- 1The Second Hospital of Jilin University, Jilin, China
- 2Orthopedic Medical Center, The Second Hospital of Jilin University, Jilin, China
Osteochondral lesions are common pathological alterations in synovial joints. Different techniques have been designed to achieve osteochondral repair, and tissue-engineered osteochondral grafts have shown the most promise. Histological assessments and related scoring systems are crucial for evaluating the quality of regenerated tissue, and the interpretation and comparison of various repair techniques require the establishment of a reliable and widely accepted histological method. To date, there is still no consensus on the type of histological assessment and scoring system that should be used for osteochondral repair. In this review, we summarize common osteochondral staining methods, discuss the criteria regarding high-quality histological images, and assess the current histological scoring systems for osteochondral regeneration. Safranin O/Fast green is the most widely used staining method for the cartilage layer, whereas Gomori and Van Gieson staining detect new bone formation. We suggest including the graft–host interface and more sections together with the basic histological information for images. An ideal scoring system should analyze both the cartilage and bone regions, especially for the subchondral bone plate. Furthermore, histological assessments should be performed over a longer period of time to minimize discrepancies caused by defect size and animal species.
1 Introduction
The articular cartilage layer, the outermost portion of the osteochondral unit, provides tremendous durability and resilience, but limited repair capacity. In our joints, articular cartilage can reduce friction between articular bone and distribute various stress loading to underlying subchondral bone (Wang et al., 2021). The osteochondral unit, which consists of articular cartilage and underlies subchondral bone and the bone-cartilage interface, provides biomolecular and mechanical support to the upper cartilage layer. However, trauma-related injuries and natural wear within articular cartilage are difficult to repair and eventually lead to osteochondral lesions (Korthagen et al., 2019). To repair such lesions, various clinical strategies and techniques have been developed, such as arthroscopic debridement and lavage, marrow-stimulating techniques, osteochondral autografts or allografts, cell-implantation-based treatments, and total arthroplasty (Chen et al., 2019a; Ye et al., 2018). Unfortunately, these techniques have certain limitations during osteochondral repair and do not provide long-lasting satisfactory outcomes (Cho et al., 2020).
Osteochondral tissue engineering aims at creating cartilage-bone units or substitutes for repairing clinical osteochondral defects, and has successfully fabricated and utilized both bone and cartilage substitutes in clinical practice (Chen et al., 2018). It is generally accepted that osteochondral tissue engineering can provide better repair/regeneration than other defect-resurfacing methods. Tissue engineering can produce the appropriate shape, thickness, mechanical properties, surface integration, biocompatible properties, and self-healing and remodeling abilities, thereby showing promise for future clinical practices. These desired factors are interdependent and have been the focus of numerous osteochondral tissue engineering studies. However, to date, no consensus has been reached regarding the optimal tissue engineering design strategy for achieving satisfactory osteochondral repair (Zhu et al., 2017). An effort to combine different osteochondral graft designs and fabrication methods may provide better osteochondral repair and regeneration than other tissue-resurfacing methods.
Thus, to establish a reliable osteochondral repair method, it is necessary to compare different osteochondral tissue-engineered graft designs and fabrication methods. Currently, structural and functional analyses in translational animal models represents a standard approach to evaluating osteochondral regeneration. Successful osteochondral repair should regenerate the original structure, including the bone and cartilage regions, and subchondral bone plate. Histological assessments, micro-computed tomography, and MRI are common methods for assessing structural regeneration in vivo (Murata et al., 2022). Functional analysis, a purpose-oriented tissue-scale evaluation, focuses on the biomechanical properties and lubrication of the tissue-engineered block (Critchley et al., 2020). A satisfactory biomechanical result, such as proper stiffness and flexibility, should always mimic the mechanical properties of a natural osteochondral unit (Zhai et al., 2018). Although functional analysis determines the success of osteochondral repair, histological evaluations provide insights into repair mechanisms and accelerate the pace of “bench to bedside”.
To assess osteochondral repair, various staining methods are used, as they can reveal the anisotropy of osteochondral units. Selecting an appropriate staining method is crucial for evaluating lesions and histological scoring systems. For comparing osteochondral repair, quantitative histological scoring systems are more objective and reliable than qualitative histological images. The first scoring system for cartilage repair was established in 1986 by O’Driscoll and colleagues, who attempted to formulate a scoring system to evaluate the quality of newly formed cartilage (O'Driscoll et al., 1986). Later, O’Driscoll scoring systems were modified by the addition of a subchondral bone evaluation section to better assess osteochondral repair (Gan et al., 2019; Wu et al., 2020). Since then, numerous histological scoring systems, such as International Cartilage Repair Society (ICRS) (Xing et al., 2020), Pineda (Yucekul et al., 2017), Wakitani (Lu et al., 2018), and Sellers scoring (Lin et al., 2020), have been introduced. Although the ICRS developed a standardized scoring system for osteochondral repair, the lack of agreement regarding a unified histological evaluation system impedes comparison of different studies (Mainil-Varlet et al., 2003).
An ideal histological scoring system for osteochondral repair should consider both structural regeneration (e.g., the regeneration of subchondral bone, the subchondral bone plate, and an appropriately thick cartilage layer) and compositional restoration (e.g., restoration of the glycosaminoglycan (GAG) and collagen II gradient) of an osteochondral unit. However, no system exists that would cover all these aspects and thus provide a global view of repaired osteochondral tissue. To this end, modified scoring systems have been established, including the modified ICRS (which includes basal and lateral integration) and O’Driscoll (which includes subchondral bone) scoring systems (Oshima et al., 2019; Sun et al., 2018). Potential bias of these modified systems should be noted, as most of them remain to be validated. Some studies have verified the intra- and inter-observer reproducibility of traditional histological scoring systems; however, they have focused mostly on how to interpret histological images and convert findings into histological scores. Corresponding histological images with histological scores is difficult, and variations may impede comparisons between different studies. Thus, it is important to establish standard classifications of histological evaluation parameters or indices.
In this paper, we performed a literature review of histological assessments and scoring systems for in vivo osteochondral repair with tissue-engineered grafts. We reviewed histological scoring systems and histological images used in studies of translational osteochondral repair. Furthermore, we identified and analyzed common histological staining methods for osteochondral defects repaired by tissue engineering. Finally, we summarized current limitations and challenges associated with histological scoring systems for osteochondral repair to guide future translational studies toward clinical osteochondral regenerative medicine.
2 The osteochondral unit: architecture, composition, and function
The osteochondral unit is an anisotropic tissue block with a hierarchical architecture that enables its loading and lubrication functions (Qiao et al., 2021). It consists of articular cartilage, subchondral bone plate, and trabecular bone from superficial to deep regions (Jia et al., 2018). These three distinct tissue types have completely different structures and compositions (Figure 1). Thus, different regions should be considered separately in the evaluation of osteochondral regeneration.
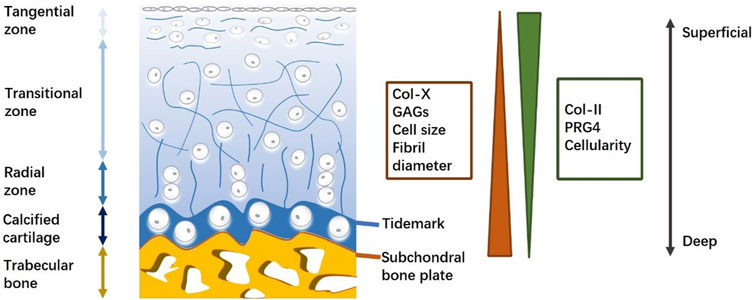
Figure 1. Schematic for osteochondral unit (structure and composition). Osteochondral structure and composition are varied with the depth from cartilage surface, displaying the heterogeneity of an osteochondral unit.
Articular cartilage, a precisely organized zonal tissue, covers the end of bones and protects joints by reducing friction between articular bone and distributing various stress loading to underlying subchondral bone (Cao et al., 2021). At the tissue level, articular cartilage thickness varies with location and local loading levels (Jia et al., 2018). At the cell level, chondrocytes that are embedded in the extracellular matrix (ECM) exhibit morphological differences across cartilage in correlation with depth (Boushell et al., 2019). At the molecular level, collagen fibers and proteoglycans weave into a network structure that provides resilience to stress, transports nutrients and signals, and enables chondrocyte adhesion (Zhu et al., 2018). Collagen II is the predominant component of collagen fibers and interacts with proteoglycans and other collagen types, including collagen IX, X, and XI (Wang et al., 2019). In contrast to collagen II, proteoglycan four expression decreases with depth, and collagen X and GAG cause adverse effects in the ECM (Hsieh et al., 2018). Collagen fibers cross-link with proteoglycan aggregates to protect cartilage against compressive and tensile forces (Wang et al., 2017). The arrangement of collagen fibers plays a significant role in the biomechanical properties of cartilage. Therefore, evaluating the orientation of regenerated collagen fibers is a promising approach to predicting the outcome of osteochondral repair.
Between the articular cartilage and subchondral bone, there exists a thin layer of calcified cartilage (Huang et al., 2021). This calcified cartilage is separated from non-calcified cartilage by a relatively irregular border known as the tidemark, which appears as a basophilic line on histological images (Zhang et al., 2017). Collagen fibers run through both the non-calcified and calcified zones. In this way, the tidemark enhances tensile strength and provides a physical barrier that protects distinct micro-environments in the cartilage and underlying bone.
The subchondral bone plate is a thin cortical lamella underlying the calcified cartilage zone that provides mechanical support for cartilage tissue. Compared with the compact structure of the subchondral bone plate, subchondral trabecular bone is a well-organized porous structure that includes osteocytes, osteoblasts, and osteoclasts (Sun et al., 2020). The vascularized and innervated trabecular zone exhibits potential endogenous healing capacity and feedback to biological and pathological conditions in the adjacent cartilage zone (Lin et al., 2020). Typical components of the trabecular zone are inorganic hydroxyapatites and organic collagen I, proteoglycans, and GAGs (Salonius et al., 2019). Proteoglycan composites, such as osteonectin, osteopontin, and osteocalcin, are specifically expressed in the trabecular bone.
The anisotropy of the osteochondral unit and the limited self-repair ability of articular cartilage make tissue engineering a promising technique for achieving osteochondral regeneration (Du et al., 2017). To date, many tissue-engineered osteochondral grafts have been evaluated in translational animal models (Kumai et al., 2019; Pérez-Silos et al., 2019; Gardner et al., 2019). However, an optimal design strategy that can achieve satisfactory osteochondral repair remains to be established. Thus, it is becoming increasingly important to establish a clear definition of successful osteochondral repair, and to compare results across different studies.
3 Methodology for osteochondral histological assessments
Histological evaluation is a widely accepted method for studying osteochondral regeneration, with the goal of analyzing regenerated tissue compositionally and structurally. The histochemical preparation of osteochondral tissue includes fixation, decalcification, processing, and cutting into sections (Chen et al., 2019b). For fixation and decalcification, osteochondral tissue should be treated differently from common connective tissues, as chondrocytes and ECM are prone to degradation by abundant fixation and decalcification agents. Thus, traditional decalcification agents (e.g., sulfuric, hydrochloric, and nitric acids) are unsuitable for preserving chondrocytes. Alternatively, organic acids and chelating agents avoid the aforementioned side effects and provide reliable decalcification. Ethylenediaminetetraacetic acid (EDTA), a chelating agent, is widely used in osteochondral tissue decalcification. When the decalcification is not urgent, EDTA is able to maintain the osteochondral tissue in a life-like state (Gong et al., 2020; Liu et al., 2017). Furthermore, for time-limited decalcification, formic acid is recommended. Fixed and decalcified tissue blocks should be embedded in paraffin to enhance their mechanical strength for further sectioning (Wang et al., 2019). Once cut, the tissue sections are fixed in warm solution, are placed on microscope slides, and undergo deparaffinization in xylene for upcoming staining (Murata et al., 2018).
Hematoxylin and eosin staining is a common method for observing pathological changes in cartilage. However, for the evaluation of cartilage repair, various other histological staining methods can be used (Li et al., 2023; Zhao et al., 2022). Currently, several histological criteria are considered to correlate with high-quality cartilage repair. For the chondral zone, the restoration of GAGs and proteoglycans (with an appropriate gradient), collagen II fibers (with an appropriate orientation and location), and chondrocytes (their morphology and quantity) should be evaluated in histological sections. Most in vivo osteochondral regeneration studies evaluate the presence of GAGs or proteoglycans with Safranin O and Alcian blue, positively charged dyes that selectively stain acid polysaccharides (Yu et al., 2022; Tamaddon et al., 2022). Safranin O and Alcian blue can stain carboxylates and sulfated polysaccharides at a pH of 2.5, whereas only Alcian blue stains more-acidic sulfated polysaccharides (e.g., with a pH of 1). Additionally, toluidine blue has been employed to stain GAGs (Cao et al., 2022). Although less commonly evaluated, collagen fibers are a vital component of cartilage and can be stained with Sirius Red (Burdis et al., 2022), Van Gieson (Cho et al., 2020), or Masson’s Trichrome (Lamparelli et al., 2021; Fang et al., 2022).
