- 1Department of Spine Surgery, First Hospital of Jilin University, Changchun, China
- 2Department of Orthopedics, Affiliated Hospital of Beihua University, Jilin, China
- 3Jilin Institute of Scientific and Technical Information, Changchun, China
- 4Department of Hand Surgery, China-Japan Union Hospital of Jilin University, Changchun, China
As the world population is aging, intervertebral disc degeneration (IDD) is becoming a global health issue of increasing concern. A variety of disc degeneration diseases (DDDs) have been proven to be associated with IDD, and these illnesses have significant adverse effects on both individuals and society. The application of stem cells in regenerative medicine, such as blood and circulation, has been demonstrated by numerous studies. Similarly, stem cells have made exciting progress in the treatment of IDD. However, due to complex anatomical structures and functional requirements, traditional stem cell injection makes it difficult to meet people’s expectations. With the continuous development of tissue engineering and biomaterials, stem cell combined with biomaterials has far more prospects than before. This review aims to objectively and comprehensively summarize the development of stem cells combined with contemporary biomaterials and the difficulties that need to be overcome.
1 Introduction
The intervertebral disc (IVD) is the cartilaginous tissue that lies between the spinal segments and plays a crucial role in maintaining the normal physiological activity of the spine. With the increase in aging populations worldwide, intervertebral disc degeneration (IDD) is becoming a health problem that is difficult to ignore or compromise. IDD could lead to disc degeneration diseases (DDDs), such as compression of blood vessels or nerves, and disc herniation (Kim H. S. et al., 2020). DDDs often cause lower back pain and may be associated with motor dysfunction and sensory abnormalities (Luoma et al., 2000). In the United States alone, back problems account for more than seven million emergency department visits and more than two million hospital admissions per year (Owens et al., 2006). In social and economic terms, the direct cost of health care and the indirect economic losses due to pain is still staggering. In 2016, lower back and neck pain accounted for the highest medical expenditure of around $ 134.5 billion among 154 medical conditions included in the United States (Dieleman et al., 2020).
The specific causes of disc degeneration are not fully understood. The prevailing view is that IDD is a combination of factors, such as age, genetics, and diabetes (Rider et al., 2019; Francisco et al., 2022). Typical pathological features of IDD include decreased water content and height of the disc, disturbed balance between synthesis and decomposition of the extracellular matrix (ECM), continuous loss of cells, and changes in the microenvironment of the IVD tissue (Binch et al., 2021). At present, the clinical treatment of DDD can be roughly categorized as either conservative or surgical. Common conservative treatments include analgesics, appropriate functional exercise, acupuncture, and massage. Conservative management may be effective in patients with mild symptoms or early-stage IDD (Corp et al., 2021). Surgery is still the only option for patients who do not respond to conservative treatment or who might have developed severe complications. The most frequently used procedures include discectomy and fusion. In recent years, the rapid development of endoscopic and postoperative rapid rehabilitation technologies has greatly shortened the length of hospital stays, in addition to reducing the possibility of many postoperative complications. However, the biomechanical changes that are brought by surgery to the overall spine are still irreversible (Ahn, 2019; Gadjradj et al., 2021). The abnormal mechanical structure will lead to further aggravation of the load on the adjacent segments, which further expands the possibility of disc degeneration of the adjacent spinal segments. In addition, the persistent neurological symptoms during long-term follow-up and the possibility of reoperation prove that surgery is not a one-and-done option (Lurie and Tomkins-Lane, 2016; Wu et al., 2021; Katz et al., 2022). In conclusion, all current treatments focus on the remedy and resolution of existing symptoms. Earlier interventions on the IDD pathological process or on the reversal of the degeneration process have received extensive attention and a lot of exploration research has been carried out in recent years.
Many promising therapeutic approaches have been developed for the various mechanisms of the IDD, such as gene therapy, biological agents, and growth factors. Some of them have been in pre-clinical trials while others have been approved for clinical use (Kamali et al., 2021). However, the treatment for single pathogeny is often difficult to meet people’s expectations in the treatment of IDD cases that are caused by multiple pathological processes. Due to the easier availability, stronger differentiation ability and extremely low immunogenicity of stem cells, these cells can target multiple pathological pathways (Zakrzewski et al., 2019; Bhujel et al., 2022). The successful application of stem cells in blood and circulatory related diseases seems to further raise the hopes for the possible application of stem cell therapy for IDD. However, even without external factors such as ethical approval, the complex anatomical structure and functional requirements make stem cells difficult to retain, not to talk about surviving in the proposed location, which undoubtedly limits further exploration of stem cell therapy for IDD. With the continuous development of tissue engineering and biomaterials, stem cells combined with biomaterials have shown promising application prospects. Biomaterials do not only provide a suitable physical and chemical environment for stem cells, but also carry drugs or cytokines, to promote better directional differentiation of the stem cells. The designed biomaterials can also have a good immobilization effect on the stem cells, in addition to preventing the leakage of the cells. This review aims to provide an overview of the current advances in the treatment of IDD with stem cells combined with biomaterials. We will also discuss the selection of stem cells and biomaterials. Finally, we will summarize the current limitations and future challenges that are associated with treatment of IDD.
2 Anatomy of intervertebral disc
IVD is the largest avascular tissue in the human body, which is mainly composed of nucleus pulposus (NP), annulus fibrosus (AF) and cartilage endplate (CEP) (Roberts et al., 2006; Smith et al., 2011). Due to its avascular nature, it has a poor ability to repair itself after injury and degeneration. To better understand the mechanism of stem cell therapy for IDD, it is necessary to understand the main physiological characteristics and functional requirements of each part in IVD (Figure 1).
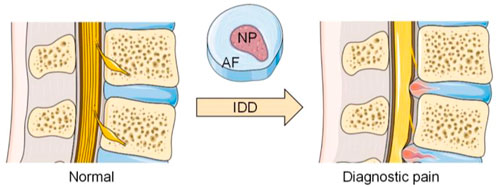
FIGURE 1. Anatomical changes between normal and degenerated discs. (The Figure was partly generated using Servier Medical Art, provided by Servier, licensed under a Creative Commons Attribution 3.0 unported license).
2.1 The nucleus pulposus
The NP is the colloidal part that is located at the center of the IVD. The NP contains more than 70% water, which reduces the effects of external pressure and prevents internal stress concentration (Raj, 2008). The remaining components of the NP are mainly proteoglycan and collagen. Aggrecan is the most abundant type of proteoglycan in NP, though other forms such as biglycan and versican are also present (Melrose et al., 2001). Proteoglycans are essential for maintaining the high-water content of NP. There is type Ⅰ and type II collagen. The NP has a higher content of type II collagen than other IVD tissues (Raj, 2008). The NP cells are present in a colloid-like matrix that is composed of proteoglycan and collagen. In general, the cell density in the NP tissue is relatively low, only 2–5*106 cells/ml, and it further decreases with age (Maroudas et al., 1975; Stairmand et al., 1991). In the early stage of IVD development, the NP has vascular distribution and notochord-derived cells (Roberts et al., 2006; de Bree et al., 2018), both of which are not found in adult NPs (Colombini et al., 2008). The presence of notochord-derived cells has been linked to self-repair abilities, while the lack of vascular distribution means that NP cells are in a relatively anoxic environment, so nutrition or drugs are difficult to transport through the blood (Risbud and Shapiro, 2011; Boubriak et al., 2013). All these suggest that NP has a fragile ability to repair itself.
As mentioned above, proteoglycans and high osmotic pressure are essential for resisting axial compressive forces and spinal pressure during daily motion and activities. The range of mechanical loads required by the NP is enormous. When lying prone, the pressure load on the NP is only 0.1 MPa, but when bending down to lift heavy objects, the pressure load will rise to 2.3 MPa (Schultz et al., 1982; Adams et al., 1996). Abnormal mechanical loads have been proved to be an important factor that contributes to IDD (Vergroesen et al., 2015). The main pathological changes in the NP are proteoglycan loss, type II collagen and osmolality reduction. In spine magnetic resonance imaging (MRI), these changes manifest as decreased disc and appear darkened to black on the T2 signal (Antoniou et al., 1996; Brinjikji et al., 2015).
2.2 The anulus fibrosus
The AF is the fibrocartilage that surrounds the NP, and can be further divided into outer and inner AF (Hsieh and Twomey, 2010). The main function of the AF is to stabilize and encapsulate the NP. In the presence of an intact AF structure, the NP tissue can maintain a high hydrostatic pressure, and the entire disc can resist the high intensity pressure that emanates from daily motion such as flexion-extension and lateral bending. The AF is a lamellar structure that is predominantly composed of type Ⅰ collagen in a highly oriented manner, with about 15–25 layers (Marchand and Ahmed, 1990). As the AF approaches the NP, the proportion of type II collagen and water content gradually increases (Feng et al., 2006). The cell density in the AF is higher than that in the NP, with a range of about 5–10*106 cells/ml (Bowles and Setton, 2017). These cells are arranged in concentric lamellae that interleave each other and are offset by 30–60° from the vertical spinal axis (Walker and Anderson, 2004; Raj, 2008). During the early stages of IVD development, the boundary between the NP and AF is not obvious but as the development approaches maturity, clear morphological boundary begins to appear (Roberts et al., 2006).
When the AF structure is damaged or fractured, the uneven distribution of stress may lead to the herniation or displacement of the IVD tissue, which may further compress the surrounding blood vessels and nerves, thereby causing obvious clinical symptoms. Despite the possibility of adequate decompression by surgical treatment, the AF structure is not repaired, and the abnormal biomechanical structure is not effectively corrected. Although AF and NP are significantly different in composition and structure, they are closely related in maintaining normal physiological functions. Moreover, any tiny damage on any single part will lead to the disorder of the overall function.
