- 1Centre for Medicines Discovery, Nuffield Department of Medicine, University of Oxford, Oxford, United Kingdom
- 2Kennedy Institute of Rheumatology, University of Oxford, Oxford, United Kingdom
- 3ExpreS2ion Biotechnologies, SCION-DTU Science Park, Hørsholm, Denmark
Recombinant protein expression in eukaryotic insect cells is a powerful approach for producing challenging targets. However, due to incompatibility with standard baculoviral platforms and existing low-throughput methodology, the use of the Drosophila melanogaster “S2” cell line lags behind more common insect cell lines such as Sf9 or High-Five™. Due to the advantages of S2 cells, particularly for secreted and secretable proteins, the lack of a simple and parallelizable S2-based platform represents a bottleneck, particularly for biochemical and biophysical laboratories. Therefore, we developed FAS2FURIOUS, a simple and rapid S2 expression pipeline built upon an existing low-throughput commercial platform. FAS2FURIOUS is comparable in effort to simple E. coli systems and allows users to clone and test up to 46 constructs in just 2 weeks. Given the ability of S2 cells to express challenging targets, including receptor ectodomains, secreted glycoproteins, and viral antigens, FAS2FURIOUS represents an attractive orthogonal approach for protein expression in eukaryotic cells.
1 Introduction
Recombinant protein expression in eukaryotic insect cell–derived systems, particularly Spodoptera frugiperda (Sf9/Sf21) and Trichoplusia ni (BTI-TN-5BI-4 or High-Five™), greatly expanded the number and complexity of proteins amenable to biochemical, structural, and functional studies. Insect cells can accommodate many posttranslational modifications and handle challenging folds, including human integral membrane proteins, and are easier, cheaper, and quicker to handle than comparable human cell–derived systems such as HEK293 or CHO. As a result, insect cell systems are routinely used for both bespoke and high-throughput protein expression, with innovative baculoviral technologies such as Bac-to-Bac®, flashBAC™, MultiBac™, and FlexiBAC allowing robust production of single proteins and multiprotein complexes (Luckow et al., 1993; Fitzgerald et al., 2006; Hitchman et al., 2010; and Lemaitre et al., 2019).
However, these baculoviral innovations failed to increase the uptake of an orthogonal insect cell line derived from Drosophila melanogaster: Schneider 2 cells (S2). Baculoviral systems rely on the Autographa californica nuclear polyhedrosis virus (AcNPV) which can only propagate in the lepidopteran species of insect cells, preventing its use in S2 and other D. melanogaster–derived cell lines. While some evidence suggests that promoter and protocol variations can rescue baculovirus-mediated gene delivery in Drosophila, nearly all commercial insect cell expression systems rely on a polyhedron promoter that is incompatible with D. melanogaster (Carbonell et al., 1985; Lee et al., 2000).
The Drosophila Expression System (DES™), marketed by Invitrogen, overcomes this challenge via bypassing the baculoviral stage and instead relies on directly co-transfecting a selection plasmid with a target-containing plasmid to select polyclonal stable cell lines. The DES™ expression system has been successfully used to generate many valuable proteins, but to our knowledge it has never been adapted to the high-throughput (HT) requirements of many structural biology laboratories (Moraes et al., 2012). At the Centre for Medicines Discovery (CMD), we routinely use and share protocols for HT baculoviral–mediated expression of proteins in Sf9 and High Five™ cells for structural characterization (Savitsky et al., 2010). However, even these robust pipelines failed as we pushed toward more challenging targets, particularly receptor ectodomains, secreted glycoproteins, and extracellular matrix components, which in preliminary experiments expressed well in S2 cells.
Secreted protein expression in S2 cells offers particular advantages for structural laboratories. Due to their smaller size, S2 cells grow much faster and to much higher densities (>6.0 × 107 cells/ml) than other insect cells, reducing culture volumes and cost (Pamboukian et al., 2008). S2 cells tolerate diverse growth conditions, including multiple serum-free and serum-containing commercially available media; all culture styles including T-flasks, shake flasks, and bio-reactors; and a wide temperature range (22–30°C) (Moraes et al., 2012). S2 cells are capable of complex eukaryotic posttranslational modifications, including mostly paucimannosidic N-linked glycosylation (Vandenborre et al., 2011). S2 cells are “powerhouse” protein secretors, with recombinant protein yields >30 mg/L allowing industrial-scale production of challenging proteins, including a malaria vaccine, West Nile virus vaccine, HER-2 vaccine, virus-like particles, and antibodies (Adriaan de Jongh et al., 2013). S2-derived proteins led to the deposition of more than 60 structures into the PDB, including receptor ectodomains, viral glycoproteins, enzymes, hormones, and growth factors. Interested readers are directed to excellent reviews which cover the details of S2 systems (Moraes et al., 2012; Adriaan de Jongh et al., 2013; Zitzmann et al., 2017).
Although S2 cells have been used to produce many cytosolic and even membrane proteins, particularly GPCRs (Perret et al., 2003), the real strength of the system, in our experience, is for secreted and “secretable” proteins. Because of their origin from Drosophila macrophages, S2 cells are uniquely tuned for protein secretion and in our hands outperform the more common High Five™ cell line, in part because S2 cells grow to significantly higher cell densities. Secreted proteins undergo packaging in the endoplasmic reticulum and Golgi apparatus, where resident chaperones can help re-fold and stabilize flexible, disulfide-bonded, partially disordered, or multidomain proteins. Proteins targeted for secretion are also subject to glycosylation, which is often critical for protein stability and activity. Furthermore, purification of secreted proteins bypasses cell lysis, simplifying the procedure and protecting fragile proteins from highly active intracellular proteases. As a result, secreting recombinant proteins can increase or even rescue target expression relative to standard cytosolic strategies. At the CMD, we found this to be particularly true for complex human proteins, particularly receptor ectodomains and extracellular matrix components, which we struggled or failed to express in the cytosol.
Motivated to implement a robust and orthogonal protein secretion platform for our challenging targets, we initiated a collaboration with the Danish company ExpreS2ion Biotechnologies. Founded in 2010, ExpreS2ion Biotechnologies markets an S2-based protein secretion system called ExpreS2, which has been used to express over 300 recombinant proteins to date. The ExpreS2 platform relies on the “pExpreS2” class of vectors which place genes of interest downstream of a strong, constitutive, and fused Actin-Hsp70 core promoter and upstream of an opie2 polyadenylation sequence. The pExpreS2 vector backbones include either a ZeocinR (pExpreS2-1) or G418R (pExpreS2-2) marker, allowing stably expressing polyclonal cell lines to be generated without co-transfection of a selection plasmid. The ExpreS2 platform also includes a flexible and insect cell–optimized proprietary transfection reagent, although other commercial transfection reagents also suffice. Recent successes with the ExpreS2 platform include the expression and structural characterization of human endothelial protein C receptor (EPCR), production of cGMP compliant P. falciparum RH5 protein vaccines, and production and structural elucidation of the three-component P. falciparum invasion complex (Lau et al., 2015; Hjerrild et al., 2016; and Wong et al., 2019). Due to the simplicity of the one-plasmid system and the reported successes from other structural laboratories, we selected the ExpreS2 system as the foundation for our secreted protein pipeline.
