- 1Department of Biosciences and Technology for Food, Agriculture and Environment, University of Teramo, Teramo, Italy
- 2Physiology of Reproduction Group, Department of Physiology, Faculty of Veterinary Medicine, International Excellence Campus for Higher Education and Research (Campus Mare Nostrum), University of Murcia, Murcia, Spain
- 3Institute of Biochemistry and Cell Biology (CNRIBBC/EMMA/Infrafrontier/IMPC), National Research Council, Rome, Italy
- 4Department of Mechanical Engineering, Politecnico di Milano, Milano, Italy
Nowadays there is an increasing demand for assisted reproductive technologies due to the growth of infertility problems. Naturally, fertilization occurs in the oviduct, where the oviductal epithelial cells (OECs) secrete many molecules that affect the embryo’s metabolism and protect it from oxidative stress. When the OECs are grown in 3D culture systems, they maintain a great part of their functional characteristics, making them an excellent model for in vitro fertilization (IVF) studies. In this work, we aimed to evaluate the suitability of different 3D-printing processes in conjunction with the corresponding set of commercially available biomaterials: extrusion-based processing using polylactic acid (PLA) and polycaprolactone (PCL) and stereolithography or digital-light processing using polyethylene-glycol-diacrylate (PEGDA) with different stiffness (PEGDA500, PEGDA200, PEGDA PhotoInk). All the 3D-printed scaffolds were used to support IVF process in a bovine embryo assay. Following fertilization, embryo development and quality were assessed in terms of cleavage, blastocyst rate at days 7 and 8, total cell number (TCN), inner cell mass/trophectoderm ratio (ICN/TE), and apoptotic cell ratio (ACR). We found a detrimental effect on cleavage and blastocyst rates when the IVF was performed on any medium conditioned by most of the materials available for digital-light processing (PEGDA200, PEGDA500). The observed negative effect could be possibly due to some leaked compound used to print and stabilize the scaffolds, which was not so evident however with PEGDA PhotoInk. On the other hand, all the extrusion-based processable materials did not cause any detrimental effect on cleavage or blastocyst rates. The principal component analysis reveals that embryos produced in presence of 3D-printed scaffolds produced via extrusion exhibit the highest similarity with the control embryos considering cleavage, blastocyst rates, TCN, ICN/TE and ACR per embryo. Conversely, all the photo-cross linkable materials or medium conditioned by PLA, lead to the highest dissimilarities. Since the use of PCL scaffolds, as well as its conditioned medium, bring to embryos that are more similar to the control group. Our results suggest that extrusion-based 3D printing of PCL could be the best option to be used for new IVF devices, possibly including the support of OECs, to enhance bovine embryo development.
1 Introduction
In recent years, the demand for artificial reproductive technologies (ARTs) is growing due to an increase in infertility, which already affects 15% of couples of reproductive age and continues to rise every year (Assidi, 2022). The high number of infertile couples, together with reproductively healthy ones seeking to prevent genetic diseases in their offspring, have contributed to an increase in the proportion of children born through ARTs in Europe, from 2.3% (De Geyter et al., 2020) to 3.5% (Gliozheni et al., 2022) in just 3 years. In human reproduction, a popular technique is intracytoplasmic sperm microinjection (ICSI) (Haddad et al., 2021), in which a sperm selected by the embryologist is directly injected into the ooplasm. With this technique, positive results are obtained despite the low motility of the sample or immaturity of the sperm (Palermo et al., 1996). However, there are main concerns about ICSI for its invasiveness since it involves the piercing of the membrane. As a result, it could induce spindle damage or the introduction of contaminating external material (Verpoest and Tournaye, 2009). Another option is in vitro fertilization (IVF), where the oocyte and sperm are co-cultured in the same plate for a certain period so that penetration occurs without human intervention. Although IVF has been associated with an increased risk of congenital diseases or developmental delay (Waynforth, 2018), this method is considered the most physiological, since the spermatozoa penetrates the oocyte by itself. In addition, the scientific community is increasingly concerned about the potential long-term effects of ARTs (Sunde et al., 2016; Fleming et al., 2018). It is known that suboptimal in vitro conditions influence the epigenetic reprogramming of embryos (Canovas et al., 2017; Ferraz et al., 2018b). In humans, it has been suggested that ARTs may be related to a higher risk of imprinting disorders such as Beckwith-Wiedemann (Maher et al., 2003) or Angelman syndrome (Manipalviratn et al., 2009), although in the latter, it is very difficult to understand whether these disorders are related to the couple´s infertility-subfertility problems or to ARTs (Pérez-Aytés et al., 2017). Moreover, differences in growth in ARTs-derived offspring in pig (París-Oller et al., 2022) and human (Ceelen et al., 2009) have also been observed.
All these above-mentioned problems could be solved by mimicking the physiological environment (i.e., the oviduct). In this organ, the oviductal epithelial cells (OECs) produce a large number of molecules that can protect embryos from oxidative stress and modify their metabolism (Ménézo et al., 2015). Indeed, two alternative strategies can be used to replicate natural conditions: 1) the use of reproductive fluid as a culture media supplement (Canovas et al., 2017) and 2) co-culture of gametes and embryos with oviductal epithelial cells (OECs) (Ferraz et al., 2018b). Two-D cultures (where cells grow in a monolayer) are the most popular for studying the physiology of the oviduct and have been used in IVF and embryo culture in several species (Kölle et al., 2020), probably due to their high reproducibility, low cost, or ease of handling (Costa et al., 2016). Indeed, when these cultures are used during embryo in vitro production (IVP), there is an enhanced developmental rate of bovine embryos (Abe and Hoshi, 1997). However, it has been shown that this 2D culture method is not the best suited for fertilization studies since the cells dedifferentiate and lose their polarity, morphology, secretory capacity, and ciliary activity (Ferraz et al., 2017). On the contrary, when cultured in 3D, these cells retain much of their natural features (Pennarossa et al., 2021), making them a better model by keeping gene and metabolic expression closer to the in vivo context than their 2D counterparts (Anton et al., 2015). When the physiological environment is mimicked using microfluidics culture during IVF, it has been shown that the epigenetic reprogramming of bovine embryos is more similar to in vivo derived embryos (Ferraz et al., 2018b). All these data are indicators of the limitations of the 2D culture methods, thus encouraging researchers to move towards 3D culture systems to improve the quality of IVP embryos. To achieve this goal, it is crucial to construct a 3D device in which it is possible to co-culture differentiated OECs with gametes/zygotes. As a matter of fact, despite the well-known relevance the oviduct in gamete maturation/activation, fertilization, and early embryo development, only a few bioengineering studies have been focused on these female reproductive structures, so far (Kessler et al., 2015; Xiao et al., 2017; Ferraz et al., 2018b; Ferraz et al., 2020; Francés-Herrero et al., 2022).
Nowadays, a great variety of 3D printable biomaterials are commercially available (Santoni et al., 2021). One popular biomaterial is polylactic acid (PLA), a promising biodegradable polymer that can be produced from renewable sources like sugarcane (Li et al., 2020). PLA-scaffolds have excellent biocompatibility (Shilov et al., 2022), and have been used for medical purposes in bone (Diomede et al., 2018; Velioglu et al., 2019) and cartilage regeneration (Rosenzweig et al., 2015). Together with PLA, polycaprolactone (PCL) is the most common biodegradable synthetic polymer used in tissue engineering (Arif et al., 2022), and it has already been employed for bone (Rumiński et al., 2018), liver (Huang et al., 2007) or skin (Ghosal et al., 2017) regenerative purposes. Similarly, photo-cross-linkable hydrogels are widely used, due to their tunable mechanical properties and to their capability to mimic native extracellular matrix (Lim et al., 2020; Zhang et al., 2022a). In fact, when viscoelasticity and stiffness properties of biomaterials can be tuned, this can represent an additional advantage to create scaffolds mimicking the native tissues with high resolution and complex architecture. Among them, polyethylene-glycol-diacrylate (PEGDA) is a synthetic polymer approved by the Food and Drug Administration (FDA) (Kim et al., 2022) that has been used in variegate studies, from bone (Rajabi et al., 2023) to cartilage (Zhang et al., 2022b) or muscle (Vannozzi et al., 2018) regeneration. In addition, PEGDA mechanical properties can be modulated by varying the molecular weight of the polymer (Nguyen et al., 2012) and it can be functionalized with cell binding motifs to enable cell adhesion (Della Sala et al., 2020). Despite the wide range of biomedical applications in which these materials have been used, no studies have been carried out so far to test the feasibility of these materials to construct a 3D-printed device for IVF.
