- 1Institute for Bioengineering, School of Engineering, The University of Edinburgh, Edinburgh, United Kingdom
- 2Centre for Synthetic and Systems Biology (SynthSys), The University of Edinburgh, Edinburgh, United Kingdom
As the fastest mode of transport, the aircraft is a major driver for globalization and economic growth. The development of alternative advanced liquid fuels is critical to sustainable development within the sector. Such fuels should be compatible with existing infrastructure and derived from second generation feedstocks to avoid competition with food markets. With properties similar to petroleum based fuels, isoprenoid derived compounds such as limonene, bisabolane, farnesane, and pinene dimers are of increasing interest as “drop-in” replacement jet fuels. In this review potential isoprenoid derived jet fuels and progress toward their microbial production was discussed in detail. Although substantial advancements have been achieved, the use of first generation feedstocks remains ubiquitous. Lignocellulosic biomass is the most abundant raw material available for biofuel production, however, technological constraints associated with its pretreatment and saccharification hinder its economic feasibility for low-value commodity production. Non-conventional microbes with novel characteristics including cellulolytic bacteria and fungi capable of highly efficient lignocellulose degradation and xylose fermenting oleaginous yeast with enhanced lignin-associated inhibitor tolerance were investigated as alternatives to traditional model hosts. Finally, innovative bioprocessing methods including consolidated bioprocessing and sequential bioreactor approaches, with potential to capitalize on such unique natural capabilities were considered.
Introduction
Transportation is critical to sustainable development and global economic growth. However, despite efforts to develop alternative fuels, reliance on fossil fuels is ongoing. In 2016, just 7.1% of all transport fuel consumed in the European Union was from a renewable source and the transport sector contributed to 27% of greenhouse gas emissions. Similarly, in the United States, biofuels currently make up just 5% of transportation fuel consumption (U.S. Energy Information Administration, 2019). Globally, bioethanol is the most widely used biofuel due to its high level natural production by microbes (Mohd Azhar et al., 2017). However, the majority is currently derived from corn or sugarcane feed stocks, which utilize vast areas of agricultural land and threaten food security. Biodiesel, produced through the transesterification of vegetable oils, is the predominant renewable transport fuel in Europe. Such biodiesel is also a first generation biofuel, requiring agricultural land that could otherwise be used to grow food crops. There is an increasing interest in low emission electric vehicles due to their reduced contribution to urban air pollution with a number of countries offering incentives for electric vehicles (UK Government (Department for Transport), 2020, 2018; Sherlock, 2019). In addition, companies including Rolls Royce, Airbus, Eviation and magniX are driving innovation to develop all electric aircrafts. Recently Eviation (2019) announced the development of Alice, a nine passenger aircraft with a range of 650 miles. Although this represents a major technological breakthrough, the limited capacity of current battery technology is a major bottleneck. The state-of-the-art lithium ion batteries used to power Alice have an energy density of 248 Wh/kg (KOKAM, 2019), which is just 2% of that of Jet A (Table 1), substantial advancements in lightweight energy storage technology are therefore necessary. As a result, for aviation, shipping and heavy good vehicles, reliance on liquid fuels is likely to continue for some time (The Royal Academy of Engineering, 2017).
The aircraft remains the fastest and most convenient means of transporting people and goods. As the aviation industry facilitates global business, international trade and tourism, it is a critical component of the global economy. The sector is experiencing immense growth and annual air passengers are expected to almost double to 7.8 billion by 2036. As air transport improves access to food, medication and educational resources, it is vital for the implementation of many of the UN’s 17 Sustainable Development Goals. However, if the environmental cost is to be eliminated, the introduction of sustainable alternatives to petroleum based aviation fuels is critical. This demand for sustainable advanced biofuels is widely recognized by national governments (UK Government (Department for Transport), 2018; Governement of India (Ministry of New and Renewable Energy), 2019), influential multinational organizations (United Nations, 2015; The European Parliament and of the Council of the European Union, 2018) and global oil and gas companies (ExxonMobil, 2018; Royal Dutch and Shell, 2019). The European Parliament and of the Council of the European Union (2018) recently introduced stringent renewable energy targets, requiring at least 32% of Europe’s energy be derived from a renewable source by 2030. In addition, first generation biofuel production must be restricted to a sustainable level of just 3.8%. The international air transport association (IATA) is a trade association with 290 airline members, representing 82% of the world’s air traffic. They have also set a number of ambitious targets for their member organizations, including a cap on net CO2 emissions from 2020 to promote carbon neutral growth and a 50% reduction in net CO2 emissions by 2050 compared to 2005 levels. The implementation of sustainable aviation fuels is imperative to the achievement of such goals. To be compatible with existing infrastructure, such fuels should have similar properties to traditional fuels (Table 1). Although similar to those used in diesel engines, a lower freezing point is paramount for aviation fuels to withstand the extreme cold.
The potential of natural medium chain length isoprenoid and fatty acid derived hydrocarbons to meet such requirements is of growing research interest (Lee et al., 2008; Jiménez-Díaz et al., 2017). Isoprenoids are the largest and most diverse class of naturally occurring organic compounds. They have a vast range of applications in the pharmaceutical, fragrance and fuel industries and have enriched the everyday lives of humans for millennia (Peralta-Yahya et al., 2011; George et al., 2015). Isoprenoid research predominantly focused on medicinal applications such as the construction of microbial pathways for the ground-breaking antimalarial (Paddon and Keasling, 2014) and anticancer drugs (Wong et al., 2018; Nowrouzi et al., 2020; Walls et al., 2020). However, the aforementioned introduction of stringent greenhouse gas emission reduction targets in recent years has increased interest in their potential as advanced biofuels (Peralta-Yahya et al., 2012; George et al., 2015; Wong et al., 2017). Isoprenoids are formed through the condensation of two or more isoprene units and are classified based on the number of units they contain (Srivastava, 2002). Many isoprenoids have great potential as high-density fuels due to their compact cyclic structures, especially those in which ring strain contributes to a high heat of combustion. The physical properties and high energy contents of many isoprenoids (Peralta-Yahya et al., 2012) are synonymous to those of petroleum-based fuels (Table 1). Monoterpene (C10) and sesquiterpene (C15) compounds have carbon chain lengths in the range of conventional jet fuels (Table 1) and are therefore of particular interest as potential replacements. Such compounds are derived from geranyl diphosphate and farnesyl diphosphate, respectively, products of the mevalonate (MVA) and MEP pathways in plants (Figure 1).
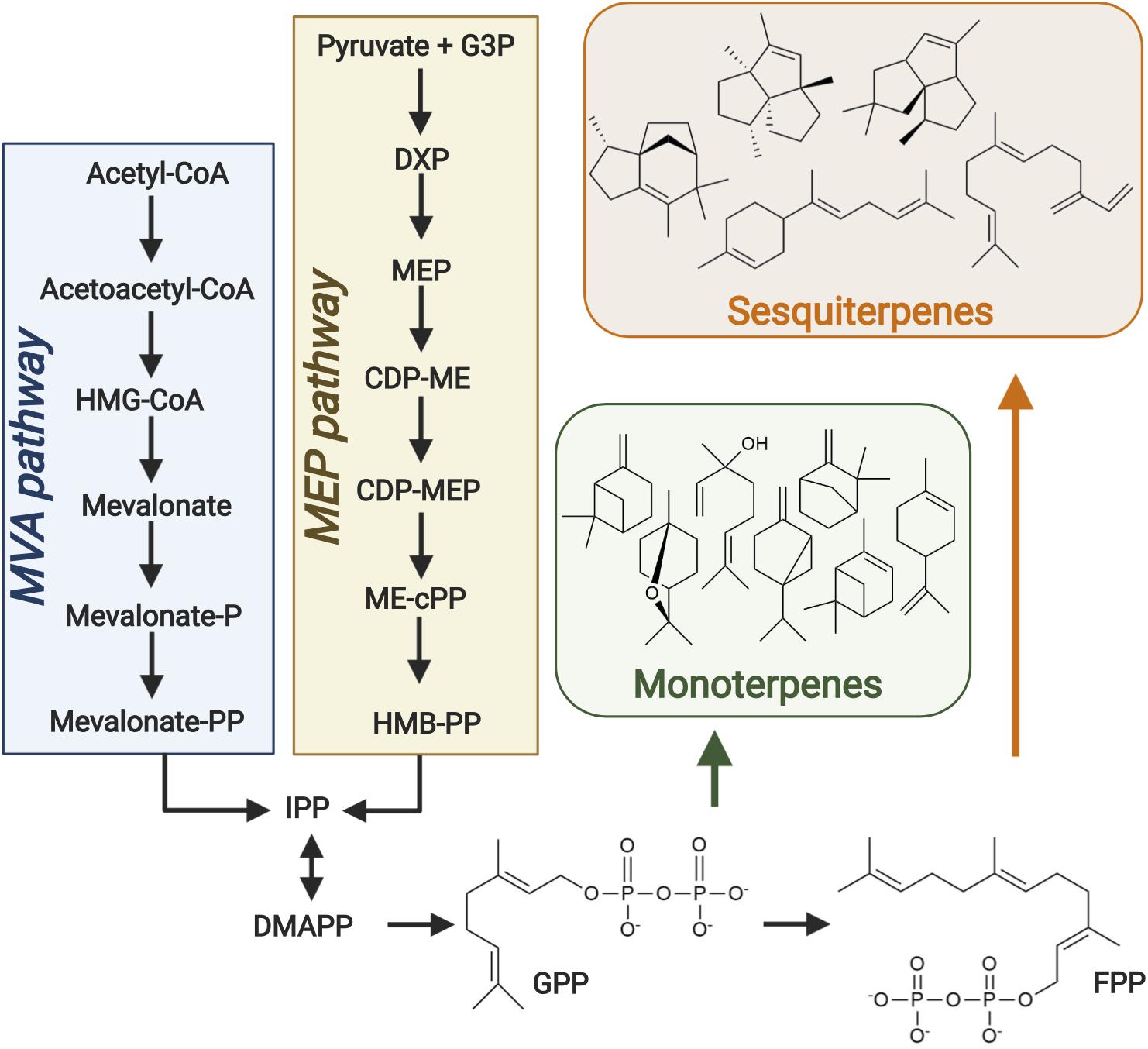
Figure 1. Isoprenoid biosynthesis. The universal monoterpene and sesquiterpene precursors geranyl diphosphate (GPP) and farnesyl diphosphate (FPP), respectively, are produced via both the mevalonate (MVA) and MEP pathways in plants. Terpene synthases then convert such precursors into a wide range of monoterpene and sesquiterpene compounds. Figure created with BioRender.com.
Low quantities of isoprenoids typically accumulate in their natural hosts. As a result, the direct extraction and isolation of the compounds is low yielding and therefore cannot be employed for large scale production. In addition, slow plant growth and susceptibility to seasonal variations further limit extraction from plant sources (Chang and Keasling, 2006; Tippmann et al., 2013). Chemical synthesis presents an alternative method for isoprenoid production. However, the complex stereochemistry of isoprenoids complicates enantioselective synthesis and typically results in high costs and undesirable yields (Immethun et al., 2013; Tippmann et al., 2013). In addition, the hazardous solvents required for chemical synthesis pose serious health and safety as well as environmental risks (Tippmann et al., 2013).
The development of strategically designed microbial cell factories for the heterologous biosynthesis of isoprenoids from low cost, renewable feedstocks has the potential to provide a sustainable solution. Thanks to advancements in synthetic and systems biology technology, progress toward the construction of such cell factories has accelerated recently and microbial production of a wide range of potential isoprenoid biojet fuel feedstocks has now been demonstrated at laboratory scale (Peralta-Yahya et al., 2011; Brennan et al., 2015; Mendez-Perez et al., 2017; Liu et al., 2018, Liu Y. et al., 2019). However, the development and scale up of economical bioprocesses for such cell factories at industrial scale remains a monumental challenge, hindering the realization of these wide-ranging benefits. One of the greatest challenges in the development of effective bioprocesses for the isoprenoid candidates, is the low value of the respective advanced biojet fuel products. In order to be economically viable at commercial scale, yields of the potential jet fuels must be substantially greater than for higher value products such as pharmaceuticals. Cell factories for biojet fuels are therefore likely to require more extensive and complex metabolic engineering (Meadows et al., 2016). In addition, the use of first-generation feedstocks, which are inherently favored by most microbial hosts, is unlikely to be feasible due to their high cost and competition with food markets. Second generation feedstocks such, which are derived from non-edible biomass sources such as lignocellulosic biomass, is the most abundant feedstock available for microbial biofuel production. As a low cost, renewable carbon source, lignocellulosic biomass is a highly attractive carbon source, however, expensive pretreatment requirements and the presence of inhibitory compounds has complicated its application to date. Novel third and fourth generation feedstocks are gaining increasing interest as feedstocks for microbial isoprenoid jet fuel production. Such generation fuels are derived from phototrophic microorganisms such as algae and cyanobacteria and therefore provide the additional environmental benefit of CO2 sequestration. However, as they are limited by the rate of photosynthesis, ensuring sufficient yields for commercial scale production is problematic.
In this review potential isoprenoid derived biojet fuel candidates and the challenges associated with the development of sustainable bioprocesses for their industrial-scale production will be discussed. Key bottlenecks including microbial host selection, feedstock selection and pretreatment requirements will be considered along with progress toward their alleviation. Finally, recent progress toward consolidated bioprocessing (CBP) will be discussed along with alternative sequential bioreactor approaches.
Isoprenoid Aviation Fuel Candidates
Monoterpene Biojet Fuels
Monoterpene are formed of two isoprene units and may be either acyclic or cyclic, however, cyclic monoterpenes are more abundant in nature and have found more industrial applications (Kang and Lee, 2016). The C10 hydrocarbon chain length of monoterpenes is in the desirable range of traditional jet fuels (C8–C16; Peralta-Yahya et al., 2012). The monocyclic monoterpene, limonene, is a promising feedstock for the biofuel industry. It is found naturally in the peel of citrus fruits and exists as two enantiomers, D-limonene which has a characteristic orange fragrance and L-limonene which has a turpentine like odor. D-limonene is a by-product of the citrus juice industry (Jongedijk et al., 2016) and has applications in the fragrance industry within perfumes and as a solvent in cleaning products. It is also a useful feedstock in the food and pharmaceutical industries (Sun, 2007). In contrast, L-limonene is predominantly used as a precursor for the biosynthesis of S-menthol, a major component of mint (Lin et al., 2017). Interest in limonene is growing as a potential bio-jet fuel candidate (Chuck and Donnelly, 2014; Zargar et al., 2017). The distillation profile of limonene is similar to that of the aviation fuel jet A-1 in blends up to 20% limonene. As a result, its blend with jet fuel would have minimal deleterious effects upon combustion quality and efficiency (Chuck and Donnelly, 2014). Limonene was found to be an excellent substitute for aviation kerosene and could potentially be used as a drop-in replacement without the need for chemical upgrading (Chuck and Donnelly, 2014). Its lower viscosity could also provide additional benefits such as increased atomization and pumping (Chuck and Donnelly, 2014).
