- 1Department of Analytical Chemistry, The Connecticut Agricultural Experiment Station, New Haven, CT, United States
- 2Fertilizer Research and Responsible Implementation (FERARI) Project, International Fertilizer Development Center (IFDC), Accra, Ghana
- 3Division of Plant Physiology, Indian Council of Agricultural Research (ICAR)-Indian Agricultural Research Institute, New Delhi, India
- 4AgroBiosciences Program, Mohammed VI Polytechnic University (UM6P), Bengeurir, Morocco
Sub-Saharan Africa (SSA) faces chronic food insecurity associated with soil degradation and the peculiar aftermath of climate change and exacerbated by rising population and historically poor agricultural practices. Notably, use of mineral fertilizers has the potential to counteract soil degradation in SSA; it drives an increased agricultural production required to feed the rising population while sustaining the quality and health of soils. However, limited financial resources deprive SSA of the promise of fertilizers, wherein application rates are historically low, and regimes are characterized by unbalanced nutrient composition and poor fertilizer quality. Although current global fertilizer use is generally characterized by low efficiency, SSA is most affected due to the already low usage and the quality of available fertilizer products. About 70% of fertilizer-nitrogen is lost through unregulated transformation to ammonia, nitrous oxide, and nitrate that are either volatilized or emitted into the atmosphere or leached into water bodies. Similarly, the preponderance of fertilizer-phosphorus is lost via run-off and leaching, unavailing it to plants while overloading streams and rivers and, together with nitrate, causing eutrophication. These environmental problems are accentuated in SSA where fertilizer quantity and quality issues are already a limiting factor. Notably, recent advances happening outside of SSA indicate that nutrients, when strategically formulated, such as by nano packaging, (bio)polymer encapsulation, and tunable to respond to environmental cues, can provide multiple outcomes, particularly, healthy soils with higher productivity. Therefore, presumably, a proper synthesis of the gamut of soil properties influencing plant nutrient release and availability, options for plant exposure and uptake is critical for realizing these benefits in SSA. Despite these possibilities, there is a lack of deeper context on fertilizer-related issues as they affect food and nutrition security and the health of soils in SSA. This paper provides an overview of the fertilizer-nutrient and associated agronomic, food insecurity and soil environmental challenges and opportunities, which though not exclusive to SSA per se, can be reasoned with the peculiarity of the region. This provides the impetus to increase fertilizer use efficiency, improve soil and environmental health, sustainable crop production, and food and nutrition security in SSA.
1 Introduction
Food security is achieved when everyone within a given geographical space at every time has physical, economic, and social access to sufficient, safe, and nutritious food that meets their food preferences and dietary needs for an active and healthy life (1). The challenge of food insecurity remains a global concern under the Sustainable Development Goals (SDGs), with about 720 (9.2%) to 811 (10.4%) million hungry people in 2020 (1). This number is even higher (21%; 278 million) in Africa, particularly the region below the Sahara Desert, SSA. Although these numbers have increased due to the Covid-19 pandemic and the ongoing Russia-Ukraine war, the global community was already off-track in achieving a zero-hunger target by 2030, particularly in the SSA regions.
The rising African population (estimated at 1.45 trillion by the end of 2023), pronouncedly so in SSA, coupled with the changing food preferences means that food production must more than double to meet the food needs in 2050 (2). There are, however, several challenges hampering food production in SSA, as with other parts of the globe. Up until recently, agriculture in SSA advanced under relatively stable climatic conditions; however, changing climate, primarily due to rising temperatures and erratic rainfall is jeopardizing the productivity and resilience of the agricultural and food systems (3–5). The rising population of the many low- and middle-income countries of SSA will worsen the diet-related environmental pressures (6). What is more is that the current diets comprise of high-calorie, nutrient-poor foods deficient in protein, vitamins, and minerals (7). The resulting hidden hunger has been devastating for the health of the SSA population, where it is carried over to the next generation, with the attendant loss in economic productivity.
One of the most compelling factors jeopardizing agricultural productivity increase and resilience, which is also a major threat to the global food and nutrition security is soil degradation (8, 9). Specifically for Africa, it was time long ago estimated that as much as 40% of the soil is degraded 10); whereas a recent estimate puts that number at 65% of the arable land (10). In general, soil degradation results from interrelated factors, including population pressure, disinvestment on land due to tenure insecurities, changing climate, agricultural land expansion, poor agronomic practices such as ploughing along slope, excessive bush burning, uncontrolled grazing, and low or excessive application of agrochemical inputs, namely mineral fertilizers, and pesticides (11, 12). All of these factors, with the exception of excessive application of agrochemicals, are more than elsewhere very peculiar to the SSA agricultural system (13), where extensification rather than intensification has continued to be the norm. Notably, soil degradation directly impacts soil health – defined as the continued capacity of soil to function as a vital living ecosystem that sustains plants, animals, and humans (14). An acceptable soil health condition is attained through maintaining a balance among critical soil properties, including microbes, plant root function, and physicochemical properties such as pH, organic carbon, moisture, and nutrients. These properties direct plant responses to fertilizer application and are in turn, themselves influenced by the chemistry of the nutrients in the fertilizer. Accordingly, for a continent that is behind in fertilizer application rates, the technology, and resources to sustain research and analytical requirements, the concept of soil health, and the ecological implications of fertilizers thereof on SSA can starkly vary from those of other regions such as South-East Asia (India-China), North America, and Western Europe.
With over 90% of food cultivated on soils on an increasing acreage of cultivated area, a cumulative of about 12 million hectares are degraded annually in SSA. There is a potential food loss of about 20 million tons of grains annually due to soil degradation (15), and which for SSA is associated with a 3% compression of GDP (10). Depending on the method of assessment, a 10 cm soil loss leads to a 4.3-26.6% reduction in crop productivity (16). Consequently, Pozza and Field (17) coined ‘soil security’ and ‘food security’ as two of the seven interlinked global challenges. Clearly, SSA is more affected by soil-related insecurities, wherein, for example, a country like Egypt decreased the land area under rice cultivation from about 0.45 million hectares in the 2016 season to only 294,117 hectares in 2018, which has remained in effect to date due to non-abating of the water scarcity problem that was induced by chronic drought. Similarly, Somalia, with devastating chronic drought. Most Somalis being nomadic pastoralists, and with livestock production diminished by the drought that has affected forage crop production, millions have had to abandon their lands. Together, drought and other natural, but also man-made, factors have resulted in a situation where one out of every five persons in SSA (20%) is estimated to retire to bed hungry, and where at least 140 million people on the continent face acute food insecurity, as of 2022. Notably, with drought arises the problem of salinity due to concentration of salts in the soil. However, there is evidence that a strategically devised fertilizer or plant nutrition regime can contribute to mitigating these effects (e.g. 19-21).
Given the above scenarios, proper management of the fertility of soils is paramount, if food security is to be achieved in SSA, but not at the cost of environmental degradation such as observed in China recently. A thoughtful of Integrated Soil Fertility Management (ISFM) that calls for the combined use of inorganic and organic fertilizers (18, 19), with other improved production practices such as rotation with leguminous crops (20, 21) and climate-smart systems can increase yields and resilience of the production system. Starting with the impact of COVID-19 in the third quarter of 2021 and then the Russian-Ukrainian war in 2022, the prices of inorganic fertilizers have increased sharply, with a negative effect on the already low fertilizer use regimes in SSA. Coupled with other challenges, this is expected to affect the gains made in reducing food insecurity in SSA. Therefore, it is necessary to delve deeper into how the continent can govern its food production through policies on fertilizer availability and use for food and nutrition security, while keeping an eye on the soil health implications of fertilizer use. Notably, research on the nexus of fertilizers, soil and agro-environmental health, and productive agriculture is limited in SSA. This review attempts to provide an overview of the nutrient and associated agronomic, environmental, and socio-economic issues that influence the SSA fertilizer use efficiency, and soil health. The subsequent sections delved specifically into nutrient stripping and its implications on soil health and food security; the role of macronutrients and micronutrients for food security; micronutrients for biofortification towards human nutrition security and soil health; rhizosphere science for soil health-compliant product development in SSA; and the general Perspectives on implications of mineral fertilization on soil health, and ways forward for SSA.
2 Nutrient stripping from the land: effects on soil health and food security
Several traits have been proposed to rate soil health, such as the inherent availability of essential nutrients, soil texture, organic matter composition, and the diversity of the contribution of soil microbes to soil fertility and plant productivity (21). Accordingly, soil health can be impacted when nutrients become deficient or excessive. In SSA, incidences of nutrient deficiency are common, relative to nutrient excessiveness (22, 23). Nutrient deficiency results from a myriad of events. Nutrient stripping (24), defined as the continued removal of native nutrients from the soil by growing plants and by grazing animals, without replenishment through fertilization, is a natural first event. Other major sources of nutrient loss are soil run-off, leaching, emission, and volatilization, and fixation of nutrients in chemical complexes that preclude their immediate availability for plant use. In Europe, North America, and most parts of Asia, nutrient stripping from soil by plants without strategic replenishment has been minimized, if not eradicated, decades ago, due to adoption of strategic fertilization regimes that involves periodic soil testing and supplementation with missing or inadequate nutrients (25, 26). Unfortunately, nutrient stripping has continued in SSA, with dire consequences on the level of agricultural productivity. As reported, the overall net N, P, and K balances for SSA is negative, -700, -100, and -450 kg/ha, compared to +2000, +700, and +1000 kg/ha, for Europe and North America over a 30-year period (27). Moreover, the strong variability in soil properties across the different SSA countries could worsen nutrient loss from soil. For example, Alfisol soils inherently high in Fe and Al oxalates increases loss of labile P fraction, causing, as reported, significant differences in the residual, plant-usable P status between countries such as Nigeria and Angola (28). Figure 1 represents a comprehensive depiction of the major features of soil nutrients paradigm in SSA, namely input, legacy stock, loss pathways and indigenous recycling options.
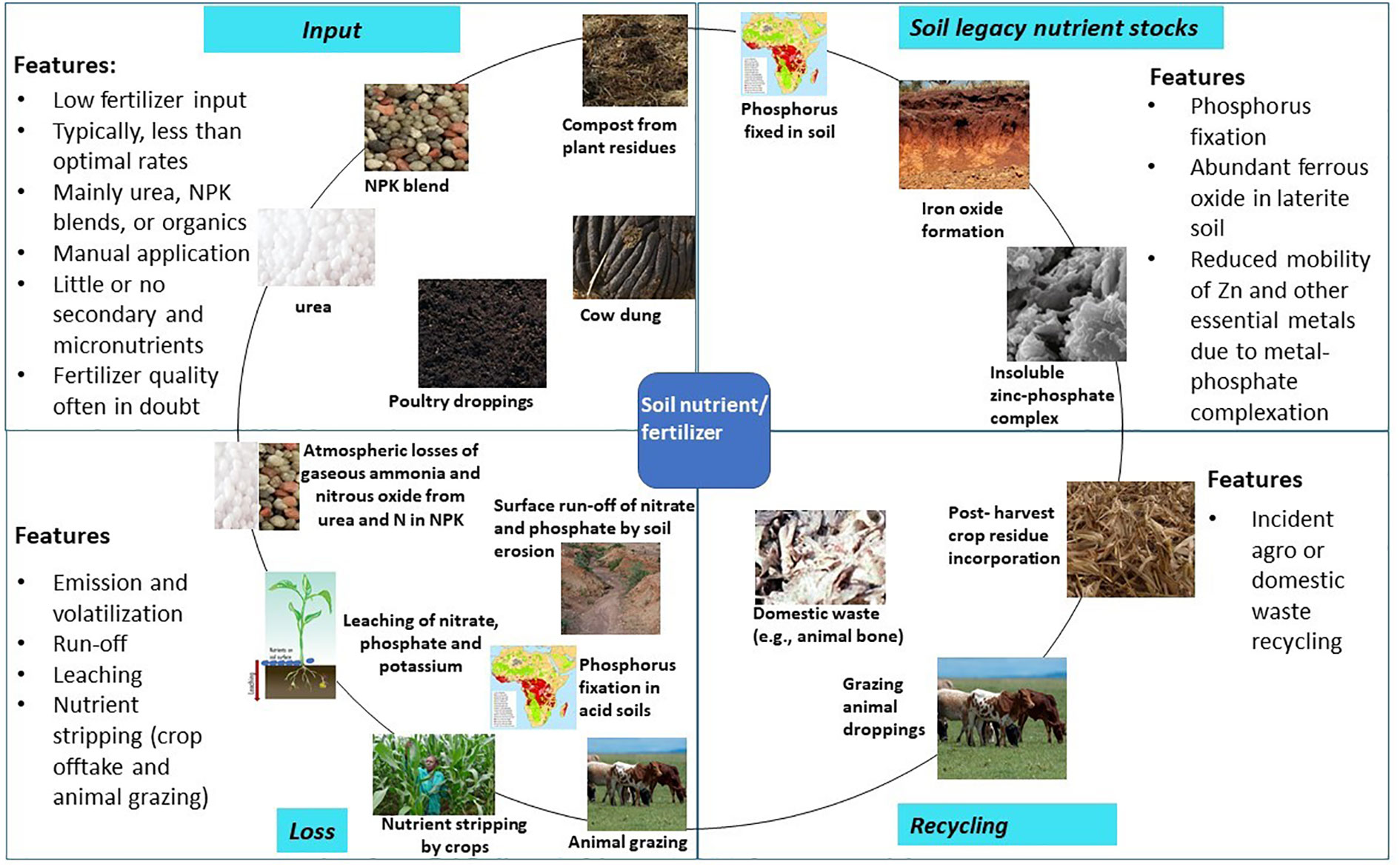
Figure 1 A comprehensive depiction of the major features of soil nutrients paradigm in SSA, namely input, legacy stock, loss pathways and recycling options, with highlights of the main components of each feature.
2.1 Nutrient input
As noted, SSA is characterized by low fertilizer input, despite a rapid increase in the fertilizer consumption in the recent years In 2006, the rate was so low at 8 kg of nutrient per hectare that the African Union member states met in Nigeria and adopted the “Abuja Declaration on Fertilizer for the African Green Revolution. There, they pledged to increase fertilizer use to 50 kg/ha by 2015, which, even at that, is far less the average level of 150 kg/ha in other regions of the world. Unfortunately, since then, the average fertilizer application rate in SSA has stagnated between 13 and 20 kg of Nutrient per hectare (29). The effect of low application rate is worsened by the unbalanced nature of the products available on the continent, whereby most farmers use mainly urea-nitrogen (N), phosphorus (P) and potassium (K), with no consideration for the 11 other essential elements that could sustain crop production under the inherent adverse climatic or edaphic conditions. The lack in soil of elements such as zinc (Zn), boron (B) and (Cu) is known to result in the phenomenon of non-responsive soils, whereby application of NPK does not engender a marked increase in yield over the control plot (30, 31). These nutrients are known to particularly facilitate N utilization by plants (32–34). The combined effects of low application rate and use of unbalanced nutrient composition are further accentuated by the quality of fertilizers in the SSA marketplace. Sanabria et al. (35, 36) noted that fertilizers sold in certain places in SSA, including Uganda and West Africa, especially Nigeria, were of low nutrient quality in terms of not meeting label claims. Product adulterations that happen within the value chain, together with poor fertilizer regulatory framework, were blamed for the poor-quality fertilizers in these places.
