- 1Department of Pharmacy, Xi’an Hospital of Traditional Chinese Medicine, Xi’an, Shaanxi, China
- 2School of Medicine, Xi’an Peihua University, Xi’an, Shaanxi, China
- 3Health Science Center, Xi’an Jiaotong University, Xi’an, Shaanxi, China
- 4Tianjin Key Laboratory of Food and Biotechnology, School of Biotechnology and Food Science, Tianjin University of Commerce, Tianjin, China
- 5Brigham and Women’s Hospital, Harvard Medical School, Boston, MA, United States
Colorectal cancer (CRC) remains a highly heterogeneous malignancy with significant morbidity and mortality worldwide. Despite advancements in surgery, chemotherapy, immunotherapy, and targeted therapy, treatment efficacy is often hampered by drug resistance and systemic toxicity. In recent years, nano-drug delivery systems (NDDS) have emerged as a promising strategy to enhance therapeutic precision, reduce adverse effects, and overcome resistance in CRC treatment. This review discusses the recent advancements in NDDS for CRC treatment, focusing on the optimization of oral drug delivery systems, the development of tumor-specific targeting strategies, and the design of intelligent delivery systems responsive to the tumor microenvironment (TME). Furthermore, we summarize current challenges in NDDS translation and explore future research directions for enhancing their clinical feasibility and therapeutic impact.
1 Introduction
Colorectal cancer (CRC) is a highly heterogeneous malignant tumor originating in mucosal epithelial cells of the colon or rectum (Liu et al., 2024). CRC remains one of the leading causes of cancer-related mortality worldwide, with particularly high incidence rates in developed countries (Hultcrantz, 2021). Epidemiological studies have shown that CRC arises from a complex interaction between genetic susceptibility and environmental influences (Wang H. et al., 2023; Sobhani et al., 2020). The genetic factors include hereditary tumor syndromes such as familial adenomatous polyposis (FAP) and Lynch syndrome (Vieira et al., 2024; Nolano et al., 2023). Environmental factors involved high-fat and low-fiber dietary patterns, obesity, sedentary lifestyle, smoking and alcohol abuse, and other controllable risk factors (Chu et al., 2025). Of note, chronic inflammatory bowel disease (IBD), particularly ulcerative colitis and Crohn’s disease, are associated with a significantly elevated CRC risk, which may be related to genomic instability caused by the chronic inflammatory microenvironment (Adami et al., 2016; Shimomura et al., 2023).
In terms of treatment strategy, the multidisciplinary integrated treatment model (MDT) was adopted for CRC, and the choice of treatment was mainly based on the TNM stage of the tumor (Mangone et al., 2024). For localized lesions, radical surgical resection remains the preferred treatment for early CRC. Postoperative adjuvant chemotherapy significantly reduces the risk of recurrence in stage II-III patients (van Erning et al., 2013). However, the current treatment of CRC still faces many challenges. The dose-limiting toxicity of chemotherapy drugs (Zhen et al., 2023a) is common, including myelosuppression, peripheral neurotoxicity, digestive tract reaction, etc., and it is easy to produce acquired drug resistance (Yue et al., 2024; Was et al., 2022). Although targeted therapy improves efficacy, its application is limited by specific molecular markers and may cause adverse reactions such as skin toxicity, hypertension, and proteinuria (Farooqui et al., 2020). Therefore, strengthening early screening (such as fecal occult blood test, and colonoscopy), optimizing individualized treatment strategies, and developing novel targeted drugs and immunotherapy (Xiong et al., 2024a; Xiong et al., 2024b) are important directions to improve the prognosis of CRC in the future.