To histologically evaluate osteochondral regeneration in bone regions, Gomori, Van Gieson, and Alizarin Red staining are used. Gomori staining reveals bone alkaline phosphatase, a typical biomarker for osteoblasts (Yu et al., 2018). Alizarin Red stains calcium deposition in the osteo-zone, revealing new bone formation (Cunniffe et al., 2019). Although researchers prefer micro-computed tomography for the evaluation of bone reconstruction, this method does not reveal all details regarding osteochondral regeneration (Mendes et al., 2018; Nie et al., 2020). Histological sections not only reveal regenerated bone but also cartilage and the tidemark, thereby demonstrating any relationships between bone and cartilage repair (Table 1).
As a complementary approach, immunohistochemical staining targets specific molecules in regenerated osteochondral tissue. Collagen I and II are two major molecules in immunohistochemical staining, as they are markers of fibrous and hyaline cartilage, respectively (Seong et al., 2017). During osteochondral repair, mechanically defective fibrous cartilage is undesirable, and thus higher collagen I expression indicates poor cartilage regeneration (Jiang et al., 2021). To date, several different markers related to articular cartilage regeneration have been evaluated. Aggrecan is the major structural component in articular cartilage (Yan et al., 2021). SOX-9, a transcription factor, plays a key role in chondrogenesis and can be detected by immunohistochemical methods (Qi et al., 2020; Lamparelli et al., 2022a). Furthermore, MMP-13 expression is less commonly evaluated, even though its increase may be related to poor-quality cartilage and chondrocytes (Liu et al., 2019). Very few studies have assessed subchondral bone regeneration with immunohistochemical methods. Nevertheless, collagen X and osteocalcin were shown to be markers of mineralized tissue, such as calcified cartilage and subchondral bone, in newly generated tissue (Daly et al., 2018; Ma et al., 2019).
4 Histological scoring systems for in vivo osteochondral regeneration
4.1 Overview of histological scoring systems
Evaluating the histological quality of osteochondral tissue is considered a reliable method to indicate osteochondral repair in translational animals. Numerous histological scoring systems were established to assess cartilage/osteochondral states in the field of cartilage tissue engineering. We found more than 20 histological scoring systems (13 original systems and their derivate systems) in studies on in vivo osteochondral regeneration (included in this review). Of these, ICRS, O’Driscoll, Wakitani, and their modified systems are most popular for evaluating in vivo osteochondral regeneration.
Nevertheless, there are still controversies regarding the selection of a histological scoring system. First, scoring systems designed with different purposes were used in the studies we reviewed, and the osteoarthritis histological system may yield unreliable results when evaluating osteochondral regeneration (Liu et al., 2023). Second, most histological scoring systems aim to assess cartilage regeneration (Tani et al., 2017; Duan et al., 2019); however, the quality of newly formed subchondral bone should be considered when assessing osteochondral unit regeneration. To fill this gap, investigators modified previous scoring systems by adding a bone-evaluating section (Ruvinov et al., 2019). However, large disparities between modified scoring systems exist and further impede comparisons between different tissue-engineering strategies (Jiang et al., 2018; Wang et al., 2018). Third, only a small number of scoring systems were validated by assessing reproducibility, sensitivity, and specificity (Duan et al., 2019).
In theory, a complex scoring system with more parameters can provide comprehensive details and yield higher sensitivity. In fact, both regenerated cartilage and bone tissue should be evaluated, as unequal numbers of cartilage and bone parameters may cause bias and misunderstandings when repair results are interpreted through histological scores. Thus, selecting and organizing osteochondral repair parameters for different tissue regions may reduce the potential biases of current histological scoring systems. A typical osteochondral unit consists of cartilage, trabecular bone, and a subchondral bone plate. Upon implantation of a tissue-engineered osteochondral graft into a defect, the local area is divided into a graft zone, a host zone, and a graft–host interface zone. In this way, an osteochondral regeneration joint model can be divided into nine regions: the graft cartilage, graft subchondral bone plate, graft trabecular bone, host cartilage, host subchondral bone plate, host trabecular bone, cartilage interface, subchondral bone plate interface, and trabecular bone interface regions (Figure 2). According to these spatial subdivisions for osteochondral regeneration, all reported histological scoring system parameters will be reviewed and summarized.
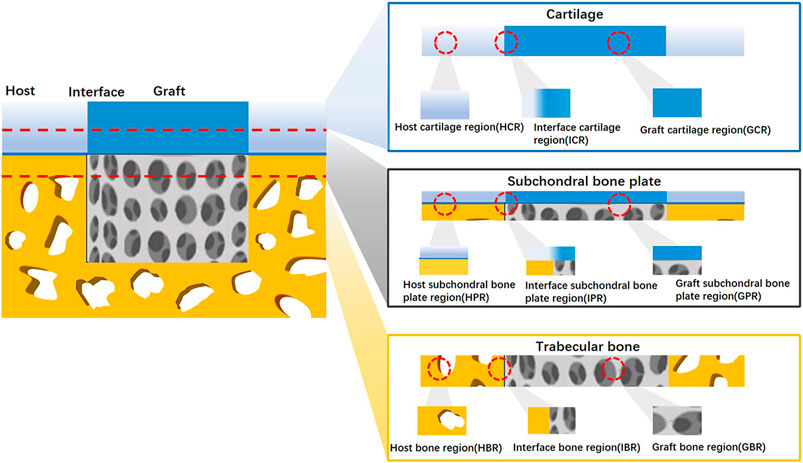
Figure 2. Spatial schematic for nine division osteochondral defect A regenerated osteochondral tissue block was divided into nine regions according to host–graft interface and cartilage-bone variation.
Among the nine regions of osteochondral regeneration, the graft cartilage region has attracted the most attention. Unlike the graft region, host region parameters are seldom included in histological scoring systems. Conversely, higher evaluation rates were found in the cartilage, subchondral bone plate, and trabecular bone region. None of the studies included in this review used a histological scoring system to evaluate the host subchondral bone plate.
4.2 Region-specific osteochondral histological evaluations
4.2.1 The graft cartilage region
Successful functional reconstruction of a joint should consist of structural and compositional cartilage regeneration. Thus, among the aforementioned nine regions, the graft cartilage region receives the most attention during osteochondral repair (Ding et al., 2022). The parameters for evaluating cartilage repair are similar across various histological scoring systems. At the tissue level, surface roughness (Chen et al., 2022; Wang et al., 2022), cartilage thickness (Zhang et al., 2022; Yan et al., 2020a), and ECM components are the most popular parameters for evaluating graft cartilage and are included in almost all scoring systems (Luo et al., 2022; Bozkurt et al., 2019). The surface of cartilage appears in a linear form that is easy to compare between slices. However, the omission of tiny fissures may be an inherent limitation in selecting sections. Thus, slides should be double-checked macroscopically (Zhang et al., 2018). Cartilage thickness is evaluated by comparisons with adjacent healthy cartilage; variable tissue thickness can negatively affect biomechanical structure and thereby cause osteochondral graft failure (Pascual-Garrido et al., 2019). In some cases, normal cartilage thickness in the graft region may not indicate high-quality osteochondral regeneration because the height of the newly formed subchondral bone plate may not be appropriate. This in turn may cause the overlying articular cartilage to be too high or low (Park et al., 2019).
ECM components surround and are produced by nearby chondrocytes, and indicate the cells’ status (Yin et al., 2018). ECM components are assessed in all the histological scoring systems that we reviewed; however, different scoring systems assess different ECM components. For example, the O’Driscoll (Widhiyanto et al., 2020) and Sellers (Lin et al., 2020) scoring systems evaluate GAGs by Safranin-O staining, whereas ICRS scoring systems evaluate GAGs by toluidine blue staining (Wang et al., 2018). As an alternative parameter, some histological scoring systems evaluate the percentage of osteochondral defect fulfillment, which is sensitive to relatively low-quality osteochondral repair with obvious defects (Petrovova et al., 2021).
At the cell level, the size, morphology (Shen et al., 2021), quantity (Mohan et al., 2017), and arrangement of chondrocytes (Wang et al., 2017) are widely used parameters in current histological scoring systems. The O’Driscoll scoring system tends to provide more details about chondrocytes and covers all the abovementioned parameters except cellularity (Cai et al., 2022). Most traditional histological scoring systems focus on cell morphology to classify cartilage, distinguishing between hyaline and fibrous cartilage. Recently, various modified ICRS and O’Driscoll scoring systems have taken chondrocyte clustering into account (Zhao et al., 2022; Yan et al., 2021). Chondrocyte clustering is an abnormal cell arrangement that correlates with immature or degenerated cartilage tissue (Xu et al., 2021). Chondrocytes and the ECM represent important aspects for histologically evaluating the graft cartilage region. During osteochondral regeneration, chondrocytes and the ECM always interact with each other, and tissue-engineering strategies attempt to influence this interaction and promote hyaline cartilage formation. Similar parameters across the various scoring systems demonstrate the consensus on histologically evaluating the graft cartilage region.
4.2.2 The cartilage interface region
Because of the intrinsic avascular and denervated properties of articular cartilage, the cartilage interface between the graft and host is the most difficult region to reconstruct (Yang et al., 2018). Insufficiently repaired chondral interface tissue is still a challenge for the translation of osteochondral repair (Nordberg et al., 2021). Histologically, successful cartilage interface regeneration should exhibit continuous ECM in both the superficial layer (with a smooth surface) and the deep layer (without gaps) (Wei et al., 2019). ECM staining that is similar to an extent to that of the adjacent host cartilage region is also needed (Xing et al., 2021). Currently, two parameters related to the cartilage interface region are included in histological scoring systems. O’Driscoll, Wakitani, Sellers, ICRS, Fortier, and some modified scoring systems have assessed graft–host cartilage integration by checking gaps or lacks of continuity on either side of the graft (Xiao et al., 2019; Kumbhar et al., 2017). Conversely, to determine cartilage interface quality, some modified ICRS scoring systems classify the tissue into fibrous and cartilage-like tissue (Wong et al., 2018). Both “tissue continuity” and “cartilage-like” characteristics are parameters that focus on ECM status. Because of the limited space between tissue-engineered grafts and host cartilage tissue, chondrocyte-related parameters are not evaluated in this region. Although parameters related to the cartilage interface region are limited, the regeneration of the cartilage interface is crucial and should never be ignored during histological evaluation.
4.2.3 The host cartilage region
Although osteochondral regeneration does not occur within the host cartilage region, ongoing regeneration affects adjacent healthy cartilage and causes further changes. In turn, even if successful osteochondral tissue is achieved, an impaired host cartilage region may cause the new osteochondral tissue block to rapidly break down. Quite a few scoring systems have taken the host cartilage region into account. The O’Driscoll scoring system analyzes chondrocyte cellularity, cluster changes, and ECM staining to determine host cartilage quality (Kim et al., 2021). Other modified scoring systems evaluate the cellularity and GAG content of adjacent host cartilage (Yan et al., 2021). Along with the cartilage tissue around the graft, the cartilage on the opposite side of a joint should also be assessed because it plays a role in sliding and loading with the graft cartilage region. However, few histological scoring systems currently evaluate this region. With the development of osteochondral tissue engineering, we believe that the host cartilage region will become another meaningful zone to analyze and record. A modified histological scoring system that includes the host cartilage region will provide more comprehensive details. Enhancing histological evaluations to include not only the defect but the entire joint will advance the translational progression of osteochondral tissue engineering.
