- 1Shanxi Medical University School and Hospital of Stomatology, Taiyuan, China
- 2State Key Laboratory of Coal Conversion, Institute of Coal Chemistry, Chinese Academy of Sciences, Taiyuan, China
- 3Department of Stomatology, The First Hospital of Shanxi Medical University, Taiyuan, China
Objective: Titanium implants are widely used in surgeries for their biocompatibility and mechanical properties. However, excessive titanium particle release can cause implant failure. This study explores Atomic Layer Deposition (ALD) to coat commercially pure titanium (Cp-Ti) with TiO2, aiming to improve its frictional and corrosion resistance while reducing particle release. By comparing TiO2 films with varying ALD cycle numbers, we assess surface properties, particle release, friction, and corrosion performance, providing insights into mitigating particle release from implants.
Methods: Cp-Ti surfaces were prepared and coated with TiO2 films of 100, 300, and 500 ALD cycles. Surface characterization involved SEM, EDX, and XRD. Friction was tested using SEM, nanoindentation, and ICP-MS. Corrosion resistance was evaluated through immersion tests and electrochemical analysis. Cytotoxicity was assessed using BMSCs.
Results: Surface characterization revealed smoother surfaces with increased ALD cycles, confirming successful TiO2 deposition. Friction testing showed reduced friction coefficients with higher ALD cycles, supported by nanoindentation results. Corrosion resistance improved with increasing ALD cycles, as evidenced by electrochemical tests and reduced titanium release. Cytotoxicity studies showed no significant cytotoxic effects.
Conclusion: ALD-coated TiO2 films significantly enhance frictional and corrosion resistance of titanium implants while reducing particle release. The study underscores the importance of ALD cycle numbers in optimizing film performance, offering insights for designing implants with improved properties.
1 Introduction
Commercially pure titanium (Cp-Ti) has been acknowledged as a premier implant material with bio-safety and nearly 1,000 tons of titanium is used annually in clinical applications in diverse applications, such as artificial joints, dental implants and heart valves (Xu et al., 2020). However, despite the excellent performance, many cases of implant Ti failure still exist. The failure rate of dental implants has been reported to be about 1%–20% (Alves et al., 2017), and more than 35% for orthopedic implants (Tobin, 2017). In 75% of these cases, implant failure was due to aseptic loosening and impaired implant fixation (Awad et al., 2022). It has been shown that titanium implants in bone can continuously release titanium species (He et al., 2016), and the release of titanium ions and titanium particles can contribute to peri-implantitis and aseptic implant loosening (Eger et al., 2017). The released Ti species are not confined to the periphery of the implant, and even migrate with the blood and progressively accumulate in distal organs (Heringa et al., 2018), which leads to systemic hypersensitivity and allergic reactions. Released Ti particles are not limited to the periphery of the implant; they can migrate with the bloodstream and gradually accumulate in distant organs. In most studies, Ti nanoparticles have been found to cause oxidative stress, tissue pathology changes, carcinogenesis, genetic toxicity, and immune disruption (Shakeel et al., 2016). Worryingly despite the reported importance of excessive titanium species release on implant success and systemic health, the problem is routinely ignored by clinicians, patients and implant manufacturers, lacking sufficient attention and preventive measures.
Mechanical friction and corrosion are the main reasons for the physical degradation of titanium implants (Mombelli et al., 2018). Mechanical friction and corrosion are the primary factors leading to the degradation of titanium implants. Even within the bone-encased portions of the implant, frictional corrosion still occurs. This phenomenon arises from minute relative vibrations between two surfaces (implant surface and bone surface) under mechanical loads (friction), leading to irreversible corrosion degradation of the implant through electrochemical interactions with the surrounding environment (Berbel et al., 2019; Prestat and Thierry, 2021). Generally, increasing the strength of Ti and Ti alloys against friction and corrosion suppresses the mechanical friction to titanium particles. On the other hand, corrosion originates from the surface chemical reaction of metallic Ti in acidic environments. Although TiO2 films formed spontaneously on Ti surfaces in contact with oxygen are resistant to corrosion when in contact with acids and reactive substances (Peron et al., 2021). However, the growth of the oxide layer is a dynamic equilibrium process involving oxidation and reduction reactions. Under certain conditions, the growth rate of the TiO2 layer may be slow or non-uniform, resulting in an unstable layer quality that is easily damaged by external factors (Lara Rodriguez et al., 2014).
Surface overcoating has attracted extensive interest from researchers in recent years due to its ability to maintain the surface morphology of the implant and to be matched to the mechanical properties. There are various coating techniques available for implant modification. Such as anodic oxidation (Fazel et al., 2018), pulsed laser deposition (PLD) (Gnanavel et al., 2018), and physical vapor deposition (PVD) (Esmaeili et al., 2017), have been implemented to improve corrosion resistance. Forming anodic oxide coatings requires precise control of multiple process parameters such as electrolyte composition, temperature, and current density (Leinenbach and Eifler, 2009) and, therefore, requires complex process control and operation techniques with poor reliability and stability. The PLD technique is subject to effects such as phase explosion, which causes large particle sputtering, thus reducing the film quality and generating localized high-temperature effects on the titanium surface, which may lead to lattice structure alterations and thermal stresses in the titanium material, which may impact the properties and structure of the material (Deng et al., 2021). In addition, the coating thickness of PVD deposition is inhomogeneous on the surface of complex-shaped implants (Mohseni et al., 2014). This could lead to delamination of the substrate with the thinner coating, and the local oxide film will break if a critical strain is reached. It is still necessary to develop implant modification technique that can achieve uniform deposition at low temperatures and is easy to handle.
Atomic layer deposition (ALD) has unique advantages in sub-nanometer film thickness and uniformity control with increasing applications in biomedical advanced materials (Yang, 2020). Atomic Layer Deposition (ALD) is a precise thin-film deposition technique that enables highly accurate control over film thickness and composition by depositing films atom by atom on the material surface. During the reaction process, precursor molecules adhere to the substrate surface in a “self-limiting” manner, forming a single-molecule layer. Once the substrate surface is fully covered, excess precursor molecules no longer adsorb, resulting in the automatic termination of the deposition process. This means that regardless of the quantity of precursor molecules provided, only enough molecules can adsorb and participate in the reaction. This ensures the uniformity and consistency of the film. Compared to PCD, ALD is based on surface self-limiting and self-saturating adsorption reactions (Kim et al., 2023), which enables precise deposition at the atomic level on the complex surface of implants (Blendinger et al., 2021), and one study reported that the thickness of the film deposited per cycle on the implant surface is only 0.097 nm (Yang et al., 2017). The uniformity and consistency of ALD film avoid problems such as surface inhomogeneity and thickness variation. Compared with PLD, which usually operates at 500°C–800°C, ALD can even be conducted at a low temperature of 100°C (Wree et al., 2023), avoiding heat damage and shape changes to the implant material and maintaining the integrity and structural stability of the implant. The homogeneous films deposited by ALD are stable in properties; consequently, ALD-deposited TiO2 films have the potential to be used for implant surface coatings (Hashemi Astaneh et al., 2021).
Herein, we have used ALD technology to overcoat the titanium surface with stable TiO2 film to enhance its frictional corrosion resistance and reduce the release of titanium particles (Figure 1). To our knowledge, this is the first study of the utilization of ALD TiO2 films to solve the problem of titanium particle release from implants. The simplicity of the ALD technique, the lower working temperature, and the uniformity and stability of the films compared to other deposition techniques make it a viable solution. In addition, to further evaluate the friction and corrosion resistance of the films with different cycles and the particle release after friction and corrosion, we deposited 100, 300, and 500 cycles of TiO2 films on Cp-Ti using the ALD technique. Through this study, we intended to provide a feasible solution to the problem of implant particle release, demonstrating that the ALD modification technique has tremendous potential in implant research to enhance the performance and long-term success of titanium implants, providing a new direction and opportunity for research to reduce titanium particle release from implants.
2 Materials and methods
2.1 Sample preparation and thin film deposition
A commercial pure Ti (CP-Ti) plate was utilized as a surrogate for titanium implants in this study. CP-Ti (10 mm × 10 mm × 5 mm) was ground sequentially with silicon carbide sandpaper of different particle sizes (600, 800 and 1200), and then cleaned sequentially with acetone and ethanol by ultrasonication for 15 min. The ALD process was carried out in a homemade closed-chamber type ALD reactor. High-purity N2 (99.999%) was utilized as a carrier gas.
