil Microorganisms. A Rev Agricult Ecosyst Environ (2017) 240:148–61. doi: 10.1016/j.agee.2017.02.019
143. Young A, Agroforestry ICfRi. Agroforestry for Soil Conservation. Wallingford, UK: CAB International (1989), 276.
144. Dollinger J, Jose S. Agroforestry for Soil Health. Agroforestry Systems (2018) 92(2):213–9. doi: 10.1007/s10457-018-0223-9
145. Price G, Gordon A. Spatial and Temporal Distribution of Earthworms in a Temperate Intercropping System in Southern Ontario, Canada. Agroforestry Systems (1998) 44(2):141–9.
146. Bergeron M, Lacombe S, Bradley RL, Whalen J, Cogliastro A, Jutras M-F, et al. Reduced Soil Nutrient Leaching Following the Establishment of Tree-Based Intercropping Systems in Eastern Canada. Agroforestry Syst (2011) 83(3):321–30. doi: 10.1007/s10457-011-9402-7
147. Kumar BM, Jose S. Phenotypic Plasticity of Roots in Mixed Tree Species Agroforestry Systems: Review With Examples From Peninsular India. Agroforestry Syst (2018) 92(1):59–69. doi: 10.1007/s10457-016-0012-2
148. Nygren P, Fernandez MP, Harmand J-M, Leblanc HA. Symbiotic Dinitrogen Fixation by Trees: An Underestimated Resource in Agroforestry Systems? Nutrient Cycling Agroecosyst (2012) 94(2):123–60. doi: 10.1007/s10705-012-9542-9
149. Borden KA, Isaac ME. Management Strategies Differentially Affect Root Functional Trait Expression in Cocoa Agroforestry Systems. Agron Sustain Dev (2019) 39(2):1–10. doi: 10.1007/s13593-019-0567-1
150. Udawatta R P, Rankoth L, Jose S. Agroforestry and Biodiversity. Sustainability (2019) 11(10):2879. doi: 10.3390/su11102879
151. Mayer S, Wiesmeier M, Sakamoto E, Hübner R, Cardinael R, Kühnel A, et al. Soil Organic Carbon Sequestration in Temperate Agroforestry Systems–A Meta-Analysis. Agricult Ecosyst Environ (2022) 323:107689. doi: 10.1016/j.agee.2021.107689
152. Gene S, Hoekstra P, Hannam C, White M, Truman C, Hanson M, et al. The Role of Vegetated Buffers in Agriculture and Their Regulation Across Canada and the United States. J Environ Management (2019) 243:12–21. doi: 10.1016/j.jenvman.2019.05.003
153. Borden KA, Mafa-Attoye TG, Dunfield KE, Thevathasan NV, Gordon AM, Isaac ME. Root Functional Trait and Soil Microbial Coordination: Implications for Soil Respiration in Riparian Agroecosystems. Front Plant Sci (2021) 1384. doi: 10.3389/fpls.2021.681113
154. Young-Mathews A, Culman SW, Sánchez-Moreno S, Toby O’Geen A, Ferris H, Hollander AD, et al. Plant-Soil Biodiversity Relationships and Nutrient Retention in Agricultural Riparian Zones of the Sacramento Valley, California. Agroforestry Syst (2010) 80(1):41–60. doi: 10.1007/s10457-010-9332-9
155. Mayer PM, Reynolds SK Jr., McCutchen MD, Canfield TJ. Meta-Analysis of Nitrogen Removal in Riparian Buffers. J Environ Quality (2007) 36(4):1172–80. doi: 10.2134/jeq2006.0462
156. Aguiar TR, Bortolozo F, Hansel F, Rasera K, Ferreira M. Riparian Buffer Zones as Pesticide Filters of No-Till Crops. Environ Sci Pollut Res (2015) 22(14):10618–26. doi: 10.1007/s11356-015-4281-5
157. Matzek V, Lewis D, O’Geen A, Lennox M, Hogan SD, Feirer ST, et al. Increases in Soil and Woody Biomass Carbon Stocks as a Result of Rangeland Riparian Restoration. Carbon Balance Management (2020) 15(1):1–15. doi: 10.1186/s13021-020-00150-7
158. Fortier J, Truax B, Gagnon D, Lambert F. Biomass Carbon, Nitrogen and Phosphorus Stocks in Hybrid Poplar Buffers, Herbaceous Buffers and Natural Woodlots in the Riparian Zone on Agricultural Land. J Environ Management (2015) 154:333–45. doi: 10.1016/j.jenvman.2015.02.039
159. Tufekcioglu A, Raich J, Isenhart T, Schultz R. Biomass, Carbon and Nitrogen Dynamics of Multi-Species Riparian Buffers Within an Agricultural Watershed in Iowa, USA. Agroforestry Syst (2003) 57(3):187–98. doi: 10.1023/A:1024898615284
