- 1Leibniz University Hannover, Institute of Continuum Mechanics, Hannover, Germany
- 2Reutlingen University, Faculty of Life Sciences, Reutlingen, Germany
- 3TU Dortmund University, Chair of Materials Test Engineering (WPT), Dortmund, Germany
- 4Clinic and Polyclinic for Dermatology and Venerology, University Medical Center Rostock (UMR), Rostock, Germany
- 5Department of Prosthetic Dentistry and Biomedical Materials Science, Hannover Medical School, Hannover, Germany
- 6Additive Manufacturing Department, Laser Zentrum Hannover e.V., Hannover, Germany
- 7Department of Oral and Maxillofacial Surgery, University Hospital Hamburg-Eppendorf, Hamburg, Germany
- 8Department of Oral and Maxillofacial Surgery, Division of Regenerative Orofacial Medicine, University Hospital Hamburg-Eppendorf, Hamburg, Germany
In the field of biomedical implants, additively manufactured titanium alloys, particularly Ti-6Al-4V, hold significant potential due to their biocompatibility and mechanical properties. This study focuses on the characterization and modeling of additively manufactured Ti-6Al-4V alloy for dental and maxillofacial implants, emphasizing fatigue behavior, surface modification, and their combined effects on cyto- and osseocompatibility. Experimental methods, including tensile, compression, and fatigue testing, were applied alongside in silico simulations to assess the long-term mechanical performance of the material. Surface properties were further modified through sandblasting and coating techniques to enhance cell adhesion and proliferation. By using in-vitro methods, the cytocompatibility of the coatings and materials was examined followed by in-vivo tests to determine osseocompatibility. Results demonstrated that appropriate surface roughness and modifications are essential in optimizing osseointegration, while the layer-by-layer additive manufacturing process influenced the fatigue life and stability. These findings contribute to the development of patient-specific implants, optimizing both mechanical integrity and biological integration for enhanced clinical outcomes. This work summarizes the investigations on additively manufactured Ti-6Al-4V alloy of the research unit 5250 “Mechanism-based characterization and modeling of permanent and bioresorbable implants with tailored functionality based on innovative in vivo, in vitro and in silico methods” funded by the Germany Research Foundation (DFG).
1 Introduction
In the field of dentistry and oral and maxillofacial surgery, the implantation of permanent or bioabsorbable implants is the preferred procedure for replacing lost hard tissue due to trauma, tumors, or malformations (Glenske et al., 2018). The success of these procedures relies on a range of factors, beyond the implant itself, including osseointegration and mechanical performance. Biocompatibility of the implant material, which is essential for preventing the body’s immune system from rejecting the implant. Furthermore, the (nano) surface characteristics and the structural topography of the implant surface, in consideration of its overall design, are of critical importance for the realization of osseointegration and the assurance of long-term stability (Parithimarkalaignan and Padmanabhan, 2013). A variety of surface modification techniques have been explored to enhance the performance of titanium alloys, including methods such as chemical etching, anodization, and advanced coatings. Notable examples include the works by Xu et al. (2021) and Xu et al. (2022), which provide innovative approaches to surface engineering of titanium alloys for biomedical applications.
Titanium alloys, such as Ti-6Al-4V, are frequently used in biomedical applications due to their exceptional mechanical properties and biocompatibility (Kotzem et al., 2023; Kotzem et al., 2021; Sidambe, 2014). However, in response to the growing complexity of patient-specific requirements in surgical procedures, additive manufacturing (AM) has emerged as a promising manufacturing process. AM enables the manufacturing of complex geometries, including lattice structures, which can be employed to modify material properties, particularly stiffness (Koju et al., 2022), and to mitigate the risk of stress shielding (Noyama et al., 2012; Niinomi and Nakai, 2011).
To mitigate this effect, lattice structures have been demonstrated to reduce the stiffness of the implant while simultaneously enhancing nutrient transport and vascularization, which are essential for osseointegration and tissue regeneration (Deering and Grandfield, 2021; Pei et al., 2022; Wang et al., 2022). Nevertheless, any reduction in stiffness and modifications to the implant’s shape must be achieved without compromising the functionality. It is therefore essential to investigate the fatigue characteristics of additively manufactured specimens to determine the optimal design (Wegner et al., 2019). The powder bed fusion laser-based process of metal (PBF-LB/M) process inherent to AM has the potential to introduce microstructural anisotropies or residual stresses, which may ultimately impact the fatigue life of the material (Chen et al., 2022; Yasin et al., 2024; Beretta and Romano, 2017; Bologna et al., 2024; Centola et al., 2023). Therefore, a comprehensive evaluation of the fatigue characteristics is essential to guarantee the long-term functionality of the material under cyclic loading conditions.
Following surgical implantations, an undisturbed healing phase is essential for the successful integration of the implant with the surrounding bone matrix growth and tissues. Implant loading conditions must be carefully controlled to prevent overstressing the bone during the early healing period, which could result in implant failure (Staedt et al., 2020). In addition to experimental studies, numerical simulations are crucial for assessing the long-term fatigue behavior of implants by replicating patient-specific loading conditions. These simulations guide focused experiments and optimize the implant design before clinical application.
The surface characteristics of the implant, including micro- and nano-roughness, structural morphology, and chemical composition, are also of critical importance in enhancing osseointegration and promoting bone formation.
Regardless of the material employed, surface topography is of primary importance for successful tissue regeneration and healing (Zhang et al., 2023). Research has demonstrated that the proliferation, differentiation, morphology, and matrix production of osteoblast cells can be markedly enhanced by optimizing surface roughness profiles (Kunzler et al., 2007; Karageoriou and Kaplan, 2005). In particular, surface roughness Ra within the range of 1–2 μm has been demonstrated to be effective in promoting osseointegration, as it closely resembles the natural topography of bone. Moreover, the integration of macro-, micro-, and nanoscale structures can enhance the directionality and temporal control of tissue regeneration at the cellular level (Yadroitsev et al., 2014).
The objective of this paper is to examine the interrelationship between the fatigue characteristics of additively manufactured Ti-6Al-4V alloy, including both experimental and simulation-based studies, surface characteristics, and their collective impact on the cyto- and osseocompatibility. These studies were performed in a team of researchers united in the research unit 5250 “Mechanism-based characterization and modeling of permanent and bioresorbable implants with tailored functionality based on innovative in vivo, in vitro and in silico methods” funded by the Germany Research Foundation (DFG).
2 Methods and materials
This section presents methods for characterizing the properties of Ti-6Al-4V specimens to promote osseointegration. The first section addresses the mechanical properties of additively manufactured Ti-6Al-4V specimens to ensure long-term stability. The second part focuses on the surface properties of various specimens, including as-built specimens with varying roughness levels controlled by additive manufacturing parameters. Additionally, machined specimens with smooth surfaces were prepared by cutting titanium rods, and some of these machined specimens were further modified through sandblasting to create rougher surfaces. Different coating strategies were applied to additively manufactured, machined, and sandblasted specimens to further enhance surface properties and promote osseointegration. This comprehensive approach allowed for the investigation of a wide range of surface roughnesses, textures, and coating effects. The section also examines the interaction between mechanical and biological properties, followed by methods used for biological characterization.
2.1 Manufacturing
2.1.1 Additive manufacturing
The Ti-6Al-4V specimens were additively manufactured via powder bed fusion laser-based process (PBF-LB/M) using the industrial machine Lasertec 12 SLM by DMG MORI at Laser Zentrum Hannover e.V. (LZH, Hannover, Germany). The machine is equipped with a 400 W fiber laser emitting at 1,070 nm in continuous wave mode, featuring a minimum spot diameter of 35 µm. The used powder specification is Ti-6Al-4V Grade 23 (ECKART TLS GmbH, Bitterfeld-Wolfen, Germany) and exhibits a predominantly spherical morphology, with particle sizes ranging from 20.0 to 53.0 µm.
Different shaped specimens were manufactured for the experiments (Figure 1).
a) for the mechanical characterization cylinders for tensile and compression testing
b) for biological experiments, discs (with 12 mm diameter and 2 mm thickness) with three different surface roughnesses (Sa).
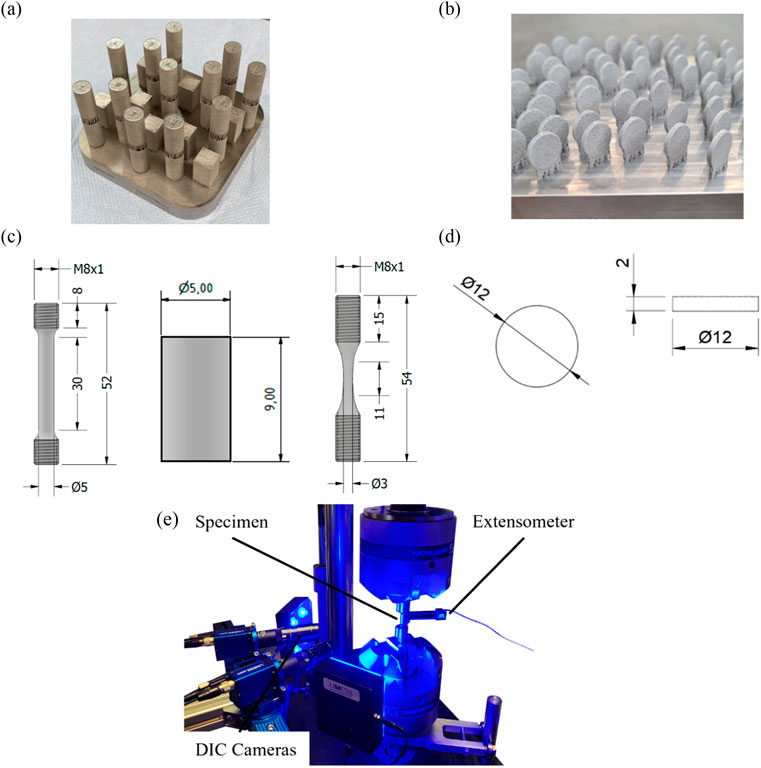
Figure 1. Additive manufacturing build job design of Ti-6Al-4V alloy: (a) Specimens for tensile and compression testing; (b) Discs for cell testing; dimensions of Ti-6Al-4V specimens for (c) tensile, compression and fatigue testing; (d) machined discs; (e) testing setup for investigations of Ti-6Al-4V.