2.3 The cartilage endplate
The CEP is composed of the hyaline cartilage and lies between the soft tissue of the IVD and the bony structure of the vertebral body (Luo et al., 2021). The hyaline cartilage is mainly composed of water, proteoglycans, and collagen. The CEPs are critical for maintaining the mechanical integrity, as well as the vascular and avascular separation of the IVDs. Due to the avascular structure of the IVD, the essential substances such as glucose and oxygen are mainly supplied by the capillaries that are around the IVD through CEP, to maintain cell activity (Urban et al., 2004; Malandrino et al., 2014; Bowles and Setton, 2017). The CEP in adults is thinner and less vascularized than the endplates in the early development stage (Harmon et al., 2020). Comparable to osteoarthritis, cartilage damage is also one of the pathological features of IDD (Francisco et al., 2022). Thinning and mineralized CEP not only makes it difficult to maintain spinal biomechanical stability, but also prevent nutrients from entering the NP and AF through the CEP (Huang et al., 2014). All these factors will further aggravate the rate of intervertebral disc degeneration. In addition to these, the increase in nerve fibers in the CEP of the degenerative segment is also thought to be related to the pain that is caused by IDD. In recent years, promoting CEP repair in a bid to enhance the nutrient supply to the IVD has become an alternative way to interfere with the progression of IDD. Having said this, stem cells have also achieved promising application prospects in promoting cartilage regeneration (Yang et al., 2018; Kangari et al., 2020).
3 Applications of stem cells in combination with biomaterials
3.1 Types and selection of stem cells and biomaterials
3.1.1 Selection of stem cells
Overall, stem cells have the advantages of low immunogenicity, strong ability to induce differentiation, and tolerance to hypoxia and low glucose. There are also some differences in the function, survival, and acquisition of different types of stem cells. Choosing the right type of stem cell is undoubtedly an important step in using stem cells to treat IDD (Table.1). At present, stem cells can be divided into mesenchymal stem cells (MSCs), pluripotent stem cells (PSCs), and IVD-derived stem cells (IVDSCs). According to their source, MSCs can be further divided into umbilical cord mesenchymal stem cells (UCMSCs), adipose-derived mesenchymal stem cells (ADMSCs), and bone marrow mesenchymal stem cells (BMSCs) (Zhang et al., 2022). UCMSCs are relatively young stem cells with excellent differentiation potential, and because they are self-provided and the means of acquisition are non-invasive, there is no need to consider ethical barriers (Chen et al., 2013; Garzón et al., 2014). Unfortunately, the retention and acquisition time of UCMSCs is short. Additionally, IDD patients lose the opportunity to obtain autologous UCMSCs when symptoms are obvious. Another thing to note is that the experimental cost of UCMSCs is high yet the treatment outcome is not significantly different from the results of using other stem cells. Considering the wide distribution and easy availability of adipose tissue in the human body, ADMSCs seem to be an ideal choice. A large number of in vivo or in vitro experiments have proved that ADMSCs can intervene or even reverse the pathological process of IDD (Lu et al., 2008; Jin et al., 2013; Clarke et al., 2014). Unfortunately, the endochondral osteogenesis ability of ASMCS is not satisfactory (Diekman et al., 2010). At present, BMSCs have attracted the most attention in stem cell therapy for IDD. BMSCs are non-hematopoietic stem cells that are located in the bone marrow. These stem cells are characterized by an ideal differentiation potential and self-renewal attributes. Although many previous studies that used animal models and some small clinical cohort studies have pointed out that BMSCs have exciting application prospects in the treatment of IDD (Cao et al., 2015; Kumar et al., 2017; Teixeira et al., 2018), the method for obtaining these stem cells is invasive. Also, there is a lack of long-term clinical cohort observation so the application of BMSCs in the treatment of IDD still needs to be carefully evaluated. PSCs include embryonic stem cells (ESCs) and induced pluripotent stem cells (IPSCs). Considering that ESCs are extracted from frozen embryos, their clinical application is subject to strict ethical restrictions. IPSCs can differentiate into NP cells in the ECM after receiving some inducing factors that are secreted by NP cells (Liu et al., 2015b). It is worth noting that the proliferation and differentiation patterns of IPSCs are similar to tumor cells, which makes them potentially carcinogenic. In recent years, the method of avoiding carcinogenicity using IPSC-based exosomes has gradually received attention (Qi et al., 2016). Comparable to MSCs, IVDSCs can be classified according to their origin from NP, AF, and CEP. Although these native tissue-derived stem cells seem to have a broad application prospect, the low separation efficiency and harsh microenvironment in the degenerated IVD hinder the further exploration of IVDSCs.
3.1.2 Selection of biomaterials
Compared with the limited selection of stem cell types, the options for materials have become more diversified due to the continuous development of materials through science and industrial technology. However, multiple options do not always simplify the problem; they sometimes even make it more complex. Considering the load-bearing requirements of a normal disc, the materials need to simulate the mechanical properties of normal tissue as much as possible (Manoukian et al., 2018; Zhu et al., 2021). In addition, targeted selection of biocompatibility such as cell adhesion should be made according to the characteristics of the corresponding tissue. Finally, cytotoxicity, immunogenicity, degradability, and manufacturing cost are inescapable problems for all implants. At present, the choice of materials is often a trade-off between the above-mentioned properties, to a certain extent. When one property is improved, another performance is usually compromised (Table 2). For example, in the selection of scaffold materials, it is often difficult to have both remarkable mechanical properties and porosity.
Hydrogels are widely used to deliver cells due to their excellent water content, which can provide a suitable microenvironment for cell survival. Hydrogels have various components that can be classified into natural and synthetic components. The natural ingredients include collagen, hyaluronic acid, chitosan, and alginate. Generally, natural components have good biocompatibility and low cytotoxicity, but their mechanical properties are often unsatisfactory. Some of the common synthetic components are poly (N-isopropyl acrylamide), poly (ethylene glycol), and poly (lactic-co-glycolic acid) copolymers. These materials can be industrially produced in large quantities and have strong mechanical properties, but their cytotoxicity and immunogenicity should be considered. Composite hydrogels can combine components with different properties to achieve more ideal performance. However, the involvement of different components bring new problems to the overall material design. The in vivo safety of various combinations of components needs to be rigorously evaluated.
Several decellularized scaffolds have been developed. To better mimic the component composition of normal disc tissue. However, the scarcity of healthy donor sources that are suitable for humans limits their further development, and the use of animal-derived substitutes requires strict safety assessment. The microsphere system can control the release of substances in the delivery system in a more precise way, in addition to providing attachment sites for cells. Some of the common microsphere materials include natural materials such as gelatin, collagen and chitosan, as well as polylactic acid-glycolic acid, poly (L-lactic acid) and polycaprolactone. Unfortunately, it is difficult for the microspheres to provide mechanical support so other materials should be introduced, though this makes system design more difficult.
In recent years, some artificial polymer materials significantly improved the performance of biomaterials, with a remarkable balance in mechanical strength and porosity. Increased understanding of the structure of IVD and continuous improvement of the material preparation technology has made it possible to imitate the structure of tissues to provide a more suitable environment for stem cells. Additionally, scaffolds that are loaded with drugs or cytokines are better applicable cell differentiation and expression of corresponding products.
3.2 Replenish cells and improve the microenvironment
Most tissues and cells in the human body are undergoing constant renewal, and sufficient cells plays a crucial role in maintaining normal tissue function and metabolism. This rule also holds true for the IVD tissue. Again, it is worth noting that the low cell density, lack of a powerful source of regenerative cells, and the harsh microenvironment after IDD make the endogenous self-cell recruitment of IVD difficult. In degenerated IVD, cell loss was reflected by higher rates of senescence, apoptosis, and pyroptosis. Direct replacement of the missing cells seems to be the most straightforward and effective method, though there are still many limitations, such as the survival rate of the implanted cells and unnecessary cell dissipation. Stem cells combined with biomaterials offer new hope for stem cell-based endogenous repair.
Many experiments have shown that various stem cells can differentiate into functional cells of IVD if they are exposed to appropriate stimulation. Although conditions such as hypoxia are often considered to be unfavorable for cell survival, appropriate hypoxia is indeed a stimulating condition for further cell differentiation. Han et al. (Han et al., 2015), co-cultured human ligamentum derived stem cells and NP cells in vitro under hypoxia conditions and successfully differentiated ligamentum derived stem cells into NP-like cells. Strassburg et al. (Strassburg et al., 2010), reported varying stimulating effects of different NP cells. BMSCs were co-cultured with NP cells from degenerated or non -degenerated discs for 7 days. Co-culturing with NP cells derived from degenerated discs enhanced the expression of the transforming growth factor (TGF) -β and cartilage-derived morphogenetic protein -1, which can regulate NP cell metabolism and promote the production of ECM (Thompson et al., 1991). In an in vivo study using rabbit model, BMSCs were transplanted into degenerated IVD and resulted in significant improvement in cell numbers, cell survival rate, and disc water content after 24 weeks (Sakai et al., 2006). Similar findings have been reported in other types of stem cells. Zhao et al. (Zhao et al., 2020), isolated NP cells from patients with severe IDD and induced apoptosis by in vitro compression. After co-culturing with UCMSCs, the apoptosis was significantly reversed. Lu et al. (Lu et al., 2008), co-cultured ADMSCs with NP cells in a hydrogel containing collagen Ⅱ. The results showed that the number of cells that were producing type II collagen was significantly increased and the aggrecan-related genes were upregulated. In another in vivo study, when ESCs were induced for differentiation by TGF-β and ascorbic acid, they were implanted into the IDD model by needle puncture (Sheikh et al., 2009). The results revealed the presence of notochord-derived cells and higher proteoglycan content was observed. In recent years, notochord-derived cells have been identified as key points in IVD regeneration (Harfe, 2022). He et al. (He et al., 2018), also reported that co-culturing cartilage endplate stem cells (CESCs) with NP cells promoted the proliferation of NP cells.
