- 1Australian Institute for Bioengineering and Nanotechnology, The University of Queensland, Saint Lucia, QLD, Australia
- 2ERA Chair in Gas Fermentation Technologies, Institute of Technology, University of Tartu, Tartu, Estonia
- 3Center for Applied Geosciences, University of Tübingen, Tübingen, Germany
- 4Queensland Node of Metabolomics Australia, The University of Queensland, Saint Lucia, QLD, Australia
- 5LanzaTech Inc., Skokie, IL, United States
Acetogenic bacteria can convert waste gases into fuels and chemicals. Design of bioprocesses for waste carbon valorization requires quantification of steady-state carbon flows. Here, steady-state quantification of autotrophic chemostats containing Clostridium autoethanogenum grown on CO2 and H2 revealed that captured carbon (460 ± 80 mmol/gDCW/day) had a significant distribution to ethanol (54 ± 3 C-mol% with a 2.4 ± 0.3 g/L titer). We were impressed with this initial result, but also observed limitations to biomass concentration and growth rate. Metabolic modeling predicted culture performance and indicated significant metabolic adjustments when compared to fermentation with CO as the carbon source. Moreover, modeling highlighted flux to pyruvate, and subsequently reduced ferredoxin, as a target for improving CO2 and H2 fermentation. Supplementation with a small amount of CO enabled co-utilization with CO2, and enhanced CO2 fermentation performance significantly, while maintaining an industrially relevant product profile. Additionally, the highest specific flux through the Wood-Ljungdahl pathway was observed during co-utilization of CO2 and CO. Furthermore, the addition of CO led to superior CO2-valorizing characteristics (9.7 ± 0.4 g/L ethanol with a 66 ± 2 C-mol% distribution, and 540 ± 20 mmol CO2/gDCW/day). Similar industrial processes are commercial or currently being scaled up, indicating CO-supplemented CO2 and H2 fermentation has high potential for sustainable fuel and chemical production. This work also provides a reference dataset to advance our understanding of CO2 gas fermentation, which can contribute to mitigating climate change.
Introduction
Gas fermentation has attractive waste carbon valorization properties, for which the need is intensifying (International Panel on Climate Change [IPCC], 2014; Emerson and Stephanopoulos, 2019). Recently, LanzaTech commercialized the first waste gas-to-ethanol process, efficiently incorporating the carbon from steel mill off-gas into fuel quality ethanol via the model acetogen Clostridium autoethanogenum. The key carbon source—carbon monoxide (CO)—accounts for a significant portion of steel mill off-gas and synthesis gas (syngas), which can be generated from multiple high-volume, non-gaseous waste feedstocks (e.g., biomass, municipal solid waste) (Liew et al., 2016). Therefore, LanzaTech's process is significant in that it valorizes waste carbon by fusing two one-carbon gas molecules (C1) into liquid fuel. Furthermore, Handler et al. (2016) found that ethanol produced by LanzaTech's process reduced greenhouse gas emissions by 67 to 98% when compared to petroleum gasoline on an energy content and “cradle-to-grave” basis (feedstock dependent). Carbon dioxide (CO2) represents a more diverse and plentiful waste stream compared to CO (International Panel on Climate Change [IPCC], 2014), thus embodying a feedstock with greater climate change mitigation and carbon recycling potential.
Increasing acetogenic carbon capture as CO2 would build on the success of commercial gas fermentation and continue the expansion of the technology as a platform for sustainable chemical production (Redl et al., 2017; Bengelsdorf et al., 2018; Müller, 2019). Compared to other CO2 valorization methods, acetogens are ideal candidates due to their high metabolic efficiency, ability to handle variable gas compositions, high product specificity, scalability, and low susceptibility to poisoning by sulfur, chlorine, and tars (Liew et al., 2016; Artz et al., 2018). However, metabolism of CO2 requires an energy source, for which some see an appropriate solution is lacking (Emerson and Stephanopoulos, 2019).
Gas fermenting acetogens harbor the Wood-Ljungdahl pathway (WLP) (Drake et al., 2008), a non-photosynthetic C1-fixation metabolic pathway with the highest-known theoretical thermodynamic efficiency (Fast and Papoutsakis, 2012; Schuchmann and Müller, 2014; Müller, 2019). Various potential energy sources exist for metabolizing CO2, primarily hydrogen, nitrates, sugars, and arginine. Yet, acetogenic CO2 valorization, which is actively being developed for industrial implementation (Tizard and Sechrist, 2015), poses challenges along with promise. These include potential adenosine triphosphate (ATP) starvation in autotrophic conditions and carbon catabolite repression in hetero/mixotrophic conditions (Emerson and Stephanopoulos, 2019).
Hydrogen (H2) is the most recognized energy source for CO2 utilization—as metabolism of sugars or nitrates cause shifts in metabolism that result in lower CO2 or H2 utilization (Liew et al., 2016; Emerson and Stephanopoulos, 2019). H2 production will also logically transition to renewable sources in the future, whereas production of sugars and nitrates are dependent on less-sustainable methods. Furthermore, levelized cost predictions for solar H2 indicate a 30% reduction by 2030, potentially becoming competitive with the current levelized cost of fossil fuel derived H2 by 2035 (Detz et al., 2018; Glenk and Reichelstein, 2019). This is in part due to rapidly decreasing solar electricity costs (IRENA, 2017) and projections of H2 electrolysis technology development (Detz et al., 2018; Glenk and Reichelstein, 2019). Similarly, atmospheric CO2 capture via direct air contact showed promising feasibility recently (Keith et al., 2018), which represents an essential development for carbon recycling (Otto et al., 2015). Various power-to-gas technologies are being discussed for mediating fluctuations in renewable power generation (Götz et al., 2016). By extension, gas fermentation to liquid products could couple mediation of renewable power fluctuations to carbon recycling (Redl et al., 2017). This provides an attractive new opportunity for bacterial artificial-photosynthesis, whereby renewable H2 supplementation facilitates acetogenic CO2 valorization (Claassens et al., 2016; Haas et al., 2018).
Continuous culture bioprocesses are preferable to batch or fed-batch fermentation bioprocesses (Hoskisson and Hobbs, 2005). Furthermore, systems-level quantification is essential for design-build-test-learn bioprocess optimization by metabolic engineering (Valgepea et al., 2017). Therefore, obtaining quantitative datasets from steady-state chemostat cultures, whose analyses are comparable between experiments, is important for development of these systems (Adamberg et al., 2015). Whilst Bengelsdorf et al. (2018) reviewed autotrophic acetogen growth on CO2 and H2 (CO2/H2), and Mock et al. (2015) provided notable insight into the CO2/H2 metabolism of C. autoethanogenum, the literature lacks a steady-state dataset where carbon flows in a CO2/H2 fermentation are quantified. Here we aimed to quantify steady-state CO2/H2 fermentation using fully instrumented chemostats and the model acetogen C. autoethanogenum. Subsequently, we showed that CO2 is a promising feedstock alternative to CO, as more than half of the substrate CO2 carbon was converted into ethanol. Furthermore, supplementation with CO at low concentrations improved fermentation performance significantly.
