- 1Department of Informatics, Bioengineering, Robotics and System Engineering, University of Genova, Genova, Italy
- 2University School for Advanced Studies (IUSS), Pavia, Italy
- 3Department of Electrical and Electronic Engineering, University of Cagliari, Cagliari, Italy
In this work, we present an alternative system to standard microelectrode arrays for monitoring the electrical activity of 3D cellular aggregates such as neurospheroids, which are known to better replicate the complex architecture and cellular interactions of native neural tissue than 2D cultures. The system is based on an ultra-sensitive organic sensor called Organic Charge-Modulated Field Effect Transistor (OCMFET) fabricated through low-resolution, low-cost fabrication techniques. This peculiar organic charge sensor offers interesting features like the absence of a reference electrode in the culture medium, a direct charge amplification, mechanical flexibility, and optical transparency. As a preliminary validation, the OCMFET system has been coupled to rtTA/Ngn2-positive human induced pluripotent stem cell (hiPSC)-derived neurospheroids and was able to reliably detect their spontaneous electrical activity exhibiting a high SNR. This preliminary validation lays the foundation for the development of simple, low-cost, and ultra-flexible organic transistor-based systems for high-performing, reliable interfacing with 3D neuronal structures.
1 Introduction
Investigating neural tissue function and dynamics is essential for understanding brain behavior and developing effective treatments for neurological disorders. Although traditional methodologies, such as standard planar cell cultures, have significantly advanced our understanding of mechanisms at the cellular and network levels, these two-dimensional (2D) models often fail to replicate the complex architecture and multicellular interactions characteristic of native neural tissue.
The emergence of three-dimensional (3D) neural cell culture models, particularly brain organoids and neurospheroids, has significantly advanced in vitro research (Zhuang et al., 2018). These models more accurately represent the in vivo environment of the neural tissue, recapitulating key characteristics, such as tissue architecture, cell organization, and both cell-cell and cell-matrix interactions. For example, cells within neurospheroids have been shown to exhibit enhanced expression of proteins that are particularly crucial for neural development and function, and generally display a more mature phenotype compared to their 2D counterparts (Simão et al., 2018; Camp et al., 2015; Jung et al., 2013).
The development of efficient methods for generating neurospheroids has further expanded their research applications. For instance, the hanging-drop technique enables the scaffold-free formation of uniform spheroidal aggregates without the need for bioreactors. This approach allows for the parallel culture of multiple neurospheroids within the same plate, reducing sample-to-sample variability and facilitating high-throughput studies (Durens et al., 2020). To effectively monitor cellular activity in these 3D cellular models, various technologies have been utilized and developed in the last years. For example, standard microelectrode arrays (MEAs), typically used for planar cultures, have been widely employed to record the electrical activity of brain organoids and neurospheroids (Muzzi et al., 2023; Jordan et al., 2024), while particular 3D MEAs have been specifically designed to interface with these complex 3D structures (Spanu et al., 2020; Yadav et al., 2023; Zips et al., 2023; Yang et al., 2024). However, MEAs passively record extracellular signals, requiring external amplification circuitry and the presence of a reference electrode, which limits system miniaturization.
Recently, organic field-effect transistors (OFETs) have emerged as a promising alternative for bioelectronic interfaces, both in vivo and in vitro (Spanu et al., 2021; Gu et al., 2019; Xie et al., 2020; Kyndiah et al., 2023). These devices exploit the unique properties of organic materials, such as flexibility, biocompatibility, and low-cost fabrication, and can be manufactured using low-temperature processes such as spin coating, inkjet printing, and screen printing (Forrest, 2004). However, conventional OFET-based biosensors integrate sensing and amplification within the same semiconductor layer, making them susceptible to environmental variations and reducing long-term stability. In this context, organic charge-modulated field-effect transistors (OCMFETs) offer distinct advantages over other MEAs and standard OFETs. Their operation relies on the modulation of the transistor channel conductivity induced by the presence of a charge on the surface of the sensing area, which can be read out as a variation of the threshold voltage of the device. Another key advantage of this organic transistor lies in its extended, floating-gate architecture and the presence of a control gate, which eliminates the need for a reference electrode in the solution, enabling further miniaturization and simplifying experimental setups (Spanu et al., 2015). In particular, OCMFETs are known to be very high-sensitive charge sensors, thanks to their double gate configuration, which provides an intrinsic signal amplification compared to standard OFETs. Additionally, unlike standard OFET-based interfaces, in the OCMFET architecture the sensing area and the organic semiconductor channel are physically separated, allowing for encapsulation that protects the organic semiconductor from degradation in humid biological environments – a crucial feature for long-term monitoring of cell cultures. Moreover, the sensing area can be specifically engineered and functionalized to detect other relevant parameters, such as metabolic cellular activity (Spanu et al., 2018). This feature is particularly convenient as it allows to develop multi-sensing platforms using the same transistor architecture (Spanu et al., 2022).