The process parameters are listed in Table 1. All specimens were manufactured in an upright position with a bidirectional scan strategy.
The final dimensions of the specimens can be extracted from Figures 1c, d.
After the manufacturing process and ultrasonic cleaning, additional heat treatment was conducted to reduce manufacturing-related residual stresses and to guarantee a homogeneous and stable microstructure based on ISO 20160. The heat treatment was performed in a vacuum furnace at 1,050°C for 4 h with conventional furnace cooling afterward (Chunze et al., 2021). The surface roughness levels were quantified using a laser-scanning confocal microscope (Keyence VK-X1,000), measuring the arithmetic mean roughness (Sa) over an area of 1.3 mm2 on the side surfaces of the samples. Preliminary investigations showed that the ratio between the roughness parameters Sz (maximum height) and Sa (arithmetic mean roughness) remained consistent across all samples. Based on this observation, Sa was chosen as the representative roughness parameter, as it effectively describes the average surface structure while facilitating consistent comparison between samples. Generally, the surface roughness of additively manufactured parts differs based on a variety of factors. Based on the process capability and the target to examine different surfaces, but using the same equipment, material and design the roughness levels were determined by only varying the process parameters. The three roughness levels represented a progressive increase of 12.52 µm (AMTi12), 14.64 µm (AMTi15) and 17.40 µm (AMTi17) to comprehensively investigate the relationship between surface roughness and biological properties.
2.1.2 Machining and sandblasting
The specimens were cut from a Ø12 mm Ti-6Al-4V rod (L. Klein SA, Biel, Switzerland) using a linear precision saw (Brillant 220, ATM Qness GmbH, Mammelzen, Germany). A diamond cutting disc (Buehler, ITW Test & Measurement GmbH, Leinfelden-Echterdingen, Germany) was used and the specimen thickness was 2 mm. The samples were deburred afterward in a rotary tumbler (Model 45C, Lortone Inc., Meridian, Idaho, United States), resulting in the geometry shown in Figure 1d).
For the sandblasted group, one side of each specimen was sandblasted using a sandblasting machine from a previous study (Finger et al., 2020), following a meander-shaped sandblasting path with a 0.3 mm offset and a travel speed of 1 mm/s, resulting in a sandblasting time of approx. 8 min per specimen. Sandblasting was carried out at an angle of 90° and a distance of 15 mm, with a pressure of 2 bar. After sandblasting, the surface roughness was measured with an optical profilometer (MicroProf 100; FRT GmbH, Bergisch-Gladbach, Germany), and the topography was analyzed using scanning electron microscopy (SEM) (EVO MA10; Carl Zeiss AG, Oberkochen, Germany). Imaging was conducted at × 500 magnification over an area of 450 × 600 μm2, enabling detailed inspection of the surface topography and assessment of microstructural changes.
2.2 Testing setup for quasi-static and fatigue experiments
To characterize the solid Ti-6Al-4V material, quasi-static compression and tensile tests, as well as fatigue loading tests were performed using Instron 8801 (Instron GmbH, Darmstadt, Germany) with load cell of ±100 kN. The specimen geometries used for the tensile, fatigue, and compression tests are illustrated in Figure 1c) and the testing setup in Figure 1e). The strains were measured using an extensometer (Instron GmbH, Darmstadt, Germany) with an initial gauge length of l0 = 12.5 mm with ± 1 mm travel as well as digital image correlation. All tests were performed according to DIN 6892–1 and DIN 50106. Specific quasi-static properties like Young’s modulus, fracture strain, and ultimate strength were determined. Fatigue tests were carried out at a frequency of f = 10 Hz under stress control.
This subsection presents a novel and effective approach for modeling fatigue, addressing the limitations of conventional cycle-by-cycle simulations, which frequently rely on extensive processing times. It presents an overview of the evolution equations that form the foundation of the material model. Thereby, it establishes the theoretical framework for fatigue life assessment and long-term stability of the additively manufactured specimens. The objective of this methodology is to enhance the accuracy of fatigue predictions while significantly reducing computational and experimental costs.
2.2.1 Extended hamilton principle
The damage model for fatigue is founded upon the extended Hamilton principle (Junker and Balzani, 2021). In the isothermal case, the extended Hamilton functional is given by
It can be defined as the summation of functionals, which include the Gibbs energy
The Gibbs energy
can be defined as the difference between the Helmholtz free energy
The dissipation functional is defined as
The remaining term for the current material model accounts of volume conservation, which is included as a constraint. Therefore, the constraint functional denotes
The stationarity of this principle results in field equations for the displacement
In this context of a quasi-static process, dynamic effects are excluded. The stationarity demands due to the independence of the respective test functions
Computing the stationarity and employing integration by parts, we obtain the generalized evolution equation for the internal variables as
It can be evaluated as soon as the dissipation function
2.2.2 Transformation of time t to cycles N
Our aim is the modeling of fatigue. Thus, the generalized evolution equation could be used to model the evolution of microstructure in the course of time. However, this approach would consume a remarkable amount of computational effort which practically prevents the simulation of high-cycle fatigue problems with millions of cycles. Hence, to reduce computational expenses, we implement a coordinate transformation from time t
For any time, derivatives
Then, the stationarity condition for the internal variables from Equation 5 is reformulated
2.2.3 Specification of the material model
For the specific model for fatigue processes, the following internal variables are introduced
As part of the Gibbs energy, the Helmholtz free energy
which include the monotonically decreasing damage function
The dissipation function is a homogeneous function of first and second order, resulting in a differential inequality for the damage function and a differential algebraic system of equations for the plastic strains. The dissipation function is defined as follows:
with
Finally, the stationarity conditions from Equation 5 and Equation 7 constitutes as
where
followed from
2.2.4 Simulation parameter
The in silico fatigue assessment was conducted to improve prediction accuracy compared to traditional methods. The assessment involved a systematic evaluation of the relationship between stress amplitude and fracture cycle count. Stress amplitudes ranging from 350 MPa to 650 MPa were applied to construct S-N (Wöhler) curves systematically. For constructing the S-N curve, individual simulations were conducted for each point on the curve, with different load amplitudes applied at each step. The hourglass shaped specimen (compare Figure 1c) is used for the fatigue simulation. The boundary conditions are applied as shown in Figure 2. These amplitudes are detailed in Supplementary Table S1. The material parameters for Young’s modulus, Poisson’s rate, and dissipation are provided in Table 2.
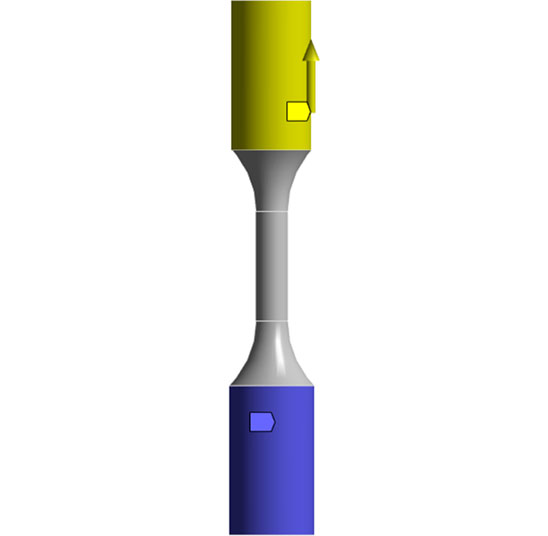
Figure 2. Boundary conditions for the hourglass specimen: fixed boundary conditions at the bottom (blue) and displacement boundary condition at the top (yellow).
The failure cycle,
2.3 Surface modification
2.3.1 Sand-blasting
In Figure 1d discs are used to modify the surface by sand-blasting. One side of each disc was sandblasted using a modified 3D printer with a sandblasting nozzle, following a meander-shaped path with a 0.3 mm offset at 1 mm/s. Sandblasting was performed at 90° and 15 mm distance with pressures of 2 bar and 6 bar, creating two distinct roughness levels. Average surface roughness was measured with an optical profilometer, and topography was assessed via scanning electron microscopy (SEM).
2.3.2 Coating
A polyelectrolyte (PE) multilayer (PEM) coating system was applied for chemical modification of the surface. It was assembled by application of a layer-by-layer technology consisting of alternating deposition of polyanion polyacrylic acid, PAA (100 kDa, 35%wt, Sigma Aldrich, Steinheim, Germany) and polycation poly (allylamine hydrochloride), PAH (120–200 kDa, Life Technologies GmbH, Darmstadt, Germany) adopting the protocol described in (Bradford, 1976). The overall coating composition was (PAA/PAH)5 and the number of five bilayers was selected to ensure that the PEM coating is homogeneous and independent of the type and properties of the substrate (Smith et al., 2011).
For physicochemical characterization, a PEM coating was applied on Si-wafers (10 × 10 mm2, CrysTec GmbH, Berlin, Germany) pre-cleaned by successive ultrasonication in acetone and 2-propanol. For cell culture experiments, the same coating was built in sterile conditions on machined Ti-6Al-4V discs (MTi), sand-blasted Ti-6Al-4V discs (SBTi), and additively manufactured Ti-6Al-4V discs (AMTi) with three different levels (Table 1) of AMTi12, AMTi15 and AMTi17, all cleaned successively in hot (60°C) solution of 10 mM SDS, acetone and 2-propanol.
The (PAA/PAH)5 coating was applied here for chemical modification of the surface. It was assembled by application of the layer-by-layer technology consisting of alternating deposition of polyanion (PAA) and polycation (PAH) adopting the protocol described in (Hartmann et al., 2013). The number of five bilayers was chosen to ensure that the PEM coating is homogeneous and independent of the type and properties of the substrate (Ladam et al., 1999).
The thickness of the coating was measured by ellipsometry (SENTECH Instruments GmbH, Berlin, Germany) in a dry state. The hydrophilicity was analyzed by static water contact angle measurements (DataPhysics Instruments GmbH, Filderstadt, Germany) by applying the Young–Laplace fitting procedure. Atomic force microscopy (AFM) (Oxford Instruments plc, Abingdon, United Kingdom) was utilized for assessing the average surface roughness. The surface density of human serum albumin (HSA) on the coating was analyzed by following the Bradford assay (Bradford, 1976).