Nevertheless, we needed to make a variety of improvements to the commercially available ExpreS2 system to facilitate a moderate-throughput pipeline for structural work. Our changes, presented and utilized herein, include the following: 1) modification of the pExpreS2 vectors to encode an N-terminal BiP secretion signal and C-terminal cleavable affinity epitopes including 10His, Twin-Strep, and Protein A; 2) standardization of the pExpreS2 vectors for high-throughput cloning; 3) quickening and miniaturizing the existing transient transfection protocol to allow parallelized expression screening; and 4) piloting the method with a variety of challenging human proteins. The resulting methodology, outlined in Figure 1, facilitates a transient test expression of up to 46 constructs in just 2 weeks without requiring bacmid or virus production, followed by stable cell line generation and large-scale purification of expressing constructs over five additional weeks. Expression testing in our pipeline is as rapid as in E. coli systems, and the final stable cell lines are cheaper, easier, and more reliable to scale-up for production than baculovirus-infected insect cells. The protocol presented herein, which we dubbed “FAS2FURIOUS” or “F2F,” should allow other structural laboratories to quickly deploy the gold-standard S2 secretion system to enable the characterization of previously intractable targets.
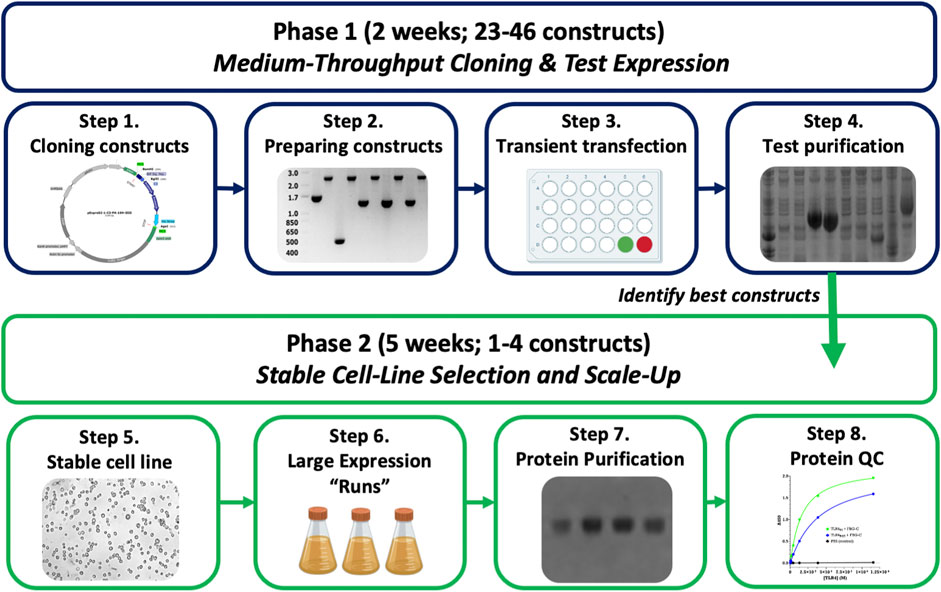
FIGURE 1. Overview of the FAS2FURIOUS method. Step 1: constructs are designed and cloned into pExpreS2-1 class vectors using a HiFi assembly in 96-well plates. Step 2: insert-containing clones are verified by colony PCR and mini-prepped on spin-columns. Step 3: constructs are transiently transfected in parallel into S2 cells in 24-well plates. Step 4: supernatants are harvested, test-purified using 96-well filter plates, and checked for expression on SDS-PAGE. Step 5: polyclonal stable cell lines are selected using Zeocin for constructs of interest. Step 6: polyclonal cell lines are scaled up in 1L shake flasks for large-scale purification.
2 Materials and Methods
2.1 Protocol and Reagent Availability
A detailed step-by-step protocol for the entirety of the FAS2FURIOUS method is available in Supplementary Material. All vectors reported and used herein are available from Addgene (Plasmids #175360, #175362, #175363, #175443, #175444, #175445, #175446, and #175447).
2.2 Molecular Biology
2.2.1 Starting Vectors
pExpreS2-1, pExpreS2-2, and pExpreS2-1-GFP were purchased from Expres2ion Biotechnologies. Drosophila codon–optimized TLR4, without a tag, and MD-2, cloned with a C-terminal thrombin-cleavable Protein A tag, were generously provided by Toshiyuki Shimizu from the University of Tokyo. Professor Shimizu’s TLR4/MD-2 constructs were provided in the pMT-BiP-V5-His (Invitrogen) backbone and cloned in-frame with the vector’s BiP signal peptide.
2.2.2 Creation of F2F Vectors
To create the pExpreS2-1-CR-PA, the cassette encoding BiP-MD-2-Thrombin-Protein A was excised from pMT-BiP-V5-His via BamHI/AgeI restriction sites and transferred into the MCS of pExpreS2-1 linearized with the same restriction enzymes. This maintained the BglII cut site in between the BiP signal peptide and MD-2 open-reading frame which was present in the Shimizu entry constructs. The MD-2 insert, along with accessory restriction cloning scars from the original cassette, was removed via PCR and the Q5 Site-Directed Mutagenesis Kit (NEB), yielding the final pExpreS2-1-CR-PA. To create the pExpreS2-1-CR-PA-10H-SIII, the pExpreS2-1-MD-2-Protein-A intermediate vector was used as a template to add a 10His-SIII tag via one cycle of PCR mutagenesis, and then the MD-2 insert was removed with a second cycle of PCR mutagenesis as before to yield pExpreS2-1-CR-PA-10H-SIII. pExpreS2-1-C3-PA and pExpreS2-1-C3-PA-10H-SIII were created similarly to their thrombin-cleavable counterparts, except the thrombin cut site which was also simultaneously mutated to a C3 cut site. Finally, to generate pExpreS2-C3-10H-SIII, both the MD-2 insert and the Protein A tag were removed from the pExpreS2-1-MD-2-Protein-A-10H-SIII intermediate vector via PCR mutagenesis. All F2F vectors were validated by Sanger sequencing.
2.2.3 Cloning Into F2F Vectors
For sub-cloning, F2F vectors were first linearized via BglII digestion, which cuts all F2F vectors in between the 5′ BiP signal peptide and the 3′ tags. The inserts were amplified via PCR, with primers containing the following HiFi Assembly overhangs:
Linearized F2F vectors and amplicons were assembled using the NEBuiler® HiFi DNA Assembly Mastermix according to the manufacturer’s recommendations, resulting in the seamless fusion of the insert with the 5′ BiP and 3′ tags. The constructs were validated using the Bioline MyTaq™ Red Colony PCR Kit with the following sequencing primers:
Validated construct DNA was purified on a spin-column and heat-sterilized for 15 min at 95°C in preparation for transfection.
2.3 Cell Culture
2.3.1 General S2 Handling
ExpreS2 cells (referred to as “S2” herein) were purchased from ExpreS2ion Biotechnologies. The S2 cells were regularly maintained in EX-CELL® 420 (Sigma Aldrich) + 1X penicillin/streptomycin. The S2 cells were routinely split every 3–4 days to a density of 8 × 106 cells/ml in round-bottomed flasks and grown at 25°C and 110 RPM.
2.3.2 Transient Test Expression
The S2 cells were split to 8 × 106 cells/ml by centrifugation (400 x g for 3 min), and 3 ml of the cells was seeded into deep-well 24-well blocks. The cells were transfected with 37.5 µl of ExpreS2 Insect-TRx5 (TRx5; ExpreS2ion Biotechnologies) and 7.5 µg of an insert-containing F2F construct. The transfected cells were allowed to grow at 500 RPM for 68–72 h at 25°C. The supernatants were harvested via centrifugation (1,500 x g for 20 min) and purified using 100 µl bed volume of the Ni-NTA resin and a 96-well deep-well filter block (ThermoFisher). The proteins were eluted in 100 µl of 1X PBS +500 mM imidazole and analyzed by SDS-PAGE. Putative target bands were confirmed using the in-gel tryptic digest LC-MS/MS.