In this work, we demonstrate the capability of OCMFETs to successfully record the electrical activity of human induced pluripotent stem cell (hiPSC)-derived neurospheroids, confirming their potential for 3D in vitro applications. Furthermore, the ability to fabricate these devices on sub-micron plastic substrates enables the development of ultra-conformable systems (Viola et al., 2018), which can seamlessly integrate with complex 3D cellular structures while maintaining high signal fidelity. Combined with their low operating voltage, which reduces power consumption and minimizes undesired electrochemical reactions, OCMFET-based systems represent a powerful and scalable solution for next-generation bioelectronic interfaces.
2 Materials and methods
2.1 Device fabrication and characterization
The devices, each comprising two OCMFETs, were fabricated on polyethylene terephthalate (PET, 175 μm, Goodfellow) substrates (Figure 1). The first gold layer was thermally evaporated onto the substrate and patterned using a low-resolution photolithographic process. This metallic film served as the floating gate in the final sensors. A 200 nm Parylene C film was deposited onto the entire substrate through chemical vapor deposition (CVD) using the Labcoater 2 SCS PDS 2010 (Specialty Coating System). Subsequently, another gold layer was evaporated and patterned using a self-alignment process to form the interdigitated source and drain electrodes, the upper plate of the control gate capacitor, and the connection pads. A via was then created in the Parylene C layer by plasma oxygen etching (Tucano, Gambetti) to expose the sensing area (200 µm
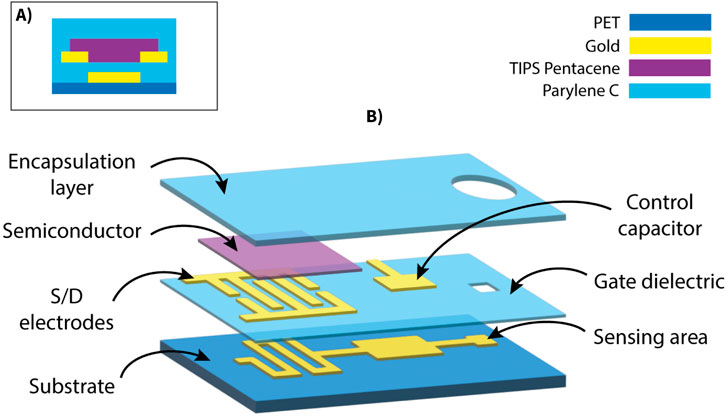
Figure 1. Structure of the OCMFET device. (A) Cross-sectional schematic showing the layered structure of the bottom-gate bottom-contact TIPS Pentacene transistor. (B) Exploded schematic view illustrating the multilayer architecture of the OCMFET.
To evaluate the performance of the devices the charge carrier mobility
where
Approximating the behavior of the OCMFET with this model, the square root of the absolute drain current can be fitted with a linear function against the gate voltage. The x-intercept of the fitted line, where
where
2.2 Neurospheroids generation and plating
The neurospheroids were derived from hiPSCs and prepared following established protocols (Muzzi et al., 2023). Specifically, this study employed a well-characterized rtTA/Ngn2-positive hiPSC line, which was derived from the fibroblasts of a healthy 30-year-old female. This hiPSC line, generated via episomal reprogramming, was obtained in frozen vials from the Coriell Institute for Medical Research (GM25256) and provided by Frega et al. The rtTA/Ngn2-positive lentiviral vectors were stably integrated into the hiPSC genome, enabling precise neuronal differentiation into early-stage excitatory cortical layer 2/3 neurons (iNeurons) through doxycycline treatment for 3 days. Maintenance of the cell line was performed following previously established protocols (Frega et al., 2017; Muzzi et al., 2021). The neurospheroids were composed of 50,000 iNeurons and astrocytes in a 1:1 ratio. The hanging-drop method was employed to create spherical aggregates of cells, aiming to generate neurospheroids in a scaffold-free culture system.
A 5 cm Petri dish was used as a “moisture chamber,” with the bottom partially filled with Dulbecco’s Phosphate-Buffered Saline (DPBS). The inverted lid of the dish was used to hold 15 µL drops of neurobasal medium, into which 15 µL drops of a mixed-cell solution (neurons and astrocytes in a 1:1 ratio, at a density of 50,000 cells per drop) were added. The dish was carefully reassembled and incubated at 37°C with 5.5% CO2. The neurobasal medium was based on Neurobasal (Thermo Fisher Scientific), supplemented with 2% B-27 supplement (Thermo Fisher Scientific), 1% GlutaMAX (Thermo Fisher Scientific), 0.1% human BDNF (Thermo Fisher Scientific), 0.1% human NT-3 (Thermo Fisher Scientific), 4 μg/mL doxycycline (Sigma Aldrich), and 1% penicillin/streptomycin (Sigma Aldrich).
On day in vitro (DIV) 1, Cytosine
At DIV 21, neurospheroids were transferred onto OCMFET devices using a pipette. Before plating, the devices were prepared by sequential cleaning with deionized water, sterilizing with ethanol, and coating with cell-adhesion promoters (poly-L-ornithine and laminin). Following deposition, the neurospheroids were incubated at 37°C with 5.5% CO2. To maintain neurospheroid viability, the culture medium was partially refreshed, replacing 50% of the volume three times per week.