The oxygenated monoterpenoid, eucalyptol (1,8-cineole), is native to a number of species of the Eucalyptus genus and is widely used across the pharmaceutical, fragrance and flavoring industries (Mendez-Perez et al., 2017). More recently is has been shown to be a useful intermediate in the synthesis of p-cymene an important component of the biojet fuel blend AMJ-700t (10% cymene, 50% limonene, and 40% farnesene) (Brennan et al., 2015; Mendez-Perez et al., 2017). After a single hydrogenation step AMJ-700t can be converted into AMJ-700, the biojet fuel produced by Amyris, which successfully fueled an Azul Brazilian Airlines demonstration flight in 2012 (Brennan et al., 2015). Both eucalyptol and limonene can be chemically upgraded to the potential jet fuel blendstock, p-menthane, which has a high energy density and desirable low freeze point (Table 2). In the case of limonene this involves a single hydrogenation step, while eucalyptol must undergo ring opening, dehydration and hydrogenation to yield p-menthane (Figure 2; Baral et al., 2019). This reliance on a series of reactions for the conversion of eucalyptol has resulted in poor selectivity toward p-menthane (Yang et al., 2017). In order to tackle this, Yang et al. (2017), developed a “one-pot” biphasic tandem catalysis process to consolidate the three steps into a single unit operation for improved efficiency and selectivity. The resulting process achieved >99% conversion of eucalyptol to p-menthane at 120°C and 10 bar in just 1 h.
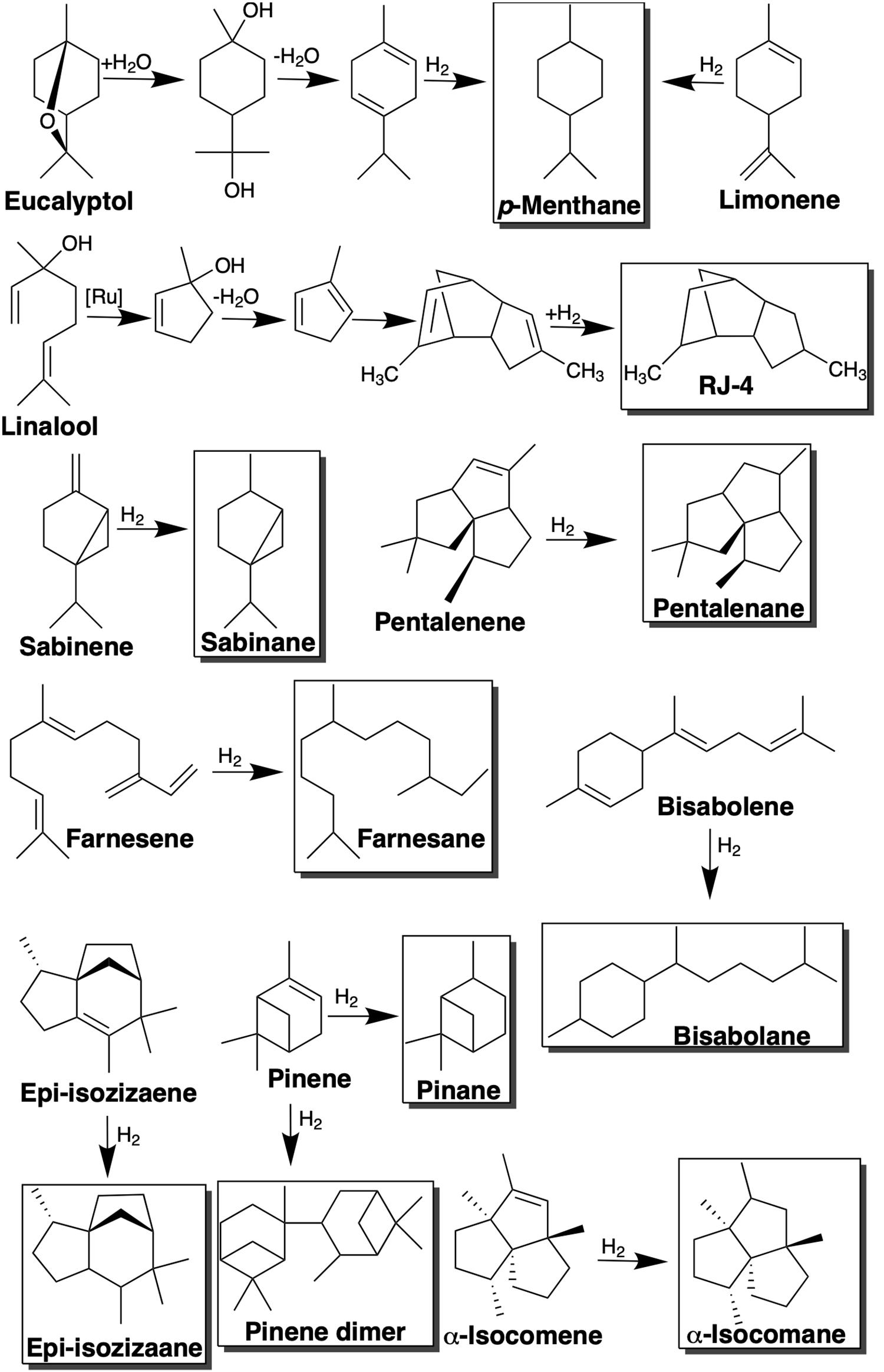
Figure 2. Conversion of monoterpene and sesquiterpene compounds into jet fuel blendstocks via hydrogenation, dimerization, or a combination of isomerization, dehydration, dimerization, and hydrogenation steps.
The acyclic monoterpene, linalool, is another industrially important fragrance chemical, which has also been used as a precursor of the high-density fuel, RJ-4, used in missiles and as a component of jet fuel (Mendez-Perez et al., 2017). Through a series of isomerization, dehydrogenation, dimerization and hydrogenation steps, linalool can be chemically upgraded to RJ-4 as shown in Figure 2. As linalool requires more extensive chemical upgrading to yield RJ-4, the hydrogenation step accounts for a significantly greater proportion of the production costs (∼12%) than for other terpene hydrogenations such as limonene to p-menthane (Baral et al., 2019).
Bicyclic monoterpenes with potential biojet fuel applications include pinene, camphene and sabinene. Pinene is found naturally in the oils of numerous species of coniferous tree and exists as two isomers, α-pinene and β-pinene which are the major constituents of turpentine. The chemical dimerization of β-pinene yields a fuel with a very high volumetric energy, comparable with that of the super-dense military aviation fuel JP-10 (Harvey et al., 2010; Sarria et al., 2014). The strained ring structure of pinenes results in a higher heating value than linear molecules of a similar molecular weight. In addition, the dimerization of pinenes can substantially increase their fuel density. The boiling points of pinene dimers are in the range of 200–350°C making them suitable for diesel and jet fuels (Jung et al., 2016). Pinene trimers, on the other hand, are not suitable for biofuel applications due to their high viscosity and high boiling temperatures. The exocyclic double bond of the β-pinene isomer is preferable for dimerization as it provides an increased reactivity compared α-pinene. The conversion of α-pinene typically results in isomers with lower dimer and oligomer yields than β-pinene (Jung et al., 2016). Although, pinene dimerization can be achieved using a range of homogeneous or heterogeneous catalysts, Jung et al. (2016), observed higher β-pinene conversions with heterogeneous catalysts. Dimerization of camphene, another bicyclic monoterpene common to conifers, yields a fuel with similar properties to JP-10 (Meylemans et al., 2012).
Sabinene is a bicyclic monoterpene which has been isolated from a variety of plants such as holm oak. Its high energy density, low flash point and low freezing point makes it another promising advanced biojet fuel feedstock candidate (Wu et al., 2020). Hydrogenation of sabinene, yields sabinane, a promising jet fuel blendstock due to its high energy density and low viscosity (Table 2).
Sesquiterpene Biojet Fuels
Sesquiterpenes are of growing research interest as potential advanced biojet fuel substitutes as a result of their high energy density and comparable cetane numbers to traditional jet fuels (Liu et al., 2018). Made up of three isoprene units, sesquiterpenes are C15 hydrocarbons and hence also fall in the desirable carbon chain length range of jet fuels (C8–C16; Peralta-Yahya et al., 2012). Since its successful commercial scale microbial production by Amyris, farnesane has become a particularly interesting jet fuel blendstock candidate (Oßwald et al., 2017). It is formed via the hydrogenation of farnesene, a naturally occurring sesquiterpene found in the coating of apples. The density and viscosity of farnesane are similar to those of diesel fuel (Table 1) and with a higher cetane number it displays superior combustion properties (Millo et al., 2014). Farnesane was recently approved for use in commercial jet fuels in blends up to 10% (ASTM International, 2020a). The viscosity of farnesene at −20°C is 14.28 mm2/s, however, above the upper limit of 8 mm2/s for standard jet fuel (ASTM International, 2020a). As a result, it is likely to require blending with other fuels for use in existing infrastructure. Despite this, farnesene can be successfully blended with other isoprenoid compounds to achieve the desired properties. For example, AMJ-700, which is the hydrogenated form of a mixture of farnesene, limonene and cymene as described in section “Monoterpene Biojet Fuels,” has a viscosity of <10 mm2/s at −20°C (Ryder, 2009) and meets the specifications of Jet A (Table 1; ASTM International, 2020a).
Bisabolene is a sesquiterpene found in a number of plant and fungal species. Its hydrogenated form, bisabolane, is another promising jet fuel candidate, its structure combines the combustion properties of a linear alkane with the increased energy density of a cyclic hydrocarbon giving it a comparable energy density to Jet-A fuel (Peralta-Yahya et al., 2011). However, hydrogenation required high pressures of around 82.7 bar and yielded a mixture of geometric isomers (Peralta-Yahya et al., 2011). In addition, as like farnesane, the viscosity of bisabolene is relatively high at ∼17 mm2/s at −20°C (Harvey, 2018), the compound would likely need to be blended with lower viscosity fuels for use in jet engines.
As the net heat of combustion of a fuel is highly dependent on its density, multicyclic sesquiterpenes are likely to be particularly suitable as high energy density fuels (Harrison and Harvey, 2017). Cederwood oil, derived from Redcedar trees, is comprised of the tricyclic sesquiterpenes α-cedrene, β-cedrene, thujopsene and the sesquiterpene alcohol, cedrol (Harrison and Harvey, 2017). Hydrogenated cedarwood oil has a high energy density of 42.67 MJ/kg and a desirable freeze point of < −80°C (Harrison and Harvey, 2017), however, its viscosity is considerably higher than the upper limits for standard jet fuel (ASTM International, 2020a) at 54 mm2/s at −20°C, which may hinder its performance as a drop in replacement. Liu et al. (2018) recently investigated three alternative novel tricyclic sesquiterpenes, epi-isozizaene, pentalenene and α-isocomene as potential jet fuel candidates. With predicted specific energies of 42.58, 42.61, and 42.78 MJ/kg, respectively, following hydrogenation, all three potential sesquiterpene fuels were envisaged to perform similarly to jet fuel A1 (42.8 MJ/kg). However, as successful hydrogenation of these sesquiterpenes is yet to be reported in literature, the potential yields and selectivity’s toward the saturated compound of interest are not known.
Microbial Production of Isoprenoid Jet Fuel Candidates From First Generation Feedstocks
The chemical and physical properties (Table 2) of the monoterpene and sesquiterpene compounds discussed in section “Isoprenoid Aviation Fuel Candidates” are comparable to those of standard jet fuel (Table 1) rendering them inherently suitable for advanced biojet fuel applications. Although such compounds are found in nature, they are typically present in minute quantities in their natural host. Research has therefore focussed on the development of metabolically engineered microbial cell factories for the overproduction of mono and sesquiterpenes for biojet fuel applications. As a result of recent advancements in synthetic and systems biology, the construction and optimization of heterologous pathways in wide ranging microbial hosts is becoming increasingly straightforward. Consequently, substantial progress toward the development of effective cell factories for the production of isoprenoid derived biojet fuel candidates has been achieved in recent times. In this section significant examples of first generation, microbial isoprenoid biofuels will be discussed.
E. coli
Escherichia coli has been successfully engineered to produce a number of isoprenoid jet fuel precursors (Peralta-Yahya et al., 2011; Mendez-Perez et al., 2017; You et al., 2017). Although this species is capable of synthesizing the critical isoprenoid building blocks, IPP and DMAPP, via its native MEP pathway (Figure 1) yields were typically insufficient. It is therefore widely recognized that pathway engineering is critical to ensuring a sufficient precursor pool for high-level heterologous isoprenoid synthesis in the host (Ward et al., 2018). Heterologous expression of the MVA pathway, found in eukaryotes including yeast and plants (Figure 1), has been found to dramatically improve isoprenoid synthesis in E. coli. Unlike the MEP pathway, it is not subject to feedback inhibition in the species allowing much greater precursor accumulation (Liu C.-L. et al., 2019). However, MVA flux must be carefully balanced to minimize accumulation of toxic intermediates (Liu C.-L. et al., 2019).
Peralta-Yahya et al. (2011), expressed an eight gene MVA pathway to convert acetyl-CoA into FPP from a single plasmid in E. coli to provide a sufficient pool of the key sesquiterpene precursor. Co-expression of a second plasmid expressing a bisabolene synthase gene yielded 388 mg/L of the potential jet fuel feedstock. Through a series of metabolic engineering steps including codon optimization, modularization and promoter optimization, bisabolene titers were improved to 912 mg/L (Peralta-Yahya et al., 2011). An analogous method was recently applied for the synthesis of the monoterpenoid biojet fuel precursors, linalool and eucalyptol, in E. coli cell factories (Mendez-Perez et al., 2017). For eucalyptol biosynthesis, eight MVA pathway genes were initially expressed along with cineole synthase from a single plasmid, resulting in an optimal titer of 228 mg/L. Further metabolic engineering efforts to redistribute the pathway genes across two plasmids and balance MVA pathway flux allowed a three-fold improvement in eucalyptol titer to 653 mg/L (Mendez-Perez et al., 2017). Using a similar approach, linalool titers were also improved to a maximum titer of 505 mg/L (Mendez-Perez et al., 2017). This modularization approach was also later applied to produce pinene with an optimized titer of 32 mg/L (Sarria et al., 2014).