Materials and Methods
Bacterial Strain, Growth Medium, and Continuous Culture Conditions
A derivate of Clostridium autoethanogenum DSM 10061 strain—DSM 19630—deposited in the German Collection of Microorganisms and Cell Cultures (DSMZ) was used in all experiments and stored as glycerol stocks at −80°C. This non-commercial strain was grown on CO2/H2 (~23% CO2, ~67% H2 and ~10% Ar; BOC Australia) and CO/CO2/H2 (~2% CO, ~23% CO2, ~65% H2, and ~10% Ar; BOC Australia) in chemically defined medium (Valgepea et al., 2017). Cells were grown under strictly anaerobic conditions at 37°C and at a pH of 5 (maintained by 5 M NH4OH). Chemostat continuous culture achieved steady-states at dilution rates (D) = 0.47 ± 0.01 (CO2/H2; specific growth rate (μ) = 0.0196 ± 0.0004 [average ± standard deviation]), 0.5 ± 0.01, and 1 ± 0.01 day−1 (CO/CO2/H2; μ = 0.021 ± 0.0004, and 0.042 ± 0.0008 h−1 respectively). See Table 1 for steady-state gas-liquid mass transfer rate data. The steady-state results reported here were collected after optical density (OD), gas uptake and production rates had been stable in chemostat mode for at least three working volumes. See Valgepea et al. (2017) for details on equipment.
Experimental Analysis and Quantification
Biomass concentration (gDCW/L) was estimated, and extracellular metabolome analysis carried out as specified in Valgepea et al. (2018).
Bioreactor off-gas was analyzed by an online Hiden HPR-20-QIC mass spectrometer. The Faraday Cup detector monitored the intensities of H2, CO, ethanol, H2S, Ar, and CO2 at 2, 14, 31, 34, 40, and 44 atomic mass units (amu), respectively, in the bioreactor off-gas. These masses were chosen so that each target compound would be represented by a unique signal. This was determined to be essential to achieve the highest confidence in quantification using preliminary experiments, as interferences from other compounds at a shared mass could not be reliably accounted for (e.g., the more intense signal from CO at 28 amu could not be used due to the uncertainty of interference at 28 amu from CO2 fragmentation). Gas from the cylinder was used as the calibration gas for each MS-cycle (i.e., “online calibration”) to achieve reliable off-gas analysis (Valgepea et al., 2017).
Gas uptake (CO, CO2, and H2) and production (ethanol) were determined using “online calibration” of the MS by analyzing the respective feed gas directly from the cylinder after each analysis cycle of the bioreactors. Specific rates (mmol/gDCW/h) were calculated by taking into account the exact composition of the respective gas, bioreactor liquid working volume, feed gas flow rate, off-gas flow rate (based on the fractional difference of the inert gas [Ar] in the feed and off-gas composition), the ideal gas molar volume, and the steady-state biomass concentration.
The carbon balances were determined at 116 ± 11%, 103 ± 12%, and 108 ± 11% for CO2/H2, and CO/CO2/H2 at D = 0.5 and 1 day−1 respectively (total C-mol products/total C-mol substrates), as specified in Valgepea et al. (2017).
Genome-Scale Metabolic Modeling With GEM iCLAU786
Model simulations were performed using genome scale model (GEM) iCLAU786 of C. autoethanogenum and flux balance analysis (FBA) (Orth and Palsson, 2011) as specified in Valgepea et al. (2018). Briefly, we used FBA to estimate intracellular fluxes (SIM1–26) by incorporating experimentally measured constraints (specific growth rate, and specific acetate, ethanol, cysteine, CO, CO2, and H2 consumption or production rates; Table S3) and employing maximization of ATP dissipation as the objective function. These values were validated by prediction of “optimal” growth phenotypes for experimental conditions (SIM27–48), which incorporated experimental constraints (specific cysteine, CO, CO2, and H2 consumption rates), ATP dissipation flux calculated above, and maximization of biomass yield as the objective function. Complete simulation results identified as SIMx (e.g., SIM1) in the text are in Table S11. SIM1-19, 27-41, and 49-55 are from Valgepea et al. (2018). Additional constraints were also used to improve the accuracy of predictive simulations were justifiable [SIM49-55; details described in Valgepea et al. (2018)]. Here, CO2 reduction to formate was forced from the formate dehydrogenase (FdhA) reaction scheme (Reaction ID: rxn00103_c0) in SIM42-48, to the correct reaction scheme in SIM56-62, as identified by Mock et al. (2015)—FdhA/Hydrogenase ABCDE complex (HytABCDE; Reaction ID: rxn08518_c0). Additionally, since extracellular metabolome analysis did not detect excretion of pyruvate by the cells, we manually blocked pyruvate export in the model (rxn05469_c0) in SIM56-62. Reaction IDs comprising letters and numbers are labels corresponding to reactions in Table S11 (follows reference to reaction/enzyme).
Results
Clostridium autoethanogenum Steady-State Fermentation of Carbon Dioxide and Hydrogen
Clostridium autoethanogenum cells reached steady-state when growing on CO2/H2 in chemostats at dilution rate (D) ~0.5 day−1 [specific growth rate (μ) ~0.02 h−1] with a biomass concentration of 0.18 ± 0.02 g dry cell weight (gDCW)/L (Figure 1A). It is important to note that attempts to reach a steady-state at D = 1 day−1 were unsuccessful. Unlike the chemostat cultures of C. autoethanogenum with CO (Valgepea et al., 2017, 2018) and CO2/H2 retentostat cultures (Mock et al., 2015), the CO2/H2 cultures could not reach stable biomass concentrations before the culture began oscillation cycles; previously observed above ~1.6 gDCW/L (Valgepea et al., 2017). The physiological reason and mechanism for such oscillatory culture behavior are under investigation, but we assumed that cell recycling is a requirement for CO2/H2 culture stability. For example, Molitor et al. (2019) showed consistent, high-biomass concentration and high-acetate CO2/H2 fermentation with Clostridium ljungdahlii in a retentostat with complete recycling.