Notably, in SSA, production systems with low mineral fertilizer input are frequently augmented with organic materials such as poultry waste, cow dung and plant biomass compost (e.g., 32, 42, 43). However, particularly for cow dung and compost, the quality of such additives is often in doubt since they originate from plant materials grown in already nutrient-compromised soils and can only return as much nutrients as they stripped from the soil, sort of garbage in, garbage out. For poultry waste, the potential for contamination with microbes, antibiotics, and heavy metals remains a concern. In addition, the strong and unpleasant odor from ammonia and hydrogen sulfide in poultry waste have precluded its widespread acceptability as a manure source (37, 38).
2.2 Nutrient legacy stock
Upon fertilizer application, the nutrients they contain are faced with a myriad of fates. For P, one such fate includes fixation in soil. In SSA, only about 5 kg P/ha is historically applied to soils (39, 40) which is projected to reach at least 23 kg/ha by 2050 (39). A huge fraction of that already low P becomes fixed in soil under acidic conditions characterized by iron (Fe) and Aluminum (Al) oxides. Simultaneous with P, acidic soils are also characterized by the presence of ferric oxide which can also potentially undergo reduction to ferrous Fe as pH is modulated by agricultural practices such as lime application. Fixation of P with Fe or Al in acidic soils reduces nutrient mobility, which can be compounded by the presence of Zn and other essential metals due to the formation of metal-phosphate complexation. Given 30% acidic soil occurrence in SSA (41, 42) and a P use inefficiency rate of up to 70%, a large proportion of the applied P is trapped in the soil, leaving a pool of so-called legacy P. P fixation is reportedly the major reason for P deficiency in SSA due to this high P fixation capacity of the soils. However, P- fixation is hardly a dead-end phenomenon; changing soil conditions can result in the ‘‘unfixing’’ of P, making it available over time. Outside of Africa, addition of lime has been for long a common practice to alter soil chemistry via changing the pH, a process that should unlock hitherto fixed P (43). Notably, liming of acid soils was not a common practice in SSA, reflecting a hitherto lack of awareness of its contribution to soil fertility (44). However, the trend has started to change in recent times following improved R&D and extension services, to the extent that countries such as South Africa, Kenya and Burundi have established factories that produce fertilizer-lime from locally abundantly available dolomitic lime. The Burundi factory established in 2019 produces up to 70,000 tons/annum, dedicated to addressing soil acidity in the country (https://esaffburundi.org/news/burundi-has-established-its-own-fertilizer-factory/).
2.3 Nutrient loss
Whereas legacy P is not lost to the soil, but rather rendered unavailable for immediate plant use, actual nutrient losses occur with N, but also with the more labile P form, under certain environmental conditions. N loss is a complex process involving losses at multiple points. This means that more frequent external supply of N via fertilization and biomass residue incorporation is critical for maintaining N in soil. The primary N loss occurs during the transformation of urea to ammonium, in which as much as 50% of N is lost as gaseous ammonia volatilization. Subsequently, another smaller fraction of N is lost as nitrous oxide emission during the transformation of ammonium to nitrate (45). The effect of such transformation is worsened in SSA, where low application rates mean most of the N is lost as gaseous volatiles or emissions. Again, ammonia volatilization, just like labile P loss, is exacerbated in alfisols with low pH buffering capacity, as reported for the East African highlands (46). Similarly, nitrous oxide emission is facilitated under acidic condition (47). Nutrient loss by run-off and leaching occurs with N and P in the form of nitrate and phosphate ions that are highly mobile and worsened in soil conditions with low organic matter and in erodible soils under high rainfall. This way, up to a significant amount of applied N or P can be lost from the soil through spatial transportation, resulting in nutrient overload in underground and surface water bodies which has increased aquatic pollution and the occurrence of eutrophication in SSA inland waters (48). As for nutrient stripping by crop offtake and animal grazing, it is estimated that a metric ton grain of maize can remove as much as 13 kg of N, 6 kg of P, and 4 kg of K from the soil (https://www.canr.msu.edu/news/nutrient_removal_rates_by_grain_crops). Notably, nutrients in edible portions of crops are completely lost from the soil as the material are exported from the field. Similarly, animal grazing can strip the soil of significant amounts of nutrients for their body mass development.
2.4 Indigenous nutrient recycling
Cutting-edge research and development efforts in nutrient recovery for recycling in agriculture such as can be said to be non-existent in SSA. However, incident agrowaste recycling has always been a natural phenomenon in SSA’s history, whereby nutrients in agriculturally derived residues are recycled by incorporation into the soil prior to, or following, composting. Literature (49) on Liberia and Ghana exemplifies a manifestation of such indigenous nutrient recovery practices in West Africa. In that case, the incorporation of biomass such as ash and char residues from cooking with wood, residues from processing palm oil, animal bones, and plant harvest residues including palm thatch, palm fruit heads, and rice straw transforms hitherto poor soils into N, P, and carbon-enriched soil dubbed “African Dark Earths” that improves crop performance, relative to adjacent soils. ‘‘African Dark Earths’’ soils contain up to 367 and 400% more P, and 93 and 42% more N, for Liberia and Ghana, respectively, at shallower depths (<0.2 m), compared to the adjacent soils; similar observations were made for P at deeper (<2.5 m) soil layers. Under the indigenous recycling system, as much nutrient as was initially taken up into the non-edible plant tissues can potentially be resupplied into the soil, especially in the case unprocessed plant harvest residues. However, given that such nutrients are in an organic form, their immediate bioavailability to plants is limited. Decay or weathering needs to happen to unleash the organically locked nutrients for plant use. Similarly direct waste droppings from live animals resupply nutrients to the soil. It is estimated that as much as 39% of the N from mineral fertilization can be recovered from cattle urine and feces and recycled in soil from cattle grazing on grass (50). It can be envisaged that the balance of N in this case is involved in the animal growth, with losses certainly occurring during the N transformation process.
2.5 Implications of nutrient stripping in SSA: soil testing and balanced fertilizer regimes
In real terms, fertilizer application as a cultural practice is directly related to the farmer’s economic ability and the ready availability of efficient and affordable fertilizer products. Thus, farms in most of SSA characterized by limited financial resources experience a net depletion of nutrients in the long term, relative to those in more agriculturally advanced regions with higher purchasing power per farmer, the industrial manufacturing base for local mineral fertilizer production, or the national financial ability to be reliant on imported fertilizers. The negative economic implication of nutrient stripping from soil is, therefore, more pronounced in countries in SSA with limited financial and material options for the soil fertility management through local production, and established soil testing and fertilization regimes. Fertilizer subsidy program have been set up in different countries; however, the overall effectiveness of such subsidy programs in improving farmers’ livelihoods remains to be seen (51).
A quantitative and qualitative understanding of nutrient stripping from soil by harvested portions of crops is a critical consideration for designing fertilizer application regimes that account for rates and balancing of the different nutrients. However, such information alone is inadequate for making fertilizer recommendations because it does not consider the ability of the soils to retain and supply nutrients. Hence, periodic soil testing is imperative to provide information related to the diversity and quantity of nutrients that may be lacking and require supplementation at any given time (32). Other related critical factors to consider alongside soil fertility improvement towards improving crop yields relate to the production climate, varieties, and management (52). Several of the countries in SSA lack soil and plant tissue testing facilities that are equipped with modern instruments to quantitatively and qualitatively determine nutrients (e.g., Skalar segmented flow analyzer for N and P; CSN combustion analyzer for N; Inductively Coupled Plasma Optical Electron Spectroscopy/Mass Spectroscopy for P and other elements, except N and Cl). Where they do have these, there is in many cases a lack of trained technicians to operate the instruments, and the costs are prohibitive for the small holder farmers. For example, a basic soil analysis for pH, %OM, %N, P, K, Mg, Ca, with recommendation costs 2000 Shillings in Kenya as of 2017 (32), which is about 16 US Dollars. Efforts to improve soil testing capability in SSA has for long been spearheaded by the International Fertilizer Development Center, with laboratory analytical training provided for different countries, especially in West Africa.
A second implication of nutrient stripping in SSA is the imbalance created by both the low rate of fertilizer application and the increased uptake of non-NPK nutrients by NPK-induced biomass proliferation. Biomass increase particularly from N fertilization increases plant biomass, which by itself is desirable for leafy vegetables and ornamentals. However, excess vegetative growth can worsen the stripping of micronutrients such as zinc (Zn) and iron (Fe). In production systems such as those of SSA lacking established fertilizer regimes that are based on soil testing of all nutrients, micronutrients could be completely stripped in the soil in the long term, with chronic negative health outcomes for animals and humans through the persistent consumption of micronutrient depleted agricultural and feed produce (53, 54). Ultimately, under little or no nutrient replenishment through fertilizer application, crop yields decline with the increasing absence of both macro and micro-nutrients. Therefore, when viewed from a broader perspective nutrient stripping has layers of ramifications for SSA, transcending the soil-plant nexus to human and environmental health and to national economic productivity.
3 Macronutrient (NPK) requirements to increase food production in SSA
As the basic nutrients required in large quantities for optimum growth and development of plants, NPK are basically the major limiting nutrients for crop production. Therefore, these nutrients must readily be available through fertilization to improve on crop yields (55, 56). While the application rates of NPK are collectively low and on the decline in Africa (Figure 2), the values varied by country (Figure 3). In addition to Nigeria and Egypt, the use of NPK for agricultural purposes is high in seven other countries (Algeria, Burkina Faso, Ghana, Kenya, Malawi, Morocco, and Zambia). Each of these macronutrients has specific role in the development and growth of plants. However, further supplementation of other nutrients including sulfur and micronutrients enhances the use efficiency of macronutrients. For instance, the application of macronutrients to maize, rice and wheat increased grain yields above zero fertilizer applications, but the yields become even higher for all three crops with the application of macronutrients with S and micronutrients (23).
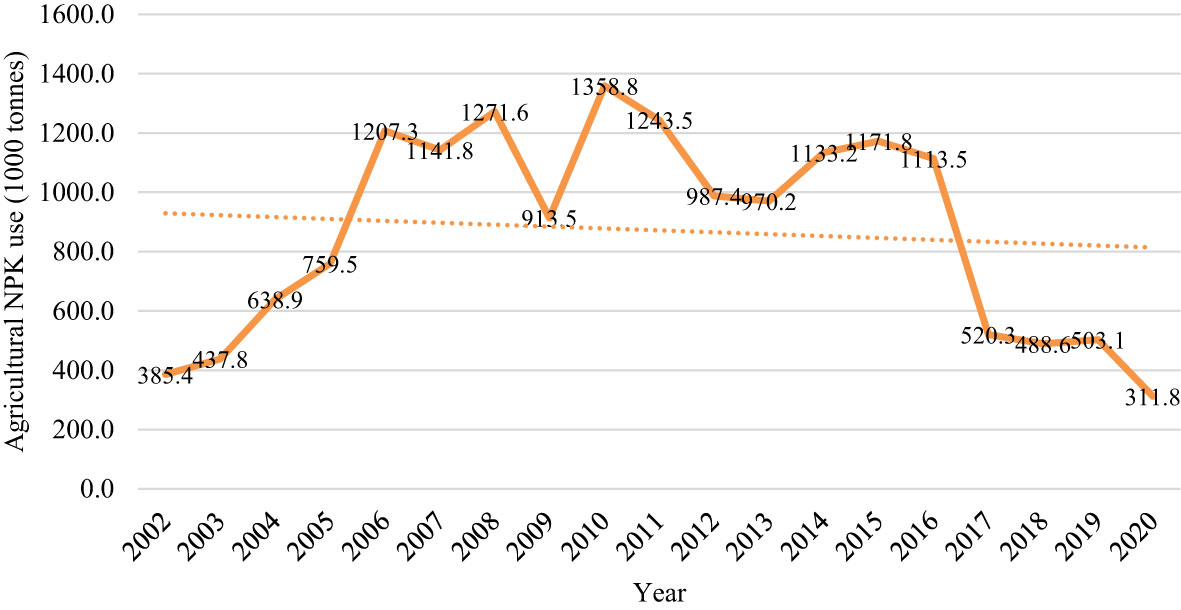
Figure 2 Total agricultural use of NPK in Africa. Source: FAO database; https://www.fao.org/faostat/en/#data/RFB.
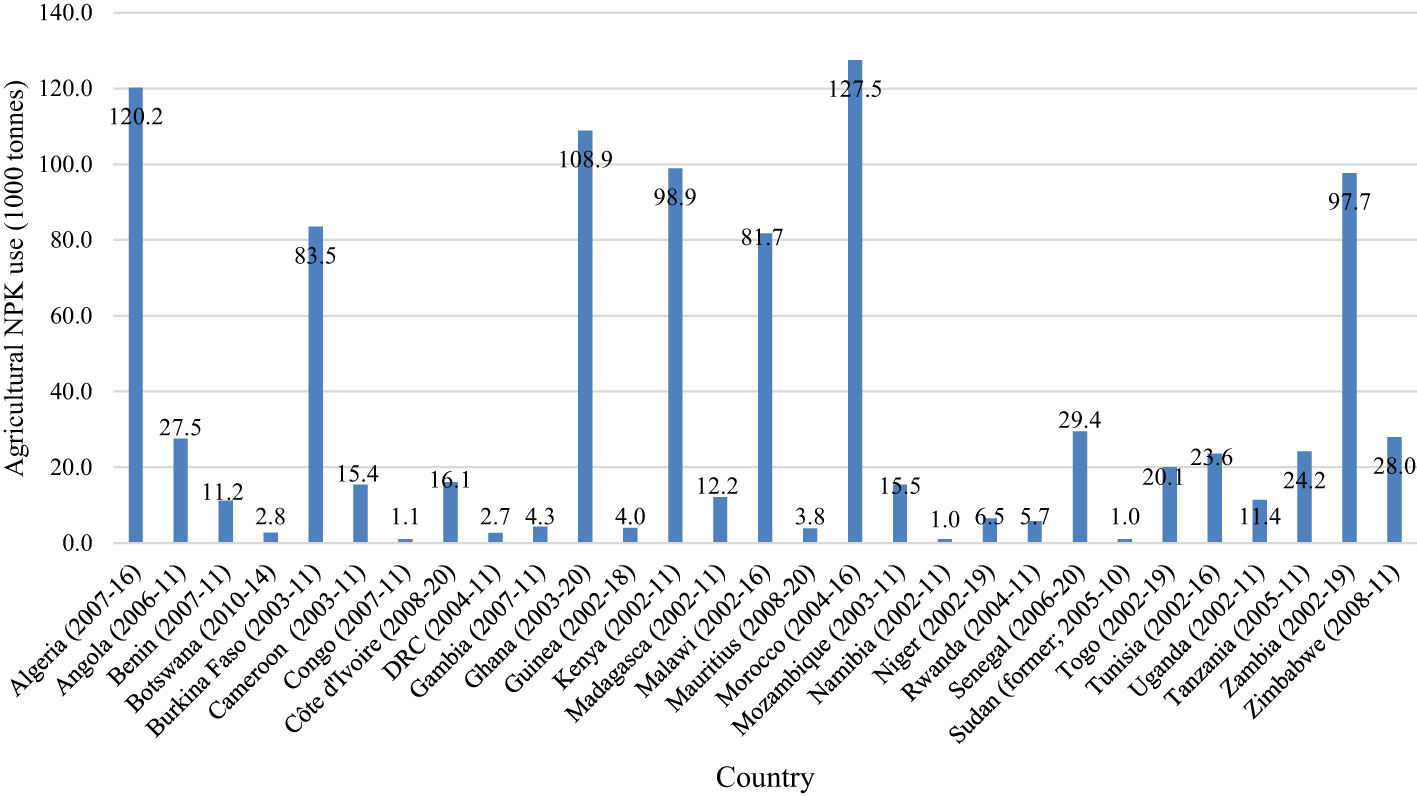
Figure 3 Average agricultural use of NPK by African countries. Burundi, Central African Republic, Eritrea, Ethiopia, Gabon, Seychelles, and South Africa had less than 1,000 tons while Nigeria and Egypt had over 200,000 tons, hence, were not included in the graph. Years considered are in parenthesis. Source: FAO database; https://www.fao.org/faostat/en/#data/RFB.