Liu et al. (2018), also employed the well established approach of expressing the MVA pathway and terpene synthase genes from separate hosts in E. coli for the production of three novel tricyclic sesquiterpenes, epi-isozizaene, pentalenene, and α-isocomene. Following effective promoter engineering maximum titers of 727.9, 780.3, and 77.5 mg/L were achieved in batch cultures of three engineered strains, respectively.
Recently, Wu et al. (2019), optimized production of another potential monoterpene jet fuel feedstock, limonene, in E. coli cell factories using a modularization approach. The limonene pathway was distributed between three modules, with the upstream module expressing MvaE and MvaS genes, responsible for the conversion of acetyl-CoA to MVA, originating from Enterococcus faecalis. The midstream module then expressed four genes responsible for the conversion of MVA to IPP/DMAPP originating from Saccharomyces cerevisiae. Finally, the downstream module contained the plant GPPS and limonene synthase genes. The up and midstream modules were carefully optimized through RBS and promoter engineering, respectively, with the optimal strain yielding 182 mg/L of limonene. Substituting the GGPP synthase gene for neryl diphosphate (NPP) synthase, which catalyzes the conversion of GPP to the alternative limonene substrate NPP, in the downstream module boosted limonene titers 2.9-fold to 695 mg/L. Finally, through fed batch cultivation of this optimized strain a maximum limonene titer of 1.29 g/L was achieved (Wu et al., 2019).
S. cerevisiae
As in E. coli, pathway engineering is critical to ensuring a sufficient precursor pool for isoprenoid overproduction in S. cerevisiae. Peralta-Yahya et al. (2011), also engineered S. cerevisiae for bisabolene production. Genes encoding a truncated HMG-CoA reductase, FPP synthase and the global sterol pathway transcription regulator were overexpressed, whilst squalene synthase was downregulated to maximize FPP availability. Bisabolene synthesis was subsequently achieved through the expression a bisabolene synthase gene from a high-copy 2 μ plasmid, of the five bisabolene synthase genes tested, the optimal produced a maximum bisabolene titer of 994 mg/L (Peralta-Yahya et al., 2011).
Commercial scale biosynthesis of isoprenoids using microbial cell factories has been achieved using S. cerevisiae. Amyris first highlighted the potential of coupling metabolic engineering and synthetic biology during the semi-synthetic artemisinin project. Artemisinic acid, a key precursor to the antimalarial drug, artemisinin, was produced at commercial scale using a strategically engineered S. cerevisiae strain (Paddon and Keasling, 2014). Through the overexpression of all MVA pathway genes and use of a fed-batch cultivation strategy, impressive amorphadiene titers of around 40 g/L could be achieved (Westfall et al., 2012). This strain was later adapted for production of the advanced biofuel precursor, farnesene. However, as a pharmaceutical product, the value of artemisinin was around $150 per kg (Benjamin et al., 2016), whereas that of jet fuels is considerably lower. Industrially feasible synthesis of lower value commodities therefore requires dramatic production cost reductions. Meadows et al. (2016), identified the low ATP/oxygen ratio of S. cerevisiae as a key bottleneck. Through the introduction of heterologous reaction stoichiometries for acetyl-CoA, redox and sugar dissimilation, the conversion of sugar to farnesene was improved 25% while oxygen demand was reduced by 75% (Meadows et al., 2016). Despite alterations to fundamental cellular processes including glycolysis, the highly engineered strain thrived under harsh industrial conditions. Titers exceeding 130 g/L were achieved using unrefined cane syrup in 200,000 L fermenters (Meadows et al., 2016). Even with substantial progress and dramatic increases in productivity, however, production costs continue to limit farnesene to specialty chemical production.
Yarrowia lipolytica
Yarrowia lipolytica is a non-conventional oleaginous yeast, which has gained substantial interest as a potential model industrial host for the production of lipid-based biofuels as it is able to accumulate large quantities of lipids, utilize low-cost renewable carbon sources, and tolerate wide range of pH and salinities (Liu Y. et al., 2019). Recently, Y. lipolytica was engineered for high level farnesene production (Liu Y. et al., 2019). Initially, three key MVA pathway genes, acetyl-CoA acetyltransferase, HMG-CoA synthase and HMG-CoA reductase were expressed from a linearized plasmid, which was subsequently randomly integrated into the genome of Y. lipolytica. A library of strains harboring these random integrations was constructed and screened for optimal MVA production. Two copies of a farnesene synthase-FPP synthase fusion along with additional copies of ERG12, ERG8, ERG19, IDI, and GPPS were subsequently integrated into the highest producing strain (1.96 g/L MVA). Cultivation of this strain resulted in a maximum farnesene titer of 1.7 g/L compared to 0.13 g/L for farnesene synthase alone. Finally, optimization of the fermentation conditions (pH, DO, and stirring speed), followed by high density fed batch fermentation led to an impressive farnesene titer of 25.55 g/L (Liu Y. et al., 2019).
Microbial Second, Third, and Fourth Generation Isoprenoid Biofuel Synthesis
Although promising titers have been achieved for a number of isoprenoid biojet fuels across different microbial hosts as discussed in section “Microbial Production of Isoprenoid Jet Fuel Candidates From First Generation Feedstocks,” the use of first generation feedstocks remains ubiquitous. As such feedstocks are derived from edible crops, there widespread use results in increased food prices and threatens global food security (Mohr and Raman, 2013). The sustainable industrialization of isoprenoid based renewable jet fuels therefore relies on the use of second, third or fourth generation feedstocks. Such feedstocks are not derived from edible crops and do not compete with food crops for arable land. Figure 3 provides a summary of potential feedstocks for biofuel production and their key features. In this section, recent progress toward the production of biofuels from non-first generation feedstocks is presented.
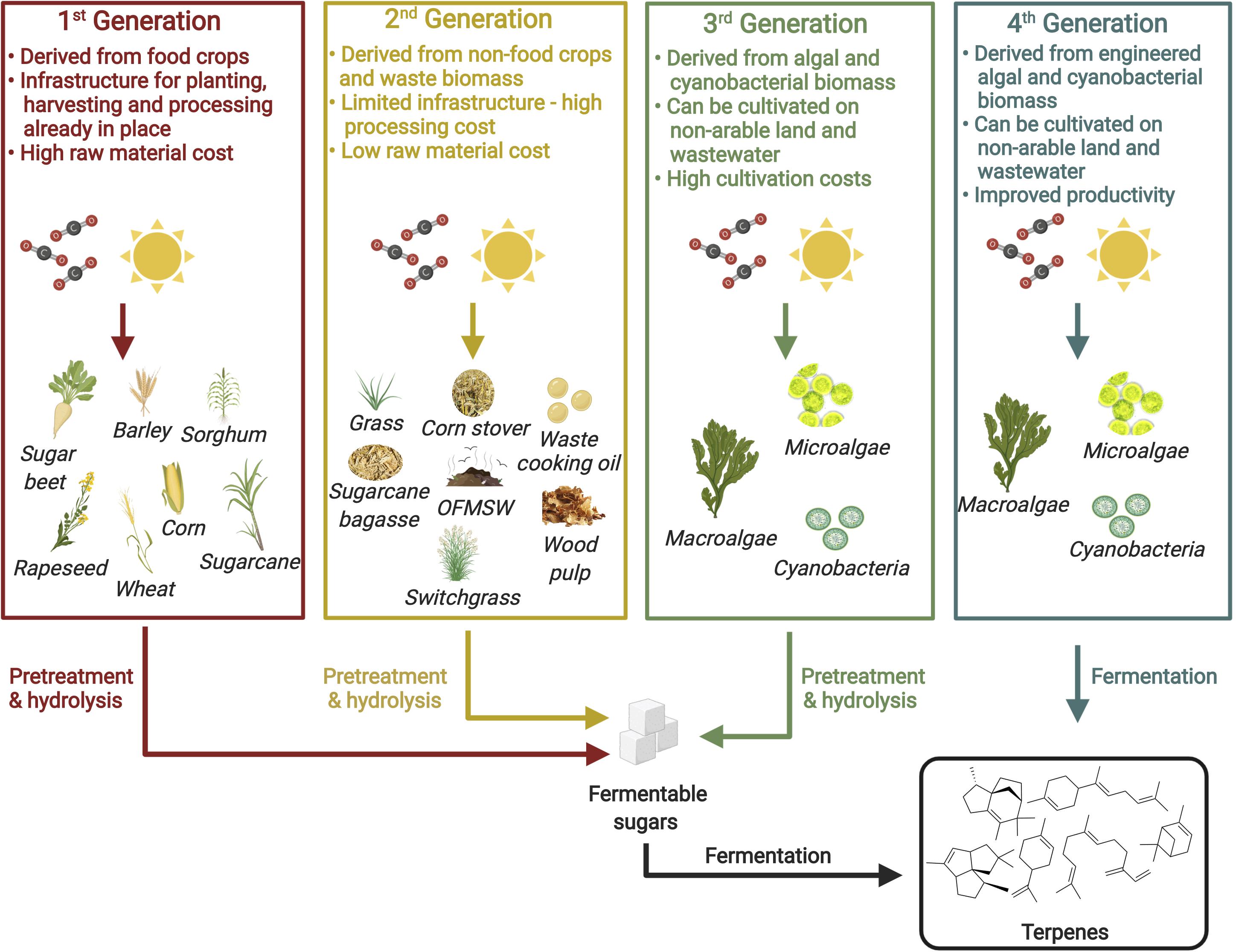
Figure 3. Potential feedstocks for biofuel production. Figure created with BioRender.com.
Second Generation Feedstocks
Lignocellulosic Biomass
Lignocellulosic biomass is the most abundant material on the planet for the production of low-cost commodities such as biofuels (Minty and Lin, 2015). Lignocellulose is comprised of the carbohydrate polymers cellulose and hemicellulose and the aromatic polymer lignin (Abdel-Hamid et al., 2013). In order to be metabolized by microbes the complex polymers must be hydrolyzed to release monomeric sugars. A major challenge in their use as feedstocks is their high xylose content, a pentose sugar which cannot naturally be metabolized by widely used microbial hosts such as E. coli and S. cerevisiae. In addition, pre-treatment leads to the release inhibitory compounds, which can greatly hinder cell viability and productivity. These include phenolic compounds (ferulic acid, p-coumaric acid), furan derivatives (furfural, 5-hydroxymethylfurfural) and small organic acids (levulinic acid, formic acid, and acetic acid) (Wang S. et al., 2017; Navarrete et al., 2020).
The non-conventional carotenogenic yeast Rhodosporidium toruloides, is able to metabolize both xylose and glucose simultaneously (Zhuang et al., 2019). R. toruloides also has the unique ability to metabolize a range of lignin associated aromatics including p-coumaric acid, ferulic acid, vanilic acid, and benzoic acid (Yaegashi et al., 2017). Such compounds inhibit the growth and productivity of S. cerevisiae (Larsson et al., 2000) and E. coli. As a result, this yeast is a promising host for the production of isoprenoids from lignocellulosic feedstocks, eliminating the need for extensive metabolic engineering to improve tolerance or expensive pre-treatment steps when using lignocellulosic feedstocks. As a result, the development of efficient metabolic engineering tools for this host is of growing research interest (Park et al., 2018; Nora et al., 2019).
Lignocellulosic corn stover waste was recently employed as a feedstock for cineole biosynthesis using an engineered R. toruloides strain. The heterologous genes were randomly integrated via NHEJ using an Agrobacterium tumefaciens mediated transformation method (Zhuang et al., 2019). The corn stover was first deacylated in dilute alkali, mechanically refined and enzymatically hydrolyzed to release high concentrations of monomeric (glucose and xylose) sugars. Following growth on this medium the engineered strain produced a maximum cineole titer of 34.6 mg/L (Zhuang et al., 2019). Although titers achieved with R. toruloides to date are lower than those achieved with the model yeast S. cerevisiae, several researchers have developed CRISPR-Cas9 based genome editing methods for R. toruloides, offering increased integration efficiency and targeted multiplex genome editing (Jiao et al., 2019; Otoupal et al., 2019; Schultz et al., 2019). The application of such tools and development of synthetic biology standards (Beal et al., 2020) to construct heterologous pathways for isoprenoid jet fuel candidate biosynthesis has the potential to boost titers from lignocellulose derived feedstocks significantly, thereby improving the economic feasibility of microbial biojet fuel production.
Glycerol
As a major waste product of biodiesel production, crude glycerol is a promising low-cost, second generation feedstock. In a recent study by You et al. (2017), the precursor to the approved jet fuel, farnesane, was produced from glycerol by an engineered E. coli strain. Although, encouraging titers of 8.7 g/L were achieved with pure glycerol, lower titers were achieved in subsequent experiments using crude glycerol. This was attributed to the presence of inhibitory compounds, indicating pre-treatment may be required to maximize productivity on crude glycerol (You et al., 2017). Glycerol was also shown to be preferred carbon source for limonene production by an engineered E. coli strain recently (Rolf et al., 2020). An impressive final limonene titer of 3.6 g/L was achieved in a glycerol limited fed-batch culture of the strain. E. coli has also been engineered for the production of the bicyclic monoterpene sabinene by Zhang et al. (2014). Process optimization studies using the engineered strain revealed the substitution of glucose with glycerol as the carbon source improved sabinene titers two-fold through fed-batch cultivation.
Cao et al. (2016) recently engineered a heterologous limonene pathway in Y. lipolytica. Through the overexpression of HMG1 along with codon optimized NPP and limonene synthase genes, followed by medium optimization, a maximum limonene titer of 23.6 mg/L was achieved from glucose. In a subsequent study by the group, an additional copy of limonene synthase was added to enhance limonene yields (Cheng et al., 2019). Of a range of carbon sources tested, interestingly glycerol was the optimal for limonene biosynthesis, with a maximum titer of 165.3 mg/L achieved in fed-batch fermentation (Cheng et al., 2019).
Waste Cooking Oil
Around 29 million tons of waste cooking oil are generated globally each year (Katre et al., 2017). As a single liter of waste cooking oil can pollute up to 500,000 liters of water (Panadare and Rathod, 2015), its safe disposal is a major environmental challenge. One potential solution, which has gained substantial interest, is the application of waste cooking oil as a feedstock for biodiesel production (Kulkarni and Dalai, 2006; Katre et al., 2017). A number of methods have been developed for the transesterification of the oil to yield biodiesel including alkali, acid and enzymatic transesterification. Alkali-catalyzed transesterification methods are widely used for the conversion of vegetable oil into biodiesel as they are considerably faster than acid catalyzed reactions. Such methods are hindered by the high levels of free fatty acids in waste cooking oil, however, which react with the catalyst forming soap. This prevents glycerol separation and dramatically reduces ester yields (Kulkarni and Dalai, 2006; Katre et al., 2017). Enzyme-catalyzed reactions can be conducted at lower temperatures with reduced pretreatment requirements and are insensitive to free fatty acids, however, the high costs associated with the required enzymes hider commercialization (Kulkarni and Dalai, 2006). In addition, the enzymes may be inactivated by methanol, the most widely used acyl acceptor for transesterification (Kulkarni and Dalai, 2006; Norjannah et al., 2016). As a result of such limitations and the vast quantities of waste cooking oil generated, alternative uses are desirable (Panadare and Rathod, 2015).