2.3 Electrophysiological recording setup
The electrical activity of the neurospheroids coupled with the OCMFET devices was recorded using a custom data acquisition system developed in collaboration with Elbatech (https://www.elbatech.it/). This system simultaneously recorded the drain current
2.4 Immunofluorescence
Following electrophysiological recordings, the samples were fixed at DIV 35 for fluorescence imaging. Fixation was carried out using a 4% paraformaldehyde (PFA, Sigma-Aldrich) solution for 20 min at room temperature, followed by three washes with phosphate-buffered saline (PBS, Sigma-Aldrich). Samples were permeabilized using 0.2% Triton X-100 (Thermo Fisher Scientific) for 15 min and subsequently blocked with a Blocking Buffer Solution (BBS) composed of 0.5% fetal bovine serum (FBS, Sigma-Aldrich) and 0.3% bovine serum albumin (BSA, Sigma-Aldrich) in PBS for 45 min at room temperature. Primary antibodies, GFAP (1:500, glial fibrillary acidic protein, Cat. 173 002 and 173 01, Synaptic System) and MAP-2 (1:500, dendritic microtubule-associated protein, Cat. 188 002 and 188,011, Synaptic System), were applied to label glial and neuronal cells, respectively. Secondary antibodies included Alexa Fluor 488 (1:700, Thermo Fisher Scientific) and Alexa Fluor 546 (1:1,000, Invitrogen), along with goat anti-mouse or goat anti-rabbit antibodies. Confocal imaging was conducted on a Leica STELLARIS 8 Falcon
2.5 Data analysis
The recorded signals were processed and analyzed using Python. The pre-processing phase consisted of filtering the raw data with a second-order zero-phase Butterworth filter to retain frequencies within the range of 10 Hz to 5 kHz, effectively reducing noise and isolating relevant signal components. Spike detection was performed using a threshold-based approach, identifying events as negative peaks exceeding 5 standard deviations below the baseline signal. For each identified negative peak, a corresponding positive peak was detected by locating the maximum positive deflection within a 150 m window following the negative peak. Three features were extracted from each detected event: duration, calculated as the time difference between the positive and negative peak positions; negative peak amplitude; and amplitude (or peak-to-peak amplitude), measured as the current difference between the positive and negative peaks;
To analyze and categorize detected events, principal component analysis (PCA) was performed on the three extracted features, reducing the dimensionality of the data to two principal components while preserving the variance in the original dataset. The resulting events in the two-dimensional representation were then clustered using the k-means algorithm
The recorded signals were also analyzed in the frequency domain to compare the spectral characteristics between the baseline (in the absence of neurospheroids) and recordings containing detected events. The power spectral density (PSD) was calculated for both conditions using Welch’s method. The signal-to-noise ratio (SNR) of the recorded signals was determined using the formula:
where
3 Results
3.1 OCMFET device characterization
The fabricated OCMFET devices were characterized prior to being used in the electrophysiology experiments. The transfer curves (Figure 2) demonstrate the low-voltage operation of the TIPS pentacene OFET, with a threshold voltage of
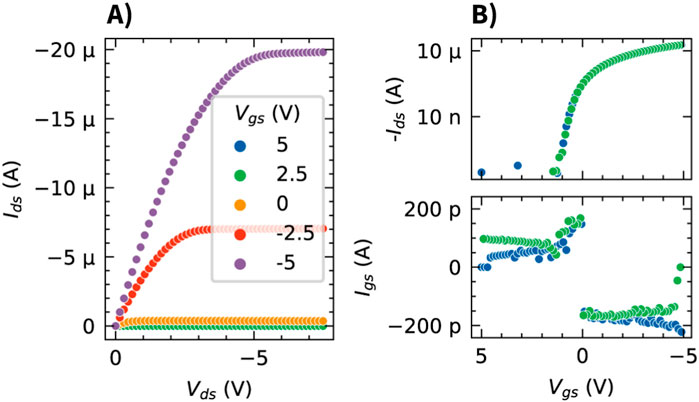
Figure 2. Electrical characterization of the TIPS pentacene based OCMFET. (A) Output characteristics (
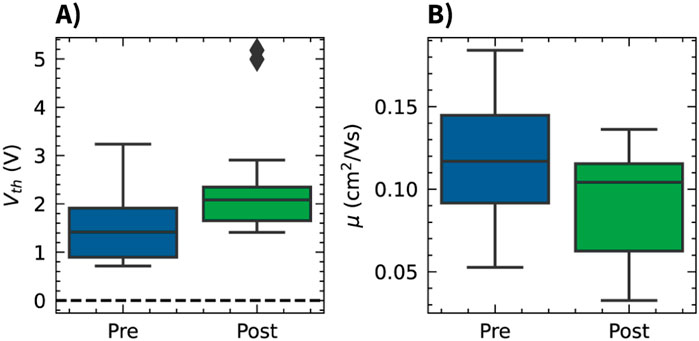
Figure 3. Effect of the Parylene C encapsulation on OCMFET electrical parameters. Box plots comparing the threshold voltage (A) and charge carrier mobility (B) distributions before (pre) and after (post) the encapsulation process.