4.2.4 The graft bone region
The graft bone region has seldom been evaluated in previous studies on cartilage or osteochondral repair. Two reasons account for the unbalanced evaluation of bone and cartilage. First, compared with cartilage tissue, bone tissue is easier to regenerate, as it contains abundant blood vessels and multi-potential cells (Shang et al., 2020). Second, to some extent, the quality of cartilage repair correlates with the quality of underlying bone repair (Filová et al., 2020). Therefore, many studies still use simple histological evaluations of cartilage. Recently, the importance of bone regeneration in osteochondral defects was demonstrated, leading to the development of suitable histological scoring systems for evaluating bone regeneration, especially the graft bone region (Dai et al., 2018). The primary parameter for evaluating bone regeneration is “defect fulfillment” in the graft bone region. Among the traditional scoring systems (Petrovova et al., 2021), the Sellers system measures the percentage of new subchondral bone, i.e., defect fulfillment (Lin et al., 2020). The modified ICRS (Fang et al., 2022) and O’Driscoll scoring systems (Cho et al., 2020) and some new systems have included this parameter for preliminary evaluations. Considering specific trabecular bone architecture, some modified scoring systems try to analyze bone tissue morphology. Most bone morphology evaluating systems are derived from the Wakitani system and also include the “bone defect fulfillment” parameter (Wang et al., 2019). Another modified ICRS scoring system also estimates bone morphology but without “bone defect fulfillment”, which may cause a relatively lower proportion of evaluations related to bone tissue (Oshima et al., 2019).
Furthermore, “inflammation” is another parameter for osteochondral repair, as exogenous material implanted in the local defect may induce rejection by the host. Although biocompatibility has already been tested ex vivo, host–graft reactions should be further investigated. Accordingly, some researchers have added an “inflammation” parameter to the O’Driscoll scoring system (Jiang et al., 2021). Our review demonstrates that graft bone regions are evaluated less often than graft cartilage regions. However, future studies should discuss and determine the degrees to which regions of graft bone versus cartilage should be analyzed in histological scoring systems.
To promote subchondral bone regeneration, tissue-engineering materials, such as hydroxyapatite, tricalcium phosphate, decalcified bone matrix, bioactive glass, and metal materials, can be incorporated into the bone region to enhance the graft’s mechanical strength. However, this may impede the formation of new subchondral bone (Zhang et al., 2021). Some semi-quantitative histological scoring systems have analyzed the “percentage degradation of the implant” during rabbit osteochondral repair (Cho et al., 2020). Material degradation parameters are specifically appropriate for assessments of grafts, especially graft bone regions. An optimal time point of graft degradation and new bone formation is desirable.
4.2.5 The interface bone and host bone region
The modified O’Driscoll scoring system is one of the few systems that analyzes the integration of the osteochondral graft with its surroundings and is thus adequate for evaluating bone interface regeneration (Du et al., 2017). Similar to the parameter of cartilage integration, the parameter “extent of new tissue bonding with adjacent bone” includes the following subitems: “complete on both edges”, “complete on one edge”, “partial on both edges”, and “without continuity on either edge”. Besides lateral integration, basal integration between the osteochondral graft and host bone tissue should be adequate. Thus, a small number of parameters were chosen for evaluating the basal interface. OsScore and ICRS II have assessed the basal integration of cartilage regeneration (Stefani et al., 2020; Bianchi et al., 2019). This review includes only four studies with modified systems that evaluate the host bone region. It seems that researchers, rather than using quantitative parameters, prefer to subjectively assess the host bone during osteochondral repair (Khanmohammadi et al., 2019; Mohan et al., 2017).
4.2.6 The graft subchondral bone plate region
The subchondral bone plate, a previously underestimated factor, influences the long-term performance of osteochondral regeneration. Previous systems seldom focused on subchondral bone plate regeneration, and a few systems analyze the osteochondral junction and tidemark reconstruction instead (Liao et al., 2017). The Pineda scoring system evaluates the reconstruction of the osteochondral junction with simple classifications: “complete reconstruction”, “almost reconstruction”, and “not close”. The Sellers system indirectly assesses the formation of new subchondral bone by measuring the extent of tidemark formation and using the following classifications: complete, 75%–99%, 50%–74%, 25%–49%, and <25% (Lin et al., 2020). A modified version of the O’Driscoll system indirectly evaluates the subchondral bone plate with the parameter “bonding of repair cartilage to de novo subchondral bone” (Du et al., 2017). However, the extent of tidemark formation is not equally representative of subchondral bone plate regeneration. Even if subchondral bone plate regeneration is successful, heterotopic osteophytes and subchondral bone protrusion into the cartilage layer may corrupt the tidemark (Feng et al., 2020a). The thickness of a newly formed subchondral bone plate is closely correlated with its mechanical properties. As such, the subchondral bone plate should be restored to a similar thickness to that of the adjacent subchondral bone plate. However, no parameter related to subchondral bone plate thickness has been used in studies on translational tissue engineering. As the increasingly predominant role of the subchondral bone plate is being recognized, more related parameters should be included in histological assessments of osteochondral regeneration.
4.2.7 The interface and host subchondral bone plate regions
Current histological scoring systems have not been used to evaluate subchondral bone plate interface formation or the host subchondral bone plate region. For multilayer or gradient tissue-engineered osteochondral grafts, specific layers or regions were designed to correspond with osteochondral bone plates (Gupta et al., 2017; Idaszek et al., 2019). These specific layers or regions have potential to transform into new osteochondral bone plates in situ. Conversely, typical elementary tissue-engineered one- or two-layered osteochondral grafts display a transitional regeneration process of subchondral bone plate from bottom to top and finally approach the host subchondral bone plate (Feng et al., 2020b; Liu et al., 2020). Therefore, plate integration is an important sign of completed osteochondral repair.
Osteochondral regeneration should also be spatially evaluated. In other words, assessments of the quality of tissue repair should also consider the relationships between different regions. Traditional histological scoring systems for osteochondral repair are result-oriented, focusing on the quality of repaired cartilage and thus potentially ignoring any mechanisms underlying osteochondral regeneration failure. For tissue-engineered grafts, assessments that determine the causes of failure are preferred. To this end, a spatially based histological scoring system shows promise. Current histological scoring system parameters were divided into nine separate regions, and the ratio of every single region in histological scoring systems and current or potential supplementary parameters were summarized (Figure 3).
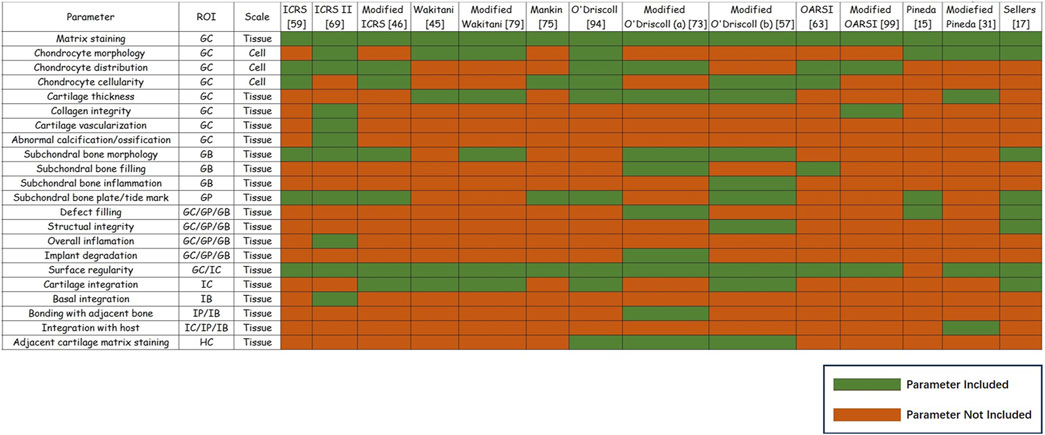
Figure 3. Summary of histological parameters for osteochondral regeneration. Summary of histological parameters for osteochondral regeneration. Parameters’ region of interest (ROI) and scale are shown. For the most widely used 14 histological scoring systems for osteochondral regeneration, the green boxes refer to specific parameters that were included in this system, while red boxes indicate that the parameter was not included in this system.
4.3 Validation of histological scoring systems
Validating histological scoring systems to improve the reliability of observations and the comparability between studies is widely accepted (Rutgers et al., 2010). However, only a few scoring systems, such as the O’Driscoll and Pineda systems, have been validated (Moojen et al., 2002). A characteristic feature of healthy cartilage integrity is the content of proteoglycans, essential biochemical factors, which have been correlated with the O’Driscoll and Pineda systems for validation (Grogan et al., 2006). Nevertheless, the reproducibility of most histological scoring systems has not been evaluated or validated to date. To validate a scoring system, the system is usually compared with already validated macro- or micro-scoring systems, biochemical factors, or other validated evaluation methods (Custers et al., 2007). Nevertheless, it is still unclear whether a histological score can be correlated with the quantity of biomolecules in repaired tissue (Orth et al., 2012a).
4.4 A temporal scale for osteochondral histological evaluation
Osteochondral regeneration usually proceeds in specific spatial and chronological patterns. However, histological evaluations for translational studies are limited by the test frequency and ignore temporal alterations of cartilage and subchondral bone compartments. An adequate experimental duration and proper observation frequency should be emphasized. Most in vivo osteochondral regeneration studies share an observational time span of 1–52 weeks. Additionally, more than half of in vivo osteochondral regeneration studies histologically evaluated regeneration only once.
Assessing the histological performance of blank groups is another potential way to compensate the drawbacks of temporal histological evaluations. The histological performance of blank groups, especially from similar in vivo osteochondral repair studies, may contribute to our understanding of the chronology of osteochondral self-repair patterns (Orth et al., 2012a). Osteochondral repair can be compared between similar species, ages, genders, defect locations, and defect sizes. Although this review includes a limited number of studies, a trend can be observed in which regeneration starts from the bottom and lateral side of osteochondral defects.
5 Determining the quality of histological images
High-quality histological images not only provide comprehensive information regarding repair but also indicate the quality of the research to some extent. Although histological assessment is a widely acknowledged routine evaluation for osteochondral repair, the quality of histological images varies across studies. The basic information that should be included in histological images is as follows. First, an ideal histological image should contain clear scale bars and display integrated regions of interest (Yan et al., 2020b). The term integrated tissue regions refers to the graft–host interface and relevant adjacent native tissues. As there are two lateral boundaries for cylindrical osteochondral defects, neither interface should be ignored. Indicators that illuminate the graft contour or interface are recommended, as they enable more precise interpretation, especially for images that only display partial defect regions (Tani et al., 2018; He et al., 2017). Second, the image’s orientation, e.g., anterior/posterior and medial/lateral, serves as meaningful information to correlate micro-features with macroscopical appearance (Nie et al., 2020). Moreover, section location on a joint is another way to facilitate the micro-to-macro correlation (Salonius et al., 2019). Third, histological sections for various staining should be selected from an adjacent location. Some studies even attempt to correspond histological images with micro-computed tomography results, which yields more details (Liu et al., 2020; Fu et al., 2016).
Conversely, for osteochondral regeneration, muti-layer grafts exhibit the original location of each layer on histological images, revealing early pathological changes in each layer with different components (Nordberg et al., 2021). Additionally, graft residues should also be recorded and marked during microscopy (Zhou et al., 2017). Histological images for evaluating osteochondral repair should be compared with other examinations. Comparing images from different staining methods, radiological examinations, and macroscopic analyses (both at the level of the graft and joint) constitute the standard of a high-quality histological evaluation (Duan et al., 2019; Gan et al., 2019; Feng et al., 2020b) (Figure 4).

Figure 4. Principles for histological image quality evaluation. (A) pictures illustrated the osteochondral regeneration with silk fibroin/collagen scaffold in New Zealand Rabbits. Host-graft interface was clearly displayed by two distinct arrows. Besides, the orientation of histological image was obviously provided by corresponding to CT images. It is also recommended to place different stained images (A6, A7, A8) from similar location. Unfortunately, these pictures do not include all the region of interest for osteochondral graft. (B, C) pictures provide the osteochondral regeneration outcomes in rabbits model, with mussel-inspired hydrogel. They utilized columnar indicator to show the intact region of interest and graft contour for interpreting the regeneration. The in vivo osteochondral regeneration using a bi-layered poly(lactide-coglycolide) porous scaffold is show in (D) pictures. For the short-term repair results, asterisk was used to indicate the graft residuals. Transplants alternation and interaction with host over time could be better interpreted. Such residues indicator contributed to a high-quality image and thus desired. Although all histological images involved in this figure are harvested from rabbits, overall appearance of (D) is totally different from (A–C) which caused by the sample sectioning. Therefore, histological images orientation, such as medial, lateral, anterior and posterior, is recommended to improve clarity and consistent of future histological display. *(A) was cited from ref Feng et al. (2020b), (B, C) was cited from ref Gan et al. (2019), and (D) was cited from ref Duan et al. (2019). And we have got copyright permission from “Royal Society of Chemistry”, “WILEY”, and “ELSEVIER”.
6 Current limitations and potential improvements of osteochondral histological evaluation
Histological evaluations are important for determining osteochondral regeneration in translational animal models. In this review, we summarized current histological methodologies and scoring systems for osteochondral tissue engineering. Currently, researchers focus on achieving acceptable osteochondral regeneration. However, there is no consensus on how to properly and objectively assess osteochondral regeneration, which may impede the progression of osteochondral tissue engineering. Any limitations and challenges of current histological scoring systems affect future translational research.