Nitrogen is an important nutrient for food production although its usage is limited in African countries in particular (57). Achieving food self-sufficiency in Africa requires that N usage increase from 35 kg/ha to 181 kg/ha per year during 2016-2050, although this will be associated with high N losses and low nitrogen use efficiency (57). In maize production, for instance, N application at 120 kg ha-1 is found optimal for yields in SSA, and beyond this application, the N is stored in the leaves and stems, making it very useful for animal fodder (58). However, considering the diversity in the soil types, the optimal rate may vary while Elrys et al. (57) admitted that considering several constraints facing the African fertilizer sector, it is impossible to attain the 181 kg N/ha per year application. For example, Essel et al. (59), observed that the application of N at 61 kg ha-1 produced optimal maize yield in the semi-deciduous zone of Ghana. The N management towards ensuring food security must comprehensively consider how to improve N use efficiency within the food system, accounting for both fertilizer-N product development and improved application regimes (60–63). Since poverty hinder the production and consumption of crops and livestock with less virtual N factors and soil N depletion factors towards soil N depletion and emission in Africa, nitrogen use efficiency measures must be promoted with a gradual shift towards foods with low virtual N factors (64). The use of locally available organic materials together with the balanced use of mineral N fertilizers can also be used in improving soil N in Africa (65). This is crucial considering the very low agronomic efficiencies of N at 14.3 to 23.9 kg additional grain yield per kg of N applied. Therefore, to achieve projected food security in 2050, the application of N must increase by 9 -15 folds to achieve the expected 49% -75% increase in maize yields 9). Ten Berge et al. (60) observed under separate scenarios that N application rates must increase in both the long and short terms to maximize maize yields in East and West Africa. Such increases must however be optimally determined to reduce N footprints. Together with the adoption of modern agricultural practices and organic inputs, the application of N at rate of 77 kg/ha/year is appropriate in SSA (57).
Phosphorus is another crucial macronutrient for crop production in SSA. Regardless of different share socioeconomic pathways (SSP) built on different assumptions, the quantity of P used in SSA must increase by 310-620% in 2050 over the 2016 usage of approximately 560,000 tons (63). An increase in P levels is necessary to guarantee that agriculture production meets the food needs of the rising population and changes in the socioeconomic characteristics of people. In addition to the amount of P applied, its pricing must be given important consideration (60, 61). Compared to N and P, maize yield response to K is low in SSA (66).
4 Role of non-NPK nutrients in crop production: effects on environmental stress mitigation, N and P utilization, and implications for SSA
Although N, P, and K constitute the primary nutrients used by farmers in SSA, other nutrients are as equally essential, and particularly beneficial for modulating plant performance under such environmentally challenged production conditions that are prevalent in SSA. These nutrients include sulfur [S], magnesium [Mg], calcium [Ca], Cu, B, Fe, manganese [Mn], molybdenum [Mo], nickel [Ni], Zn, and chlorine [Cl]. Currently, drought, disease, salinity incidences and poor nutrient use efficiency are the major factors militating against crop production in SSA. Soil moisture deficit due to drought controls nutrient availability in the soil, corroborated by a meta-analysis by He and Dijkstra (67) showing that a lack of moisture inhibited N and P uptake by plants in continuously dried conditions. Notably, 45% of the terrestrial surface of SSA is dryland (FAO; https://www.fao.org/3/XII/0169-B3.htm). A consequence of this can be illustrated by an example with teff cultivation in Ethiopia in which about 50% loss in yield is recorded (68). Salinity also affects the availability of nutrients from fertilizers in soil. A recent global soil salinity map indicated that SSA is being seriously impacted by salinity, particularly in the South-Western and Eastern parts (https://www.fao.org/3/cb7247en/cb7247en.pdf). In terms of numbers, approximately 80 million hectares of land are salt affected in SSA, representing about 8.6% of the global salinity burden (reviewed in 76). For South Africa, for example, the number was earlier reported to be up to 32% of the country’s land surface (69). At the high ionic strength of highly saline systems, nutrient imbalances occur because of competition by Na and Cl ions with nutrients such as K, Ca, and N (in the nitrate form). Disease incidences in SSA are no less prevalent as drought and salinity. According to FAO estimates, as much as 40% of crop yield is affected by disease, costing SSA about 200 billion in economic loss annually (https://guardian.ng/features/agro-care/nigeria-other-african-countries-lose-200b-to-plant-pests-diseases-yearly/). About 12% teff crop loss is consistently reported in Ethiopia due to disease burden; over $1 billion in losses are reported for cassava due to mosaic virus in East Africa (68), to cite a few specific examples. The prevalent SSA environmental conditions of ideal warm temperature, high relative humidity, and even soil infertility (as with the case of the parasitic weed, Striga hermonthica) can exacerbate disease occurrence. Though conventional fertilization in general keeps plant healthy; and healthy plants can better tolerate or resist environmental stresses, recent advances in agrochemical packaging, especially with respect to nanotechnology (the exploitation and design of materials at the nanoscale, ≤ 100 nanometers, resulting in materials with higher reactivity and improved functionality), have elucidated important new information on alternative nutrient assimilation and functionality in the form of nanoparticles (e.g., 78, 79, and references therein) with potential relevance in addressing environmental stress in plant. Such novel applications have ranged from deciphering non-NPK nutrients involvement in N metabolism, photosynthesis, to antioxidative responses and biotic stress regulation that enable them to contribute to sustaining plant performance under adverse production conditions associated with climate change, such as nutrient deficiency, drought stress, salinity stress, and pathogen infestation. Mechanistically, these nutrients engender greater elicitation of appropriate molecular responses that reflect in observable agronomic outcomes. Unfortunately, studies conducted in SSA evaluating these nutrients under these environmental stress conditions are sorely limited. However, we will briefly highlight here some of the novel advances in these non-NPK nutrients that are related to tangible agronomic outcomes under stressful production conditions to, hopeful, spur research efforts to validate them under various SSA agroecological conditions.
4.1 Nutrients in drought stress mitigation
The influence of nano Zn on crop yield and N uptake under drought was reported, wherein soybean biomass production, grain yield, and N uptake were reduced by 27, 54, and 43%, respectively by drought but were alleviated by 33, 36, and 28% when Zn was applied to the plants (32). Similar findings were reported for drought-stressed sorghum, whereby flag leaf and spike emergence were delayed 6-17 days grain yield was reduced by 76%, grain N and K translocation was reduced by 57% and 71%. However, augmenting the plant nutrition with nano Zn reduced flag leaf and grain head emergence delay to 4-5 days, improved grain yield by as much as 183%, improved grain N translocation by 84%, and improved grain K acquisition by 123% (34). Under an Egyptian field condition with 60% evapotranspiration (hence, drought stress), plant membrane stability, relative water content, and photosynthetic efficiency were all negatively affected, resulting in reduced eggplant growth and yield. These effects were ameliorated by fertilization with nano Zn, leading up to a 23% fruit yield increase (70). Such findings for Zn and other nutrients, including Cu and Fe (reported for e.g., in 81, 82), clearly evidence the mitigation of a critical aftermath of a changing climate, drought, by non-NPK nutrients. Specifically, for Zn such role is related to the upregulation mechanism of on abscisic acid (ABA) production in plants (71, 72), the effects of which enhances stomatal regulation under water stress condition (73). It is also likely that the role of Zn in evoking this soil-health indicative rhizospheric activities via modulating microbial metabolism and enzyme activity (74, 75). Undoubtedly, the ability of Zn to accelerate plant phenological development, promote grain yield, improve N acquisition under drought stress and contribute to plant resistance or tolerance to abiotic stresses has strong implications for increasing cropping systems resilience under adverse production conditions. These findings, therefore, deserve to be validated in SSA and other regions of Africa, where drought has become endemic. Unlike N where synergistic interactions with micronutrients under drought are reported, the interaction of P with Zn, Fe, Mn and other metallic micronutrients show mostly antagonistic effects on all involved nutrients (Reviewed, for e.g., in 88). And such outcome can be worsened under drought (67). Studies (32, 34) corroborate this in soybean and sorghum, wherein P uptake from soil under drought was reduced by 33% and 23%, respectively, in the presence of nano Zn.
In the P-metallic nutrient interface, P mobility in soil, uptake in the roots, and potential translocation within the shoots can be affected due to the formation of metallo-phosphate complexes (75). Findings from greenhouse plant growth supports accumulation of P in soil in the presence of Mn (76). From a P sustainability standpoint, the question arises as to what the best choice is between stocking P (legacy P) and losing P? With a low P application rate in SSA, but high P leaching events, as reported for East Africa (77), an argument can be made that metallic micronutrient complexation with P can be a way to reduce P loss and attendant pollution of the aquatic ecosystem by building up legacy P that could subsequently be harnessed.
4.2 Nutrients in salinity stress mitigation
Soil salinity negatively affects the availability of nutrients to plants due to competition between salts and dissolved nutrients. Other nutrients such as Zn and Cu, among other metals will aggregate at low ionic strength (78), potentially reducing their mobility and availability as well. As noted in the preamble, accumulation of salts in the soil promotes plant assimilation of Na and Cl, affecting crop productivity and soil health, with significant effects in SSA given the high prevalence of soil salinity. Inadvertent use of contaminated water for irrigation is one of the leading causes of soil salinization across Africa, both in the coastal zones abutting inland rivers with reported intrusion by sea water (e.g., Nile River) and in the central hinterlands in places like Nigeria (79). Notably, fertilization of salinity-stressed crops with nutrients other than NPK has been demonstrated to alleviate salinity-induced loss in productivity under both field and greenhouse conditions (reviewed in 93). Salinity studies from Egypt involving irrigation of bean plants with water containing up to 4000 mg/l NaCl indicate that foliar spraying the plants with 12% conventional Fe, Zn and Mn could negate the salinity-induced effects on dry weight and nutrient accumulation (80). Extensive literature search reveals a lack of studies demonstrating novel effects of nano-based nutrients on salinity mitigation in SSA. However, the few studies conducted outside the region in Africa and elsewhere show several promising outcomes. For example, in Egypt, broad bean stressed by salinity with up to 150 mM NaCl and treated with a foliar spray of nano Zn at up to 100 mg/l were ameliorated of the oxidative stress induced by salinity via evocation of several metabolic responses, including accumulation of antioxidants, osmolytes, and secondary metabolites, that contributed to enhancing plant growth, compared to plants stressed with salinity but with no nano Zn fertilization (81). Similarly, nano Fe at 25 mg/l were evaluated in the tree species, Eucalyptus tereticornis, for effect on salinity (300 mM NaCl), resulting in several subtle molecular-level changes that led to increased shoot length, chlorophyll content and the resolution of iron deficiency symptoms under salt stress (82). The later study has implications for addressing a critical environmental issue in agroforestry, warranting exploration by international organizations such as the International Center for Research in Agroforestry (ICRAF) based in SSA. Viewed more broadly, soil salinity clearly has significant implications for farmers’ livelihood, causing, for example, crop yield losses of up to 27.3 billion US$ globally (83). Therefore, the translation of the utility of fertilization to mitigate these impacts in the African context can contribute to soil and environmental health improvement.
4.3 Nutrients in plant health promotion: disease and toxic elements stress mitigation
Among nutrients with role in improving plant health, S has long ago been shown to increase crop yield when added to soils in West Africa (e.g., 98). However, Wang et al. (84) elucidated novel functions of S in plant disease suppression using nano particles of S. In greenhouse (GH) pot experiments they observed a reduction in Fusarium disease infection in tomatoes up to 56% that is concomitant with nano S-type and time-dependent S translocation patterns suggestive of a different uptake mechanism from the traditional sulfate accumulation pathway. Translocation of S into the plant was associated with enhanced biosynthesis of disease resistance and plant immunity-related metabolites. When these researchers replicated the nano S treatment in the field, the GH-based mechanistic findings could explain increases in tomato fruit yield under the Fusarium disease burden. Subsequent field data with these nano S applications (85) show that while disease reduced plant yield by 77%, soil fertilization with S under disease conditions improved yield by 98% with conventional S (bulk S and sulfate), and by 160% with nano S, relative to the control. Sulfur deficiency is prevalent in SSA soils (86) A lot of the S in soils is derived from acid rain from fossil fuel processing and which occurs in places like Nigeria, Algeria, and Angola, among several others. However, with the current improvements in fossil fuel refining technologies, S deposition from acid rain would reduce; up to 90% reduction is already reported by the US EPA (https://www.epa.gov/acidrain/acid-rain-program-results). While reducing S deposition from fossil fuel refining is a good development from a soil health perspective, it would also facilitate S deficiency in soil, in the absence of a strategic S fertilization regime such as the use of nano S fertilizers that allow lower nutrient application rates that could control S-induced soil acidification.
A role for Mg in enhancing N use efficiency by increasing crop N uptake was recently demonstrated (87). Mg fertilization with nano-packed fertilizers has been demonstrated as capable of inducing disease resistance in plants against stress from both fungi and bacterial infections. The effect is related to boosting the plant immune system and sustaining production under biotic stress, particularly when administered before the stress emergence (e.g., 88, 89). Mg deficiency may be thought to not be a concern in SSA; however, it is likely that soil testing for this nutrient would reveal the true status of Mg in SSA soils, as suggested by the National Soil Testing Maps for Burundi (https://ifdc.org/2022/12/08/soil-acidity-and-fertility-mapping-in-burundi-2022-edition/). Moreover, the prevalence of soil acidity in SSA intuitively indicates that Mg and Ca, even when present in soil, would be unavailable for plant use in such soils. The above studies with both S and Mg involving seedling exposure to the nutrients indicate that pretreatment prior to disease infestation is critical for optimizing disease resistance by acting as a preventative therapy and should be evaluated under the agroecological conditions of SSA. Ample evidence also exists for the involvement of Zn in disease suppression. Prior studies show Zn capable of directly reducing fungal growth in vitro (90). Notably, applications in real-plant-path systems also evince similar outcomes reviewed in (91, 92). Exemplified in this regard is ongoing work by researchers at the Connecticut Agricultural Experiment Station (CAES) in New Haven and collaborators at the University of Central Florida, United States. Here, the soil-borne fungus, Fusarium, is shown to devastate watermelon production under field conditions, whereas the application of zinc (this product is currently under patent application) mitigates the impact, similarly to a commercial fungicide (Figure 4).