The selection of Titanium tetraisopropoxide (TTIP) and ultrapure water as precursors offers advantages such as usability, affordability, chemical stability, availability, low maintenance, safety, and a lower deposition temperature range (150°C–300°C) (Niemelä et al., 2017). Deposition at 160°C prevents thermal damage to the substrate material at high temperatures, which holds significant importance for the structure and applications of titanium implants. TTIP and water were kept at 80°C and 25°C, respectively. The pulse, exposure, and purge times for titanium tetraisopropoxide were 1, 8, and 20 s, and those for H2O were 0.1, 8, and 25 s, respectively. To explore the role of different number of cycles on particle release, we deposited 100, 300, and 500 cycles of ALD layers.
2.2 Surface characterization
Scanning Electron Microscopy (SEM) using the JSM-7900F was employed to meticulously observe the surface morphology of thin films, providing crucial insights into the microstructure and surface features of different cyclically deposited films. Additionally, Focused Ion Beam (FIB) was used to cut samples for Transmission Electron Microscopy (TEM), enabling the measurement of nanofilm thickness and cross-sectional elemental distribution.
Energy-Dispersive X-ray Spectroscopy (EDX) was utilized to quantitatively analyze the elemental composition of the films, helping determine whether variations in titanium-to-oxygen ratios exist across different cycles. The combination of SEM, FIB-TEM, and EDX provides a comprehensive understanding of the morphology and elemental distribution of TiO2 coatings.
Research findings indicate that rutile-type TiO2 nanoparticles cause more significant damage to murine bone tissue compared to anatase-type TiO2 nanoparticles (Cheng et al., 2021). By comparing measured XRD patterns with standard reference cards in databases, the phase structure can be accurately determined. Therefore, we employed a PANalytical X’Pert Pro X-ray Diffractometer (XRD) to examine the film’s structure within an angular range of 20°–60°.
2.3 Friction tests
In the oral environment, dental implants are exposed to various components present in saliva, including organic substances, dissolved oxygen, inorganic anions (such as Cl−, HPO42-, HCO3-), and cations (such as Na+, K+, Ca2+, Mg2+), as well as amino acids and proteins. These constituents may contribute to the degradation of the TiO2 layer (Ossowska and Zieliński, 2020). To study oral implants, we conducted friction-corrosion tests simulating the oral microenvironment, also known as bio-tribocorrosion. In these experiments, we selected artificial saliva as the medium to better understand the performance and durability of titanium-based implants and prostheses in the oral environment.
According to ASTM G119-93 (ASTM, 1993), a material surface property tester (MFT-4000, Huahui, China) was used to perform the friction corrosion test under artificial saliva immersion. In this study, GCr15 steel balls were used as the friction counterpart because the hardness of GCr15 bearing steel can reach HRC 58–63 after heat treatment, which is much higher than that of titanium alloy (HRC 28–33) which would not cause plastic deformation of the friction counterpart leading to wear and thus affect the experimental results during the tribocorrosion experiments (Zhou et al., 2019). A normal force of 5 N was applied to the counterpart, using the Hertzian contact stress model, corresponding to a maximum contact pressure of about 950 MPa, while the radius of the wear track was set to 5 mm, the speed of the sample table was set to 200 mm/min, and the test time was 10 min.
Two different methods were employed to assess the frictional performance. Scratch experiments were conducted using an ultra-nanoindenter with a diamond probe featuring a radius of 1 µm and an angle of 60°. The experiments were performed under a constant load of 500 μN, with a scratch distance of 10 µm and a duration of 1 min.
The surfaces of the four sample groups after friction were examined for surface scratches and particle detachment using SEM. In the presence of titanium nanoparticles in the sample, the distribution of elements no longer exhibits uniformity but appears as discrete atomic clusters. This non-uniformity hinders accurate quantitative analysis. Addressing this challenge, we employed the high-sensitivity ICP-MS (Inductively Coupled Plasma Mass Spectrometry) technique, specifically designed for elemental analysis in samples (Laborda et al., 2014). In this experiment, we collected titanium nanoparticles from artificial saliva dissolved in nitric acid and utilized ICP-MS for precise quantitative and statistical analysis of titanium content in the solution. To gain a more detailed insight into the morphology and size of titanium particles, we further employed SEM.
2.4 Corrosion tests
2.4.1 Corrosion resistance test
Infection, medication, dietary factors, periodontal disease, smoking, and systemic illnesses can potentially decrease the pH of normal saliva from 6.3–7.0 to <6.0 (Licausi et al., 2013). Under acidic conditions, the oxide layer on implant surfaces may be compromised, resulting in surface erosion and eventual release of titanium particles in the absence of wear. Commonly used oral medications, such as citric acid, tetracycline, and sodium fluoride, can lower the solution’s pH to <3 (Wheelis et al., 2016), causing rapid breakdown of the implant surface oxide layer and release of Ti particles. Therefore, this experiment simulated different acidic environments in the oral cavity using artificial saliva with pH values of 2.5, 5.5, and 7.0.
Each set of samples was immersed in 2 mL artificial saliva with different pH values, at 37°C for 7 days. SEM was utilized to compare the corrosion status of the samples before and after immersion, and EDX was employed to detect elemental changes on the corroded surface. To assess titanium release in highly acidic conditions, artificial saliva with a pH of 2.5 was collected, and the titanium content was quantified using ICP-MS, followed by statistical analysis. TEM was employed to investigate the morphology and size of particles that might exist in the solution.
2.4.2 Electrochemical tests
The electrochemical workstation (CHI6600E, Chinstruments, China) was used to perform the electrochemical tests on four sets of samples, respectively. A three-electrode setup was used, with the sample as the working electrode, the saturated glyceryl electrode as the reference electrode, and the platinum plate electrode as the counter electrode. Artificial saliva at pH 6.5°C and 37°C was used as the electrolyte. All tests were performed in a simulated oral environment at 37°C ± 1°C. Electrochemical tests were performed according to ASTM-G61 standard. The first step was system stabilization, and after 3600s of open circuit potential (OCP) monitoring, dynamic potential polarization tests and electrochemical impedance spectroscopy (EIS) tests were performed at a stable OCP. EIS tests were performed in the frequency range of 100 kHz-0.005 Hz, and Nyquist plots were plotted using EIS data. The dynamic potential polarization test was performed at a scan rate of 0.5 mV/s, and the corrosion potential (Ecorr) and the corresponding current density (Icorr) were estimated using Tafel curves. The measurements were repeated three times for each condition.
2.5 Cytotoxic studies
2.5.1 Cell cultures
Biocompatibility tests were performed according to ISO 7405–2018 (ISO, 2018). The cells used in this test were bone marrow mesenchymal stem cells (BMSCs)Cultivate under conditions of 37°C, 5% CO2, and saturated humidity until cells reach 70%–80% growth. Perform passage using 0.25% trypsin. Cells cultivated up until passage 3 (P3) then ready for experimental use. The titanium samples were placed in complete medium and in a 37°C for 72 h to obtain the extracts for the cell experiments.
2.5.2 Fluorescent staining
Cells at a density of 1×105 cells/mL were incubated in confocal dishes for 24 h. Cells were seen to adhere to the wall under an inverted microscope, the original culture medium was discarded, an immersion fluid exchange was performed, and the plates were incubated in an incubator for 24 h. And then rinsed three times with PBS. Add 150 μL of staining working solution A, incubate for 30 min at 37°C protected from light, rinse three times with PBS, add 150 μL of staining working solution B, and incubate for 20 min at 37°C protected from light. Observe the cell morphology at 488 nm under a laser confocal microscope (FV3000, Olympus, Japan).
2.5.3 LDH tests
The cytotoxicity of the samples was assessed by quantifying the release of lactate dehydrogenase (LDH) from BMSCs. Following cultivation, cells were seeded into a 96-well plate and treated with different material extracts. After 12 h of incubation, LDH release reagent was added, and the cells were incubated for an additional hour. The cell culture plate was then centrifuged at 400g for 5 min using a multi-well plate centrifuge. Supernatant (120 µL) from each well was transferred to a new 96-well plate, and absorbance was measured at 490 nm.