The adhesion strength of PEM coatings on MTi and AMTi15 materials was evaluated using an automatic pull-off adhesion tester (PosiTest AT-A, DeFelsko) in accordance with ASTM F1147-05 standards. This method measures the tensile force required to cause adhesive or cohesive failure between two adhesive pull-off cylinders: one AMTi cylinder coated with the PEM coating and one uncoated control cylinder. Both cylinders were bonded using a high-adhesive epoxy glue, with uncoated cylinders serving as controls.
2.4 In vitro cytocompatibility
Cells viability and proliferation on the uncoated and PEM-coated Ti-6Al-4V specimen (machined, sand-blasted and additively manufactured) with different roughness levels were investigated with 4 cell types - L929 fibroblasts (cell lines service GmbH, Eppelheim, Germany, Cat. No.: 400260–817), MC3T3 preosteoblasts (American Type Culture Collection, Virginia, United States, Cat. No.: CRL-2593), human dental pulp stem cells (hDPSCs), and human osteoblasts (NHOst) (Lonza Group AG, Basel, Switzerland, Cat No.: CC-2538) based on previously described protocols (Alkildani et al., 2021; Jung et al., 2020). Extracts from each specimen and control group were incubated for 24 h in the appropriate cell type specific medium under cell culture conditions (37°C, 5% CO2 and 95% humidity). The cells were then incubated with the extracts for a further 24 h and analyzed immediately. The following established methods were employed in order to ascertain the cytocompatibility of these cell types: Lactate dehydrogenase (LDH) activity assays were performed to assess the adhesion and cell growth on PEM-coated versus uncoated titanium and on smoother versus rougher titanium. LDH assay was carried out following the protocol described in detail in (Longhitano et al., 2018). 2,3-bis-(2-methoxy-4-nitro-5-sulfophenyl)-2H-tetrazolium-5-carboxanilide (XTT) reduction assay was performed according to the protocol provided by the manufacturer (Thermo Fisher Scientific, Waltham, Massachusetts, United States).
XTT assay and LDH assay are commonly used methods to assess cytotoxicity and cell viability in biomedical research. The XTT assay measures the metabolic activity of cells by quantifying the reduction of a colorimetric dye, providing an indirect measurement of cell proliferation and viability. In contrast, the LDH assay detects the release of lactate dehydrogenase (LDH), an enzyme presents in the cytoplasm, upon cell membrane damage or lysis, indicating cellular toxicity or damage. Both assays offer valuable insights into the health and functionality of cells when exposed to various treatments or materials, aiding in the evaluation of biocompatibility and potential cytotoxic effects.
The cultivation and differentiation of hDPSCs is considered a stem cell model with potential for osteogenic, chondrogenic, and adipogenic differentiation (Al-Sanabani et al., 2013) (Supplementary Figure S1).
2.5 In vivo biocompatibility
The subcutaneous implantation study was performed via an established methodology (Barbeck et al., 2023; Alkildani et al., 2022; Barbeck et al., 2024). Briefly, the additively manufactured Ti-6Al-4V with three different roughnesses were inserted in a subcutaneous tissue pocket using 30 female 5-week-old Wistar rats (n = 5 animals for 10 and 30 days post implantation) after the approval of the Local Ethical Committee of the Faculty of Medicine at the University of Niš, Serbia, based on the approval of the Veterinary Directorate of the Ministry of Agriculture, Forestry and Water Management of the Republic of Serbia (approval number 323–07-01762/2019–05/9; date of approval: 01 March 2019). Thereby, experimental animals were housed using standard conditions with regular mouse pellets, access to water ad libitum, and an artificial light-dark cycle of 12 h each. After intraperitoneal anesthesia [10 mL of ketamine (50 mg/mL) with 1.6 mL of 2% xylazine] the subcutaneous implantation included the insertion of the Ti-6Al-4V into a preformed subcutaneous pocket within the rostral subscapular region under sterile conditions followed by wound closure with Prolene 6.0 (Ethicon, Somerville, NJ, United States). After the respective study time points, the experimental animals were sacrificed by an overdose of ketamine, xylazine, and the implanted biomaterials were extracted together with the peri-implant tissue and fixated in 4% formalin for 24 h. The explants were embedded in paraffin, cut with a rotation microtome (Leica, Wetzlar, Germany) in 3–5 μm thick slices, and stained with hematoxylin and eosin (H&E). Afterward, the tissue sections were analyzed histologically with a conventional diagnostic microscope (Eclipse 80i, Nikon, Tokyo, Japan) in combination with a digital camera (DS-Fi1, Nikon, Tokyo, Japan) with a digital sight control unit (Nikon, Tokyo, Japan).
3 Results and discussion
3.1 Mechanical behavior
The heat-treated and therefore stress-relieved condition of the specimen shown in Figure 1c) was initially characterized in the case of quasi-static compression and tensile behavior to evaluate the material response in different loading conditions. Figure 3 presents the tensile test results which align with the findings of previous studies (Longhitano et al., 2018; Nes et al., 2022). Focusing on the determined properties, Young’s modulus of E ≈ 110,000 MPa was reached and is comparable to other studies (Nes et al., 2022; Kasperovich and Hausmann, 2015; Tao et al., 2018). Further parameters like fracture strain (FS) were determined with FS ≈ 14.8% while the ultimate tensile strength (UTS) was estimated with UTS ≈ 870 MPa. When comparing the tensile and compression behavior of the same material condition, an increased ultimate compression strength to UCS ≈ −1,250 MPa can be detected. As a result of the compression tests, the elongation at break (FS) also increases up to FS ≈ 24% which is comparable to the literature (Lee et al., 2022).
In addition to quasi-static tensile and compression tests, microstructural analyses were performed to initially characterize the phase distribution after heat treatment. Figure 4a) shows the microstructure of Ti-6Al-4V Grade 23 (ELI) with a homogenous structure. It also indicates an α+β microstructure that can be reached after super-transus heat treatment (Okabe and Hero, 1995).
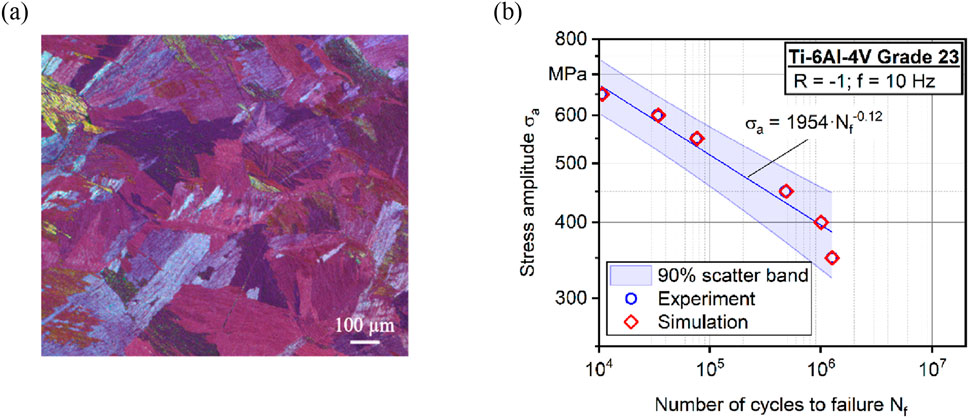
Figure 4. (a) Microstructure after heat treatment and (b) experimental S-N (Woehler) curve of Ti-6Al-4V alloy.
To evaluate the performance under cyclic loading, additional fatigue tests were carried out. Figure 4b) shows the results of constant amplitude tests (CAT) in high cycle fatigue (HCF) regime. The samples exhibit a strong correlation with the Basquin fit, effectively capturing the fatigue behavior of Ti-6Al-4V. This correlation enables an accurate description of the material’s properties under cyclic loading.
Damage models and models for tissue and implant interface are essential for the appropriate design of patient-specific implants. The fatigue results are used for experimental verification of numerical simulations.
3.2 Numerical behavior
The in silico fatigue assessment yielded a significant improvement in prediction accuracy compared to traditional methods. The relationship between stress amplitude and number of cycles to failure was systematically evaluated. The S-N curve shown in Figure 4b) illustrates the fatigue properties as a function of stress amplitude.
At a stress amplitude of 350 MPa, the material withstood approx.
3.3 Surface roughness
3.3.1 Ti-6Al-4V specimens
Surface characteristics of the implanted materials such as chemical composition, surface energy (hydrophilicity), and roughness should be considered to ensure successful and long-term osseointegration (Boyan et al., 2017). Among them, the micro- (<100 μm), submicron (<1 μm), and nanoscale roughness (<100 nm) were shown to have the primary role (Boyan et al., 2003).
Five Ti-6Al-4V specimens with various roughness levels, each with the geometry shown in Figure 1d), were produced and studied: smooth MTi-discs with average Sa of 0.50 µm, SBTi-discs with Sa of 2.06 µm, and three types of AMTi-discs with Sa of 12.52 µm, 14.64 µm and 17.40 µm (Table 1). Si-wafers were used in some cases as a model substrate.
SEM images in Figure 5 demonstrate significant differences in both macro- and microtopography of the Ti-6Al-4V discs substrates. Machined Ti-6Al-4V discs (Figure 5a) display an anisotropically grooved surface. On the contrary, both sandblasted (Figure 5b) and additively manufactured (Figure 5c) samples exhibit a highly rugged and anisotopically irregular surface.
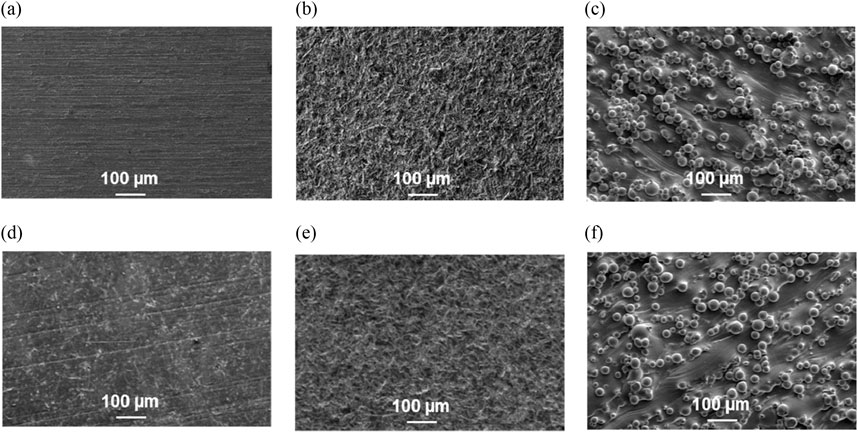
Figure 5. SEM images of (a, d) MTi surface (b, e) SBTi surface (c, f) AMTi15 surface; uncoated (upper row) and PEM coated (lower row).