Because of this lack of information, our study aims to evaluate the biocompatibility of different materials (PLA, PCL, PEGDA500, PEGDA200, and PEGDA PhotoInk) to support IVF, using bovine embryo development parameters (cleavage, blastocyst rates at day 7 and 8). In addition, to assess the quality of the in vitro produced embryos, we examined three fundamental parameters (Wydooghe et al., 2014) the cell number/embryo (TCN), the inner cell mass/trophectoderm (ICM/TE) ratio, and the apoptotic cell ratio (ACR).
2 Materials and methods
2.1 Experimental design
To evaluate the feasibility of different materials (PLA, PCL, PEGDA500, PEGDA200, and PEGDA PhotoInk) to support IVF and their effects on bovine embryo development, three experimental groups were settled for each of the materials tested:
- Control group: the IVF was performed, without having any contact with the materials (Figure 1A).
- Rinse group: to assess if these materials could release some unknown substances that could have adverse effects in IVF or embryo development, the IVF was carried out in a Fert-TALP medium conditioned by the scaffold of each material during 24 h (Figure 1B).
- Scaffold group: prior to fertilization, the same scaffold used to condition the IVF media, was transferred to another well with new media and the IVF was performed in the presence of the scaffold (Figure 1C).
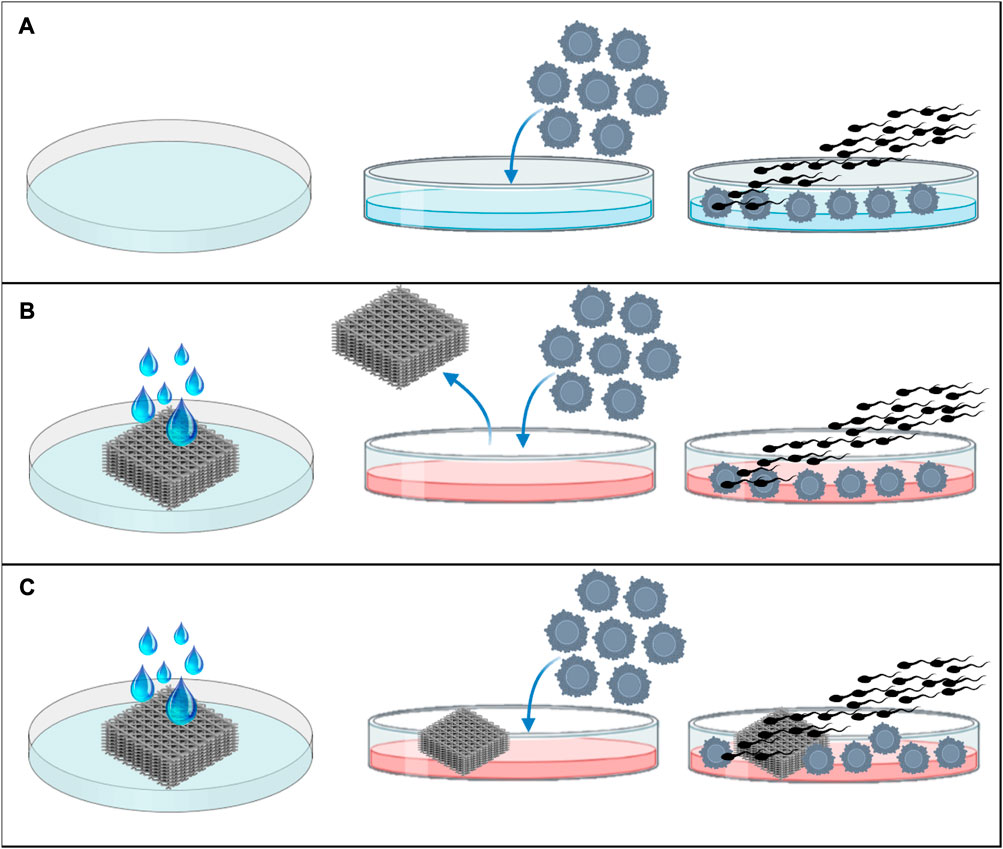
FIGURE 1. Experimental design. (A) IVF in normal Fert-TALP medium, without conditioning or scaffold, (B) IVF in Fert-TALP medium conditioned by scaffold for 24 h, and (C) IVF performed in presence of the rinsed scaffold.
The number of oocytes was n = 2892 distributed for each group as follows: n = 585 for control, n = 297 for scaffold PCL, n = 293 for rinse PCL, n = 196 for scaffold PLA, n = 201 for rinse PLA, n = 131 for scaffold PEGDA500, n = 143 for rinse PEGDA500, n = 248 for scaffold PEGDA200, n = 256 for rinse PEGDA200, n = 269 for scaffold PEGDA PhotoInk, n = 273 for rinse PEGDA PhotoInk. We carried out 11 replicates, the control group was present in every single replicate, while PCL material was present in 6 of them, the PLA material in 4, the PEGDA500 material in 3, and the PEGDA200 and PEGDA PhotoInk materials were present in 5 replicates.
2.2 Culture media reagents.
All chemicals were purchased from Sigma-Aldrich Quimica, S.A. (Madrid, Spain) unless otherwise indicated.
2.3 3D printing materials
3D printed structures were produced to test the biocompatibility of the material and the effect of the 3D architecture on cells. All structures were designed using SolidWorks software (Dassault Systèmes SE, Vélizy-Villacoublay, France) and exported as an STL file. Depending on the printer used, the STL file was directly loaded on the printer or sliced using PrusaSlicer (Prusa Research, Prague, Czech Republic) to obtain the gcode file.
The 3D models were printed using different materials with different stiffness (Table 1) and 3D printing methods. PLA filaments were purchased from Sharebot, Italy; PCL pellet (Mn = 50.000 g/mol), PEGDA200, PEGDA500, and PEGDA Photoink were purchased from Cellink, Sweeden. PLA filaments were printed via extrusion-based processing (FFF, Fused Filament Fabrication) using a Sharebot 42 3D printer (Sharebot, Italy) with a 0.4 mm diameter nozzle. PCL (CELLINK, Gothenburg, Sweden) structures were 3D printed using a BioX, a pneumatic extrusion-based 3D bioprinter (CELLINK, Gothenburg, Sweden) using a 0.4 mm nozzle, a pressure of 180 kPa, a velocity in a range 15–20 mm/s, and a temperature of 180°C according to suggested printing protocol. PEGDA500 Photoink, PEGDA200 Photoink, and PEGDA Photoink hydrogels (listed in decreasing order of stiffness) were 3D printed using a LumenX bioprinter based on stereolithography via digital light processing (CELLINK, Gothenburg, Sweden) considering a 50 µm layer height for the slicing and 20 mWatt/cm2 power, 3x as first layer time scale factor, and a variable time of 2/3/12 s depending on the formulation, respectively, according to printing protocol.
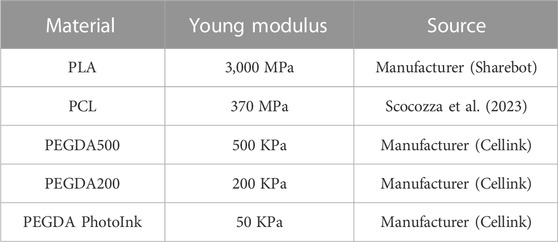
TABLE 1. Hardness of different materials (PLA, PCL, PEGDA500, PEGDA200, and PEGDA PhotoInk) expressed by Young’s modulus.
2.4 Material sterilization
PLA, PCL, PEGDA500, PEGDA200, and PEGDA PhotoInk were sterilized following the manufacturer’s instructions. Briefly, they were immersed in 70% ethanol for 5 min, then submerged twice in PBS (30 min each), and finally washed for 24 h with Fert-TALP (Parrish et al., 1986) culture medium supplemented con 175 U/mL heparin, 6 mg/mL BSA, 0.20 mM Na-pyruvate and 50 μg/mL gentamicin. Fert-TALP medium consisted of 114 mM sodium chloride, 3.2 mM potassium chloride, 0.3 Mm sodium phosphate monobasic monohydrate, 10 mM sodium lactate, 2.0 mM calcium chloride dihydrate, 0.5 mM magnesium chloride hexahydrate and 25 mM sodium bicarbonate.