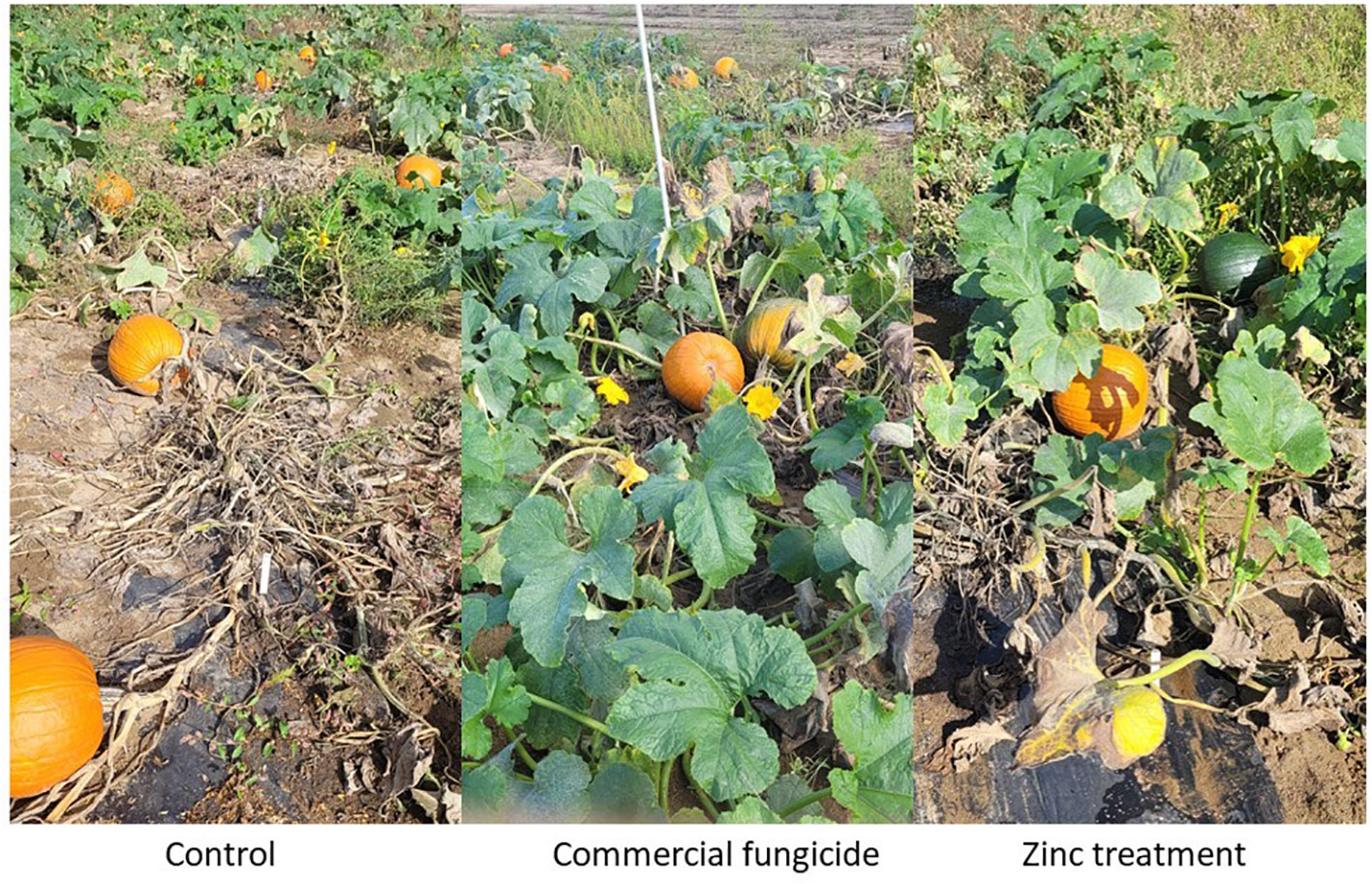
Figure 4 Effect of zinc treatment on watermelon vegetative and reproductive productivity (image courtesy Dr. Wade Elmer, CAES).
Nevertheless, Cu, particularly in its nanoform, has frequently been demonstrated to show higher efficacy than Zn for disease suppression (e.g., 93, 94). Indeed, Cu is an important co-factor for key proteins, including plastocyanin, peroxidases, and multi-Cu oxidases involved in plant response to pathogenic infections. Notably, Cu nutrition not only directly inhibits pathogen growth indicative of the traditional role of Cu as an age-long pesticide, but it also engenders plant systemic resistance against future disease outbreaks, providing a preventive remedy, as noted for Mg as well. Elmer and coworkers (e.g., 93–96) have in multiple studies demonstrated the role of Cu in this regard under greenhouse and field conditions. For example, in watermelon, cucumber, eggplant, and tomato, treatment of Cu significantly reduces disease progression, as indicated by the lowered area under the disease progress curves (AUDPC) of up to 60% in cases. The genes induced in plants in response to disease and Cu were polyphenol oxidase (PPO) and pathogenesis-related gene1 (PR1). Taken together, the Cu effect under the disease condition could translate to increased yield and economic profitability reviewed in (97). It must be noted, however, that use of Cu at higher concentrations as an agrochemical can pose long-term soil health problems in terms of downregulating soil microbial activity and functions (98, 99). Notably, the applied quantities (1-2 ml/plant of a 500 µg/ml stock solution) in the studies by Elmer et al. (93, 94, 96) are relatively small. Moreover, the products are formulated to alter undesirable chemical properties and applied as foliar sprays. As such, minimal, if any, inimical effects on soil health are anticipated. Plant pathogenic burden in the soil causing disease in plants is a soil health problem, as root health is compromised. A compromised root function negates soil-root chemical communications in terms of mutual release and assimilation of organic molecules between plants and plant-associated beneficial microbes. Therefore, fertilization with nutrients involved in maintaining rhizosphere health under disease pressure contributes to sustaining overall soil health.
A separate but related dimension of the non-NPK nutrient effect on plant health is the mitigation of toxic elements in the food system. Several toxic elements are implicated in compromising the quality of soil, plant, and environmental health, including cadmium, lead and arsenic (As). However, worthy of more in-depth discussion in the paper is the potential for the SSA food systems contamination by As, due to reported widespread environmental occurrence at elevated levels in Ethiopia, South Africa and several countries of Western and Eastern Africa (reviewed in 114). As is a carcinogen, capable of causing cancer, heart disease, type 2 diabetes and decreased cognition when consumed in the inorganic form in agricultural produce reviewed by (100). The rice crop has been identified as an efficient absorber and accumulator of As from irrigation water, soil or paddy field reviewed in (101). Notably, rice has also become the most staple food crop consumed by more than half of the population in SSA, with production increasing in the region from over 17 million tons in 2017 to more than 24 million tons in 2021 (https://www.statista.com/statistics/1294234/production-volume-of-rice-in-africa/), led in the order of production by Nigeria, Madagascar, Côte d’Ivoire, Tanzania, Mali, Guinea, Sierra Leone, and Senegal. Regular consumption of rice contaminated with As would, therefore, increase the risk of the above-mentioned ailments IN SSA. Furthermore, As contamination in soil has been associated with low rice crop yield and productivity of paddies, in addition to increased crop grain contamination (reviewed for e.g., in 102). This raises the risk of crop failure and unsustainable farming in SSA. Accordingly, reducing As exposure in rice have become a very major concern for the scientific community, to address both ecosystem health and crop production. In that context, several studies show the effective mitigation of arsenic effect on crop yield and/or accumulation of As in rice by nano nutrients, including nano Fe, nano Cu, and nano Zn (103–105). Mechanistically, these nano-scale nutrients mitigate the accumulation of As into plants by adsorbing and sequestering inorganic forms of the toxic element in soil, thereby reducing their mobility (106). The availability of local resources in SSA to design nanosystems for addressing As removal from the environment was recently articulated (107).
Taken together, these studies clearly indicate that the mitigation of drought, salinity, disease stresses, arsenic bioavailability and effect on crop production with a nutritional strategy based on nanotechnology is a good practice for both soil and human health and food security that should be adapted and adopted for SSA, where these environmental issues are commonplace. Notably, some of these studies did not rigorously compare nano nutrients with their conventional counterparts to establish the basis for adoption and commercialization of nano-scale solutions for addressing the above discussed environmental stresses in SSA. This, therefore, is an aspect that must be included in any valorization in SSA.
5 Micronutrients for biofortification towards human nutrition security and soil health
Perhaps critically important for Fe and Zn is their role in the agronomic fortification of food to address human health challenges. Functionally, these nutrients dictate key aspects of human health related to general well-being and cognitive development and are discussed in the millennium/sustainable development goals’ realm, in the context of hidden hunger and its socio-economic implications at the individual, continental, and global levels (e.g., 108, 109). The term hidden hunger describes the immediately indiscernible deficiency of micronutrients in human diets that leads to long-term future effects on human health. Micronutrients are naturally present in agricultural soils. However, they are highly deficient in many soils in SSA. In particular, Zn deficiency in SSA soils has been linked to human Zn deficiency, arising from the endemic consumption of staple crops grown in Zn-deficient soils (reviewed for e.g., in 29). A study (110) focused specifically on Ethiopia and using soil Zn map and the country’s national micronutrient survey, show a clear correlation among soil Zn, human serum Zn deficiency, and child nutritional status. In other SSA countries, human Zn deficiency ranges from 24% in Nigeria, 51% in Kenya, 66% in Zambia, and 28% in Ghana, indicating significant continental-scale human health ramifications (111, 112). However, the addition of Zn as fertilizers to cropped soils can increase the Zn contents of aerial plant tissues, warranting an agronomic fortification approach to address micronutrient-induced human health challenges. Notably, Zn is relatively immobile within plants due to its positive charge that lends to the tendency to be bound up by negatively charged ligands, including proteins, enzymes, organic acids, and biochelators (113–115).
While increased plant shoot Zn content directly improve the nutritional quality of leafy vegetables for human consumption, Zn translocation to edible grains and seeds can present a different scenario than shoot uptake. In some cases, micronutrients can be efficiently translocated to the grain/seed, while it is not so much so in other cases. An example of differential efficiency translocation of micronutrients to the grain can be demonstrated with Zn in three different crops, namely soybean, sorghum and wheat (Table 1). Regardless of the differences, the overall data show that the Zn amendment in soil can strongly improve the Zn nutritional quality of different crops. In that regard, grain Zn fortification via fertilization represents a significant nutritional outcome for critical populations that depend on grain stapes for meeting their Zn dietary needs.

Table 1 Relationship between Zn application (soil) rate and grain Zn in different crops (data adapted from Dimkpa et al., 37- 39).
Nutrient deficiencies in human diets, including those of Zn and Fe, are being exacerbated by climate change events such as water deficiency (116). Data from a previous study (34) is adapted in Table 2 to buttress the contribution of agronomic Zn fortification to human Zn nutrition under drought stress production conditions. Here, drought lowered average sorghum grain Zn concentration by 32%, but fertilization with Zn improved the grain Zn concentration under drought by 94%. Relating these findings to the human-recommended dietary allowance (RDA) for Zn, a 192 g serving of sorghum can supply only about 29% of the daily Zn RDA based on information from Sorghum Facts and Health Benefits (https://www.healthbenefitstimes.com/sorghum/). However, the Zn amendment in the non-drought plants provided ≈ 60% of the daily RDA based on a serving of the sorghum grain. Notably, drought imposition (with no Zn amendment) lowered the daily RDA to 36%. However, with Zn fertilization under drought, Zn RDA increased to between 48 and 52%, depending on the Zn concentration applied (1, 3, and 5 mg Zn/kg soil). Such estimates demonstrate that Zn amendment can mitigate the concerns related to grain Zn quantity in crops produced under drought conditions. Grain Zn fortification via soil fertilization thus represents a significant nutritional outcome for critical populations that depend on grain stapes such as sorghum in the face of persistent climatic events such as drought such as in parts of SSA. A similar estimate can also be generated for Fe; aridity has been described as affecting the availability of Fe to humans (116).
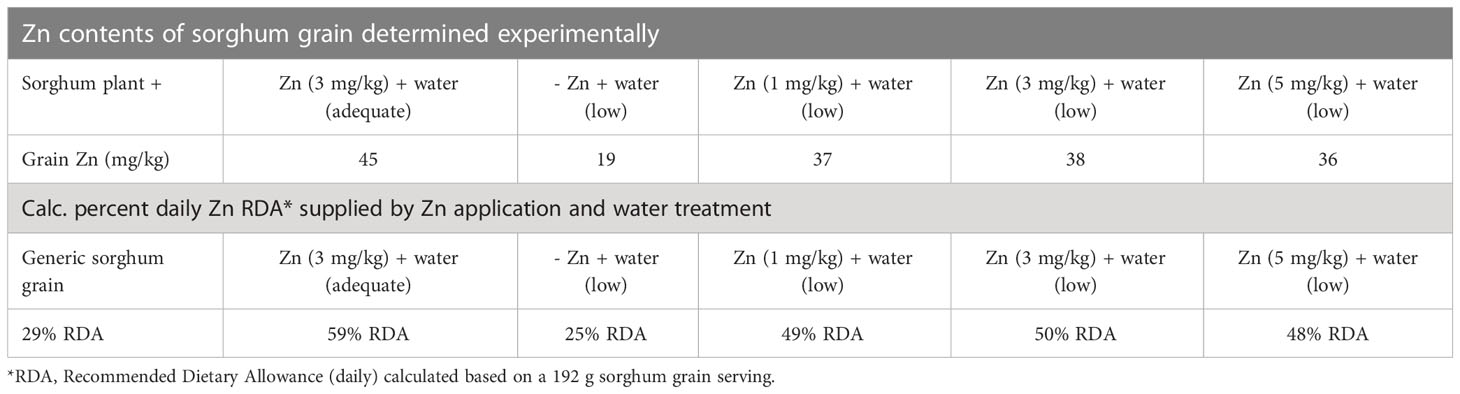
Table 2 Estimation of daily dietary recommendation for Zn in sorghum grain using agronomic fortification under drought stress growth condition (adapted from ref 39).
Despite a lack of Zn in the soils in SSA that results in crop and human Zn deficiencies, there are several large Zn mineral deposits in the Democratic Republic of Congo, Nigeria, Zambia, and Namibia, in addition to even larger deposits along the Moroccan-Algerian boarder, that are currently being mined for use in non-agricultural purposes. To alleviate both agricultural and public health aftermath of soil Zn deficiency, it is pertinent that some of the Zn from these mining activities be deployed to the agricultural sector by way of Zn-fertilizer product development. This then should be dovetailed with the screening of vegetable and seed-bearing crop species or varieties with high shoot Zn uptake and seed translocation efficiencies, respectively, to optimize a new Zn-enabled fertilization regime.
6 Revisiting rhizosphere science for soil health-compliant product development in SSA
The rhizosphere is habitat to billons of living microbes cohabiting within the root (endophytes) for nutrient transfer and outside the root for nutrient recycling for plant uptake. The largest group of soil microbes are bacteria accounting for 75%; fungi (13%) and other groups are less than 10%. The largest microbial contribution of nutrients to plants is from bacteria via converting biological N2 fixation (BNF) from atmosphere into NH3 usable by legume plants (117). The BNF process is arguably one of the most important beneficial roles of soil microbes in agriculture performed by bacteria such as Rhizobia spp., Azospirillum spp., Frankia spp., Azotobacter and vinelandii. As much as 300 kg N2/ha/yr. can be fixed by Rhizobia, depending on the plant, soils, and efficacies of the resident and introduced microbes (58, 59). BNF is environmentally beneficial and allows notable carbon sequestration and soil health maintenance in the short and long run from crop residues decomposition and accumulation in soil (118). Research on BNF in SSA have received considerable attention, with long-term R&D projects such as N2AFRICA, focused on ‘‘putting nitrogen fixation to work for smallholder legume farmers’’ in DR Congo, Ethiopia, Ghana, Kenya, Malawi, Mozambique, Nigeria, Rwanda, Tanzania, and Uganda by identifying compatible rhizobia strains for different leguminous plants. However, the challenges to a successful BNF regimen in Africa include the low adoption by farmers, and how to develop microbial inoculants with stable shelf lives. Other factors critical to BNF adoption are the proper engagement of private sectors for investment on rhizobia inoculant plants and favorable country-scale regulations to support effective product commercialization.