As a number of microorganisms are capable of utilizing waste cooking oils as a carbon source, they are gaining interest as an alternative feedstock for the production of heterologous products (Panadare and Rathod, 2015). Pang et al. (2019) recently capitalized on this ability in the non-conventional yeast Y. lipolytica. Using an engineered Y. lipolytica strain, the researchers successfully produced limonene from waste cooking oil. However, the resulting D-limonene and L-limonene titers were low at 2.5 and 2.7 mg/L, respectively, using 70% waste cooking oil as the carbon source. Despite this, titers obtained using cooking oil were over 10% higher than those using glucose indicating titers may be improved through MVA pathway optimization in the strain. Recently, Zhao et al. (2020) overexpressed ten genes of the MVA pathway in Y. lipolytica to enhance heterologous bisabolene synthesis. Expression of a heterologous efflux pump and supplementation of the medium with additional Mg2+ had a positive effect on productivity through reducing toxicity and increasing enzyme co-factor availability, respectively. Using waste cooking oil as a carbon source the optimal engineered strain achieved a maximum bisabolene titer of 973 mg/L (Zhao et al., 2020). A combination of waste cooking oil (carbon source) and corn steep liquor (sugar, nitrogen, amino acids, and vitamins source) has also been employed as a feedstock for the production of carotene by the fungus, Blakeslea trisporai (Nanou et al., 2017). Cultivation in a bubble column reactor resulted in a maximum carotene titer of 980 mg/L. The gram-negative bacterium, Pseudomonas aeruginosa, has also been cultivated on a number of waste oils for the production of biosurfactants (Pérez-Armendáriz et al., 2019). An impressive rhamnolipid titer of 3.6 g/L was achieved when the strain was grown on media containing just waste canola oil and a combination of four salts (Pérez-Armendáriz et al., 2019). Although research into the microbial conversion of waste cooking oil into isoprenoid products is in its infancy, its low cost and high abundance renders it an attractive feedstock for the sustainable production of advanced biojet fuels.
Third and Fourth Generation Feedstocks
Third and fourth generation feedstocks, which are derived from photosynthetic organisms including algae and cyanobacteria, are of growing interest for biofuel production as they exhibit higher rates of photosynthesis and growth than terrestrial plants (Dutta et al., 2014). As phototrophs, the application of such hosts would also eliminate the need for costly carbon sources. Furthermore, many microalgae and cyanobacterial species can be grown in wastewater, where they would utilize harmful nitrogen and phosphorus for growth, resulting in effective bioremediation and a reduced reliance on freshwater (Abedi et al., 2019; Hernández-García et al., 2019). In the case of third generation terpene biojet fuel production the biomass would provide the carbon source for an engineered host (Figure 3). Microalgae, macroalgae and cyanobacterial biomass has been employed for the production of third generation bioethanol (de Farias Silva and Bertucco, 2016; del Río et al., 2019). Following biomass pretreatment and hydrolysis, to degrade the cell wall and release fermentable sugars, the resulting sugar was then fermented by S. cerevisiae yielding bioethanol. Fourth generation terpene biojet fuel production on the other hand, would involve an engineered algae or cyanobacterium capable of synthesizing the terpene of interest directly (Figure 3). As the primary carbon source of such organisms is CO2, the environmental impact of fourth generation biofuels is likely to be reduced dramatically compared to bacterial or yeast hosts. The cyanobacteria, Synechocystis sp., is a non-conventional host under investigation as a potential host for fourth generation advanced biofuel production. Synechocystis sp. was recently engineered for the production of bisabolene, however, even after extensive metabolic engineering efforts, the rate of photosynthesis remained limiting and 36 days of growth were required to produce just 22 mg/L of bisabolene (Sebesta and Peebles, 2020). The species has also been engineered for the production of the monoterpene, limonene, with a maximum titer of 6.7 mg/L achieved following 7 days of cultivation (Lin et al., 2017).
Other microorganisms with potential as third and fourth generation feedstocks include acetogens such as Clostridium autoethanogenum, which are capable of producing ethanol from syngas and industrial waste gasses (Heffernan et al., 2020). Such organisms provide a means of capturing and recycling carbon from waste gases that would otherwise be released into the atmosphere and contribute to environmental pollution. Lanzatech are striving to capitalize on the potential of the species to develop wide-ranging sustainable fuels and chemicals (LanzaTech, 2017). They recently commercialized an innovative waste gas to bioethanol process using C. autoethanogenum (Heffernan et al., 2020). The company have also successfully engineered the species for the production of farnesene from a carbon monoxide containing waste gas (Yiting Chen et al., 2015).
A summary of each of the discussed fuel candidates along with the microbial host and feedstock is provided in Table 3.
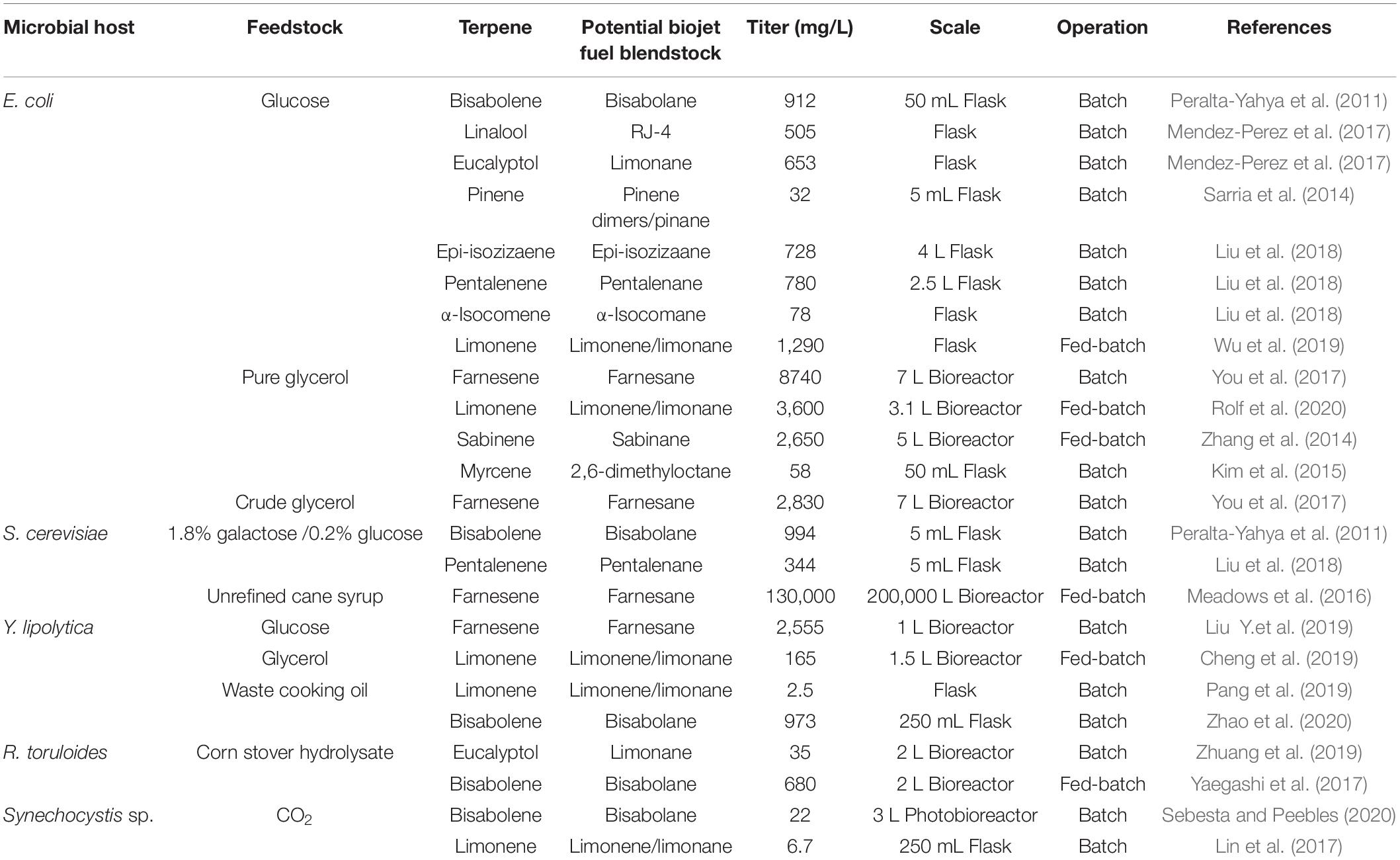
Table 3. Summary of recent progress in microbial biosynthesis of isoprenoid-derived advanced jet fuel candidates.
Bioprocessing Strategies for Biojet Fuel Production From Lignocellulosic Feedstocks
As over half of the carbon in the biosphere is in the form of cellulose, lignocellulosic biomass is the most abundant, low cost feedstock available for commercial microbial biofuel production (Minty and Lin, 2015). However, the high costs associated with its pre-treatment and enzymatic hydrolysis and the inhibitory compounds generated during these steps currently hinders the economic feasibility of their widespread use at commercial scale. Firstly, the biomass is typically subjected to physical, chemical, biological or a combination of the latter pre-treatment steps to separate and solubilize the complex components for improved digestibility (Minty and Lin, 2015; Chen et al., 2017). The pretreated biomass then undergoes saccharification, in which the cellulose and hemicellulose are enzymatically hydrolyzed releasing monomeric sugars. The monomeric sugars can then be metabolized by the engineered host to produce the biojet fuel feedstock of interest (Figure 4). Using a traditional approach each of the aforementioned steps are performed in a separate unit operation (multiple units, Figure 4) resulting in high capital and operational costs thus hindering the economic feasibility of low value commodity production from lignocellulosic feedstocks. Each gram of crystalline cellulose requires 30–50 mg of commercial enzymes (Ali et al., 2016), such high loading requirements and the slow rate of digestion are particular bottlenecks. CBP is a promising alternative approach, in which cellulase production, enzymatic saccharification and microbial fermentation are consolidated in a single unit operation (consolidated bioprocess, Figure 4) (Minty and Lin, 2015). Such approaches could improve the economic feasibility of jet biofuel synthesis through reduced feedstock processing complexity, lower energy requirements and increased conversion efficiency compared to multiple units (Levin et al., 2015).
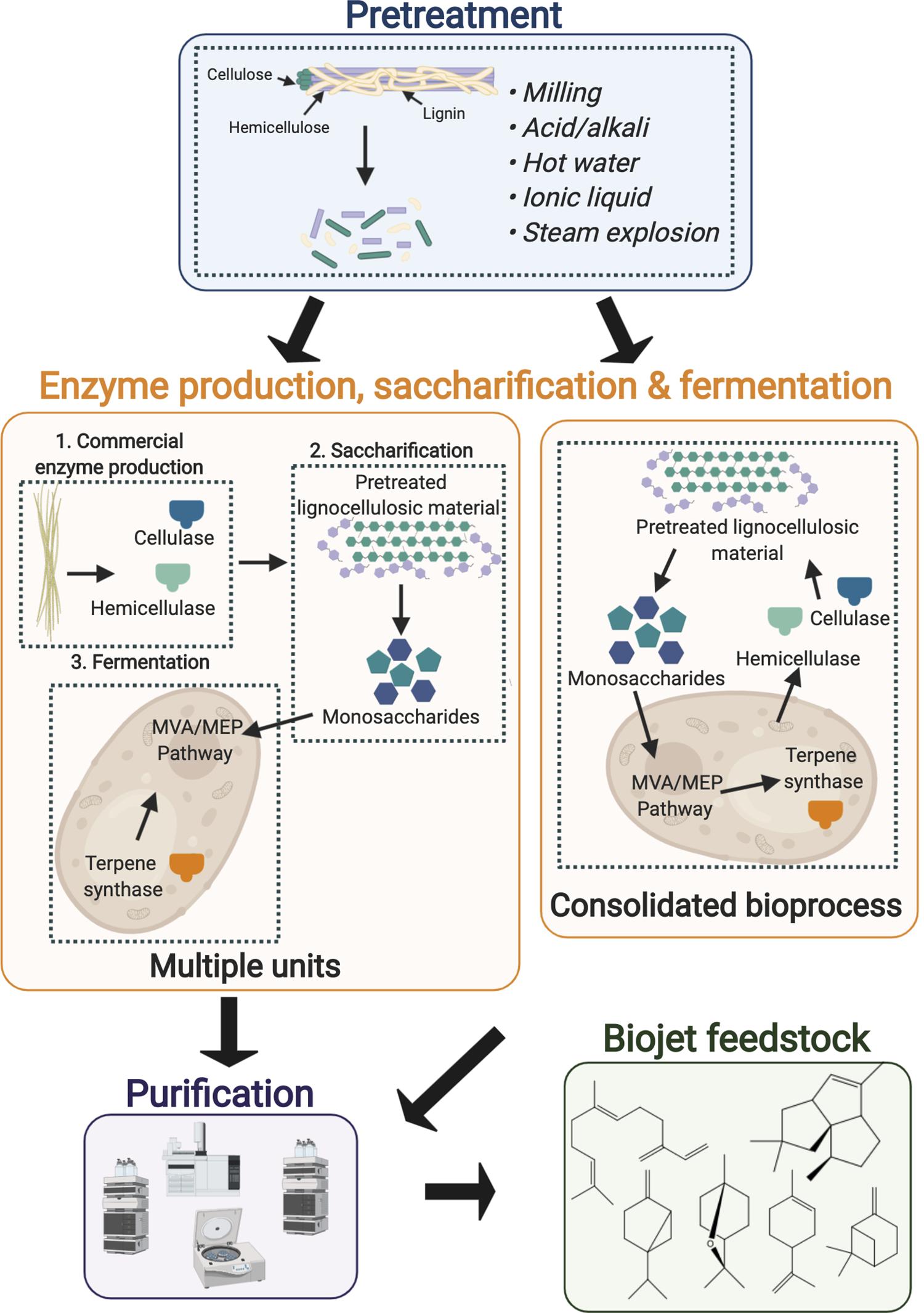
Figure 4. Bioprocessing methods for the conversion of lignocellulosic biomass to advanced jet fuel feedstocks. Firstly the raw biomass is pretreated to reduce crystallinity and lignin content and to increase the surface area prior to enzymatic hydrolysis. The pretreated biomass then undergoes saccharification to release the monomeric sugars, which can be converted into the product of interest by the microbial host. The enzyme production, saccharification and fermentation steps may be performed separately (multiple units) or consolidated into a single unit (consolidated bioprocess). Dashed lines represent single unit operations. Figure created with BioRender.com.