2.5 In vitro maturation
Ovaries from 1 year old cows were transported from the local slaughterhouse to the laboratory in physiological saline solution (0.9% w/vol) supplemented with 100 mg/L kanamycin sulfate at 38.5°C within two hours of slaughter. Once in the laboratory, the ovaries were washed with a 0.04% cetrimide solution and twice with saline. In vitro maturation was performed as previously described (Lopes et al., 2019) with minor modifications. Briefly, follicles between 2- and 8-mm diameter were aspirated. Only Cumulus-Oocyte Complexes (COCs) with at least three cumulus cell layers and with a homogeneous cytoplasm were selected and then washed three times in handling medium, consisting of TCM 199 supplemented with 4.2 mM sodium bicarbonate, 10 mM HEPES, 2 mM glutamine, 1% w/v polyvinyl alcohol, 50 IU/mL penicillin and 50 μg/mL streptomycin. Subsequently, COCs were washed once in a maturation medium, consisting of TCM 199 (with Hanks’ salts) supplemented with 4.2 mM sodium bicarbonate, 2 mM glutamine, 50 IU/mL gentamicin, 10% v/v of bovine follicular fluid (BFF, NaturARTs-BFF, Embryocloud, Murcia, Spain), 10 IU/mL equine chorionic gonadotropin (Foligon, Intervet International BV, Netherlands) and 10 IU/mL human chorionic gonadotropin (Veterin Corion, Divasa Farmavic, Spain) and incubated in 500 μL of maturation medium in groups of 50–55 COCs in a four well dish at 38.5°C with a humidity-saturated atmosphere with 5% CO2 for 22 h.
2.6 In vitro fertilization
After maturation and 30 min before IVF, the oocytes were washed once in Fert-TALP medium supplemented con 175 U/mL heparin, 6 mg/mL BSA, 0.20 mM Na-pyruvate and 50 μg/mL gentamicin. For fertilization, frozen-thawed semen from three bulls of proven fertility was used. The straw was thawed in a water bath at 38.5°C for 30 s. Once thawed, a Bovipure gradient (Nidacon, Sweden) was performed, centrifuging at 300 g for 10 min and removing the supernatant. Before insemination, sperm cells were washed once in modified Sperm-TALP medium (Parrish et al., 1988) (114 mM sodium chloride, 3.2 mM potassium chloride, 0.3 Mm sodium phosphate monobasic monohydrate, 10 mM sodium lactate, 2.0 mM calcium chloride dihydrate, 0.5 mM magnesium chloride hexahydrate, 25 mM sodium bicarbonate and 10 mM HEPES) supplemented with 6 mg/mL BSA, 1.0 mM Na-pyruvate and 50 μg/mL gentamicin, by centrifuging at 300 g during 3 min and removing the supernatant. Insemination was performed in medium conditioned by the scaffold, in the presence of scaffold and in fresh medium, in a final concentration of 1 × 106 spz/mL. Oocytes were coincubated with the spermatozoa for 22 h at 38.5°C with a humidity-saturated atmosphere with 5% CO2.
2.7 Embryo culture
Twenty-two hours after insemination, the presumptive zygotes were moved into a 15 mL Falcon tube with a handling medium and vortexed for 4 min for decumulation. Zygotes were then washed once in Synthetic Oviductal Fluid medium (SOF) (Holm et al., 1999) and transferred into 50 μL microdrops of the same media covered by paraffin oil (Nidoil, Nidacon) in groups of 25 embryos per drop and cultured during 8 days at 38.5°C, 5% CO2 and 5% O2. Evaluation of embryo development occurred 48 h post insemination as the percentage of cleaved embryos out of presumptive zygotes, and at 7 and 8 days post insemination (dpi). In this study, only embryos with quality 1–2 according to the criteria of the International Embryo Technology Society (IETS) (summarized in Bó and Mapletoft, 2013) have been considered.
2.8 Differential apoptotic staining
To assess the total cell number (TCN), the inner cell mass/trophectoderm ratio (ICM/TE), and the apoptotic cell ratio (ACR), differential staining was performed as described previously (Wydooghe et al., 2011) with minor modifications. Briefly, day 8 blastocysts were fixed in 4% paraformaldehyde for 20 min at RT and conserved in 2% paraformaldehyde at 4°C until the moment of staining. The embryos were permeabilized with 0.5% Triton-X and 0.05% Tween in PBS overnight at 4°C. On the second day, blastocysts were washed three times for 10 min in PBS containing 0.5% BSA (washing solution). Subsequently, the DNA of the cells was denatured with 2N HCl for 20 min followed by 100 Mm trisHCL (pH = 8.5) for 10 min. After denaturation, the embryos were washed three times in washing solution and transferred to blocking solution (10% goat serum and 0.05% tween in PBS) overnight at 4°C. After blocking, the blastocysts were washed three times in washing solution and incubated in ready-to-use mouse anti-CDX2 primary antibody (Biogenex, San Ramon, United States) for overnight at 4°C, while two embryos remained in blocking solution as negative control. After this incubation, test embryos were washed three times in washing solution and incubated 1:500 dilution of rabbit anti -active caspase-3 primary antibody (Cell Signaling Technology, Leiden, Netherlands) in blocking solution overnight at 4°C. On the last day, all blastocysts (negative and test) were washed three times for 10 min in washing solution, and incubated with 1:100 goat anti-mouse TRICT (Abcam, Cambridge, United Kingdom) in blocking solution for 1 h at RT. After another three-wash step, the embryos were incubated in 1:200 goat anti-rabbit FITC secondary antibody (Abcam, Cambridge, United Kingdom) in blocking solution for 1 h at RT. Finally, the blastocysts were washed, stained with Hoechst 33342 for 15 min, washed for the last time, mounted in Dabco (1,4-Diazabicyclo[2.2.2]octane solution), and evaluated under fluorescence microscopy (Eclipse Ti Series, Nikon, Japan). A representative image of embryo was taken using Nikon A1r laser confocal scanning microscope.
2.9 Statistical analysis
For statistical analysis, GraphPad Prism 8 Software (La Jolla, CA, United States) was used. Data were checked for normal distribution with Shapiro-Wilk normality test prior to perform the comparison with parametric tests. In all cases the differences among groups were considered statistically significant when p < 0.05.
For Principal Component Analysis, Past 4.13 (Oslo, Norway) was used to evaluate the effect of different materials on Cleavage, blastocyst rate at day 7, blastocyst rate at day 8, TCN, ICM/TE and AC ratio.
3 Results
3.1 Effect of the different materials on cleavage
We observed a significant lower cleavage rate in rinse PEGDA500 (45% ± 15%), scaffold PEGDA500 (50% ± 23%), and rinse PEGDA200 (63% ± 8%) groups vs. CTRL group (84% ± 8%), while we did not observe any difference between the cleavage rate of rinse PCL (82% ± 10%), scaffold PCL (80% ± 10%), rinse PLA (77% ± 7%), scaffold PLA (81% ± 6%), scaffold PEGDA200 (73% ± 11%), rinse PEGDA PhotoInk (71% ± 14%) or scaffold PEGDA PhotoInk (77% ± 10%) compared to the control (Figure 2).
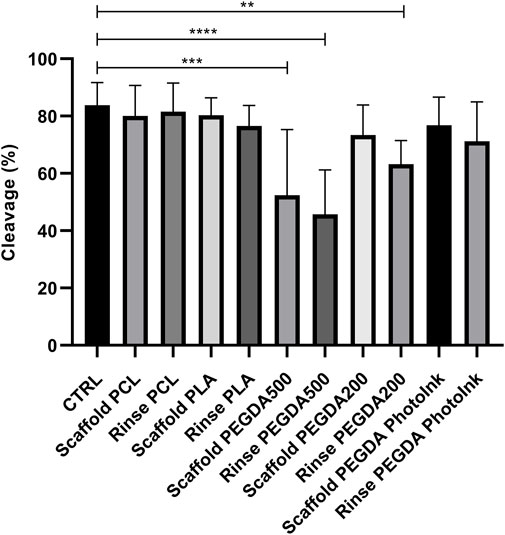
FIGURE 2. Effect of different materials on the percentage of cleaved embryos. The histograms show the cleavage rate when the IVF was performed with an unconditioned medium (CTRL), with medium conditioned by each material (rinse groups) or when different scaffolds were present (scaffold groups). The data show significant differences (p < 0.05) in CTRL vs. Scaffold PEGDA500, CTRL vs. Rinse PEGDA500 and CTRL vs. Rinse PEGDA200. The data are presented as the mean ± SD of 11 independent experiments. Data were analyzed using Dunnett’s test. **p < 0.01, ***p < 0.005, ****p < 0.0001 versus control.