Like BNF, phosphate-solubilizing bacteria (PSB) have been well studied for their ability to solubilize P from rock phosphate with otherwise low solubility that limits direct use in agriculture as fertilizer reviewed in (119, 120). PSBs such as Pseudomonas, Bacillus and Rhizobium, among others, harness their ability to produce and release gluconic acid and/or phosphatases into the soil to solubilize rock phosphate or chemically fixed insoluble phosphate forms. Studies based on tricalcium phosphate show a wide range of P-solubilizing abilities among bacterial species, with Gordonia sp. producing as little as 32 mg/L at pH 6, and Arthrobacter sp. being able to produce as much as 520 mg/L of soluble P at pH 4.9 (121). Most plants require P at<100 mg/kg fertilizer application, suggesting that PSBs such as Arthrobacter could potentially fulfill the required amount of P for plant growth, assuming a strategy to provide and validate them as soil amendments under field conditions. Compared to N and P, less is known about K-solubilizing microbes. However, findings suggest these microbes assimilate K for themselves prior to release to plants, a process that engenders increased soil dehydrogenase enzyme activities because of K nutrition. Dehydrogenase enzyme activity is a measure of microbial oxidative functions in soil that is related to K nutrition and more broadly to soil quality (122, 123). Root-associated microbes, including Pseudomonas and Bacillus species, that solubilize or oxidize S, Zn, Mn and others non-NPK nutrients also have been identified (e.g., 124–126). However, inoculation of plants with root-colonizing bacterium is known to regulate micronutrients uptake by plants from Zn and Cu (127–129). This effect may be related to the competitive binding of these nutrients by microbially produced siderophores and may alleviate nutrient toxicity or worsen nutrient deficiency. When soil microbes orchestrate plant root decay, the resultant organic matter possesses an overwhelming negative charge due to the strong presence of hydroxyls and carboxylic moieties. The acids on the surface of organic matter, thus, offer charge-dependent interactions with soil nutrients and by so doing can modulate the fate and effect of fertilizers through both inducing chemical changes on the fertilizer’s surface and by chelating or complexing nutrient-ions released from the fertilizer. Organic acids on biomasses cause the dissolution of particulate nutrients, as noted for ZnO where particle size decreased due to coating with the acid, resulting in greater Zn bioavailability and uptake by cucumber plants (130). Bean plants similarly accumulated more Zn from ZnO in the presence of organic matter (131), presumably by altering the aggregate size. The chelation of Zn by organic acids is related to the reduction of toxicity of ZnO to a soil-borne bacterium, Pseudomonas putida (132), implying a positive outcome for soil health, given this bacterium is beneficial for plant productivity enhancement. Further, organic acid could increase soil contents of total and available N, P, and K, which could be correlated with heightened expression of key soil health indicators, namely, urease, sucrase, and phosphatase activities, all microbially driven processes Li et al. (133). Similarly, plant uptake of N, P, Fe, Zn, and Mn in the presence of organic acid under field growth conditions increased due to enhanced cation exchange capacity of the soil, gradual release of nutrients, and influence on soil bio-physico-chemical properties (134). These studies imply that a facile functionalization of nutrients with post-harvest biomaterials such as decaying plant residues containing functional organic acids can be used as ‘‘organic’’ and environmentally friendly fertilizer development strategy. This approach is described in several papers (e.g., 135–137) and worthy of evaluation under SSA conditions, as a low-cost, locally sourced approach to addressing soil fertility problems.
The trace elements Cu, Fe, Mn, Mo, Ni, Zn function in soil microbial metabolisms that support soil health via enhancing soil enzyme activities and maintaining a health soil microbial community. Many of the roles of rhizosphere microbes in nutrient cycling, organic matter degradation, decomposition of biodegradable industrial wastes, and production of secondary metabolites with plant functions are driven by enzymes having Cu, Fe, Mn, Mo, Ni, or Zn as a co-factor. Some of these nutrients are already being evaluated in SSA in the context of addressing the ‘‘non-responsiveness’’ of soils to NPK (e.g., 23, 53, 138, 139). However, the mechanisms by which they act in the soil have not been well interrogated in the SSA context, with the goal of fertilizer product development. Several microbes are known to mobilize and avail plants of nutrients, including N, P, K, Fe, S, Mn, and Zn, using a variety of mechanisms (reviewed for e.g., in 140–142). These bacteria, fungi, or by proxy their exudates, such as siderophores and organic acids, can thus, influence the fate and effects of applied fertilizers in the rhizosphere; they regulate nutrients levels by remodeling nutrient release via complexation, solubilization, reduction or oxidation (e.g., 143–146).
Another application of soil microbes is in their use for bioremediation of heavy metal contaminated soil that affect soil and food quality. In this respect, endophytic microbes are receiving growing attention as successful, low-cost, and long-term tools for the management of chemical contaminants in the environment. Because of their nature, bacteria often spend most of their asymptomatic life cycle within the tissue of plants, causing harm to the host (147). To circumvent the metal stress, endophytic bacteria have evolved several types of mechanisms, through which they alleviate the toxicity of metal ions. These mechanisms include the efflux of metal ions to the exterior to the cell, the transformation of metal ions to less toxic forms, the sequestration of metals on the cell surface or in intracellular polymers, and precipitation, adsorption/desorption, or biomethylation (148). Similarly, the ability to develop tiny accumulates in various locations of plant tissues where nutrients are transferred, induce the metabolic changes from host plant and microbes and offering potential to improve plant growth and health (149). Mining activities is bourgeoning in many SSA countries and heavy metal wastes will become an additional source of pollution, reducing soil and human health quality.
7 Perspectives on implications of mineral fertilization on soil health, and ways forward for SSA
As discussed in greater detail (150) and reiterated in the foregoing, agricultural production in SSA must increase substantially in the coming decades if the continent must sustainably feed its growing population. A prior estimate placed such food production increase at 120% (139). Large swaths of the continent are faced with problems of soil acidity, aridity, salinity, drought, and a variety of biotic stresses. Therefore, it is important that achieving such a leap in food production should come with no further damage to pristine ecosystems through the intensification of agricultural production, rather than through extensification via increasing the land hectarage under cultivation. Agrochemicals such as fertilizers are critical inputs for agricultural intensification. Notably, balancing the macro and micronutrients in fertilizers could further increase productivity and lower crop yield gaps under certain SSA soil conditions (32, 151). However, there is no doubt that fertilizer over-application has negative consequences for soil and environmental health. Elrys et al. (65) estimated that the plant derived N footprint proportion in SSA will be 72%, assuming all farms used fertilizers, and 44% if no farm used fertilizers. The increased application of N fertilizer leads to significant increase in nitrous oxide emissions (152, 153) especially through nitrification and denitrification processes. Globally, the supply chain of mineral N fertilizer was responsible for a greenhouse gas emission of about 1129.1 Mt CO2e in 2018 with China, India, USA and EU28 accounting for 62% of the total (154). This is made up of 38.8% 58.6% and 2.6% emissions associated with production, fertilizer use in agriculture, and transportation, respectively. While Africa contributes only about 1% to the global N emissions, East-Asia led by China, and Latin America led by Brazil, contributes 29% and 22%, respectively (154).
Nitrate and phosphate leaching into groundwater causes undesirable hazards for biological systems. Ammonia volatilization from urea-N has human health implications, and as noted above nitrous oxide emission is a source of greenhouse gas that contributes to climate change. Fertilizers in general cause short- and long-term, sometimes negative, shifts in microbial population and diversity; and micronutrients such as Cu used at high levels as agrochemicals are toxic to soil biota reviewed in (155, 156). These negative effects, while mostly not being directly of great implications to SSA due to traditional agrochemicals underapplication on the continent, warrants be noted as a preventative measure against the experiences of North America and Asian countries where fertilizer overdose is of major concern. To this end, researchers in advanced agricultural regions are continuously working to improve fertilizer use efficiency via the development of enhanced efficiency fertilizers (EEFs) that are balanced in their composition, functionalized on their surface or in their core to promote regulated (slow or controlled) release of the nutrients, and tunable to respond to different soil chemistries such as acidity and alkalinity and specific crop needs (61, 157, 158). It can, thus, be said that while increasing the rate of mineral fertilizers use to achieve the desired food security objectives is being advocated for SSA, the eventual amount would depend on advances made in developing EEFs that permit the use of lower rates of nutrients with higher plant uptake, but without compromising crop yield and other fertilizer related ecosystem functions. Still, such products must be affordable to SSA farmers, preferably be produced locally, and be designed with soil health enhancement in mind, such as combining organic and inorganic options. To achieve this implies that SSA must expand its R&D efforts towards EEFs to address both the agronomic and environmental implications of the anticipated fertilizer use intensification that must come with increased food production. EEFs developed via nanotechnology-, microbial (e.g., NFBs and PSBs), and biomass -based formulations, or their combinations thereof can allow for using lower nutrient application rates. In addition to avoiding immediate wastage, lower application rates invariably reduce the long-term mineral fertilizer environmental footprint and the need for constant inputs of new mineral resources into the ecosystem. Furthermore, it can reduce the cost of agrochemical input on the farmer, thereby increasing farm profit margins. Such benefits typically come with no loss in yield loss as shown in field studies of maize with Zn fertilizers (e.g., 159). The judicious use of EEFs would also support several positive outcomes for fertilizers at the plant-soil level. They increase plant biomass which helps with carbon sequestration. They positively remodel soil enzyme activities due to the inclusion of elements that are co-factors of important rhizosphere functioning enzymes. They help to reduce plant pathogenic disease progression in soil, and to mitigate abiotic stress effects on plant productivity. Such improvements, accompanied by reduced field application rates, allow for an increase nutrient uptake by plants, reduce run-off and leaching, and thus contribute to improving overall soil health. For SSA, the promise of EEFs can be fast-tracked by harnessing the abundant mineral resources to promote a home-grown fertilizer technology that is farmer centric. Indeed, SSA needs to look inward for fertilizer solutions using local materials. The existing phosphate technology can be modified to cater for small and medium-scale production, where for example, the abundantly available insoluble rock phosphate is formulated with water-soluble P to generate hybrid P products with phased plant availability. The abundantly available agro-wastes from discarded inedible plant materials and sea food wastes can be used to generate high-value biomaterials such as nanocellulose and chitosan for use in developing EEFs. Biochar production and functionalization can be pursued both as a source of nutrients and for formulating high-value EEFs, whereas novel mineral resources such as pyrite (FeS2) can be harnessed as nutrients (160–163). The mineral pyrite (FeS2) has a significant presence in Southern and Central Africa; and facile nanotechnology based on ball-milling can generate nanoscale pyrite for use as a fertilizer. This mineral has been shown in studies from India to generate an ‘‘NPK-equivalent’’ nutritional effect in rice that may permit less use of NPK fertilizer (164). Such findings should be valorized under African rice production systems. Recycling and repurposing other wastes such as tripolyphosphate in wastewater to generate P; and farm-scale N fertilizer plants (Haber-Bosch process on the farm (124); to produce N on-farm, can contribute to reducing the risks associated with fertilizer importation from outside the continent. In pursuit of these advances, the potential for incorporating biomaterials and leveraging the chemistry of novel minerals for repurposing as fertilizers must be assessed, to avoid compromising native rhizosphere functions which can deteriorate soil health. Overall, the advancement of home-grown solutions for the SSA region can optimally ensure sustainability in increasing fertilizer use efficiency mindful of maintaining soil health and quality. Notably, a Pan African working group on nanotechnology application in several applications including agriculture was recently set up, comprising of scientists from Mauritius, South Africa, Botswana, Tunisia, Egypt, Nigeria and several other SSA countries. Coordinated by the United Nations Economic Commission for Africa (UNECA), the main objectives of the Working Group are to (i) set up a consortium of Pan-African experts who would partner in the mounting and delivery of a nanotech program at Masters level; (ii) identify areas of strength in nanotech focusing on resources and raw materials, capacity of production/manufacturing, and markets; and (iii) develop nanotechnology research and technology clusters across Africa. Achieving these objectives would provide an opportunity for addressing public good (e.g., environmental, agricultural, human health and resource use) using nanoscience and technology tools.
Evidently, harnessing technology alone cannot solve the food insecurity and poor soil health problem of SSA. Field-based rice studies in Uganda indicate that good agricultural practices with a goal towards intensification, such as timely weed and water management, in conjunction with fertilizer application, can close the huge crop yield gaps in SSA (139). Therefore, in addition to a sound fertilizer regime, good crop production activities or farm management practices are crucial in improving crop yield and soil health. Soil management is critical for improving several ecosystem services including the regulation of water flow, regulation of biodiversity and greenhouse gas emissions (165). Farm management practices including crop diversity, low mechanical disturbance of soil and use of organic amendments improves soil health indicators particularly the aggregate stability and autoclaved-citrate extractable soil protein of the soil (166). The incorporation of cover crops into crop rotation system helps in improving nitrogen recycling by lowering the risk of nitrate leaching, improvement in the soil carbon levels, and weed suppression, while also, soil amendments are critical in enhancing the three properties- physical, chemical, and biological -of the soil (167). Again, conservative farming involving mulching, reduced tillage and crop rotation significantly improves not only the soil organic carbon content of the soil and allowing improved inputs of roots but also, other soil parameters including pH, cation exchange capacity, and potential nutrification rates becomes well improved (168). Soil structure, water infiltration, soil evaporation and weed management are improved under conservative farming practices. According to studies in Zimbabwe by Mashavakure et al. (169), minimum tillage with reduced plant residue retention improves biodiversity and its associated biological benefits. Farm management practices also play significant roles in the fertilizer use efficiency as they provide a conducive environment for the fertilizers to exhibit their full potential. This is evident in Adzawla et al. (170) where mulched farms had higher fertilizer use efficiency than non-mulched farms, and in Adzawla and Alhassan (171) where the production of maize under crop rotation and row planting significantly improves the production efficiency of the farmers. The adoption of sound production practices is therefore vital for sustainable food production in SSA. Notably, a new impetus on conserving soil organic matter to reverse the low levels of carbon (C) sequestered in SSA soils is being provided (172). In this respect, proper use of the available fertilizer increases both above and below ground plant biomasses, thereby enhancing the sequestration of additional C in soils, and, thus, contributing to SSA’s response to climate change mitigation. This is a positive development, as SSA holds great promise as a major player in addressing climate change. While continued deforestation of the continent will increase CO2 released into the atmosphere, proper management of agro ecosystems for sustainable agricultural intensification, including use of fertilizers, will reduce the content of CO2 in the atmosphere and increase CO2 sequestration in soils. Improved fertilizer use in SSA by harnessing technologies will undoubtedly make it an important contributor to the fight against climate change.
Author contributions
CD, WA and RP were involved in conceptualization, original drafting of manuscript and validation. WKA, and AKK provided resources. MJ was involved in validation. PSB was involved in conceptualization, validation and supervision. All authors contributed to the article and approved the submitted version.
Acknowledgment
Many thanks to the OCP and the Mohammed VI Polytechnic University (UM6P) Morocco, for funds provided for the Fertilizer Research and Responsible Implementation (FERARI) project in support of this paper.
Conflict of interest
The authors declare that the research was conducted in the absence of any commercial or financial relationships that could be construed as a potential conflict of interest.
Publisher’s note
All claims expressed in this article are solely those of the authors and do not necessarily represent those of their affiliated organizations, or those of the publisher, the editors and the reviewers. Any product that may be evaluated in this article, or claim that may be made by its manufacturer, is not guaranteed or endorsed by the publisher.
References
1. FAO. The state of food security and nutrition in the world: Transforming food systems for food security, improved nutrition and affordable healthy diets for all. Rome: FAO (2021).
2. FAO. The future of food and agriculture – trends and challenges. Rome: FAO (2017). Available at: https://www.fao.org/3/i6583e/i6583e.pdf.