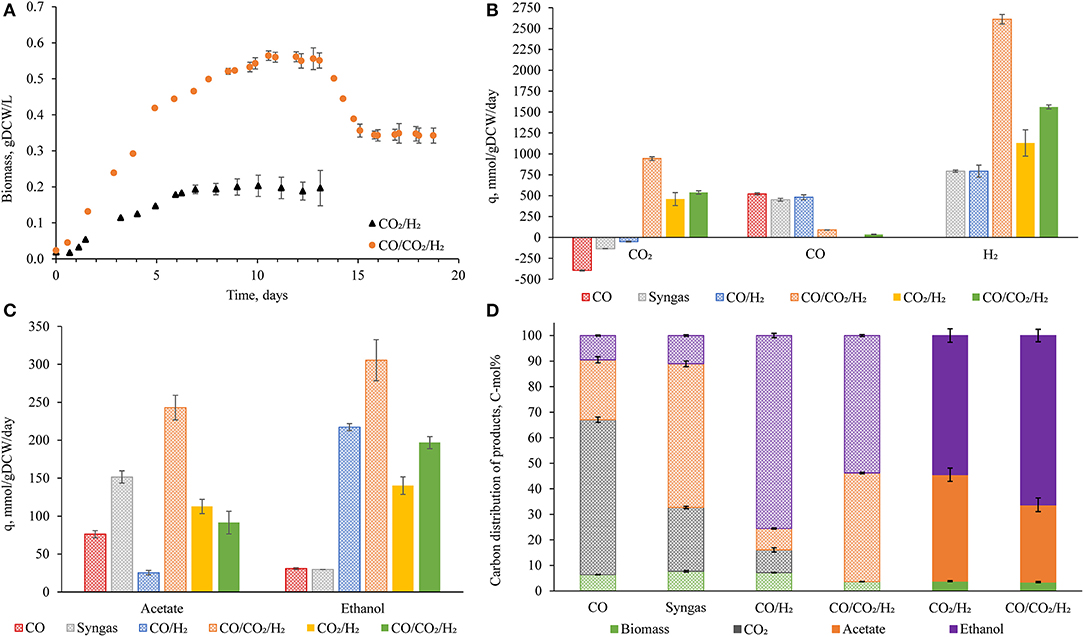
Figure 1. Important fermentation characteristics of Clostridium autoethanogenum in autotrophic chemostats. Results from Valgepea et al. (2018) are also displayed (B–D), the conditions of all fermentations are summarized in Table 1. Growth curves of novel fermentations with standard deviation at steady-state (A). Specific rates of uptake (B) and production (C) for important metabolites. Product carbon balances (D). Values represent the average ± standard deviation between biological replicates. Number of biological replicates, and detailed gas composition for each fermentation are available in Table 1. Patterned bars indicate a D of 1 day−1, full bars indicate a D of 0.5 day−1 (B–D). q, specific rate; DCW, dry cell weight.
Despite the attempt to reach a steady-state at D = 1 day−1, cells reached steady-state at dilution rate = 0.5 day−1, with a specific rate of carbon incorporation (i.e., qCO2) of 480 ± 80 mmol/gDCW/day (Figure 1B). Furthermore, the specific production rates of ethanol and acetate were 140 ± 10 and 113 ± 9 mmol/gDCW/day, respectively (Figure 1C). Strikingly, this meant around half of the captured carbon was converted to ethanol (54 ± 3 C mol%) (Figure 1D and Table S4). Fermentation conditions and titers are available in Table 1, showing an impressive ethanol concentration compared to previous fermentations where CO was the main carbon and energy source.
Despite the different dilution rate, the CO2/H2 results generated were compared to previously published chemostat cultures of C. autoethanogenum grown on CO, syngas, and CO/H2 (Valgepea et al., 2018) at similar biomass concentrations (~0.5 gDCW/L) (Figures 1B–D). Specific rates of acetate and ethanol production achieved here for CO2/H2 cultures fell between those for syngas () and CO/H2 (
) cultures (Figures 1B,D). However, the specific rate of carbon incorporation was higher for CO2/H2 (Figure 1C). We found that more than half of the captured CO2 was converted into ethanol (Figure 1D). These results were encouraging, especially as ethanol production has unfavorable stoichiometry compared to acetate (Mock et al., 2015). Furthermore, the H2 specific uptake rate (1,130 ± 160 mmol/gDCW/day) showed that higher H2 uptake rates are achievable (compared to old datasets). These results show that higher carbon yields are possible (Valgepea et al., 2018). To investigate further the metabolic demand and the feasibility of CO2/H2 fermentation, we utilized the measured specific consumption and production rates and specific growth rate from the steady-state dataset as constraints for the genome-scale metabolic model (GEM) to find candidate mechanisms for improving CO2/H2 fermentation using the metabolic model iCLAU786.
Metabolic Model of Carbon Dioxide and Hydrogen Fermentation
Estimation of intracellular processes constrained by in vivo datasets represents an important developmental step for progressing acetogenic CO2 valorization. Here, we used the GEM to compare intracellular metabolic flux distributions on CO2/H2 and CO-containing gases (Figure 2). See Figures S2, S3 and Tables S8–S10 for further flux comparison summaries.
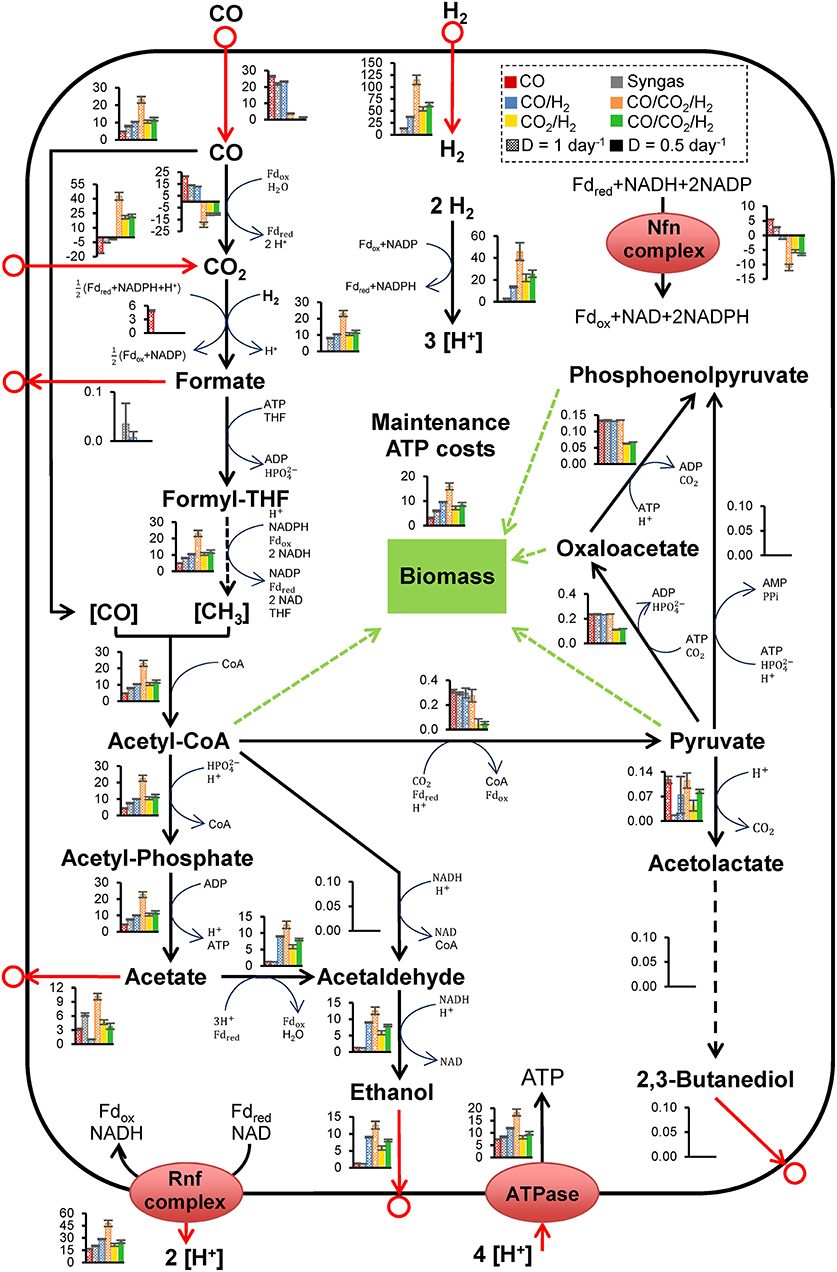
Figure 2. Intracellular metabolic fluxes in Clostridium autoethanogenum growing on various gas mixes, estimated using the metabolic model iCLAU786 and flux balance analysis. Bar charts show specific flux rates (mmol/gDCW/h) from Tables S10, S11 and represent the average ± standard deviation between biological Replicates from SIM: 1–4 (CO), 9–10 (Syngas), 13–15 (CO/H2), 20–21 (CO/CO2/), 22–24 (CO2/H2), and 25–26 (CO/CO2/). Results for CO, syngas, and CO/H2 are low biomass condition data from Valgepea et al. (2018), the conditions of these fermentations are summarized in Table 1. Number of biological replicates, and detailed gas composition for each fermentation are available in Table 1. Arrows show the direction of calculated fluxes; red arrows denote uptake or secretion, dashed arrows denote a series of reactions. Brackets denote metabolites bound by an enzyme. Refer to Figures S1, S2 for enzyme involvement, metabolite abbreviations, and complete flux balance analysis datasets.