One major drawback of current histological scoring systems is the lack of consistent standards for the evaluation of bone. For the reconstruction of a smooth articular surface, previous studies focused on the regeneration of the cartilage layer, which was analyzed by the O’Driscoll (Wang et al., 2022), Wakitani (Zhang et al., 2022), and Mankin scoring systems (Peng et al., 2019). These scoring systems provide widely acknowledged parameters for cartilage repair, from compositional to structural reconstruction. However, parameters regarding the bone region are not included in these systems. With the growing recognition of the importance of subchondral bone during osteochondral regeneration, histological scoring systems have been supplemented with additional parameters, such as subchondral bone plate reconstruction and trabecular bone morphology and fulfillments (Cho et al., 2020). Nevertheless, parameters regarding the subchondral bone plate and subchondral trabecular bone still share only a small part of the entire scoring system. Such systems may underestimate the function of bone during the repair of osteochondral units. Although CT provides details of bone tissue, our still-lacking understanding of the relationships between subchondral bone and overlying cartilage on CT images limits interpretations regarding inadequate cartilage regeneration (Gu et al., 2022). Therefore, future histological scoring systems that place more importance on subchondral bone, especially the subchondral bone plate, are necessary.
In addition to the reconstruction of the subchondral bone plate, the height and integration of the subchondral bone plate have also been suggested to be included in osteochondral scoring systems. Bone plate heights of self-healing osteochondral defects vary among animal species and defect types; however, a height similar to that of the host subchondral bone plate is ideal (Orth et al., 2012b). Both subchondral bone that protrudes into cartilage and a concaved subchondral bone plate impair the long-term performance of tissue-engineered osteochondral grafts.
Current histological scoring systems indicate a trend of evaluating regeneration at the joint level rather than the defect level. Along with this transition, several systems have started to consider the host region as well (Cai et al., 2022). It is now encouraged to histologically evaluate host bone and synovium to determine the effect of heterogenous tissue-engineered grafts on surrounding micro-environments. Nevertheless, current scoring systems seldom include these host-related parameters, which may be due to overlaps with in vitro biocompatibility studies (Tian et al., 2021; Lamparelli et al., 2022b). For tissue-engineered osteochondral grafts, any graft residues should also be recorded, as the occupying effect and degradation products will influence local regeneration (Ran et al., 2021).
Another limitation of current histological scoring systems for osteochondral repair is chronological inadequacy. Most studies usually select two timepoints to represent short-term and long-term outcomes separately (Gu et al., 2022). However, it may be incorrect to draw conclusions from single timepoints. A promising solution may be to summarize the morphology of regenerated tissue, especially of cartilage and the subchondral bone plate, from more timepoints. Some studies have already summarized histological classifications of cartilage and subchondral bone during osteochondral repair (Orth et al., 2012b; Qiu et al., 2003). Such classifications can enable the grouping of similarly repaired tissues. Collecting regenerated tissues with similar pathological changes into groups can reveal potential risk factors that cause poor regeneration (Figure 5).
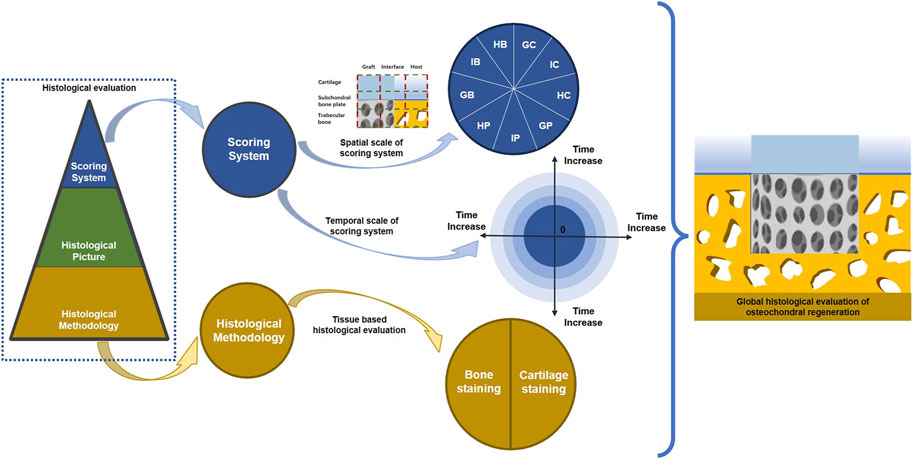
Figure 5. Potential improvement for current osteochondral regeneration evaluation. Global histological evaluation is recommended for osteochondral regeneration, which consists of spatial and temporal integrity for the scoring system. Furthermore, staining methods for cartilage and bone should be standardized for the histological scoring system.
There are a number of limitations inherent to osteochondral histological scoring systems. Regenerated tissue is displayed on histologically stained images, whereas histological scoring systems are textually described. Variations in understanding the textually described scoring criteria are inevitable between different observers, even though scoring systems have been validated to ensure their reliability and comparability. A single histological section may still be interpreted in different ways, even when the same textual criteria are used. To overcome this limitation, the ICRS II scoring system offers image-based criteria, which can be found on the internet (Mainil-Varlet et al., 2010).
High-quality images are fundamental for histological assessments and include appropriate staining methods for specific tissue components, appropriate magnification to detect details, complete regions of interest, and appropriate selection of histological sections that correspond with radiological evaluations. Common staining methods for osteochondral units have been discussed in our review. Selecting the appropriate stain can facilitate the analysis of the newly formed tissues’ quality. Certain scoring systems provide suggestions for selecting the appropriate staining technique (Gao et al., 2021). The Sellers scoring system requires Safranin O/Fast green for cartilage matrix staining (Katagiri et al., 2017), whereas the O’Driscoll scoring system merely suggests Safranin O staining for the same purpose (Lin et al., 2019). Conversely, the Fortier scoring system requires toluidine blue staining to evaluate cartilage-related parameters (Tschon et al., 2021). Additionally, numerous scoring systems do not indicate any specific staining method for histological evaluation. No consensus exists on whether a standard stain is necessary for histological scoring systems for osteochondral repair; however, this should be investigated in future studies to determine the effects of staining methods on histological results.
In addition to histological scoring systems, immunohistochemical analysis is another method to evaluate osteochondral regeneration (Browe et al., 2022). Immunohistochemical staining uses antibodies to indicate the presence of specific molecules. For a comprehensive evaluation of osteochondral unit regeneration and hyaline layer quality, collagen II was mainly used as a hyaline cartilage marker (Xia et al., 2022; Abedin et al., 2022). Conversely, collagen I, an undesired component during osteochondral repair, indicates fibrous cartilage and was used to determine the quality of cartilage by immunohistochemistry (Wang et al., 2020). Because of the increasingly recognized role of subchondral bone, studies have focused on collagen X (Li et al., 2020) and osteocalcin (Zhu et al., 2020) to detect cartilage calcification and bone growth and finally to provide a complete picture of osteochondral regeneration. Conversely, although aggrecan (Kondo et al., 2021) and Sox9 (Zhang et al., 2019) represent alternative markers for investigating cartilaginous tissue, few studies have analyzed them (Lamparelli et al., 2022a). This review reveals that current histological scoring systems do not include immunohistochemical staining. The potential reasons for this are explained below. First, most histological scoring systems were established before the wide use of immunohistochemical staining in osteochondral regeneration. Most scoring systems considered in this review were established before or around 2000, whereas immunohistochemical evaluations became widely used only afterwards (Rutgers et al., 2010). Second, the original design purpose of scoring systems may also impede the inclusion of immunohistochemical evaluation. Certain systems, such as OARSI and Mankin scores, were developed for osteoarthritis assessment, whereas other systems, such as ICRS and OSWESTRY, were developed for clinical purposes. Such original purposes for scoring systems make immunohistochemical evaluation non-essential. Third, some proteins of interest, which are present in the cell membrane and cell matrix, may limit quantitative assessments of immunohistochemical staining (Ming et al., 2018). However, researchers usually compare immunohistochemical results to histological results. Considering that methodological advancements have enabled the immunohistochemical detection of specific proteins, such new approaches should facilitate future investigations of osteochondral regeneration (Lamparelli et al., 2021). Accordingly, future scoring systems should include immunohistochemical staining for a comprehensive evaluation of osteochondral regeneration.
In vivo osteochondral regeneration is a pivotal process for tissue-engineered osteochondral grafts before clinical application. During this translational process, animal models first consist of smaller animals, such as rats (Ming et al., 2018), rabbits (Rastegar Adib et al., 2022), and dogs (Stefani et al., 2020), and then consist of larger animals, such as horses (Murata et al., 2022), goats (Kostešić et al., 2022), and pigs (Zlotnick et al., 2022). The need to assess models at the joint scale has complicated histological processing. Longer decalcification, paraffin embedding, and complex sample dissection are time-consuming yet inevitable (Favreau et al., 2020). Additionally, it is difficult to fully include larger osteochondral defects in one histological image, especially at higher magnifications (Bothe et al., 2019). As such, incomplete defect regions will omit important details and hinder interpretation. To minimize these drawbacks, samples should be cut with a detailed plan to prevent potential tissue loss. Graft–host interface indicators or graft contours should be provided on histological images to facilitate quick and accurate positioning. Another possible method to improve the evaluation of osteochondral repair in large animals is to analyze their gait (Ding et al., 2022). As gait parameters better represent the clinical situation, gait analysis may be a promising method to verify histological scoring systems for large-scale osteochondral defect models in the future.
The processing of samples, but not the results of sample staining, may be influenced by the animal species. Osteochondral units share structural and compositional similarities in synovial joints across different mammalian species (Mendes et al., 2020). Therefore, staining methods may remain consistent across different animal models. Nevertheless, small animals tend to receive higher histological scores than large animals owing to their relatively smaller joints. Thus, similar histological scores between small and large animals do not necessarily indicate similar osteochondral regeneration. As such, osteochondral tissue engineering should consider different animal models. To begin with, smaller animal models should be used for screening and preliminary evaluations. This should be followed by the use of larger animal models for preclinical assessments (Nie et al., 2019). Another potential explanation for inconsistent histological scoring criteria is the variability among staining methods (Lamparelli et al., 2022a). To address this, future histological scoring systems should specify staining methods that adequately convey compositional regeneration.
7 Conclusion
In this review, we reported the current framework of histological assessments of in vivo osteochondral repair with tissue-engineered grafts. Common staining methods were divided into two main categories for the chondral and subchondral bone part. Safranin O/Fast green is the most widely used staining method for the cartilage layer, whereas Gomori, Van Gieson, and Alizarin Red staining detect new bone formation. Moreover, appropriately displaying histological images with comprehensive details is crucial for interpreting the quality of osteochondral tissue. Finally, there is still no widely accepted histological scoring system for osteochondral regeneration. An ideal system should analyze both cartilage and bone regions equally. Furthermore, performing histological observations over longer periods of time may minimize discrepancies caused by defect size and animal models. Histological classification of repaired cartilage and subchondral bone plate is a promising method for predicting the long-term performance of osteochondral tissue-engineered grafts.
Author contributions
MC: Investigation, Writing–original draft, Data curation, Formal Analysis, Methodology, Software. YS: Data curation, Methodology, Software, Resources, Writing–review and editing. XZ: Data curation, Writing–review and editing, Resources, Formal Analysis. PY: Data curation, Writing–review and editing, Methodology, Software. WJ: Writing–review and editing, Conceptualization, Funding acquisition, Investigation, Project administration, Supervision, Validation, Visualization, Writing–original draft.
Funding
The author(s) declare that financial support was received for the research, authorship, and/or publication of this article. This work was funded by the Natural Science Foundation of Jilin Province (YDZJ202201ZYTS281).
Conflict of interest
The authors declare that the research was conducted in the absence of any commercial or financial relationships that could be construed as a potential conflict of interest.
Publisher’s note
All claims expressed in this article are solely those of the authors and do not necessarily represent those of their affiliated organizations, or those of the publisher, the editors and the reviewers. Any product that may be evaluated in this article, or claim that may be made by its manufacturer, is not guaranteed or endorsed by the publisher.
Abbreviations
Col, collagen; ECM, extracellular matrix; EDTA, ethylenediaminetetraacetic acid; GAGs, glycosaminoglycans; ICRS, International Cartilage Repair Society; IHC, immunohistochemical; PRGs, proteoglycans.