Due to the complexity of the biological barriers and the tumor microenvironment (Zhen et al., 2024), drugs are often limited by the low permeability at the tumor site, making it difficult for them to accumulate. At the same time, the hypoxic, acidic environment, and high levels of reactive oxygen species in the tumor microenvironment may affect the stability of the drugs. In recent years, the significant advantages of nanotechnology in cancer treatment have garnered considerable attention from researchers. The core benefits are primarily reflected in two key areas: precise targeting and intelligent controlled release (Chehelgerdi et al., 2023; Sun et al., 2023). Firstly, through surface modification techniques (such as antibodies, peptides, or specific ligands), nanomaterials can accurately identify and bind to markers overexpressed on the surface of cancer cells, thereby achieving targeted drug delivery (Di et al., 2020). This precision not only markedly enhances therapeutic efficacy, but also significantly mitigates the toxic side effects of conventional chemotherapy drugs on healthy tissues. Secondly, by engineering carrier materials with environmental responsiveness (such as pH-sensitive, temperature-sensitive, or enzyme-sensitive materials), the nano-delivery system can achieve controlled drug release in response to specific stimuli within the tumor microenvironment. This intelligent release mechanism prolongs drug action time, reduces dosing frequency, and improves patient compliance (Li X. et al., 2023; Wang et al., 2021).
Nano-drug delivery systems (NDDS) represent an advanced class of nanomaterials, derived from either natural or synthetic polymers (Safari and Zarnegar, 2014). These systems encompass solid particles and gel-like structures. By integrating anticancer agents with nano-delivery platforms, various functional formulations such as liposomes, polymer microspheres, micelles, and in situ gels can be developed (Sandha et al., 2025; Ni et al., 2020; Zhang et al., 2023b; Si et al., 2021). These nanotherapeutics significantly enhance drug targeting and bioavailability while effectively mitigating off-target effects that often lead to damage in normal tissues, a common issue with traditional small-molecule anticancer drugs (Chuang et al., 2022). With the rapid advancement of nanotechnology, its application in cancer theranostics has expanded considerably, leading to breakthroughs in treatment efficacy and demonstrating substantial potential for early diagnosis and real-time monitoring, thereby offering new hope and improved survival prospects for cancer patients (Bano et al., 2023; Seyfoori et al., 2023).
In this review, we systematically delineate the advancements in NDDS for CRC treatment, with a focus on three pivotal innovative directions: the optimization of oral drug delivery systems, the development of tumor-specific targeting strategies, and the design of tumor microenvironment-responsive intelligent delivery systems. Through a comprehensive analysis of the groundbreaking progress achieved by nanocarriers in enhancing oral drug bioavailability, improving tumor-targeting specificity, and enabling microenvironment-regulated drug release, we aim to provide novel insights and a robust theoretical foundation for the development of next-generation intelligent NDDS. The integration of these cutting-edge nanotechnologies is anticipated to significantly enhance the precision and efficacy of CRC treatment. By improving therapeutic outcomes and reducing the risk of recurrence, these advancements not only promise to elevate the treatment experience and quality of life for patients, but also hold the potential to extend overall survival rates. Collectively, these innovations pave the way for transformative approaches in the clinical management of CRC.
2 Recent advancements in NDDS for CRC treatment
2.1 Oral drug delivery systems
Oral administration is a preferred route for drug delivery due to its non-invasiveness, patient compliance, and suitability for both local and systemic therapies (Liu et al., 1997). This mode of drug delivery utilizes the extensive mucosal absorption surface area of the intestine and prolonged drug retention time, making it an ideal choice for the management of gastrointestinal diseases (Chai et al., 2025). Its non-invasive nature, convenience, and high patient compliance further underscore its clinical appeal (Chen et al., 2023). However, traditional oral drugs face significant challenges in the complex microenvironment of the digestive tract, which can severely compromise their efficacy (Ejazi et al., 2023) (Figure 1A). Factors such as the highly acidic gastric environment (pH 1.0–3.0), enzymatic degradation by gastrointestinal digestive enzymes, and the barrier properties of the gastrointestinal mucosa insignificantly reduce drug bioavailability (Bashiardes and Christodoulou, 2024). To address these limitations, the development of novel intelligent oral NDDS with environment-responsive characteristics has emerged as a critical scientific challenge in the field of drug delivery. These advanced systems are designed to effectively protect drug activity and enhance targeted delivery efficiency. The successful development of such innovative delivery platforms is anticipated to not only significantly improve drug bioavailability, but also provide transformative solutions for the precision treatment of gastrointestinal diseases.