3.3.2 PEM-coated Ti-6Al-4V specimens
Polyelectrolyte multilayers (PEMs) are assembled through the layer-by-layer deposition of oppositely charged polyelectrolytes, offering a versatile platform for surface modification in biomedical applications. Their tunable physicochemical properties, including thickness, roughness, and mechanical stiffness, can be precisely controlled by adjusting parameters such as pH, ionic strength, and the specific polyelectrolytes used during assembly. This adaptability makes PEMs particularly suitable for tailoring surface characteristics to enhance cellular interactions and promote tissue integration. In the context of titanium-based implants, such as Ti-6Al-4V alloys, surface biofunctionalization is crucial to improve biocompatibility and encourage osseointegration. Among various PEM systems, those composed PAA and PAH have been extensively studied due to their ability to form stable multilayers with controllable properties. The assembly pH significantly influences the film’s characteristics; for instance, PEMs assembled at higher pH values tend to exhibit increased stiffness and promote better cell adhesion compared to those assembled at lower pH levels (Thompson et al., 2005). In tissue engineering, the PAA/PAH films have shown superior cell adhesion properties compared to other PEMs. When assembled at optimal pH levels, these multilayers promote excellent adhesion of fibroblasts and endothelial cells, making them suitable for biomedical applications (Thompson et al., 2005; Hajicharalambous et al., 2009). This enhanced cell compatibility is not universally observed in all PEM systems.
The (PAA/PAH)5 as applied on model Si-wafers is just a few nanometers thin (12.2 ± 2.1 nm) and mildly hydrophilic (static water contact angle 73 ± 2°, Table 3). Consistent with the low thickness of the coating, its average roughness is also minimal (1.7 ± 0.4 nm). AFM images in Supplementary Figure S2 demonstrate the homogeneous and nanostructured topography of the PEM coating. Both the PAH and PAA chains are fully ionized at pH 7.0, which ends up with a strong repulsion between the COO− groups in the PAA chains and NH3+ groups in the PAH chains. This causes the polymer chains to adopt a fully extended conformation in solution, which is retained during surface adsorption, leading to extremely low thickness and roughness of the PAA/PAH films assembled at neutral pH. Applying nano-thin, nano-smooth PEM coating to MTi, SBTi, and AMTi substrates effectively preserves their inherent micromorphology (Figures 5d–f). Coating smooths surface contours, eliminates loosely bound titanium particles from AMTi materials, and enhances the surface chemical homogeneity. The PEM coating exhibits strong adhesion, conforming seamlessly to the Ti surfaces without defects such as scratches or delamination.
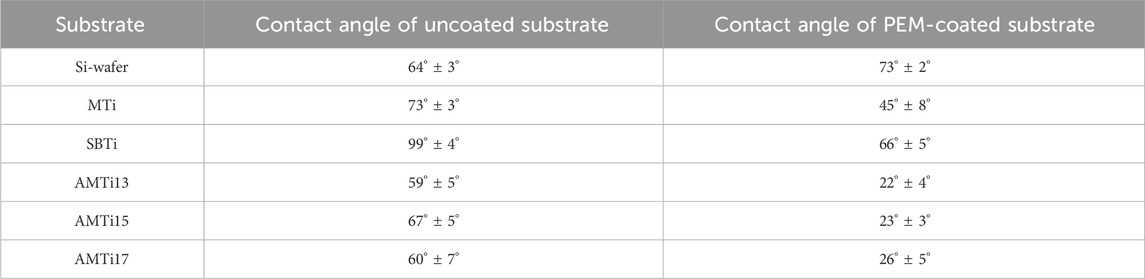
Table 3. Static water contact angles (CA, in degrees) of model Si-wafers and on Ti-6Al-4V discs with five different Sa values, uncoated and coated with (PAA/PAH)5 PEM coating.
It is known that the surface roughness contributes to the material hydrophilicity, therefore we assessed the hydrophilicity of the uncoated MTi, SBTi and AMTi discs with different roughness, as well as the same discs coated with the PEM film by measuring the water contact angle (Table 3, representative contact angle images are presented in Supplementary Figure S3). All uncoated Ti-substrates are moderately hydrophilic, except SBTi, which is slightly hydrophobic, presumably due to modification of the surface chemical composition during sandblasting. Although ultra-thin, the PEM coating significantly enhances the hydrophilicity of all Ti-6Al-4V surfaces without altering their micro-roughness and morphology. The adhesion between a coating and an implant’s surface is paramount among mechanical properties, as it dictates the implant’s performance and functionality. If the coating detaches from the substrate - a phenomenon known as delamination - it can result in implantation failure and compromised long-term stability. To assess this adhesion strength, we employed the pull-off test, which quantifies the force per unit area necessary to separate the coating from its substrate. This method aligns with the ASTM F1147-05 standard, designed to evaluate the adhesion of coatings to solid metal substrates or the internal cohesion of a coating under tension perpendicular to the surface plane.
The tensile load applied to detach the uncoated AMTi cylinder from the control cylinder, also known as adhesive strength, was 18.8 ± 0.1 MPa. The adhesive strength of the PAA/PAH-coated AMTi-cylinder was 19.5 ± 0.2 MPa, higher than that of the uncoated cylinder. The adhesive strength of the uncoated MTi sample was 25.3 ± 2.1 MPa and remained unchanged after the application of PAA/PAH coatings (25.4 ± 2.5 MPa). According to ISO standards, hydroxyapatite coatings applied to solid metal substrates must exhibit a minimum adhesion strength of 15 MPa to be deemed suitable for implant applications. The PEM coating discussed herein demonstrate adhesion strengths that exceeds this requirement, positioning it as promising candidate for application on medical devices.
3.3.3 Effect of roughness and PEM coating on the cell behavior
There is a number of publications on the effect of surface roughness on cell adhesion, proliferation, viability, migration, and differentiation (Celles and dos Reis, 2024; Lu et al., 2020; Metwally et al., 2020). Despite the large amount of data collected, they led to controversial conclusions that might originate from the various ranges of nano- and micro-roughness, topographies, materials, and cell types studied. Here we investigated, on the one hand, the effect of roughness of AMTi-materials at a narrower micro-scale (12 μm, 15 μm, and 17 µm), and on the other hand, the effect of roughness at a much broader nano-to micro-scale (0.5 µm, 2 μm and 15 µm), on cell adhesion and proliferation of different cell types. The effect of surface biofunctionalization with the PAA/PAH coating was also investigated.
3.3.3.1 Micro-scale
A notable aspect of this study is the assessment of both the individual and combined impacts of varying microscale surface roughness (12 μm, 15 μm, and 17 µm) and the application of a nano-thick PAA/PAH coating on the cytotoxicity and cytocompatibility of additively manufactured titanium materials. This was evaluated by studying the viability of L929 fibroblasts, MC3T3 preosteoblasts, and human dental pulp stem cells (hDPSCs). hDPSCs are a relevant model for evaluating osteogenic potential and can differentiate into various cell lineages, including osteoblasts (Awais et al., 2020). The use of hDPSCs offers valuable insights into the interaction between biomaterials and cells which are important for bone regeneration and repair.
The cell cytotoxicity and viability of the 3 cell types studied on AMTi-materials were assessed by LDH and XTT assays. Both assays revealed that all cell types keep their viability on non-coated and PEM-coated AMTi-substrates equal to that on the non-toxic negative control (Figure 6) indicating a robust adherence on the specimens. Different roughness, as well as PAA/PAH coating, have neither an inhibitory effect on cell adhesion or viability nor a toxic effect on the cells.
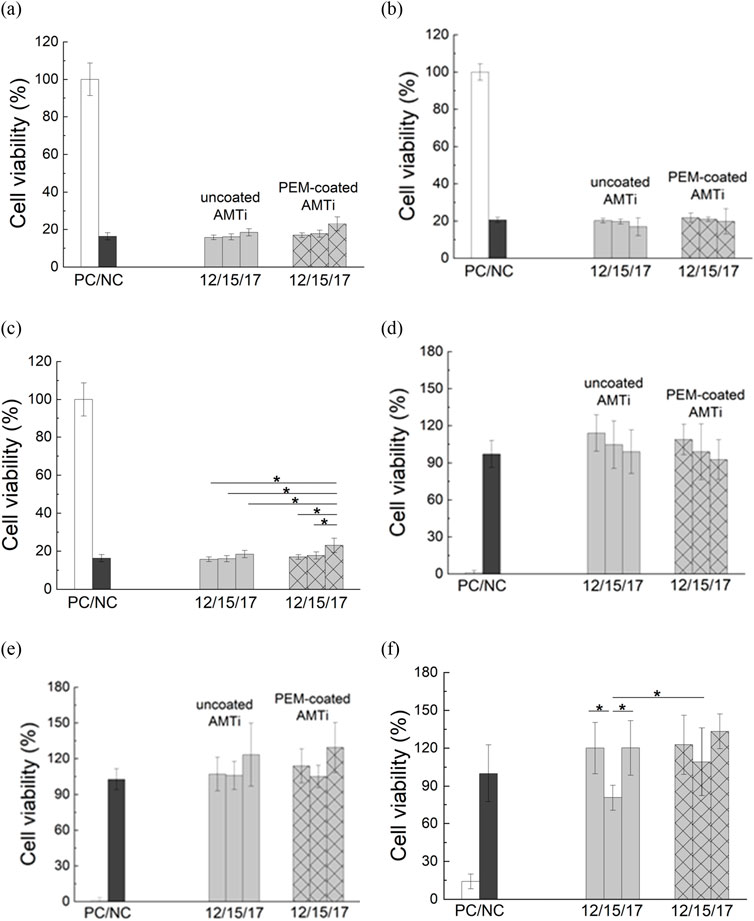
Figure 6. Cell viability of (a, d) L929 murine fibroblast (b, e) MC3T3 preosteoblasts; and (c, f) human dental pulp stem cells (hDPSCs) on toxic positive control (white columns), non-toxic negative control (dark grey columns), non-coated AMTi discs with roughness 12 μm, 15 μm and 17 µm (light gray columns), and PAA/PAH-coated AMTi discs with roughness 12 μm, 15 μm and 17 µm (light gray hatched columns), estimated by LDH assay (a-c) and XTT assay (d-f), (* designates values that are significantly different, p < 0.05).