3.2 Effect of the different materials on blastocyst rate at day 7
Compared to the CTRL group (23% ± 6%), blastocyst rates were significantly lower (p < 0.05) in the rinse PEGDA500 (4% ± 4%), scaffold PEGDA500 (7% ± 7%), and rinse PEGDA200 (8% ± 7%) groups on day 7 (Figure 3). The scaffold PCL group had a 25% ± 10% blastocyst yield, which was not statistically different (p > 0.05) vs. the CTRL, while scaffold PLA (17% ± 7%), scaffold PEGDA200 (14% ± 7%), and scaffold PEGDA PhotoInk (16% ± 4%) showed similar blastocyst rates. On the other hand, rinse groups have decreased embryo development compared to control but not significantly less than their scaffold groups, being 18% ± 4% for rinse PCL, 13% ± 4% for rinse PLA, and 16% ± 7% for rinse PEGDA PhotoInk (Figure 3).
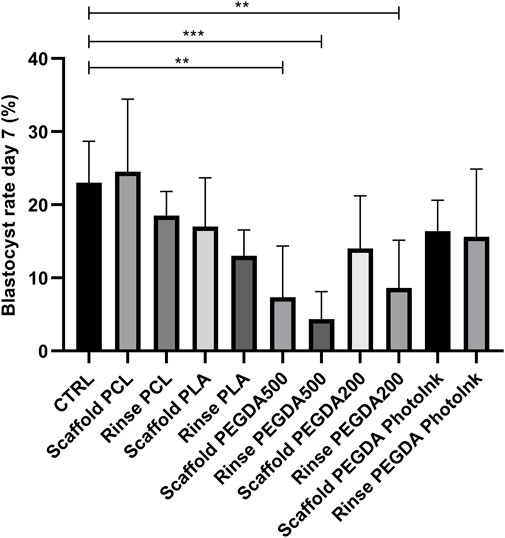
FIGURE 3. Effect of different materials on blastocyst rate at day 7. The histograms show the blastocyst rate at day 7 when the IVF was performed with unconditioned medium (CTRL), with medium conditioned by each material (rinse groups) or when different scaffolds were present (scaffold groups). The data show significant differences (p < 0.05) in CTRL vs. Scaffold PEGDA500, CTRL vs. Rinse PEGDA500 and CTRL vs. Rinse PEGDA200. The data are presented as the mean of 11 independent experiments. Data were analyzed using the Dunnett’s test. **p < 0.01, ***p < 0.005 versus control.
3.3 Effect of the different materials on blastocyst rate at day 8
Blastocyst rate at day 8 were significantly lower in rinse PEGDA500 (6% ± 6%), scaffold PEGDA500 (10% ± 9%), and rinse PEGDA200 (12% ± 9%) groups vs. CTRL group (25% ± 6%). On the scaffold’s groups, we had not statistical differences (p > 0.05) for blastocyst yield in the scaffold PCL group with 29% ± 8%, while the scaffold PLA (14% ± 2%), scaffold PEGDA200 (20% ± 9%) and scaffold PhotoInk (19% ± 5%) groups showed similar blastocyst rates. In addition, rinse groups did not present significant differences vs. the CTRL, being the blastocyst rates 22% ± 6% for rinse PCL, 14% ± 2% for rinse PLA, and 17% ± 11% for rinse PEGDA PhotoInk (Figure 4).
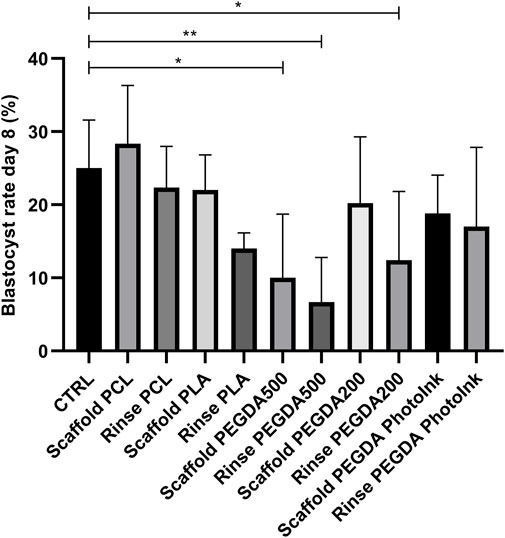
FIGURE 4. Effect of different materials scaffolds on blastocyst rate at day 8. The histograms show the blastocyst rate at day 8 when the IVF was performed with unconditioned medium (CTRL), with medium conditioned by each material (rinse groups) or when different scaffolds were present (scaffold groups). The data shows significant differences (p < 0.05) in CTRL vs. Scaffold PEGDA500, CTRL vs. Rinse PEGDA500 and CTRL vs. Rinse PEGDA200. The data are presented as mean of 11 independent experiments. Data were analyzed using the Dunnett’s test. *p < 0.05, **p < 0.01 versus control.
3.4 Principal component analysis of the different materials considering all variables studied
The total cell number, the trophectoderm and the apoptosis were evaluated under fluorescence microscopy (Figure 5) and the ICM/TE ratio and ACR were calculated. Since we studied several biological factors (cleavage, blastocyst rate at day 7 and 8, TCN, ICM/TE ratio and ACR), we used the Principal Component Analysis (PCA) as a multivariable analysis to simplify the data analysis and interpretation by reducing the complexity (Jollife and Cadima, 2016a). This analysis allows to reduce the amount of information needed since the system works with more compact representation of the data by retaining the relevant information and highlighting the underlying patterns and structures (Jollife and Cadima, 2016b) PCA showed how the scaffold PCL, rinse PCL, scaffold PLA and control groups were more similar among them than to the other groups (scaffold PEGDA500, rinse PEGDA500, rinse PEGDA200, scaffold PEGDA200, rinse PLA, rinse PhotoInk and scaffold PhotoInk) (Figure 6).
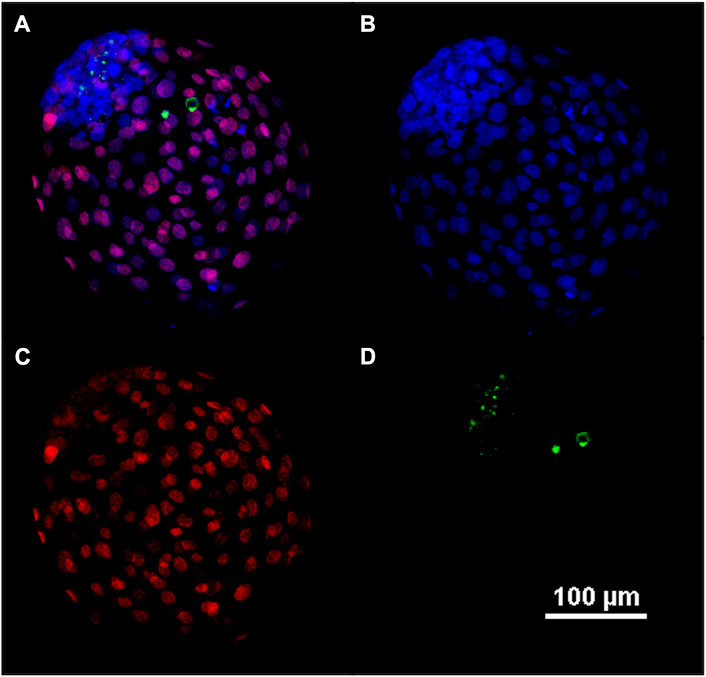
FIGURE 5. Representative confocal image of blastocyst at day 8. Fluorescent image of differential apoptotic staining (A–D). At day 8, bovine blastocysts were fixed, dyed with Hoechst 33342 for nuclei (B), immune-stained for CDX2 for the trophectoderm (C), and for active caspase-3 for the apoptosis (D). In (A) an overlay (B–D) is provided.