References
Abedin, D. S., Hanaee-Ahvaz, H., Irani, S., Soleimani, M., Khatami, S. M., and Sohi, A. N. (2022). A composite bilayer scaffold functionalized for osteochondral tissue regeneration in rat animal model. J. Tissue Eng. Regen. Med. 16 (6), 559–574. doi:10.1002/term.3297
Asensio, G., Benito-Garzón, L., Ramírez-Jiménez, R. A., Guadilla, Y., Gonzalez-Rubio, J., Abradelo, C., et al. (2021). Biomimetic gradient scaffolds containing hyaluronic acid and Sr/Zn folates for osteochondral tissue engineering. Polym. (Basel) 14 (1), 12. doi:10.3390/polym14010012
Bianchi, V. J., Lee, A., Anderson, J., Parreno, J., Theodoropoulos, J., Backstein, D., et al. (2019). Redifferentiated chondrocytes in fibrin gel for the repair of articular cartilage lesions. Am. J. Sports Med. 47 (10), 2348–2359. doi:10.1177/0363546519857571
Bothe, F., Deubel, A. K., Hesse, E., Lotz, B., Groll, J., Werner, C., et al. (2019). Treatment of focal cartilage defects in minipigs with zonal chondrocyte/mesenchymal progenitor cell constructs. Int. J. Mol. Sci. 20 (3), 653. doi:10.3390/ijms20030653
Boushell, M. K., Mosher, C. Z., Suri, G. K., Doty, S. B., Strauss, E. J., Hunziker, E. B., et al. (2019). Polymeric mesh and insulin-like growth factor 1 delivery enhance cell homing and graft-cartilage integration. Ann. N. Y. Acad. Sci. 1442 (1), 138–152. doi:10.1111/nyas.14054
Bozkurt, M., Aşık, M. D., Gürsoy, S., Türk, M., Karahan, S., Gümüşkaya, B., et al. (2019). Autologous stem cell-derived chondrocyte implantation with bio-targeted microspheres for the treatment of osteochondral defects. J. Orthop. Surg. Res. 14 (1), 394. doi:10.1186/s13018-019-1434-0
Browe, D. C., Díaz-Payno, P. J., Freeman, F. E., Schipani, R., Burdis, R., Ahern, D. P., et al. (2022). Bilayered extracellular matrix derived scaffolds with anisotropic pore architecture guide tissue organization during osteochondral defect repair. Acta Biomater. 143, 266–281. doi:10.1016/j.actbio.2022.03.009
Burdis, R., Chariyev-Prinz, F., Browe, D. C., Freeman, F. E., Nulty, J., McDonnell, E. E., et al. (2022). Spatial patterning of phenotypically distinct microtissues to engineer osteochondral grafts for biological joint resurfacing. Biomaterials 289, 121750. doi:10.1016/j.biomaterials.2022.121750
Cai, Z., Tang, Y., Wei, Y., Wang, P., and Zhang, H. (2022). Double - network hydrogel based on exopolysaccharides as a biomimetic extracellular matrix to augment articular cartilage regeneration. Acta Biomater. 152, 124–143. doi:10.1016/j.actbio.2022.08.062
Cao, R., Xu, Y., Xu, Y., Brand, D. D., Zhou, G., Xiao, K., et al. (2022). Development of tri-layered biomimetic atelocollagen scaffolds with interfaces for osteochondral tissue engineering. Adv. Healthc. Mater 11 (11), e2101643. doi:10.1002/adhm.202101643
Cao, R., Zhan, A., Ci, Z., Wang, C., She, Y., Xu, Y., et al. (2021). A biomimetic biphasic scaffold consisting of decellularized cartilage and decalcified bone matrixes for osteochondral defect repair. Front. Cell Dev. Biol. 9, 639006. doi:10.3389/fcell.2021.639006
Chen, L., Deng, C., Li, J., Yao, Q., Chang, J., Wang, L., et al. (2019a). 3D printing of a lithium-calcium-silicate crystal bioscaffold with dual bioactivities for osteochondral interface reconstruction. Biomaterials 196, 138–150. doi:10.1016/j.biomaterials.2018.04.005
Chen, L., Shi, Y., Zhang, X., Hu, X., Shao, Z., Dai, L., et al. (2019b). CaAlg hydrogel containing bone morphogenetic protein 4-enhanced adipose-derived stem cells combined with osteochondral mosaicplasty facilitated the repair of large osteochondral defects. Knee Surg. Sports Traumatol. Arthrosc. 27 (11), 3668–3678. doi:10.1007/s00167-019-05418-1
Chen, T., Bai, J., Tian, J., Huang, P., Zheng, H., and Wang, J. (2018). A single integrated osteochondral in situ composite scaffold with a multi-layered functional structure. Colloids Surf. B Biointerfaces 167, 354–363. doi:10.1016/j.colsurfb.2018.04.029
Chen, Y., Chen, Y., Xiong, X., Cui, R., Zhang, G., Wang, C., et al. (2022). Hybridizing gellan/alginate and thixotropic magnesium phosphate-based hydrogel scaffolds for enhanced osteochondral repair. Mater Today Bio 14, 100261. doi:10.1016/j.mtbio.2022.100261
Cho, H., Kim, J., Kim, S., Jung, Y. C., Wang, Y., Kang, B. J., et al. (2020). Dual delivery of stem cells and insulin-like growth factor-1 in coacervate-embedded composite hydrogels for enhanced cartilage regeneration in osteochondral defects. J. Control Release 327, 284–295. doi:10.1016/j.jconrel.2020.08.002
Critchley, S., Sheehy, E. J., Cunniffe, G., Diaz-Payno, P., Carroll, S. F., Jeon, O., et al. (2020). 3D printing of fibre-reinforced cartilaginous templates for the regeneration of osteochondral defects. Acta Biomater. 113, 130–143. doi:10.1016/j.actbio.2020.05.040
Cunniffe, G. M., Díaz-Payno, P. J., Sheehy, E. J., Critchley, S. E., Almeida, H. V., Pitacco, P., et al. (2019). Tissue-specific extracellular matrix scaffolds for the regeneration of spatially complex musculoskeletal tissues. Biomaterials 188, 63–73. doi:10.1016/j.biomaterials.2018.09.044
Custers, R. J., Creemers, L. B., Verbout, A. J., van Rijen, M. H., Dhert, W. J., and Saris, D. B. (2007). Reliability, reproducibility and variability of the traditional histologic/histochemical grading system vs the new OARSI osteoarthritis cartilage histopathology assessment system. Osteoarthr. Cartil. 15 (11), 1241–1248. doi:10.1016/j.joca.2007.04.017
Dai, Y., Shen, T., Ma, L., Wang, D., and Gao, C. (2018). Regeneration of osteochondral defects in vivo by a cell-free cylindrical poly(lactide-co-glycolide) scaffold with a radially oriented microstructure. J. Tissue Eng. Regen. Med. 12 (3), e1647–e1661. doi:10.1002/term.2592
Daly, A. C., Pitacco, P., Nulty, J., Cunniffe, G. M., and Kelly, D. J. (2018). 3D printed microchannel networks to direct vascularisation during endochondral bone repair. Biomaterials 162, 34–46. doi:10.1016/j.biomaterials.2018.01.057
Ding, X., Gao, J., Yu, X., Shi, J., Chen, J., Yu, L., et al. (2022). 3D-Printed porous scaffolds of hydrogels modified with TGF-β1 binding peptides to promote in vivo cartilage regeneration and animal gait restoration. ACS Appl. Mater Interfaces 14 (14), 15982–15995. doi:10.1021/acsami.2c00761
Du, Y., Liu, H., Yang, Q., Wang, S., Wang, J., Ma, J., et al. (2017). Selective laser sintering scaffold with hierarchical architecture and gradient composition for osteochondral repair in rabbits. Biomaterials 137, 37–48. doi:10.1016/j.biomaterials.2017.05.021
Duan, P., Pan, Z., Cao, L., Gao, J., Yao, H., Liu, X., et al. (2019). Restoration of osteochondral defects by implanting bilayered poly(lactide-co-glycolide) porous scaffolds in rabbit joints for 12 and 24 weeks. J. Orthop. Transl. 19, 68–80. doi:10.1016/j.jot.2019.04.006
Fang, J., Liao, J., Zhong, C., Lu, X., and Ren, F. (2022). High-strength, biomimetic functional chitosan-based hydrogels for full-thickness osteochondral defect repair. ACS Biomater. Sci. Eng. 8 (10), 4449–4461. doi:10.1021/acsbiomaterials.2c00187
Favreau, H., Pijnenburg, L., Seitlinger, J., Fioretti, F., Keller, L., Scipioni, D., et al. (2020). Osteochondral repair combining therapeutics implant with mesenchymal stem cells spheroids. Nanomedicine 29, 102253. doi:10.1016/j.nano.2020.102253
Feng, X., Xu, P., Shen, T., Zhang, Y., Ye, J., and Gao, C. (2020a). Age-related regeneration of osteochondral and tibial defects by a fibrin-based construct in vivo. Front. Bioeng. Biotechnol. 8, 404. doi:10.3389/fbioe.2020.00404
Feng, X., Xu, P., Shen, T., Zhang, Y., Ye, J., and Gao, C. (2020b). Influence of pore architectures of silk fibroin/collagen composite scaffolds on the regeneration of osteochondral defects in vivo. J. Mater Chem. B 8 (3), 391–405. doi:10.1039/c9tb01558b
Filová, E., Tonar, Z., Lukášová, V., Buzgo, M., Litvinec, A., Rampichová, M., et al. (2020). Hydrogel containing anti-CD44-labeled microparticles, guide bone tissue formation in osteochondral defects in rabbits. Nanomater. (Basel) 10 (8), 1504. doi:10.3390/nano10081504
Fu, N., Liao, J., Lin, S., Sun, K., Tian, T., Zhu, B., et al. (2016). PCL-PEG-PCL film promotes cartilage regeneration in vivo. Cell Prolif. 49 (6), 729–739. doi:10.1111/cpr.12295
Gan, D., Wang, Z., Xie, C., Wang, X., Xing, W., Ge, X., et al. (2019). Mussel-inspired tough hydrogel with in situ nanohydroxyapatite mineralization for osteochondral defect repair. Adv. Healthc. Mater 8 (22), e1901103. doi:10.1002/adhm.201901103
Gao, J., Ding, X., Yu, X., Chen, X., Zhang, X., Cui, S., et al. (2021). Cell-Free bilayered porous scaffolds for osteochondral regeneration fabricated by continuous 3D-printing using nascent physical hydrogel as ink. Adv. Healthc. Mater 10 (3), e2001404. doi:10.1002/adhm.202001404
Gardner, O. F., Juneja, S. C., Whetstone, H., Nartiss, Y., Sieker, J. T., Veillette, C., et al. (2019). Effective repair of articular cartilage using human pluripotent stem cell-derived tissue. Eur. Cell Mater 38, 215–227. doi:10.22203/ecm.v038a15
Gong, L., Li, J., Zhang, J., Pan, Z., Liu, Y., Zhou, F., et al. (2020). An interleukin-4-loaded bi-layer 3D printed scaffold promotes osteochondral regeneration. Acta Biomater. 117, 246–260. doi:10.1016/j.actbio.2020.09.039
Grogan, S. P., Barbero, A., Winkelmann, V., Rieser, F., Fitzsimmons, J. S., O'Driscoll, S., et al. (2006). Visual histological grading system for the evaluation of in vitro-generated neocartilage. Tissue Eng. 12 (8), 2141–2149. doi:10.1089/ten.2006.12.2141
Gu, X., Zha, Y., Li, Y., Chen, J., Liu, S., Du, Y., et al. (2022). Integrated polycaprolactone microsphere-based scaffolds with biomimetic hierarchy and tunable vascularization for osteochondral repair. Acta Biomater. 141, 190–197. doi:10.1016/j.actbio.2022.01.021
Gupta, V., Lyne, D. V., Laflin, A. D., Zabel, T. A., Barragan, M., Bunch, J. T., et al. (2017). Microsphere-based osteochondral scaffolds carrying opposing gradients of decellularized cartilage and demineralized bone matrix. ACS Biomater. Sci. Eng. 3 (9), 1955–1963. doi:10.1021/acsbiomaterials.6b00071
He, A., Liu, L., Luo, X., Liu, Y., Liu, Y., Liu, F., et al. (2017). Repair of osteochondral defects with in vitro engineered cartilage based on autologous bone marrow stromal cells in a swine model. Sci. Rep. 7, 40489. doi:10.1038/srep40489
Hsieh, Y. H., Shen, B. Y., Wang, Y. H., Lin, B., Lee, H. M., and Hsieh, M. F. (2018). Healing of osteochondral defects implanted with biomimetic scaffolds of poly(ε-caprolactone)/hydroxyapatite and glycidyl-methacrylate-modified hyaluronic acid in a minipig. Int. J. Mol. Sci. 19 (4), 1125. doi:10.3390/ijms19041125