Intracellular metabolic fluxes estimated using the model iCLAU786 and flux balance analysis (FBA) (Figure 2) showed remarkable similarity to the combined theoretical stoichiometry of acetate and ethanol production (Mock et al., 2015) and indicated energetic cofactor circuits with mapping close to 1:1 (experimental:theoretical stoichiometry; Figure S4). Ethanol production likely occurred via acetaldehyde:ferredoxin oxidoreductase (AOR; ReactionID: leq000004; see Materials and Methods) under autotrophic conditions, with the HytABCDE (leq000001) and Nfn complex (leq000002) likely facilitating cofactor production via electron bifurcation (Figure 2) (Valgepea et al., 2018). This is a mechanism for minimization of free energy loss employed by C. autoethanogenum and may play a key role in sustaining proton motive force by balancing acetate, ethanol, and ATP production (Mock et al., 2015; Valgepea et al., 2018). Engineering acetogens to redirect this energy toward cellular growth, sacrificing some ethanol production, could be beneficial for CO2 fermentation (Emerson and Stephanopoulos, 2019).
It was notable that, unlike CO fermentations, the intracellular pyruvate:ferredoxin oxidoreductase (PFOR; rxn05938_c0; acetyl-CoA ↔ pyruvate) flux was not significantly in the direction of pyruvate (Figure 2) (Valgepea et al., 2018). Under autotrophic conditions, PFOR links the WLP to anabolic pathways associated with biomass (Furdui and Ragsdale, 2000), and therefore this indicated high cell-specific energetic limitation. From this observation, we hypothesized that CO supplementation could provide a potential solution, as CO oxidation would generate additional Fdred. Furthermore, an ATP/H2 flux ratio of ~0.15 was estimated using metabolic modeling here compared to an ATP/CO ratio of ~0.28 in CO only fermentations (Valgepea et al., 2018). Considering CO/H2 and CO2/H2 fermentations had equal carbon-flux through the WLP (~10 mmol/gDCW/h; Figure 2), supplementation with renewable CO from CO2 electrolysis could aid biomass formation and culture stability. A similar process (but CO fermentation) was detailed by Haas et al. (2018).
Clostridium autoethanogenum Steady-State Fermentation of Carbon Dioxide and Hydrogen Supplemented With Carbon Monoxide
To validate our modeling hypothesis, Clostridium autoethanogenum was cultured with a low concentration of carbon monoxide in addition to CO2 and H2 (CO/CO2/H2) in chemostats. A steady-state was reached at D = 0.5 day−1 (μ ~0.02 h−1), and at D =1 day−1 (μ ~0.04 h−1; Figure 1A; biomass concentrations of 0.54 ± 0.01 and 0.34 ± 0.02 gDCW/L respectively). CO/CO2/H2 fermentations at a D = 1 day−1 (CO/CO2/; superscript 1 denotes D of 1 day−1) and at a D = 0.5 day−1 (CO/CO2/; superscript 0.5 denotes D of 0.5 day−1) showed simultaneous uptake of CO (89 ± 2 and 36 ± 4 mmol/gDCW/day, respectively) and CO2 (940 ± 20 and 540 ± 20 mmol/gDCW/day, respectively) (Figure 1B). The co-utilization of both C1 gases is, to the best of our knowledge, an unquantified phenomenon. This led to a specific carbon incorporation (CO/CO2/1030 ± 30 mmol/gDCW/day) larger than any other gas type (maximum of ~450 mmol/gDCW/day for fermentations with CO in Valgepea et al. (2018) or CO2/H2 in this work). This also resulted in significant improvements to culture performance compared to CO2/H2 fermentations.
Compared to CO2/H2, CO/CO2/ showed higher acetate and ethanol titers (Table 1) and specific productivities (Figure 1C), and a higher ethanol/acetate ratio (2.15 vs. 1.24 mol/mol respectively; Tables S1, S2). While at a similar biomass concentration (CO/CO2/ best comparison due to similarity in dilution rate), acetate and ethanol titers (Table 1), and specific productivities (Figure 1C) are greater than during fermentation of other CO-containing gases. When comparing to high biomass (~1.4 gDCW/L) CO cultures, CO-supplementation still performs impressively—CO/H2 fermentation achieved a higher ethanol titer (11.6 ± 0.4 g/L), while CO and syngas fermentations were similar (3.9 ± 0.2 and 5.4 ± 0.3 g/L respectively; Table S5). Otherwise, all specific productivities were higher for CO/CO2/ (Figure S3). Furthermore, the distribution of carbon to ethanol was still >50% (53.8 ± 0.4% and 66 ± 2% for CO/CO2/ and CO/CO2/, respectively; Figure 1D and Table S4).