Taken together, the results from the LDH assay indicate that the samples can be classified as biocompatible according to the cytotoxicity threshold defined in EN ISO 10993–5 (≥70% cell viability). The variation of the surface roughness of additively manufactured Ti-6Al-4V in the range of 12–17 µm has no statistically significant impact on the cytotoxicity of any of the 3 cell types investigated. The XTT assay shows that cell viability, proliferation, and metabolic activity is not significantly influenced either by the variation of surface micro-roughness not by the application of PAA/PAH coating.
Prior studies unite around the statement that osteoblastic cell adhesion, growth, and proliferation are positively correlated to surface roughness (Nöth et al., 1997). Rough surfaces encourage the entrapment of proteins and adhesion of osteogenic cells (Linez-Bataillon et al., 2002). However other studies report a reverse linear correlation between osteoblasts proliferation rate and surface roughness like the one (Boyan et al., 1999; Anselme, 2000). The discrepancies in the various studies may be attributable to differences in the examined cell species and the range of roughness. The results generated in this study refer to specimens with a high macro-topological level (Ra from 12 to 17 µm) therefore comparison with results described in the literature might not be relevant.
3.3.3.2 Submicron-to-micron-scale
A straightforward technique that is frequently used to prepare the surface of dental implants is sandblasting accelerating osteoblast attachment, thus leading to an increase in osseointegration (Vogler, 1998). Here, we used sandblasting as a method to develop surfaces with an intermediate roughness between that of machined and additively manufactured Ti-6Al-4V (see Table 3).
It is evident from Figure 7a) that NHOst adhesion was not affected by the significant increase in surface roughness for either the uncoated or PEM-coated Ti-6Al-4V specimen. It looks like the PEM-coating enhanced the initial cell attachment on the Ti-6Al-4V specimen but statistical analysis revealed that significant increase of osteoblast adhesion (by 40%) is observed only on sandblasted Ti-6Al-4V specimens relative to the uncoated SBTi. As for the proliferation, a dramatical suppression was found for the uncoated SBTi and AMTi compared to the smooth MTi samples. All PEM-coated samples demonstrated the same level of cell viability regardless the surface roughness.
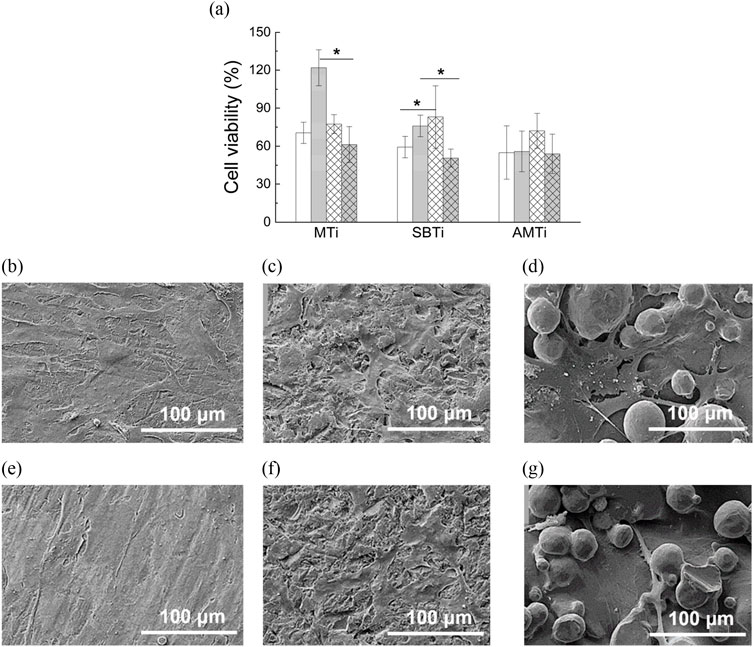
Figure 7. (a) NHOst viability (relative to the positive control) after 24 h of cultivation (white columns), showing the extent of cell adhesion and after 72 h of cultivation (gray columns), showing the extent of cell proliferation, examined on MTi, SBTi and AMTi discs, non-coated (normal bars) and coated with PEM coating (hatched bars) (* designates values that are significantly different, p < 0.05). SEM images of NHOst cultured for 24 h (upper row) and 72 h (bottom row) on Ti-6Al-4V discs with increasing roughness: (b, e) MTi, (c, f) SBTi, and (d, g) AMTi. Magnification 1,200×.
The findings regarding the relationship between surface hydrophilicity and the behavior of osteoblasts are in agreement with the so-called Berg limit (Vogler et al., 1995), which was established in the context of protein adsorption and cell adhesion. This limit states that surfaces lose their ability to bind hydrophilic and hydrophobic proteins when their contact angle falls below 60°–65° (Vogler et al., 1995). The functionalization of MTi and AMTi biomaterials with the PAA/PAH coating lowered the contact angle much below this threshold, thus suppressing the strength of cell adhesion, and consequently the proliferation ability. Uncoated SBTi biomaterial appeared hydrophobic and numerous scientific researches reveal suppressed cell adhesion and spreading on hydrophobic surfaces (Barrias et al., 2009; Glenske et al., 2018). The adsorption of proteins that promote cell adhesion, such as fibronectin and vitronectin, is hampered by hydrophobic surfaces’ generally low surface energy (Barrias et al., 2009). PEM coating increases the hydrophilicity of the originally hydrophobic SBTi substrate, thereby enhancing NHOst adhesion.
Our results on the NHOst behavior on uncoated Ti-6Al-4V specimen with increasing submicron-to-micron roughness align well with the literature. Evidence is accumulating to suggest a favorable cellular response to submicron and nanoscale roughness compared to micron-scale roughness. Alveolar bone osteoblasts cultured on surface-treated zirconia polycrystals with varying submicron average roughness (from 0.3 to 0.8 µm) and morphology showed comparable initial attachment efficiency, regardless of the surface properties (Rabel et al., 2020). Through deep correlation analysis, it was found that proliferation is related not so much to surface roughness as to surface enlargement and texture, with lower surface expansion and surface roughness anisotropy supporting better cell proliferation (Rabel et al., 2020). A systematic decrease in the number of attached cells, as well as proliferation levels, has been reported for MG-63 human osteoblast-like cells grown on Ti-6Al-4V discs with increasing roughness (Martin et al., 1995).
In this study, the SBTi and especially the AMTi are characterized by relatively high surface enlargement and anisotropy of the surface structures, both contributing to the suppression of NHOst proliferation. Regarding the AMTi surfaces, we assume that lateral space limitations restrict the osteoblasts’ ability to adhere effectively and proliferate. As shown in Figures 7b–g), approximately 50% of the AMTi surface is covered with Ti-6Al-4V beads, leaving only the remaining 50% available for the cells.
SEM images reveal that NHOst cells are closely spread and fully flattened on the MTi surface, exhibiting numerous cytoplasmic extensions and filopodia that follow the direction of the grooves (Figures 7b, e). On the SBTi surfaces the cells spread less and in more isotropic directions, developing fewer cytoplasmic extensions compared to the MTi substrates (Figures 7c, f). In contrast, for AMTi, the lack of wide smooth areas causes osteoblasts to be suspended by their filopodia attached to the Ti-6Al-4V beads, resulting in weak adhesion and suppressed proliferation (Figures 7d, e). SEM images of NHOst cells on the same three surfaces functionalized with PAA/PAH coating are not shown because they appear similar to the images presented in Figures 7b–g).
Finally, the in vivo results showed that all the Ti-6Al-4V specimens induced comparable tissue reactions without any differences related to the roughnesses (Figure 8). At day 10 post-implantation, a moderate inflammatory tissue reaction pattern including mainly macrophages at the material-tissue-interfaces and within the surrounding connective tissue beside lower numbers of granulocytes and lymphocytes in concert with a mild vascularization was detectable in all study groups (Figure 8). This reaction pattern especially at this early time point has already been described and seems to be more related to the surgical procedure and the early wound healing processes around the implants. This is even more evident from the tissue reaction on day 30, which shows a flattening of the reaction or a slightly fibrotic tissue reaction with a reduced number of reactive cells in all study groups. However, this has already been shown in the case of other biomaterials and does not indicate either altered biocompatibility or reduced regenerative functionality (Vogler, 1998).
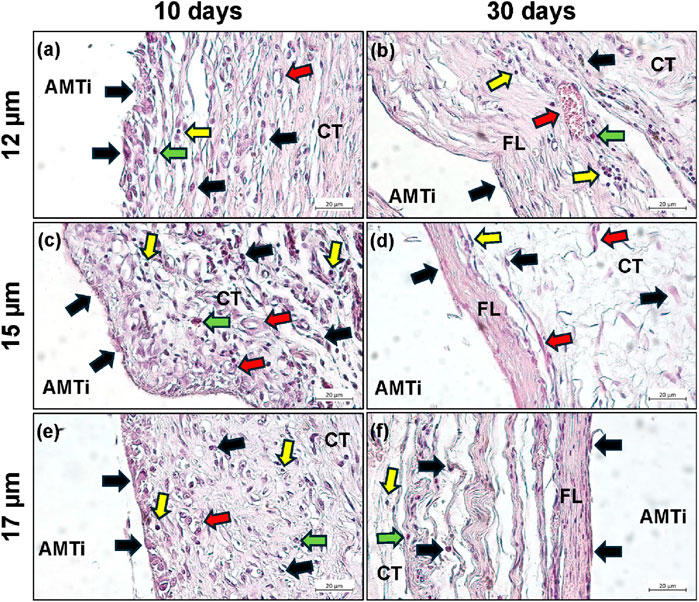
Figure 8. Representative histological microphotographs of the tissue reactions to the additive manufactured Ti-6Al-4V discs (uncoated- AMTi) at day 10 (left column) (a,c,e) and day 30 (b,d,f) post-implantation (right column) within the subcutaneous connective tissue (CT). Black arrows = macrophages, red arrows = blood vessels, green arrows = granulocytes, yellow arrows = lymphocytes (HE-stainings, magnification x400, scalebars = 20 µm).