2.6 Statistical analysis
OriginPro 8.5 and Graphpad Prism9 software have been used for all graphical and statistical analyses. All experiments were conducted three times, except for the LDH assay, which was performed four times. All repeated measurements were expressed as standard deviation (SD). The statistical significance of the data was estimated using a one-way analysis of variance (ANOVA). p < 0.05 was considered statistically significant for significance levels, * for p < 0.05 and ** for p < 0.01.
3 Results
3.1 Surface characterization
The surface morphologies of the modified samples are shown in Figure 2. The SEM results (Figures 2A–D) showed that the surface of the TiO2 films generated by ALD was significantly smoother as the number of cycles increased. Since the thinness of TiO2 nanofilm increases with the ALD cycle number, a thick enough TiO2 film improves the surface smoothness. TEM was employed to examine the 300-cycle group, and the results are depicted in Figures 2E, F. The nanofilm exhibited a thickness of 26.7 nm, with an average TiO2 film thickness of 0.089 nm per cycle. Figure 2F illustrates the even distribution of titanium and oxygen elements across the cross-section of the film. The upper layer, containing platinum for detection purposes, is clearly distinguished from the lower layer, representing the pure titanium substrate without additional elements.
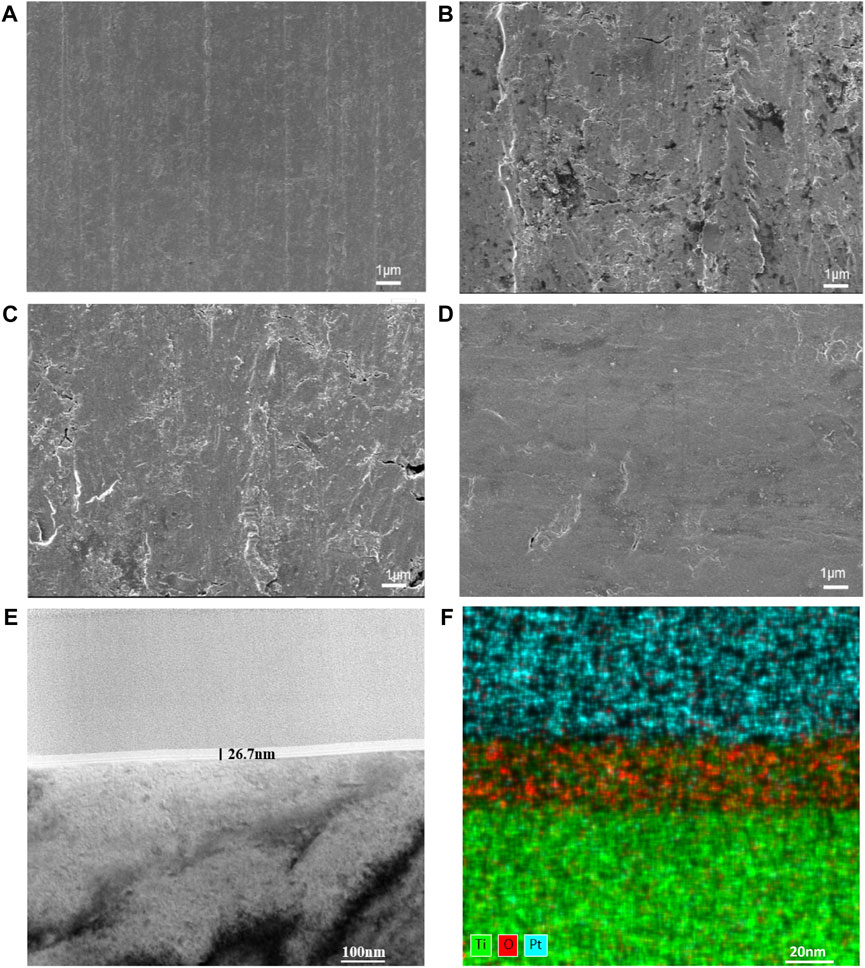
Figure 2. Surface morphologies of CP-Ti with different ALD cycles. (A) CP-Ti, (B) 100ALD-Ti, (C) 300ALD-Ti and (D) 500ALD-Ti of SEM. (E) TEM and (F) mapping for 300ALD-Ti.
The EDX spectra (Figures 3A–D) show the elemental distribution of titanium and oxygen on the surfaces of each group. Table 1 and Figure 3E indicates the titanium and oxygen content of the sample surface. The increase of oxygen ratio on the surface suggests the covering of TiO2 on the Ti surface. Since the oxygen content is not changed after 300 cycles of TiO2, a uniform overcoating is realized. The XRD spectra of the samples are in Figure 3F. All diffraction peaks are in good agreement with the standard JCPDS data. The anatase TiO2 films had strong diffraction peaks at 2θ = 25.4° and 48.0°, while none of the three groups of ALD-Ti saw this diffraction peak, indicating that the increase in the number of cycles at the deposition temperature of 160°C did not change the crystalline shape of TiO2.
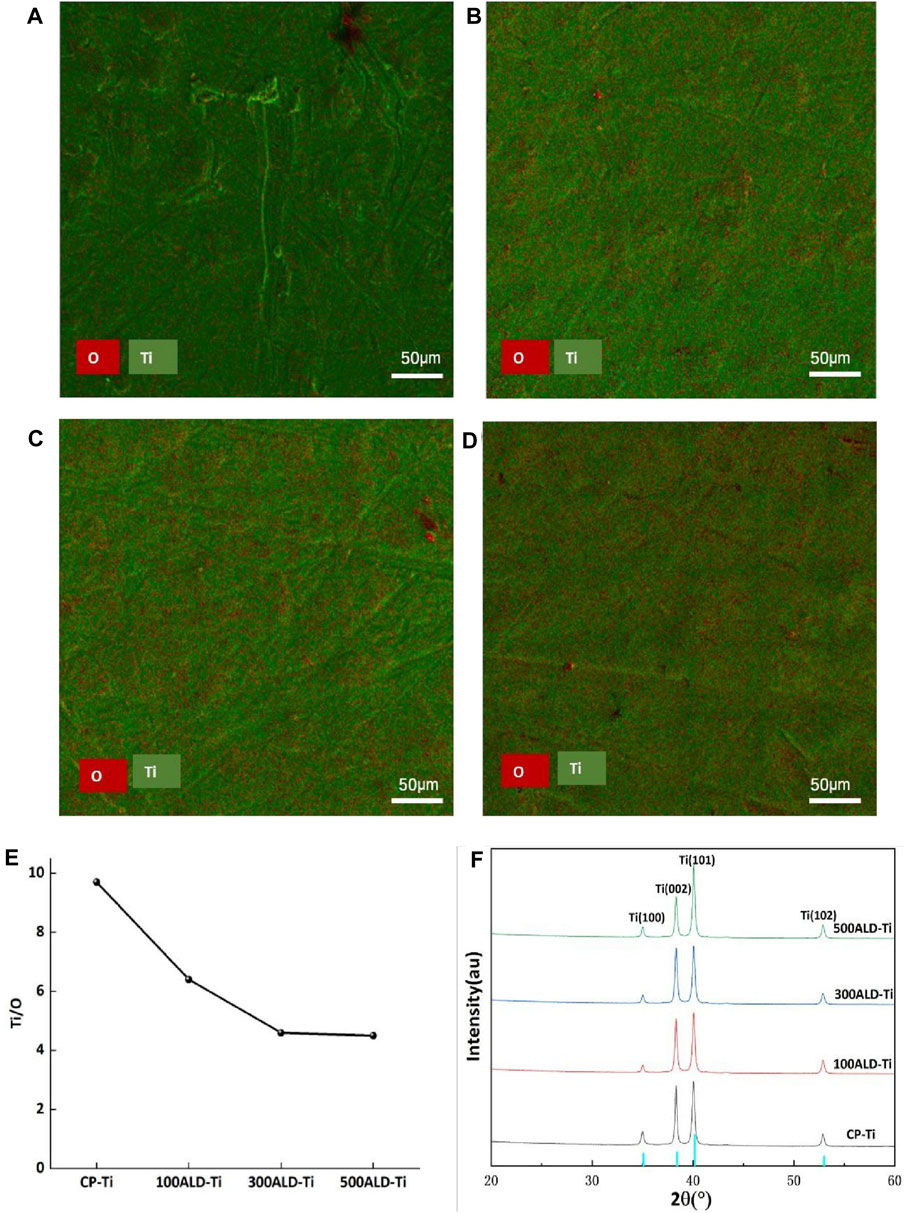
Figure 3. Phase composition and elemental distribution. (A) CP-Ti, (B) 100ALD-Ti, (C) 300ALD-Ti and (D) 500ALD-Ti is the mapping diagram after EDX, with O in red and Ti in green. (E) Titanium oxygen ratio by EDX spectra. (F) XRD patterns. The blue color of the horizontal axis indicates Amorphous TiO2 type.