To understand the metabolic effects of supplementing CO, FBA was performed using the same conditions and alterations as for CO2/H2 (Figure 2). Notably, the WLP specific flux throughput for CO/CO2/ was ~2-fold greater than for any other gas type (including high-biomass Valgepea et al., 2018). Furthermore, for CO2 fermentations, Nfn complex flux direction was opposite that of CO and syngas fermentations. CO/CO2/ also showed significantly greater flux through the AOR, whilst specific WLP productivity was insignificantly different compared to CO2/H2.
Discussion
Achieving steady-state continuous cultures using CO2/H2 mixtures, without cell recycling here, was challenging. Yet, compared to other organisms fermenting CO2/H2 with continuous medium exchange, Clostridium autoethanogenum performs well (Table 2). No direct comparisons can be made to other experiments due to variations in conditions, but C. autoethanogenum clearly achieves the highest ethanol production, with comparable quantities of carbonous products also. Acetobacterium woodii, along with Sporomusa ovata, were shown to perform well when compared to a wide range of acetogens under batch CO2/H2 conditions (Groher and Weuster-Botz, 2016). Yet, as evidenced by omission of S. ovata from Table 2, few continuous culture characterizations of acetogens are available—an essential step for validation of industrial robustness in gas fermentation. As discussed by Molitor et al. (2019), the lack of yeast extract or C≥2 substrates is also distinguishing between fermentations.
Notably, CO2/H2 cultures displayed higher variability between biological replicates compared to those of CO-containing gas mixtures (Figure 1) (Valgepea et al., 2017). This may indicate variable organism fitness, a trait previously discussed for C. autoethanogenum by Liew et al. (2016), who extensively covered numerous techniques used for enhancing gas fermentation including—coupling to other processes, adaptive laboratory evolution, and metabolic engineering of acetogens using genetic tools. CO-supplementation could be a valuable option for enhancement as it overcomes inherent problems linked to engineering acetogens. Supplementation of low quantities of CO here stabilized the culture, enabled culturing at D = 1 day−1, and achieved higher biomass concentration with a carbon incorporation larger than any other gas type—all without compromising by-product distribution.
While, Valgepea et al. (2018) found that syngas fermentation lead to CO-only fermentation at steady-state, we observed co-utilization of CO and CO2. Tizard and Sechrist (2015) have also shown co-utilization for C. autoethanogenum continuous cultures, and it seems that co-uptake may also occur for some points of syngas batch fermentation (Infantes et al., 2020). Co-utilization of sugars was found for E. coli in chemostats—where inhibition of consumption, but no change in induction time was observed (Standing et al., 1972). The WLP is most likely no different, in that metabolism of CO is preferential, yet the pathway can co-consume CO2 under certain conditions.
Various efforts have been made toward enhancing CO2(+H2) fermentation to C≥2 products (Table 2) (Emerson and Stephanopoulos, 2019). Braun and Gottschalk (1981) first discovered the potential for enhancement when Acetobacterium woodii simultaneously consumed fructose and a headspace of CO2/H2 during batch cultivation. Growth and acetate production was high but no characterization of the headspace was performed. More recently, continuous glucose-supplemented CO2/H2 fermentation of Moorella thermoacetica by Park et al. (2019) did not lead to net uptake of CO2. Furthermore, Jones et al. (2016) did not show net CO2 uptake for a wide range of acetogens (not A. woodii) fermenting syngas and fructose. A. woodii generates a sodium ion (Na+) gradient (Hess et al., 2013) rather than a proton (H+) gradient for membranous ATP generation (Pierce et al., 2008; Poehlein et al., 2015; Bengelsdorf et al., 2018). This may highlight an important metabolic difference from other model acetogens—decoupling the resources of the WLP and membranous ATP generation pathways could facilitate fermentation of sugar and CO2/H2 simultaneously.
Other enhancements have also struggled to achieve net CO2 uptake. Co-culture of C. acetobutylicum and C. ljungdahlii showed syntrophic metabolic coupling when fermenting glucose, fructose, and CO2/H2, but no net CO2 uptake (Charubin and Papoutsakis, 2019). Addition of nitrate to batch CO2/H2 fermentation by C. ljungdahlii, increased biomass concentration and subsequently volumetric productivity of acetate (Emerson et al., 2019). However, the specific WLP productivity decreased, meaning lower utilization of CO2. Other organisms not recognized as gas fermenters can also use mixotrophy to minimize carbon loss, such as Clostridium beijerinckii but have not displayed net CO2 uptake either (Sandoval-Espinola et al., 2017). To the best of our knowledge, this is the first report where supplementation of a substrate other than H2, increased productivities of continuous acetogenic CO2 fermentation while maintaining net CO2 utilization. Furthermore, the effect of CO supplementation on CO2 utilization was superlinear, indicating a synergistic mechanism (Park et al., 2019). This is encouraging for development of bioprocesses valorizing CO2.
Comparisons between fermentation datasets enables us to speculate about the positive effect of CO-supplementation on CO2/H2 fermentation. Although, addition of CO led to minimal metabolic shifts (Figure 2—CO2/H2 vs. CO/CO2/ and Figure S2), FBA showed that CO supplementation caused significant increases to the reduced ferredoxin consumption by AOR and Rnf complex (leq000004 and M002, respectively) compared to CO2/H2 (Figure 2). The overflow model proposed by Richter et al. (2016) suggests that high NADH production via Rnf and Nfn complexes (leq000002) is also important for reducing AOR product inhibition. In this way, NADH facilitates fast metabolism of acetaldehyde to ethanol via alcohol dehydrogenase (Adh(E); rxn00543_c0). Decreasing the acetate concentration reduces acidification and the ATP cost for excreting acetate (Valgepea et al., 2018). Including acetaldehyde conversion to ethanol and association to acetic acid, this also leads to consumption of 2 H+ (4 here vs. 2 produced via CODH). Therefore, CO consumption decreases the intracellular H+ pool, and following Le Chatelier's principle, drives HytABCDE activity. Indeed, the change in specific H2 uptake relative to specific CO2 uptake is greater than that of CO (for CO2/H2 vs. CO/CO2/, Table S7). Subsequently, the relative gain in free energy from H2 is ~ 4-fold greater than CO. We speculate this is ultimately responsible for the improved fitness of CO-supplemented CO2/H2 fermentation by C. autoethanogenum. We propose the following five critical factors to this enhanced metabolism: [1] metabolism of CO increases the intracellular pool of reduced ferredoxin; [2] this stimulates oxidation of ferredoxin, which if consumed by the AOR; [3] reduces ATP costs; and [4] decreases the H+ pool/acidification; which therefore [5] drives H2 uptake for further reduction of ferredoxin. Evidently, additional understanding of acetogenic redox metabolism, from a thermodynamic perspective, is important for developing acetogenic CO2-valorization as a platform industrial bioprocess (Cueto-Rojas et al., 2015).