2.3.3 Stable Cell Line Selection
To select stable cell lines of well-expressing constructs, the S2 cells were split to 2 × 106 cells/ml by centrifugation (400 x g for 3 min) and 5 ml of the cells was seeded into T25 flasks. The cells were transfected with 50 µl of TRx5 and 12.5 µg of construct DNA and rested at 25°C for 4 h before the addition of 1 ml FBS. For a positive control, one flask was transfected with pExpreS2-1-GFP, which can be used to track transfection efficiency (often >50%). For a negative control, one flask was transfected with TE. After 24 h, a selection reagent was added. For pExpreS2-1 class vectors, 2 mg/ml Zeocin was used. For pExpreS2-2 class vectors, 4 mg/ml G418 was used. For co-selection, 1.5 mg/ml Zeocin +4 mg/ml G418 was used. The cells were selected for 24 total days, during which every 3–4 days, the cells were split back to 1 × 106 cells/ml in EX-CELL® 420 + 10% FBS + selection reagent. After 24 days, all cells in the positive control were GFP-positive and all cells in the negative control were dead. At the end of the selection, the cells were transferred in 15 ml to a T75 flask with 10% FBS (without a selection marker) and then scaled up again to 25 ml in a 125-ml shake flask without FBS. Stable cell lines were then scaled-up, passaged, and frozen down in 10% DMSO as recommended by ExpreS2ion.
2.4 Purification of Recombinant Proteins
2.4.1 Commercially Available Proteins
The recombinant human CD44 ectodomain derived from mammalian cells was purchased from Abcam (ab173996). Recombinant human TLR4 ectodomain, derived from mammalian cells, was purchased from R&D systems.
2.4.2 TLR4 and MD-2 From S2 Cells
Drosophila codon–optimized human TLR4 ectodomain (UniProtKB O00206, P23-M629) and MD-2 (UniProtKB Q9Y6Y9, Q19-N160) received from Toshiyuki Shimizu (University of Tokyo) were cloned into pExpreS2-1-CRPA and stably expressed in S2 cells. Every 3–4 days, 0.5–1 L of the supernatant from stable cell lines was harvested and purified on a 5 ml column of IgG Sepharose 6 Fast Flow Affinity Resin (GE). The protein was eluted in 0.5M acetic acid pH = 3.4 and then immediately neutralized by desalting into 1X PBS. TLR4 was incubated with 10 U/mg human recombinant thrombin protease (EMD Millipore) for 4 h at room temperature, while MD-2 was routinely left tagged for stability. TLR4 was further purified via size-exclusion chromatography on a Superdex 200 16/60 column into 1X PBS, while MD-2 was polished into 25 mM Bicine pH = 8.5 + 250 mM NaCl. The proteins were concentrated to ∼5 mg/ml and frozen at −80°C for downstream applications. Protein identity was validated by intact mass spectrometry for MD-2 and tryptic digest LC-MS/MS for TLR4.
2.4.3 FBG-C From E. coli
The C-terminal fibrinogen-like globe domain of human tenascin C (FBG-C; UniProtKB P24821 Isoform 1, I1974-A2201) was cloned using ligation-independent cloning into pNIC-NHStIIT, which places FBG-C downstream of a TEV-cleavable tandem 6His + Twin-Strep-tag®. FBG-C was expressed in BL21 (DE3) Tuner + CyDisCo pMJS226 E. coli cell lines. pMJS226, the plasmid encoding the CyDisCo components, was supplied from Prof. Lloyd Ruddock and allows the soluble expression of disulfide-containing proteins such as FBG-C (Gaciarz et al., 2017). The protein was expressed overnight at 18°C in “TB-PLUS” media (1X Terrific Broth +15 ml/L glycerol +0.01% Antifoam +1 mM MgSO4 + 10 mM (NH4)2SO4 + 0.5% (w/v) glucose + 1X E. coli trace metals). The cells were broken by sonication, and FBG-C was purified using StrepTactinXT™ affinity resin and eluted with 50 mM HEPES pH = 7.5, 250 mM NaCl, 5% glycerol, and 50 mM D-biotin. FBG-C was cleaved with TEV for 4 h at room temperature before a final purification step was performed on a Superdex 75 16/60 column into 20 mM HEPES pH = 7.5, 250 mM NaCl, 2% glycerol. FBG-C was concentrated to ∼20 mg/ml and flash-frozen in liquid nitrogen before storage at −80°C for downstream applications. The presence of two disulfide bonds and identity of the protein was confirmed by intact mass spectrometry.
2.4.4 CD44-v10 From S2 Cells
The ectodomain of the human CD44 v10 isoform (UniProtKB P16070 Isoform 11, A20-P335) was cloned into pExpreS2-C3-10H-SIII alongside a non-cleavable, C-terminal 6His tag and a stop codon before the tags supplied in the vector. A polyclonal stable cell line was generated as before and was grown for 4 days before harvesting 50 ml of the supernatant. Imidazole was added to the supernatant (10 mM) prior to the addition of 0.5 ml of equilibrated Ni–NTA resin. Binding to the resin occurred on a rotating wheel for 1 h at 8°C, before batch-washing beads twice with 100 ml of 50 mM HEPES pH = 7.5, 500 NaCl, and 10 mM imidazole. The resin was loaded onto a poly-prep column and further washed with 5 ml 50 mM HEPES pH = 7.5, 500 NaCl and 25 mM imidazole. CD44-v10 was eluted with 2 ml of 50 mM HEPES pH = 7.5, 500 NaCl, and 250 mM imidazole. CD44-v10 was further purified on a Superdex 200 16/60 column into 20 mM HEPES pH = 7.5 and 200 mM NaCl before being concentrated to 1.5 mg/ml using a centrifugal filtration unit and stored at 80°C. The protein identity was validated by tryptic digest LC-MS/MS.
2.4.5 CD44-Link From E. coli
The ectodomain of human CD44 containing only the Link domain (UniProtKB P16070 Isoform 1, A20-N172) was cloned into pNIC28-Bsa4, which places CD44-Link downstream of a TEV-cleavable 6His tag. CD44-Link was expressed as inclusion bodies in 1 L of Terrific Broth from BL21 (DE3) Rosetta cells. After harvesting the cells, the inclusion bodies were re-suspended into 20 ml of 20 mM Tris pH = 8.0. Washing of the inclusion bodies (up to four times) was performed by sonication (10 min), centrifugation (9,000 x g, 20 min, 4°C), and re-suspension into 30 ml of 50 mM Tris pH = 8.0, 100 mM NaCl, and 0.5% Triton X-100. A final wash in 50 mM Tris pH = 8.0 and 100 mM NaCl was performed to remove Triton X-100. The protein was solubilized in 10 ml of 8 M urea, 50 mM MES pH = 6.5, 0.1 mM EDTA, and 0.1 mM DTT at 4°C overnight on a rotating wheel. The protein was re-folded over 2 days at 4°C by rapid dilution into 250 mM L-arginine, 100 mM Tris–HCl pH = 8.5, 2.5 mM reduced glutathione, 2.5 mM oxidized glutathione, and 1:200 dilution of Pierce Complete EDTA-free protease inhibitors to give a final protein concentration of 50 µg/ml (typically using 1–2 L of buffer). The re-folded protein was passed through a 0.2-µm filter before being concentrated using a Vivaflow 200 (Sartorius) tangential flow filtration unit (to 50 ml), followed by a centrifugal filtration unit. Further purification and removal of aggregates were performed on a Superdex 75 16/60 column into 20 mM Tris–HCl pH = 8.0 and 150 mM NaCl. Peak fractions were concentrated to 7 mg/ml prior to storing at −80°C. The protein identity was validated by using intact mass spectrometry, revealing the correct formation of the three disulfide bonds within the CD44-Link.
2.5 In Vitro Assays
2.5.1 Analytical Size Exclusion Chromatography
The TLR4:MD-2 complex was analyzed using a Superdex 200 Increase 30/100 on a Dionex Ultimate 3000 UHPLC. A280 traces reported from 100-µl injections containing 200 µg of TLR4, 400 µg of MD-2, or 200 µg of TLR4 + 400 µg MD-2.