There are three potential approaches to developing a CBP for the microbial conversion of lignocellulosic biomass to advanced jet biofuels as follows:
1. Engineer a naturally highly efficient cellulolytic microbe to produce the desired product
2. Engineer a microbe with a high product titer to express hydrolytic enzymes to efficiently solubilize the lignocellulosic substrate and to consume both hexose and pentose sugars
3. Engineer a microbial consortia allowing labor to be divided across multiple microorganisms
Engineering Cellulolytic Microbial Hosts for Heterologous Terpene Biosynthesis
Filamentous fungi such as Trichoderma are the largest producers of lignocellulolytic enzymes in industry (Liu and Qu, 2019) and produce a wide range of volatile organic compounds (VOC) as secondary metabolites (Stoppacher et al., 2010; Contreras-Cornejo et al., 2014). A significant proportion of these VOCs are terpenes, mostly monoterpenes and sesquiterpenes. For example, Trichoderma atroviride was found to naturally produce a number of the aforementioned advanced biojet fuel candidates including β-farnesene, α-farnesene, and β-bisabolene (Stoppacher et al., 2010). Similarly, a number of endophytic fungi have also been demonstrated to be promising CBP hosts for the production of advanced biofuels (Wu W. et al., 2017). Wu W. et al. (2017), successfully cultivated four endophytic fungi using a number of lignocellulosic materials as the sole carbon sources including switchgrass, corn stover and eucalyptus mill powder. The fungi naturally accumulated a wide range of VOC as secondary metabolites, of which a significant proportion (up to 63%) were terpenes. The majority of the produced terpenes were monoterpenes or sesquiterpenes, and included some known biofuel candidates such as α-pinene, β-pinene, limonene and cineole (Wu W. et al., 2017). In another endophytic fungus, Annulohypoxylon sp., the advanced jet fuel precursor, eucalyptol, comprised up to 94% of the VOCs produced depending on the ligncellulosic waste used as the carbon source (Wang K.Y. et al., 2017). Although relatively low titers will necessitate significant metabolic engineering to reach commercial production levels, the natural ability of certain fungi to both metabolize lignocellulosic materials and synthesize advanced biojet fuel feedstocks renders them excellent candidate CBP hosts.
Recently, Pang et al. (2017) isolated a novel cellulolytic E. coli strain from bovine rumen. With naturally evolved extracellular cellulase ethanol production, the strain effectively metabolized corn straw, representing a promising potential CBP host (Pang et al., 2017). The thermophilic bacteria, C. thermocellumas, is another promising CBP host candidate due to its remarkably high enzymatic hydrolysis efficiency (Akinosho et al., 2014). The species grows well at temperatures between 50 and 68°C, minimizing contamination risks. However, as it is unable to metabolize pentose sugars, further metabolic engineering would likely be necessary to maximize substrate conversion.
The non-conventional yeast Spathaspora passalidarum is capable of fermenting xylose faster than glucose and can co-metabolize glucose and xylose under both aerobic and anaerobic conditions (Chen et al., 2015; Morales et al., 2017). However, the lack of genetic engineering tools and relatively poor tolerance of this strain to lignin associated inhibitory compounds has hindered microbial cell factory development to date. Yaegashi et al. (2017) recently demonstrated a novel single-unit lignocellulose pre-treatment, saccharification, and fermentation process for heterologous bisabolene biosynthesis using the non-conventional yeast, R. toruloides. Initially the corn stover feedstock was pre-treated using the biocompatible ionic liquid, choline α-ketoglutarate, to reduce cellulose crystallinity and lignin content. The pH of the resulting mixture was reduced to five for the subsequent enzyme hydrolysis step, in which cellulase and hemicellulase were added to release the monomeric sugars. The result of this step was a medium containing 17.1 g/L glucose, 9.1 g/L xylose, and 383 mg/L of p-coumaric acid (Yaegashi et al., 2017). Subsequent cultivation of the engineered R. toruloides strain in this medium resulted in a final bisabolene titer of 261 mg/L. Interestingly the titer achieved using this hydrolysate was over two-fold higher than in the synthetic control medium containing equal quantities of glucose, xylose, and p-coumaric acid (Yaegashi et al., 2017). Although this represents substantial progress toward the development of a CBP through combining pre-treatment, saccharification and fermentation into a single unit operation, the process relied on costly commercial enzymes for substrate hydrolysis.
Engineering Model Hosts to Produce Cellulases and Advanced Biofuels
Recently Amyris undertook an ambitious project in collaboration with Renmatix and Total to engineer their industrial farnesene overproducing S. cerevisiae cell factories to grow on lignocellulosic feedstocks with the ultimate goal of reducing production costs to $2 per liter (Mitrovich and Amyris, 2019). This involved extensive metabolic engineering of the S. cerevisiae cell factories to facilitate xylose catabolism along with resistance toward growth inhibitors. However, even following an extensive engineering effort, the predicted minimum production cost was $4–5/L resulting in premature termination of the project.
A number of recombinant S. cerevisiae strains with the ability to metabolize xylose have been successfully constructed. S. cerevisiae was recently engineered for the production of a carotenoid, lycopene, from a mixed glucose-xylose substrate (Su et al., 2020). Through engineering heterologous pathways for xylose consumption and lycopene synthesis a maximum titer of 903 mg/L was achieved in fed-batch culture on the mixed substrate (Su et al., 2020). However, preference for glucose and slow and repressive consumption of xylose during co-consumption of the two sugars remain major bottlenecks (Chen et al., 2015). In addition, such strains typically still rely on the use of commercial enzymes for saccharification.
Saccharomyces cerevisiae was also recently engineered for the heterologous expression of α-amylase and glucoamylase to develop a consolidated enzyme production, saccharification and glucose fermentation process using raw starch as a substrate (Cripwell et al., 2019). The resulting strain achieved yields in excess of 80% of the theoretical maximum.
Division of Labor
Microbial Consortia
Microbial consortia are ubiquitous in nature and play important roles in nutrient recycling and maintaining the health of humans, animals and plants (McCarty and Ledesma-Amaro, 2019). Such organisms are capable of performing tasks too complex for any single organism to complete alone through the strategic division of labor. Many natural consortia are innately capable of converting lignocellulosic biomass to a plethora of natural products, however, the predominant products are organic acids, CO2 and methane which are not suitable for advanced liquid biofuel applications (Zuroff et al., 2013). The assembly of effective synthetic microbial consortia for the pre-treatment, saccharification and fermentation of lignocellulosic biomass into terpene fuels could help alleviate some of the major bottlenecks associated with monocultures. A co-culture of the thermophilic bacteria, C. thermocellum and C. thermolacticum, showed improved ethanol production compared to mono-cultures of the strains (Xu and Tschirner, 2011). This was attributed to combining the higher enzymatic hydrolysis efficiency of C. thermocellum and ability of C. thermolacticum to metabolize all of the hydrolysis products including xylose. The ethanol yield from cellulose of resulting co-culture was 75% of the theoretical maximum (Xu and Tschirner, 2011). An E. coli–E. coli co-culture has been engineered for the conversion of switchgrass biomass into the monoterpene, pinene (Bokinsky et al., 2011). The plant biomass was first pre-treated through dissolution in an ionic liquid to reduce crystallinity and lignin content thereby improving digestibility. Two engineered E. coli strains were subsequently employed for pinene synthesis, the first harboring genes for xylan hydrolysis and the second for pinene synthesis. The resulting co-culture yielded 1.7 ± 0.6 mg/L of pinene (Bokinsky et al., 2011). Although this yield is relatively low, a large proportion of the switch grass was not metabolized indicating the process could be improved through further metabolic engineering.
The construction of stable synthetic consortia is, however, limited by a lack of understanding of how most species interact (Peng et al., 2016). In addition, those strains engineered for high level terpene production are highly domesticated to thrive independently under laboratory conditions, which may hinder their ability to form robust co-cultures with cellulolytic microorganisms.
Division of Labor Across Separate Units
One potential solution recently proposed by Navarrete et al. (2020) involves the use of sequential bioreactors, in the first feedstock degradation occurs, whilst in the second biochemical production is performed. This two stage approach has been demonstrated for lipid biosynthesis (Xu et al., 2015). The first stage involved the anaerobic digestion of macroalgae biomass (30 g/L) by microbes obtained from anaerobic digester sludge from a wastewater treatment plant. The sludge was heat-treated to inactivate methanogens prior to use as the inoculum for the macroalgae fermentation. This produced a mixture of volatile fatty acids (VFAs) (acetic acid 8.24 g/L, propionic acid 1.11 g/L, and butyric acid 0.45 g/L), which subsequently provided the carbon source for a second reactor containing either Y. lipolytica, Cryptococcus curvatus, or Trichosporon dermatis. Of the yeast species investigated, Cr. curvatus and T. dermatis successfully consumed all of the acetic acid during 60 h cultivations and produced 1.3 and 1.3 g/L of lipids, respectively (Xu et al., 2015).
Ruminants are an excellent example of a naturally evolved CBP. The rumen microbiome is a highly evolved symbiotic community of bacteria, fungi, protozoa, bacteriophages and methanogens capable of exceptionally efficient lignocellulose degradation (Ribeiro et al., 2016). This consortia break down the lignocellulosic biomass releasing VFA, mainly acetic, propionic and butyric acids, which are subsequently used as the primary source of carbon by the animal to produce valuable products (i.e., meat and milk). As a result, the rumen is of growing interest as a potential source of CBP hosts and lignocellulose degrading enzymes. Predominant species of ruminal bacteria include Ruminococcus albus, Ru. flavefaciens, and Fibrobacter succinogenes (Weimer et al., 2009; Sharma et al., 2019). The rumen bacteria, Ru. albus has also been employed for the depolymerization of sorghum and bagasse wastes to release fermentable sugars for the subsequent conversion to ethanol by S. cerevisiae (Mutalik et al., 2012). Fermentation of the filtered hydrolyzed wastes resulted in ethanol titers of 17.4 and 19.8 g/L, respectively (Mutalik et al., 2012).
A number of oleaginous yeasts including R. toruloides (Huang et al., 2016), Y. lipolytica (Llamas et al., 2020), Cr. curvatus (Liu et al., 2017), and T. dermatis (Xu et al., 2015) have been employed for the production of lipids using VFA as the sole carbon source. However, optimal conditions vastly different with rumen bacteria performing optimally under anaerobic conditions at temperatures between 37 and 39°C (Sari et al., 2017) and circumneutral pH (Wu C. et al., 2017), while growth of the oleaginous yeast requires aeration and is typically optimal under acidic conditions at around 30°C (Dai et al., 2019; Hackenschmidt et al., 2019). In addition, at high concentrations lignocellulosic hydrolysate components such as acetic acid (∼40 g/L; Huang et al., 2016), formic acid (2 g/L; Zhao et al., 2012). and vanillin (1.5 g/L; Zhao et al., 2012) have an inhibitory effect on R. toruloides. Such discrepancies would likely hinder effective simultaneous enzyme production, saccharification and fermentation to advanced biojet fuels by a consortia of this nature. A sequential bioprocess could therefore be a promising approach for the production of advanced biojet fuels from lignocellulosic biomass using such microbes (Figure 5). In the first reactor a cellulolytic bacterium or consortia would be employed for efficient lignocellulose degradation. F. succinogenes, is a rumen cellulolytic bacterium capable of robust and efficient lignocellulosic biomass degradation (Raut et al., 2019). It ferments the resulting cellobiose and glucose products generating VFAs. Interestingly the species possesses xylanase activity and is therefore capable of converting xylan into xylose despite being unable to metabolize the sugar (Forano et al., 2008; Suen et al., 2011). F. succinogenes could therefore be a promising microorganism for this stage. The resulting product comprised of VFA and xylose would provide the carbon source for a yeast species such as Cr. curvatus or R. toruloides capable of efficient xylose and VFA metabolism and engineered for terpene overproduction in a second reactor. This approach would allow the optimal conditions for each microorganism to be maintained in their respective reactors thereby maximizing productivity. In addition, a fed-batch strategy could be applied for the terpene synthesis stage to prevent VFA and lignin aromatics accumulating to inhibitory concentrations.