3. Derbile EK, Bonye SZ, Yiridomoh GY. Mapping vulnerability of smallholder agriculture in Africa: Vulnerability assessment of food crop farming and climate change adaptation in Ghana. Environ Challenges (2022) 8:100537. doi: 10.1016/j.envc.2022.100537
4. Kummu M, Heino M, Taka M, Varis O, Viviroli D. Climate change risks pushing one-third of global food production outside the safe climatic space. One Earth (2021) 4(5):720–9. doi: 10.1016/j.oneear.2021.04.017
5. Dhanarajan A. Sustainable agriculture towards food security. In: Sustainable agriculture towards food security. Singapore: Springer Nature Singapore Pte Ltd (2017). doi: 10.1007/978-981-10-6647-4
6. FAO, IFAD, UNICEF, WFP, WHO. The state of food security and nutrition in the world 2020. In: Transforming food systems for affordable healthy diets. Rome: FAO (2020). doi: 10.4060/ca9692en
7. Mustafa MA, Mabhaudhi T, Massawe F. Building a resilient and sustainable food system in a changing world – a case for climate-smart and nutrient dense crops. In: Glob food security (2021) 28:100477. doi: 10.1016/j.gfs.2020.100477
8. Gomiero T. Soil degradation, land scarcity and food security: Reviewing a complex challenge. Sustain. (2016) 8(3):1–41. doi: 10.3390/su8030281
9. Bindraban PS, van der Velde M, Ye L, van den Berg M, Materechera S, Kiba DI, et al. Assessing the impact of soil degradation on food production. Curr Opin Environ Sustain (2012) 4(5):478–88. doi: 10.1016/j.cosust.2012.09.015
10. Mansourian S, Berrahmouni N. Review of forest and landscape restoration in Africa. Rome, Italy: Accra. FAO and AUDA-NEPAD (2021). doi: 10.4060/cb6111en
11. Wassie SB. Natural resource degradation tendencies in Ethiopia: a review. Environ Syst Res (2020) 9(1):1–29. doi: 10.1186/s40068-020-00194-1
12. Gomiero T. Soil degradation, land scarcity and food security: Reviewing a complex challenge. Sustainability (2016) 8(3):1–41. doi: 10.3390/su8030281
13. Smaling E. The balance may look fine when there is nothing you can mine: nutrient stocks and flows in west African soils. In: Use of phosphate rock for sustainable agriculture in West africa. proceedings of a seminar on the use of local mineral resources for sustainable agriculture in West Africa, held at IFDC-Africa. Ghana: IFDC (1994). p. 21–3.
14. Lehmann J, Bossio DA, Kögel-Knabner I, Rillig MC. The concept and future prospects of soil health. Nat Rev Earth Environ (2020) 1:544–53. doi: 10.1038/s43017-020-0080-8
15. Rickson RJ, Deeks LK, Graves A, Harris JAH, Kibblewhite MG, Sakrabani R. Input constraints to food production: the impact of soil degradation. Food Secur (2015) 7(2):351–64. doi: 10.1007/s12571-015-0437-x
16. Bakker MM, Govers G, Rounsevell MDA. The crop productivity-erosion relationship: An analysis based on experimental work. Catena (2004) 57(1):55–76. doi: 10.1016/j.catena.2003.07.002
17. Pozza LE, Field DJ. The science of soil security and food security. Soil Secur (2020) 1. doi: 10.1016/j.soisec.2020.100002
18. Ghosh M, Devi A. Assessment of crop growth, soil properties and crop yield in an upland acidic soil with inorganic fertilizer blended with organic amendments in summer rice cropping seasons. Int J Recycl Org Waste Agric (2019) 8(s1):1–9. doi: 10.1007/s40093-019-0252-z
19. Zhao J, Ni T, Li J, Lu Q, Fang Z, Huang Q, et al. Effects of organic-inorganic compound fertilizer with reduced chemical fertilizer application on crop yields, soil biological activity and bacterial community structure in a rice-wheat cropping system. Appl Soil Ecol (2016) 99:1–12. doi: 10.1016/j.apsoil.2015.11.006
20. Jiang Y, Zhang J, Manuel DB, Op de Beeck M, Shahbaz M, Chen Y, et al. Rotation cropping and organic fertilizer jointly promote soil health and crop production. J Environ Manage (2022) 315:1–3. doi: 10.1016/j.jenvman.2022.115190
21. Trasar-Cepeda C, Leirós MC, Labandeira SS, Gil-Sotres F. Limitation of soil enzymes as indicators of soil pollution. soil biol biochem. (2000) 32:1867–75. doi: 10.1016/S0038-0717(00)00160-7
22. Butler BM, Palarea-Albaladejo J, Shepherd KD, Nyambura KM, Towett EK, Sila AM, et al. Mineral-nutrient relationships in African soils assessed using cluster analysis of X-ray powder diffraction patterns and compositional methods. Geoderma. (2020) 375:114474. doi: 10.1016/j.geoderma.2020.114474
23. Kihara J, Sileshi GW, Nziguheba G, Kinyua M, Zingore S, Sommer R. Application of secondary nutrients and micronutrients increases crop yields in sub-Saharan Africa. Agron Sustain Dev (2017) 37(4). doi: 10.1007/s13593-017-0431-0
24. Jones DL, Cross P, Withers PJA, DeLuca TH, Robinson DA, Quilliam RS, et al. Review: nutrient stripping: the global disparity between food security and soil nutrient stocks. J Appl Ecol (2013) 50:851–62. doi: 10.1111/1365-2664.12089
25. Dimkpa C, Bindraban P, McLean JE, Gatere L, Singh U, Hellums D. Methods for rapid testing of plant and soil nutrients. In: Lichtfouse E, editor. Sustainable agriculture reviews. Switzerland: Springer International Publishers (2017). doi: 10.1007/978-3-319-58679-3_1
26. Wortmann S, Esilaba C O, Kaizzi A C, Kibunja K, Magario C,W, Maman K,N. Fertilizer use issues for smallholder agriculture in tropical Africa. In: Sustainable crop production. UK: IntechOpen Limited (2020). doi: 10.5772/intechopen.89040
27. Bayu W, Rethman NFG, Hammes PS. The role of animal manure in sustainable soil fertility management in sub-Saharan Africa: A review. J Sustain Agric (2005) 25:2:113–36. doi: 10.1300/J064v25n02_09
28. Magnone D, Bouwman AF, van der Zee SE, Sattari SZ, Beusen AH. Efficiency of phosphorus resource use in Africa as defined by soil chemistry and the impact on crop production. Energy Proc (2017) 123:97–104. doi: 10.1016/j.egypro.2017.07.264
29. Sheahan M, Barrett C. Ten striking facts about agricultural input use in Sub-Saharan Africa. Food Policy (2017) 67:12–25. doi: 10.1016/j.foodpol.2016.09.010
30. Vanlauwe B, Bationo A, Chianu J, Giller KE, Merckx R, Mokwunye U, et al. Integrated soil fertility management: operational definition and consequences for implementation and dissemination. Outlook Agr (2010) 39:17–24. doi: 10.5367/000000010791169998
31. Ichami SM, Shepherd KD, Sila AM, Stoorvogel JJ, Hoffland E. Fertilizer response and nitrogen use efficiency in African smallholder maize farms. Nutr Cycl. Agroecosyst. (2019) 113:1–19. doi: 10.1007/s10705-018-9958-y
32. Dimkpa C, Bindraban P, McLean JE, Gatere L, Singh U, Hellums D. Methods for rapid testing of plant and soil nutrients. In: Lichtfouse E, editor. Sustainable agriculture reviews. Switzerland: Springer International Publishers (2017). doi: 10.1007/978-3-319-58679-3_1
33. Dimkpa CO, Singh U, Bindraban PS, Elmer WH, Gardea-Torresdey JL, White JC. Exposure to weathered and fresh nanoparticle and ionic zn in soil promotes grain yield and modulates nutrient acquisition in wheat (Triticum aestivum l.). J Agric Food Chem (2018) 66:9645–56. doi: 10.1021/acs.jafc.8b03840
34. Dimkpa CO, Singh U, Bindraban PS, Elmer WH, Gardea-Torresdey JL, White JC. Zinc oxide nanoparticles alleviate drought-induced alterations in sorghum performance, nutrient acquisition, and grain fortification. SciTotal Environ (2019) 688:926–34. doi: 10.1016/j.scitotenv.2019.06.392
35. Sanabria J, Dimithe G, Alognikou EKM. The quality of fertilizer traded in West Africa: Evidence for stronger control. Muscle Shoals, AL: IFDC (2013). Available at: https://ifdc.org/research-papers-and-studies/.
36. Sanabria J, Ariga J, Fugice J, Mose D. Fertilizer quality assessment in markets of uganda. IFDC report. Muscle Shoals, AL: IFDC (2018). p. 2018. Available at: https://ifdc.org/Uganda-Fertilizer-Quality-Assessment.
37. Ngogang MP, Ernest T, Kariuki J, Mouiche MM, Ngogang J, Wade A, et al. Microbial contamination of chicken litter manure and antimicrobial resistance threat in an urban area setting in Cameroon. Antibiotics (2020) 10(1):20. doi: 10.3390/antibiotics10010020
38. Kyakuwaire M, Olupot G, Amoding A, Nkedi-Kizza P, Basamba TA. How safe is chicken litter for land application as an organic fertilizer? a review. Int J Environ Res Public Health (2019) 16(19):3521. doi: 10.3390/ijerph16193521
39. Sattari SZ, Bouwman AF, Giller KE, van Ittersum MK. Residual soil phosphorus as the missing piece in the global phosphorus crisis puzzle. PNAS (2012) 109:6348–54. doi: 10.1073/pnas.1113675109
40. Ciceri D, Allanore A. Local fertilizers to achieve food self-sufficiency in Africa. Sci Total Environ (2019) 648:669–80. doi: 10.1016/j.scitotenv.2018.08.154
41. Agegnehu G, Amede T, Erkossa T, Yirga C, Henry C, Tyler R, et al. Extent and management of acid soils for sustainable crop production system in the tropical agroecosystems: a review. Acta Agric Scand B — Soil Plant Sci (2021) 71:9:852–69. doi: 10.1080/09064710.2021.1954239
42. De Pauw E. The management of acid soils in Africa. Outlook Agric (1994) 23:11–6. doi: 10.1177/003072709402300104
43. Margenot AJ, Singh BR, Rao IM, Sommer R. Phosphorus fertilization and management in soils of Sub-Saharan Africa (2016). Available at: https://margenot.cropsciences.illinois.edu/wp-content/uploads/2018/12/phosphorus-fertilization-and-management-in-soils-of-sub-saharan-africa.pdf.
44. Okalebo JR, Othieno CO, Nekesa AO, Ndungu-Magiroi KW, KifukoKoech MN. Potential for agricultural lime on improved soil health and agricultural production in Kenya. Afr Crop Sci J. (2009). p. 339–41.
45. Angle SJ, Singh U, Dimkpa CO, Bindraban PS, Hellum DT. Role of ffertilizers for climate-resilient agriculture. Proc Int Fertiliser Soc (2017) 802:44.
46. Zheng J, Kilasara MM, Mmari WN, Funakawa S. Ammonia volatilization following urea application at maize fields in the East African highlands with different soil properties. Biol Fertil Soils (2018) 54:411–22. doi: 10.1007/s00374-018-1270-0
47. Lemarpe SE, Musafiri CM, Macharia JM, Kiboi MN, Ng’etich OK, Shisanya CA, et al. Nitrous oxide emissions from smallholders’ cropping systems in Sub-Saharan Africa. Adv Agric (2021) 2021:13. doi: 10.1155/2021/4800527
48. Nyenje PM, Foppen JW, Uhlenbrook S, Kulabako A. Muwanga, eutrophication and nutrient release in urban areas of sub-Saharan Africa — a review. Sci Total Environ (2010) 408:447–55. doi: 10.1016/j.scitotenv.2009.10.020
49. Solomon D, Lehmann J, Fraser JA, Leach M, Amanor K, Frausin V, et al. Indigenous African soil enrichment as a climate- smart sustainable agriculture alternative. Front Ecol Environ (2016) 14(2):71–6. doi: 10.1002/fee.1226
50. Garcia L, Dubeux JCB, Sollenberger LE, Vendramini JMB, DiLorenzo N, Santos ERS, et al. Nutrient excretion from cattle grazingnitrogen-fertilized grass or grass–legume pastures. J Agron (2021) 113:1–14. doi: 10.1002/agj2.20675
51. Druilhe Z, Barreiro-Hurlé J. Fertilizer subsidies in sub-Saharan Africa ESA working paper no. 12. In: Agricultural development economics division food and agriculture organization of the united nations. Rome, Italy: FAO (2012). Available at: www.fao.org/economic/es.
52. Bindraban PS, Kouame AKK, Kissiedu IN, Atakora WK, El Mejahed K. Identifying drivers of variability of maize yield in Ghana. (2022). doi: 10.2139/ssrn.4093525
53. Kihara J, Bolo P, Kinyua M, Rurinda J, Piikki K. Micronutrient deficiencies in African soils and the human nutritional nexus: opportunities with staple crops. Environ Geochem Health (2020) 42(9):3015–33. doi: 10.1007/s10653-019-00499-w
54. Steffan JJ, Brevik EC, Burgess LC, Cerdà A. The effect of soil on human health: an overview. Eur J Soil Sci (2018) 69(1):159–71. doi: 10.1111/ejss.12451
55. Ray K, Banerjee H, Dutta S, Sarkar S, Murrell TS, Singh VK, et al. Macronutrient management effects on nutrient accumulation, partitioning, remobilization, and yield of hybrid maize cultivars. Fron Plant Sci (2020) 11:1307. doi: 10.3389/fpls.2020.01307
56. Ain NU, Naveed M, Hussain A, Mumtaz MZ, Rafique M, Bashir MA, et al. Impact of coating of urea with bacillus-augmented zinc oxide on wheat grown under salinity stress. Plants (2020) 9(1375). doi: 10.3390/plants9101375
57. Elrys AS, Metwally MS, Raza S, Alnaimy MA, Shaheen SM, Chen Z, et al. How much nitrogen does Africa need to feed itself by 2050? J Environ Manage (2020) 268:(110488). doi: 10.1016/j.jenvman.2020.110488
58. Bojtor C, Mousavi SMN, Illés Á., Golzardi F, Széles A, Szabó A, et al. Nutrient composition analysis of maize hybrids affected by different nitrogen fertilisation systems. Plants (2022) 11(12). doi: 10.3390/plants11121593
59. Essel B, Abaidoo RC, Opoku A, Ewusi-Mensah N. Economically optimal rate for nutrient application to maize in the semi-deciduous forest zone of Ghana. J Soil Sci Plant Nutr (2020) 20(4):1703–13. doi: 10.1007/s42729-020-00240-y
60. Ten Berge HFM, Hijbeek R, van Loon MP, Rurinda J, Tesfaye K, Zingore S, et al. Maize crop nutrient input requirements for food security in sub-Saharan Africa. Glo Food Sec (2019) 23:9–21. doi: 10.1016/j.gfs.2019.02.001
61. Dimkpa CO, Fugice J, Singh U, Lewis T. Development of fertilizers for enhanced nitrogen use efficiency – trends and perspectives. Sci Total Environ (2020) 731:139113. doi: 10.1016/j.scitotenv.2020.139113
62. Masso C, Baijukya F, Ebanyat P, Bouaziz S, Wendt J, Bekunda M, et al. Dilemma of nitrogen management for future food security in sub-Saharan Africa – a review. Soil Res (2017) 55:425–34. doi: 10.1071/SR16332
63. Magnone D, Niasar VJ, Bouwman AF, Beusen AHW, van der Zee S. E. A. T. M., Sattari SZ. The impact of phosphorus on projected Sub-Saharan Africa food security futures. Nat Commun (2022) 13(1). doi: 10.1038/s41467-022-33900-x
64. Elrys AS, Desoky EM, Alnaimy MA, Zhang H, Zhang J, Cai Z, et al. The food nitrogen footprint for African countries under fertilized and unfertilized farms. J Environ Manage (2021) 279:(111599). doi: 10.1016/j.jenvman.2020.111599
65. Elrys AS, Abdel-fattah MK, Raza S, Chen Z, Zhou J. Spatial trends in the nitrogen budget of the African agro-food system over the past five decades. Environ Rese Lett (2019) 14(124091). doi: 10.1088/1748-9326/ab5d9e
66. Rurinda J, Zingore S, Jibrin JM, Balemi T, Masuki K, Andersson JA, et al. Science-based decision support for formulating crop fertilizer recommendations in sub-Saharan Africa. Agric Syst (2020) 180:102790. doi: 10.1016/j.agsy.2020.102790
67. He M, Dijkstra FA. Drought effect on plant nitrogen and phosphorus: a meta-analysis. New Phytol (2014) 204:924–31. doi: 10.1111/nph.12952
68. Ristaino J, Anderson P, Bebber D, Brauman K, Cunniffe N, Fedoroff N. The persistent threat of emerging plant disease pandemics to global food security. Proc Natl Acad Sci (2021) 118:e2022239118. doi: 10.1073/pnas.2022239118
69. Villiers MC, Nell JP, Barnard RO, Henning A. Salt-affected soils: South africa. report number: FAO contract No.PR 26897. ISCW project GW/56/003/20. Rome, Italy: FAO (2003). doi: 10.13140/2.1.2529.8722.