4 Discussion
4.1 Influence of mechanical properties on implant performance
The investigation of the mechanical properties and surface modifications of Ti-6Al-4V highlights the asymmetry between tensile and compressive behavior, which has been observed in various studies (Suresh et al., 2021). This asymmetry remains crucial and should be further considered in the development of medical implants, especially since the reduced Young’s modulus in the compressive direction could influence the long-term mechanical performance of implants subjected to repeated loading.
The α-β microstructure observed after heat treatment not only confirms previous findings but also emphasizes its role in improving the material’s mechanical properties, such as hardness. This enhancement is particularly important for applications where high strength and wear resistance are critical, such as load-bearing medical implants. The stability and hardness of this microstructure suggest its ability to withstand long-term mechanical stresses in clinical settings. However, future studies should focus on the effects of this microstructure on the long-term stability of implants under various clinical conditions.
The fatigue results confirm the reliability of Ti-6Al-4V for applications requiring long-term cyclic loading, such as in load-bearing implants. Within our research group, models for predicting material damage and understanding the tissue-implant interface are central to designing patient-specific implants. The fatigue properties obtained in this study serve as valuable experimental benchmarks for validating numerical simulations, providing essential insights for improving implant design, durability, and safety in medical applications.
4.2 Fatigue resistance and computational model efficiency
A particularly notable finding in this study is the high computational efficiency of the proposed model. To enhance computational efficiency, we implemented a time-to-cycle transformation, enabling the prediction of high-cycle fatigue behavior without the need for exhaustive cycle-by-cycle simulations. Quasi-static processes were assumed to simplify calculations, with the material model accounting for damage evolution, plastic strain, and hardening effects. Simulations were completed in just 18,024-time steps for a stress amplitude of 350 MPa, significantly reducing the number of time steps compared to the 1,254,380 required in conventional cycle-by-cycle approaches. The simulation results were rigorously validated against experimental S-N curves derived from high-cycle fatigue tests. The predicted fatigue life demonstrated a strong correlation with experimental data, with an error margin of less than 3%. This consistency underscores the reliability of the simulation framework for predicting fatigue behavior under realistic conditions. This efficiency, combined with consistent accuracy, highlights the model’s potential for predicting fatigue behavior under realistic loading conditions in additively manufactured materials. These findings support the application of the model to optimize implant designs, where long-term fatigue performance is critical, enabling precise, reliable predictions while reducing computational demands.
4.3 Surface hydrophilicity and roughness effects
The investigation of the surface hydrophilicity of PEM-coated MTi samples with low surface roughness shows that surface roughness significantly impacts the contact angle, with increasing Ra values enhancing the hydrophilicity of the samples. The sand-blasted Ti-6Al-4V samples are more hydrophobic than the machined samples. The additively manufactured Ti-6Al-4V samples, however, are significantly more hydrophilic than the machined and sand-blasted. According to the Wenzel model accounting for the contribution of the surface roughness component to the contact angle, increasing the roughness exaggerates the hydrophilicity of hydrophilic surfaces with the same chemical composition. Our data on the contact angle of non-coated as well as coated MTi and AMTi agree with the Wenzel rule, the impact of roughness being more pronounced on the PEM-coated samples due to their increased hydrophilicity compared to the non-coated samples (Table 3). However, the sandblasted samples behave somewhat differently and the reason can be found in the changed surface composition of these samples due to the traces of the blasted particles left on the treated surface (Guo et al., 2015). These findings highlight the importance of surface characteristics in modulating material hydrophilicity, a factor crucial for osseointegration in biomedical applications.
4.4 Cellular responses to surface roughness
Prior studies unite around the statement that osteoblastic cell adhesion, growth, and proliferation are positively correlated to surface roughness (Nöth et al., 1997). Rough surfaces encourage the entrapment of proteins and adhesion of osteogenic cells (Linez-Bataillon et al., 2002). However other studies report a reverse linear correlation between osteoblasts proliferation rate and surface roughness like the one (Boyan et al., 1999; Anselme, 2000). The discrepancies in the various studies may be attributable to differences in the examined cell species and the range of roughness. The results generated in this study refer to specimens with a high macro-topological level (Ra from 12 μm to 17 µm) therefore comparison with results described in the literature might not be relevant.
The study’s findings support the notion that surface roughness significantly influences cellular responses on Ti-6Al-4V implants, though effects vary depending on the roughness scale and surface treatment. While sandblasting enhanced initial osteoblast adhesion, it also decreased proliferation, a phenomenon consistent with findings that hydrophobic surfaces and high surface roughness reduce protein adsorption and cell spreading (Rabel et al., 2020; Martin et al., 1995). Furthermore, AMTis highly anisotropic and enlarged surface structures contributed to reduced proliferation, as limited lateral space restricted cell attachment.
Although nanoscale and submicron roughness are widely reported to promote osteogenic cell attachment, the high micro-scale roughness in this study resulted in diminished cell proliferation, highlighting the need for precise control of surface topography.
4.5 Challenges and limitations
Precise manufacturing of patient-specific implants requires advanced technologies such as additive manufacturing, but they are prone to defects and process variability. From a biological perspective, the long-term stability and integration of an implant mostly depend on the biological compatibility and the custom-fit design to match the individual patient’s anatomical requirements. Additionally, the customized implants’ design and manufacturing process is time-consuming, which can be particularly problematic in urgent clinical situations. Nevertheless, the production and development of personalized implants are currently cost-intensive and difficult to scale up. For the authorization process of patient-specific implants, strict regulatory requirements include extensive testing and verification.
4.6 Implications and future directions
Overall, this study highlights how both, the mechanical and surface properties of Ti-6Al-4V implants contribute to their performance in biomedical applications. Mechanical strength, fatigue resistance, and computational efficiency are critical for developing reliable load-bearing implants. Surface characteristics, especially roughness and hydrophilicity, directly influence cell adhesion and proliferation, impacting osseointegration. Future research should continue to investigate these properties to enhance implant safety, durability, and biocompatibility, with an emphasis on refining surface topography for optimal biological response.
5 Conclusions and outlook
This study demonstrated that powder bed fusion laser-based process (PBF-LB/M) Ti-6Al-4V is a viable material for dental and maxillofacial implants, exhibiting strong mechanical performance and excellent fatigue resistance. Quasi-static tests revealed a Young’s modulus of approximately 110 GPa, and tensile strength of 870 MPa, with superior compressive strength of 1,250 MPa. Fatigue life modeling and experimental validation confirmed the material’s long-term stability under cyclic loading. Surface modifications like sandblasting, enhanced cell viability and osseointegration by reducing the roughness values of 12.5 µm–17.4 µm, as observed in in-vitro studies. These findings support the use of Ti-6Al-4V for custom-made implants, ensuring both mechanical reliability and enhanced biological integration for improved patient outcomes.
Applying advanced modeling techniques could further refine predictions of implant longevity, enhancing the reliability and patient specificity of solutions for dental and maxillofacial applications. Future studies should also focus on assessing long-term mechanical stability under physiological loading conditions to ensure optimal clinical safety and effectiveness of patient-specific implants.
Data availability statement
The original contributions presented in the study are publicly available. This data can be found here: https://doi.org/10.25835/6nqow7m4.
Ethics statement
The animal study was approved by Local Ethical Committee of the Faculty of Medicine at the University of Niš, Serbia, based on the approval of the Veterinary Directorate of the Ministry of Agriculture, Forestry and Water Management of the Republic of Serbia (approval number 323–07-01762/2019–05/9. The study was conducted in accordance with the local legislation and institutional requirements.
Author contributions
HK: Conceptualization, Formal Analysis, Methodology, Supervision, Validation, Writing–original draft, Writing–review and editing. TA: Conceptualization, Formal Analysis, Methodology, Resources, Supervision, Writing–original draft, Writing–review and editing. SS: Conceptualization, Data curation, Methodology, Project administration, Validation, Writing–original draft, Writing–review and editing. CR: Conceptualization, Data curation, Methodology, Writing–original draft, Writing–review and editing. OA: Methodology, Validation, Visualization, Writing–original draft, Writing–review and editing. AJ: Methodology, Validation, Writing–original draft, Writing–review and editing. FG: Data curation, Methodology, Writing–original draft, Writing–review and editing. SF: Methodology, Writing–original draft, Writing–review and editing. MT: Methodology, Validation, Writing–review and editing. MS: Data curation, Methodology, Validation, Writing–review and editing. MM: Project administration, Writing–review and editing. AK: Project administration, Writing–review and editing. OJ: Supervision, Writing–review and editing. MB: Writing–review and editing. AG: Formal Analysis, Funding acquisition, Supervision, Writing–review and editing. RS: Formal Analysis, Supervision, Writing–review and editing. JH: Formal Analysis, Funding acquisition, Supervision, Writing–review and editing. RK: Funding acquisition, Supervision, Writing–review and editing. PJ: Formal Analysis, Funding acquisition, Methodology, Supervision, Writing–review and editing. MS: Formal Analysis, Funding acquisition, Supervision, Writing–review and editing. FW: Formal Analysis, Funding acquisition, Project administration, Supervision, Writing–review and editing.
Funding
The author(s) declare that financial support was received for the research, authorship, and/or publication of this article. The authors gratefully acknowledge the funding by the German Research Foundation (Deutsche Forschungsgemeinschaft, DFG) for the research unit 5250 “Mechanism-based characterization and modeling of permanent and bioresorbable implants with tailored functionality based on innovative invivo, in-vitro and in-silico methods” (project no. 449916462).
Conflict of interest
The authors declare that the research was conducted in the absence of any commercial or financial relationships that could be construed as a potential conflict of interest.
Generative AI statement
The authors declare that no Generative AI was used in the creation of this manuscript.
Publisher’s note
All claims expressed in this article are solely those of the authors and do not necessarily represent those of their affiliated organizations, or those of the publisher, the editors and the reviewers. Any product that may be evaluated in this article, or claim that may be made by its manufacturer, is not guaranteed or endorsed by the publisher.