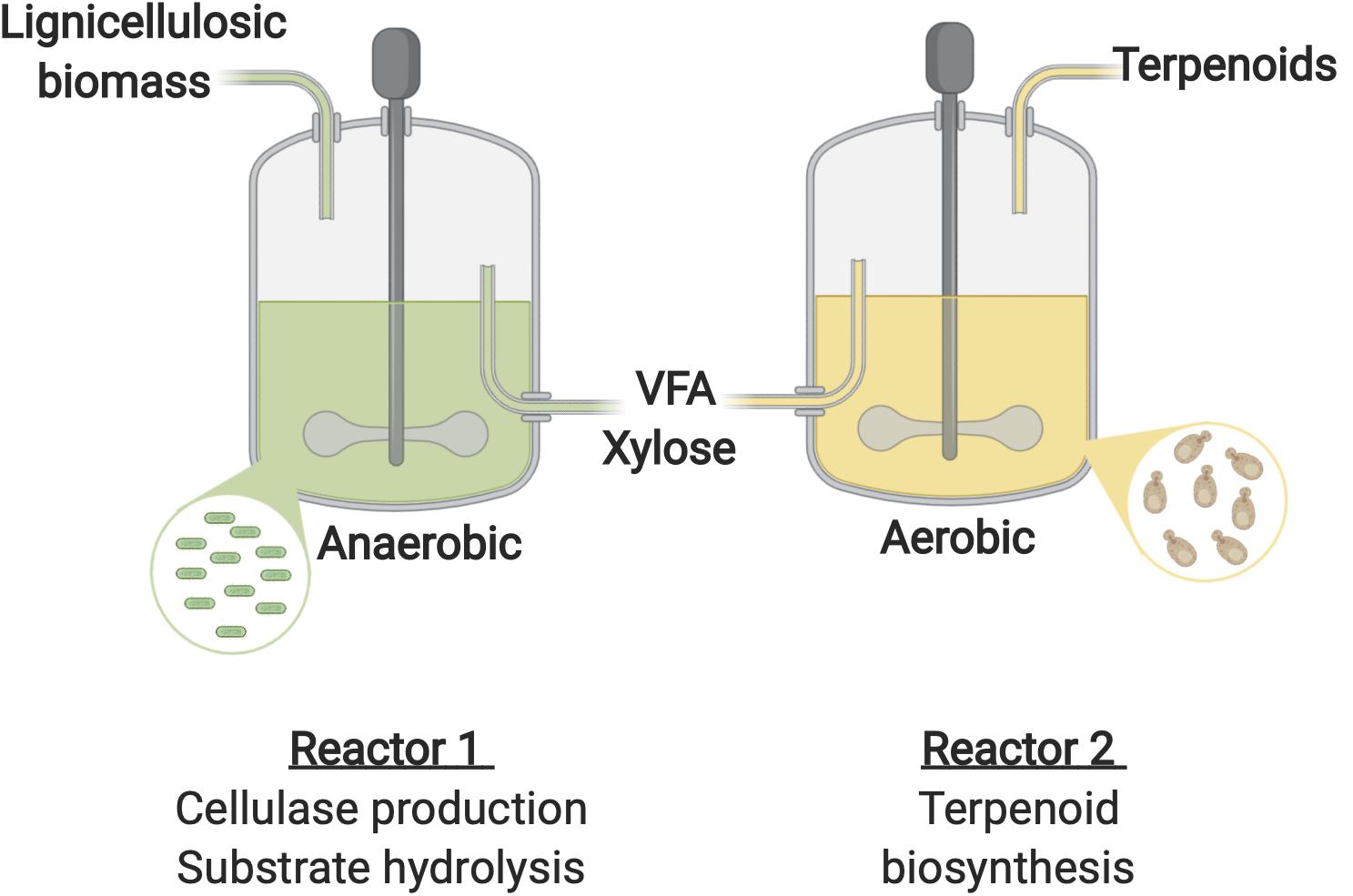
Figure 5. Proposed sequential bioprocessing strategy for terpene production from lignocellulosic biomass. Figure created with BioRender.com.
Although all of the methods discussed in this section, demonstrate potential for improving the sustainability of microbial isoprenoid biojet fuel production, a holistic approach is likely to be necessary to ensure economic viability at industrial scale. Through the coupling of non-conventional hosts, which are naturally capable of growing on abundant, low cost feedstocks and engineered to overproduce the terpene fuel of interest, with strategic bioprocessing methods such as those discussed here, critical commercial bottlenecks such as high raw material and/or pre-treatment costs could be effectively alleviated. Both economic and environmental sustainability would be improved dramatically, allowing the feasibility of industrial scale isoprenoid derived biojet fuel production to be enhanced.
Conclusion
This review focused on potential isoprenoid derived advanced biojet fuel candidates and progress toward their microbial production. Such compounds have properties similar to traditional aviation fuels and are compatible with existing infrastructure. Although extensive progress toward microbial production of isoprenoid derived advanced biofuels has been made recently, the use of first generation feedstocks remains ubiquitous. The development of an economically and environmentally sustainable bioprocess is likely to require the use of waste derived feedstocks. Examples including crude glycerol, waste cooking oil, and lignocellulosic biomass, which have recently been demonstrated as effective substrates for isoprenoid biosynthesis were considered in this review. Emphasis was made on the most abundant renewable feedstock, lignocellulosic biomass and major bottlenecks, which have hindered its widespread application to date. The high costs associated with the pretreatment and saccharification of this complex feedstock limits its economic feasibility for low-value commodity production. In addition, lignocellulosic biomass is comprised of up to 40% pentose sugars, which cannot be naturally metabolized by S. cerevisiae or E. coli and the lignin associated compounds released during lignocellulosic biomass pretreatment inhibit growth of these model hosts. The potential of a number of innovative bioprocessing methods such as CBP and sequential bioreactors to alleviate such bottlenecks were evaluated in this review. Opportunities to capitalize on the robust and efficient lignocellulosic degradation capability of novel cellulolytic bacteria and filamentous fungi were investigated. Non-conventional yeasts such as, R. toruloides, with the ability to metabolize xylose faster than glucose and both tolerate and metabolize lignin associated compounds were considered as alternative hosts for the conversion of lignocellulose to advanced jet fuels. The development of an effective bioprocess for high level advanced biojet fuel production from lignocellulosic feedstocks using traditional model hosts has proven unsuccessful to date. Despite this, through the adoption of novel and synergistic approaches such as those discussed in this review, there is great potential to improve the sustainability of microbial isoprenoid biojet fuel production. Future research should focus on improving the economic viability and productivity of second, third or fourth generation isoprenoid biojet fuels, as such fuels promise substantial progress toward several of the UN’s Sustainable Development Goals and IATA’s commitment to reducing CO2 emissions by 50% by 2050.
Author Contributions
All authors listed have made a substantial, direct and intellectual contribution to the work, and approved it for publication.
Funding
This work was supported by the Engineering and Physical Sciences Research Council [grant number EP/R513209/1] and British Council, Newton Fund Grant Number: P83153.
Conflict of Interest
The authors declare that the research was conducted in the absence of any commercial or financial relationships that could be construed as a potential conflict of interest.
References
Abdel-Hamid, A. M., Solbiati, J. O., and Cann, I. K. O. (2013). Chapter One - Insights into Lignin Degradation and its Potential Industrial Applications. Cambridge, MA: Academic Press, 1–28. doi: 10.1016/B978-0-12-407679-2.00001-6
Abedi, S., Astaraei, F. R., Ghobadian, B., Tavakoli, O., Jalili, H., Greenwell, H. C., et al. (2019). Decoupling a novel Trichormus variabilis-Synechocystis sp. interaction to boost phycoremediation. Sci. Rep. 9:2511. doi: 10.1038/s41598-019-38997-7
Akinosho, H., Yee, K., Close, D., and Ragauskas, A. (2014). The emergence of Clostridium thermocellum as a high utility candidate for consolidated bioprocessing applications. Front. Chem. 2:66. doi: 10.3389/fchem.2014.00066
Ali, S. S., Nugent, B., Mullins, E., and Doohan, F. M. (2016). Fungal-mediated consolidated bioprocessing: the potential of Fusarium oxysporum for the lignocellulosic ethanol industry. AMB Exp. 6:13. doi: 10.1186/s13568-016-0185-0
ASTM International (2020a). ASTM D7566-20: Standard Specification for Aviation Turbine Fuel Containing Synthesized Hydrocarbons. West Conshohocken, PA: ASTM International.
ASTM International (2020b). ASTM D975-20a: Standard Specification for Diesel Fuel. West Conshohocken, PA: ASTM International.
Baral, N. R., Kavvada, O., Mendez-Perez, D., Mukhopadhyay, A., Lee, T. S., Simmons, B. A., et al. (2019). Techno-economic analysis and life-cycle greenhouse gas mitigation cost of five routes to bio-jet fuel blendstocks. Energy Environ. Sci. 12, 807–824. doi: 10.1039/C8EE03266A
Beal, J., Goñi-Moreno, A., Myers, C., Hecht, A., de Vicente, M. D. C., Parco, M., et al. (2020). The long journey towards standards for engineering biosystems. EMBO Rep. 21:e50521. doi: 10.15252/embr.202050521
Benjamin, K. R., Silva, I. R., Cherubim, J. P., McPhee, D., and Paddon, C. J. (2016). Developing commercial production of semi-synthetic artemisinin, and of β-Farnesene, an isoprenoid produced by fermentation of brazilian sugar. J. Braz. Chem. Soc. 27, 1339–1345.
Bokinsky, G., Peralta-Yahya, P. P., George, A., Holmes, B. M., Steen, E. J., Dietrich, J., et al. (2011). Synthesis of three advanced biofuels from ionic liquid-pretreated switchgrass using engineered Escherichia coli. Proc. Natl. Acad. Sci. U.S.A. 108, 19949–19954. doi: 10.1073/pnas.1106958108
Brennan, T. C. R., Williams, T. C., Schulz, B. L., Palfreyman, R. W., Krömer, J. O., and Nielsen, L. K. (2015). Evolutionary engineering improves tolerance for replacement jet fuels in Saccharomyces cerevisiae. Appl. Environ. Microbiol. 81, 3316–3325. doi: 10.1128/AEM.04144-14
Cao, X., Lv, Y.-B., Chen, J., Imanaka, T., Wei, L.-J., and Hua, Q. (2016). Metabolic engineering of oleaginous yeast Yarrowia lipolytica for limonene overproduction. Biotechnol. Biofuels 9:214. doi: 10.1186/s13068-016-0626-7
Chang, M. C. Y., and Keasling, J. D. (2006). Production of isoprenoid pharmaceuticals by engineered microbes. Nat. Chem. Biol. 2, 674–681. doi: 10.1038/nchembio836
Chen, H., Liu, J., Chang, X., Chen, D., Xue, Y., Liu, P., et al. (2017). A review on the pretreatment of lignocellulose for high-value chemicals. Fuel Process. Technol. 160, 196–206. doi: 10.1016/j.fuproc.2016.12.007
Chen, Y., Huang, W., Fu, G., Guo, J., Liu, M., Guo, X., et al. (2015). A genetic transformation protocol for the xylose-fermenting yeast Spathaspora passalidarum. Eng. Life Sci. 15, 550–555. doi: 10.1002/elsc.201400232
Cheng, B.-Q., Wei, L.-J., Lv, Y.-B., Chen, J., and Hua, Q. (2019). Elevating limonene production in oleaginous yeast Yarrowia lipolytica via genetic engineering of limonene biosynthesis pathway and optimization of medium composition. Biotechnol. Bioprocess Eng. 24, 500–506. doi: 10.1007/s12257-018-0497-9
Chuck, C. J., and Donnelly, J. (2014). The compatibility of potential bioderived fuels with Jet A-1 aviation kerosene. Appl. Energy 118, 83–91. doi: 10.1016/j.apenergy.2013.12.019
Contreras-Cornejo, H. A., Macías-Rodríguez, L., and López-Bucio, J. S. (2014). Chapter 36 - Enhanced Plant Immunity Using Trichoderma. Amsterdam: Elsevier, 495–504. doi: 10.1016/B978-0-444-59576-8.00036-9
Coordinating Research Council Inc (1983). Handbook of Aviation Fuel Properties. Alpharetta, GA: Coordinating Research Council Inc.
Cripwell, R. A., Rose, S. H., Favaro, L., and van Zyl, W. H. (2019). Construction of industrial Saccharomyces cerevisiae strains for the efficient consolidated bioprocessing of raw starch. Biotechnol. Biofuels 12:201. doi: 10.1186/s13068-019-1541-5
Dai, X., Shen, H., Li, Q., Rasool, K., Wang, Q., Yu, X., et al. (2019). Microbial lipid production from corn stover by the oleaginous yeast Rhodosporidium toruloides using the PreSSLP process. Energies 12:1053. doi: 10.3390/en12061053
de Farias Silva, C. E., and Bertucco, A. (2016). Bioethanol from microalgae and cyanobacteria: a review and technological outlook. Process Biochem. 51, 1833–1842. doi: 10.1016/j.procbio.2016.02.016
del Río, P. G., Domínguez, E., Domínguez, V. D., Romaní, A., Domingues, L., and Garrote, G. (2019). Third generation bioethanol from invasive macroalgae Sargassum muticum using autohydrolysis pretreatment as first step of a biorefinery. Renew. Energy 141, 728–735. doi: 10.1016/j.renene.2019.03.083
Dutta, K., Daverey, A., and Lin, J.-G. (2014). Evolution retrospective for alternative fuels: first to fourth generation. Renew. Energy 69, 114–122. doi: 10.1016/j.renene.2014.02.044
Eviation (2019). Alice. Available online at: https://www.eviation.co/alice/ (accessed September 6, 2019).
ExxonMobil (2018). Advanced Biofuels And Algae Research: Targeting The Technical Capability To Produce 10,000 barrels per day by 2025. Available online at: https://corporate.exxonmobil.com/research-and-innovation/advanced-biofuels/advanced-biofuels-and-algae-research (accessed October 21, 2020).
Forano, E., Delort, A.-M., and Matulova, M. (2008). Carbohydrate metabolism in Fibrobacter succinogenes: what NMR tells us. Microb. Ecol. Health Dis. 20, 94–102. doi: 10.1080/08910600802106517
George, K. W., Alonso-Gutierrez, J., Keasling, J. D., and Lee, T. S. (2015). “Isoprenoid Drugs, Biofuels, and Chemicals—Artemisinin, Farnesene, and Beyond BT - Biotechnology of Isoprenoids,” in Biotechnology of Isoprenoids, eds J. Schrader and J. Bohlmann (Cham: Springer International Publishing), 355–389. doi: 10.1007/10_2014_288
Governement of India (Ministry of New and Renewable Energy) (2019). Press Information Bureau. Available online at: http://pib.nic.in/newsite/pmreleases.aspx?mincode = 28 (accessed July 18, 2019).