Huang, Y., Fan, H., Gong, X., Yang, L., and Wang, F. (2021). Scaffold with natural calcified cartilage zone for osteochondral defect repair in minipigs. Am. J. Sports Med. 49 (7), 1883–1891. doi:10.1177/03635465211007139
Idaszek, J., Costantini, M., Karlsen, T. A., Jaroszewicz, J., Colosi, C., Testa, S., et al. (2019). 3D bioprinting of hydrogel constructs with cell and material gradients for the regeneration of full-thickness chondral defect using a microfluidic printing head. Biofabrication 11 (4), 044101. doi:10.1088/1758-5090/ab2622
Jia, S., Wang, J., Zhang, T., Pan, W., Li, Z., He, X., et al. (2018). Multilayered scaffold with a compact interfacial layer enhances osteochondral defect repair. ACS Appl. Mater Interfaces 10 (24), 20296–20305. doi:10.1021/acsami.8b03445
Jiang, G., Li, S., Yu, K., He, B., Hong, J., Xu, T., et al. (2021). A 3D-printed PRP-GelMA hydrogel promotes osteochondral regeneration through M2 macrophage polarization in a rabbit model. Acta Biomater. 128, 150–162. doi:10.1016/j.actbio.2021.04.010
Jiang, X., Liu, J., Liu, Q., Lu, Z., Zheng, L., Zhao, J., et al. (2018). Therapy for cartilage defects: functional ectopic cartilage constructed by cartilage-simulating collagen, chondroitin sulfate and hyaluronic acid (CCH) hybrid hydrogel with allogeneic chondrocytes. Biomater. Sci. 6 (6), 1616–1626. doi:10.1039/c8bm00354h
Katagiri, H., Mendes, L. F., and Luyten, F. P. (2017). Definition of a critical size osteochondral knee defect and its negative effect on the surrounding articular cartilage in the rat. Osteoarthr. Cartil. 25 (9), 1531–1540. doi:10.1016/j.joca.2017.05.006
Khanmohammadi, M., Golshahi, H., Saffarian, Z., Montazeri, S., Khorasani, S., and Kazemnejad, S. (2019). Repair of osteochondral defects in rabbit knee using menstrual blood stem cells encapsulated in fibrin glue: a good stem cell candidate for the treatment of osteochondral defects. Tissue Eng. Regen. Med. 16 (3), 311–324. doi:10.1007/s13770-019-00189-9
Kim, J. K., Bae, H. C., Ro, D. H., Lee, S., Lee, M. C., and Han, H. S. (2021). Enhancement of cartilage regeneration of synovial stem cells/hydrogel by using transglutaminase-4. Tissue Eng. Part A 27 (11-12), 761–770. doi:10.1089/ten.tea.2020.0271
Kondo, M., Kameishi, S., Kim, K., Metzler, N. F., Maak, T. G., Hutchinson, D. T., et al. (2021). Safety and efficacy of human juvenile chondrocyte-derived cell sheets for osteochondral defect treatment. NPJ Regen. Med. 6 (1), 65. doi:10.1038/s41536-021-00173-9
Korthagen, N. M., Brommer, H., Hermsen, G., Plomp, S. G. M., Melsom, G., Coeleveld, K., et al. (2019). A short-term evaluation of a thermoplastic polyurethane implant for osteochondral defect repair in an equine model. Vet. J. 251, 105340. doi:10.1016/j.tvjl.2019.105340
Kostešić, P., Vukasović Barišić, A., Erjavec, I., Pušić, M., Hudetz, D., Matičić, D., et al. (2022). Characterisation of subchondral bone repair following transplantation of bioreactor-manufactured autologous osteochondral graft in a sheep model. Eur. Cell Mater 43, 79–97. doi:10.22203/ecm.v043a08
Kumai, T., Yui, N., Yatabe, K., Sasaki, C., Fujii, R., Takenaga, M., et al. (2019). <p>A novel, self-assembled artificial cartilage&ndash;hydroxyapatite conjugate for combined articular cartilage and subchondral bone repair: histopathological analysis of cartilage tissue engineering in rat knee joints</p>. Int. J. Nanomedicine 14, 1283–1298. doi:10.2147/ijn.s193963
Kumbhar, J. V., Jadhav, S. H., Bodas, D. S., Barhanpurkar-Naik, A., Wani, M. R., Paknikar, K. M., et al. (2017). In vitro and in vivo studies of a novel bacterial cellulose-based acellular bilayer nanocomposite scaffold for the repair of osteochondral defects. Int. J. Nanomedicine 12, 6437–6459. doi:10.2147/ijn.s137361
Lamparelli, E. P., Casagranda, V., Pressato, D., Maffulli, N., Della Porta, G., and Bellini, D. (2022b). Synthesis and characterization of a novel composite scaffold based on hyaluronic acid and equine type I collagen. Pharmaceutics 14 (9), 1752. doi:10.3390/pharmaceutics14091752
Lamparelli, E. P., Ciardulli, M. C., Giudice, V., Scala, P., Vitolo, R., Dale, T. P., et al. (2022a). 3D in-vitro cultures of human bone marrow and Wharton's jelly derived mesenchymal stromal cells show high chondrogenic potential. Front. Bioeng. Biotechnol. 10, 986310. doi:10.3389/fbioe.2022.986310
Lamparelli, E. P., Lovecchio, J., Ciardulli, M. C., Giudice, V., Dale, T. P., Selleri, C., et al. (2021). Chondrogenic commitment of human bone marrow mesenchymal stem cells in a perfused collagen hydrogel functionalized with hTGF-β1-Releasing PLGA microcarrier. Pharmaceutics 13 (3), 399. doi:10.3390/pharmaceutics13030399
Li, Y., Li, L., Li, Y., Feng, L., Wang, B., Wang, M., et al. (2023). Enhancing cartilage repair with optimized supramolecular hydrogel-based scaffold and pulsed electromagnetic field. Bioact. Mater 22, 312–324. doi:10.1016/j.bioactmat.2022.10.010
Li, Z., Wu, N., Cheng, J., Sun, M., Yang, P., Zhao, F., et al. (2020). Biomechanically, structurally and functionally meticulously tailored polycaprolactone/silk fibroin scaffold for meniscus regeneration. Theranostics 10 (11), 5090–5106. doi:10.7150/thno.44270
Liao, J., Tian, T., Shi, S., Xie, X., Ma, Q., Li, G., et al. (2017). The fabrication of biomimetic biphasic CAN-PAC hydrogel with a seamless interfacial layer applied in osteochondral defect repair. Bone Res. 5, 17018. doi:10.1038/boneres.2017.18
Lin, D., Cai, B., Wang, L., Cai, L., Wang, Z., Xie, J., et al. (2020). A viscoelastic PEGylated poly(glycerol sebacate)-based bilayer scaffold for cartilage regeneration in full-thickness osteochondral defect. Biomaterials 253, 120095. doi:10.1016/j.biomaterials.2020.120095
Lin, H., Beck, A. M., Shimomura, K., Sohn, J., Fritch, M. R., Deng, Y., et al. (2019). Optimization of photocrosslinked gelatin/hyaluronic acid hybrid scaffold for the repair of cartilage defect. J. Tissue Eng. Regen. Med. 13 (8), 1418–1429. doi:10.1002/term.2883
Liu, B., Zhao, Y., Zhu, T., Gao, S., Ye, K., Zhou, F., et al. (2020b). Biphasic double-network hydrogel with compartmentalized loading of bioactive glass for osteochondral defect repair. Front. Bioeng. Biotechnol. 8, 752. doi:10.3389/fbioe.2020.00752
Liu, Q., Yu, Y., Reisdorf, R. L., Qi, J., Lu, C. K., Berglund, L. J., et al. (2019). Engineered tendon-fibrocartilage-bone composite and bone marrow-derived mesenchymal stem cell sheet augmentation promotes rotator cuff healing in a non-weight-bearing canine model. Biomaterials 192, 189–198. doi:10.1016/j.biomaterials.2018.10.037
Liu, S., Jia, Y., Yuan, M., Guo, W., Huang, J., Zhao, B., et al. (2017). Repair of osteochondral defects using human umbilical cord wharton's jelly-derived mesenchymal stem cells in a rabbit model. Biomed. Res. Int. 2017, 1–12. doi:10.1155/2017/8760383
Liu, X., Wei, Y., Xuan, C., Liu, L., Lai, C., Chai, M., et al. (2020a). A biomimetic biphasic osteochondral scaffold with layer-specific release of stem cell differentiation inducers for the reconstruction of osteochondral defects. Adv. Healthc. Mater 9, e2000076. doi:10.1002/adhm.202000076
Liu, Z., Nan, H., Chiou, Y. S., Zhan, Z., Lobie, P. E., and Hu, C. (2023). Selective Formation of osteogenic and vasculogenic tissues for cartilage regeneration. Adv. Healthc. Mater 12 (5), e2202008. doi:10.1002/adhm.202202008
Lu, J., Shen, X., Sun, X., Yin, H., Yang, S., Lu, C., et al. (2018). Increased recruitment of endogenous stem cells and chondrogenic differentiation by a composite scaffold containing bone marrow homing peptide for cartilage regeneration. Theranostics 8 (18), 5039–5058. doi:10.7150/thno.26981
Luo, M., Chen, M., Bai, J., Chen, T., He, S., Peng, W., et al. (2022). A bionic composite hydrogel with dual regulatory functions for the osteochondral repair. Colloids Surf. B Biointerfaces 219, 112821. doi:10.1016/j.colsurfb.2022.112821
Ma, F., Ge, Y., Liu, N., Pang, X., Shen, X., and Tang, B. (2019). In situ fabrication of a composite hydrogel with tunable mechanical properties for cartilage tissue engineering. J. Mater Chem. B 7 (15), 2463–2473. doi:10.1039/c8tb01331d
Mainil-Varlet, P., Aigner, T., Brittberg, M., Bullough, P., Hollander, A., Hunziker, E., et al. (2003). Histological assessment of cartilage repair: a report by the histology endpoint committee of the international cartilage repair society (ICRS). J. Bone Jt. Surg. Am. 85 (85-A Suppl. 2), 45–57. doi:10.2106/00004623-200300002-00007
Mainil-Varlet, P., Van Damme, B., Nesic, D., Knutsen, G., Kandel, R., and Roberts, S. (2010). A new histology scoring system for the assessment of the quality of human cartilage repair: ICRS II. Am. J. Sports Med. 38 (5), 880–890. doi:10.1177/0363546509359068
Mendes, L. F., Bosmans, K., Van Hoven, I., Viseu, S. R., Maréchal, M., and Luyten, F. P. (2020). Developmental engineering of living implants for deep osteochondral joint surface defects. Bone 139, 115520. doi:10.1016/j.bone.2020.115520
Mendes, L. F., Katagiri, H., Tam, W. L., Chai, Y. C., Geris, L., Roberts, S. J., et al. (2018). Advancing osteochondral tissue engineering: bone morphogenetic protein, transforming growth factor, and fibroblast growth factor signaling drive ordered differentiation of periosteal cells resulting in stable cartilage and bone formation in vivo. Stem Cell Res. Ther. 9 (1), 42. doi:10.1186/s13287-018-0787-3
Ming, L., Zhipeng, Y., Fei, Y., Feng, R., Jian, W., Baoguo, J., et al. (2018). Microfluidic-based screening of resveratrol and drug-loading PLA/Gelatine nano-scaffold for the repair of cartilage defect. Artif. Cells Nanomed Biotechnol. 46 (Suppl. 1), 336–346. doi:10.1080/21691401.2017.1423498
Mohan, N., Mohanan, P. V., Sabareeswaran, A., and Nair, P. (2017). Chitosan-hyaluronic acid hydrogel for cartilage repair. Int. J. Biol. Macromol. 104 (Pt B), 1936–1945. doi:10.1016/j.ijbiomac.2017.03.142
Moojen, D. J., Saris, D. B., Auw Yang, K. G., Dhert, W. J., and Verbout, A. J. (2002). The correlation and reproducibility of histological scoring systems in cartilage repair. Tissue Eng. 8 (4), 627–634. doi:10.1089/107632702760240544
Murata, D., Akieda, S., Misumi, K., and Nakayama, K. (2018). Osteochondral regeneration with a scaffold-free three-dimensional construct of adipose tissue-derived mesenchymal stromal cells in pigs. Tissue Eng. Regen. Med. 15 (1), 101–113. doi:10.1007/s13770-017-0091-9
Murata, D., Ishikawa, S., Sunaga, T., Saito, Y., Sogawa, T., Nakayama, K., et al. (2022). Osteochondral regeneration of the femoral medial condyle by using a scaffold-free 3D construct of synovial membrane-derived mesenchymal stem cells in horses. BMC Vet. Res. 18 (1), 53. doi:10.1186/s12917-021-03126-y