3.2 Friction tests
The surface morphology of the sample after friction is shown in Figures 4A–D. It is evident that all four groups showed friction marks on the surface after friction. However, as the number of cycles increased, the friction marks became shallower, with the 500 ALD-Ti exhibiting the shallowest marks among the four groups.
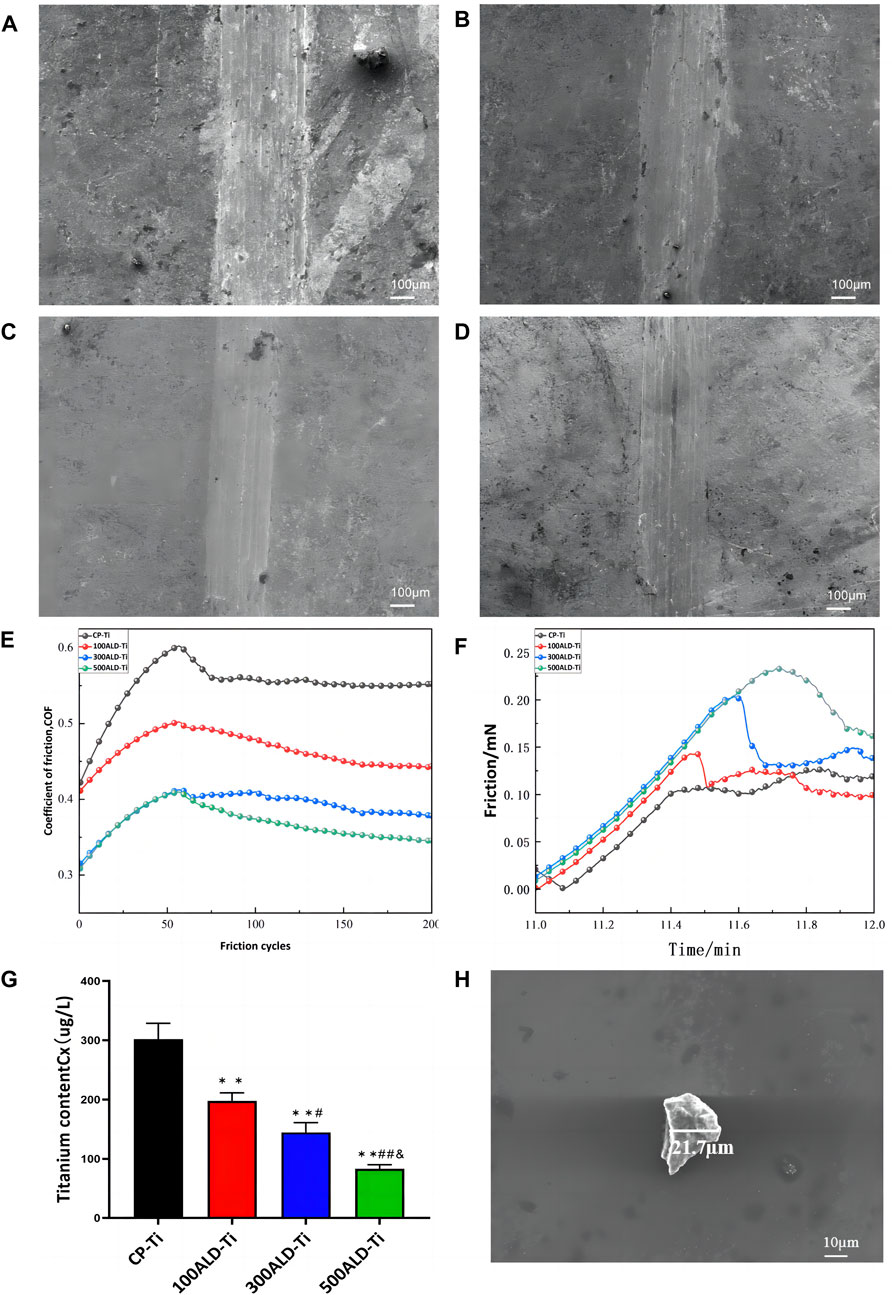
Figure 4. Friction performance. SEM images of (A) CP-Ti, (B) 100ALD-Ti, (C) 300ALD-Ti and (D) 500ALD-Ti. (E) Influence of different deposition cycles on coefficient of friction. (F) Friction analysis of nano scratch testing. (G) ICP-MS of post-friction solutions. The results represent the average of three experiments. (n = 3, *p < 0.05, **p < 0.001 compared with the CP-Ti group; #p < 0.05, ##p < 0.001 compared with the 100ALD-Ti group; and p < 0.001 compared with the 300ALD-Ti group). (H) SEM of particles in solution.
To facilitate the observation of trends in friction resistance, the friction tests data were smoothed and then plotted, and the relationship between the number of cycles and the coefficient of friction (COF) is illustrated in Figure 4E. Evidently, both the experimental and control groups displayed a progressive increase in friction coefficient followed by a tendency to stabilize. This pattern arises from initial challenges like surface roughness and uneven contact, resulting in a COF increase. As the friction surface adapts and readjusts, the COF gradually stabilizes. And COF is inversely proportional to the friction resistance (Matijošius et al., 2020), from the smooth segment in the graph. As the cycle increases, COF becomes smaller, indicating that the friction resistance increases. The nanoscratch results, as shown in Figure 4F, indicate that the titanium dioxide nanofilm enhances the friction performance, with an improvement observed as the number of cycles increases.
To quantify the titanium species shed from the sample surface into the solution, the solution was acid dissolved and subjected to ICP-MS. The test results are summarized in Figure 4G. Analysis of the data showed that the release of titanium particles was significantly reduced in each experimental group compared to the control group (p < 0.001), indicating that the ALD-deposited TiO2 coating was able to substantially reduce the release of titanium particles from the implant surface. The reduction in titanium particle release with increasing number of cycles was also observed between the experimental groups (p < 0.05), which suggests that the improvement of titanium particle release by the coating is closely related to the number of ALD cycles. SEM (Figure 4H) was employed to determine the size of residual particles in the immersion liquid. A total of 20 particles were identified and measured in the liquid. The particle sizes were predominantly in the micrometer range, exhibiting diverse shapes and forms.
3.3 Corrosion tests
3.3.1 Corrosion resistance test
Figures 5A–D show the SEM comparisons before and after corrosion. As can be seen from the figures, there is no change in the four groups of samples before and after corrosion at a PH of 7.0 and 5.5. 100ALD-Ti corrosion traces are more obvious at a PH of 2.5, followed by the 300ALD-Ti. While the 500ALD-Ti and CP-Ti groups had almost no corrosion. This indicates that the corrosion resistance increases with the increase in the number of ALD cycles. The basically unchanged morphology of the CP-Ti before and after corrosion is due to the uniform distribution of the CP-Ti surface and the consistent corrosion resistance, which results in no significant change in the overall surface structure before and after corrosion.