70. Semida WM, Abdelkhalik A, Mohamed GF, Abd El-Mageed TA, Abd El-Mageed SA, Rady MM, et al. Foliar application of zinc oxide nanoparticles promotes drought stress tolerance in eggplant (Solanum melongena l.). Plants (2021) 10:421. doi: 10.3390/plants10020421
71. Song C, Yan Y, Rosado A, Zhang Z, Castellarin SD. And accumulation of zinc in grapevine (Vitis vinifera l.) by inducing expression of ZIP and detoxification-related genes. Fron Plant Sci (2019) 10:872. doi: 10.3389/fpls.2019.00872
72. Bharath P, Gahir S, Raghavendr AS. Abscisic acid-induced stomatal closure: an important component of plant defense against abiotic and biotic stress. Fron Plant Sci (2021) 12:615114. doi: 10.3389/fpls.2021.615114
73. Ayyar S, Appavoo S, Basker M, Pandiyarajan P, Kavimani R. Effect of zinc and microbial inoculation on soil enzyme activities for maize (Zea mays l.) in black soil. Int J Curr Microbiol Appl Sci (2019) 8:1804–14. doi: 10.20546/ijcmas.2019.808.213
74. Kumar A, Rana KS, Choudhary AK, Bana RS, Sharma VK, Rajpoot SK, et al. Sole- or dual-crop basis residue mulching and zn fertilization lead to improved productivity, rhizo-modulation and soil health in zero-tilled pigeonpea–wheat cropping system. J Soil Sci Plant Nutr (2022) 22:1193–214. doi: 10.1007/s42729-021-00723-6
75. Cao X, Wahbi A, Ma L, Li B, Yang Y. Immobilization of zn, Cu, and Pb in contaminated soils using phosphate rock and phosphoric acid. J Hazard Mater (2009) 164:555–64. doi: 10.1016/j.jhazmat.2008.08.034
76. Dimkpa CO, Singh U, Adisa IO, Bindraban PS, Elmer WH, Gardea-Torresdey JL, et al. Effects of manganese nanoparticle exposure on nutrient acquisition in wheat (Triticum aestivum l.). Agronomy (2018) 8:158. doi: 10.3390/agronomy8090158
77. Tamatamah R, Hecky R, Duthie H. The atmospheric deposition of phosphorus in lake Victoria (East Africa). Biogeochemistry (2005) 73:325–44. doi: 10.1007/s10533-004-0196-9
78. Stewart J, Hansen T, Mclean JE, Mcmanus P, Das S, Britt DW, et al. Salts affect the interaction of ZnO or CuO nanoparticles with wheat. Environ Toxicol Chem (2015) 34:(9). doi: 10.1002/etc.3037
79. Kayode OT, Aizebeokhai A, Odukoya AM. Soil salinity and its implications on sustainable agriculture in southern and northcentral states of Nigeria. IOP Conf Series: Earth Environ Sci (2021) 655:12077. doi: 10.1088/1755-1315/655/1/012077
80. El-Fouly M, Abou El-Nour E. Foliar feeding with micronutrients to overcome adverse salinity effects on growth and nutrients uptake of bean (Phaseolus vulgaris). Egypt J Agron (2021) 43(1):1–12. doi: 10.21608/agro.2021.49359.1238
81. Mogazy AM, Hanafy RS. Foliar spray of biosynthesized zinc oxide nanoparticles alleviate salinity stress effect on Vicia faba plants. J Soil Sci Plant Nutr (2022) 22:2647–62. doi: 10.1007/s42729-022-00833-9
82. Singh D, Sillu D, Kumar A, Agnihotri S. Dual nanozyme characteristics of iron oxide nanoparticles alleviate salinity stress and promote the growth of an agroforestry tree, eucalyptus tereticornis Sm. Environ Sci: Nano (2021) 8(5):1308–25. doi: 10.1039/D1EN00040C
83. Dimkpa CO, McLean JE, Britt DW, Anderson AJ. Antifungal activity of ZnO nanoparticles and their interactive effect with a biocontrol bacterium on growth antagonism of the plant pathogen. Fusarium graminearum. BioMetals (2013) 26:913–24. doi: 10.1007/s10534-013-9667-6
84. Wang Y, Deng C, Elmer WH, Dimkpa CO, Sharma S, Navarro G, et al. Therapeutic delivery of nanoscale sulfur to suppress disease in tomatoes: In vitro imaging and orthogonal mechanistic investigation. ACS Nano (2022) 16:11204–17. doi: 10.1021/ACSNANO.2C04073
85. Wang Y, Deng C, Shen Y, Borgatta J, Dimkpa CO, Xing B, et al. Surface coated sulfur nanoparticles suppress fusarium disease in field grown tomato: increased yield and nutrient biofortification. J Agric Food Chem (2022) 70:14377–85. doi: 10.1021/ACS.JAFC.2C05255
86. Buri MM, Masunaga T, Wakatsuki T. Sulfur and zinc levels as limiting factors to rice production in West Africa lowlands. Geoderma (2000) 94:23–42. doi: 10.1016/S0016-7061(99)00076-2
87. Potarzycki J, Grzebisz W, Szczepaniak W. Magnesium fertilization increases nitrogen use efficiency in winter wheat (Triticum aestivum l.). Plants (2022) 11:2600. doi: 10.3390/plants11192600
88. Fujikawa I, Takehara Y, Ota M, Imada K, Sasaki K, Kajihara H, et al. Magnesium oxide induces immunity against fusarium wilt by triggering the jasmonic acid signaling pathway in tomato. J Biotechnol (2021) 325:100–8. doi: 10.1016/j.jbiotec.2020.11.012
89. Imada K, Sakai S, Kajihara H, Tanaka S, Ito S. Magnesium oxide nanoparticles induce systemic resistance in tomato against bacterial wilt disease. Plant Pathol J (2015) 65:551–60. doi: 10.1111/ppa.12443
90. Cabot C, Martos S, Llugany M, Gallego B, Tolrà R, Poschenrieder C. A role for zinc in plant defense against pathogens and herbivores. Fron Plant Sci (2019) 10:1171. doi: 10.3389/fpls.2019.01171
91. Dimkpa CO, Elmer W, Duffy B. Zinc and plant disease. In: Datnoff LE, Elmer WH, Huber DM, editors. Mineral nutrition and plant disease, 2nd Edition. MN, USA: The American Phytopathological Society (2022).
92. Borgatta J, Ma C, Hudson-Smith N, Elmer W, Pérez CDP, de la Torre-Roche R, et al. Copper based nanomaterials suppress root fungal disease in watermelon (Citrullus lanatus): role of particle morphology, composition and dissolution behavior. ACS Sustain Chem Eng (2018) 6:14847–56. doi: 10.1021/acssuschemeng.8b03379
93. Elmer W, de la Torre-Roche R, Pagano L, Majumdar S, Zuverza-Mena N, Dimkpa C, et al. Effect of metalloid and metallic oxide nanoparticles on Fusarium wilt of watermelon. Plant Dis (2018) 102:1394–401. doi: 10.1094/PDIS-10-17-1621-RE
94. Elmer WH, Roche R, Zuverza-Mena N, Dimkpa CO, White JL. Influence of single and combined mixtures of metal oxide nanoparticles on eggplant growth, yield, and verticillium wilt severity. Plant Dis (2021) 105:1153–61. doi: 10.1094/PDIS-07-20-1636-RE
95. Elmer WH, White JC. The use of metallic oxide nanoparticles to enhance growth of tomatoes and eggplants in disease infested soil or soilless medium. Environ Sci: Nano (2016) 3:1072–9. doi: 10.1039/C6EN00146G
96. Servin A, Elmer W, Mukherjee A, de la Torre-Roche R, Hamdi H, White JC, et al. A review of the use of engineered nanomaterials to suppress plant disease and enhance crop yield. J Nanopart Res (2015) 17:92. doi: 10.1007/s11051-015-2907-7
97. Peixoto S, Henriques I, Loureiro S. Long-term effects of Cu(OH)2 nanopesticide exposure on soil microbial communities. Environ pollut (2021) 269:116113. doi: 10.1016/j.envpol.2020.116113
98. Shaw JLA, Ernakovich JG, Judy JD, Farrell M, Whatmuff M, Kirby J. Long-term effects of copper exposure to agricultural soil function and microbial community structure at a controlled and experimental field site. Environ pollut (2020) 263(Pt A):114411. doi: 10.1016/j.envpol.2020.114411
99. Muthayyz S, Rah JH, Sugimoto JD, Roos FF, Kraemer K, Black RE. The global hidden hunger indices and maps: an advocacy tool for action. PloS One (2013) 8:e67860. doi: 10.1371/journal.pone.0067860
100. Niazi NK, Environmental Science and Engineering. Global arsenic hazard and sustainable development. In: Niazi NK, Bibi I, Aftab T, editors. Global arsenic hazard. Switzerland: Springer, Cham (2023). doi: 10.1007/978-3-031-16360-9_1
101. Rokonuzzaman M, Li WC, Man YB, Tsang YF, Ye Z. Arsenic accumulation in rice: sources, human health impact and probable mitigation approaches. Rice Sci (2022) 29(4):309–27. doi: 10.1016/j.rsci.2022.02.002
102. Murugaiyan V, Zeibeg F, Anumalla M, Siddiq SA, Frei M, Murugaiyan J, et al. Arsenic stress responses and accumulation in rice. In: Ali J, Wani SH, editors. Rice improvement. Switzerland: Springer, Cham (2021). doi: 10.1007/978-3-030-66530-2_9
103. Khan S, Akhtar N, Rehman SU, Shujah S, Rha ES, Jamil M. Biosynthesized iron oxide nanoparticles (Fe3O4 NPs) mitigate arsenic toxicity in rice seedlings. Toxics (2021) 9:2. doi: 10.3390/toxics9010002
104. Yan S, Wu F, Zhou S, Yang J, Tang X, Ye W. Zinc oxide nanoparticles alleviate the arsenic toxicity and decrease the accumulation of arsenic in rice (Oryza sativa l.). BMC Plant Biol (2021) 21:150. doi: 10.1186/s12870-021-02929-3
105. Liu J, Simms M, Song S, King RS, Cobb GP. Physiological effects of copper oxide nanoparticles and arsenic on the growth and life cycle of rice (Oryza sativa japonica ‘Koshihikari’). Environ Sci Technol (2018) 52:13728–37. doi: 10.1021/acs.est.8b03731
106. Liu C-H, Chuang Y-H, Chen T-Y, Tian Y, Li H, Wang M-K, et al. Mechanism of arsenic adsorption on magnetite nanoparticles from water: thermodynamic and spectroscopic studies. Environ Sci Technol (2015) 49:7726–34. doi: 10.1021/acs.est.5b00381
107. Irunde R, Ijumulana J, Ligate F, Maity JP, Ahmad A, Mtamba J, et al. Arsenic in Africa: Potential sources, spatial variability, and the state of the art for arsenic removal using locally available materials. Groundwater Sust Dev (2022) 18:100746. doi: 10.1016/j.gsd.2022.100746
108. Ekholuenetale M, Tudeme G, Onikan A, Ekholuenetale CE. Socioeconomic inequalities in hidden hunger, undernutrition, and overweight among under-five children in 35 sub-Saharan Africa countries. J Egypt Public Health Assoc (2020) 95(9). doi: 10.1186/s42506-019-0034-5
109. Joy EJM, Stein AJ, Young SD, Ander EL, Watts MJ, Broadley MR. Zinc-enriched fertilisers as a potential public health intervention in Africa. Plant Soil (2015) 389(1-2):1–24. doi: 10.1007/s11104-015-2430-8
110. De Groote H, Tessema M, Gameda S, Gunaratna NS. Soil zinc, serum zinc, and the potential for agronomic biofortification to reduce human zinc deficiency in Ethiopia. Sci Rep (2021) 11. doi: 10.1038/s41598-021-88304-6
111. Pobee RA, Aguree S, Colecraft EK, Gernand AD, Murray-Kolb LE. Food insecurity and micronutrient status among ghanaian women planning to become pregnant. Nutrients (2020) 12(2):1–12. doi: 10.3390/nu12020470
112. Kaur H, Garg N. Zinc toxicity in plants: a review. Planta (2021) 253(129). doi: 10.1007/s00425-021-03642-z
113. Kobayashi T, Nozoye T, Nishizawa NK. Iron transport and its regulation in plants. Free Radic Biol Med (2019) 133:11–20. doi: 10.1016/j.freeradbiomed.2018.10.439
114. Shinozaki D, Yoshimoto K. Autophagy balances the zinc–iron seesaw caused by zn-stress. Trends Plant Sci (2021) 26):882–4. doi: 10.1016/j.tplants.2021.06.014
115. Moreno-Jiménez E, Plaza C, Saiz H, Manzano R, Flagmeier M, Maestre FT. Aridity and reduced soil micronutrient availability in global drylands. Nat Sustain (2019) 2:371–7. doi: 10.1038/s41893-019-0262-x
116. Sanchez P.A.Agricultural productivity must improve in sub-Saharan Africa. Sci (2021) 372:1045–7. doi: 10.1126/science.abf5413
117. Buernor AB, Kabiru MR, Bechtaoui N, Jibrin JM, Asante M, Bouraqqadi A, et al. Grain legume yield responses to rhizobia inoculants and phosphorus supplementation under Ghana soils: A meta-synthesis. Front Plant Sci (2022) 13:877433. doi: 10.3389/fpls.2022.877433
118. Kihara J, Bolo P, Kinyua M, Nyawira SS, Sommer R. Soil health and ecosystem services: Lessons from sub-Sahara Africa (SSA). Geoderma (2020) 370:114342. doi: 10.1016/j.geoderma.2020.114342
119. Berde CP, Rawool P, Berde VB. Phosphate-solubilizing bacteria: recent trends and applications in agriculture in recent advancement in microbial biotechnology. Mandal SD, Passari AK, editors. Elsevier, Inc. New York: Academic Press (2021) p. 27–47.