2.5.2 TLR4-Binding ELISA
The TLR4-binding ELISA was implemented essentially as previously reported (Zuliani-Alvarez et al., 2017). Briefly, 1 µg/ml of FBG-C was coated overnight onto microlon medium binding plates (Greiner Bio One 655080) before blocking for 2 h with 10% BSA in PBST. A concentration series of TLR4, dissolved in PBST +2% BSA, was prepared for both in-house–purified Drosophila-derived TLR4 and commercially available TLR4 from mammalian cells (R&D). After incubating for 2 h at 37°C, the plate was probed for TLR4 binding using a primary antibody (Invivogen, mAbg-hTLR4) in PBST +2% BSA for 2 h at RT. After thorough washing, the anti-rabbit HRP-coupled secondary antibody (BioRad STAR13B) was used for 1 h at room temperature before colorimetric readout using TMB. OD450 was measured using a FluoStar Omega plate reader.
2.5.3 CD44-Binding ELISA
Detection of hyaluronic acid (HA) binding to CD44 was performed using a modified ELISA assay, essentially as previously described (Banerji et al., 1998). Briefly, the CD44 protein was diluted into 15 mM sodium carbonate buffer (pH 9.3), and 100 μl was added to a well of a 96-well Nunc-MaxiSorp flat-bottomed plate. Adsorption of the protein to the well was performed for 6 h at room temperature. Further adsorption was blocked by the incubation of the wells with 1% BSA in PBS and 0.05% Tween-20 at 4°C overnight. The wells were washed with PBS containing 0.05% Tween-20 prior to incubation with 100 μl of 10-kDa HA (Creative PEGWorks; HA-6011) diluted to 5 μg/ml for 1 h at room temperature. After washing, the wells were incubated for 30 min with 100 μl of streptavidin-conjugated HRP at 100 ng/ml. After a further wash, 100 μl o-phenylenediamine substrate (0.4 mg/ml in 0.4 mg/ml urea hydrogen peroxide, 0.05 M phosphate-citrate pH = 5.0) was added to the wells for 20 min. HRP activity was terminated by the addition of 50 μl 2.5 M sulfuric acid prior to the measuring activity at 492 nm.
3 Results
3.1 Modification of the pExpreS2-1 Vector for Structural Biology
The original pExpreS2-1 backbone (Figure 2A) contains only a restriction endonuclease–based (RE) multiple cloning site (MCS), which is unsuitable for high-throughput cloning due to the off-target cleavage of DNA templates (Festa et al., 2013). Furthermore, because of decreased secretion efficiencies observed for constructs with “scars” between the signal peptide and gene of interest (data not shown), we preferred a seamless cloning strategy. Therefore, we made our F2F vectors compatible with the NEBuilder® HiFi DNA Assembly (HiFi), which builds upon standard Gibson Assembly methodologies and allows a seamless, one-step, and high-efficiency assembly of DNA fragments (Gibson et al., 2009). HiFi assemblies are also compatible with un-purified PCR reaction mixtures, allowing us to bypass tedious PCR clean-up steps and assemble the constructs in only 1 day. The main drawback of HiFi assemblies is the cost, which we partially mitigate by halving the recommended reaction volume without compromising assembly efficiency (Figure 3A).
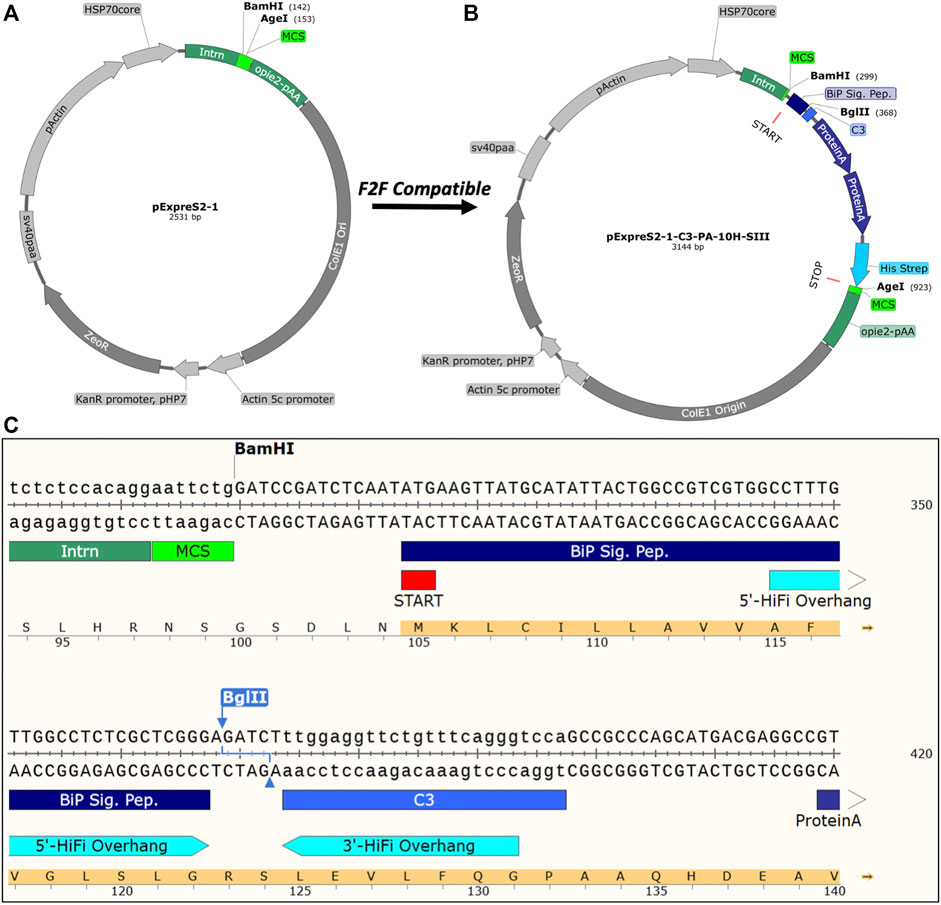
FIGURE 2. Vectors and construct design. (A) pExpreS2-1 vector backbone included in the ExpreS2ion’s commercially available kit. (B) Exemplar F2F vector, pExpreS2-1-C3-PA-10H-SIII, modified for high-throughput structural applications. (C) Sequence-level details of pExpreS2-1-C3-PA-10H-SIII showing the BglII linearization sequence, recommended HiFi overhangs, N-terminal secretion peptide, and C-terminal affinity tags. An overview of all F2F vectors created for this study can be found in Table 1.
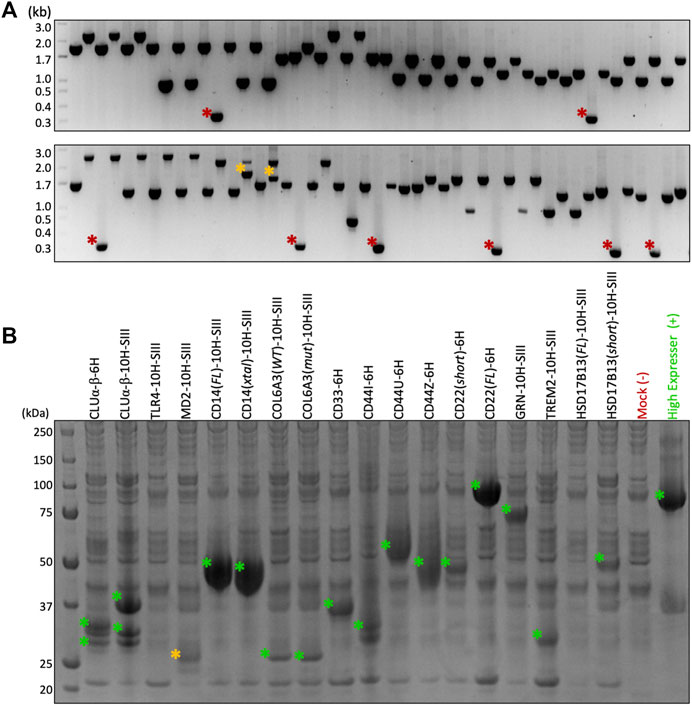
FIGURE 3. Cloning and test expression results. (A) Colony PCR after selecting 94 colonies from 18 different constructs demonstrates >90% assembly efficiency using HiFi. Red asterisk = vector alone; orange asterisk = unidentified products. (B) SDS-PAGE after 3-ml test expression and Ni-NTA pull-down. Eighteen constructs and two controls tested in parallel. Green asterisk = target band confirmed by in-gel tryptic digest MSMS; orange asterisk = putative target band.