160. Hoogwijk M, Faaij A, Van Den Broek R, Berndes G, Gielen D, Turkenburg W. Exploration of the Ranges of the Global Potential of Biomass for Energy. Biomass Bioenerg (2003) 25(2):119–33. doi: 10.1016/S0961-9534(02)00191-5
161. Liu T, McConkey B, Ma Z, Liu Z, Li X, Cheng L. Strengths, Weaknessness, Opportunities and Threats Analysis of Bioenergy Production on Marginal Land. Energy Procedia (2011) 5:2378–86. doi: 10.1016/j.egypro.2011.03.409
162. Nijsen M, Smeets E, Stehfest E, van Vuuren DP. An Evaluation of the Global Potential of Bioenergy Production on Degraded Lands. Gcb Bioenerg (2012) 4(2):130–47. doi: 10.1111/j.1757-1707.2011.01121.x
163. Singh S, Jaiswal DK, Krishna R, Mukherjee A, Verma JP. Restoration of Degraded Lands Through Bioenergy Plantations. Restor Ecol (2020) 28(2):263–6. doi: 10.1111/rec.13095
164. Lemus R, Lal R. Bioenergy Crops and Carbon Sequestration. Crit Rev Plant Sci (2005) 24(1):1–21. doi: 10.1080/07352680590910393
165. Beringer T, Lucht W, Schaphoff S. Bioenergy Production Potential of Global Biomass Plantations Under Environmental and Agricultural Constraints. Gcb Bioenerg (2011) 3(4):299–312. doi: 10.1111/j.1757-1707.2010.01088.x
166. Chimento C, Almagro M, Amaducci S. Carbon Sequestration Potential in Perennial Bioenergy Crops: The Importance of Organic Matter Inputs and Its Physical Protection. Gcb Bioenerg (2016) 8(1):111–21. doi: 10.1111/gcbb.12232
167. Yang Y, Tilman D. Soil and Root Carbon Storage Is Key to Climate Benefits of Bioenergy Crops. Biofuel Res J (2020) 7(2):1143–8. doi: 10.18331/BRJ2020.7.2.2
168. De Deyn GB, Cornelissen JH, Bardgett RD. Plant Functional Traits and Soil Carbon Sequestration in Contrasting Biomes. Ecol Lett (2008) 11(5):516–31. doi: 10.1111/j.1461-0248.2008.01164.x
169. Immerzeel DJ, Verweij PA, van der Hilst F, Faaij AP. Biodiversity Impacts of Bioenergy Crop Production: A State-of-the-Art Review. Gcb Bioenerg (2014) 6(3):183–209. doi: 10.1111/gcbb.12067
170. Chambers JC, Eldredge EP, Snyder KA, Board DI, de Queiroz TF, Hubbard V. Restoring Abandoned Agricultural Lands in Cold Desert Shrublands: Tradeoffs Between Water Availability and Invasive Species. Invasive Plant Sci Management (2014) 7(1):176–89. doi: 10.1614/IPSM-D-13-00046.1
171. Yang Y, Reilly EC, Jungers JM, Chen J, Smith TM. Climate Benefits of Increasing Plant Diversity in Perennial Bioenergy Crops. One Earth (2019) 1(4):434–45. doi: 10.1016/j.oneear.2019.11.011
172. Nabel M, Schrey SD, Temperton VM, Harrison L, Jablonowski ND. Legume Intercropping With the Bioenergy Crop Sida Hermaphrodita on Marginal Soil. Front Plant Sci (2018) 9:905. doi: 10.3389/fpls.2018.00905
173. York LM, Cumming JR, Trusiak A, Bonito G, von Haden AC, Kalluri UC, et al. Bioenergy Underground: Challenges and Opportunities for Phenotyping Roots and the Microbiome for Sustainable Bioenergy Crop Production. Plant Phenome J (2022) 5(1):e20028. doi: 10.1002/ppj2.20028
174. Moyle J, Brooks L, McCrea B, Brown W. On-Farm Assessment of Switchgrass Bedding. J Appl Poultry Res (2016) 25(2):272–6. doi: 10.3382/japr/pfw011
175. Dessureault-Rompré J, Libbrecht C, Caron J. Biomass Crops as a Soil Amendment in Cultivated Histosols: Can We Reach Carbon Equilibrium? Soil Sci Soc America J (2020) 84(2):597–608. doi: 10.1002/saj2.20051
176. Pidlisnyuk V, Newton RA, Mamirova A. Miscanthus Biochar Value Chain-A Review. J Environ Management (2021) 290:112611. doi: 10.1016/j.jenvman.2021.112611
177. Alexopoulou E. Perennial Grasses for Bioenergy and Bioproducts: Production, Uses, Sustainability and Markets for Giant Reed, Miscanthus, Switchgrass, Reed Canary Grass and Bamboo. London: Academic Press (2018).
178. Dessureault-Rompré J. The Ultimate Veg Patch Can Phytotechnology Save Cultivated Peatlands? Res Outreach (2021) 124):78–81.