3.2 Neurospheroid characterization
The neurospheroids used in this study were characterized using both phase-contrast and fluorescence microscopy. Phase-contrast imaging revealed compact, spherical structures with well-defined boundaries positioned on the sensing areas of the devices (Figure 4A). To confirm the cellular composition of the neurospheroids, immunofluorescence staining was performed using antibodies against MAP-2 (Microtubule-Associated Protein 2) to label neurons and GFAP (Glial Fibrillary Acid Protein) to label astrocytes. These were visualized using Alexa Fluor 488 (green) and Alexa Fluor 546 (red) secondary antibodies, respectively. This analysis confirmed the presence of both neurons and astrocytes within the neurospheoroid structure (Figure 4B). Confocal z-stack images revealed a three-dimensional cellular organization that is consistent with previous reports of hiPSC-derived neurospheroids, with astrocytes predominantly distributed along the periphery of the spheroids (Figure 4C).
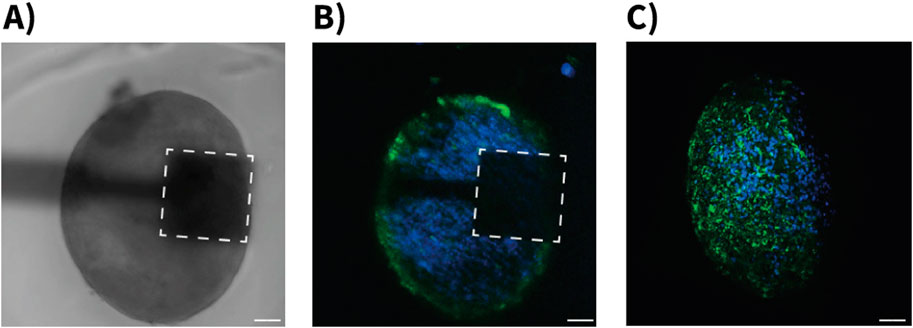
Figure 4. Morphological characterization of hiPSC-derived neurospheroids on OCMFET devices. (A) Phase-contrast microscopy image showing a neurospheroid positioned on the sensing area (dashed square) of a OCMFET (scale bar: 100 µm). (B) Confocal fluorescence microscopy image of the neurospheroid on device, showing the distribution of neurons (blue, MAP-2) and glial cells (green, GFAP) (scale bar: 100 µm). (C) Projection of confocal z-stack of a neurospheroid after device detachment, showing the three-dimensional cellular organization with neuronal (blue) and glial (green) components throughout the spheroid structure (scale bar: 100 µm).
3.3 Activity features and spectral analysis
Analysis of the recorded signals revealed distinct spike-like events in the OCMFET recordings. Using a threshold set at 5 standard deviations from the baseline, consistent bi-phasic spikes characterized by a negative deflection followed by a positive peak were detected (Figure 5). The distribution of the extracted features (event amplitude, duration, and negative peak magnitude) across all recorded events is shown in Figures 6A–C. To analyze the heterogeneity of the detected events and address potential redundancy in the extracted features, principal component analysis (PCA) was applied. Particularly amplitude and negative peak amplitude were hypothesized to be correlated. The application of PCA revealed three distinct classes visible in the scatter plot of events projected onto this new feature space. Another motivation for this analysis was to facilitate the visualization of the average event waveform. Given the apparent variability in the shapes of the events, averaging all detected events together would have provided a non-representative picture of the diverse shapes of the detected events. K-means clustering identified three representative patterns within the recorded events (Figure 6D). These clusters might represent different cellular populations or varying forms of network activity within the 3D neural tissue. The contributions of the extracted features (duration, amplitude, and negative peak amplitude) to the two principal components (PC1 and PC2), as depicted in Figure 6E, show PC1 exclusively driven by the event duration with a loading of 1.00, while PC2 exhibits a positive loading of 0.75 for amplitude and a negative loading of −0.66 for negative peak amplitude. This confirms the initial hypothesis that amplitude and negative peak amplitude contributed to a common source of variance, captured by PC2.
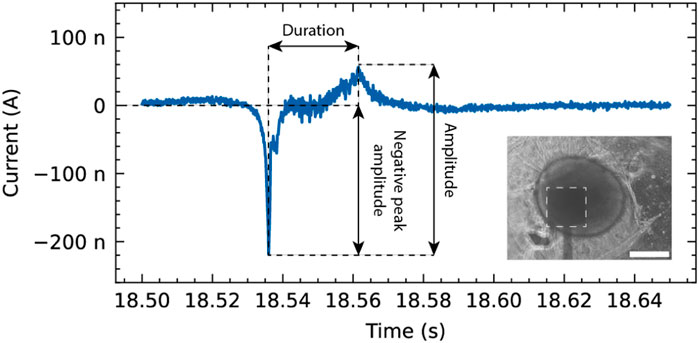
Figure 5. Representative event recorded from a neurospheroid using the OCMFET device. The trace shows the temporal evolution of the recorded signal, highlighting key features such as duration, amplitude, and negative peak amplitude. Inset: Phase-contrast microscopy image showing the neurospheroid positioned on the sensing area (dashed square) of the device (scale bar: 200 µm).