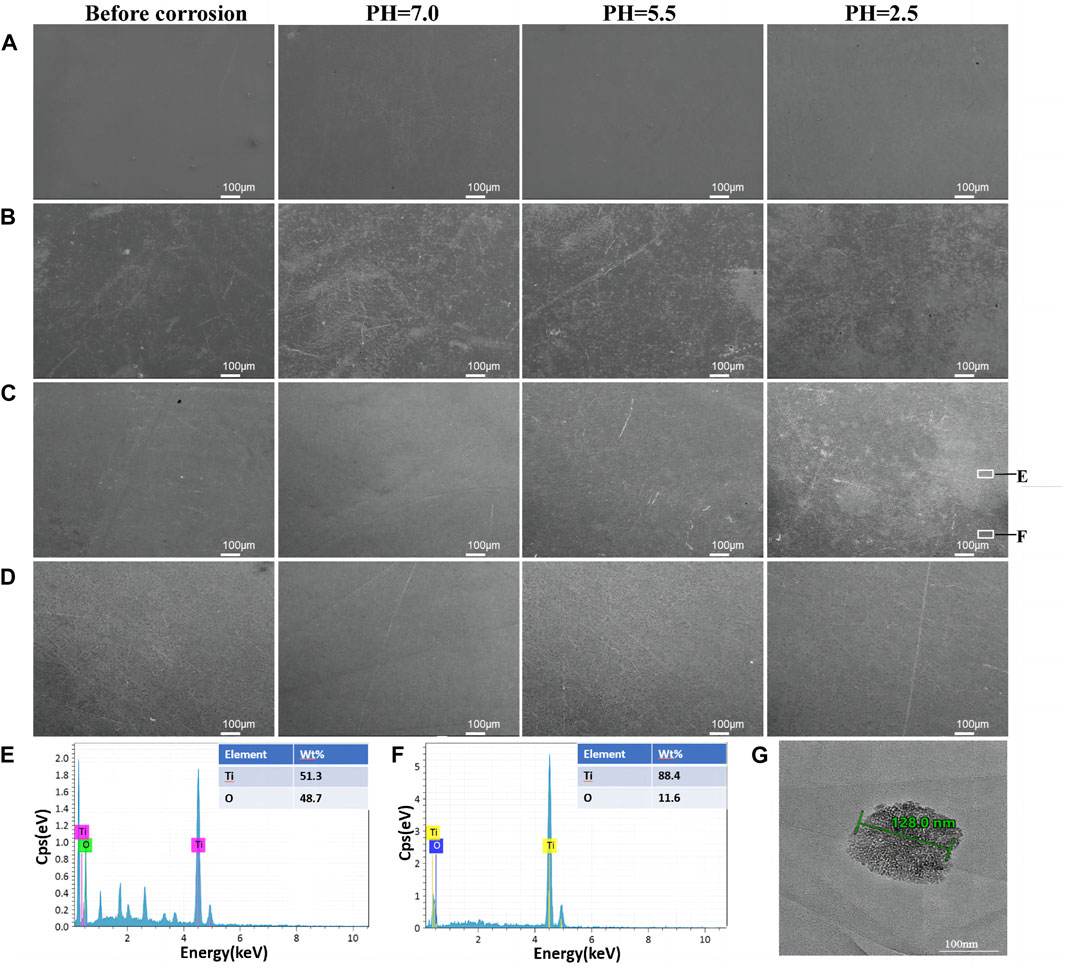
Figure 5. Surface morphology after corrosion. SEM images of (A)CP-Ti, (B) 100ALD-Ti, (C) 300ALD-Ti and (D) 500ALD-Ti. (E,F) correspond to the EDX spectra of the white boxed region in the last image (C). (G) TEM image of Ti particles.
Further EDX analysis of the corroded samples shows that the darker areas in Figure 5C image have a low titanium-to-oxygen ratio (Figure 5E), which corresponds to the TiO2 coating. While the brighter region has a high titanium-to-oxygen ratio (Figure 5F), which corresponds to a pure titanium substrate. This suggests that when the titanium implant comes into contact with an acidic environment, the TiO2 coating is the first to be corroded, providing protection for the underlying titanium. In addition, as the number of ALD cycles increases, the coatings become thicker, exhibit better corrosion resistance, and provide stronger protection for the titanium. The TEM results (Figure 5G) revealed the presence of nanoscale titanium particles in the solution, exhibiting diverse morphologies and shapes.
To determine the titanium ions released from the sample surface into the solution, artificial saliva at a pH of 2.5 was collected and subjected to ICP-MS testing. The results, shown in Figure 6A, revealed that compared to the control group, all experimental groups exhibited a decrease in titanium content (p < 0.05). However, in comparison to the other two sample groups, the 500ALD-Ti exhibited a significant reduction in titanium ion release (p < 0.001). This finding is consistent with the SEM images, indicating that the decrease in titanium content in the experimental groups may be attributed to the protective effect of the TiO2 coating on the underlying titanium substrate. The 500ALD-Ti, which had the thickest deposited TiO2 coating, demonstrated the strongest protective effect on the substrate, resulting in the least amount of titanium ion release.
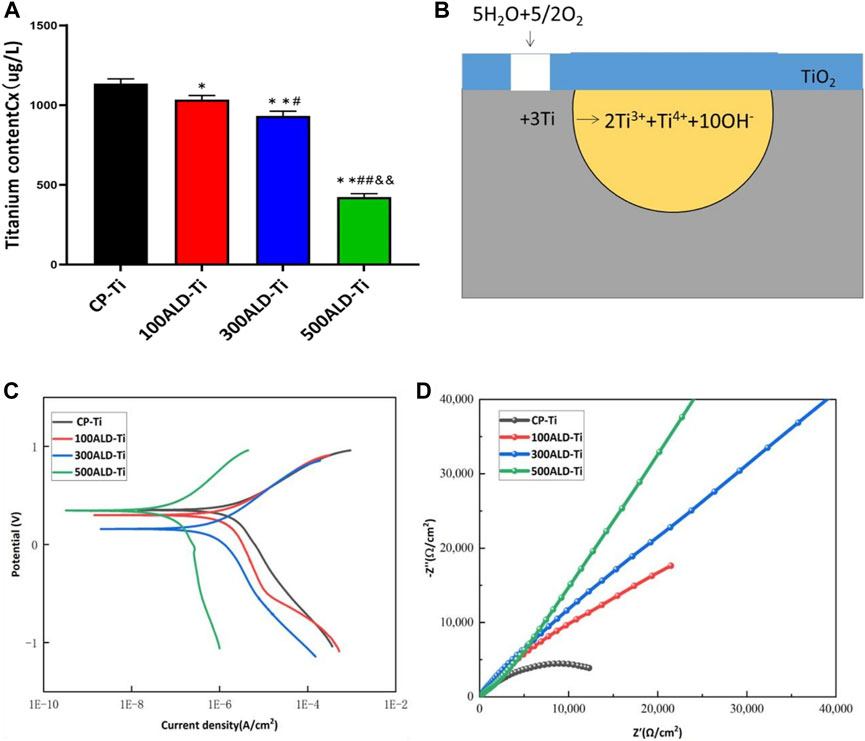
Figure 6. Corrosion performance. (A) ICP-MS of post-corrosion solution. The results represent the average of three experiments. (n = 3, *p < 0.05, **p < 0.001 compared with the CP-Ti group; #p < 0.05, ##p < 0.001 compared with the 100ALD-Ti group; and&p < 0.001 compared with the 300ALD-Ti group). (B) The reaction route for titanium corrosion. (C) Potentiodynamic polarization (Tafel) plots and (D) Nyquist plots for electrochemical corrosion.
3.3.2 Electrochemical tests
Figure 6B depicts the typical electrochemical reactions that may occur during the corrosion process of uncoated titanium implants without protective coatings. In this process, water molecules play a role in providing hydroxide ions (OH−) while oxygen acts as the oxidizing agent. In such a corrosive environment, titanium metal is oxidized into various oxidation states, releasing electrons. It is essential to note that this is just a simplified electrochemical reaction equation representing one possible aspect of the complex process of biocorrosion. The actual scenario is influenced by various factors such as the composition of oral fluids, temperature, pH levels, among others. Therefore, this simplified reaction equation only represents a potential aspect of the corrosion process.
Figure 6C reports the dynamic potential polarization curves of the experimental and control groups. The corrosion potentials of the samples in the experimental group were all shifted to the left compared to the control group. This may indicate a positive effect of the coating on the corrosion resistance of CP-Ti. According to the Tafel equation, the average values of corrosion potential (Ecorr) and corrosion current density (Icorr) are shown in Table 2. The lower the corrosion current density, the lower the corrosion rate. The higher the corrosion potential in the case of similar currents, the lower the corrosion tendency, it can be observed that the corrosion current of experimental group are lower than the control group, indicating that the presence of TiO2 coating increases the corrosion resistance of the material. And the number of cycles increased corrosion resistance also increased, 500ALD-Ti shows the best ability of corrosion resistance.