Although the growth and differentiation of stem cells are necessary for the stem cell-based therapy, the differentiation of abnormal location and direction caused by stem cell leakage often reduces the therapeutic effect, sometimes leading to serious consequences such as tumorigenesis. Therefore, the introduction of biomaterials cannot only prevent stem cell leakage but also provide suitable spatial structure for stem cells. In addition, the combination of drugs can provide further support for stem cells. Bertolo et al. (Bertolo et al., 2015), developed an injectable microcarrier with a size between 100 and 1,500 µm based on collagen, which achieved significant cell proliferation under 5% hypoxia. Andrea et al. (Friedmann et al., 2021), implanted collagen-based scaffolds loaded with ADMSCs into a sheep IDD model and made observations for up to 1 year. The researchers noted a stabilization of disc degeneration but did not observe recovery of disc height (Figure 2). Similarly, Zhang et al. (Zhang et al., 2014), used chitosan and alginate to fabricate an injectable 3D scaffold and achieved good growth and differentiation of ADMSCs under 2% hypoxia. Daisuke et al. (Ukeba et al., 2020), combined ultra-pure alginate-based gel with BMSCs. Compared with the gel-free control group, stem cells that were cultured in vitro showed higher expression of growth factors and ECM-related genes, and the stem cells combined with the gel group effectively induced IVD regeneration in vivo. Upon further comparison of four kinds of commercial scaffolds approved for medical use, Alessandro et al. (Bertolo et al., 2012), indicated that collagen and gelation-based scaffolds had better cell survival rate and aggrecan expression. Peroglio et al. (Peroglio et al., 2013), developed a hyaluronic acid-based thermoreversible hydrogel, which showed better differentiation induction than the alginate in vitro culture. The hydrogel maintained 90% of MSCs viability in nucleotomized IVD for at least 1 week. To compensate for the lack of cell binding sites in alginate-based materials and the unsatisfactory mechanical properties of collagen-based materials, Guillaume et al. (Guillaume et al., 2015), developed an alginate-collagen composite scaffold with the property of shape memory. In addition, the composite scaffold showed better cell fixation ability than alginate only scaffold and maintained the viability of BMSCs for 5 weeks.
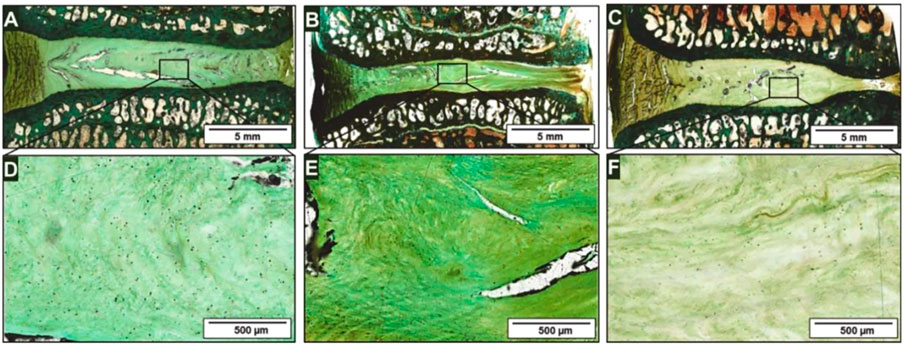
FIGURE 2. After 12 months of experiment, Masson-Goldner histological light microscopy was used to observe the representative IVD segments in a sheep model. The healthy segment view (A) and enlarged view of the NP region (D); The IDD segment (B) and enlarged view of the NP region (E); The IDD segment receiving scaffolds injection (C) and enlarged view of the NP region (F). Reproduced with permission from (Friedmann et al., 2021).
The high pressure in human IVD may cause the displacement of liquid cell carrier, which has higher requirements on the mechanical properties of the material. Zeng et al. (Zeng et al., 2015), loaded the alginate precursor encapsulated with ADMSCs into Poly (ethylene glycol) diacrylate (PEGDA). This thermos-responsive hydrogel improved cell survival rate while preventing cell leakage. Moreover, a better reduction in degeneration was observed than in other treatment groups in an IDD dog model after 6 months. In 2017, Diba et al. (Diba et al., 2017), assembled silica and gelatin nanoparticles to form a colloidal gel with excellent mechanical properties and impressive self-healing ability upon shear failure. In their subsequent study, they used this colloidal gel as the carrier to deliver BMSCs and injected it into rabbit IDD models. The gel showed excellent biocompatibility and degradability in vivo. Besides, the colloidal gel effectively prevented cell leakage, in addition to providing a favorable environment for the growth and differentiation of the BMSCs. Apart from the characteristics of the material itself, external physical stimulation can also affect stem cells. Elsaadany et al. (Elsaadany et al., 2017), evaluated the effects of different equiaxial mechanical strains and frequencies on the survival of ADMSCs in the scaffold and indicated that under suitable loading, ECM protein secretion and AD marker gene expression were significantly increased. Similarly, Li et al. (Li et al., 2021), used poly-caprolactone and nano-hydroxyapatite to fabricate scaffolds to load BMSCs, prior to treating these scaffolds with a sinusoidal electromagnetic field. The results indicated that stimulated BMSCs exhibited excellent osteogenic potential (Figure 3). Artificial polymer materials are easy to work with so they can be used to determine the relationship between material properties and stem cells. Tasi et al. (Tsai et al., 2014), used poly-l-lactic acid and poly-caprolactone to construct a fibrous scaffold mimicking the AF structure. Zhu et al. (Zhu et al., 2016a), used a biodegradable poly (ether carbonate urethane) urea material to achieve adjustable scaffold elasticity. AFSCs exhibited significant differences in protein expression on different elastic scaffolds. Chu et al. (Chu et al., 2019), further used this scaffold to demonstrate that the mechanical properties, topography, and geometric characteristics of the material affect the growth and differentiation of AFSCs.
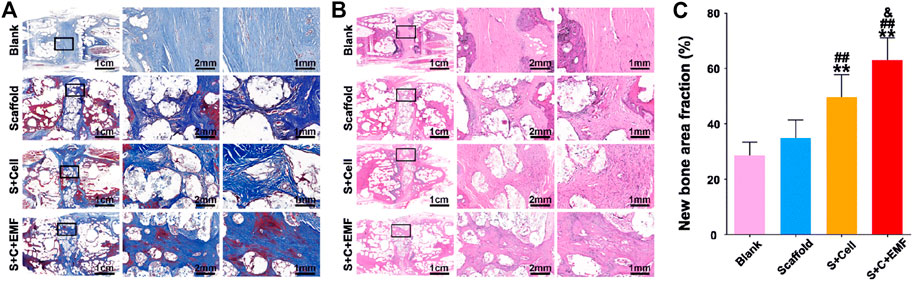
FIGURE 3. Masson trichrome staining (A) and HE staining (B) were used to observe the new bone formation in the scaffolds after 12 weeks of implantation. Quantification analysis of new bone fraction in four groups after 12 weeks of implantation (n = 6) (C). ** means p < 0.01 compared to the blank group, ## means p < 0.01 compared to the only scaffold group, &means p < 0.05 compared to the S + Cell group. EMF: electromagnetic field. Reproduced with permission from (Li et al., 2021).
Adding cytokines or drugs to the material offers more possibilities to control stem cell growth and differentiation in the material. TGF is an important signal factor for IVD development and repair, considering that it can stimulate ECM anabolism, induce stem cell differentiation, and inhibit inflammatory response and ECM catabolism (Watabe and Miyazono, 2009; Yang et al., 2015; Chen et al., 2019). Liang et al. (Liang et al., 2013), reported a cell carrier by adding nanoparticles containing dexamethasone and TGF-β3 to poly (lactide-co-glycolide) microspheres loaded with ADMSCs. At week 24 post-transplantation, the mice models showed significant disc height recovery and proteoglycan accumulation. Similar results were reported by Kim et al., (Kim M. J. et al., 2020), who engineered porous particles with leaf-stack structures to simultaneously load BMSCs and TGF-β3; such particles released TGF-β3 continuously for up to 18 days. As a result, significant disc regeneration was observed in dog IDD models. To further overcome the defects of rapid clearance and short half-life of TGF in vivo, Shen et al. (Shen et al., 2020), used graphene oxide nanosheets to achieve slow release of TGF. Whether cultured in vitro or implanted subcutaneously, the addition of this material caused BMSCs in the hydrogel to express more cartilage matrix. In addition, the initial compression strength of the hydrogel was also enhanced by graphene oxide nanosheets. Furthermore, the repair stimulatory effect of TGF on the NP and AF cells has also been demonstrated (Guillaume et al., 2014; Ligorio et al., 2021). Platelet-rich plasma (PRP) obtained by autologous whole blood centrifugation can provide a greater variety of bioactive factors than a single TGF component, and its application in regeneration medicine has received extensive attention in recent years (Everts et al., 2020; Everts et al., 2021). Chen et al. (Chen et al., 2009), used the culture system containing PRP to culture MSCs and observed the upregulation of genes that are related to type II collagen, aggrecan, and an increased cartilage matrix deposition in vivo. Wang et al. (Wang et al., 2018), further compared the effects of different doses of PRP and the presence or absence of leukocytes in PRP on NP stem cells, and at a 10% dose, PRP without leukocytes exerted the best differentiation effects on NP stem cells. Russo et al. (Russo et al., 2021), further developed a hydrogel composed of PRP and hyaluronic acid as the carrier to deliver the BMSCs, and this could integrate well with the surrounding tissue while maintaining cell viability (Figure 4).