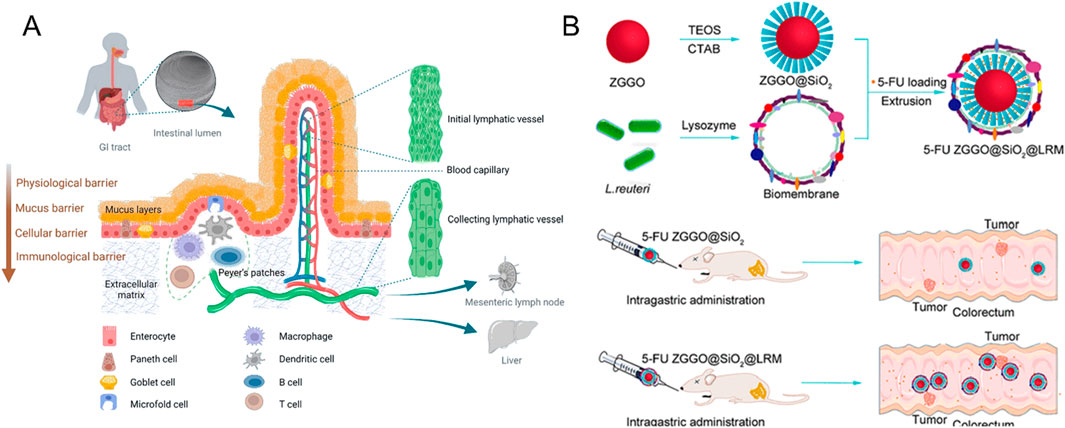
Figure 1. (A) Schematic diagram based on the transit pathway of nanoparticles in the gastrointestinal tract after oral administration (Reproduced with permission from the reference: Mechanisms of Nanoparticle Transport across Intestinal Tissue: An Oral Delivery Perspective). Adapted from Ejazi et al., ACS Nano, 2023, under a Creative Commons CC BY license. (B) Schematic Diagram of 5-Fu ZGGO@SiO2@LRM Nanoparticles for Colorectal Cancer Imaging and Chemotherapy (Reproduced with permission from the reference: Bacterial Biofilm Bioinspired Persistent Luminescence Nanoparticles with Gut-Oriented Drug Delivery for Colorectal Cancer Imaging and Chemotherapy). Adapted from Wang et al., ACS Applied Materials and Interfaces, 2019, under a Creative Commons CC BY license.
2.1.1 NDDS developed based on low stomach pH
There were significant pH gradients in different anatomical parts of the digestive tract. Physiologically, the stomach cavity maintains a strongly acidic environment (pH 1.0–3.0), while as chyme advances into the distal digestive tract, the pH of the duodenal-ileum segment gradually increases to the weakly alkaline range (pH 6.0–7.4) (Collnot et al., 2012; Koziolek et al., 2015). Notably, the CRC tissue microenvironment is typically weakly acidic (pH 6.5–7.2) (Gerweck and Seetharaman, 1996). Based on this unique pH distribution in the digestive tract, researchers have successfully developed a variety of PH-responsive smart delivery materials, including polymethacrylate polymers, natural polysaccharides chitosan, and synthetic polymers such as Eudragit (Turanlı and Acartürk, 2022; Noreen et al., 2024; Gupta et al., 2023). These functional materials can undergo controlled physicochemical changes such as swelling, dissolution, or degradation at specific pH thresholds, thereby achieving precise controlled release of therapeutic drugs at target sites, improving drug bioavailability, and reducing systemic toxicity (Wathoni et al., 2020).
Bajracharya et al. developed an innovative colon-targeted drug delivery system using methotrexate (MTX) as a model drug. The system was constructed by decorating gold nanoparticles onto aminoclay (AC-Au) and coating them with pH-sensitive methacrylic acid-co-methyl methacrylate (1:2) copolymer. Experimental results demonstrated that the functionalized nanoparticles exhibited excellent pH-dependent release characteristics: In the simulated gastric environment (pH 1.2), only approximately 23% of the drug was released, while in the simulated colonic environment (pH 7.4), the drug release rate reached 75%, which was attributed to the pH-responsive dissolution of the outer polymeric coating (Bajracharya et al., 2023). This novel pH-responsive delivery system significantly enhances the targeting efficiency and in vivo antitumor efficacy of MTX in CRC treatment.