After selecting the overall cloning strategy, we then functionalized the pExpreS2-1 backbone with the requisite purification epitopes. All of our F2F vectors rely on the Drosophila immunoglobulin heavy chain–binding protein (BiP) signal sequence, which is commonly used for efficient protein secretion in Drosophila (Munro and Pelham, 1986; Sonoda et al., 2012; and Ohmuro-Matsuyama and Yamaji, 2018). At the C-terminus, we added a selection of cleavable tandem-affinity purification (TAP) tags, which enhance purification flexibility, improve final protein purity, and diversify downstream biochemical applications (Li, 2011). Alongside a standard 10His tag, which itself is sufficient for small-scale expression testing using immobilized metal-affinity chromatography (IMAC; Figure 4B), we also included two high-affinity tags designed for pull downs from dilute cell culture media samples: Protein A and Twin-Strep-tag®. Because of its relatively large size, the Protein A tag, derived from Staphylococcal protein A, can increase solubility and proteolytic stability of fusion partners; furthermore, with a Kd of just 10 nM for IgG, even dilute cell culture supernatants can be affinity-purified on IgG Sepharose (Kimple et al., 2013). The Twin-Strep-tag®, which relies on an engineered streptavidin-binding peptide, is specifically designed for the purification of recombinant proteins from dilute supernatants using the Strep-Tactin® affinity resin (Schmidt et al., 2013). Elution from the Strep-Tactin® affinity resin relies on excess free biotin and, therefore, is much gentler than elution from IgG Sepharose, which requires low pH. Because of these benefits, the TAP “His-Twin-Strep” tag is the workhorse affinity epitope at the CMD, and it routinely performs well across soluble, secreted, and membrane proteins.
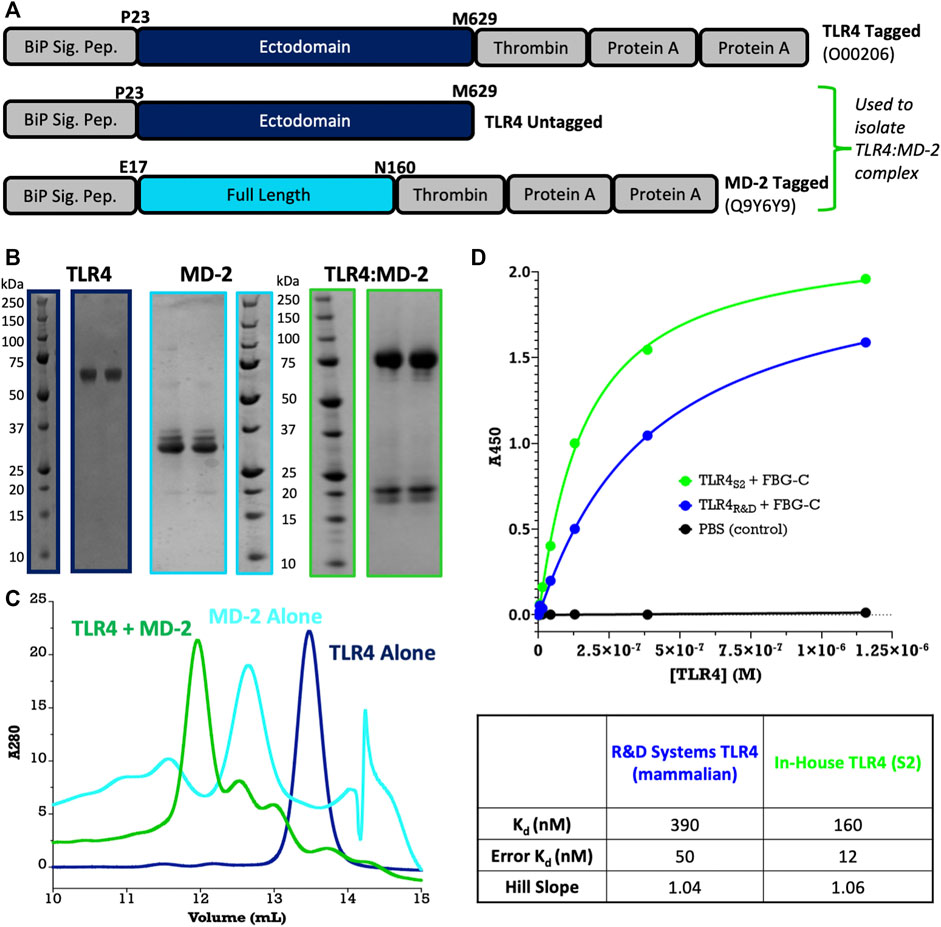
FIGURE 4. Large-scale purification of TLR4 and MD-2. (A) Construct design using pExpreS2-1-CRPA and pExpreS2-2. (B) Final purity for TLR4, MD-2, and TLR4:MD-2 complex after IgG pull-down and size-exclusion chromatography (SEC). In the TLR4 and TLR4:MD-2 samples, the Protein A tag has been removed, while in the MD-2 sample, the tag is retained for stability. All SDS-PAGE bands were confirmed by in-gel tryptic digest MSMS. (C) Analytical SEC binding assay shows that individually purified TLR4 and MD-2 associate into a complex as expected. It is to be noted that MD-2 exists as a mixed homo-oligomer by itself in solution and, therefore, elutes before the larger TLR4 monomer. (D) Activity assay confirms that TLR4:FBG-C binding is similar between S2-derived and commercially available mammalian cell-derived TLR4 from R&D Systems. Data are presented from a single biological replicate and were fit via nonlinear regression to a one-to-one saturation binding model using GraphPad Prism.
In between the regions coding for the N-terminal BiP signal peptide and the C-terminal affinity tag(s), we placed a BglII cut site that is used to linearize the vector during cloning and is removed during the HiFi assembly step when our recommended overhangs are used. In addition, we included either a thrombin or human rhinovirus 3C (HRV 3C) protease site, which is used to cleave the purification tags from the final recombinant protein. HRV 3C protease, also marketed as PreScission™ protease, outperforms the more commonly used tobacco etch virus (TEV) protease across a variety of conditions, including in high-imidazole IMAC elution buffers and at 4°C (Ullah et al., 2016). Thrombin protease has a higher specific activity than HRV C3 protease; however, it sometimes suffers from off-target cleavage activity and is not available in a tagged form for easy removal (Waugh, 2011). Figure 2B shows a representative F2F vector, pExpreS2-1-C3-PA-10H-SIII, and Figure 2C details the architecture of the HiFi cloning region. Table 1 provides an overview of all the vectors used herein.
3.2 Parallelization of Transient Test Expressions
Using the new F2F vector backbones, initial experiments achieved excellent expression of a handful of difficult targets using the recommended commercial methodology for transient transfections. However, in order to fully deploy ExpreS2 as a pipeline secretion system for moderate-throughput structural applications, we needed to miniaturize and parallelize the reported protocols. ExpreS2ion Biotechnologies recommends either 1) an 8 ml transient transfection in 50-ml conical tubes using 20 µg of DNA at >0.6 µg/µl or 2) a 10 ml transient transfection in 100-ml flat-bottomed flasks using 25 µg of DNA at >0.6 µg/µl. These protocols require midi- or maxi-prepping constructs, and parallelizing transfections in falcon tubes or flasks is cumbersome. Using the established methodology, we could reasonably test just 3–4 constructs in a week, preventing us from deploying the system at the requisite scale.