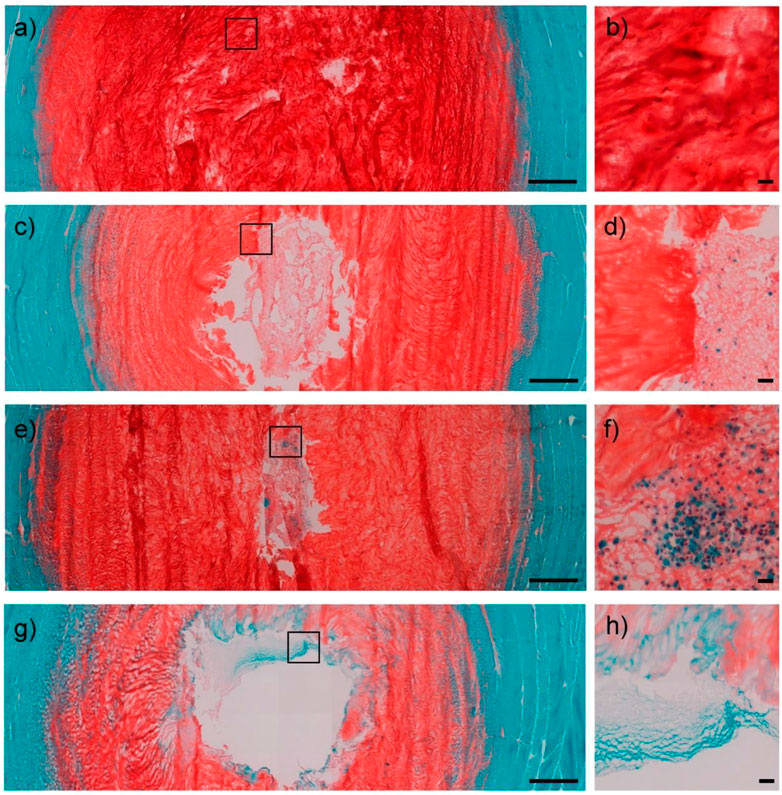
FIGURE 4. IVD cross-sections were visualized using safranin O/fast green staining. Healthy disc controls in low (1.25×) (A) and high (20×) (B) magnification images. Low cell density hydrogel (1 million MSCs) in low (1.25×) (C) and high (20×) (D) magnification images. High cell density hydrogel (2 million MSCs) in low (1.25×) (E) and high (20×) (F) magnification images. Only hydrogel group in low (1.25×) (G) and high (20×) (H) magnification images. Scale bar = 1,000 μm (low magnification); 50 μm (high magnification). Reproduced with permission from (Russo et al., 2021).
New tissue engineering technologies allow people to design and manipulate material properties more accurately. In recent years, an emerging technology called 3D bioprinting has attracted much attention in tissue engineering, a scenario that improves the control of the spatial distribution of materials to a new level (Shapira and Dvir, 2021; Zhu et al., 2016b; D'Este et al., 2018). Serra et al. (Serra et al., 2016), used 3D bioprinting to design an anatomical lumbar spine skeleton with similar mechanical properties to trabecular bone and demonstrated ideal biocompatibility in preliminary cell culture. Tarafder et al. (Tarafder et al., 2016), achieved precise spatial control of growth factor release by exploiting the differences in the melting points of various materials, in addition to interchanging dispensing cartridges during a single printing process. Electrospinning is a technology that can be used to produce ultrathin fibers. It makes it possible to reduce the diameter of ultrathin fibers to nanometer levels (Hong et al., 2019; Xue et al., 2019). Liu et al. (Liu et al., 2015a), fabricated an aligned fiber-polyurethane scaffold carrying AF stem cells using the electrospinning technology, and the AFSCs on this kind of scaffold were more aligned and produced more collagen Ⅰ and aggrecan (Figure 5). Wang et al. (Wang et al., 2020), compared the effects of static culture, intermittent centrifugation culture, and dynamic bioreactor on the infiltration ability of BMSCs in low-porosity electrospinning scaffolds. Zhu et al. (Zhu et al., 2021), combined 3D printing and electrospinning technology to construct a composite scaffold, which simulate the structure and properties of the NP and AF at the same time. The BMSCs that are distributed in the scaffold maintain ideal cell viability. In an in vivo experiment, new ECM production was observed and IVD height was maintained.
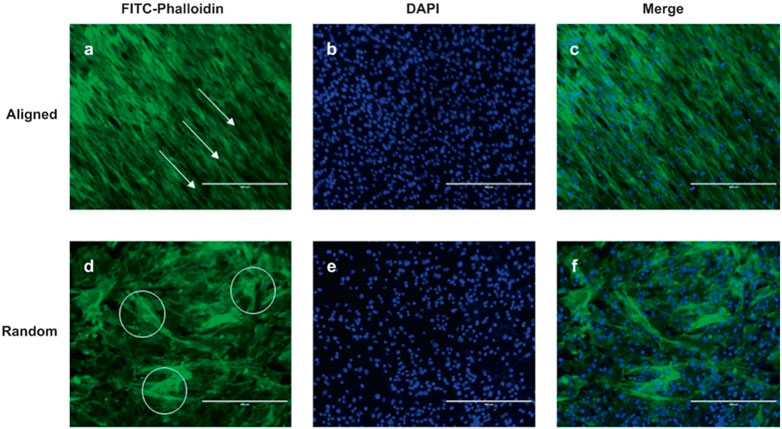
FIGURE 5. AF stem cell cytoskeletal images of aligned (A–C) and random (D–F) scaffolds were processed using FITC-phalloidin (green) and DAPI (blue) staining. White arrow and circle are the selected represent area. Scale bar = 400 μm. DAPI (4′,6-diamidino-2-phenylindole). Reproduced with permission from (Liu et al., 2015a).
3.3 New directions based on stem cells
Considering the unavoidable ethical and cell survival problems of stem cell therapy, extracellular vesicles based on stem cells have attracted attention as a new treatment method for IDD in recent years. Extracellular vesicles can be roughly divided into exosomes, microvesicles, and apoptotic bodies, according to their size. Exosomes are the smallest category, with a size of about 30–150 nm (Vader et al., 2016; Li et al., 2019). Apoptotic bodies are the largest, with a size of between 50 nm and 5 μm. Microvescicles are in the middle with a size of about 50–100 nm. Of all the extracellular vesicles, exosomes have been the most extensively studied. Almost all cells will produce exosomes, the outer layer is the lipid double layer, which contains a variety of substances like cytokines, proteins, lipids, mRNA, among others (DiStefano et al., 2022a; Bhujel et al., 2022). Due to their cell origin, exosomes have extremely low immunogenicity. As a carrier of intercellular information, exosomes can act on a variety of signal transduction pathways, such as fusion, endocytosis, and soluble signaling pathways (Zhang et al., 2019). A remarkable number of studies confirmed that stem cell-derived exosomes interfere with the pathological development of IDD through various mechanisms. Liao et al. (Liao et al., 2019), demonstrated that exosome-derived BMSCs could ameliorate excessive apoptosis in IDD, through endoplasmic reticulum stress. Similarly, Xiang et al. (Xiang et al., 2020), indicated that urine-derived stem cells could also regulate endoplasmic reticulum stress. Zhu et al. (Guo et al., 2021), further demonstrated that exosomes from urine-derived stem cells have the effect of promoting cell proliferation and ECM synthesis by regulating TGF-β. In an in vivo experiment using a rabbit IDD model, exosomes derived from MSCs also exhibited anti-oxidative and anti-inflammatory effects and prevented further degeneration. The combination of exosomes and biomaterials also has a broad application prospect. Luo et al. (Luo et al., 2022), designed a cartilage ECM hydrogel that was loaded with CEP stem cells and injected the hydrogel near the CEPs in mouse models. They observed that the exosomes produced by the stem cells penetrated the AFs to reach the NP cells and attenuated the development of IDD. Xing et al. (Xing et al., 2021), designed a thermosensitive hydrogel loaded with ADSC exosomes. The hydrogel provides a suitable environment for the growth of NP cells, and the exosomes regulate the anabolism and catabolism of the ECM by regulating metalloproteinases (Figure 6). DiStefano et al. (DiStefano et al., 2022b), loaded exosomes produced by MSCs under different oxygen concentrations into poly (lactic-co-glycolic acid) microspheres and showed that AF cells were more sensitive to the production of exosomes under hypoxic conditions.