Figure 6D reveals the Nyquist (real vs. imaginary impedance) plots used to study the EIS data. The Nyquist plot reveals a significant increase in capacitance loop for the experimental group compared to the control group. A larger capacitance loop diameter correlates with higher corrosion resistance. This indicates that the 500ALD-Ti coating has the highest corrosion resistance and is considerably higher than the control group. 100ALD-Ti has the smallest semicircular ring and therefore has the lowest corrosion resistance in the experimental group. However, 100ALD-Ti still has corrosion resistance compared to the control group.
3.4 Cytotoxic studies
Because BMSCs are sensitive to external environmental changes and materials, and can reflect the effect of implant modification materials on cell activity and function, BMSCs are involved in the bone tissue regeneration and repair process in vivo, which is important for implant compatibility and bioactivity, BMSCs were selected for this test. To analyze the cytotoxicity, confocal microscopy experiments were performed using both xanthophyll-AM and propidium iodide (PI) solutions, which were used to stain live and dead cells, respectively, and the results are displayed in Figures 7A–D. There were fewer dead cells in both the control and experimental groups.
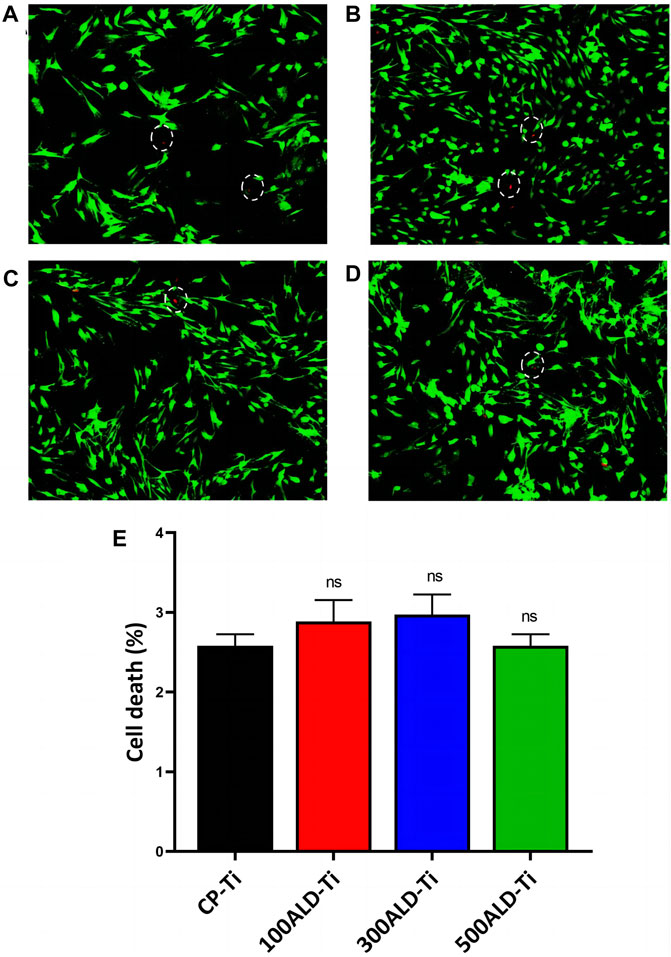
Figure 7. Cytotoxic assays. Fluorescence images after live/dead staining of bone marrow mesenchymal stem cells (BMSC) after culturing for 48 h (A) CP-Ti (B) 100ALD-Ti (C) 300ALD-Ti and (D) 500ALD-Ti. (E) LDH assay of BMSC. The results represent the average of three experiments. (n = 4, ns mean p > 0.05).
In LDH test on BMSCs indicated that both pure titanium and ALD-deposited TiO2 films were not cytotoxic and were not correlated with the number of cycles. The results of the cytotoxicity test are shown in Figure 7E. Compared to the CP-Ti group, the cell death rates in all other groups did not show a statistically significant increase.
4 Discussion
Implant surface degradation in the human body is caused by both friction (resulting in mechanical particle breakdown) and corrosion (leading to chemical degradation of soluble metal ions) (Delgado-Ruiz and Romanos, 2018). Titanium implants with TiO2 coatings exhibit strong resistance to friction and corrosion (Matijošius et al., 2020). To address the issue of particle release due to friction and corrosion of these implants, this study deposited thin TiO2 films using the ALD technique to create protective layers. ALD’s step-by-step deposition process allows precise control at the atomic level, ensuring uniformity and accurate thickness control of the film (Kim et al., 2021).
The crystalline shape of TiO2 deposited by ALD is related to the deposition temperature (Jolivet et al., 2023). The XRD results show that TiO2 is an amorphous structure when the temperature is 160°C, consistent with literature reports (Liu et al., 2017). Moreover, the TiO2 of different cycles did not show anatase titanium dioxide, which indicates that the crystalline form is not related to the number of cycles but to the working temperature. Anatase TiO2 may be toxic to osteoblasts (Bernier et al., 2012). So the amorphous TiO2 films in this study can avoid the toxicity problem. Furthermore, different cycle counts of ALD have been reported in the literature to have an effect on the corrosion resistance of zirconia films (Yang et al., 2017), but there is little literature comparing the friction and corrosion resistance of TiO2 deposited on the surface of titanium implants with different ALD cycles. This study was therefore conducted with three gradients of cycle numbers to investigate the relationship between cycle numbers and friction and corrosion resistance.
In this study, we assessed how different ALD cycles affect titanium implant performance, specifically focusing on species release and friction corrosion resistance. We simulated intraoral conditions with artificial saliva at 37°C. We found that the TiO2 coating initially experienced wear and corrosion, but it effectively protected the underlying titanium substrate. Thicker TiO2 coatings showed greater resistance to both wear and corrosion, providing better protection for the titanium implant.
ICP analysis of the immersion solution confirmed reduced ion levels, with the 500ALD-Ti group having significantly lower titanium ion concentrations compared to other groups. This aligns with our friction and corrosion results, confirming that the titanium dioxide film effectively limits the release of titanium species from the implant’s surface. Overall, increasing the number of ALD cycles enhances friction corrosion resistance and reduces titanium species release.
Despite the small thickness of TiO2 overcoating layers, they significantly contributed to the corrosion behavior of CP-Ti. The smaller corrosion current (Icorr) values of the coated samples obtained by fitting the dynamic potential polarization curves using Tafel curves indicate that the coatings effectively reduced the corrosion rate of Ti and protected its surface from electrochemical reactions. And the 500-cycle TiO2 films showed the smallest Icorr compared to the other two sets of coatings, a trend consistent with that reported in the literature (Kania et al., 2021). Moreover, Nyquist plots of impedance spectra showed that the experimental group had a larger semicircle diameter than the control group, while the 500-cycle group had the largest, which suggested that the films improved the insulating properties of the surface. In other words, the ALD films are better capacitors with a higher protection level than pure titanium. This may be due to the fact that the standard electrode potential of TiO2 is the same as that of titanium, which slows down the oxidation rate. This paper used three different methods to evaluate the corrosion resistance: dynamic polarization curves, EIS spectroscopy and immersion experiments. The results of the three methods are basically consistent. By increasing the number of ALD cycles of the TiO2 film, the corrosion resistance of the titanium surface can be significantly improved, the corrosion rate reduced, the corrosion area minimized, and the ions release reduced, providing an effective method for the corrosion protection of titanium materials in various applications.
It is worth mentioning that although the cell activity of the experimental group in the in vitro cell test was higher than that of the control group and increased with cycling, the cell activity of all four groups of samples was smaller than that of the blank group, and there was no statistical difference between the groups. Therefore, the experimental results can only show that TiO2 is not cytotoxic, but cannot prove that the coating can increase cellular activity. This is inconsistent with the report of Liu et al. on the ability of TiO2 to increase cytocompatibility (Liu et al., 2017), which may be due to the fact that the number of cycles in the literature is 2500, while the number of cycles in this experiment is less, and the uncoated pure titanium can also show high biocompatibility and stimulate cell differentiation in the osteogenic direction, so the difference between the experimental group and the control group is smaller.
While Atomic Layer Deposition (ALD) technology demonstrates significant advantages in surface modification, it still encounters challenges in large-scale manufacturing. The layer-by-layer deposition process of ALD results in a relatively slow preparation speed, as each layer needs to go through a complete cycle. This limitation hampers the technology compared to other surface modification techniques. To address this issue, optimizing the reaction conditions of ALD to enhance the efficiency of each cycle and consequently increase the preparation speed could be considered. Another significant challenge is reducing the cost associated with ALD in manufacturing. The ALD process requires substantial energy for heating and vacuum, making optimization in throughput crucial (Zhuiykov et al., 2017). Adjusting the reactor to maximize precursor utilization and reducing precursor costs is a potential solution. Additionally, exploring more economical and readily available precursor materials can effectively alleviate cost pressures.