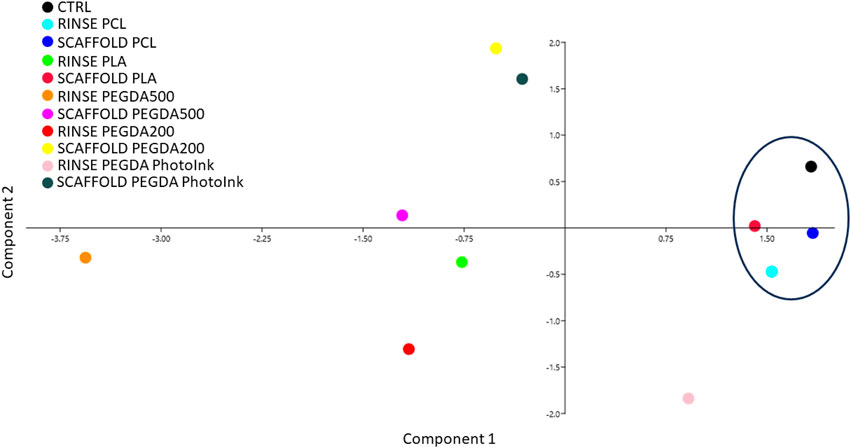
FIGURE 6. Principal component analysis performed by assessing the different parameters studied (Cleavage, blastocyst rate at days 7 and 8, TCN, ICM/TE and ACR). Principal component analysis shows no separation among groups. However, we observed that the closest groups to control are scaffold PLA, scaffold PCL and rinse PCL.
4 Discussion
In the present study we performed a bovine embryo assay to evaluate the potential toxicity of PLA, PCL, PEGDA500, PEGDA200, and PEGDA PhotoInk biomaterials in the embryo IVP during IVF. In addition, we tested not only different materials but also different printing methods, using for each material the most suitable method for the scaffold construction needs. We chose this animal model since it has already been used for embryo assay (Ieda et al., 2018) and it represents a valuable model for IVF improvement trials (Ménézo and Hérubel, 2002). It is also well-known that IVP produces suboptimal embryos with a lower yield of blastocysts and lower developmental capacity than their in vivo counterparts (Heras et al., 2016; Canovas et al., 2017; Ferraz et al., 2018b).
The first step in producing a device that could improve the IVP is the choice of biomaterial. All the materials we propose have been used in cell culture and have shown a good biocompatibility (Eslahi et al., 2013; Biagini et al., 2021; Di Berardino et al., 2022; Testore et al., 2022). However, as the cytotoxicity of the materials such as PLA (Biagini et al., 2021) could be different according to the cell types, the biocompatibility should be tested in regard to gametes, zygotes and embryos.
Our results suggest that the only material that has toxic effects is PEGDA500. This material had detrimental effect on bovine embryo development, promoting lower cleavage and lower blastocyst rate at day 7 and 8. This is an unexpected effect since PEGDA hydrogels have been suggested as effective candidates to carry out studies for embryogenesis and organogenesis due to their low cost, high reproducibility, and ease fabrication (Hribar et al., 2015). This is not the first time that biomaterials have shown unexpected negative effects when in contact with embryos. MacDonald et al. (2016) showed that VisiJet Crystal material (belonging to the strictest class for plastic biocompatibility) had a detrimental effect on zebrafish embryos (MacDonald et al., 2016). The materials E-shell200 and E-shell300 have also shown a deleterious effect on bovine embryo development, even having been considered biocompatible according to ISO 10993 (Ferraz et al., 2018a). Furthermore, we must take into consideration the eventuality that our materials might not be exactly the same in chemistry as those used in previously works, since the full chemical composition may vary from one company to another. Another plausible factor could be the fact that in the 3D printed scaffolds could be found some residues of toxic compounds that have been used to stabilize and print the devices. Indeed, several studies have observed that different chemical species are leaked by 3D-printed scaffolds (Oskui et al., 2016; Ferraz et al., 2018a).
Additionally, we detected significant differences between the rinse group of PEGDA200 and CTRL group, but no differences when PEGDA200 scaffolds were compared to the controls. This result suggests that the PEGDA200 may require longer washing than the other PEGDA hydrogels, since this type of scaffold had no detrimental effects on embryo development during IVF after being washed for 24 h and rinsed for another 24 h. However, all these hydrogels might not be the best option for the IVF device manufacturing because they were very fragile, and their rupture could be a relevant inconvenience during sterilization and handling.
Conversely, neither PLA nor PCL have shown detrimental effects on cleavage and blastocyst rate parameters. The PLA synthetic polymer has been suggested as an optimal candidate for scaffold fabrication due to its high biocompatibility, low cost, and mechanical properties (Serra et al., 2013; Di Prima et al., 2016). To our knowledge, this is the first study testing those materials to support bovine IVF, showing high biocompatibility. This is an expected result since both PLA and PCL biomaterials have been used in an emerging field called REPROTEN, the discipline that applies tissue engineering to restore fertility (Amorim, 2017). It has been shown that PLA is a suitable material to create a nanofiber scaffold that enhance the in vitro cluster formation of mouse spermatogonia stem cells, allowing their proliferation and differentiation (Eslahi et al., 2013; Ghorbani et al., 2019). As well, PCL has been used to culture spermatogonia stem cells (Talebi et al., 2019; Ghorbani et al., 2022), obtaining the same successful results as PLA. Furthermore, recent works have used PCL scaffolds as devices to carry out folliculogenesis in sheep (Di Berardino et al., 2022) and pig (Liverani et al., 2019).
We observed worse results with the increase of the PEGDA material stiffness (PEGDA Photoink vs PEGDA200 vs. PEGDA500). Previously, it has been shown that the stiffness of different substrates can affect in vitro embryo development in mice (Kolahi et al., 2012), but in our case the lower efficiency could be due to the chemistry employed to promote higher stiffness of the material, since even when the scaffold is absent during IVF, the rinse groups showed lower efficiency. One possible explanation for the worse performance of the rinse groups could be that the scaffolds release toxic compounds during the rinse period culture, so when the scaffolds are used during IVF the release of these toxic chemicals is much lower or absent. However, to confirm this hypothesis mass spectrometry analysis should be performed.
Altogether, these data suggest that the materials printed with stereolithography (PEGDAs) are less biocompatible than extrusion-printed materials (PLA and PCL). On the other hand, the TCN, the ICM/TE ratio and the ACR are three important parameters of embryo quality and in recent years, several studies have shown that the rate of ICM/TE is a strong predictor of live birth (Ai et al., 2021; Sivanantham et al., 2022). When we analyzed all these parameters, the principal component analysis (PCA) showed that the embryos produced in presence of PLA and PCL scaffolds are the most comparable to the control group. Regarding the PCL biopolymer, the rinse and the scaffold groups are both closer to the control ones, in terms of the analyzed parameters. While a different situation using the PLA biomaterial has been observed, since the scaffold group exhibited similar behavior to the control, contrary to the rinse group of the same biopolymer. For this reason, we consider PCL as the most suitable material for in vitro bovine embryo production.
Considering that we have not identified any negative effects on bovine embryo development when PCL is present during IVF, its implementation in the construction of a device compatible with microfluidics systems becomes a promising possibility. The combination of these microfluidics systems with the above-mentioned devices could allow the creation of an in vitro model of the oviduct (Romar et al., 2019). This innovative application could have a significant impact on the research and understanding of sperm selection by mimicking rheotaxis, chemotaxis and thermotaxis (Pérez-Cerezales et al., 2018; Ramal-Sanchez et al., 2021), fertilization and early development processes, providing a controlled and reproducible environment for experimental studies, without jeopardizing early embryo development.
In conclusion, the utilization of PCL in the construction of an IVF device holds great promise for the improvement of ARTs in the near future. However, further research and development are necessary to test the biocompatibility with OECs, optimize the design and functionality of this PCL-based IVF devices, ensuring their long-term effectiveness and safety. Nonetheless, the outcomes of our study strongly support the potential of the PCL biomaterial and open the way for advancements in the field of ARTs.
Data availability statement
The original contributions presented in the study are included in the article/Supplementary Material, further inquiries can be directed to the corresponding author.
Ethics statement
Ethical approval was not required for the studies involving animals in accordance with the local legislation and institutional requirements because the ovaries were collected from cows slaughtered for feed purposes. Written informed consent was obtained from the owners for the participation of their animals in this study.
Author contributions
RB-P: Data curation, Formal Analysis, Methodology, Writing–original draft, Writing–review and editing. SH: Methodology, Writing–review and editing. CC: Data curation, Formal Analysis, Writing–review and editing. JR-A: Methodology, Writing–review and editing. LV: Writing–review and editing. AC: Writing–review and editing. BC: Methodology, Writing–review and editing. SS: Methodology, Writing–review and editing. BB: Funding acquisition, Supervision, Writing–review and editing. NB: Conceptualization, Data curation, Formal Analysis, Funding acquisition, Supervision, Writing–review and editing. PC: Conceptualization, Funding acquisition, Supervision, Writing–review and editing.