Hackenschmidt, S., Bracharz, F., Daniel, R., Thürmer, A., Bruder, S., and Kabisch, J. (2019). Effects of a high-cultivation temperature on the physiology of three different Yarrowia lipolytica strains. FEMS Yeast Res. 19:foz068. doi: 10.1093/femsyr/foz068
Harrison, K. W., and Harvey, B. G. (2017). Renewable high density fuels containing tricyclic sesquiterpanes and alkyl diamondoids. Sustain. Ener. Fuels 1, 467–473. doi: 10.1039/C6SE00108D
Harvey, B. G. (2018). Fuels and Lubricants from Bisaboline. U.S. Patent No US10053643B1. Washington, DC: U.S. Patent and Trademark Office. doi: 10.1039/c6se00108d
Harvey, B. G., Wright, M. E., and Quintana, R. L. (2010). High-density renewable fuels based on the selective dimerization of pinenes. Energy Fuels 24, 267–273. doi: 10.1021/ef900799c
Heffernan, J. K., Valgepea, K., de Souza Pinto, Lemgruber, R., Casini, I., Plan, M., et al. (2020). Enhancing CO2-valorization using Clostridium autoethanogenum for sustainable fuel and chemicals production. bioRxiv[Preprint]. doi: 10.1101/2020.01.23.917666
Hernández-García, A., Velásquez-Orta, S. B., Novelo, E., Yáñez-Noguez, I., Monje-Ramírez, I., and Orta Ledesma, M. T. (2019). Wastewater-leachate treatment by microalgae: biomass, carbohydrate and lipid production. Ecotoxicol. Environ. Saf. 174, 435–444. doi: 10.1016/j.ecoenv.2019.02.052
Huang, X.-F., Liu, J.-N., Lu, L.-J., Peng, K.-M., Yang, G.-X., and Liu, J. (2016). Culture strategies for lipid production using acetic acid as sole carbon source by Rhodosporidium toruloides. Bioresour. Technol. 206, 141–149. doi: 10.1016/j.biortech.2016.01.073
Immethun, C., Hoynes-O’Connor, A., Balassy, A., and Moon, T. A. E. S. (2013). Microbial production of isoprenoids enabled by synthetic biology. Front. Microbiol 4:75. doi: 10.3389/fmicb.2013.00075
Jiao, X., Zhang, Y., Liu, X., Zhang, Q., Zhang, S., and Zhao, Z. K. (2019). Developing a CRISPR/Cas9 system for genome editing in the basidiomycetous yeast Rhodosporidium toruloides. Biotechnol. J. 14:1900036. doi: 10.1002/biot.201900036
Jiménez-Díaz, L., Caballero, A., Pérez-Hernández, N., and Segura, A. (2017). Microbial alkane production for jet fuel industry: motivation, state of the art and perspectives. Microb. Biotechnol. 10, 103–124. doi: 10.1111/1751-7915.12423
Jongedijk, E., Cankar, K., Buchhaupt, M., Schrader, J., Bouwmeester, H., and Beekwilder, J. (2016). Biotechnological production of limonene in microorganisms. Appl. Microbiol. Biotechnol. 100, 2927–2938. doi: 10.1007/s00253-016-7337-7
Jung, J. K., Lee, Y., Choi, J.-W., Jae, J., Ha, J.-M., Suh, D. J., et al. (2016). Production of high-energy-density fuels by catalytic β-pinene dimerization: effects of the catalyst surface acidity and pore width on selective dimer production. Energy Convers. Manag. 116, 72–79. doi: 10.1016/j.enconman.2016.02.053
Kang, A., and Lee, T. S. (2016). Chapter 2 - Secondary Metabolism for Isoprenoid-based Biofuels. Amsterdam: Elsevier, 35–71. doi: 10.1016/B978-0-444-63475-7.00002-9
Katre, G., Ajmera, N., Zinjarde, S., and RaviKumar, A. (2017). Mutants of Yarrowia lipolytica NCIM 3589 grown on waste cooking oil as a biofactory for biodiesel production. Microb. Cell Fact. 16, 176. doi: 10.1186/s12934-017-0790-x
Kim, E.-M., Eom, J.-H., Um, Y., Kim, Y., and Woo, H. M. (2015). Microbial synthesis of myrcene by metabolically engineered Escherichia coli. J. Agric. Food Chem. 63, 4606–4612. doi: 10.1021/acs.jafc.5b01334
KOKAM (2019). KOKAM Li-ion/Polymer Cell. Available online at: http://kokam.com/data/2019_Kokam_Cell_ver_4.2.pdf (Accessed September 6, 2019).
Kulkarni, M. G., and Dalai, A. K. (2006). Waste cooking Oil An economical source for biodiesel:? a review. Ind. Eng. Chem. Res. 45, 2901–2913. doi: 10.1021/ie0510526
LanzaTech (2017). LanzaTech Presentation. Available online at: https://www.energy.gov/sites/prod/files/2017/07/f35/BETO_2017WTE-Workshop_SeanSimpson-LanzaTech.pdf
Larsson, S., Quintana-Sáinz, A., Reimann, A., Nilvebrant, N.-O., and Jönsson, L. J. (2000). Influence of lignocellulose-derived aromatic compounds on oxygen-limited growth and ethanolic fermentation by Saccharomyces cerevisiae. Appl. Biochem. Biotechnol. 84, 617–632. doi: 10.1385/abab:84-86:1-9:617
Lee, S. K., Chou, H., Ham, T. S., Lee, T. S., and Keasling, J. D. (2008). Metabolic engineering of microorganisms for biofuels production: from bugs to synthetic biology to fuels. Curr. Opin. Biotechnol. 19, 556–563. doi: 10.1016/j.copbio.2008.10.014
Levin, D. B., Verbeke, T. J., Munir, R., Islam, R., Ramachandran, U., Lal, S., et al. (2015). “Chapter 17 - Omics Approaches for Designing Biofuel Producing Cocultures for Enhanced Microbial Conversion of Lignocellulosic Substrates,” in Direct Microbial Conversion of Biomass to Advanced Biofuels, ed. A. B. Himmel (Amsterdam: Elsevier), 335–363. doi: 10.1016/B978-0-444-59592-8.00017-8
Lin, P.-C., Saha, R., Zhang, F., and Pakrasi, H. B. (2017). Metabolic engineering of the pentose phosphate pathway for enhanced limonene production in the cyanobacterium Synechocystis sp. PCC 6803. Sci. Rep. 7:17503. doi: 10.1038/s41598-017-17831-y
Liu, C.-L., Bi, H.-R., Bai, Z., Fan, L.-H., and Tan, T.-W. (2019). Engineering and manipulation of a mevalonate pathway in Escherichia coli for isoprene production. Appl. Microbiol. Biotechnol. 103, 239–250. doi: 10.1007/s00253-018-9472-9
Liu, C.-L., Tian, T., Alonso-Gutierrez, J., Garabedian, B., Wang, S., Baidoo, E. E. K., et al. (2018). Renewable production of high density jet fuel precursor sesquiterpenes from Escherichia coli. Biotechnol. Biofuels 11:285. doi: 10.1186/s13068-018-1272-z
Liu, G., and Qu, Y. (2019). Engineering of filamentous fungi for efficient conversion of lignocellulose: tools, recent advances and prospects. Biotechnol. Adv. 37, 519–529. doi: 10.1016/j.biotechadv.2018.12.004
Liu, J., Yuan, M., Liu, J.-N., and Huang, X.-F. (2017). Bioconversion of mixed volatile fatty acids into microbial lipids by Cryptococcus curvatus ATCC 20509. Bioresour. Technol. 241, 645–651. doi: 10.1016/j.biortech.2017.05.085
Liu, Y., Jiang, X., Cui, Z., Wang, Z., Qi, Q., and Hou, J. (2019). Engineering the oleaginous yeast Yarrowia lipolytica for production of α-farnesene. Biotechnol. Biofuels 12:296. doi: 10.1186/s13068-019-1636-z
Llamas, M., Tomás-Pejó, E., and González-Fernández, C. (2020). Volatile fatty acids from organic wastes as novel low-cost carbon source for Yarrowia lipolytica. New Biotechnol. 56, 123–129. doi: 10.1016/j.nbt.2020.01.002
Martel, C. R. (1987). Military Jet Fuels, 1944-1987, Report Number: AFWAL-TR-87-2062, Wright-Patterson Air Force Base, OH
McCarty, N. S., and Ledesma-Amaro, R. (2019). Synthetic biology tools to engineer microbial communities for biotechnology. Trends Biotechnol. 37, 181–197. doi: 10.1016/j.tibtech.2018.11.002
Meadows, A. L., Hawkins, K. M., Tsegaye, Y., Antipov, E., Kim, Y., Raetz, L., et al. (2016). Rewriting yeast central carbon metabolism for industrial isoprenoid production. Nature 537, 694–697. doi: 10.1038/nature19769
Mendez-Perez, D., Alonso-Gutierrez, J., Hu, Q., Molinas, M., Baidoo, E. E. K., Wang, G., et al. (2017). Production of jet fuel precursor monoterpenoids from engineered Escherichia coli. Biotechnol. Bioeng. 114, 1703–1712. doi: 10.1002/bit.26296
Meylemans, H. A., Quintana, R. L., and Harvey, B. G. (2012). Efficient conversion of pure and mixed terpene feedstocks to high density fuels. Fuel 97, 560–568. doi: 10.1016/j.fuel.2012.01.062
Millo, F., Bensaid, S., Fino, D., Castillo Marcano, S. J., Vlachos, T., and Debnath, B. K. (2014). Influence on the performance and emissions of an automotive Euro 5 diesel engine fueled with F30 from Farnesane. Fuel 138, 134–142. doi: 10.1016/j.fuel.2014.07.060
Minty, J. J., and Lin, X. N. (2015). “Chapter 18 - Engineering Synthetic Microbial Consortia for Consolidated Bioprocessing of Ligonocellulosic Biomass into Valuable Fuels and Chemicals,” in, ed. M. E. B. T.-D. M. C. of B. to A. B. Himmel (Amsterdam. Elsevier, 365–381. doi: 10.1016/B978-0-444-59592-8.00018-X
Mitrovich, Q., and Amyris. (2019). MegaBio: An Integrated Process For Production Of Farnesene, A Versatile Platform Chemical, From Domestic Lignocellulosic Feedstock. Available online at: https://www.energy.gov/sites/prod/files/2019/03/f61/Integrated process for commercial production of farnesene a versatile platform chemical from domestic_EE0007729_v2.pdf (accessed October 21, 2020).
Mohd Azhar, S. H., Abdulla, R., Jambo, S. A., Marbawi, H., Gansau, J. A., Mohd Faik, A. A., et al. (2017). Yeasts in sustainable bioethanol production: a review. Biochem. Biophys. Rep. 10, 52–61. doi: 10.1016/j.bbrep.2017.03.003
Mohr, A., and Raman, S. (2013). Lessons from first generation biofuels and implications for the sustainability appraisal of second generation biofuels. Energy Pol. 63, 114–122. doi: 10.1016/j.enpol.2013.08.033
Morales, P., Gentina, J. C., Aroca, G., and Mussatto, S. I. (2017). Development of an acetic acid tolerant Spathaspora passalidarum strain through evolutionary engineering with resistance to inhibitors compounds of autohydrolysate of Eucalyptus globulus. Ind. Crops Prod. 106, 5–11. doi: 10.1016/j.indcrop.2016.12.023
Mutalik, S., Kumar, C. S. V., Swamy, S., and Manjappa, S. (2012). Hydrolysis of lignocellulosic feed stock by Ruminococcus albus in production of biofuel ethanol. Ind. J. Biotechnol. 11, 453–457.
Nanou, K., Roukas, T., Papadakis, E., and Kotzekidou, P. (2017). Carotene production from waste cooking oil by Blakeslea trispora in a bubble column reactor: the role of oxidative stress. Eng. Life Sci. 17, 775–780. doi: 10.1002/elsc.201600228
Navarrete, C., Jacobsen, I. H., Martínez, J. L., and Procentese, A. (2020). Cell factories for industrial production processes: current issues and emerging solutions. Processes 8:768. doi: 10.3390/pr8070768
Nora, L. C., Wehrs, M., Kim, J., Cheng, J.-F., Tarver, A., Simmons, B. A., et al. (2019). A toolset of constitutive promoters for metabolic engineering of Rhodosporidium toruloides. Microb. Cell Fact. 18:117. doi: 10.1186/s12934-019-1167-0
Norjannah, B., Ong, H. C., Masjuki, H. H., Juan, J. C., and Chong, W. T. (2016). Enzymatic transesterification for biodiesel production: a comprehensive review. RSC Adv. 6, 60034–60055. doi: 10.1039/C6RA08062F
Nowrouzi, B., Li, R. A., Walls, L. E., d’Espaux, L., Malcı, K., Liang, L., et al. (2020). Enhanced production of taxadiene in Saccharomyces cerevisiae. Microb. Cell Fact. doi: 10.1186/s12934-020-01458-2
Osmont, A., Gökalp, I., and Catoire, L. (2006). Evaluating Missile Fuels. Propellants, Explos. Pyrotech. 31, 343–354. doi: 10.1002/prep.200600043
Otoupal, P. B., Ito, M., Arkin, A. P., Magnuson, J. K., Gladden, J. M., and Skerker, J. M. (2019). Multiplexed CRISPR-Cas9-based genome editing of Rhodosporidium toruloides. mSphere 4, e0099-19. doi: 10.1128/mSphere.00099-19
Oßwald, P., Whitside, R., Schäffer, J., and Köhler, M. (2017). An experimental flow reactor study of the combustion kinetics of terpenoid jet fuel compounds: farnesane, p-menthane and p-cymene. Fuel 187, 43–50. doi: 10.1016/j.fuel.2016.09.035
Paddon, C. J., and Keasling, J. D. (2014). Semi-synthetic artemisinin: a model for the use of synthetic biology in pharmaceutical development. Nat. Rev. Microbiol. 12:355. doi: 10.1038/nrmicro3240
Panadare, D. C., and Rathod, V. K. (2015). Applications of waste cooking oil other than biodiesel: a review. Iran. J. Chem. Eng. 12, 55–76.
Pang, J., Liu, Z.-Y., Hao, M., Zhang, Y.-F., and Qi, Q.-S. (2017). An isolated cellulolytic Escherichia coli from bovine rumen produces ethanol and hydrogen from corn straw. Biotechnol. Biofuels 10:165. doi: 10.1186/s13068-017-0852-7
Pang, Y., Zhao, Y., Li, S., Zhao, Y., Li, J., Hu, Z., et al. (2019). Engineering the oleaginous yeast Yarrowia lipolytica to produce limonene from waste cooking oil. Biotechnol. Biofuels 12:241. doi: 10.1186/s13068-019-1580-y
Park, Y.-K., Nicaud, J.-M., and Ledesma-Amaro, R. (2018). The engineering potential of Rhodosporidium toruloides as a workhorse for biotechnological applications. Trends Biotechnol. 36, 304–317. doi: 10.1016/j.tibtech.2017.10.013
Peng, X. N., Gilmore, S. P., and O’Malley, M. A. (2016). Microbial communities for bioprocessing: lessons learned from nature. Curr. Opin. Chem. Eng. 14, 103–109. doi: 10.1016/j.coche.2016.09.003
Peralta-Yahya, P. P., Ouellet, M., Chan, R., Mukhopadhyay, A., Keasling, J. D., and Lee, T. S. (2011). Identification and microbial production of a terpene-based advanced biofuel. Nat. Commun. 2:483.
Peralta-Yahya, P. P., Zhang, F., del Cardayre, S. B., and Keasling, J. D. (2012). Microbial engineering for the production of advanced biofuels. Nature 488, 320–328. doi: 10.1038/nature11478
Pérez-Armendáriz, B., Cal-y-Mayor-Luna, C., El-Kassis, E. G., and Ortega-Martínez, L. D. (2019). Use of waste canola oil as a low-cost substrate for rhamnolipid production using Pseudomonas aeruginosa. AMB Express 9, 61. doi: 10.1186/s13568-019-0784-7
Pires, A. P. P., Han, Y., Kramlich, J., and Garcia-Perez, M. (2018). Chemical composition and fuel properties of alternative jet fuels. Bioresource 13, 2632–2657.