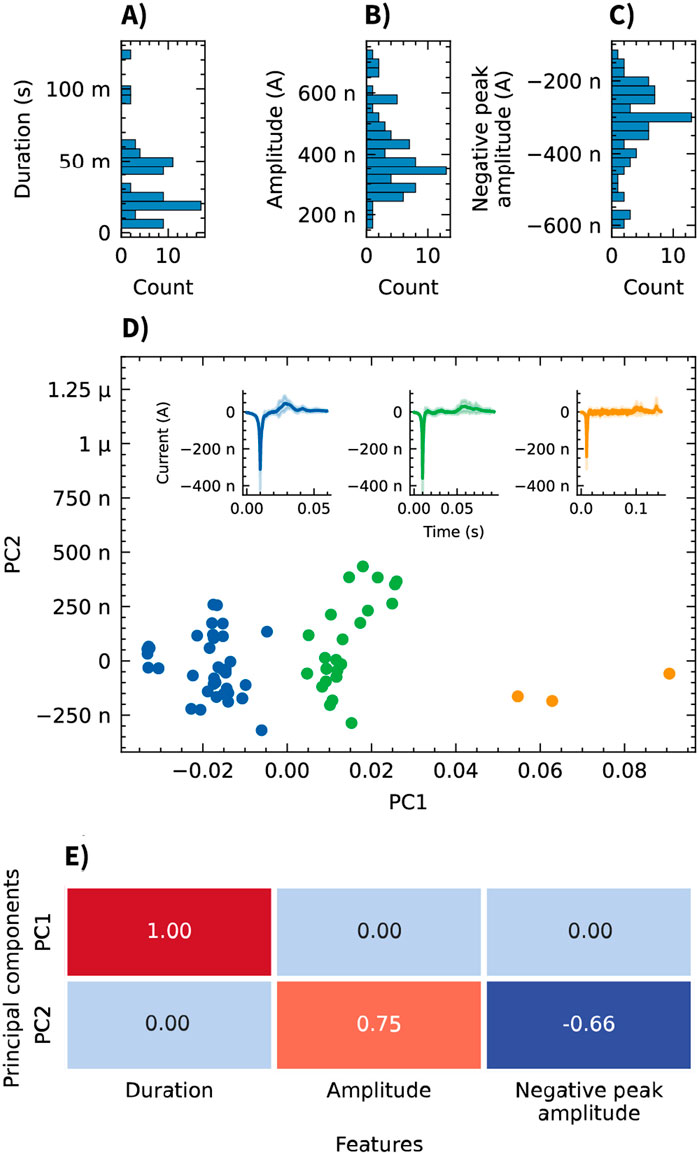
Figure 6. Analysis of event features recorded from neurospheroids using OCMFETs. (A–C) Histograms showing the distribution of event features: duration (A), amplitude (B), and negative peak amplitude (C). (D) Principal component analysis (PCA) of the three features reveals three clusters (blue, green, and orange). Insets show the average event waveforms (solid lines) and standard deviations (shaded areas) for each cluster. (E) Heatmap of the PCA loadings showing the contributions of each feature (duration, amplitude, and negative peak amplitude) to PC1 and PC2.
Power spectral density (PSD) analysis showed a clear difference between recordings with and without neurospheroids present on the sensing area of the OCMFET, with significantly higher power observed when cells were present (Figure 7). The PSD of neurospheroids recordings exceeded the baseline by approximately two orders of magnitude at low frequencies (
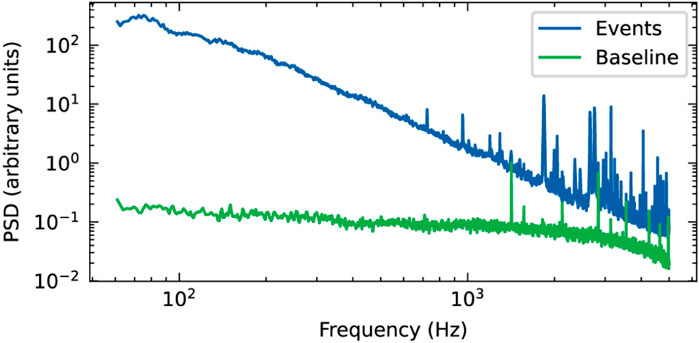
Figure 7. Power spectral density (PSD) analysis of OCMFET recordings from neurospheroids. Comparison of frequency components with (events, blue) and without (baseline, green) neurospheroid.