Funding
The author(s) declare that no financial support was received for the research, authorship, and/or publication of this article.
Conflict of interest
The authors declare that the research was conducted in the absence of any commercial or financial relationships that could be construed as a potential conflict of interest.
Publisher’s note
All claims expressed in this article are solely those of the authors and do not necessarily represent those of their affiliated organizations, or those of the publisher, the editors and the reviewers. Any product that may be evaluated in this article, or claim that may be made by its manufacturer, is not guaranteed or endorsed by the publisher.
References
Abe, H., and Hoshi, H. (1997). Bovine oviductal epithelial cells: their cell culture and applications in studies for reproductive biology. Cytotechnology 23, 171–183. doi:10.1023/A:1007929826186
Ai, J., Jin, L., Zheng, Y., Yang, P., Huang, B., and Dong, X. (2021). The morphology of inner cell mass is the strongest predictor of live birth after a frozen-thawed single embryo transfer. Front. Endocrinol. (Lausanne) 12, 621221. doi:10.3389/fendo.2021.621221
Amorim, C. A. (2017). Special issue devoted to a new field of regenerative medicine: reproductive tissue engineering. Ann. Biomed. Eng. 45, 1589–1591. doi:10.1007/s10439-017-1862-0
Anton, D., Burckel, H., Josset, E., and Noel, G. (2015). Three-dimensional cell culture: A breakthrough in vivo. Int. J. Mol. Sci. 16, 5517–5527. doi:10.3390/ijms16035517
Arif, Z. U., Khalid, M. Y., Noroozi, R., Sadeghianmaryan, A., Jalalvand, M., and Hossain, M. (2022). Recent advances in 3D-printed polylactide and polycaprolactone-based biomaterials for tissue engineering applications. Int. J. Biol. Macromol. 218, 930–968. doi:10.1016/j.ijbiomac.2022.07.140
Assidi, M. (2022). Infertility in men: advances towards a comprehensive and integrative strategy for precision theranostics. Cells 11, 1711. doi:10.3390/cells11101711
Biagini, G., Senegaglia, A. C., Pereira, T., Berti, L. F., Marcon, B. H., and Stimamiglio, M. A. (2021). 3D poly(lactic acid) scaffolds promote different behaviors on endothelial progenitors and adipose-derived stromal cells in comparison with standard 2D cultures. Front. Bioeng. Biotechnol. 9, 700862. doi:10.3389/FBIOE.2021.700862
Bó, G. A., and Mapletoft, R. J. (2013). Evaluation and classification of bovine embryos. Anim. Reprod. Ar. 10, 344–348.
Canovas, S., Ivanova, E., Romar, R., García-Martínez, S., Soriano-Úbeda, C., García-Vázquez, F. A., et al. (2017). DNA methylation and gene expression changes derived from assisted reproductive technologies can be decreased by reproductive fluids. Elife 6, e23670. doi:10.7554/eLife.23670
Ceelen, M., Van Weissenbruch, M. M., Prein, J., Smit, J. J., Vermeiden, J. P. W., Spreeuwenberg, M., et al. (2009). Growth during infancy and early childhood in relation to blood pressure and body fat measures at age 8–18 years of IVF children and spontaneously conceived controls born to subfertile parents. Hum. Reprod. 24, 2788–2795. doi:10.1093/HUMREP/DEP273
Costa, E. C., Moreira, A. F., de Melo-Diogo, D., Gaspar, V. M., Carvalho, M. P., and Correia, I. J. (2016). 3D tumor spheroids: an overview on the tools and techniques used for their analysis. Biotechnol. Adv. 34, 1427–1441. doi:10.1016/J.BIOTECHADV.2016.11.002
De Geyter, C., Calhaz-Jorge, C., Kupka, M. S., Wyns, C., Mocanu, E., Motrenko, T., et al. (2020). ART in Europe, 2015: results generated from european registries by eshre. Hum. Reprod. Open 2020, hoz038. doi:10.1093/HROPEN/HOZ038
Della Sala, F., Biondi, M., Guarnieri, D., Borzacchiello, A., Ambrosio, L., and Mayol, L. (2020). Mechanical behavior of bioactive poly(ethylene glycol) diacrylate matrices for biomedical application. J. Mech. Behav. Biomed. Mater 110, 103885. doi:10.1016/J.JMBBM.2020.103885
Di Berardino, C., Liverani, L., Peserico, A., Capacchietti, G., Russo, V., Bernabò, N., et al. (2022). When electrospun fiber support matters: in vitro ovine long-term folliculogenesis on poly (epsilon caprolactone) (pcl)-patterned fibers. Cells 11, 1968. doi:10.3390/cells11121968
Di Prima, M., Coburn, J., Hwang, D., Kelly, J., Khairuzzaman, A., and Ricles, L. (2016). Additively manufactured medical products - the FDA perspective. 3D Print Med. 2, 1. doi:10.1186/S41205-016-0005-9
Diomede, F., Gugliandolo, A., Cardelli, P., Merciaro, I., Ettorre, V., Traini, T., et al. (2018). Three-dimensional printed PLA scaffold and human gingival stem cell-derived extracellular vesicles: A new tool for bone defect repair. Stem Cell Res. Ther. 9, 104–121. doi:10.1186/s13287-018-0850-0
Eslahi, N., Hadjighassem, M. R., Joghataei, M. T., Mirzapour, T., Bakhtiyari, M., Shakeri, M., et al. (2013). The effects of poly L-lactic acid nanofiber scaffold on mouse spermatogonial stem cell culture. Int. J. Nanomedicine 8, 4563–4576. doi:10.2147/IJN.S45535
Ferraz, M. A. M. M., Henning, H. H. W., Da Costa, P. F., Malda, J., Le Gac, S., Bray, F., et al. (2018a). Potential health and environmental risks of three-dimensional engineered polymers. Environ. Sci. Technol. Lett. 5, 80–85. doi:10.1021/acs.estlett.7b00495
Ferraz, M. A. M. M., Henning, H. H. W., Stout, T. A. E., Vos, P. L. A. M., and Gadella, B. M. (2017). Designing 3-dimensional in vitro oviduct culture systems to study mammalian fertilization and embryo production. Ann. Biomed. Eng. 45, 1731–1744. doi:10.1007/s10439-016-1760-x
Ferraz, M. A. M. M., Nagashima, J. B., Venzac, B., Le Gac, S., and Songsasen, N. (2020). A dog oviduct-on-a-chip model of serous tubal intraepithelial carcinoma. Sci. Rep. 10, 1–11. doi:10.1038/s41598-020-58507-4
Ferraz, M. A. M. M., Rho, H. S., Hemerich, D., Henning, H. H. W., van Tol, H. T. A., Hölker, M., et al. (2018b). An oviduct-on-a-chip provides an enhanced in vitro environment for zygote genome reprogramming. Nat. Commun. 9, 4934. doi:10.1038/s41467-018-07119-8
Fleming, T. P., Watkins, A. J., Velazquez, M. A., Mathers, J. C., Prentice, A. M., Stephenson, J., et al. (2018). Origins of lifetime health around the time of conception: causes and consequences. Lancet 391, 1842–1852. doi:10.1016/S0140-6736(18)30312-X
Francés-Herrero, E., Lopez, R., Hellström, M., De Miguel-Gómez, L., Herraiz, S., Brännström, M., et al. (2022). Bioengineering trends in female reproduction: A systematic review. Hum. Reprod. Update 28, 798–837. doi:10.1093/humupd/dmac025
Ghorbani, M., Nourani, M. R., Alizadeh, H., and Goodarzi, V. (2022). Evaluation of the growth and differentiation of spermatogonial stem cells on a 3D polycaprolactone/multi-walled carbon nanotubes-based microfibrous scaffold. J. Appl. Biotechnol. Rep. 9, 846–855. doi:10.30491/JABR.2022.312357.1463
Ghorbani, S., Eyni, H., Khosrowpour, Z., Salari Asl, L., Shabani, R., Nazari, H., et al. (2019). Spermatogenesis induction of spermatogonial stem cells using nanofibrous poly(l-lactic acid)/multi-walled carbon nanotube scaffolds and naringenin. Polym. Adv. Technol. 30, 3011–3025. doi:10.1002/PAT.4733