179. Dessaux Y, Grandclément C, Faure D. Engineering the Rhizosphere. Trends Plant Sci (2016) 21(3):266–78. doi: 10.1016/j.tplants.2016.01.002
180. Gregory PJ, Bengough AG, George TS, Hallett PD. Rhizosphere Engineering by Plants: Quantifying Soil–Root Interactions. Enhancing Understanding and Quantification of Soil–Root Growth Interactions. ASA, CSSA, SSSA. Eds Timlin D, Ahuja LR (2013) 4:1–30.
181. Ryan PR, Dessaux Y, Thomashow LS, Weller DM. Rhizosphere Engineering and Management for Sustainable Agriculture. Plant Soil (2009) 321(1):363–83. doi: 10.1007/s11104-009-0001-6
182. Ahkami AH, White RA III, Handakumbura PP, Jansson C. Rhizosphere Engineering: Enhancing Sustainable Plant Ecosystem Productivity. Rhizosphere (2017) 3:233–43. doi: 10.1016/j.rhisph.2017.04.012
183. Najar GR, Ganie MA, Tahir A. Biochar for Sustainable Soil Health: A Review of Prospects and Concerns. Pedosphere (2015) 25(5):639–53. doi: 10.1016/S1002-0160(15)30045-X
184. D’Hose T, Cougnon M, De Vliegher A, Vandecasteele B, Viaene N, Cornelis W, et al. The Positive Relationship Between Soil Quality and Crop Production: A Case Study on the Effect of Farm Compost Application. Appl Soil Ecol (2014) 75:189–98. doi: 10.1016/j.apsoil.2013.11.013
185. Jamiołkowska A, Skwaryło-Bednarz B, Patkowska E, Buczkowska H, Gałązka A, Grządziel J, et al. Effect of Mycorrhizal Inoculation and Irrigation on Biological Properties of Sweet Pepper Rhizosphere in Organic Field Cultivation. Agronomy (2020) 10(11):1693. doi: 10.3390/agronomy10111693
186. Singh VK, Singh AK, Singh PP, Kumar A. Interaction of Plant Growth Promoting Bacteria With Tomato Under Abiotic Stress: A Review. Agricult Ecosyst Environ (2018) 267:129–40. doi: 10.1016/j.agee.2018.08.020
187. Ramakrishna W, Rathore P, Kumari R, Yadav R. Brown Gold of Marginal Soil: Plant Growth Promoting Bacteria to Overcome Plant Abiotic Stress for Agriculture, Biofuels and Carbon Sequestration. Sci Total Environ (2020) 711:135062. doi: 10.1016/j.scitotenv.2019.135062
188. Van Deynze A, Zamora P, Delaux P-M, Heitmann C, Jayaraman D, Rajasekar S, et al. Nitrogen Fixation in a Landrace of Maize is Supported by a Mucilage-Associated Diazotrophic Microbiota. PloS Biol (2018) 16(8):e2006352. doi: 10.1371/journal.pbio.2006352
189. Preece C, Peñuelas J. A Return to the Wild: Root Exudates and Food Security. Trends Plant Sci (2020) 25(1):14–21. doi: 10.1016/j.tplants.2019.09.010
190. Bommarco R, Kleijn D, Potts SG. Ecological Intensification: Harnessing Ecosystem Services for Food Security. Trends Ecol Evol (2013) 28(4):230–8. doi: 10.1016/j.tree.2012.10.012
191. Tittonell P. Ecological Intensification of Agriculture—Sustainable by Nature. Curr Opin Environ Sustainability (2014) 8:53–61. doi: 10.1016/j.cosust.2014.08.006
192. Dubey PK, Singh A, Raghubanshi A, Abhilash P. Steering the Restoration of Degraded Agroecosystems During the United Nations Decade on Ecosystem Restoration. J Environ Management (2021) 280:111798. doi: 10.1016/j.jenvman.2020.111798
Keywords: rhizosphere, roots, soil functions, land management, soil restoration, agroecosystems, phytotechnology toolbox
Citation: Dessureault-Rompré J (2022) Restoring Soil Functions and Agroecosystem Services Through Phytotechnologies. Front. Soil Sci. 2:927148. doi: 10.3389/fsoil.2022.927148
Received: 23 April 2022; Accepted: 23 June 2022;
Published: 13 July 2022.
Edited by:
Sabine Grunwald, University of Florida, United StatesReviewed by:
Vishal Tripathi, GLA University, IndiaSara Ondoño, Spanish National Research Council (CSIC), Spain
Copyright © 2022 Dessureault-Rompré. This is an open-access article distributed under the terms of the Creative Commons Attribution License (CC BY). The use, distribution or reproduction in other forums is permitted, provided the original author(s) and the copyright owner(s) are credited and that the original publication in this journal is cited, in accordance with accepted academic practice. No use, distribution or reproduction is permitted which does not comply with these terms.
*Correspondence: Jacynthe Dessureault-Rompré, Jacynthe.dessureault-rompre@fsaa.ulaval.ca