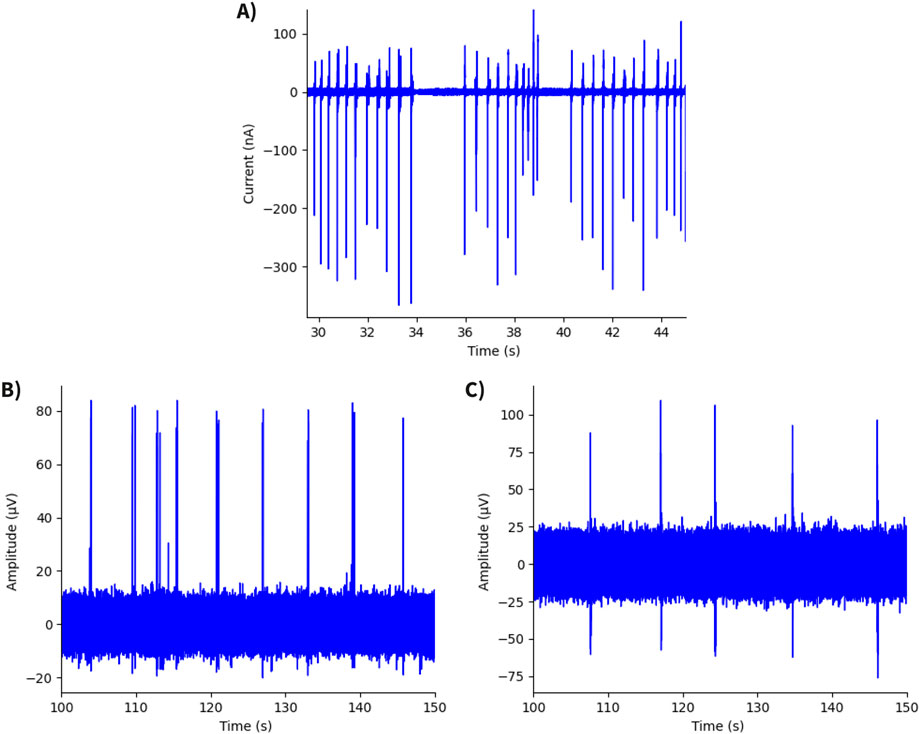
Figure 8. Time traces of neurospheroid recordings. (A) Recording from hiPSC-derived neurospheroid using an OCMFET device, showing a SNR of 589. (B) Recording from hiPSC-derived neurospheroid using an MEA60 system (Multichannel Systems), with a SNR of 45. (C) Recording from rat cortical neurospheroid using an MEA60 system, exhibiting the lowest value of SNR (Yang et al., 2024).
4 Discussion
In this work, we have tested organic charge-modulated field-effect transistors (OCMFETs) for recording the spontaneous, synchronized electrical activity of human induced pluripotent stem cell (hiPSC)-derived neurospheroids. The proposed devices show good electrical performance at low operating voltages, an important aspect when dealing with in-liquid measurements in that it helps to slow down the degradation of the sensing area material, it reduces unwanted perturbation of cells activity, and it allows for ultra-low power consumption.
The recorded events captured the spontaneous electrical activity of the hiPSC-derived neurospheroids, demonstrating the ability of OCMFET devices to transduce the electrophysiological responses of these 3D cell cultures. A key next step in the validation of this platform involves testing these devices across different neural models and stimulation protocols, including pharmacological modulation. In parallel, scaling down the dimensions of the sensitive areas will enable higher density mapping of electrical activity within tissues.
Although preliminary, this evaluation highlighted excellent performance in terms of signal transduction, thus laying the groundwork for future iterations of the OCMFET technology. In particular, this solution, thanks to the versatility of the transduction principle and the possibility of fabricating these devices on ultra-flexible, possibly conformable substrates, will drive the development of a new generation of high-performing devices for recording the electrical activity of 3D complex cellular aggregates in vitro, thus enabling innovative applications in precision medicine, drug discovery, and high-throughput screening.
Data availability statement
The raw data supporting the conclusions of this article will be made available by the authors, without undue reservation.
Ethics statement
Ethical approval was not required for the studies on humans in accordance with the local legislation and institutional requirements because only commercially available established cell lines were used.
Author contributions
FT: Conceptualization, Data curation, Methodology, Software, Validation, Visualization, Writing – original draft, Formal Analysis, Investigation, Writing – review and editing. FV: Data curation, Investigation, Methodology, Validation, Writing – original draft, Writing – review and editing. DD: Data curation, Investigation, Methodology, Visualization, Writing – original draft. PM: Conceptualization, Methodology, Supervision, Writing – review and editing. SM: Conceptualization, Project administration, Resources, Supervision, Validation, Writing – review and editing. AB: Funding acquisition, Supervision, Writing – review and editing. AS: Conceptualization, Funding acquisition, Methodology, Project administration, Supervision, Validation, Writing – original draft, Writing – review and editing.