From the comprehensive experimental results, it is worth affirming that the ALD-deposited TiO2 coating can both enhance the frictional corrosion resistance of pure titanium and reduce the release of titanium species. In this experiment, three sets of gradient cycle times were set, so it is presumed that the increase of ALD cycle numbers has positively affects the reduction of species release. However, no conclusion has been reached on the optimal number of cycles for the friction resistance and corrosion performance; therefore, the optimal number of cycles for the friction resistance and corrosion performance will be further investigated. Furthermore, the relationship between the biocompatibility of ALD-TiO2 films and their resistance to frictional corrosion remains uncertain. To address this uncertainty, we plan to conduct additional biocompatibility experiments in subsequent studies, examining the biological performance of TiO2 films under different frictional corrosion conditions. Through these experiments, we aim to determine the optimal number of TiO2 film cycles to achieve the best performance in various aspects.
5 Conclusion
In this study, TiO2 films were prepared on the titanium surface by the ALD technique, which significantly improved the friction corrosion resistance of the material and thus reduced the release of titanium species. Three different cycle designs demonstrated that the increase in the number of ALD cycles significantly impacted the morphology and properties of the TiO2 films, significantly improving the friction corrosion resistance of the TiO2 films and reducing release of titanium species. This provides important guidance for designing and preparing titanium implants with more resistance to friction and corrosion. Future studies can further explore optimizing ALD parameters and selecting coating materials to achieve better friction corrosion resistance and biocompatibility.
Data availability statement
The original contributions presented in the study are included in the article/Supplementary material, further inquiries can be directed to the corresponding authors.
Ethics statement
The animal study was approved by the Ethics Committee of The School of Stomatology of Shanxi Medical University. The study was conducted in accordance with the local legislation and institutional requirements.
Author contributions
XZo: Conceptualization, Methodology, Writing–original draft. XZg: Conceptualization, Methodology, Writing–original draft. ZZ: Formal Analysis, Methodology, Writing–review and editing. FM: Formal Analysis, Writing–review and editing. RL: Formal Analysis, Investigation, Writing–review and editing. MZ: Formal Analysis, Investigation, Writing–review and editing. YH: Formal Analysis, Investigation, Writing–review and editing. QX: Formal Analysis, Investigation, Writing–review and editing. XS: Supervision, Writing–review and editing. BZ: Supervision, Writing–review and editing. XW: Conceptualization, Supervision, Validation, Writing–review and editing.
Funding
The author(s) declare financial support was received for the research, authorship, and/or publication of this article. This work was supported by National Natural Science Foundation of China (82071155, 82271023), the Project of Shanxi Province Key Laboratory of Oral Diseases Prevention and New Materials [grant number RC2021-02 and RC202301], the Shanxi Applied Basic Research Program Outstanding Youth Cultivation Project Fund [grant numbers 202203021223006], Graduate Education Innovation Project of Shanxi Province (2021Y432, 2023SJ170), and R&D Program of Beijing Municipal Education Commission (KM202010025013).
Conflict of interest
The authors declare that the research was conducted in the absence of any commercial or financial relationships that could be construed as a potential conflict of interest.
Publisher’s note
All claims expressed in this article are solely those of the authors and do not necessarily represent those of their affiliated organizations, or those of the publisher, the editors and the reviewers. Any product that may be evaluated in this article, or claim that may be made by its manufacturer, is not guaranteed or endorsed by the publisher.
References
Alves, S. A., Patel, S. B., Sukotjo, C., Mathew, M. T., Filho, P. N., Celis, J.-P., et al. (2017). Synthesis of calcium-phosphorous doped TiO2 nanotubes by anodization and reverse polarization: a promising strategy for an efficient biofunctional implant surface. Appl. Surf. Sci. 399, 682–701. doi:10.1016/j.apsusc.2016.12.105
Awad, K., Young, S., Aswath, P., and Varanasi, V. (2022). Interfacial adhesion and surface bioactivity of anodized titanium modified with SiON and SiONP surface coatings. Surf. Interfaces 28, 101645. doi:10.1016/j.surfin.2021.101645
Berbel, L. O., Banczek, E. D. P., Karoussis, I. K., Kotsakis, G. A., and Costa, I. (2019). Determinants of corrosion resistance of Ti-6Al-4V alloy dental implants in an in vitro model of peri-implant inflammation. PLoS One 14, e0210530. doi:10.1371/journal.pone.0210530
Bernier, M. C., El Kirat, K., Besse, M., Morandat, S., and Vayssade, M. (2012). Preosteoblasts and fibroblasts respond differently to anatase titanium dioxide nanoparticles: a cytotoxicity and inflammation study. Colloids Surf. B Biointerfaces 90, 68–74. doi:10.1016/j.colsurfb.2011.09.044
Blendinger, F., Seitz, D., Ottenschlager, A., Fleischer, M., and Bucher, V. (2021). Atomic layer deposition of bioactive TiO2 thin films on polyetheretherketone for orthopedic implants. ACS Appl. Mater Interfaces 13, 3536–3546. doi:10.1021/acsami.0c17990
Cheng, W., Xu, X., Lang, Y., Cheng, Z., Rizwan, M., Tang, X., et al. (2021). Anatase and rutile TiO2 nanoparticles lead effective bone damage in young rat model via the igf-1 signaling pathway. Int. J. Nanomedicine 16, 7233–7247. doi:10.2147/ijn.s333632
Delgado-Ruiz, R., and Romanos, G. (2018). Potential causes of titanium particle and ion release in implant dentistry: a systematic review. Int. J. Mol. Sci. 19, 3585. doi:10.3390/ijms19113585
Deng, Z., Jia, Q., Feng, B., and Liu, L. J. J. C. L. (2021). Research progress on fabrication and applications of high-performance films by pulsed laser deposition. J. Nanomater. 8, 0802010. doi:10.3788/cjl202148.0802010
Eger, M., Sterer, N., Liron, T., Kohavi, D., and Gabet, Y. (2017). Scaling of titanium implants entrains inflammation-induced osteolysis. Sci. Rep. 7, 39612. doi:10.1038/srep39612
Esmaeili, M. M., Mahmoodi, M., and Imani, R. J. I. (2017). Tantalum carbide coating on Ti-6Al-4V by electron beam physical vapor deposition method: study of corrosion and biocompatibility behavior. Int. J. Appl. Ceram. Technol. 14, 374–382. doi:10.1111/ijac.12658
Fazel, M., Salimijazi, H. R., and Shamanian, M. (2018). Improvement of corrosion and tribocorrosion behavior of pure titanium by subzero anodic spark oxidation. ACS Appl. Mater Interfaces 10, 15281–15287. doi:10.1021/acsami.8b02331
Gnanavel, S., Ponnusamy, S., Mohan, L., and Muthamizhchelvan, C. J. J. (2018). In vitro corrosion behaviour of Ti–6Al–4V and 316L stainless steel alloys for biomedical implant applications. J. Bio- Tribo-Corrosion 4, 1–8. doi:10.1007/s40735-017-0118-8
Hashemi Astaneh, S., Faverani, L. P., Sukotjo, C., and Takoudis, C. G. (2021). Atomic layer deposition on dental materials: processing conditions and surface functionalization to improve physical, chemical, and clinical properties - a review. Acta Biomater. 121, 103–118. doi:10.1016/j.actbio.2020.11.024