Ghosal, K., Manakhov, A., Zajíčková, L., and Thomas, S. (2017). Structural and surface compatibility study of modified electrospun poly(ε-caprolactone) (PCL) composites for skin tissue engineering. AAPS PharmSciTech 18, 72–81. doi:10.1208/S12249-016-0500-8
Gliozheni, O., Hambartsoumian, E., Strohmer, H., Petrovskaya, E., Tishkevich, O., de Neubourg, D., et al. (2022). ART in Europe, 2018: results generated from european registries by eshre. Hum. Reprod. Open 2022, hoac022. doi:10.1093/HROPEN/HOAC022
Haddad, M., Stewart, J., Xie, P., Cheung, S., Trout, A., Keating, D., et al. (2021). Thoughts on the popularity of ICSI. J. Assist. Reprod. Genet. 38, 101–123. doi:10.1007/S10815-020-01987-0
Heras, S., De Coninck, D. I. M., Van Poucke, M., Goossens, K., Bogado Pascottini, O., Van Nieuwerburgh, F., et al. (2016). Suboptimal culture conditions induce more deviations in gene expression in male than female bovine blastocysts. BMC Genomics 17, 72. doi:10.1186/S12864-016-2393-Z
Holm, P., Booth, P. J., Schmidt, M. H., Greve, T., and Callesen, H. (1999). High bovine blastocyst development in a static in vitro production system using SOFaa medium supplemented with sodium citrate and myo-inositol with or without serum-proteins. Theriogenology 52, 683–700. doi:10.1016/S0093-691X(99)00162-4
Hribar, K. C., Finlay, D., Ma, X., Qu, X., Ondeck, M. G., Chung, P. H., et al. (2015). Nonlinear 3D projection printing of concave hydrogel microstructures for long-term multicellular spheroid and embryoid body culture. Lab. Chip 15, 2412–2418. doi:10.1039/c5lc00159e
Huang, H., Oizumi, S., Kojima, N., Niino, T., and Sakai, Y. (2007). Avidin-biotin binding-based cell seeding and perfusion culture of liver-derived cells in a porous scaffold with a three-dimensional interconnected flow-channel network. Biomaterials 28, 3815–3823. doi:10.1016/J.BIOMATERIALS.2007.05.004
Ieda, S., Akai, T., Sakaguchi, Y., Shimamura, S., Sugawara, A., Kaneda, M., et al. (2018). A microwell culture system that allows group culture and is compatible with human single media. J. Assist. Reprod. Genet. 35, 1869–1880. doi:10.1007/s10815-018-1252-z
Jollife, I. T., and Cadima, J. (2016a). Principal component analysis: A review and recent developments. Philosophical Trans. R. Soc. A Math. Phys. Eng. Sci. 374, 20150202. doi:10.1098/rsta.2015.0202
Jollife, I. T., and Cadima, J. (2016b). Principal component analysis: A review and recent developments. Philosophical Trans. R. Soc. A Math. Phys. Eng. Sci. 374, 20150202. doi:10.1098/rsta.2015.0202
Kessler, M., Hoffmann, K., Brinkmann, V., Thieck, O., Jackisch, S., Toelle, B., et al. (2015). The Notch and Wnt pathways regulate stemness and differentiation in human fallopian tube organoids. Nat. Commun. 6, 8989. doi:10.1038/NCOMMS9989
Kim, S., Lee, H., Choi, H., Yoo, K. Y., and Yoon, H. (2022). Investigation on photopolymerization of PEGDA to fabricate high-aspect-ratio microneedles. RSC Adv. 12, 9550–9555. doi:10.1039/D2RA00189F
Kolahi, K. S., Donjacour, A., Liu, X., Lin, W., Simbulan, R. K., Bloise, E., et al. (2012). Effect of substrate stiffness on early mouse embryo development. PLoS One 7, e41717. doi:10.1371/JOURNAL.PONE.0041717
Kölle, S., Hughes, B., and Steele, H. (2020). Early embryo-maternal communication in the oviduct: A review. Mol. Reprod. Dev. 87, 650–662. doi:10.1002/MRD.23352
Li, G., Zhao, M., Xu, F., Yang, B., Li, X., Meng, X., et al. (2020). Synthesis and biological application of polylactic acid. Molecules 25, 5023. doi:10.3390/MOLECULES25215023
Lim, K. S., Galarraga, J. H., Cui, X., Lindberg, G. C. J., Burdick, J. A., and Woodfield, T. B. F. (2020). Fundamentals and applications of photo-cross-linking in bioprinting. Chem. Rev. 120, 10662–10694. doi:10.1021/ACS.CHEMREV.9B00812
Liverani, L., Raffel, N., Fattahi, A., Preis, A., Hoffmann, I., Boccaccini, A. R., et al. (2019). Electrospun patterned porous scaffolds for the support of ovarian follicles growth: A feasibility study. Sci. Rep. 9 (19), 1150–1214. doi:10.1038/s41598-018-37640-1
Lopes, J. S., Canha-Gouveia, A., París-Oller, E., and Coy, P. (2019). Supplementation of bovine follicular fluid during in vitro maturation increases oocyte cumulus expansion, blastocyst developmental kinetics, and blastocyst cell number. Theriogenology 126, 222–229. doi:10.1016/j.theriogenology.2018.12.010
MacDonald, N. P., Zhu, F., Hall, C. J., Reboud, J., Crosier, P. S., Patton, E. E., et al. (2016). Assessment of biocompatibility of 3D printed photopolymers using zebrafish embryo toxicity assays. Lab. Chip 16, 291–297. doi:10.1039/c5lc01374g
Maher, E. R., Brueton, L. A., Bowdin, S. C., Luharia, A., Cooper, W., Cole, T. R., et al. (2003). Beckwith-Wiedemann syndrome and assisted reproduction technology (ART). J. Med. Genet. 40, 62–64. doi:10.1136/JMG.40.1.62
Manipalviratn, S., DeCherney, A., and Segars, J. (2009). Imprinting disorders and assisted reproductive technology. Fertil. Steril. 91, 305–315. doi:10.1016/J.FERTNSTERT.2009.01.002
Ménézo, Y., Guérin, P., and Elder, K. (2015). The oviduct: A neglected organ due for re-assessment in IVF. Reprod. Biomed. Online 30, 233–240. doi:10.1016/j.rbmo.2014.11.011
Ménézo, Y. J. R., and Hérubel, F. (2002). Mouse and bovine models for human IVF. Reprod. Biomed. Online 4, 170–175. doi:10.1016/S1472-6483(10)61936-0
Nguyen, Q. T., Hwang, Y., Chen, A. C., Varghese, S., and Sah, R. L. (2012). Cartilage-like mechanical properties of poly (ethylene glycol)-diacrylate hydrogels. Biomaterials 33, 6682–6690. doi:10.1016/J.BIOMATERIALS.2012.06.005
Oskui, S. M., Diamante, G., Liao, C., Shi, W., Gan, J., Schlenk, D., et al. (2016). Assessing and reducing the toxicity of 3D-printed parts. Environ. Sci. Technol. Lett. 3, 1–6. doi:10.1021/acs.estlett.5b00249
Palermo, G. D., Schlegel, P. N., Colombero, L. T., Zaninovic, N., Moy, F., and Rosenwaks, Z. (1996). Aggressive sperm immobilization prior to intracytoplasmic sperm injection with immature spermatozoa improves fertilization and pregnancy rates. Hum. Reprod. 11, 1023–1029. doi:10.1093/OXFORDJOURNALS.HUMREP.A019290
París-Oller, E., Soriano-Úbeda, C., Belda-Pérez, R., Sarriás-Gil, L., Lopes, J. S., Canha-Gouveia, A., et al. (2022). Reproductive fluids, added to the culture media, contribute to minimizing phenotypical differences between in vitro-derived and artificial insemination-derived piglets. J. Dev. Orig. Health Dis. 13, 593–605. doi:10.1017/S2040174421000702
Parrish, J. J., Susko-Parrish, J. L., Leibfried-Rutledge, M. L., Critser, E. S., Eyestone, W. H., and First, N. L. (1986). Bovine in vitro fertilization with frozen-thawed semen. Theriogenology 25, 591–600. doi:10.1016/0093-691X(86)90143-3
Parrish, J. J., Susko-Parrish, J., Winer, M. A., and First, N. L. (1988). Capacitation of bovine sperm by heparin. Biol. Reprod. 38, 1171–1180. doi:10.1095/biolreprod38.5.1171
Pennarossa, G., Arcuri, S., De Iorio, T., Gandolfi, F., and Brevini, T. A. L. (2021). Current advances in 3D tissue and organ reconstruction. Int. J. Mol. Sci. 22, 830–926. doi:10.3390/IJMS22020830