Therefore, we optimized and parallelized the transfection methodology by implementing three key improvements. First, we reduced the cell volume to just 3 ml per construct, allowing us to perform transient test expressions in 24-well deep-well blocks. Second, we reduced the amount and concentration of DNA required to 7.5 µg at >200 ng/µl, which we routinely achieved with a standard miniprep of the F2F vectors. Finally, we implemented a 96-well filter-plate–based test purification protocol, which supports all HT work at the CMD and allows rapid analysis of the expression levels. Coupled with the HT-cloning methodology outlined previously, the F2F method allows either 22 (one mini-prep cycle and one test expression plate) or 46 (two mini-prep cycles and two test expression plates) constructs to be tested in parallel with the appropriate controls. The cloning and test expression stage of F2F takes only 2 weeks and does not require bacmid preparation or virus amplification; therefore, F2F is most similar to E. coli expression protocols and is by far the fastest eukaryotic pipeline deployed at the CMD.
3.3 Pilot of the Entire F2F Method
To validate the F2F method and demonstrate its robustness, we cloned and test-expressed 18 constructs containing 11 challenging gene targets, many of which expressed poorly using other systems. These “pilot” genes included receptor ectodomains such as toll-like receptor 4 (TLR4), monocyte differentiation antigen CD14 (CD14), B-cell receptor CD22 (CD22), myeloid surface antigen CD33 (CD33), CD44 antigen (CD44), and triggering receptor expressed on myeloid cells 2 (TREM2); naturally secreted proteins such as lymphocyte antigen 96 (MD-2), collagen alpha-3(VI) (COL6A3), clusterin (CLU), and progranulin (GRN); and the cytosolic protein 17-beta-hydroxysteroid dehydrogenase 13 (HSD17B13).
Figure 3A demonstrates the greater than 90% HiFi Assembly efficiency we typically observe when cloning into the F2F vectors, which means just two colonies need to be checked to ensure finding a positive clone. Figure 3B shows the results of our small-scale transient test expression, with MSMS confirming the expression of 15/18 constructs and 9/11 genes. It is to be noted that the expressions of TLR4 (lane 3) and MD-2 (lane 4) can be rescued by switching the His-Twin-Strep tag for a Protein A tag (see Figure 4); therefore, we eventually expressed all 11 of our pilot targets using the F2F methodology.
3.4 Representative Stable Cell Lines Yield Homogenous, Active Protein
Following a positive result in the transient test transfection stage, it is necessary to select polyclonal stable cell lines which can be used for large-scale purification. At this stage, constructs can also be co-expressed with a binding partner cloned into pExpreS2-2, which is G418R (see Table 1). The F2F method includes no optimization of the protocol for selecting stable cell lines as ExpreS2ion’s recommended method is sufficient for our applications and works routinely in our hands. The selection of stable cell lines requires 5 weeks, with the actual hands-on time less than 3 h per week. The resulting polyclonal cell lines can be frozen in DMSO for long-term storage without a pronounced decrease in target expression. Stable cell lines can be harvested and passaged twice a week for up to 6 weeks before the expression levels decrease, facilitating relatively simple and high yielding purification “runs.” Given that the expression levels in ExpreS2 are often >10 mg/L and cell supernatants can be harvested twice a week, it is reasonable to expect >50 mg of the purified protein after a 4-week purification run for well-expressing targets.
To demonstrate the utility of purifying in bulk from stable cell lines, we scaled up two of our pilot targets, TLR4 and MD-2. MD-2 is the obligate co-receptor for the innate immune receptor TLR4, and the two proteins are known to assemble into a high-affinity two-way complex at the cell surface, which is responsible for the detection of endotoxin (LPS) (Park et al., 2009). Despite testing >100 constructs, we could not express TLR4 or MD-2 in E. coli, Sf9, High-Five™, or Expi293(TM) cells. However, using F2F we could express TLR4 and MD-2, both alone and in complex, using a thrombin-cleavable Protein A tag (Figure 4A). The purified proteins are homogenous and monodisperse after size-exclusion chromatography (Figure 4B) and separately purified TLR4 and MD-2 assemble into a complex as expected (Figure 4C). Furthermore, in a solid-phase ELISA that measures TLR4 binding to a known ligand, the fibrinogen-like globe domain of tenascin C (FBG-C) our S2-derived TLR4 is comparable, if not superior, to commercially available mammalian-derived TLR4 from R&D Systems (Figure 4D).
Furthermore, we also scaled up the ectodomain of CD44, which expresses insolubly and with low refolded yields in E. coli. Using a non-cleavable 6His tag (Figure 5A), which is standard for this target, we obtained monodisperse proteins at good yields (10 mg/L) following an affinity pull-down on Ni-NTA resin and a gel-filtration clean-up step (Figure 5B). We also showed that S2-derived CD44 binds its ligand, hyaluronic acid (HA), with higher affinity than either E. coli–derived re-folded protein or commercially available mammalian-derived protein from Abcam (Figure 5C). Therefore, even for previously intractable targets such as TLR4 and MD-2 and difficult refolded targets such as CD44, stably expressing ExpreS2 cell lines yield homogenous, active protein in excellent yields (final purified yield greater than 5 mg/L in all cases).
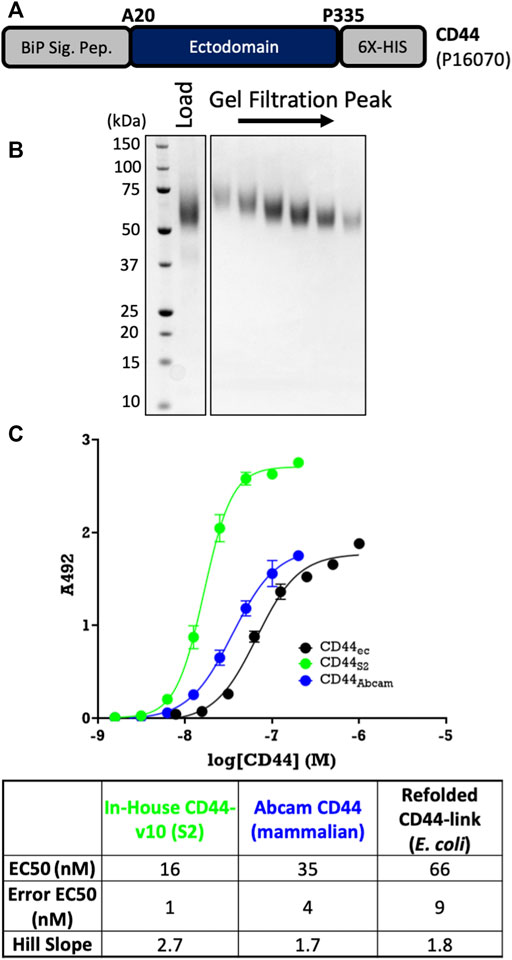
FIGURE 5. Large-scale purification of CD44. (A) Construct design using pExpreS2-1-C3-10H-SIII. (B) CD44 is pure and monodisperses after SEC. (C) Binding assay confirms that S2-derived CD44 binds to its ligand hyaluronic acid (HA) with similar affinity as E. coli– or mammalian-derived CD44. Data are presented from a single biological replicate and were fit via four component (variable slope) nonlinear regression using GraphPad Prism.