Supplementary material
The Supplementary Material for this article can be found online at: https://www.frontiersin.org/articles/10.3389/fbioe.2025.1526873/full#supplementary-material
References
Alkildani, S., Jung, O., and Barbeck, M. (2021). in vitro investigation of jellyfish collagen as a tool in cell culture and (bone) tissue engineering. Anticancer Res. 41, 707–717. doi:10.21873/anticanres.14822
Alkildani, S., Mandule, A., Radenković, M., Najman, S., Stojanovic, S., Jung, O., et al. (2022). in vivo analysis of the immune response to strontium- and copper-doped bioglasses. vivo. doi:10.20944/preprints202205.0286.v1
Al-Sanabani, J. S., Madfa, A. A., and Al-Sanabani, F. A. (2013). Application of calcium phosphate materials in dentistry. Int. J. Biomaterials 2013, 1–12. doi:10.1155/2013/876132
Anselme, K. (2000). Osteoblast adhesion on biomaterials. Biomaterials 21, 667–681. doi:10.1016/s0142-9612(99)00242-2
Awais, S., Balouch, S. S., Riaz, N., and Choudhery, M. S. (2020). Human dental pulp stem cells exhibit osteogenic differentiation potential. Open Life Sci. 15, 229–236. doi:10.1515/biol-2020-0023
Barbeck, M., Andreeva, T., Krastev, R., Jung, O., and Schneider-Stock, R. (2024). Validation of the chick chorioallantoic membrane (cam) model for biocompatibility analysis of implant materials. Trans. Addit. Manuf. Meets Med. Available online at: https://doi.org/10.18416/AMMM.2024.24091875
Barbeck, M., Pissarek, J., Alkildani, S., Jung, O., and Unger, R. E. (2023). “Biology of resorbable bone substitutes: CaP-Based and polymers,” in Surgical research in implant dentistry. Springer International Publishing. doi:10.1007/978-3-031-37234-6_17
Barrias, C. C., Martins, M. C. L., Almeida-Porada, G., Barbosa, M. A., and Granja, P. L. (2009). The correlation between the adsorption of adhesive proteins and cell behaviour on hydroxyl-methyl mixed self-assembled monolayers. Biomaterials 30, 307–316. doi:10.1016/j.biomaterials.2008.09.048
Beretta, S., and Romano, S. (2017). A comparison of fatigue strength sensitivity to defects for materials manufactured by AM or traditional processes. Int. J. Fatigue 94, 178–191. doi:10.1016/j.ijfatigue.2016.06.020
Blaszczyk, M., Jantos, D. R., and Junker, P. (2022). Application of Taylor series combined with the weighted least square method to thermodynamic topology optimization. Comput. Methods Appl. Mech. Eng. 393, 114698. doi:10.1016/j.cma.2022.114698
Bologna, O., Cecchel, S., Cornacchia, G., Avanzini, A., Sepe, R., Berto, F., et al. (2024). Investigating post-processing impact on fatigue performance of LPBF Ti6Al4V with heat treatment, high pressure heat treatment, and dry electropolishing strategies. Int. J. Fatigue 185, 108365. doi:10.1016/j.ijfatigue.2024.108365
Boyan, B. D., Lotz, E. M., and Schwartz, Z. (2017). *Roughness and hydrophilicity as osteogenic biomimetic surface properties. Tissue Eng. Part A 23, 1479–1489. doi:10.1089/ten.tea.2017.0048
Boyan, B. D., Lotz, E. M., Schwartz, Z., Zhao, G., Lohmann, C., Cochran, D., et al. (2003). Osteoblasts generate an osteogenic microenvironment when grown on surfaces with rough microtopographies. Eur. Cells Mater. 6, 22–27. doi:10.22203/ecm.v006a03
Boyan, B. D., Sylvia, V. L., Yuhong, L., Sagun, R., Cochran, D. L., Lohmann, C. H., et al. (1999). Surface roughness mediates its effects on osteoblasts via protein kinase A and phospholipase A2. Biomaterials 20, 2305–2310. doi:10.1016/s0142-9612(99)00159-3
Bradford, M. M. (1976). A rapid and sensitive method for the quantitation of microgram quantities of protein utilizing the principle of protein-dye binding. Anal. Biochem. 72, 248–254. doi:10.1016/0003-2697(76)90527-3
Celles, C. A. S., and dos Reis, A. C. (2024). Titanium: a systematic review of the relationship between crystallographic profile and cell adhesion. J. Biomed. Mater. Res. Part B Appl. Biomaterials 112, e35450. doi:10.1002/jbm.b.35450
Centola, A., Ciampaglia, A., Tridello, A., and Paolino, D. S. (2023). “Machine learning methods to predict the fatigue life of selectively laser melted Ti6Al4V components,” in Fatigue and fracture of engineering materials and structure. doi:10.1111/ffe.14125
Chen, S., Gao, H., Zhang, Y., Wu, Q., Gao, Z., and Zhou, X. (2022). Review on residual stresses in metal additive manufacturing: formation mechanisms, parameter dependencies, prediction and control approaches. J. Mater. Res. Technol. 17, 2950–2974. doi:10.1016/j.jmrt.2022.02.054
Chunze, Y., Yan, L., Hao, L., Yang, L., Hussein, A. Y., Young, P. G., et al. (2021). Triply periodic minimal surface lattices additively manufactured by selective laser melting-melting a volume in 3D printing technology series. Elsevier. doi:10.1016/c2020-0-01253-3
Deering, J., and Grandfield, K. (2021). Current interpretations on the in vivo response of bone to additively manufactured metallic porous scaffolds: a review. Biomaterials Biosyst. 2, 100013. doi:10.1016/j.bbiosy.2021.100013
Finger, C., Stiesch, M., Eisenburger, M., Breidenstein, B., Busemann, S., and Greuling, A. (2020). Effect of sandblasting on the surface roughness and residual stress of 3Y-TZP (zirconia). SN Appl. Sci. 2, 1700. doi:10.1007/s42452-020-03492-6
Glenske, K., Donkiewicz, P., Köwitsch, A., Milosevic-Oljaca, N., Rider, P., Rofall, S., et al. (2018). Applications of metals for bone regeneration. Int. J. Mol. Sci. 19, 826. doi:10.3390/ijms19030826
Guo, C. Y., Matinlinna, J. P., Tsoi, J. K., and Hong Tang, A. T. (2015). Residual contaminations of silicon-based glass, alumina and aluminum grits on a titanium surface after sandblasting. Silicon 11, 2313–2320. doi:10.1007/s12633-015-9287-6
Hajicharalambous, C. S., Lichter, J., Hix, W. T., Swierczewska, M., Rubner, M. F., and Rajagopalan, P. (2009). Nano- and sub-micron porous polyelectrolyte multilayer assemblies: biomimetic surfaces for human corneal epithelial cells. Biomaterials 30, 4029–4036. doi:10.1016/j.biomaterials.2009.03.020
Hartmann, H., Hossfeld, S., Schlosshauer, B., Mitternacht, U., Pêgo, A. P., Dauner, M., et al. (2013). Hyaluronic acid/chitosan multilayer coatings on neuronal implants for localized delivery of siRNA nanoplexes. J. Control. Release 168, 289–297. doi:10.1016/j.jconrel.2013.03.026
Jung, O., Radenkovic, M., Stojanović, S., Lindner, C., Batinic, M., Görke, O., et al. (2020). in vitro and in vivo biocompatibility analysis of a new transparent collagen-based wound membrane for tissue regeneration in different clinical indications. vivo 34, 2287–2295. doi:10.21873/invivo.12040
Junker, P., and Balzani, D. (2021). An extended Hamilton principle as unifying theory for coupled problems and dissipative microstructure evolution. Continuum Mech. Thermodyn. 33, 1931–1956. doi:10.1007/s00161-021-01017-z
Junker, P., and Wick, T. (2023). Space-time variational material modeling: a new paradigm demonstrated for thermo-mechanically coupled wave propagation, visco-elasticity, elasto-plasticity with hardening, and gradient-enhanced damage. Comput. Mech. 73, 365–402. doi:10.1007/s00466-023-02371-2
Karageoriou, V., and Kaplan, D. (2005). „Porosity of 3D biomaterial scaffolds and osteogenesis. Biomaterials. doi:10.1016/j.biomaterials.2005.02.002
Kasperovich, G., and Hausmann, J. (2015). Improvement of fatigue resistance and ductility of TiAl6V4 processed by selective laser melting. J. Mater. Process. Technol. 220, 202–214. doi:10.1016/j.jmatprotec.2015.01.025
Koju, N., Niraula, S., and Fotovvati, B. (2022). Additively manufactured porous Ti6Al4V for bone implants: a review. Metals 12, 687. doi:10.3390/met12040687
Kotzem, D., Arold, T., Bleicher, K., Raveendran, R., Niendorf, T., and Walther, F. (2023). Ti6Al4V lattice structures manufactured by electron beam powder bed fusion - microstructural and mechanical characterization based on advanced in situ techniques. J. Mater. Res. Technol. 22, 2111–2130. doi:10.1016/j.jmrt.2022.12.075
Kotzem, D., Höffgen, A., Raveendran, R., Stern, F., Möhring, K., and Walther, F. (2021). Position-dependent mechanical characterization of the PBF-EB-manufactured Ti6Al4V alloy. Prog. Addit. Manuf. 7, 249–260. doi:10.1007/s40964-021-00228-9
Kunzler, T. P., Drobek, T., Schuler, M., and Spencer, N. D. (2007). Systematic study of osteoblast and fibroblast response to roughness by means of surface-morphology gradients. Biomaterials 28, 2175–2182. doi:10.1016/j.biomaterials.2007.01.019
Ladam, G., Schaad, P., Voegel, J. C., Schaaf, P., Decher, G., and Cuisinier, F. (1999). In situ determination of the structural properties of initially deposited polyelectrolyte multilayers. Langmuir 16, 1249–1255. doi:10.1021/la990650k