120. Zeng Q, Ding X, Wang J, Han X, Iqbal HMN, Bilal M. Insight into soil nitrogen and phosphorus availability and agricultural sustainability by plant growth-promoting rhizobacteria. Environ Sci Pollut Res (2022) 29:45089–106. doi: 10.1007/s11356-022-20399-4
121. Chen YP, Rekha PD, Arun AB, Shen FT, Lai W-A, Young CC. Phosphate solubilizing bacteria from subtropical soil and their tricalcium phosphate solubilizing abilities. Appl Soil Ecol (2006) 34:33–41. doi: 10.1016/j.apsoil.2005.12.002
122. Wolinskà A, Stępniewska Z, Canuto RA. Dehydrogenase activity in the soil environment. In: Dehydrogenases. London, UK: InTech Publishers (2012). p. 183–210. doi: 10.5772/48294
123. Symanowicz B, Kalembasa S, Toczko M, Skwarek K. The effect of different potassium fertilization of forecrop on the enzymatic activity of soil in spring barley cultivation. Acta Agrophys (2018) 25:85–94. doi: 10.31545/aagr0007
124. Goteti PK, Emmanuel LDA, Desai S, Mir MHA. Prospective zinc solubilizing bacteria for enhanced nutrient uptake and growth promotion in maize (Zea mays l.). Int J Microbiol (2013) 2013:869697. doi: 10.1155/2013/869697
125. Meldau DG, Meldau S, Hoang LH, Underberg S, Wunsche H, Baldwin IT. Dimethyl disulfide produced by the naturally associated bacterium Bacillus sp B55 promotes Nicotiana attenuata growth by enhancing sulfur nutrition. Plant Cell (2013) 25:2731–47. doi: 10.1105/tpc.113.114744
126. Ijaz A, Mumtaz MZ, Wang X, Ahmad M, Saqib M, Maqbool H, et al. Insights into manganese solubilizing bacillus spp. for improving plant growth and manganese uptake in maize. Front Plant Sci (2021) 12:12. doi: 10.3389/fpls.2021.719504
127. Dimkpa CO, Hansen T, Stewart J, McLean JE, Britt DW, Anderson AJ. ZnO nanoparticles and root colonization by a beneficial pseudomonad influence essential metal responses in bean (Phaseolus vulgaris). Nanotoxicology (2015) 9:271–8. doi: 10.3109/17435390.2014.900583
128. Wright M, Adams J, Yang K, McManus P, Jacobson A, Gade A, et al. A root-colonizing pseudomonad lessens stress responses in wheat imposed by CuO nanoparticles. PloS One (2016) 11(10). doi: 10.1371/journal.pone.0164635
129. Etiang J, Uzatunga I, Barekye A, Turyamureeba G, Mwesige R, Kwikiriza G, et al. Effect of macro-nutrient combinations on yield and economic returns of potato. J Soil Sci Plant Health (2018) 2(2). doi: 10.4172/JSPH.1000112
130. Moghaddasi S, Fotovat A, Khoshgoftarmanesh AH, Karimzadeh F, Khazaei HR, Khorassani R. Bioavailability of coated and uncoated ZnO nanoparticles to cucumber in soil with or without organic matter. Ecotoxicol Environ Safe (2017) 144:543–51. doi: 10.1016/j.ecoenv.2017.06.074
131. Medina-Velo IA, Dominguez OE, Ochoa L, Barrios AC, Hernández-Viezcas JA, White JC, et al. Nutritional quality of bean seeds harvested from plants grown in different soils amended with coated and uncoated zinc oxidenanomaterials. Environ Sci: Nano (2017) 4:2336–47. doi: 10.1039/C7EN00495H
132. Ouyang K, Yu X, Zhu Y, Gao C, Huang Q, Cai P. Effects of humic acid on the interactions between zinc oxide nanoparticles and bacterial biofilms. environ pollut. 231 (2017) 231:1104–11. doi: 10.1016/j.envpol.2017.07.003
133. Li Y, Fang F, Wei J, Wu X, Cui R, Li G, et al. Humic acid fertilizer improved soil properties and soil microbial diversity of continuous cropping peanut: A three-year experiment. Sci Rep (2019) 9. doi: 10.1038/s41598-019-48620-4
134. Rasouli F, Nasiri Y, Asadi M, Hassanpouraghdam MB, Golestaneh S, Pirsarandib Y. Fertilizer type and humic acid improve the growth responses, nutrient uptake, and essential oil content on Coriandrum sativum l. Sci Rep (2022) 12:7437. doi: 10.1038/s41598-022-11555-4
135. Michalak I, Witek-Krowiak A, Chojnacka K, Bhatnagar A. Advances in biosorption of microelements – the starting point for the production of new agrochemicals. Rev Inorg Chem (2015) 35:115–33. doi: 10.1515/revic-2015-0003
136. Izydorczyk G, Sienkiewicz-Cholewa U, Baśladyńska S, Kocek D, Mironiuk M, Chojnacka K. New environmentally friendly bio-based micronutrient fertilizer by biosorption: From laboratory studies to the field. Sci Total Environ (2020) 710:136061. doi: 10.1016/j.scitotenv.2019.136061
137. Mikula K, Izydorczyk G, Skrzypczak D, Mironiuk M, Moustakas K, Witek-Krowiak A, et al. Controlled release micronutrient fertilizers for precision agriculture – a review. Sci Total Environ (2019) 712:136365. doi: 10.1016/j.scitotenv.2019.136365
138. Sanabria J, Wendt J. Statistical analysis of non-replicated experiments in farmers’ fields. a case of balanced fertilization trials for bean in Burundi. J Agron (2019) 111. doi: 10.2134/agronj2018.10.0655
139. Awio T, Senthilkumar K, Dimkpa CO, Otim-Nape GW, Struik PC, Stomph TJ. Yields and yield gaps in lowland rice systems and options to improve smallholder production. Agron (2022) 12:552. doi: 10.3390/agronomy12030552
140. Bindraban PS, Dimkpa C, Nagarajan L, Roy A, Rabbinge R. Revisiting fertilisers and fertilisation strategies for improved nutrient uptake by plants. Biol Fertil Soils (2015) 51:897–911. doi: 10.1007/s00374-015-1039-7
141. Mitter EK, Tosi M, Obregón D, Dunfield KE, Germida JJ. Rethinking crop nutrition in times of modern microbiology: Innovative biofertilizer technologies. Front Sustain Food Syst (2021) 5:606815. doi: 10.3389/fsufs.2021.606815
142. Mumtaz MZ, Ahmad M, Etesami H, Mustafa A. Editorial: Mineral solubilizing microorganisms (MSM) and their applications in nutrient availability, weathering and bioremediation. Front Microbiol (2023) 14:1101426. doi: 10.3389/fmicb.2023.1101426
143. Dimkpa C, Weinand T, Asch F. Plant-rhizobacteria interactions alleviate abiotic stress conditions. Plant Cell Environ (2009) 32:1682–94. doi: 10.1111/j.1365-3040.2009.02028.x
144. Dimkpa C. Microbial siderophores: production, detection and application in agriculture and environment. Endocytobiosis Cell Res (2016) 27:7–16.
145. Jacoby R, Peukert M, Succurro A, Koprivova A, Kopriva S. The role of soil microorganisms in plant mineral nutrition-current knowledge and future directions. Front Plant Sci (2017) 19:1617. doi: 10.3389/fpls.2017.01617
146. Sahu A, Bhattacharjya S, Mandal A, Thakur JK, Atoliya N, Sahu N, et al. Microbes: A sustainable approach for enhancing nutrient availability in agricultural soils. In: Meena V, editor. Role of rhizospheric microbes in soil. Singapore: Springer (2018). doi: 10.1007/978-981-13-0044-8_2
147. Sharma P, Kumar S. Bioremediation of heavy metals from industrial effluents by endophytes and their metabolic activity: Recent advances. Bioresour. Technol (2021) 339:125589. doi: 10.1016/j.biortech.2021.125589
148. Ma Y, Rajkumar M, Zhang C, Freitas H. Beneficial role of bacterial endophytes in heavy metal phytoremediation. J Environ Manage (2016) 174:14–25. doi: 10.1016/j.jenvman.2016.02.047
149. Pérez-Jaramillo JE, Carrión VJ, de Hollander M, Raaijmakers JM. The wild side of plant microbiomes. Microbiome (2018) 6:4–9. doi: 10.1186/s40168-018-0519-z
150. Alexandratos N, Bruinsma J. World agriculture towards 2030/2050: The 2012 revision. ESA working paper. 12–03. Rome: FAO (2012).
151. Kim DG, Grieco E, Bombelli A, Hickman JE, Sanz-Cobena A. Challenges and opportunities for enhancing food security and greenhouse gas mitigation in smallholder farming in sub-Saharan Africa. A review. Food Secur (2021) 13(2):457–76. doi: 10.1007/s12571-021-01149-9
152. Bell MJ, Cloy JM, Topp CFE, Ball BC, Bagnall A, Rees RM, et al. Quantifying N2O emissions from intensive grassland production: The role of synthetic fertilizer type, application rate, timing and nitrification inhibitors. J Agric Sci (2016) 154(5):812–27. doi: 10.1017/S0021859615000945
153. Menegat S, Ledo A, Tirado R. Greenhouse gas emissions from global production and use of nitrogen synthetic fertilisers in agriculture. Sci Rep (2022) 12(1):1–13. doi: 10.1038/s41598-022-18773-w
154. Angle SJ, Singh U, Dimkpa CO, Bindraban PS, Hellum DT. Role of ffertilizers for climate-resilient agriculture. In: Proceedings of the international fertiliser society, vol. 802. . London, U.K: International Fertiliser Society (2017). p. 44.
155. Rehman M, Liu L, Wang Q, Saleem MH, Bashir S, Ullah S, et al. Copper environmental toxicology, recent advances, and future outlook: a review. Environ Sci pollut Res (2019) 26(18):18003–16. doi: 10.1007/s11356-019-05073-6
156. Monreal CM, DeRosa M, Mallubhotla SC, Bindraban PS, Dimkpa C. Nanotechnologies for increasing the crop use efficiency of fertilizer-micronutrients. Biol Fertil Soils (2016) 52:423–37. doi: 10.1007/s00374-015-1073-5
157. Lam SK, Wille U, Hu HW, Caruso F, Mumford K, Liang X, et al. Next-generation enhanced-efficiency fertilizers for sustained food security. Nat Food (2022) 3:575–80. doi: 10.1038/s43016-022-00542-7
158. Subbaiah LV, Prasad T.N.V.K.V., Krishna TG, Sudhakar P, Reddy BR, Pradeep T. Novel effects of nanoparticulate delivery of zinc on growth, productivity, and zinc biofortification in maize (Zea mays l.). J Agric Food Chem (2016) 64:3778–88. doi: 10.1021/acs.jafc.6b00838
159. Ciceri D, Allanore A. Local fertilizers to achieve food self-sufficiency in Africa. Sci Total Environ (2019) 648:669–80. doi: 10.1016/j.scitotenv.2018.08.154
160. França D, Siqueira G, Nyström G, Clemens F, Souza CF, Faez R. Charged-cellulose nanofibrils as a nutrient carrier in biodegradable polymers for enhanced efficiency fertilizers. Carbohydr Polym (2022) 296:119934. doi: 10.1016/j.carbpol.2022.119934
161. Sashidhar P, Kochar M, Singh B, Gupta M, Cahill D, Adholeya A, et al. Biochar for delivery of agri-inputs: Current status and future perspectives. Sci Total Environ (2020) 703:134892. doi: 10.1016/j.scitotenv.2019.134892
162. Prajapati D, Pal A, Dimkpa C, Singh U, Devi KA, Choudhary JL, et al. Chitosan nanomaterials: A prelim of next-generation fertilizers; existing and future prospects. Carbohydr Polym (2022) 288:119356. doi: 10.1016/j.carbpol.2022.119356
163. Das CK, Jangir H, Kumar J, Verma S, Mahapatra SS, Philip D, et al. Nano-pyrite seed dressing: a sustainable design for NPK equivalent rice production. Nanotechnol Environ Eng (2018) 3:(14). doi: 10.1007/s41204-018-0043-1
164. Comer BA, Fuentes P, Dimkpa CO, Liu Y-H, Fernandez C, Arora P, et al. Prospects and challenges for solar fertilizers. Joule (2019) 3:1578–605. doi: 10.1016/j.joule.2019.05.001
165. Kihara J, Bolo P, Kinyua M, Nyawira SS, Sommer R. Soil health and ecosystem services: Lessons from sub-Sahara Africa (SSA). Geoderma (2020) 370:114342. doi: 10.1016/j.geoderma.2020.114342
166. Williams H, Colombi T, Keller T. The influence of soil management on soil health: An on-farm study in southern Sweden. Geoderma (2020) 360. doi: 10.1016/j.geoderma.2019.114010
167. Norris CE, Congreves KA. Alternative management practices improve soil health indices in intensive vegetable cropping systems: A review. Front Environ Sci (2018) 6:50. doi: 10.3389/fenvs.2018.00050
168. Martinsen V, Munera-echeverri JL, Obia A, Cornelissen G, Mulder J. Significant build-up of soil organic carbon under climate-smart conservation farming in Sub-Saharan acrisols. Sci Total Environ (2019) 660(3):97–104. doi: 10.1016/j.scitotenv.2018.12.452
169. Mashavakure N, Mashingaidze AB, Musundire R, Nhamo N, Gandiwa E, Thierfelder C, et al. Soil dwelling beetle community response to tillage, fertilizer and weeding intensity in a sub-humid environment in Zimbabwe. Appl Soil Ecol (2019) 135:120–8. doi: 10.1016/j.apsoil.2018.12.001
170. Adzawla W, Bindraban PS, Atakora WK, Gouzaye A, Camara O, Dogbe. R. Fertilizer use among maize farmers in Guinea savannah zone of Ghana: The role of topdressing fertilizer. In: IFDC FERARI research report no. 9. Ghana: IFDC (2022).
171. Adzawla W, Alhassan H. Effects of climate adaptation on technical efficiency of maize production in Northern Ghana. Agric Food Econ (2021) 9(14):1–18. doi: 10.1186/s40100-021-00183-7
Keywords: fertilizer-nutrients, fertilizer use efficiency, fertilizers for food and nutrition security, nano fertilizers, rhizospheric microbial composition, soil health, sub-Saharan Africa
Citation: Dimkpa C, Adzawla W, Pandey R, Atakora WK, Kouame AK, Jemo M and Bindraban PS (2023) Fertilizers for food and nutrition security in sub-Saharan Africa: An overview of soil health implications. Front. Soil Sci. 3:1123931. doi: 10.3389/fsoil.2023.1123931
Received: 14 December 2022; Accepted: 06 March 2023;
Published: 16 March 2023.
Edited by:
Hassan Ragab El-Ramady, Kafrelsheikh University, EgyptReviewed by:
Amitava Rakshit, Banaras Hindu University, IndiaAhmed Salah Elrys, Hainan University, China
Dr. Abhijit Sarkar, Indian Institute of Soil Science (ICAR), India
Copyright © 2023 Dimkpa, Adzawla, Pandey, Atakora, Kouame, Jemo and Bindraban. This is an open-access article distributed under the terms of the Creative Commons Attribution License (CC BY). The use, distribution or reproduction in other forums is permitted, provided the original author(s) and the copyright owner(s) are credited and that the original publication in this journal is cited, in accordance with accepted academic practice. No use, distribution or reproduction is permitted which does not comply with these terms.
*Correspondence: Christian Dimkpa, Q2hyaXN0aWFuLkRpbWtwYUBjdC5nb3Y=; William Adzawla, d2FkemF3bGFAaWZkYy5vcmc=