Raut, M. P., Couto, N., Karunakaran, E., Biggs, C. A., and Wright, P. C. (2019). Deciphering the unique cellulose degradation mechanism of the ruminal bacterium Fibrobacter succinogenes S85. Sci. Rep. 9:16542. doi: 10.1038/s41598-019-52675-8
Ribeiro, G. O., Gruninger, R. J., Badhan, A., and McAllister, T. A. (2016). Mining the rumen for fibrolytic feed enzymes. Anim. Front. 6, 20–26. doi: 10.2527/af.2016-0019
Rolf, J., Julsing, M. K., Rosenthal, K., and Lütz, S. (2020). A gram-scale limonene production process with engineered Escherichia coli. Molecules 25:1881. doi: 10.3390/molecules25081881
Royal Dutch, and Shell. (2019). Biofuels. Available online at: https://www.shell.com/energy-and-innovation/new-energies/biofuels.html#iframe = L3dlYmFwcHMvMjAxOV9CaW9mdWVsc19pbnRlcmFjdGl2ZV9tYXAv (accessed September 6, 2019).
Ryder, J. A. (2009). Jet fuel compositions. U.S. Patent No US7935156B2. Washington, DC: U.S. Patent and Trademark Office.
Sari, W. N., Safika, Darmawi, and Fahrimal, Y. (2017). Isolation and identification of a cellulolytic Enterobacter from rumen of Aceh cattle. Vet. world 10, 1515–1520. doi: 10.14202/vetworld.2017.1515-0
Sarria, S., Wong, B., Martín, H. G., Keasling, J. D., and Peralta-Yahya, P. (2014). Microbial Synthesis of Pinene. ACS Synth. Biol. 3, 466–475. doi: 10.1021/sb4001382
Schultz, J. C., Cao, M., and Zhao, H. (2019). Development of a CRISPR/Cas9 system for high efficiency multiplexed gene deletion in Rhodosporidium toruloides. Biotechnol. Bioeng. 116, 2103–2109. doi: 10.1002/bit.27001
Sebesta, J., and Peebles, C. A. M. (2020). Improving heterologous protein expression in Synechocystis sp. PCC 6803 for alpha-bisabolene production. Metab. Eng. Commun. 10, e00117. doi: 10.1016/j.mec.2019.e00117
Sharma, H. K., Xu, C., and Qin, W. (2019). Biological pretreatment of lignocellulosic biomass for biofuels and bioproducts: an overview. Waste Biomass Valor. 10, 235–251. doi: 10.1007/s12649-017-0059-y
Sherlock, M. F. (2019). The Plug-In Electric Vehicle Tax Credit. Available online at: https://fas.org/sgp/crs/misc/IF11017.pdf (accessed October 21, 2020).
Soriano, J. A., Agudelo, J. R., López, A. F., and Armas, O. (2017). Oxidation reactivity and nanostructural characterization of the soot coming from farnesane - A novel diesel fuel derived from sugar cane. Carbon N. Y. 125, 516–529. doi: 10.1016/j.carbon.2017.09.090
Speight, J. G. (2011). “Chapter 3 - Fuels for Fuel Cells,” in Fuel Cells: Technologies for Fuel Processing, eds D. Shekhawat, J. J. Spivey, and F. P. Berry (Amsterdam: Elsevier), 29–48. doi: 10.1016/B978-0-444-53563-4.10003-3
Srivastava, L. M. (2002). “Gibberellins,” in Plant Growth and Development, ed. D. Srivastava (San Diego: Academic Press), 171–190. doi: 10.1016/B978-012660570-9/50148-9
Stoppacher, N., Kluger, B., Zeilinger, S., Krska, R., and Schuhmacher, R. (2010). Identification and profiling of volatile metabolites of the biocontrol fungus Trichoderma atroviride by HS-SPME-GC-MS. J. Microbiol. Methods 81, 187–193. doi: 10.1016/j.mimet.2010.03.011
Su, B., Song, D., and Zhu, H. (2020). Metabolic engineering of Saccharomyces cerevisiae for enhanced carotenoid production from Xylose-Glucose mixtures. Front. Bioeng. Biotechnol 8:435. doi: 10.3389/fbioe.2020.00435
Suen, G., Weimer, P. J., Stevenson, D. M., Aylward, F. O., Boyum, J., Deneke, J., et al. (2011). The complete genome sequence of Fibrobacter succinogenes S85 reveals a cellulolytic and metabolic specialist. PLoS One 6:e0018814. doi: 10.1371/journal.pone.0018814
The European Parliament and of the Council of the European Union (2018). Directive (EU) 2018/2001 of the European Parliament and of the Council of 11 December 2018 on the promotion of the use of energy from renewable sources (recast). Off. J. Eur. Union 328, 82–209. Available online at: https://eur-lex.europa.eu/legal-content/EN/TXT/PDF/?uri=CELEX:32018L2001&from=EN
The Royal Academy of Engineering (2017). Sustainability of Liquid Biofuels. London: The Royal Academy of Engineering.
Tippmann, S., Chen, Y., Siewers, V., and Nielsen, J. (2013). From flavors and pharmaceuticals to advanced biofuels: production of isoprenoids in Saccharomyces cerevisiae. Biotechnol. J. 8, 1435–1444. doi: 10.1002/biot.201300028
U.S. Energy Information Administration (2019). Monthly Energy Review. Washington, DC: U.S. Energy Information Administration
UK Government (Department for Transport) (2020). Low-Emission Vehicles Eligible For A Plug-In Grant. Available online at: https://www.gov.uk/plug-in-car-van-grants (accessed June 30, 2020).
UK Government (Department for Transport) (2018). Renewable Transport Fuel Obligation Guidance Part One. (Process)Guidance. Available online at: https://assets.publishing.service.gov.uk/government/uploads/system/uploads/attachment_data/file/694277/rtfo-guidance-part-1-process-guidance-year-11.pdf (accessed July 18, 2019).
United Nations (2015). Transforming our World: the 2030 Agenda for Sustainable Development. New York, NY: United Nations.
Walls, L. E., Malci, K., Nowrouzi, B., Li, R., D’Espaux, L., Wong, J., et al. (2020). Optimizing the biosynthesis of oxygenated and acetylated Taxol precursors in Saccharomyces cerevisiae using advanced bioprocessing strategies. Biotechnol. Bioeng. 117, 1–15. doi: 10.1002/bit.27569
Wang, K. Y., Strobel, G. A., and Yan, D. H. (2017). The production of 1,8-Cineole, a Potential Biofuel, from an Endophytic Strain of Annulohypoxylon sp. FPYF3050 When Grown on Agricultural Residues. J. Sustain. Bioener. Syst. 7, 65–84. doi: 10.4236/jsbs.2017.72006
Wang, S., Wang, Z., Wang, Y., Nie, Q., Yi, X., Ge, W., et al. (2017). Production of isoprene, one of the high-density fuel precursors, from peanut hull using the high-efficient lignin-removal pretreatment method. Biotechnol. Biofuels 10:297. doi: 10.1186/s13068-017-0988-5
Ward, V. C. A., Chatzivasileiou, A. O., and Stephanopoulos, G. (2018). Metabolic engineering of Escherichia coli for the production of isoprenoids. FEMS Microbiol. Lett. 365, doi: 10.1093/femsle/fny079
Weimer, P. J., Russell, J. B., and Muck, R. E. (2009). Lessons from the cow: what the ruminant animal can teach us about consolidated bioprocessing of cellulosic biomass. Bioresour. Technol. 100, 5323–5331. doi: 10.1016/j.biortech.2009.04.075
Westfall, P. J., Pitera, D. J., Lenihan, J. R., Eng, D., Woolard, F. X., Regentin, R., et al. (2012). Production of amorphadiene in yeast, and its conversion to dihydroartemisinic acid, precursor to the antimalarial agent artemisinin. Proc. Natl. Acad. Sci. U.S.A. 109, E111–E118. doi: 10.1073/pnas.1110740109
Wong, J., de Rond, T., d’Espaux, L., van der Horst, C., Dev, I., Rios-Solis, L., et al. (2018). High-titer production of lathyrane diterpenoids from sugar by engineered Saccharomyces cerevisiae. Metab. Eng. 45, 142–148. doi: 10.1016/j.ymben.2017.12.007
Wong, J., Rios-Solis, L., and Keasling, J. D. (2017). “Microbial Production of Isoprenoids BT - Consequences of Microbial Interactions with Hydrocarbons, Oils, and Lipids: Production of Fuels and Chemicals,” in Handbook of Hydrocarbon and Lipid Microbiology, ed. S. Y. Lee (Cham: Springer International Publishing), 359–382. doi: 10.1007/978-3-319-50436-0_219.
Woodroffe, J.-D., and Harvey, B. G. (2020). High-performance, biobased, jet fuel blends containing hydrogenated monoterpenes and synthetic paraffinic kerosenes. Energy Fuels 34, 5929–5937. doi: 10.1021/acs.energyfuels.0c00274
Wu, C., Spike, T., Klingeman, D. M., Rodriguez, M., Bremer, V. R., and Brown, S. D. (2017). Generation and characterization of acid tolerant Fibrobacter succinogenes S85. Sci. Rep. 7:2277. doi: 10.1038/s41598-017-02628-w
Wu, J., Cheng, S., Cao, J., Qiao, J., and Zhao, G.-R. (2019). Systematic optimization of limonene production in engineered Escherichia coli. J. Agric. Food Chem. 67, 7087–7097. doi: 10.1021/acs.jafc.9b01427
Wu, T., Liu, J., Li, M., Zhang, G., Liu, L., Li, X., et al. (2020). Improvement of sabinene tolerance of Escherichia coli using adaptive laboratory evolution and omics technologies. Biotechnol. Biofuels 13:79. doi: 10.1186/s13068-020-01715-x
Wu, W., Davis, R. W., Tran-Gyamfi, M. B., Kuo, A., LaButti, K., Mihaltcheva, S., et al. (2017). Characterization of four endophytic fungi as potential consolidated bioprocessing hosts for conversion of lignocellulose into advanced biofuels. Appl. Microbiol. Biotechnol. 101, 2603–2618. doi: 10.1007/s00253-017-8091-1
Xu, L., and Tschirner, U. (2011). Improved ethanol production from various carbohydrates through anaerobic thermophilic co-culture. Bioresour. Technol. 102, 10065–10071. doi: 10.1016/j.biortech.2011.08.067
Xu, X., Kim, J. Y., Cho, H. U., Park, H. R., and Park, J. M. (2015). Bioconversion of volatile fatty acids from macroalgae fermentation into microbial lipids by oleaginous yeast. Chem. Eng. J. 264, 735–743. doi: 10.1016/j.cej.2014.12.011
Yaegashi, J., Kirby, J., Ito, M., Sun, J., Dutta, T., Mirsiaghi, M., et al. (2017). Rhodosporidium toruloides: a new platform organism for conversion of lignocellulose into terpene biofuels and bioproducts. Biotechnol. Biofuels 10:241. doi: 10.1186/s13068-017-0927-5
Yang, X., Li, T., Tang, K., Zhou, X., Lu, M., Ounkham, W. L., et al. (2017). Highly efficient conversion of terpenoid biomass to jet-fuel range cycloalkanes in a biphasic tandem catalytic process. Green Chem. 19, 3566–3573. doi: 10.1039/C7GC00710H
Yiting Chen, W., Liew, F., and Koepke, M. (2015). Microbial fermentation for the production of terpenes. U.S. Patent No. US20180142265. Washington, DC: U.S. Patent and Trademark Office doi: 10.1039/c7gc00710h
You, S., Yin, Q., Zhang, J., Zhang, C., Qi, W., Gao, L., et al. (2017). Utilization of biodiesel by-product as substrate for high-production of β-farnesene via relatively balanced mevalonate pathway in Escherichia coli. Bioresour. Technol. 243, 228–236. doi: 10.1016/j.biortech.2017.06.058
Zargar, A., Bailey, C. B., Haushalter, R. W., Eiben, C. B., Katz, L., and Keasling, J. D. (2017). Leveraging microbial biosynthetic pathways for the generation of ‘drop-in’ biofuels. Curr. Opin. Biotechnol. 45, 156–163. doi: 10.1016/j.copbio.2017.03.004
Zhang, H., Liu, Q., Cao, Y., Feng, X., Zheng, Y., Zou, H., et al. (2014). Microbial production of sabinene–a new terpene-based precursor of advanced biofuel. Microb. Cell Fact 13:20. doi: 10.1186/1475-2859-13-0
Zhao, X., Peng, F., Du, W., Liu, C., and Liu, D. (2012). Effects of some inhibitors on the growth and lipid accumulation of oleaginous yeast Rhodosporidium toruloides and preparation of biodiesel by enzymatic transesterification of the lipid. Bioprocess Biosyst. Eng. 35, 993–1004. doi: 10.1007/s00449-012-0684-6
Zhao, Y., Zhu, K., Li, J., Zhao, Y., Li, S., Zhang, C., et al. (2020). High-Efficiency Production of the Bisabolene from Waste Cooking Oil By Metabolically Engineered Yarrowia Lipolytica (Preprint). Res. Sq. Preprint doi: 10.21203/rs.3.rs-71409/v1
Zhuang, X., Kilian, O., Monroe, E., Ito, M., Tran-Gymfi, M. B., Liu, F., et al. (2019). Monoterpene production by the carotenogenic yeast Rhodosporidium toruloides. Microb. Cell Fact. 18:54. doi: 10.1186/s12934-019-1099-8
Keywords: advanced biojet fuel, isoprenoid, monoterpene, sesquiterpene, lignocellulosic biomass, consolidated bioprocessing
Citation: Walls LE and Rios-Solis L (2020) Sustainable Production of Microbial Isoprenoid Derived Advanced Biojet Fuels Using Different Generation Feedstocks: A Review. Front. Bioeng. Biotechnol. 8:599560. doi: 10.3389/fbioe.2020.599560
Received: 27 August 2020; Accepted: 09 October 2020;
Published: 30 October 2020.
Edited by:
Eduardo Jacob-Lopes, Federal University of Santa Maria, BrazilReviewed by:
Lei Zhao, Harbin Institute of Technology, ChinaKeikhosro Karimi, Isfahan University of Technology, Iran
Copyright © 2020 Walls and Rios-Solis. This is an open-access article distributed under the terms of the Creative Commons Attribution License (CC BY). The use, distribution or reproduction in other forums is permitted, provided the original author(s) and the copyright owner(s) are credited and that the original publication in this journal is cited, in accordance with accepted academic practice. No use, distribution or reproduction is permitted which does not comply with these terms.
*Correspondence: Leonardo Rios-Solis, TGVvLlJpb3NAZWQuYWMudWs=