Wang and his team pioneered the design and synthesis of deoxycholic acid and hydroxybutyl decorated chitosan nanoparticles (DAHBC NPs), establishing a groundbreaking oral drug delivery system aimed at enhancing the bioavailability of the poorly soluble drug curcumin (CUR) (Wang T. et al., 2019). These nanocarriers exhibit a distinctive temperature-responsive behavior, characterized by a low critical solution temperature (LCST) ranging from 27°C to 33°C, which is notably below physiological temperature (37°C). In simulated gastric conditions (pH 1.2, 37°C), DAHBC NPs demonstrate remarkable stability, primarily attributed to the dynamic equilibrium between pH-responsive expansion and thermal-responsive contraction. Upon transitioning to simulated intestinal conditions (pH 7.0–7.4, 37°C), the thermal-responsive contraction properties of DAHBC NPs become predominant, facilitating the rapid release of CUR. Experimental findings reveal that this delivery system effectively modulates CUR release in the stomach, with a release rate of merely approximately 10%. Notably, DAHBC NPs enhance the intestinal absorption efficiency and bioavailability of CUR by approximately tenfold compared to free CUR, underscoring the immense potential of this nano-delivery system in augmenting the oral absorption of insoluble drugs.
Eudragit, a pH-sensitive enteric polymer, demonstrates excellent solubility at pH 5.5 due to its unique solubility characteristics (Kendall et al., 2009). This polymer exhibits superior drug retention capabilities compared to other similar polymers, offering significant advantages in pharmaceutical applications. Its distinctive properties not only effectively shield active pharmaceutical ingredients from degradation in the acidic gastric environment, thereby enhancing drug bioavailability, but also enable precise drug release at targeted intestinal sites (Nikam et al., 2023). In a groundbreaking study, the Kassem developed an innovative drug delivery system by utilizing Eudragit®-S100 (EUS-100) for surface modification of functionalized silica samples (Kassem, et al., 2024). Their research demonstrated that the EUS-100 coating effectively prevents premature release of encapsulated catechins (CHT) in the acidic gastrointestinal environment while maintaining the chemical stability of catechins against pH variations. Notably, the experimental results revealed that approximately 90% of catechins were specifically released at the colon site, highlighting the crucial role and application potential of Eudragit in colon-targeted drug delivery systems.
In addition, bionics has demonstrated significant application potential in the development of functional nanomaterials (Tang et al., 2024). For instance, certain acid-tolerant bacteria can survive the harsh stomach environment and colonize the gut, offering valuable insights for the design of nanomedicine delivery systems (Li X. et al., 2025). Leveraging this bionic principle, Wang and his research team innovatively coated Lactobacillus reuteri biofilm (LRM) onto the surface of near-infrared persistent luminescent mesoporous zinc gallogermanate (ZGGO), successfully constructing nanoparticles with bacterial bionic properties (ZGGO@SiO2@LRM) (Wang Z.-H. et al., 2019) (Figure 1B). This nano-system not only withstands the acidic stomach environment, but also achieves targeted delivery of 5-fluorouracil (5-FU) to the colorectal region. Experimental results revealed that the LRM coating significantly enhanced the colonization of nanomaterials in the colorectal area and markedly prolonged the retention time of drugs at the target site. In vivo tracking demonstrated that the nanomaterial could still be detected in the colorectal region 24 h post-administration. This groundbreaking study provides novel insights and a theoretical foundation for the development of oral targeted colorectal drug delivery systems, holding substantial promise for clinical applications.