Funding
The author(s) declare that financial support was received for the research and/or publication of this article. FT and AS acknowledge financial support under the National Recovery and Resilience Plan (NRRP), Mission 4, Component 2, Investment 1.1, Call for tender No. 1409 published on 14.9.2022 by the Italian Ministry of University and Research (MUR), funded by the European Union - NextGenerationEU - Project Title ANALYSER - CUP I53D23005640001 - Grant Assignment Decree No. 960 adopted on 30/06/2023 by the Italian Ministry of Ministry of University and Research (MUR). FAV and AB acknowledge financial support under the National Recovery and Resilience Plan (NRRP), Mission 4, Component 2, Investment 1.5, Creazione e Rafforzamento di “Ecosistemi dell’Innovazione per la Sostenibilitá”, Creazione di “Leader Territoriali di R&S″, finanziato dall’Unione Europea–NextgenerationEU Spoke four e 9, Cascade grant of “THE - TUSCANY HEALTH ECOSYSTEM” ECS00000017, CUP I53C22000780001 - NEXTGENERATIONEU - SPOKE 4 ″Nanotechnologies for diagnosis and therapy” Project Title: MISCELL–CUP 13C24000460006. FAV and AB acknowledge the financial support of The Italian Ministry of Health - Piano Operativo Salute - Traiettoria 4, project “Hybrid Hub (H2UB): Modelli cellulari e computazionali, micro e nanotecnologie per la personalizzazione di terapie innovative”. The fabrication of the devices has been partially carried out at Biosensors Lab, Dipartimento di Scienze Biomediche (DISB), University of Cagliari.
Conflict of interest
The authors declare that the research was conducted in the absence of any commercial or financial relationships that could be construed as a potential conflict of interest.
The author(s) declared that they were an editorial board member of Frontiers, at the time of submission. This had no impact on the peer review process and the final decision.
Generative AI statement
The author(s) declare that no Generative AI was used in the creation of this manuscript.
Publisher’s note
All claims expressed in this article are solely those of the authors and do not necessarily represent those of their affiliated organizations, or those of the publisher, the editors and the reviewers. Any product that may be evaluated in this article, or claim that may be made by its manufacturer, is not guaranteed or endorsed by the publisher.
References
Camp, J. G., Badsha, F., Florio, M., Kanton, S., Gerber, T., Wilsch-Bräuninger, M., et al. (2015). Human cerebral organoids recapitulate gene expression programs of fetal neocortex development. Proc. Natl. Acad. Sci. 112, 15672–15677. doi:10.1073/pnas.1520760112
Durens, M., Nestor, J., Williams, M., Herold, K., Niescier, R. F., Lunden, J. W., et al. (2020). High-throughput screening of human induced pluripotent stem cell-derived brain organoids. J. Neurosci. Methods 335, 108627. doi:10.1016/j.jneumeth.2020.108627
Forrest, S. R. (2004). The path to ubiquitous and low-cost organic electronic appliances on plastic. Nature 428, 911–918. doi:10.1038/nature02498
Frega, M., Van Gestel, S. H. C., Linda, K., Van Der Raadt, J., Keller, J., Van Rhijn, J. R., et al. (2017). Rapid neuronal differentiation of induced pluripotent stem cells for measuring network activity on micro-electrode arrays. J. Vis. Exp., 54900. doi:10.3791/54900
Gu, X., Yeung, S. Y., Chadda, A., Poon, E. N. Y., Boheler, K. R., and Hsing, I. M. (2019). Organic electrochemical transistor arrays for in vitro electrophysiology monitoring of 2D and 3D cardiac tissues. Adv. Biosyst. 3, 1800248. doi:10.1002/adbi.201800248
Jordan, F. D., Kutter, M., Comby, J. M., Brozzi, F., and Kurtys, E. (2024). Open and remotely accessible Neuroplatform for research in wetware computing. Front. Artif. Intell. 7, 1376042. doi:10.3389/frai.2024.1376042
Jung, G. S., Lee, K. M., Park, J. K., Choi, S. K., and Jeon, W. B. (2013). Morphogenetic and neuronal characterization of human neuroblastoma multicellular spheroids cultured under undifferentiated and all-trans-retinoic acid-differentiated conditions. BMB Rep. 46, 276–281. doi:10.5483/BMBRep.2013.46.5.196
Kyndiah, A., Dipalo, M., Molazemhosseini, A., Viola, F. A., Modena, F., Iachetta, G., et al. (2023). Direct recording of action potentials of cardiomyocytes through solution processed planar electrolyte-gated field-effect transistors. Sensors Actuators B Chem. 393, 134227. doi:10.1016/j.snb.2023.134227
Lagomarsini, C., Jean-Mistral, C., Kachroudi, A., Monfray, S., and Sylvestre, A. (2020). Outstanding performance of parylene polymers as electrets for energy harvesting and high-temperature applications. J. Appl. Polym. Sci. 137, 48790. doi:10.1002/app.48790