He, X., Reichl, F. X., Wang, Y., Michalke, B., Milz, S., Yang, Y., et al. (2016). Analysis of titanium and other metals in human jawbones with dental implants - a case series study. Dent. Mater 32, 1042–1051. doi:10.1016/j.dental.2016.05.012
Heringa, M. B., Peters, R. J. B., Bleys, R., van der Lee, M. K., Tromp, P. C., van Kesteren, P. C. E., et al. (2018). Detection of titanium particles in human liver and spleen and possible health implications. Part Fibre Toxicol. 15, 15. doi:10.1186/s12989-018-0251-7
Jolivet, A., Labbé, C., Frilay, C., Debieu, O., Marie, P., Horcholle, B., et al. (2023). Structural, optical, and electrical properties of TiO2 thin films deposited by ALD: impact of the substrate, the deposited thickness and the deposition temperature. Appl. Surf. Sci. 608, 155214. doi:10.1016/j.apsusc.2022.155214
Kania, A., Szindler, M. M., and Szindler, M. J. C. (2021). Structure and corrosion behavior of TiO2 thin films deposited by ALD on a biomedical magnesium alloy. Coatings 11, 70. doi:10.3390/coatings11010070
Kim, H.-M., Kim, D.-G., Kim, Y.-S., Kim, M., and Park, J. (2023). Atomic layer deposition for nanoscale oxide semiconductor thin film transistors: review and outlook. Int. J. Extreme Manuf. 5, 012006. doi:10.1088/2631-7990/acb46d
Kim, Y., Woo, W. J., Kim, D., Lee, S., Chung, S. M., Park, J., et al. (2021). Atomic-layer-deposition-based 2D transition metal chalcogenides: synthesis, modulation, and applications. Adv. Mater 33, e2005907. doi:10.1002/adma.202005907
Laborda, F., Bolea, E., and Jimenez-Lamana, J. (2014). Single particle inductively coupled plasma mass spectrometry: a powerful tool for nanoanalysis. ACS Publications 86 (5), 2270-2278. doi:10.1021/ac402980q
Lara Rodriguez, L., Sundaram, P. A., Rosim-Fachini, E., Padovani, A. M., and Diffoot-Carlo, N. (2014). Plasma electrolytic oxidation coatings on γTiAl alloy for potential biomedical applications. J. Biomed. Mater Res. B Appl. Biomater. 102, 988–1001. doi:10.1002/jbm.b.33079
Leinenbach, C., and Eifler, D. (2009). Influence of oxidation treatment on fatigue and fatigue-induced damage of commercially pure titanium. Acta Biomater. 5, 2810–2819. doi:10.1016/j.actbio.2009.03.029
Licausi, M.-P., Muñoz, A. I., and Borrás, V. (2013). Influence of the fabrication process and fluoride content on the tribocorrosion behaviour of Ti6Al4V biomedical alloy in artificial saliva. J. Mech. Behav. Biomed. Mater 20, 137–148. doi:10.1016/j.jmbbm.2013.01.019
Liu, L., Bhatia, R., and Webster, T. J. (2017). Atomic layer deposition of nano-TiO(2) thin films with enhanced biocompatibility and antimicrobial activity for orthopedic implants. Int. J. Nanomedicine 12, 8711–8723. doi:10.2147/IJN.S148065
Matijošius, T., Pivoriūnas, A., Čebatariūnienė, A., Tunaitis, V., Staišiūnas, L., Stalnionis, G., et al. (2020). Friction reduction using Nanothin Titanium layers on anodized aluminum as potential bioceramic material. Ceram. Int. 46, 15581–15593. doi:10.1016/j.ceramint.2020.03.105
Mohseni, E., Zalnezhad, E., and Bushroa, A. R. (2014). Comparative investigation on the adhesion of hydroxyapatite coating on Ti–6Al–4V implant: a review paper. Int. J. Adhesion Adhesives 48, 238–257. doi:10.1016/j.ijadhadh.2013.09.030
Mombelli, A., Hashim, D., and Cionca, N. (2018). What is the impact of titanium particles and biocorrosion on implant survival and complications? A critical review. Clin. Oral Implants Res. 29 (18), 37–53. doi:10.1111/clr.13305
Niemelä, J.-P., Marin, G., and Karppinen, M. J. S. (2017). Titanium dioxide thin films by atomic layer deposition: a review. A Rev. 32, 093005. doi:10.1088/1361-6641/aa78ce
Ossowska, A., and Zieliński, A. J. C. (2020). The mechanisms of degradation of titanium dental implants. Coatings 10, 836. doi:10.3390/coatings10090836
Peron, M., Cogo, S., Bjelland, M., Bin Afif, A., Dadlani, A., Greggio, E., et al. (2021). On the evaluation of ALD TiO2, ZrO2 and HfO2 coatings on corrosion and cytotoxicity performances. J. Magnesium Alloys 9, 1806–1819. doi:10.1016/j.jma.2021.03.010
Prestat, M., and Thierry, D. (2021). Corrosion of titanium under simulated inflammation conditions: clinical context and in vitro investigations. Acta Biomater. 136, 72–87. doi:10.1016/j.actbio.2021.10.002
Shakeel, M., Jabeen, F., Shabbir, S., Asghar, M. S., Khan, M. S., and Chaudhry, A. S. (2016). Toxicity of nano-titanium dioxide (TiO2-NP) through various routes of exposure: a review. a Rev. 172, 1–36. doi:10.1007/s12011-015-0550-x
Tobin, E. J. (2017). Recent coating developments for combination devices in orthopedic and dental applications: a literature review. Adv. Drug Deliv. Rev. 112, 88–100. doi:10.1016/j.addr.2017.01.007
Wheelis, S. E., Gindri, I. M., Valderrama, P., Wilson Jr, T. G., Huang, J., and Rodrigues, D. C. J. (2016). Effects of decontamination solutions on the surface of titanium: investigation of surface morphology, composition, and roughness. Clin. Oral Implants Res. 27, 329–340. doi:10.1111/clr.12545
Wree, J. L., Rogalla, D., Ostendorf, A., Schierbaum, K. D., and Devi, A. (2023). Plasma-Enhanced atomic layer deposition of molybdenum oxide thin films at low temperatures for hydrogen gas sensing. ACS Appl. Mater Interfaces. doi:10.1021/acsami.2c19827
Xu, J., Aoki, H., Kasugai, S., and Otsuka, M. (2020). Enhancement of mineralization on porous titanium surface by filling with nano-hydroxyapatite particles fabricated with a vacuum spray method. Mater Sci. Eng. C Mater Biol. Appl. 111, 110772. doi:10.1016/j.msec.2020.110772
Yang, B. (2020). Applications of titania atomic layer deposition in the biomedical field and recent updates. Am. J. Biomed. Sci. Res. 8, 465–468. doi:10.34297/ajbsr.2020.08.001321
Yang, Q., Yuan, W., Liu, X., Zheng, Y., Cui, Z., Yang, X., et al. (2017). Atomic layer deposited ZrO(2) nanofilm on Mg-Sr alloy for enhanced corrosion resistance and biocompatibility. Acta Biomater. 58, 515–526. doi:10.1016/j.actbio.2017.06.015
Zhou, J., Sun, Y., Shu, H., Jie, S., Jing, L., Emmanuel, A. B. J. O., et al. (2019). Effect of laser peening on friction and wear behavior of medical Ti6Al4V alloy. Opt. Laser Technol. 109, 263–269. doi:10.1016/j.optlastec.2018.08.005
Keywords: atomic layer deposition, titanium dioxide, titanium implant, particles release, friction and corrosion
Citation: Zhao X, Zhang X, Zhou Z, Meng F, Liu R, Zhang M, Hao Y, Xie Q, Sun X, Zhang B and Wang X (2024) Atomic layer deposited TiO2 nanofilm on titanium implant for reduced the release of particles. Front. Bioeng. Biotechnol. 12:1346404. doi: 10.3389/fbioe.2024.1346404
Received: 29 November 2023; Accepted: 11 April 2024;
Published: 26 April 2024.
Edited by:
Hasan Uludag, University of Alberta, CanadaReviewed by:
Kui Xu, Anhui University of Chinese Medicine, ChinaRuiqiang Hang, Taiyuan University of Technology, China
Copyright © 2024 Zhao, Zhang, Zhou, Meng, Liu, Zhang, Hao, Xie, Sun, Zhang and Wang. This is an open-access article distributed under the terms of the Creative Commons Attribution License (CC BY). The use, distribution or reproduction in other forums is permitted, provided the original author(s) and the copyright owner(s) are credited and that the original publication in this journal is cited, in accordance with accepted academic practice. No use, distribution or reproduction is permitted which does not comply with these terms.
*Correspondence: Xiaojun Sun, eGlzMTk3MnN1bkAxNjMuY29t; Bin Zhang, emhhbmdiaW4yMDA5QHN4aWNjLmFjLmNu; Xing Wang, a3F3eDEwMEAxNjMuY29t
†These authors have contributed equally to this work