Nguyen, T. T., Hu, C. C., Sakthivel, R., Nabilla, S. C., Huang, Y. W., Yu, J., et al. (2022). Preparation of gamma poly-glutamic acid/hydroxyapatite/collagen composite as the 3D-printing scaffold for bone tissue engineering. Biomater. Res. 26 (1), 21. doi:10.1186/s40824-022-00265-7
Nie, X., Chuah, Y. J., He, P., and Wang, D. A. (2019). Engineering a multiphasic, integrated graft with a biologically developed cartilage-bone interface for osteochondral defect repair. J. Mater Chem. B 7 (42), 6515–6525. doi:10.1039/c9tb00822e
Nie, X., Yang, J., Chuah, Y. J., Zhu, W., Peck, Y., He, P., et al. (2020). Full-scale osteochondral regeneration by sole graft of tissue-engineered hyaline cartilage without Co-engraftment of subchondral bone substitute. Adv. Healthc. Mater 9 (2), e1901304. doi:10.1002/adhm.201901304
Nordberg, R. C., Huebner, P., Schuchard, K. G., Mellor, L. F., Shirwaiker, R. A., Loboa, E. G., et al. (2021). The evaluation of a multiphasic 3D-bioplotted scaffold seeded with adipose derived stem cells to repair osteochondral defects in a porcine model. J. Biomed. Mater Res. B Appl. Biomater. 109 (12), 2246–2258. doi:10.1002/jbm.b.34886
O'Driscoll, S. W., Keeley, F. W., and Salter, R. B. (1986). The chondrogenic potential of free autogenous periosteal grafts for biological resurfacing of major full-thickness defects in joint surfaces under the influence of continuous passive motion. An experimental investigation in the rabbit. J. Bone Jt. Surg. Am. 68 (7), 1017–1035. doi:10.2106/00004623-198668070-00008
Orth, P., Cucchiarini, M., Kaul, G., Ong, M. F., Gräber, S., Kohn, D. M., et al. (2012b). Temporal and spatial migration pattern of the subchondral bone plate in a rabbit osteochondral defect model. Osteoarthr. Cartil. 20 (10), 1161–1169. doi:10.1016/j.joca.2012.06.008
Orth, P., Zurakowski, D., Wincheringer, D., and Madry, H. (2012a). Reliability, reproducibility, and validation of five major histological scoring systems for experimental articular cartilage repair in the rabbit model. Tissue Eng. Part C Methods 18 (5), 329–339. doi:10.1089/ten.tec.2011.0462
Oshima, T., Nakase, J., Toratani, T., Numata, H., Takata, Y., Nakayama, K., et al. (2019). A scaffold-free allogeneic construct from adipose-derived stem cells regenerates an osteochondral defect in a rabbit model. Arthroscopy 35 (2), 583–593. doi:10.1016/j.arthro.2018.08.033
Park, I. S., Jin, R. L., Oh, H. J., Truong, M. D., Choi, B. H., Park, S. H., et al. (2019). Sizable scaffold-free tissue-engineered articular cartilage construct for cartilage defect repair. Artif. Organs 43 (3), 278–287. doi:10.1111/aor.13329
Pascual-Garrido, C., Aisenbrey, E. A., Rodriguez-Fontan, F., Payne, K. A., Bryant, S. J., and Goodrich, L. R. (2019). Photopolymerizable injectable cartilage mimetic hydrogel for the treatment of focal chondral lesions: a proof of concept study in a rabbit animal model. Am. J. Sports Med. 47 (1), 212–221. doi:10.1177/0363546518808012
Peng, L., Zhou, Y., Lu, W., Zhu, W., Li, Y., Chen, K., et al. (2019). Characterization of a novel polyvinyl alcohol/chitosan porous hydrogel combined with bone marrow mesenchymal stem cells and its application in articular cartilage repair. BMC Musculoskelet. Disord. 20 (1), 257. doi:10.1186/s12891-019-2644-7
Pérez-Silos, V., Moncada-Saucedo, N. K., Peña-Martínez, V., Lara-Arias, J., Marino-Martínez, I. A., Camacho, A., et al. (2019). A cellularized biphasic implant based on a bioactive silk fibroin promotes integration and tissue organization during osteochondral defect repair in a porcine model. Int. J. Mol. Sci. 20 (20), 5145. doi:10.3390/ijms20205145
Petrovova, E., Tomco, M., Holovska, K., Danko, J., Kresakova, L., Vdoviakova, K., et al. (2021). PHB/CHIT scaffold as a promising biopolymer in the treatment of osteochondral defects-an experimental animal study. Polym. (Basel) 13 (8), 1232. doi:10.3390/polym13081232
Qi, Y., Zhang, W., Li, G., Niu, L., Zhang, Y., Tang, R., et al. (2020). An oriented-collagen scaffold including Wnt5a promotes osteochondral regeneration and cartilage interface integration in a rabbit model. Faseb J. 34 (8), 11115–11132. doi:10.1096/fj.202000280r
Qiao, Z., Lian, M., Han, Y., Sun, B., Zhang, X., Jiang, W., et al. (2021). Bioinspired stratified electrowritten fiber-reinforced hydrogel constructs with layer-specific induction capacity for functional osteochondral regeneration. Biomaterials 266, 120385. doi:10.1016/j.biomaterials.2020.120385
Qiu, Y. S., Shahgaldi, B. F., Revell, W. J., and Heatley, F. W. (2003). Observations of subchondral plate advancement during osteochondral repair: a histomorphometric and mechanical study in the rabbit femoral condyle. Osteoarthr. Cartil. 11 (11), 810–820. doi:10.1016/s1063-4584(03)00164-x
Ran, J., Fei, Y., Wang, C., Ruan, D., Hu, Y., Zheng, Z., et al. (2021). An off-the-shelf tissue engineered cartilage composed of optimally sized pellets of cartilage progenitor/stem cells. ACS Biomater. Sci. Eng. 7 (3), 881–892. doi:10.1021/acsbiomaterials.9b01863
Rastegar Adib, F., Bagheri, F., and Sharifi, A. M. (2022). Osteochondral regeneration in rabbit using xenograft decellularized ECM in combination with different biological products; platelet-rich fibrin, amniotic membrane extract, and mesenchymal stromal cells. J. Biomed. Mater Res. B Appl. Biomater. 110 (9), 2089–2099. doi:10.1002/jbm.b.35063
Rutgers, M., van Pelt, M. J., Dhert, W. J., Creemers, L. B., and Saris, D. B. (2010). Evaluation of histological scoring systems for tissue-engineered, repaired and osteoarthritic cartilage. Osteoarthr. Cartil. 18 (1), 12–23. doi:10.1016/j.joca.2009.08.009
Ruvinov, E., Tavor Re'em, T., Witte, F., and Cohen, S. (2019). Articular cartilage regeneration using acellular bioactive affinity-binding alginate hydrogel: a 6-month study in a mini-pig model of osteochondral defects. J. Orthop. Transl. 16, 40–52. doi:10.1016/j.jot.2018.08.003
Salonius, E., Muhonen, V., Lehto, K., Järvinen, E., Pyhältö, T., Hannula, M., et al. (2019). Gas-foamed poly(lactide-co-glycolide) and poly(lactide-co-glycolide) with bioactive glass fibres demonstrate insufficient bone repair in lapine osteochondral defects. J. Tissue Eng. Regen. Med. 13 (3), 406–415. doi:10.1002/term.2801
Seong, Y. J., Kang, I. G., Song, E. H., Kim, H. E., and Jeong, S. H. (2017). Calcium phosphate-collagen scaffold with aligned pore channels for enhanced osteochondral regeneration. Adv. Healthc. Mater 6 (24). doi:10.1002/adhm.201700966
Shang, L., Ma, B., Wang, F., Li, J., Shen, S., Li, X., et al. (2020). Nanotextured silk fibroin/hydroxyapatite biomimetic bilayer tough structure regulated osteogenic/chondrogenic differentiation of mesenchymal stem cells for osteochondral repair. Cell Prolif. 53 (11), e12917. doi:10.1111/cpr.12917
Shen, T., Dai, Y., Li, X., Xu, S., Gou, Z., and Gao, C. (2018). Regeneration of the osteochondral defect by a wollastonite and macroporous fibrin biphasic scaffold. ACS Biomater. Sci. Eng. 4 (6), 1942–1953. doi:10.1021/acsbiomaterials.7b00333
Shen, Y., Xu, Y., Yi, B., Wang, X., Tang, H., Chen, C., et al. (2021). Engineering a highly biomimetic chitosan-based cartilage scaffold by using short fibers and a cartilage-decellularized matrix. Biomacromolecules 22 (5), 2284–2297. doi:10.1021/acs.biomac.1c00366
Stefani, R. M., Lee, A. J., Tan, A. R., Halder, S. S., Hu, Y., Guo, X. E., et al. (2020). Sustained low-dose dexamethasone delivery via a PLGA microsphere-embedded agarose implant for enhanced osteochondral repair. Acta Biomater. 102, 326–340. doi:10.1016/j.actbio.2019.11.052
Sun, J., Lyu, J., Xing, F., Chen, R., Duan, X., and Xiang, Z. (2020). A biphasic, demineralized, and Decellularized allograft bone-hydrogel scaffold with a cell-based BMP-7 delivery system for osteochondral defect regeneration. J. Biomed. Mater Res. A 108 (9), 1909–1921. doi:10.1002/jbm.a.36954
Sun, X., Yin, H., Wang, Y., Lu, J., Shen, X., Lu, C., et al. (2018). In situ articular cartilage regeneration through endogenous reparative cell homing using a functional bone marrow-specific scaffolding system. ACS Appl. Mater Interfaces 10 (45), 38715–38728. doi:10.1021/acsami.8b11687
Tamaddon, M., Blunn, G., Tan, R., Yang, P., Sun, X., Chen, S. M., et al. (2022). In vivo evaluation of additively manufactured multi-layered scaffold for the repair of large osteochondral defects. Biodes Manuf. 5 (3), 481–496. doi:10.1007/s42242-021-00177-w
Tani, Y., Sato, M., Maehara, M., Nagashima, H., Yokoyama, M., Yokoyama, M., et al. (2017). The effects of using vitrified chondrocyte sheets on pain alleviation and articular cartilage repair. J. Tissue Eng. Regen. Med. 11 (12), 3437–3444. doi:10.1002/term.2257
Tani, Y., Sato, M., Yokoyama, M., Yokoyama, M., Takahashi, T., Toyoda, E., et al. (2018). Intra-articular administration of EP2 enhances the articular cartilage repair in a rabbit model. J. Tissue Eng. Regen. Med. 12 (11), 2179–2187. doi:10.1002/term.2748
Tian, G., Jiang, S., Li, J., Wei, F., Li, X., Ding, Y., et al. (2021). Cell-free decellularized cartilage extracellular matrix scaffolds combined with interleukin 4 promote osteochondral repair through immunomodulatory macrophages: in vitro and in vivo preclinical study. Acta Biomater. 127, 131–145. doi:10.1016/j.actbio.2021.03.054
Tschon, M., Brogini, S., Parrilli, A., Bertoldi, S., Silini, A., Parolini, O., et al. (2021). Assessment of the in vivo biofunctionality of a biomimetic hybrid scaffold for osteochondral tissue regeneration. Biotechnol. Bioeng. 118 (1), 465–480. doi:10.1002/bit.27584
Wang, C., Liu, G., Zhang, W., Wang, W., Ma, C., Liu, S., et al. (2017). Cartilage oligomeric matrix protein improves in vivo cartilage regeneration and compression modulus by enhancing matrix assembly and synthesis. Colloids Surf. B Biointerfaces 159, 518–526. doi:10.1016/j.colsurfb.2017.08.008
Wang, H. C., Lin, T. H., Chang, N. J., Hsu, H. C., and Yeh, M. L. (2019b). Continuous passive motion promotes and maintains chondrogenesis in autologous endothelial progenitor cell-loaded porous PLGA scaffolds during osteochondral defect repair in a rabbit model. Int. J. Mol. Sci. 20 (2), 259. doi:10.3390/ijms20020259
Wang, H. C., Lin, T. H., Hsu, C. C., and Yeh, M. L. (2021). Restoring osteochondral defects through the differentiation potential of cartilage stem/progenitor cells cultivated on porous scaffolds. Cells 10 (12), 3536. doi:10.3390/cells10123536