Muzzi, L., Di Lisa, D., Arnaldi, P., Aprile, D., Pastorino, L., Martinoia, S., et al. (2021). Rapid generation of functional engineered 3D human neuronal assemblies: network dynamics evaluated by micro-electrodes arrays. J. Neural Eng. 18, 066030. doi:10.1088/1741-2552/ac3e02
Muzzi, L., Di Lisa, D., Falappa, M., Pepe, S., Maccione, A., Pastorino, L., et al. (2023). Human-derived cortical neurospheroids coupled to passive, high-density and 3D MEAs: a valid platform for functional tests. Bioengineering 10, 449. doi:10.3390/bioengineering10040449
Simão, D., Silva, M. M., Terrasso, A. P., Arez, F., Sousa, M. F. Q., Mehrjardi, N. Z., et al. (2018). Recapitulation of human neural microenvironment signatures in iPSC-derived NPC 3D differentiation. Stem Cell Rep. 11, 552–564. doi:10.1016/j.stemcr.2018.06.020
Spanu, A., Colistra, N., Farisello, P., Friz, A., Arellano, N., Rettner, C. T., et al. (2020). A three-dimensional micro-electrode array for in-vitro neuronal interfacing. J. Neural Eng. 17, 036033. doi:10.1088/1741-2552/ab9844
Spanu, A., Lai, S., Cosseddu, P., Tedesco, M., Martinoia, S., and Bonfiglio, A. (2015). An organic transistor-based system for reference-less electrophysiological monitoring of excitable cells. Sci. Rep. 5, 8807. doi:10.1038/srep08807
Spanu, A., Martines, L., and Bonfiglio, A. (2021). Interfacing cells with organic transistors: a review of in vitro and in vivo applications. Lab a Chip 21, 795–820. doi:10.1039/D0LC01007C
Spanu, A., Martines, L., Tedesco, M., Martinoia, S., and Bonfiglio, A. (2022). Simultaneous recording of electrical and metabolic activity of cardiac cells in vitro using an organic charge modulated field effect transistor array. Front. Bioeng. Biotechnol. 10, 945575. doi:10.3389/fbioe.2022.945575
Spanu, A., Tedesco, M. T., Martines, L., Martinoia, S., and Bonfiglio, A. (2018). An organic neurophysiological tool for neuronal metabolic activity monitoring. Apl. Bioeng. 2, 046105. doi:10.1063/1.5050170
Viola, F. A., Spanu, A., Ricci, P. C., Bonfiglio, A., and Cosseddu, P. (2018). Ultrathin, flexible and multimodal tactile sensors based on organic field-effect transistors. Sci. Rep. 8, 8073. doi:10.1038/s41598-018-26263-1
Xie, K., Wang, N., Lin, X., Wang, Z., Zhao, X., Fang, P., et al. (2020). Organic electrochemical transistor arrays for real-time mapping of evoked neurotransmitter release in vivo. eLife 9, e50345. doi:10.7554/eLife.50345
Yadav, N., Lisa, D. D., Giacomozzi, F., Cian, A., Giubertoni, D., Martinoia, S., et al. (2023). Development of multi-depth probing 3D microelectrode array to record electrophysiological activity within neural cultures. J. Micromechanics Microengineering 33, 115002. doi:10.1088/1361-6439/acf940
Yang, X., Forró, C., Li, T. L., Miura, Y., Zaluska, T. J., Tsai, C. T., et al. (2024). Kirigami electronics for long-term electrophysiological recording of human neural organoids and assembloids. Nat. Biotechnol. 42, 1836–1843. doi:10.1038/s41587-023-02081-3
Zhuang, P., Sun, A. X., An, J., Chua, C. K., and Chew, S. Y. (2018). 3D neural tissue models: from spheroids to bioprinting. Biomaterials 154, 113–133. doi:10.1016/j.biomaterials.2017.10.002
Keywords: neurospheroids, OCMFET, hiPSC, bioelectronics, neural recording, organic transistors, 3D cell cultures
Citation: Terranova F, Viola FA, Di Lisa D, Massobrio P, Martinoia S, Bonfiglio A and Spanu A (2025) Organic charge-modulated transistor for electrophysiological measurements of human-derived neurospheroids. Front. Bioeng. Biotechnol. 13:1571011. doi: 10.3389/fbioe.2025.1571011
Received: 04 February 2025; Accepted: 02 May 2025;
Published: 16 May 2025.
Edited by:
Guangli Li, Hunan University of Technology, ChinaReviewed by:
Russell J. Andrews, Independent Researcher, Los Gatos, United StatesAndrea Idili, University of Rome Tor Vergata, Italy
Copyright © 2025 Terranova, Viola, Di Lisa, Massobrio, Martinoia, Bonfiglio and Spanu. This is an open-access article distributed under the terms of the Creative Commons Attribution License (CC BY). The use, distribution or reproduction in other forums is permitted, provided the original author(s) and the copyright owner(s) are credited and that the original publication in this journal is cited, in accordance with accepted academic practice. No use, distribution or reproduction is permitted which does not comply with these terms.
*Correspondence: Andrea Spanu, YW5kcmVhLnNwYW51QGl1c3NwYXZpYS5pdA==
†These authors share first authorship