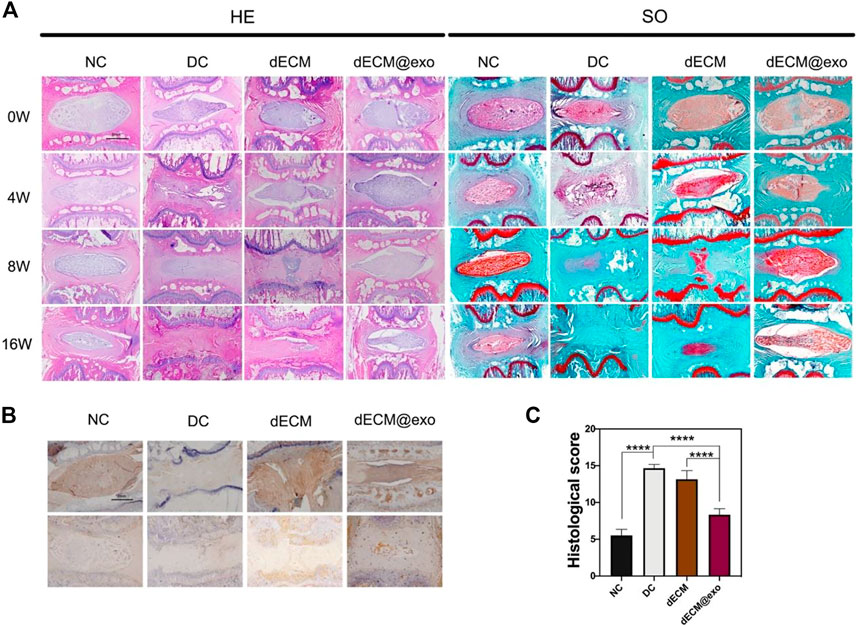
FIGURE 6. Images of each group were obtained by H&E and saffron O staining method at different times (A). Scale bar = 1 mm. Immunohistochemical images of aggrecan and collagen II were examined (B). Quantitative analysis of histological grade in each group at 16 weeks (C). *means p < 0.05. NC: normal disc group. DC: degenerated disc group. dECM: only the hydrogel was injected. dECM@exo: hydrogel containing exosomes were injected. Reproduced with permission from (Xing et al., 2021).
Generally, the exosome strategy avoids many problems that are difficult for stem cells to circumvent while retaining most of the advantages of the stem cell strategy. Although exosomes brought a new direction to regenerative medicine, their application in IDD is still in its infancy. Its formal application in clinical practice still requires extensive evaluation. The metabolism, distribution, appropriate dosage, and administration frequency of exosomes in the human body still need a lot of research. In addition, the differences in the expression of genetic information between different individuals may lead to significant variations in the therapeutic effects of exosomes. Therefore, a comprehensive and multi-level evaluation system is necessary. From the point of view of drug production, their large-scale preparation is still difficult, not to talk of a lack of unified standards. The storage and transportation of exosome also need to be considered.
4 Conclusion
The improvement effect of stem cells combined with biomaterials on IDD has been fully proved through a wealth of experiments. However, there are still many problems that are worth considering besides the benefits that comes with the application of the “stem cell-biomaterial” combo the human body. First, the structure of the IVD and the types of functional cells that are involved are complex. The pathological mechanism of IDD is also multifaceted. It is often difficult to obtain satisfactory results for the repair and supplementation of a tissue or cell. Second, despite the increasing understanding of various stem cells and the continuous developments in tissue engineering, choosing a personalized match according to the actual situation of patients still requires extensive pre-clinical testing. In addition to long-standing issues such as safety, cell origin, and ethics, large-scale preparation and production costs are key factors in that need to be carefully considered in practical applications. Finally, considering that IDD is not always accompanied by obvious symptoms, the procedure for screening patients with early IDD in a manner that is comprehensive enough to persuade them to employ timely interventions is a problem that needs to dealt with in clinical application.
Future research may focus on the following aspects: 1) A deeper understanding of the pathological mechanism of IDD and more effective therapeutic targets will provide more succinct directions for selecting drugs and preparing materials; 2) Further exploration of stem cell repair mechanisms, as well as efficient and stable differentiation induction methods; 3) Identification of materials with good properties that can withstand different mechanical requirements in various areas of IVD, in addition to providing a suitable microenvironment for cells; 4) Development of IDD models that closely imitate human IVD environment development and the corresponding in vivo experiments; 5) Earlier, faster, more economical screening techniques for IDD, as well as comprehensive and easy-to-accept patient education.
Author contributions
ZL provided the idea and wrote the original manuscript. YB revised the manuscript. GW and CF provided the idea and revised the manuscript. All authors have read and agreed to the published version of the manuscript.
Acknowledgments
The authors would like to express their gratitude to EditSprings (https://www.editsprings.cn) for the expert linguistic services provided.
Conflict of interest
The authors declare that the research was conducted in the absence of any commercial or financial relationships that could be construed as a potential conflict of interest.
Publisher’s note
All claims expressed in this article are solely those of the authors and do not necessarily represent those of their affiliated organizations, or those of the publisher, the editors and the reviewers. Any product that may be evaluated in this article, or claim that may be made by its manufacturer, is not guaranteed or endorsed by the publisher.
References
Adams, M. A., Mcnally, D. S., and Dolan, P. (1996). 'Stress' distributions inside intervertebral discs. The effects of age and degeneration. J. Bone Jt. Surg. Br. volume 78, 965–972. doi:10.1302/0301-620x.78b6.0780965
Ahn, Y. (2019). Endoscopic spine discectomy: Indications and outcomes. Int. Orthop. 43, 909–916. doi:10.1007/s00264-018-04283-w
Antoniou, J., Steffen, T., Nelson, F., Winterbottom, N., Hollander, A. P., Poole, R. A., et al. (1996). The human lumbar intervertebral disc: Evidence for changes in the biosynthesis and denaturation of the extracellular matrix with growth, maturation, ageing, and degeneration. J. Clin. Invest. 98, 996–1003. doi:10.1172/jci118884
Bertolo, A., HäFNER, S., Taddei, A. R., Baur, M., PöTZEL, T., Steffen, F., et al. (2015). Injectable microcarriers as human mesenchymal stem cell support and their application for cartilage and degenerated intervertebral disc repair. Eur. Cell. Mat. 29, 70–81. doi:10.22203/ecm.v029a06
Bertolo, A., Mehr, M., Aebli, N., Baur, M., Ferguson, S. J., and Stoyanov, J. V. (2012). Influence of different commercial scaffolds on the in vitro differentiation of human mesenchymal stem cells to nucleus pulposus-like cells. Eur. Spine J. 21 (6), S826–S838. doi:10.1007/s00586-011-1975-3
Bhujel, B., Shin, H. E., Choi, D. J., and Han, I. (2022). Mesenchymal stem cell-derived exosomes and intervertebral disc regeneration: Review. Int. J. Mol. Sci. 23, 7306. doi:10.3390/ijms23137306
Binch, A. L. A., Fitzgerald, J. C., Growney, E. A., and Barry, F. (2021). Cell-based strategies for IVD repair: Clinical progress and translational obstacles. Nat. Rev. Rheumatol. 17, 158–175. doi:10.1038/s41584-020-00568-w
Boubriak, O. A., Watson, N., Sivan, S. S., Stubbens, N., and Urban, J. P. (2013). Factors regulating viable cell density in the intervertebral disc: Blood supply in relation to disc height. J. Anat. 222, 341–348. doi:10.1111/joa.12022
Bowles, R. D., and Setton, L. A. (2017). Biomaterials for intervertebral disc regeneration and repair. Biomaterials 129, 54–67. doi:10.1016/j.biomaterials.2017.03.013
Brinjikji, W., Diehn, F. E., Jarvik, J. G., Carr, C. M., Kallmes, D. F., Murad, M. H., et al. (2015). MRI findings of disc degeneration are more prevalent in adults with low back pain than in asymptomatic controls: A systematic review and meta-analysis. AJNR. Am. J. Neuroradiol. 36, 2394–2399. doi:10.3174/ajnr.a4498
Cao, C., Zou, J., Liu, X., Shapiro, A., Moral, M., Luo, Z., et al. (2015). Bone marrow mesenchymal stem cells slow intervertebral disc degeneration through the NF-κB pathway. Spine J. 15, 530–538. doi:10.1016/j.spinee.2014.11.021
Chen, H., Zhang, Y., Yang, Z., and Zhang, H. (2013). Human umbilical cord Wharton's jelly-derived oligodendrocyte precursor-like cells for axon and myelin sheath regeneration. Neural Regen. Res. 8, 890–899. doi:10.3969/j.issn.1673-5374.2013.10.003
Chen, S., Liu, S., Ma, K., Zhao, L., Lin, H., and Shao, Z. (2019). TGF-β signaling in intervertebral disc health and disease. Osteoarthr. Cartil. 27, 1109–1117. doi:10.1016/j.joca.2019.05.005
Chen, W. H., Liu, H. Y., Lo, W. C., Wu, S. C., Chi, C. H., Chang, H. Y., et al. (2009). Intervertebral disc regeneration in an ex vivo culture system using mesenchymal stem cells and platelet-rich plasma. Biomaterials 30, 5523–5533. doi:10.1016/j.biomaterials.2009.07.019
Chu, G., Yuan, Z., Zhu, C., Zhou, P., Wang, H., Zhang, W., et al. (2019). Substrate stiffness- and topography-dependent differentiation of annulus fibrosus-derived stem cells is regulated by Yes-associated protein. Acta Biomater. 92, 254–264. doi:10.1016/j.actbio.2019.05.013
Clarke, L. E., Mcconnell, J. C., Sherratt, M. J., Derby, B., Richardson, S. M., and Hoyland, J. A. (2014). Growth differentiation factor 6 and transforming growth factor-beta differentially mediate mesenchymal stem cell differentiation, composition, and micromechanical properties of nucleus pulposus constructs. Arthritis Res. Ther. 16, R67. doi:10.1186/ar4505