2.1.2 NDDS developed based on different enzyme systems in the gastrointestinal tract
The gastrointestinal tract harbors an intricate enzymatic network that functions as highly specialized molecular shears, cleaving chemical bonds in most pharmaceutical compounds and rendering them biologically inactive prior to reaching their target sites (Udenigwe et al., 2021). To circumvent this enzymatic degradation and ensure site-specific drug delivery, polysaccharides including chitosan, guar gum, and pectin are extensively employed as rate-controlling excipients in colon-targeted drug formulations (Shahdadi Sardou et al., 2019; Shao et al., 2025; Udaipuria and Bhattacharya, 2025). These polysaccharide-based matrices demonstrate remarkable resistance to gastric and intestinal enzymatic activity, maintaining structural integrity throughout the upper gastrointestinal tract. However, upon reaching the colonic environment, they undergo selective degradation by anaerobic bacterial flora, thereby initiating controlled drug release (Salyers et al., 1978). This sophisticated drug delivery strategy not only enhances site-specificity, but also optimizes therapeutic efficacy through targeted pharmacological action.
Chitosan is a linear polysaccharide formed by randomly distributed N-acetyl-D-glucosamine and D-glucosamine units connected by β (1–4) glucoside bonds (Zamboulis et al., 2020). Its unique molecular structure enables it to be degraded by specific enzymes secreted by the colon microbial community, which makes chitosan a significant application in the targeted therapy of CRC (Ray, 2019). In an innovative study by Zhou et al., the research team successfully developed a novel oral drug delivery system (Zhou et al., 2024) (Figure 2A). The system uses rhamnolipid (RHL) to encapsulate the chemotherapy drug 5-FU and the photothermic agent bismuth nanosheets (BiNS), and chitosan (CS) is used as the shell of the nanoparticles. The BiNS@RHL-CS/5-FU nanoparticles (NPs) were constructed. The delivery system has unique pH response characteristics: During gastrointestinal transport, the double protective barrier formed by rhamnose-lipids and chitosan can effectively prevent the premature release of BiNS and 5-FU. When the nanoparticles reach the colonic site, β-glycosidase can specifically degrade the chitosan shell under the condition of elevated microenvironmental pH. This process not only reduces the size of nanoparticles, enhances their penetration and absorption ability in tumor tissues, but also significantly improves the tumor targeting efficiency of 5-FU, and effectively reduces the toxic effect of the drug on normal tissues.
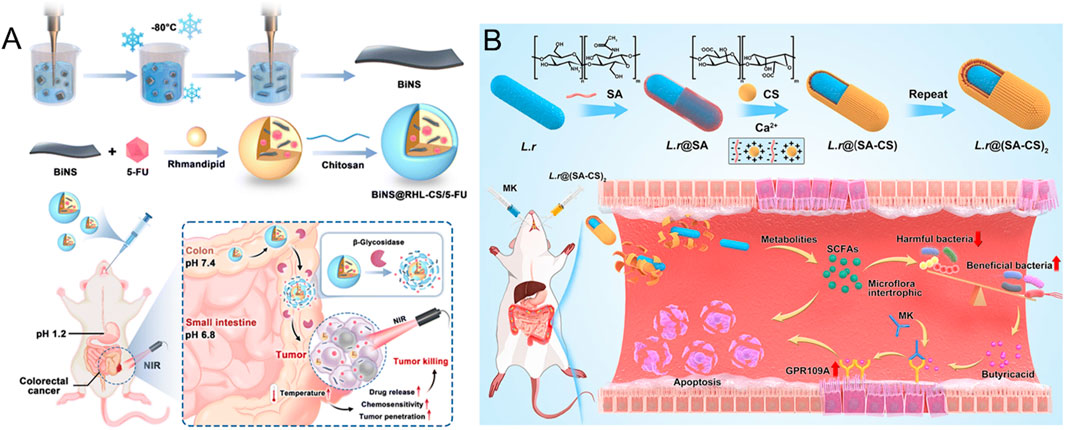
Figure 2. (A) The mode and mechanism of action of BiNS@RHL-CS/5-FU NPs (Reproduced with permission from the reference: β-Glycosidase sensitive oral nanoparticles for combined photothermal and chemo treatment of colorectal cancer). Adapted from Zhou et al., Journal of Materials Chemistry B, 2024, under a Creative Commons CC BY license. (B) Schematic illustration of L.r @(SA-CS)2 suppressed tumor growth by modulating intestinal flora (Reproduced with permission from the reference: Taking SCFAs produced by Lactobacillus reuteri orally reshapes gut microbiota and elicits antitumor responses). Adapted from Li et al., Journal of Nanobiotechnology, 2024, under a Creative Commons CC BY license.