Physicochemical properties could also play a key role in CO-supplementation enabling to achieve a stable CO2/H2 chemostat culture at D = 1 day−1. Generation of a stable and large non-equilibrium is what drives microbial growth (Qian and Beard, 2005; Igamberdiev and Kleczkowski, 2009; Quéméner and Bouchez, 2014) and gas-liquid mass transfer (Ma et al., 2005). For continuous culture of gas fermenting microbes, an inherent relationship between substrate mass transfer and culture growth exists (Supplementary Materials Note 2.1). An important parameter for these systems is the Gibb's free energy of a system (Cueto-Rojas et al., 2015). This describes the thermodynamic favorability of the reaction system—termed spontaneity. Here, analysis of experimental flux and Gibbs free energy suggests that CO2/H2 fermentation is infeasible (), whereas CO-supplemented CO2/H2 fermentation is feasible (; Table S6). Though these calculations use standard conditions, they do indicate how close CO2/H2 fermentation is to the thermodynamic limit of metabolism. Theoretically, minute and unobservable changes to chemostat CO2/H2 fermentation can disrupt the culture (Henry and Martin, 2016). Thus, increasing the free energy of central metabolism with CO-supplementation appears to keep metabolism in a spontaneous and stable state by increasing reduced ferredoxin production.
The mechanisms for achieving the 2-fold higher specific WLP flux throughput for CO/CO2/ compared to others is less clear but appears to be linked to the difference in primary substrate. CO/CO2/ and CO/H2 are the most similar CO2 and CO fermentations, respectively (D ~1 day−1 and carbon to hydrogen feed ratio (~1:3); Table 1), and the maximum carbon incorporation per cell for CO/H2 was roughly half of that of CO/CO2/ (~450 vs. ~1,000 mmol/gDCW). Theoretically, cells will maximize carbon-to-redox metabolism by minimizing thermodynamic losses. CO supplementation to a CO2/H2 culture seems to facilitate this as (H2/carbon)feed–(H2/carbon)flux was ~0 mol/mol for CO/CO2/H2 fermentations only (Table S2)—an indication of the relative magnitude of carbon and redox metabolism. This suggests that high specific fluxes for CO/CO2/ may be a result of (close to) optimal co-factor recycling by C. autoethanogenum's WLP and redox pathway. Thus, the lower energy associated with CO2 fermentation may, counterintuitively, stimulate specific WLP activity when in the presence of appropriate energy-containing substrates. Further quantifications of CO2 metabolism and characterizations of enzyme activities are required to confirm these hypotheses (Supplementary Materials Note 2.2), as they assist our ability to engineer the links between redox and carbon metabolisms.
We established a dataset quantifying steady-state of the model acetogen C. autoethanogenum during autotrophic-CO2/H2 growth in chemostat cultures. This enabled analysis via FBA, and highlighted CO as a potential supplement. CO supplementation successfully improved metabolic stability and CO2 utilization. This was the first time that intracellular fluxes for net uptake of CO2 (with enhancement) where characterized. Industry is actively developing gas fermentation to valorize CO2 (Tizard and Sechrist, 2015; Haas et al., 2018). Previously, genetic and process engineering of gas fermentation successfully developed the technology for industrial CO valorization (Liew et al., 2016). Therefore, progression to industrial CO2 valorization is foreseeable, and CO supplementation may play a role in the continuing diversification of industrial gas fermentation.
Data Availability Statement
All datasets generated for this study are included in the article/Supplementary Material.
Author Contributions
All authors viewed and approved the manuscript and contributed significantly to the work. KV, EM, and LN conceived the project. JH, KV, and EM designed the experiments and analyzed the results. JH and KV performed experiments, supported by RS, IC, MP, and EM. JH wrote the manuscript with the help of KV, EM, RT, SS, MK, and LN.
Funding
This study was funded by a Grant from the Australian Research Council, partly funded by LanzaTech (ARC LP140100213).
Conflict of Interest
LanzaTech has interest in commercializing gas fermentation with C. autoethanogenum. RT, SS, and MK are employees of LanzaTech.
Acknowledgments
Elements of this research utilized equipment and support provided by the Queensland node of Metabolomics Australia, an initiative of the Australian Government being conducted as part of the NCRIS National Research Infrastructure for Australia. IC would like to acknowledge support from the German Academic Exchange Service (DAAD) through the DAAD Kurzstipendien für Doktoranden. We thank the following investors in LanzaTech's technology: Sir Stephen Tindall, Khosla Ventures, Qiming Venture Partners, Softbank China, the Malaysian Life Sciences Capital Fund, Mitsui, Primetals, CICC Growth Capital Fund I, L.P. and the New Zealand Superannuation Fund. There was no funding support from the European Union for the experimental part of the study. However, KV acknowledges support also from the European Union's Horizon 2020 research and innovation programme under grant agreement N810755.