Pérez-Aytés, A., Arcos-Machancoses, J. V., Marin Reina, P., Jimenez Busselo, M. T., and Martínez, F. (2017). Artificial reproductive techniques and epigenetic alterations: additional comments to the article by arcos-machancoses et al. Am. J. Med. Genet. A 173, 1983–1984. doi:10.1002/AJMG.A.38273
Pérez-Cerezales, S., Ramos-Ibeas, P., Acuna, O. S., Avilés, M., Coy, P., Rizos, D., et al. (2018). The oviduct: from sperm selection to the epigenetic landscape of the embryo. Biol. Reprod. 98, 262–276. doi:10.1093/BIOLRE/IOX173
Rajabi, M., Cabral, J. D., Saunderson, S., and Ali, M. A. (2023). 3D printing of chitooligosaccharide-polyethylene glycol diacrylate hydrogel inks for bone tissue regeneration. J. Biomed. Mater Res. A 111, 1468–1481. doi:10.1002/JBM.A.37548
Ramal-Sanchez, M., Bernabò, N., Valbonetti, L., Cimini, C., Taraschi, A., Capacchietti, G., et al. (2021). Role and modulation of TRPV1 in mammalian spermatozoa: an updated review. Int. J. Mol. Sci. 22, 4306. doi:10.3390/IJMS22094306
Romar, R., Cánovas, S., Matás, C., Gadea, J., and Coy, P. (2019). Pig in vitro fertilization: where are we and where do we go? Theriogenology 137, 113–121. doi:10.1016/j.theriogenology.2019.05.045
Rosenzweig, D. H., Carelli, E., Steffen, T., Jarzem, P., and Haglund, L. (2015). 3D-Printed ABS and PLA scaffolds for cartilage and nucleus pulposus tissue regeneration. Int. J. Mol. Sci. 16, 15118–15135. doi:10.3390/IJMS160715118
Rumiński, S., Ostrowska, B., Jaroszewicz, J., Skirecki, T., Włodarski, K., Święszkowski, W., et al. (2018). Three-dimensional printed polycaprolactone-based scaffolds provide an advantageous environment for osteogenic differentiation of human adipose-derived stem cells. J. Tissue Eng. Regen. Med. 12, e473–e485. doi:10.1002/TERM.2310
Santoni, S., Gugliandolo, S. G., Sponchioni, M., Moscatelli, D., and Colosimo, B. M. (2021). 3D bioprinting: current status and trends—a guide to the literature and industrial practice. Bio-Design Manuf. 5 (15), 14–42. doi:10.1007/S42242-021-00165-0
Scocozza, F., Di Gravina, G. M., Bari, E., Auricchio, F., Torre, M. L., and Conti, M. (2023). Prediction of the mechanical response of a 3D (bio)printed hybrid scaffold for improving bone tissue regeneration by structural finite element analysis. J. Mech. Behav. Biomed. Mater 142, 105822. doi:10.1016/J.JMBBM.2023.105822
Serra, T., Planell, J. A., and Navarro, M. (2013). High-resolution PLA-based composite scaffolds via 3-D printing technology. Acta Biomater. 9, 5521–5530. doi:10.1016/J.ACTBIO.2012.10.041
Shilov, S. Y., Rozhkova, Y. A., Markova, L. N., Tashkinov, M. A., Vindokurov, I. V., and Silberschmidt, V. V. (2022). Biocompatibility of 3D-printed PLA, PEEK and PETG: adhesion of bone marrow and peritoneal lavage cells. Adhesion Bone Marrow Perit. Lavage Cells 14, 3958. doi:10.3390/polym14193958
Sivanantham, S., Saravanan, M., Sharma, N., Shrinivasan, J., and Raja, R. (2022). Morphology of inner cell mass: A better predictive biomarker of blastocyst viability. PeerJ 10, e13935. doi:10.7717/peerj.13935
Sunde, A., Brison, D., Dumoulin, J., Harper, J., Lundin, K., Magli, M. C., et al. (2016). Time to take human embryo culture seriously: table i. Hum. Reprod. 31, 2174–2182. doi:10.1093/HUMREP/DEW157
Talebi, A., Sadighi Gilani, M. A., Koruji, M., Ai, J., Rezaie, M. J., Navid, S., et al. (2019). Colonization of mouse spermatogonial cells in modified soft agar culture system utilizing nanofibrous scaffold: A new approach. Galen Med. J. 8, 1319. doi:10.31661/gmj.v8i0.1319
Testore, D., Zoso, A., Kortaberria, G., Sangermano, M., and Chiono, V. (2022). Electroconductive photo-curable PEGDA-gelatin/PEDOT:PSS hydrogels for prospective cardiac tissue engineering application. Front. Bioeng. Biotechnol. 10, 897575. doi:10.3389/fbioe.2022.897575
Vannozzi, L., Yasa, I. C., Ceylan, H., Menciassi, A., Ricotti, L., and Sitti, M. (2018). Self-folded hydrogel tubes for implantable muscular tissue scaffolds. Macromol. Biosci. 18, 1700377. doi:10.1002/MABI.201700377
Velioglu, Z. B., Pulat, D., Demirbakan, B., Ozcan, B., Bayrak, E., and Erisken, C. (2019). 3D-printed poly(lactic acid) scaffolds for trabecular bone repair and regeneration: scaffold and native bone characterization. Connect. Tissue Res. 60, 274–282. doi:10.1080/03008207.2018.1499732
Verpoest, W., and Tournaye, H. (2009). ICSI: hype or hazard? Hum. Fertil. (Camb) 9, 81–92. doi:10.1080/14647270500422158
Waynforth, D. (2018). Effects of conception using assisted reproductive technologies on infant health and development: an evolutionary perspective and analysis using uk millennium cohort data. Yale J. Biol. Med. 91, 225–235.
Wydooghe, E., Heras, S., Dewulf, J., Piepers, S., Van Den Abbeel, E., De Sutter, P., et al. (2014). Replacing serum in culture medium with albumin and insulin, transferrin and selenium is the key to successful bovine embryo development in individual culture. Reprod. Fertil. Dev. 26, 717–724. doi:10.1071/RD13043
Wydooghe, E., Vandaele, L., Beek, J., Favoreel, H., Heindryckx, B., De Sutter, P., et al. (2011). Differential apoptotic staining of mammalian blastocysts based on double immunofluorescent CDX2 and active caspase-3 staining. Anal. Biochem. 416, 228–230. doi:10.1016/J.AB.2011.05.033
Xiao, S., Coppeta, J. R., Rogers, H. B., Isenberg, B. C., Zhu, J., Olalekan, S. A., et al. (2017). A microfluidic culture model of the human reproductive tract and 28-day menstrual cycle. Nat. Commun. 8, 14584. doi:10.1038/ncomms14584
Zhang, Q., Bei, H. P., Zhao, M., Dong, Z., and Zhao, X. (2022a). Shedding light on 3D printing: printing photo-crosslinkable constructs for tissue engineering. Biomaterials 286, 121566. doi:10.1016/j.biomaterials.2022.121566
Keywords: IVF, bovine, embryo culture, biomaterials, PCL, PEGDA, PLA
Citation: Belda-Perez R, Heras S, Cimini C, Romero-Aguirregomezcorta J, Valbonetti L, Colosimo A, Colosimo BM, Santoni S, Barboni B, Bernabò N and Coy P (2023) Advancing bovine in vitro fertilization through 3D printing: the effect of the 3D printed materials. Front. Bioeng. Biotechnol. 11:1260886. doi: 10.3389/fbioe.2023.1260886
Received: 18 July 2023; Accepted: 15 September 2023;
Published: 19 October 2023.
Edited by:
Reza Hedayati, Delft University of Technology, NetherlandsReviewed by:
Mozhgan Keshavarz, University of California, Irvine, United StatesAnna Lange-Consiglio, University of Milan, Italy
Copyright © 2023 Belda-Perez, Heras, Cimini, Romero-Aguirregomezcorta, Valbonetti, Colosimo, Colosimo, Santoni, Barboni, Bernabò and Coy. This is an open-access article distributed under the terms of the Creative Commons Attribution License (CC BY). The use, distribution or reproduction in other forums is permitted, provided the original author(s) and the copyright owner(s) are credited and that the original publication in this journal is cited, in accordance with accepted academic practice. No use, distribution or reproduction is permitted which does not comply with these terms.
*Correspondence: Pilar Coy, cGNveUB1bS5lcw==