4 Discussion
Protein secretion represents a powerful method for expressing challenging targets, particularly disulfide-bonded, posttranslationally modified, or partially disordered proteins as well as receptor ectodomains and naturally secreted proteins. S2 cells, derived from Drosophila macrophages, are uniquely tuned protein secretors, and in our hands express ∼90% of “secreted or secretable” targets. Figure 6 provides a sampling of more than 300 proteins that have now been expressed in the ExpreS2 system, many of which were intractable in orthogonal eukaryotic expression systems.
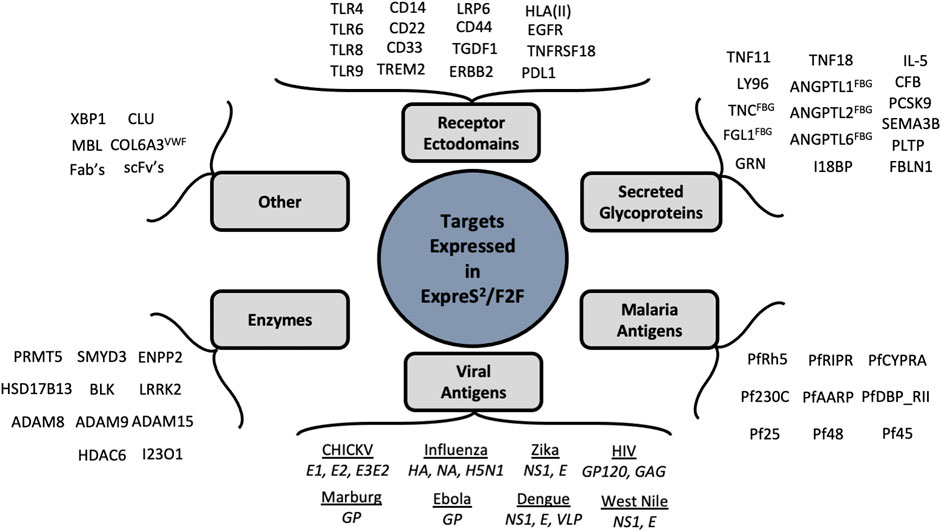
FIGURE 6. Diverse targets expressed in S2 Cells. Representative examples of proteins expressed with the ExpreS2/F2F system both in-house and at ExpreS2ion Biotechnologies.
The FAS2FURIOUS method represents a step forward versus previously reported S2 systems, with rapid and parallelizable expression testing now feasible for up to 46 constructs in just 2 weeks. We include in Supplementary Material a detailed step-by-step protocol which outlines the entirety of the F2F protocol in granular detail, and all F2F vectors are freely available from Addgene. F2F lays the foundation for the broad implementation of secreted expression pipelines, especially in structural and biophysical laboratories, and should greatly expand the targets amenable to characterization. Given the benefits of F2F in terms of simplicity, yield, speed, and versatility, we expect protein expression in S2 to “catch-up” to more common approaches in Sf9 and High Five™ cells, especially for the most challenging targets.
Data Availability Statement
The datasets presented in this study can be found in online repositories. The names of the repository/repositories and accession number(s) can be found in the article.
Author Contributions
JC designed, implemented, and optimized the F2F method; created 4/5 F2F vectors; purified and characterized TLR4/MD-2; and wrote the manuscript. MF created the other F2F vector. VK purified and characterized CD44. AS developed the TLR4 ELISA protocol and assisted JC with assay optimization. SC, BF, and WdJ provided support, guidance, and standard operating procedures for the ExpreS2 system and supplying target expression data shared in Figure 6. OG, NB-B, BM, KM, and WY provided supervision and suggestions, and BM, KM, and WY assisted with manuscript preparation.
Funding
The Centre for Medicines Discovery, previously the Structural Genomics Consortium, is a registered charity (Number 1097737) that receives funds from AbbVie, Bayer Pharma AG, Boehringer Ingelheim, Canada Foundation for Innovation, Eshelman Institute for Innovation, Genome Canada, Innovative Medicines Initiative (EU/EFPIA) (ULTRA-DD grant no. 115766), Janssen, Merck & Co., Novartis Pharma AG, Ontario Ministry of Economic Development and Innovation, Pfizer, São Paulo Research Foundation-FAPESP, Takeda, and Wellcome Trust (092809/Z/10/Z). BM was supported by the Kennedy Trust for Rheumatology Research (KTRR). JC is grateful for funding received from the Nuffield Department of Medicine’s Prize DPhil Studentship.
Conflict of Interest
SC, BF, and WdJ were employed by ExpreS2ion Biotechnologies, which markets the ExpreS2ion system that was used as a starting point to create FAS2FURIOUS.
The remaining authors declare that the research was conducted in the absence of any commercial or financial relationships that could be construed as a potential conflict of interest.
Publisher’s Note
All claims expressed in this article are solely those of the authors and do not necessarily represent those of their affiliated organizations or those of the publisher, the editors, and the reviewers. Any product that may be evaluated in this article, or claim that may be made by its manufacturer, is not guaranteed or endorsed by the publisher.
Acknowledgments
The authors would like to especially thank Toshiyuki Shimizu from the University of Tokyo for generously providing the initial TLR4 and MD-2 constructs.
Supplementary Material
The Supplementary Material for this article can be found online at: https://www.frontiersin.org/articles/10.3389/fbioe.2022.871933/full#supplementary-material
References
Adriaan de Jongh, W., Salgueiro, S., and Dyring, C. (2013). The Use ofDrosophilaS2 Cells in R&D and Bioprocessing. Pharm. Bioprocess. 1, 197–213. doi:10.4155/pbp.13.18
Banerji, S., Day, A. J., Kahmann, J. D., and Jackson, D. G. (1998). Characterization of a Functional Hyaluronan-Binding Domain from the Human CD44 Molecule Expressed inEscherichia Coli. Protein Expr. Purif. 14, 371–381. doi:10.1006/prep.1998.0971
Carbonell, L. F., Klowden, M. J., and Miller, L. K. (1985). Baculovirus-mediated Expression of Bacterial Genes in Dipteran and Mammalian Cells. J. Virol. 56, 153–160. doi:10.1128/jvi.56.1.153-160.1985
Festa, F., Steel, J., Bian, X., and Labaer, J. (2013). High-throughput Cloning and Expression Library Creation for Functional Proteomics. Proteomics 13, 1381–1399. doi:10.1002/pmic.201200456
Fitzgerald, D. J., Berger, P., Schaffitzel, C., Yamada, K., Richmond, T. J., and Berger, I. (2006). Protein Complex Expression by Using Multigene Baculoviral Vectors. Nat. Methods 3, 1021–1032. doi:10.1038/nmeth983
Gaciarz, A., Khatri, N. K., Velez-Suberbie, M. L., Saaranen, M. J., Uchida, Y., Keshavarz-Moore, E., et al. (2017). Efficient Soluble Expression of Disulfide Bonded Proteins in the Cytoplasm of Escherichia coli in Fed-Batch Fermentations on Chemically Defined Minimal media. Microb. Cel Fact. 16, 1–12. doi:10.1186/s12934-017-0721-x
Gibson, D. G., Young, L., Chuang, R.-Y., Venter, J. C., Hutchison, C. A., and Smith, H. O. (2009). Enzymatic Assembly of DNA Molecules up to Several Hundred Kilobases. Nat. Methods 6, 343–345. doi:10.1038/nmeth.1318
Hitchman, R. B., Possee, R. D., Crombie, A. T., Chambers, A., Ho, K., Siaterli, E., et al. (2010). Genetic Modification of a Baculovirus Vector for Increased Expression in Insect Cells. Cell Biol. Toxicol. 26, 57–68. doi:10.1007/s10565-009-9133-y
Hjerrild, K. A., Jin, J., Wright, K. E., Brown, R. E., Marshall, J. M., Labbé, G. M., et al. (2016). Production of Full-Length Soluble Plasmodium Falciparum RH5 Protein Vaccine Using a Drosophila melanogaster Schneider 2 Stable Cell Line System. Sci. Rep. 6, 1–15. doi:10.1038/srep30357