Wang, J., Sun, X., Zhang, Z., Wang, Y., Huang, C., Yang, C., et al. (2019a). Silk fibroin/collagen/hyaluronic acid scaffold incorporating pilose antler polypeptides microspheres for cartilage tissue engineering. Mater Sci. Eng. C Mater Biol. Appl. 94, 35–44. doi:10.1016/j.msec.2018.09.017
Wang, K. H., Wan, R., Chiu, L. H., Tsai, Y. H., Fang, C. L., Bowley, J. F., et al. (2018a). Effects of collagen matrix and bioreactor cultivation on cartilage regeneration of a full-thickness critical-size knee joint cartilage defects with subchondral bone damage in a rabbit model. PLoS One 13 (5), e0196779. doi:10.1371/journal.pone.0196779
Wang, T., Xu, W., Zhao, X., Bai, B., Hua, Y., Tang, J., et al. (2022). Repair of osteochondral defects mediated by double-layer scaffolds with natural osteochondral-biomimetic microenvironment and interface. Mater Today Bio 14, 100234. doi:10.1016/j.mtbio.2022.100234
Wang, Z., Han, L., Sun, T., Ma, J., Sun, S., Ma, L., et al. (2020). Extracellular matrix derived from allogenic decellularized bone marrow mesenchymal stem cell sheets for the reconstruction of osteochondral defects in rabbits. Acta Biomater. 118, 54–68. doi:10.1016/j.actbio.2020.10.022
Wang, Z., Li, Z., Li, Z., Wu, B., Liu, Y., and Wu, W. (2018b). Cartilaginous extracellular matrix derived from decellularized chondrocyte sheets for the reconstruction of osteochondral defects in rabbits. Acta Biomater. 81, 129–145. doi:10.1016/j.actbio.2018.10.005
Wei, X., Liu, B., Liu, G., Yang, F., Cao, F., Dou, X., et al. (2019). Mesenchymal stem cell-loaded porous tantalum integrated with biomimetic 3D collagen-based scaffold to repair large osteochondral defects in goats. Stem Cell Res. Ther. 10 (1), 72. doi:10.1186/s13287-019-1176-2
Widhiyanto, L., Utomo, D. N., Perbowo, A. P., and Hernugrahanto, K. D. (2020). Macroscopic and histologic evaluation of cartilage regeneration treated using xenogenic biodegradable porous sponge cartilage scaffold composite supplemented with allogenic adipose derived mesenchymal stem cells (ASCs) and secretome: an in vivo experimental study. J. Biomater. Appl. 35 (3), 422–429. doi:10.1177/0885328220934938
Wong, C. C., Chen, C. H., Chiu, L. H., Tsuang, Y. H., Bai, M. Y., Chung, R. J., et al. (2018). Facilitating in vivo articular cartilage repair by tissue-engineered cartilage grafts produced from auricular chondrocytes. Am. J. Sports Med. 46 (3), 713–727. doi:10.1177/0363546517741306
Wu, Y., Yang, Z., Denslin, V., Ren, X., Lee, C. S., Yap, F. L., et al. (2020). Repair of osteochondral defects with predifferentiated mesenchymal stem cells of distinct phenotypic character derived from a nanotopographic platform. Am. J. Sports Med. 48 (7), 1735–1747. doi:10.1177/0363546520907137
Xia, P., Yan, S., Li, G., and Yin, J. (2022). Preparation of assemblable chondral and subchondral bone microtissues for osteochondral tissue engineering. ACS Appl. Mater Interfaces 14 (10), 12089–12105. doi:10.1021/acsami.2c00997
Xiao, S. P., Tang, L. S., Chen, J. Y., Li, Z. T., Cheng, G. H., Chen, Q. Q., et al. (2019). Effect of cross-linked hyaluronate scaffold on cartilage repair: an in vivo study. Orthop. Surg. 11 (4), 679–689. doi:10.1111/os.12508
Xing, D., Liu, W., Li, J. J., Liu, L., Guo, A., Wang, B., et al. (2020). Engineering 3D functional tissue constructs using self-assembling cell-laden microniches. Acta Biomater. 114, 170–182. doi:10.1016/j.actbio.2020.07.058
Xing, J., Peng, X., Li, A., Chen, M., Ding, Y., Xu, X., et al. (2021). Gellan gum/alginate-based Ca-enriched acellular bilayer hydrogel with robust interface bonding for effective osteochondral repair. Carbohydr. Polym. 270, 118382. doi:10.1016/j.carbpol.2021.118382
Xu, D., Cheng, G., Dai, J., and Li, Z. (2021). Bi-Layered composite scaffold for repair of the osteochondral defects. Adv. Wound Care New Rochelle. 10 (8), 401–414. doi:10.1089/wound.2019.1140
Yan, J., Liu, C., Tu, C., Zhang, R., Tang, X., Li, H., et al. (2021). Hydrogel-hydroxyapatite-monomeric collagen type-I scaffold with low-frequency electromagnetic field treatment enhances osteochondral repair in rabbits. Stem Cell Res. Ther. 12 (1), 572. doi:10.1186/s13287-021-02638-6
Yan, W., Xu, X., Xu, Q., Sun, Z., Jiang, Q., and Shi, D. (2020a). Platelet-rich plasma combined with injectable hyaluronic acid hydrogel for porcine cartilage regeneration: a 6-month follow-up. Regen. Biomater. 7 (1), 77–90. doi:10.1093/rb/rbz039
Yan, W., Xu, X., Xu, Q., Sun, Z., Lv, Z., Wu, R., et al. (2020b). An injectable hydrogel scaffold with kartogenin-encapsulated nanoparticles for porcine cartilage regeneration: a 12-month follow-up study. Am. J. Sports Med. 48 (13), 3233–3244. doi:10.1177/0363546520957346
Yang, J., Liu, Y., He, L., Wang, Q., Wang, L., Yuan, T., et al. (2018). Icariin conjugated hyaluronic acid/collagen hydrogel for osteochondral interface restoration. Acta Biomater. 74, 156–167. doi:10.1016/j.actbio.2018.05.005
Ye, K., Traianedes, K., Robins, S. A., Choong, P. F. M., and Myers, D. E. (2018). Osteochondral repair using an acellular dermal matrix-pilot in vivo study in a rabbit osteochondral defect model. J. Orthop. Res. 36 (7), 1919–1928. doi:10.1002/jor.23837
Yin, H., Wang, Y., Sun, X., Cui, G., Sun, Z., Chen, P., et al. (2018). Functional tissue-engineered microtissue derived from cartilage extracellular matrix for articular cartilage regeneration. Acta Biomater. 77, 127–141. doi:10.1016/j.actbio.2018.07.031
Yu, F., Li, M., Yuan, Z., Rao, F., Fang, X., Jiang, B., et al. (2018). Mechanism research on a bioactive resveratrol&ndash;PLA&ndash;gelatin porous nano-scaffold in promoting the repair of cartilage defect. Int. J. Nanomedicine 13, 7845–7858. doi:10.2147/ijn.s181855
Yu, H., Feng, M., Mao, G., Li, Q., Zhang, Z., Bian, W., et al. (2022). Implementation of photosensitive, injectable, interpenetrating, and kartogenin-modified GELMA/PEDGA biomimetic scaffolds to restore cartilage integrity in a full-thickness osteochondral defect model. ACS Biomater. Sci. Eng. 8 (10), 4474–4485. doi:10.1021/acsbiomaterials.2c00445
Yucekul, A., Ozdil, D., Kutlu, N. H., Erdemli, E., Aydin, H. M., and Doral, M. N. (2017). Tri-layered composite plug for the repair of osteochondral defects: in vivo study in sheep. J. Tissue Eng. 8, 204173141769750. doi:10.1177/2041731417697500
Zhai, C., Fei, H., Hu, J., Wang, Z., Xu, S., Zuo, Q., et al. (2018). Repair of articular osteochondral defects using an integrated and biomimetic trilayered scaffold. Tissue Eng. Part A 24 (21-22), 1680–1692. doi:10.1089/ten.tea.2018.0086
Zhang, T., Zhang, H., Zhang, L., Jia, S., Liu, J., Xiong, Z., et al. (2017). Biomimetic design and fabrication of multilayered osteochondral scaffolds by low-temperature deposition manufacturing and thermal-induced phase-separation techniques. Biofabrication 9 (2), 025021. doi:10.1088/1758-5090/aa7078
Zhang, W., Zhang, Y., Zhang, A., Ling, C., Sheng, R., Li, X., et al. (2021). Enzymatically crosslinked silk-nanosilicate reinforced hydrogel with dual-lineage bioactivity for osteochondral tissue engineering. Mater Sci. Eng. C Mater Biol. Appl. 127, 112215. doi:10.1016/j.msec.2021.112215
Zhang, Y., Feng, G., Xu, G., and Qi, Y. (2019). Microporous acellular extracellular matrix combined with adipose-derived stem cell sheets as a promising tissue patch promoting articular cartilage regeneration and interface integration. Cytotherapy 21 (8), 856–869. doi:10.1016/j.jcyt.2019.02.005
Zhang, Y., Han, Y., Peng, Y., Lei, J., and Chang, F. (2022). Bionic biphasic composite scaffolds with osteochondrogenic factors for regeneration of full-thickness osteochondral defects. Biomater. Sci. 10 (7), 1713–1723. doi:10.1039/d2bm00103a
Zhang, Y., Liu, S., Guo, W., Wang, M., Hao, C., Gao, S., et al. (2018). Human umbilical cord Wharton's jelly mesenchymal stem cells combined with an acellular cartilage extracellular matrix scaffold improve cartilage repair compared with microfracture in a caprine model. Osteoarthr. Cartil. 26 (7), 954–965. doi:10.1016/j.joca.2018.01.019
Zhao, Z., Li, J., Bai, X., Wang, Y., Wang, Q., Lv, N., et al. (2022). Microfracture augmentation with direct in situ radial shockwave stimulation with appropriate energy has comparable repair performance with tissue engineering in the porcine osteochondral defect model. Am. J. Sports Med. 50 (13), 3660–3670. doi:10.1177/03635465221125936
Zhou, F., Zhang, X., Cai, D., Li, J., Mu, Q., Zhang, W., et al. (2017). Silk fibroin-chondroitin sulfate scaffold with immuno-inhibition property for articular cartilage repair. Acta Biomater. 63, 64–75. doi:10.1016/j.actbio.2017.09.005
Zhu, S., Chen, P., Chen, Y., Li, M., Chen, C., and Lu, H. (2020). 3D-Printed extracellular matrix/polyethylene glycol diacrylate hydrogel incorporating the anti-inflammatory phytomolecule honokiol for regeneration of osteochondral defects. Am. J. Sports Med. 48 (11), 2808–2818. doi:10.1177/0363546520941842
Zhu, W., Guo, D., Peng, L., Chen, Y. F., Cui, J., Xiong, J., et al. (2017). Repair of rabbit cartilage defect based on the fusion of rabbit bone marrow stromal cells and Nano-HA/PLLA composite material. Artif. Cells Nanomed Biotechnol. 45 (1), 115–119. doi:10.3109/21691401.2016.1138482
Zhu, X., Chen, T., Feng, B., Weng, J., Duan, K., Wang, J., et al. (2018). Biomimetic bacterial cellulose-enhanced double-network hydrogel with excellent mechanical properties applied for the osteochondral defect repair. ACS Biomater. Sci. Eng. 4 (10), 3534–3544. doi:10.1021/acsbiomaterials.8b00682
Keywords: osteochondral repair, tissue engineering, osteochondral grafts, histological assessment, histological scoring system
Citation: Cui M, Sun Y, Zhang X, Yang P and Jiang W (2024) Osteochondral tissue engineering in translational practice: histological assessments and scoring systems. Front. Bioeng. Biotechnol. 12:1434323. doi: 10.3389/fbioe.2024.1434323
Received: 17 May 2024; Accepted: 15 July 2024;
Published: 02 August 2024.
Edited by:
Valentina Giudice, University of Salerno, ItalyReviewed by:
Erwin Pavel Lamparelli, University of Salerno, ItalyZhen Yang, Peking University People’s Hospital, China
Copyright © 2024 Cui, Sun, Zhang, Yang and Jiang. This is an open-access article distributed under the terms of the Creative Commons Attribution License (CC BY). The use, distribution or reproduction in other forums is permitted, provided the original author(s) and the copyright owner(s) are credited and that the original publication in this journal is cited, in accordance with accepted academic practice. No use, distribution or reproduction is permitted which does not comply with these terms.
*Correspondence: Weibo Jiang, amlhbmd3ZWlib0BqbHUuZWR1LmNu