Lee, J., Lee, M., Yeon, S. M., Kang, D., and Jun, T. (2022). Influence of heat treatment and loading direction on compressive deformation behaviour of Ti–6Al–4V ELI fabricated by powder bed fusion additive manufacturing. Mater. Sci. Eng. A 831, 142258. doi:10.1016/j.msea.2021.142258
Linez-Bataillon, P., Monchau, F., Bigerelle, M., and Hildebrand, H. F. (2002). in vitro MC3T3 osteoblast adhesion with respect to surface roughness of Ti6Al4V substrates. Biomol. Eng. 19, 133–141. doi:10.1016/s1389-0344(02)00024-2
Longhitano, G. A., Larosa, M. A., Jardini, A. L., Zavaglia, C., and Ierardi, M. (2018). Correlation between microstructures and mechanical properties under tensile and compression tests of heat-treated Ti-6Al–4 V ELI alloy produced by additive manufacturing for biomedical applications. J. Mater. Process. Technol. 252, 202–210. doi:10.1016/j.jmatprotec.2017.09.022
Lu, A., Gao, Y., Jin, T., Luo, X., Zeng, Q., and Shang, Z. (2020). Effects of surface roughness and texture on the bacterial adhesion on the bearing surface of bio-ceramic joint implants: an in vitro study. Ceram. Int. 46, 6550–6559. doi:10.1016/j.ceramint.2019.11.139
Martin, J. Y., Schwartz, Z., Hummert, T. W., Schraub, D. M., Simpson, J., Lankford, J., et al. (1995). Effect of titanium surface roughness on proliferation, differentiation, and protein synthesis of human osteoblast-like cells (MG63). J. Biomed. Mater. Res. 29, 389–401. doi:10.1002/jbm.820290314
Metwally, S., Ferraris, S., Spriano, S., Krysiak, Z. J., Kaniuk, Ł., Marzec, M. M., et al. (2020). Surface potential and roughness controlled cell adhesion and collagen formation in electrospun PCL fibers for bone regeneration. Mater. and Des. 194, 108915. doi:10.1016/j.matdes.2020.108915
Nes, E. V., Hovig, E. W., Feitosa, L., and Sørby, K. (2022). “Effect of building orientation in mechanical properties of ti6al4v produced with laser powder bed fusion”, in Advanced Manufacturing and Automation XI. Singapore: Springer. doi:10.1007/978-981-19-0572-8_30
Niinomi, M., and Nakai, M. (2011). Titanium-based biomaterials for preventing stress shielding between implant devices and bone. Int. J. Biomaterials 2011, 1–10. doi:10.1155/2011/836587
Nöth, U., Hendrich, C., Scheddin, D., Altvater, T., Eulert, J., and Thull, R. (1997). Der Effekt von Titanoberflächen unterschiedlicher Rauhigkeit auf Zellproliferation, Zelldifferenzierung und Proteinsynthese humaner fetaler Osteoblasten (hFOB 1.19). Biomed. Tech. Berl. 42, 367–368. doi:10.1515/bmte.1997.42.s2.367
Noyama, Y., Miura, T., Ishimoto, T., Itaya, T., Niinomi, M., and Nakano, T. (2012). Bone loss and reduced bone quality of the human femur after total hip arthroplasty under stress-shielding effects by titanium-based implant. Mater. Trans. 53, 565–570. doi:10.2320/matertrans.m2011358
Okabe, T., and Hero, H. (1995). The use of titanium in dentistry. Cells Mater. Available online at: https://api.semanticscholar.org/CorpusID:59476954
Parithimarkalaignan, S., and Padmanabhan, T. V. (2013). Osseointegration: an update. J Indian Prosthodont Soc 13 (1):2–6. doi:10.1007/s13191-013-0252-z
Pei, X., Wang, L., Zhou, C., Wu, L., Lei, H., Fan, S., et al. (2022). Ti6Al4V orthopedic implant with biomimetic heterogeneous structure via 3D printing for improving osteogenesis. Mater. and Des. 221, 110964. doi:10.1016/j.matdes.2022.110964
Rabel, K., Kohal, R., Steinberg, T., Tomakidi, P., Rolauffs, B., Adolfsson, E., et al. (2020). Controlling osteoblast morphology and proliferation via surface micro-topographies of implant biomaterials. Sci. Rep. 10, 12810. doi:10.1038/s41598-020-69685-6
Sidambe, A. (2014). Biocompatibility of advanced manufactured titanium implants - a review. Materials 7, 8168–8188. doi:10.3390/ma7128168
Smith, S. M., Wunder, M. B., Norris, D. A., and Shellman, Y. G. (2011). A simple protocol for using a LDH-based cytotoxicity assay to assess the effects of death and growth inhibition at the same time. Plos One 6, e26908. doi:10.1371/journal.pone.0026908
Staedt, H., Rossa, M., Lehmann, K. M., Al-Nawas, B., Kämmerer, P. W., and Heimes, D. (2020). Potential risk factors for early and late dental implant failure: a retrospective clinical study on 9080 implants. Int. J. Implant Dent. 6, 81. doi:10.1186/s40729-020-00276-w
Suresh, S., Sun, C.-N., Tekumalla, S., Rosa, V., Ling Nai, S. M., and Wong, R. C. W. (2021). Mechanical properties and in vitro cytocompatibility of dense and porous Ti–6Al–4V ELI manufactured by selective laser melting technology for biomedical applications. J. Mech. Behav. Biomed. Mater. 123, 104712. doi:10.1016/j.jmbbm.2021.104712
Tao, P., Li, H.-X., Huang, B.-Y., Hu, Q.-D., Gong, S.-L., and Xu, Q.-Y. (2018). Tensile behavior of Ti-6Al-4V alloy fabricated by selective laser melting: effects of microstructures and as-built surface quality. China Foundry 15, 243–252. doi:10.1007/s41230-018-8064-8
Thompson, M. T., Berg, M. C., Tobias, I. S., Rubner, M. F., and Van Vliet, K. J. (2005). Tuning compliance of nanoscale polyelectrolyte multilayers to modulate cell adhesion. Biomaterials 26, 6836–6845. doi:10.1016/j.biomaterials.2005.05.003
Vogel, A., and Junker, P. (2020). Adaptive and highly accurate numerical treatment for a gradient-enhanced brittle damage model. Int. J. Numer. Methods Eng. 121, 3108–3131. doi:10.1002/nme.6349
Vogler, E. A. (1998). Structure and reactivity of water at biomaterial surfaces. Adv. Colloid Interface Sci. 74, 69–117. doi:10.1016/S0001-8686(97)00040-7
Vogler, E. A., Graper, J. C., Sugg, H. W., Lander, L. M., and Brittain, W. J. (1995). Contact activation of the plasma coagulation cascade. II. Protein adsorption to procoagulant surfaces. J. Biomed. Mater. Res. 29, 1017–1028. doi:10.1002/jbm.820290814
Wang, Z., Zhang, M., Liu, Z., Wang, Y., Dong, W., Zhao, S., et al. (2022). Biomimetic design strategy of complex porous structure based on 3D printing Ti-6Al-4V scaffolds for enhanced osseointegration. Mater. and Des. 218, 110721. doi:10.1016/j.matdes.2022.110721
Wegner, N., Kotzem, D., Wessarges, Y., Emminghaus, N., Hoff, C., Tenkamp, J., et al. (2019). Corrosion and corrosion fatigue properties of additively manufactured magnesium alloy WE43 in comparison to titanium alloy Ti-6Al-4V in physiological environment. Materials 12, 2892. doi:10.3390/ma12182892
Xu, C., Chen, L., Zheng, C., Zhang, H., Zhao, C., Wang, Z., et al. (2021). Improved wear and corrosion resistance of microarc oxidation coatings on Ti–6Al–4V alloy with ultrasonic assistance for potential biomedical applications. Adv. Eng. Mater. 23. doi:10.1002/adem.202001433
Xu, C., Chen, L., Zheng, C., Zhang, Z., Li, R., Yang, H., et al. (2022). Bioactive performances of surface modification of Ti–6Al–4V jointly using ultrasonic-assisted microarc oxidation and hydrothermal treatment. Adv. Eng. Mater. 24. doi:10.1002/adem.202200674
Yadroitsev, I., Krakhmalev, P., and Yadroitsava, I. (2014). Selective laser melting of Ti6Al4V alloy for biomedical applications: temperature monitoring and microstructural evolution. J. Alloys Compd. 583, 404–409. doi:10.1016/j.jallcom.2013.08.183
Yasin, M. S., Stonaker, K., Shao, S., and Shamsaei, N. (2024). Mechanical performance of laser powder bed fused Ti-6Al-4V: the influence of filter condition and part location. Addit. Manuf. Lett. 11, 100255. doi:10.1016/j.addlet.2024.100255
Keywords: additive manufacturing, Ti-6Al-4V alloy, osseointegration, dental implants, surface modification, fatigue behavior, finite element method
Citation: Kök HI, Andreeva T, Stammkötter S, Reinholdt C, Akbas O, Jahn A, Gamon F, Fuest S, Teschke M, Schäfer M, Müller M, Koch A, Jung O, Barbeck M, Greuling A, Smeets R, Hermsdorf J, Krastev R, Junker P, Stiesch M and Walther F (2025) Characterization and modeling of additively manufactured Ti-6Al-4V alloy with modified surfaces for medical applications. Front. Bioeng. Biotechnol. 13:1526873. doi: 10.3389/fbioe.2025.1526873
Received: 12 November 2024; Accepted: 24 February 2025;
Published: 07 April 2025.
Edited by:
Mona Kamal Marei, Alexandria University, EgyptReviewed by:
Ahmed El-Fiqi, National Research Centre, EgyptLiang-Yu Chen, Jiangsu University of Science and Technology, China
Copyright © 2025 Kök, Andreeva, Stammkötter, Reinholdt, Akbas, Jahn, Gamon, Fuest, Teschke, Schäfer, Müller, Koch, Jung, Barbeck, Greuling, Smeets, Hermsdorf, Krastev, Junker, Stiesch and Walther. This is an open-access article distributed under the terms of the Creative Commons Attribution License (CC BY). The use, distribution or reproduction in other forums is permitted, provided the original author(s) and the copyright owner(s) are credited and that the original publication in this journal is cited, in accordance with accepted academic practice. No use, distribution or reproduction is permitted which does not comply with these terms.
*Correspondence: Hüray Ilayda Kök, a29la0Bpa20udW5pLWhhbm5vdmVyLmRl