Kimple, M. E., Brill, A. L., and Pasker, R. L. (2013). Overview of Affinity Tags for Protein Purification. Curr. Protoc. Protein Sci. 73, 9.9.1–9.9.23. doi:10.1002/0471140864.ps0909s73
Lau, C. K. Y., Turner, L., Jespersen, J. S., Lowe, E. D., Petersen, B., Wang, C. W., et al. (2015). Structural Conservation Despite Huge Sequence Diversity Allows EPCR Binding by the Pfemp1 Family Implicated in Severe Childhood Malaria. Cell Host Microbe 17, 118–129. doi:10.1016/j.chom.2014.11.007
Lee, D.-F., Chen, C.-C., Hsu, T.-A., and Juang, J.-L. (2000). A Baculovirus Superinfection System: Efficient Vehicle for Gene Transfer into Drosophila S2 Cells. J. Virol. 74, 11873–11880. doi:10.1128/jvi.74.24.11873-11880.2000
Lemaitre, R. P., Bogdanova, A., Borgonovo, B., Woodruff, J. B., and Drechsel, D. N. (2019). FlexiBAC: A Versatile, Open-Source Baculovirus Vector System for Protein Expression, Secretion, and Proteolytic Processing. BMC Biotechnol. 19, 1–11. doi:10.1186/s12896-019-0512-z
Li, Y. (2011). The Tandem Affinity Purification Technology: An Overview. Biotechnol. Lett. 33, 1487–1499. doi:10.1007/s10529-011-0592-x
Luckow, V. A., Lee, S. C., Barry, G. F., and Olins, P. O. (1993). Efficient Generation of Infectious Recombinant Baculoviruses by Site-specific Transposon-Mediated Insertion of Foreign Genes into a Baculovirus Genome Propagated in Escherichia coli. J. Virol. 67, 4566–4579. doi:10.1128/jvi.67.8.4566-4579.1993
Moraes, Â. M., Jorge, S. A. C., Astray, R. M., Suazo, C. A. T., Calderón Riquelme, C. E., Augusto, E. F. P., et al. (2012). Drosophila melanogaster S2 Cells for Expression of Heterologous Genes: From Gene Cloning to Bioprocess Development. Biotechnol. Adv. 30, 613–628. doi:10.1016/j.biotechadv.2011.10.009
Munro, S., and Pelham, H. R. B. (1986). An Hsp70-like Protein in the ER: Identity with the 78 Kd Glucose-Regulated Protein and Immunoglobulin Heavy Chain Binding Protein. Cell 46, 291–300. doi:10.1016/0092-8674(86)90746-4
Ohmuro-Matsuyama, Y., and Yamaji, H. (2018). Modifications of a Signal Sequence for Antibody Secretion from Insect Cells. Cytotechnology 70, 891–898. doi:10.1007/s10616-017-0109-0
Pamboukian, M. M., Jorge, S. A. C., Santos, M. G., Yokomizo, A. Y., Pereira, C. A., and Tonso, A. (2008). Insect Cells Respiratory Activity in Bioreactor. Cytotechnology 57, 37–44. doi:10.1007/s10616-007-9118-8
Park, B. S., Song, D. H., Kim, H. M., Choi, B.-S., Lee, H., and Lee, J.-O. (2009). The Structural Basis of Lipopolysaccharide Recognition by the TLR4-MD-2 Complex. Nature 458, 1191–1195. doi:10.1038/nature07830
Perret, B. G., Wagner, R., Lecat, S., Brillet, K., Rabut, G., Bucher, B., et al. (2003). Expression of EGFP-Amino-Tagged Human Mu Opioid Receptor in Drosophila Schneider 2 Cells: A Potential Expression System for Large-Scale Production of G-Protein Coupled Receptors. Protein Expr. Purif. 31, 123–132. doi:10.1016/S1046-5928(03)00140-2
Savitsky, P., Bray, J., Cooper, C. D. O., Marsden, B. D., Mahajan, P., Burgess-Brown, N. A., et al. (2010). High-throughput Production of Human Proteins for Crystallization: The SGC Experience. J. Struct. Biol. 172, 3–13. doi:10.1016/j.jsb.2010.06.008
Schmidt, T. G. M., Batz, L., Bonet, L., Carl, U., Holzapfel, G., Kiem, K., et al. (2013). Development of the Twin-Strep-Tag and its Application for Purification of Recombinant Proteins from Cell Culture Supernatants. Protein Expr. Purif. 92, 54–61. doi:10.1016/j.pep.2013.08.021
Sonoda, H., Kumada, Y., Katsuda, T., and Yamaji, H. (2012). Production of Single-Chain Fv-Fc Fusion Protein in Stably Transformed Insect Cells. Biochem. Eng. J. 67, 77–82. doi:10.1016/j.bej.2012.05.005
Ullah, R., Shah, M. A., Tufail, S., Ismat, F., Imran, M., Iqbal, M., et al. (2016). Activity of the Human Rhinovirus 3C Protease Studied in Various Buffers, Additives and Detergents Solutions for Recombinant Protein Production. PLoS One 11, e0153436. doi:10.1371/journal.pone.0153436
Vandenborre, G., Smagghe, G., Ghesquière, B., Menschaert, G., Nagender Rao, R., Gevaert, K., et al. (2011). Diversity in Protein Glycosylation Among Insect Species. PLoS One 6, e16682. doi:10.1371/journal.pone.0016682
Waugh, D. S. (2011). An Overview of Enzymatic Reagents for the Removal of Affinity Tags. Protein Expr. Purif. 80, 283–293. doi:10.1016/j.pep.2011.08.005
Wong, W., Huang, R., Menant, S., Hong, C., Sandow, J. J., Birkinshaw, R. W., et al. (2019). Structure of Plasmodium Falciparum Rh5-CyRPA-Ripr Invasion Complex. Nature 565, 118–121. doi:10.1038/s41586-018-0779-6
Zitzmann, J., Sprick, G., Weidner, T., Schreiber, C., and Czermak, P. (2017). “Process Optimization for Recombinant Protein Expression in Insect Cells,” in New Insights into Cell Culture Technology. London: InTech. doi:10.5772/67849
Keywords: insect cells, recombinant proteins, S2 cells, glycoproteins, secreted proteins, structural biology
Citation: Coker JA, Katis VL, Fairhead M, Schwenzer A, Clemmensen SB, Frandsen BU, de Jongh WA, Gileadi O, Burgess-Brown NA, Marsden BD, Midwood KS and Yue WW (2022) FAS2FURIOUS: Moderate-Throughput Secreted Expression of Difficult Recombinant Proteins in Drosophila S2 Cells. Front. Bioeng. Biotechnol. 10:871933. doi: 10.3389/fbioe.2022.871933
Received: 09 February 2022; Accepted: 04 April 2022;
Published: 05 May 2022.
Edited by:
Jose Ruben Morones-Ramirez, Autonomous University of Nuevo León, MexicoReviewed by:
Feng Wang, Southwest University, ChinaKathrin Göritzer, St George’s University of London, United Kingdom
Copyright © 2022 Coker, Katis, Fairhead, Schwenzer, Clemmensen, Frandsen, de Jongh, Gileadi, Burgess-Brown, Marsden, Midwood and Yue. This is an open-access article distributed under the terms of the Creative Commons Attribution License (CC BY). The use, distribution or reproduction in other forums is permitted, provided the original author(s) and the copyright owner(s) are credited and that the original publication in this journal is cited, in accordance with accepted academic practice. No use, distribution or reproduction is permitted which does not comply with these terms.
*Correspondence: Wyatt W. Yue, d3lhdHQueXVlQGNtZC5veC5hYy51aw==