Supplementary Material
The Supplementary Material for this article can be found online at: https://www.frontiersin.org/articles/10.3389/fbioe.2020.00204/full#supplementary-material
References
Adamberg, K., Valgepea, K., and Vilu, R. (2015). Advanced continuous cultivation methods for systems microbiology. Microbiology 161, 1707–1719. doi: 10.1099/mic.0.000146
Artz, J., Müller, T. E., Thenert, K., Kleinekorte, J., Meys, R., Sternberg, A., et al. (2018). Sustainable conversion of carbon dioxide: an integrated review of catalysis and life cycle assessment. Chem. Rev. 118, 434–504. doi: 10.1021/acs.chemrev.7b00435
Bengelsdorf, F. R., Beck, M. H., Erz, C., Hoffmeister, S., Karl, M. M., Riegler, P., et al. (2018). Bacterial anaerobic synthesis gas (syngas) and CO2 + H2 fermentation. Adv. Appl. Microbiol. 103, 143–221. doi: 10.1016/bs.aambs.2018.01.002
Braun, K., and Gottschalk, G. (1981). Effect of molecular hydrogen and carbon dioxide on chemo-organotrophic growth of Acetobacterium woodii and Clostridium aceticum. Arch. Microbiol. 128, 294–298. doi: 10.1007/BF00422533
Charubin, K., and Papoutsakis, E. T. (2019). Direct cell-to-cell exchange of matter in a synthetic Clostridium syntrophy enables CO2 fixation, superior metabolite yields, and an expanded metabolic space. Metab. Eng. 52, 9–19. doi: 10.1016/j.ymben.2018.10.006
Claassens, N. J., Sousa, D. Z., dos Santos, V. A. P. M., de Vos, W. M., and van der Oost, J. (2016). Harnessing the power of microbial autotrophy. Nat. Rev. Microbiol. 14, 692–706. doi: 10.1038/nrmicro.2016.130
Cueto-Rojas, H. F., van Maris, A. J., Wahl, S. A., and Heijnen, J. J. (2015). Thermodynamics-based design of microbial cell factories for anaerobic product formation. Trends Biotechnol. 33, 534–546. doi: 10.1016/j.tibtech.2015.06.010
Detz, R. J., Reek, J. N. H., and Van Der Zwaan, B. C. C. (2018). The future of solar fuels: when could they become competitive? Energy Environ. Sci. 11, 1653–1669. doi: 10.1039/C8EE00111A
Drake, H. L., Gößner, A. S., and Daniel, S. L. (2008). Old acetogens, new light. Ann. N. Y. Acad. Sci. 1125, 100–128. doi: 10.1196/annals.1419.016
Emerson, D. F., and Stephanopoulos, G. (2019). Limitations in converting waste gases to fuels and chemicals. Curr. Opin. Biotechnol. 59, 39–45. doi: 10.1016/j.copbio.2019.02.004
Emerson, D. F., Woolston, B. M., Liu, N., Donnelly, M., Currie, D. H., and Stephanopoulos, G. (2019). Enhancing hydrogen-dependent growth of and carbon dioxide fixation by Clostridium ljungdahli through nitrate supplementation. Biotechnol. Bioeng. 116, 294–306. doi: 10.1002/bit.26847
Fast, A. G., and Papoutsakis, E. T. (2012). Stoichiometric and energetic analyses of non-photosynthetic CO2-fixation pathways to support synthetic biology strategies for production of fuels and chemicals. Curr. Opin. Chem. Eng. 1, 380–395. doi: 10.1016/j.coche.2012.07.005
Furdui, C., and Ragsdale, S. W. (2000). The role of pyruvate ferredoxin oxidoreductase in pyruvate synthesis during autotrophic growth by the Wood-Ljungdahl pathway. J. Biol. Chem. 275, 28494–28499. doi: 10.1074/jbc.M003291200
Glenk, G., and Reichelstein, S. (2019). Economics of converting renewable power to hydrogen. Nat. Energy 4, 216–222. doi: 10.1038/s41560-019-0326-1
Götz, M., Lefebvre, J., Mörs, F., McDaniel Koch, A., Graf, F., Bajohr, S., et al. (2016). Renewable power-to-gas: a technological and economic review. Renew. Energy 85, 1371–1390. doi: 10.1016/j.renene.2015.07.066
Groher, A., and Weuster-Botz, D. (2016). Comparative reaction engineering analysis of different acetogenic bacteria for gas fermentation. J. Biotechnol. 228, 82–94. doi: 10.1016/j.jbiotec.2016.04.032
Haas, T., Krause, R., Weber, R., Demler, M., and Schmid, G. (2018). Technical photosynthesis involving CO2 electrolysis and fermentation. Nat. Catal. 1, 32–39. doi: 10.1038/s41929-017-0005-1
Handler, R. M., Shonnard, D. R., Griffing, E. M., Lai, A., and Palou-Rivera, I. (2016). Life cycle assessments of ethanol production via gas fermentation: anticipated greenhouse gas emissions for cellulosic and waste gas feedstocks. Ind. Eng. Chem. Res. 55, 3253–3261. doi: 10.1021/acs.iecr.5b03215
Henry, A., and Martin, O. C. (2016). Short relaxation times but long transient times in both simple and complex reaction networks. J. R. Soc. Interface 13:20160388. doi: 10.1098/rsif.2016.0388
Hess, V., Schuchmann, K., and Müller, V. (2013). The ferredoxin: NAD+ oxidoreductase (Rnf) from the acetogen acetobacterium woodii requires na+ and is reversibly coupled to the membrane potential. J. Biol. Chem. 288, 31496–31502. doi: 10.1074/jbc.M113.510255
Hoffmeister, S., Gerdom, M., Bengelsdorf, F. R., Linder, S., Flüchter, S., Öztürk, H., et al. (2016). Acetone production with metabolically engineered strains of Acetobacterium woodii. Metab. Eng. 36, 37–47. doi: 10.1016/j.ymben.2016.03.001
Hoskisson, P. A., and Hobbs, G. (2005). Continuous culture - making a comeback? Microbiology 151, 3153–3159. doi: 10.1099/mic.0.27924-0
Hu, P., Chakraborty, S., Kumar, A., Woolston, B. M., Liu, H., Emerson, D., et al. (2016). Integrated bioprocess for conversion of gaseous substrates to liquids. Proc. Natl. Acad. Sci. U.S.A. 113, 2–7. doi: 10.1073/pnas.1516867113
Igamberdiev, A. U., and Kleczkowski, L. A. (2009). Metabolic systems maintain stable non-equilibrium via thermodynamic buffering. Bioessays 31, 1091–1099. doi: 10.1002/bies.200900057
Infantes, A., Kugel, M., and Neumann, A. (2020). Effect of cysteine, yeast extract, pH regulation and gas flow on acetate and ethanol formation and growth profiles of clostridium ljungdahlii syngas fermentation. BioRxiv[Preprint]. doi: 10.1101/2020.01.13.904292
International Panel on Climate Change [IPCC] (2014). Climate Change 2014: Mitigation of Climate Change.
IRENA (2017). Renewable Power Generation Costs in 2017. Abu Dhabi: International Renewable Energy Agency.