Colombini, A., Lombardi, G., Corsi, M. M., and Banfi, G. (2008). Pathophysiology of the human intervertebral disc. Int. J. Biochem. Cell Biol. 40, 837–842. doi:10.1016/j.biocel.2007.12.011
Corp, N., Mansell, G., Stynes, S., Wynne-Jones, G., Morsø, L., Hill, J. C., et al. (2021). Evidence-based treatment recommendations for neck and low back pain across europe: A systematic review of guidelines. Eur. J. Pain 25, 275–295. doi:10.1002/ejp.1679
D'Este, M., Eglin, D., and Alini, M. (2018). Lessons to be learned and future directions for intervertebral disc biomaterials. Acta Biomater. 78, 13–22. doi:10.1016/j.actbio.2018.08.004
De Bree, K., De Bakker, B. S., and Oostra, R. J. (2018). The development of the human notochord. PLoS One 13, e0205752. doi:10.1371/journal.pone.0205752
Diba, M., Wang, H., Kodger, T. E., Parsa, S., and Leeuwenburgh, S. C. (2017). Highly elastic and self-healing composite colloidal gels. Adv. Mat. 29, 1604672. doi:10.1002/adma.201604672
Diekman, B. O., Rowland, C. R., Lennon, D. P., Caplan, A. I., and Guilak, F. (2010). Chondrogenesis of adult stem cells from adipose tissue and bone marrow: Induction by growth factors and cartilage-derived matrix. Tissue Eng. Part A 16, 523–533. doi:10.1089/ten.tea.2009.0398
Dieleman, J. L., Cao, J., Chapin, A., Chen, C., Li, Z., Liu, A., et al. (2020). US health care spending by payer and health condition, 1996-2016. Jama 323, 863–884. doi:10.1001/jama.2020.0734
Distefano, T. J., Vaso, K., Danias, G., Chionuma, H. N., Weiser, J. R., and Iatridis, J. C. (2022a). Extracellular vesicles as an emerging treatment option for intervertebral disc degeneration: Therapeutic potential, translational pathways, and regulatory considerations. Adv. Healthc. Mat. 11, e2100596. doi:10.1002/adhm.202100596
Distefano, T. J., Vaso, K., Panebianco, C. J., Danias, G., Chionuma, H. N., Kunnath, K., et al. (2022b). Hydrogel-Embedded poly(lactic-co-glycolic acid) microspheres for the delivery of hMSC-derived exosomes to promote bioactive annulus fibrosus repair. Cartilage 13, 194760352211139. doi:10.1177/19476035221113959
Elsaadany, M., Winters, K., Adams, S., Stasuk, A., Ayan, H., and Yildirim-Ayan, E. (2017). Equiaxial strain modulates adipose-derived stem cell differentiation within 3D biphasic scaffolds towards annulus fibrosus. Sci. Rep. 7, 12868. doi:10.1038/s41598-017-13240-3
Everts, P. A., Van Erp, A., Desimone, A., Cohen, D. S., and Gardner, R. D. (2021). Platelet rich plasma in orthopedic surgical medicine. Platelets 32, 163–174. doi:10.1080/09537104.2020.1869717
Everts, P., Onishi, K., Jayaram, P., Lana, J. F., and Mautner, K. (2020). Platelet-rich plasma: New performance understandings and therapeutic considerations in 2020. Int. J. Mol. Sci. 21, 7794. doi:10.3390/ijms21207794
Feng, H., Danfelter, M., StröMQVIST, B., and HeinegåRD, D. (2006). Extracellular matrix in disc degeneration. J. Bone Jt. Surg. 88 (2), 25–29. doi:10.2106/jbjs.e.01341
Francisco, V., Pino, J., GonzáLEZ-Gay, M., Lago, F., Karppinen, J., Tervonen, O., et al. (2022). A new immunometabolic perspective of intervertebral disc degeneration. Nat. Rev. Rheumatol. 18, 47–60. doi:10.1038/s41584-021-00713-z
Friedmann, A., Baertel, A., Schmitt, C., Ludtka, C., Milosevic, J., Meisel, H. J., et al. (2021). Intervertebral disc regeneration injection of a cell-loaded collagen hydrogel in a sheep model. Int. J. Mol. Sci. 22, 4248. doi:10.3390/ijms22084248
Gadjradj, P. S., Harhangi, B. S., Amelink, J., Van Susante, J., Kamper, S., Van Tulder, M., et al. (2021). Percutaneous transforaminal endoscopic discectomy versus open microdiscectomy for lumbar disc herniation: A systematic review and meta-analysis. Spine (Phila Pa 1976) 46, 538–549. doi:10.1097/brs.0000000000003843
GarzóN, I., MartíN-Piedra, M. A., Alfonso-RodríGUEZ, C., GonzáLEZ-Andrades, M., Carriel, V., MartíNEZ-GóMEZ, C., et al. (2014). Generation of a biomimetic human artificial cornea model using Wharton's jelly mesenchymal stem cells. Invest. Ophthalmol. Vis. Sci. 55, 4073–4083. doi:10.1167/iovs.14-14304
Guillaume, O., Daly, A., Lennon, K., Gansau, J., Buckley, S. F., and Buckley, C. T. (2014). Shape-memory porous alginate scaffolds for regeneration of the annulus fibrosus: Effect of TGF-β3 supplementation and oxygen culture conditions. Acta Biomater. 10, 1985–1995. doi:10.1016/j.actbio.2013.12.037
Guillaume, O., Naqvi, S. M., Lennon, K., and Buckley, C. T. (2015). Enhancing cell migration in shape-memory alginate-collagen composite scaffolds: In vitro and ex vivo assessment for intervertebral disc repair. J. Biomater. Appl. 29, 1230–1246. doi:10.1177/0885328214557905
Guo, Z., Su, W., Zhou, R., Zhang, G., Yang, S., Wu, X., et al. (2021). Exosomal MATN3 of urine-derived stem cells ameliorates intervertebral disc degeneration by antisenescence effects and promotes NPC proliferation and ECM synthesis by activating TGF-β. Oxid. Med. Cell. Longev. 2021, 5542241–5542318. doi:10.1155/2021/5542241
Han, X. B., Zhang, Y. L., Li, H. Y., Chen, B., Chang, X., Zhang, W., et al. (2015). Differentiation of human ligamentum flavum stem cells toward nucleus pulposus-like cells induced by coculture system and hypoxia. Spine (Phila Pa 1976) 40, E665–E674. doi:10.1097/brs.0000000000000882
Harfe, B. D. (2022). Intervertebral disc repair and regeneration: Insights from the notochord. Semin. Cell Dev. Biol. 127, 3–9. doi:10.1016/j.semcdb.2021.11.012
Harmon, M. D., Ramos, D. M., Nithyadevi, D., Bordett, R., Rudraiah, S., Nukavarapu, S. P., et al. (2020). Growing a backbone - functional biomaterials and structures for intervertebral disc (IVD) repair and regeneration: Challenges, innovations, and future directions. Biomater. Sci. 8, 1216–1239. doi:10.1039/c9bm01288e
He, Z., Jia, M., Yu, Y., Yuan, C., and Wang, J. (2018). Roles of SDF-1/CXCR4 axis in cartilage endplate stem cells mediated promotion of nucleus pulposus cells proliferation. Biochem. Biophys. Res. Commun. 506, 94–101. doi:10.1016/j.bbrc.2018.10.069
Hong, J., Yeo, M., Yang, G. H., and Kim, G. (2019). Cell-electrospinning and its application for tissue engineering. Int. J. Mol. Sci. 20, 6208. doi:10.3390/ijms20246208
Hsieh, A. H., and Twomey, J. D. (2010). Cellular mechanobiology of the intervertebral disc: New directions and approaches. J. Biomech. 43, 137–145. doi:10.1016/j.jbiomech.2009.09.019
Huang, Y. C., Urban, J. P., and Luk, K. D. (2014). Intervertebral disc regeneration: Do nutrients lead the way? Nat. Rev. Rheumatol. 10, 561–566. doi:10.1038/nrrheum.2014.91
Jin, E. S., Min, J., Jeon, S. R., Choi, K. H., and Jeong, J. H. (2013). Analysis of molecular expression in adipose tissue-derived mesenchymal stem cells : Prospects for use in the treatment of intervertebral disc degeneration. J. Korean Neurosurg. Soc. 53, 207–212. doi:10.3340/jkns.2013.53.4.207
Kamali, A., Ziadlou, R., Lang, G., Pfannkuche, J., Cui, S., Li, Z., et al. (2021). Small molecule-based treatment approaches for intervertebral disc degeneration: Current options and future directions. Theranostics 11, 27–47. doi:10.7150/thno.48987
Kangari, P., Talaei-Khozani, T., Razeghian-Jahromi, I., and Razmkhah, M. (2020). Mesenchymal stem cells: Amazing remedies for bone and cartilage defects. Stem Cell Res. Ther. 11, 492. doi:10.1186/s13287-020-02001-1
Katz, J. N., Zimmerman, Z. E., Mass, H., and Makhni, M. C. (2022). Diagnosis and management of lumbar spinal stenosis: A review. Jama 327, 1688–1699. doi:10.1001/jama.2022.5921
Kim, H. S., Wu, P. H., and Jang, I. T. (2020). Lumbar degenerative disease Part 1: Anatomy and pathophysiology of intervertebral discogenic pain and radiofrequency ablation of basivertebral and sinuvertebral nerve treatment for chronic discogenic back pain: A prospective case series and review of literature. Int. J. Mol. Sci. 21, 1483. doi:10.3390/ijms21041483
Kim, M. J., Lee, J. H., Kim, J. S., Kim, H. Y., Lee, H. C., Byun, J. H., et al. (2020). Intervertebral disc regeneration using stem cell/growth factor-loaded porous particles with a leaf-stacked structure. Biomacromolecules 21, 4795–4805. doi:10.1021/acs.biomac.0c00992
Kumar, H., Ha, D. H., Lee, E. J., Park, J. H., Shim, J. H., Ahn, T. K., et al. (2017). Safety and tolerability of intradiscal implantation of combined autologous adipose-derived mesenchymal stem cells and hyaluronic acid in patients with chronic discogenic low back pain: 1-year follow-up of a phase I study. Stem Cell Res. Ther. 8, 262. doi:10.1186/s13287-017-0710-3
Li, J. J., Hosseini-Beheshti, E., Grau, G. E., Zreiqat, H., and Little, C. B. (2019). Stem Cell-Derived Extracellular Vesicles for Treating Joint Injury and Osteoarthritis, 9. Nanomaterials (Basel).