Jones, S. W., Fast, A. G., Carlson, E. D., Wiedel, C. A., Au, J., Antoniewicz, M. R., et al. (2016). CO2 fixation by anaerobic non-photosynthetic mixotrophy for improved carbon conversion. Nat. Commun. 7:12800. doi: 10.1038/ncomms12800
Kantzow, C., Mayer, A., and Weuster-Botz, D. (2015). Continuous gas fermentation by Acetobacterium woodii in a submerged membrane reactor with full cell retention. J. Biotechnol. 212, 11–18. doi: 10.1016/j.jbiotec.2015.07.020
Keith, D. W., Holmes, G., Angelo, D. St., and Heidel, K. (2018). A process for capturing CO2 from the atmosphere. Joule 2, 1573–1594. doi: 10.1016/j.joule.2018.05.006
Klask, C., Kliem-kuster, N., Molitor, B., and Angenent, L. T. (2019). An open-source multiple-bioreactor system for replicable gas- fermentation experiments: nitrate feed results in stochastic inhibition events, but improves ethanol production of Clostridium ljungdahlii with CO2 and H2. BioRxiv[Preprint]. doi: 10.1101/2019.12.15.877050
Liew, F. M., Martin, E., Tappel, R., Heijstra, B., Mihalcea, C., and Köpke, M. (2016). Gas fermentation – a flexible platform for commercial scale production of low carbon fuels and chemicals from waste and renewable feedstocks. Front. Microbiol. 7:694. doi: 10.3389/fmicb.2016.00694
Ma, Y., Yu, G., and Li, H. Z. (2005). Note on the mechanism of interfacial mass transfer of absorption processes. Int. J. Heat Mass Transf. 48, 3454–3460. doi: 10.1016/j.ijheatmasstransfer.2005.03.008
Mock, J., Zheng, Y., Mueller, A. P., Ly, S., Tran, L., Segovia, S., et al. (2015). Energy conservation associated with ethanol formation from H2and CO2in Clostridium autoethanogenum involving electron bifurcation. J. Bacteriol. 197, 2965–2980. doi: 10.1128/JB.00399-15
Molitor, B., Mishra, A., and Angenent, L. T. (2019). Power-to-protein: converting renewable electric power and carbon dioxide into single cell protein with a two-stage bioprocess. Energy Environ. Sci. 12, 3515–3521. doi: 10.1039/C9EE02381J
Morinaga, T., and Kawada, N. (1990). The production of acetic acid from carbon dioxide and hydrogen by an anaerobic bacterium. J. Biotechnol. 14, 187–194. doi: 10.1016/0168-1656(90)90007-X
Müller, V. (2019). New horizons in acetogenic conversion of one-carbon substrates and biological hydrogen storage. Trends Biotechnol. 37, 1344–1354. doi: 10.1016/j.tibtech.2019.05.008
Orth, J. D., and Palsson, B. Ø. (2011). What is flux balance analysis? Nat. Biotechnol. 28, 245–248. doi: 10.1038/nbt.1614
Otto, A., Grube, T., Schiebahn, S., and Stolten, D. (2015). Closing the loop: captured CO2 as a feedstock in the chemical industry. Energy Environ. Sci. 8, 3283–3297. doi: 10.1039/C5EE02591E
Park, J. O., Liu, N., Holinski, K. M., Emerson, D. F., Qiao, K., Woolston, B. M., et al. (2019). Synergistic substrate cofeeding stimulates reductive metabolism. Nat. Metab. 1, 643–651. doi: 10.1038/s42255-019-0077-0
Pierce, E., Xie, G., Barabote, R. D., Saunders, E., Han, C. S., Detter, J. C., et al. (2008). The complete genome sequence of Moorella thermoacetica (f. Clostridium thermoaceticum). Environ. Microbiol. 10, 2550–2573. doi: 10.1111/j.1462-2920.2008.01679.x
Poehlein, A., Cebulla, M., Ilg, M. M., Bengelsdorf, F. R., Schiel-bengelsdorf, B., Whited, G., et al. (2015). The complete genome sequence of Clostridium aceticum: a missing link between Rnf- and cytochrome-containing autotrophic acetogens. MBio 6:e01168-15. doi: 10.1128/mBio.01168-15
Qian, H., and Beard, D. A. (2005). Thermodynamics of stoichiometric biochemical networks in living systems far from equilibrium. Biophys. Chem. 114, 213–220. doi: 10.1016/j.bpc.2004.12.001
Quéméner, E. D. Le., and Bouchez, T. (2014). A thermodynamic theory of microbial growth. ISME J. 8, 1747–1751. doi: 10.1038/ismej.2014.7
Redl, S., Diender, M., Jensen, T. Ø., Sousa, D. Z., and Nielsen, A. T. (2017). Exploiting the potential of gas fermentation. Ind. Crops Prod. 106, 21–30. doi: 10.1016/j.indcrop.2016.11.015
Richter, H., Molitor, B., Wei, H., Chen, W., Aristilde, L., and Angenent, L. T. (2016). Ethanol production in syngas-fermenting Clostridium ljungdahlii is controlled by thermodynamics rather than by enzyme expression. Energy Environ. Sci. 9, 2392–2399. doi: 10.1039/C6EE01108J
Sakai, S., Nakashimada, Y., Inokuma, K., Kita, M., Okada, H., and Nishio, N. (2005). Acetate and ethanol production from H2 and CO2 by Moorella sp. using a repeated batch culture. J. Biosci. Bioeng. 99, 252–258. doi: 10.1263/jbb.99.252
Sandoval-Espinola, W. J., Chinn, M. S., Thon, M. R., and Bruno-Bárcena, J. M. (2017). Evidence of mixotrophic carbon-capture by n-butanol-producer Clostridium beijerinckii. Sci. Rep. 7, 1–13. doi: 10.1038/s41598-017-12962-8
Schuchmann, K., and Müller, V. (2014). Autotrophy at the thermodynamic limit of life: a model for energy conservation in acetogenic bacteria. Nat. Rev. Microbiol. 12, 809–821. doi: 10.1038/nrmicro3365
Standing, C. N., Fredrickson, A. G., and Tsuchiya, H. M. (1972). Batch- and continuous-culture transients for two substrate systems. Appl. Microbiol. 23, 354–359. doi: 10.1128/AEM.23.2.354-359.1972
Tizard, J. H., and Sechrist, P. A. (2015). Carbon Capture in Fermentation. U.S. Patent No 0,111,266. Washington, DC: U.S. Patent and Trademark Office. Available online at: https://patents.justia.com/patent/20150111266
Valgepea, K., De Souza Pinto Lemgruber, R., Abdalla, T., Binos, S., Takemori, N., Takemori, A., et al. (2018). H2 drives metabolic rearrangements in gas-fermenting Clostridium autoethanogenum. Biotechnol. Biofuels 11:55. doi: 10.1186/s13068-018-1052-9
Keywords: gas fermentation, Clostridium autoethanogenum, carbon dioxide, valorization, carbon recycling, fuel and chemical platforms
Citation: Heffernan JK, Valgepea K, de Souza Pinto Lemgruber R, Casini I, Plan M, Tappel R, Simpson SD, Köpke M, Nielsen LK and Marcellin E (2020) Enhancing CO2-Valorization Using Clostridium autoethanogenum for Sustainable Fuel and Chemicals Production. Front. Bioeng. Biotechnol. 8:204. doi: 10.3389/fbioe.2020.00204
Received: 18 July 2019; Accepted: 02 March 2020;
Published: 27 March 2020.
Edited by:
Christoph Herwig, Vienna University of Technology, AustriaReviewed by:
Claudio Martin Pereira De Pereira, Federal University of Pelotas, BrazilFrank Robert Bengelsdorf, University of Ulm, Germany
Copyright © 2020 Heffernan, Valgepea, de Souza Pinto Lemgruber, Casini, Plan, Tappel, Simpson, Köpke, Nielsen and Marcellin. This is an open-access article distributed under the terms of the Creative Commons Attribution License (CC BY). The use, distribution or reproduction in other forums is permitted, provided the original author(s) and the copyright owner(s) are credited and that the original publication in this journal is cited, in accordance with accepted academic practice. No use, distribution or reproduction is permitted which does not comply with these terms.
*Correspondence: Esteban Marcellin, ZS5tYXJjZWxsaW5AdXEuZWR1LmF1
†These authors have contributed equally to this work