Li, W., Huang, C., Ma, T., Wang, J., Liu, W., Yan, J., et al. (2021). Low-frequency electromagnetic fields combined with tissue engineering techniques accelerate intervertebral fusion. Stem Cell Res. Ther. 12, 143. doi:10.1186/s13287-021-02207-x
Liang, C. Z., Li, H., Tao, Y. Q., Peng, L. H., Gao, J. Q., Wu, J. J., et al. (2013). Dual release of dexamethasone and TGF-β3 from polymeric microspheres for stem cell matrix accumulation in a rat disc degeneration model. Acta Biomater. 9, 9423–9433. doi:10.1016/j.actbio.2013.08.019
Liao, Z., Luo, R., Li, G., Song, Y., Zhan, S., Zhao, K., et al. (2019). Exosomes from mesenchymal stem cells modulate endoplasmic reticulum stress to protect against nucleus pulposus cell death and ameliorate intervertebral disc degeneration in vivo. Theranostics 9, 4084–4100. doi:10.7150/thno.33638
Ligorio, C., O'Brien, M., Hodson, N. W., Mironov, A., Iliut, M., Miller, A. F., et al. (2021). TGF-β3-loaded graphene oxide - self-assembling peptide hybrid hydrogels as functional 3D scaffolds for the regeneration of the nucleus pulposus. Acta Biomater. 127, 116–130. doi:10.1016/j.actbio.2021.03.077
Liu, C., Zhu, C., Li, J., Zhou, P., Chen, M., Yang, H., et al. (2015a). The effect of the fibre orientation of electrospun scaffolds on the matrix production of rabbit annulus fibrosus-derived stem cells. Bone Res. 3, 15012. doi:10.1038/boneres.2015.12
Liu, Y., Fu, S., Rahaman, M. N., Mao, J. J., and Bal, B. S. (2015b). Native nucleus pulposus tissue matrix promotes notochordal differentiation of human induced pluripotent stem cells with potential for treating intervertebral disc degeneration. J. Biomed. Mat. Res. A 103, 1053–1059. doi:10.1002/jbm.a.35243
Lu, Z. F., Doulabi, B. Z., Wuisman, P. I., Bank, R. A., and Helder, M. N. (2008). Influence of collagen type II and nucleus pulposus cells on aggregation and differentiation of adipose tissue-derived stem cells. J. Cell. Mol. Med. 12, 2812–2822. doi:10.1111/j.1582-4934.2008.00278.x
Luo, L., Gong, J., Wang, Z., Liu, Y., Cao, J., Qin, J., et al. (2022). Injectable cartilage matrix hydrogel loaded with cartilage endplate stem cells engineered to release exosomes for non-invasive treatment of intervertebral disc degeneration. Bioact. Mat. 15, 29–43. doi:10.1016/j.bioactmat.2021.12.007
Luo, L., Jian, X., Sun, H., Qin, J., Wang, Y., Zhang, J., et al. (2021). Cartilage endplate stem cells inhibit intervertebral disc degeneration by releasing exosomes to nucleus pulposus cells to activate Akt/autophagy. Stem Cells 39, 467–481. doi:10.1002/stem.3322
Luoma, K., RiihimäKI, H., Luukkonen, R., Raininko, R., Viikari-Juntura, E., and Lamminen, A. (2000). Low back pain in relation to lumbar disc degeneration. Spine (Phila Pa 1976) 25, 487–492. doi:10.1097/00007632-200002150-00016
Lurie, J., and Tomkins-Lane, C. (2016). Management of lumbar spinal stenosis. Bmj 352, h6234. doi:10.1136/bmj.h6234
Malandrino, A., Lacroix, D., Hellmich, C., Ito, K., Ferguson, S. J., and Noailly, J. (2014). The role of endplate poromechanical properties on the nutrient availability in the intervertebral disc. Osteoarthr. Cartil. 22, 1053–1060. doi:10.1016/j.joca.2014.05.005
Manoukian, O. S., Aravamudhan, A., Lee, P., Arul, M. R., Yu, X., Rudraiah, S., et al. (2018). Spiral layer-by-layer micro-nanostructured scaffolds for bone tissue engineering. ACS Biomater. Sci. Eng. 4, 2181–2192. doi:10.1021/acsbiomaterials.8b00393
Marchand, F., and Ahmed, A. M. (1990). Investigation of the laminate structure of lumbar disc anulus fibrosus. Spine (Phila Pa 1976) 15, 402–410. doi:10.1097/00007632-199005000-00011
Maroudas, A., Stockwell, R. A., Nachemson, A., and Urban, J. (1975). Factors involved in the nutrition of the human lumbar intervertebral disc: Cellularity and diffusion of glucose in vitro. J. Anat. 120, 113–130.
Melrose, J., Ghosh, P., and Taylor, T. K. (2001). A comparative analysis of the differential spatial and temporal distributions of the large (aggrecan, versican) and small (decorin, biglycan, fibromodulin) proteoglycans of the intervertebral disc. J. Anat. 198, 3–15. doi:10.1046/j.1469-7580.2001.19810003.x
Owens, P. L., Woeltje, M., and Mutter, R. (2006). Emergency department visits and inpatient stays related to back problems, 2008. Healthcare Cost and utilization project (HCUP) statistical briefs. Rockville (MD): Agency for Healthcare Research and Quality (US.
Peroglio, M., Eglin, D., Benneker, L. M., Alini, M., and Grad, S. (2013). Thermoreversible hyaluronan-based hydrogel supports in vitro and ex vivo disc-like differentiation of human mesenchymal stem cells. Spine J. 13, 1627–1639. doi:10.1016/j.spinee.2013.05.029
Qi, X., Zhang, J., Yuan, H., Xu, Z., Li, Q., Niu, X., et al. (2016). Exosomes secreted by human-induced pluripotent stem cell-derived mesenchymal stem cells repair critical-sized bone defects through enhanced angiogenesis and osteogenesis in osteoporotic rats. Int. J. Biol. Sci. 12, 836–849. doi:10.7150/ijbs.14809
Raj, P. P. (2008). Intervertebral disc: Anatomy-physiology-pathophysiology-treatment. Pain Pract. 8, 18–44. doi:10.1111/j.1533-2500.2007.00171.x
Rider, S. M., Mizuno, S., and Kang, J. D. (2019). Molecular mechanisms of intervertebral disc degeneration. Spine Surg. Relat. Res. 3, 1–11. doi:10.22603/ssrr.2017-0095
Risbud, M. V., and Shapiro, I. M. (2011). Notochordal cells in the adult intervertebral disc: New perspective on an old question. Crit. Rev. Eukaryot. Gene Expr. 21, 29–41. doi:10.1615/critreveukargeneexpr.v21.i1.30
Roberts, S., Evans, H., Trivedi, J., and Menage, J. (2006). Histology and pathology of the human intervertebral disc. J. Bone Jt. Surg. 88 (2), 10–14. doi:10.2106/jbjs.f.00019
Russo, F., Ambrosio, L., Peroglio, M., Guo, W., Wangler, S., Gewiess, J., et al. (2021). A hyaluronan and platelet-rich plasma hydrogel for mesenchymal stem cell delivery in the intervertebral disc: An organ culture study. Int. J. Mol. Sci. 22, 2963. doi:10.3390/ijms22062963
Sakai, D., Mochida, J., Iwashina, T., Hiyama, A., Omi, H., Imai, M., et al. (2006). Regenerative effects of transplanting mesenchymal stem cells embedded in atelocollagen to the degenerated intervertebral disc. Biomaterials 27, 335–345. doi:10.1016/j.biomaterials.2005.06.038
Schultz, A., Andersson, G., Ortengren, R., Haderspeck, K., and Nachemson, A. (1982). Loads on the lumbar spine. Validation of a biomechanical analysis by measurements of intradiscal pressures and myoelectric signals. J. Bone Jt. Surg. 64, 713–720. doi:10.2106/00004623-198264050-00008
Serra, T., Capelli, C., Toumpaniari, R., Orriss, I. R., Leong, J. J., Dalgarno, K., et al. (2016). Design and fabrication of 3D-printed anatomically shaped lumbar cage for intervertebral disc (IVD) degeneration treatment. Biofabrication 8, 035001. doi:10.1088/1758-5090/8/3/035001
Shapira, A., and Dvir, T. (2021). 3D tissue and organ printing-hope and reality. Adv. Sci. (Weinh). 8, 2003751. doi:10.1002/advs.202003751
Sheikh, H., Zakharian, K., De La Torre, R. P., Facek, C., Vasquez, A., Chaudhry, G. R., et al. (2009). In vivo intervertebral disc regeneration using stem cell-derived chondroprogenitors. J. Neurosurg. Spine 10, 265–272. doi:10.3171/2008.12.spine0835
Shen, H., Lin, H., Sun, A. X., Song, S., Wang, B., Yang, Y., et al. (2020). Acceleration of chondrogenic differentiation of human mesenchymal stem cells by sustained growth factor release in 3D graphene oxide incorporated hydrogels. Acta Biomater. 105, 44–55. doi:10.1016/j.actbio.2020.01.048
Smith, L. J., Nerurkar, N. L., Choi, K. S., Harfe, B. D., and Elliott, D. M. (2011). Degeneration and regeneration of the intervertebral disc: Lessons from development. Dis. Model. Mech. 4, 31–41. doi:10.1242/dmm.006403
Stairmand, J. W., Holm, S., and Urban, J. P. (1991). Factors influencing oxygen concentration gradients in the intervertebral disc. A theoretical analysis. Spine (Phila Pa 1976) 16, 444–449. doi:10.1097/00007632-199104000-00010
Strassburg, S., Richardson, S. M., Freemont, A. J., and Hoyland, J. A. (2010). Co-culture induces mesenchymal stem cell differentiation and modulation of the degenerate human nucleus pulposus cell phenotype. Regen. Med. 5, 701–711. doi:10.2217/rme.10.59
Tarafder, S., Koch, A., Jun, Y., Chou, C., Awadallah, M. R., and Lee, C. H. (2016). Micro-precise spatiotemporal delivery system embedded in 3D printing for complex tissue regeneration. Biofabrication 8, 025003. doi:10.1088/1758-5090/8/2/025003
Teixeira, G. Q., Pereira, C. L., Ferreira, J. R., Maia, A. F., Gomez-Lazaro, M., Barbosa, M. A., et al. (2018). Immunomodulation of human mesenchymal stem/stromal cells in intervertebral disc degeneration: Insights from a proinflammatory/degenerative ex vivo model. Spine (Phila Pa 1976) 43, E673–e682. doi:10.1097/brs.0000000000002494
Thompson, J. P., Oegema, T. R., and Bradford, D. S. (1991). Stimulation of mature canine intervertebral disc by growth factors. Spine (Phila Pa 1976) 16, 253–260. doi:10.1097/00007632-199103000-00001
Tsai, T. L., Nelson, B. C., Anderson, P. A., Zdeblick, T. A., and Li, W. J. (2014). Intervertebral disc and stem cells cocultured in biomimetic extracellular matrix stimulated by cyclic compression in perfusion bioreactor. Spine J. 14, 2127–2140. doi:10.1016/j.spinee.2013.11.062
Ukeba, D., Sudo, H., Tsujimoto, T., Ura, K., Yamada, K., and Iwasaki, N. (2020). Bone marrow mesenchymal stem cells combined with ultra-purified alginate gel as a regenerative therapeutic strategy after discectomy for degenerated intervertebral discs. EBioMedicine 53, 102698. doi:10.1016/j.ebiom.2020.102698
Urban, J. P., Smith, S., and Fairbank, J. C. (2004). Nutrition of the intervertebral disc. Spine (Phila Pa 1976) 29, 2700–2709. doi:10.1097/01.brs.0000146499.97948.52
Vader, P., Mol, E. A., Pasterkamp, G., and Schiffelers, R. M. (2016). Extracellular vesicles for drug delivery. Adv. Drug Deliv. Rev. 106, 148–156. doi:10.1016/j.addr.2016.02.006
Vergroesen, P. P., Kingma, I., Emanuel, K. S., Hoogendoorn, R. J., Welting, T. J., Van Royen, B. J., et al. (2015). Mechanics and biology in intervertebral disc degeneration: A vicious circle. Osteoarthr. Cartil. 23, 1057–1070. doi:10.1016/j.joca.2015.03.028
Walker, M. H., and Anderson, D. G. (2004). Molecular basis of intervertebral disc degeneration. Spine J. 4, 158s–166s. doi:10.1016/j.spinee.2004.07.010
Wang, S., He, Y. F., Ma, J., Yu, L., Wen, J. K., and Ye, X. J. (2020). Dynamic bioreactor culture for infiltration of bone mesenchymal stem cells within electrospun nanofibrous scaffolds for annulus fibrosus repair. Orthop. Surg. 12, 304–311. doi:10.1111/os.12615
Wang, S. Z., Fan, W. M., Jia, J., Ma, L. Y., Yu, J. B., and Wang, C. (2018). Is exclusion of leukocytes from platelet-rich plasma (PRP) a better choice for early intervertebral disc regeneration? Stem Cell Res. Ther. 9, 199. doi:10.1186/s13287-018-0937-7
Watabe, T., and Miyazono, K. (2009). Roles of TGF-beta family signaling in stem cell renewal and differentiation. Cell Res. 19, 103–115. doi:10.1038/cr.2008.323
Wu, X., Ma, Y., Ding, R., Xiao, X., and Yang, D. (2021). Should adjacent asymptomatic lumbar disc herniation be simultaneously rectified? A retrospective cohort study of 371 cases that received an open fusion or endoscopic discectomy only on symptomatic segments. Spine J. 21, 411–417. doi:10.1016/j.spinee.2020.09.003
Xiang, H., Su, W., Wu, X., Chen, W., Cong, W., Yang, S., et al. (2020). Exosomes derived from human urine-derived stem cells inhibit intervertebral disc degeneration by ameliorating endoplasmic reticulum stress. Oxid. Med. Cell. Longev. 2020, 1–21. doi:10.1155/2020/6697577
Xing, H., Zhang, Z., Mao, Q., Wang, C., Zhou, Y., Zhou, X., et al. (2021). Injectable exosome-functionalized extracellular matrix hydrogel for metabolism balance and pyroptosis regulation in intervertebral disc degeneration. J. Nanobiotechnology 19, 264. doi:10.1186/s12951-021-00991-5
Xue, J., Wu, T., Dai, Y., and Xia, Y. (2019). Electrospinning and electrospun nanofibers: Methods, materials, and applications. Chem. Rev. 119, 5298–5415. doi:10.1021/acs.chemrev.8b00593
Yang, H., Cao, C., Wu, C., Yuan, C., Gu, Q., Shi, Q., et al. (2015). TGF-Βl suppresses inflammation in cell therapy for intervertebral disc degeneration. Sci. Rep. 5, 13254. doi:10.1038/srep13254
Yang, Y., Lin, H., Shen, H., Wang, B., Lei, G., and Tuan, R. S. (2018). Mesenchymal stem cell-derived extracellular matrix enhances chondrogenic phenotype of and cartilage formation by encapsulated chondrocytes in vitro and in vivo. Acta Biomater. 69, 71–82. doi:10.1016/j.actbio.2017.12.043
Zakrzewski, W., Dobrzyński, M., Szymonowicz, M., and Rybak, Z. (2019). Stem cells: Past, present, and future. Stem Cell Res. Ther. 10, 68. doi:10.1186/s13287-019-1165-5
Zeng, Y., Chen, C., Liu, W., Fu, Q., Han, Z., Li, Y., et al. (2015). Injectable microcryogels reinforced alginate encapsulation of mesenchymal stromal cells for leak-proof delivery and alleviation of canine disc degeneration. Biomaterials 59, 53–65. doi:10.1016/j.biomaterials.2015.04.029
Zhang, W., Sun, T., Li, Y., Yang, M., Zhao, Y., Liu, J., et al. (2022). Application of stem cells in the repair of intervertebral disc degeneration. Stem Cell Res. Ther. 13, 70. doi:10.1186/s13287-022-02745-y
Zhang, Y., Liu, Y., Liu, H., and Tang, W. H. (2019). Exosomes: Biogenesis, biologic function and clinical potential. Cell Biosci. 9, 19. doi:10.1186/s13578-019-0282-2
Zhang, Z., Li, F., Tian, H., Guan, K., Zhao, G., Shan, J., et al. (2014). Differentiation of adipose-derived stem cells toward nucleus pulposus-like cells induced by hypoxia and a three-dimensional chitosan-alginate gel scaffold in vitro. Chin. Med. J. 127, 314–321.
Zhao, Y. T., Qin, Y., Yang, J. S., Huang, D. G., Hu, H. M., Wang, X. D., et al. (2020). Wharton's Jelly-derived mesenchymal stem cells suppress apoptosis of nucleus pulposus cells in intervertebral disc degeneration via Wnt pathway. Eur. Rev. Med. Pharmacol. Sci. 24, 9807–9814. doi:10.26355/eurrev_202010_23190
Zhu, C., Li, J., Liu, C., Zhou, P., Yang, H., and Li, B. (2016a). Modulation of the gene expression of annulus fibrosus-derived stem cells using poly(ether carbonate urethane)urea scaffolds of tunable elasticity. Acta Biomater. 29, 228–238. doi:10.1016/j.actbio.2015.09.039
Zhu, M., Tan, J., Liu, L., Tian, J., Li, L., Luo, B., et al. (2021). Construction of biomimetic artificial intervertebral disc scaffold via 3D printing and electrospinning. Mater. Sci. Eng. C 128, 112310. doi:10.1016/j.msec.2021.112310
Keywords: intervertebral disc, intervertebral disc degeneration, low back pain, stem cell, biomaterial, regenerative medicine
Citation: Liu Z, Bian Y, Wu G and Fu C (2022) Application of stem cells combined with biomaterial in the treatment of intervertebral disc degeneration. Front. Bioeng. Biotechnol. 10:1077028. doi: 10.3389/fbioe.2022.1077028
Received: 22 October 2022; Accepted: 18 November 2022;
Published: 25 November 2022.
Edited by:
Jinshan Guo, Southern Medical University, ChinaReviewed by:
Pingqiang Cai, Nanjing University, ChinaJun Yue, Sun Yat-sen University, China
Meihan Tao, Southern Medical University, China
Copyright © 2022 Liu, Bian, Wu and Fu. This is an open-access article distributed under the terms of the Creative Commons Attribution License (CC BY). The use, distribution or reproduction in other forums is permitted, provided the original author(s) and the copyright owner(s) are credited and that the original publication in this journal is cited, in accordance with accepted academic practice. No use, distribution or reproduction is permitted which does not comply with these terms.
*Correspondence: Guangzhi Wu, d3VndWFuZ3poaUBqbHUuZWR1LmNu; Changfeng Fu, ZnVjZkBqbHUuZWR1LmNu