Tramontano et al. found that although gelatine-coated diatomite nanoparticles (diatomite NPs) show potential applications in drug encapsulation and cell delivery, their oral administration effects are significantly limited (Tramontano et al., 2022). This is mainly due to the degradation of gelatin by digestive enzymes in the gastrointestinal tract, resulting in premature release of the active ingredient before the target site. To solve this problem, the researchers innovatively adopted microfluidic nanoprecipitation technology to further encapsulate NPs in hydroxypropyl methylcellulose acetate succinate (HPMC). This enteric polymer not only effectively protects the gelatin coating on the surface of the NPs from gastric enzymatic hydrolysis, but also enables the precise release of the drug in the specific pH environment of the intestine, thus significantly improving its therapeutic effect on colon cancer.
In fact, very few unmodified proteins survive in the stomach and make it to the intestines. Pepsin is activated at low pH and efficiently hydrolyzes peptide bonds in proteins, breaking them down into smaller peptide fragments (Rivera del Rio et al., 2021). As a result, unprotected proteins are often quickly digested in the stomach, making it difficult to maintain their integrity and biological activity. However, binding with specific carrier molecules or encapsulation in acid-resistant NPs is an important strategy to avoid the inactivation of protein drugs during oral administration. In the study of Elmorshedy et al., they developed an NDDS based on this idea, and the construction of the system adopted advanced nanotechnology: First, two active molecules docetaxel (DTX) and atorvastatin (ATR) were loaded onto lactoferrin (Lf) nanocapsules to form stable nanoparticles through chemical conjugations; Subsequently, the molecular interaction between carboxymethyl inulin (CMI) or carboxymethyl cellulose (CMC) and zeololysin (ZN) was utilized to further microencapsulated the nanoparticles and construct colon-targeting NDDS (Elmorshedy et al., 2023). In vitro release experiments showed that the delivery system showed good stability in the simulated gastric fluid environment, and no drug leakage was detected. In the colon environment, the nanoparticles were released efficiently and showed significant cancer cell targeting and excellent anti-tumor effect.
2.1.3 NDDS developed based on the dense mucus barrier of the gastrointestinal tract
In addition to the well-known extreme pH and complex enzymolysis barriers, the unique physiological characteristics of gastrointestinal mucosal tissue constitute another important obstacle. Specifically, the surface of the gastrointestinal tract is covered by a mucous layer about 50–450 μm thick, with a pore size of about 50–1800 nm and a negative charge, forming a complex physicochemical barrier (das Neves et al., 2020; Damianos et al., 2025). Drug molecules must first overcome the electrostatic rejection and steric hindrance of the mucus layer, and interact with mucin specifically or non-specifically in order to achieve effective penetration, and then reach the epithelial cells to play a role (Subramanian et al., 2022). In recent years, the breakthrough of nanotechnology in the field of drug delivery has provided a new way to solve this problem. Studies have shown that nanoparticle delivery systems in the size range of 50–200 nm exhibit excellent mucus penetration (Öztürk et al., 2024). Currently, biomaterials commonly used to improve mucus permeability include: chitosan (cationic properties and bioadhesion), hyaluronic acid (good biocompatibility and mucus affinity), alginate (excellent gel formation ability), and other polysaccharide substances with specific functional groups (Lu et al., 2020; Zhang, Y. et al., 2023a; Taipaleenmäki et al., 2020).
Chitosan can enhance the transepithelial permeability of anticancer drugs by temporarily opening tight junctions between epithelial cells (Cole et al., 2018). Wang et al. developed an innovative hydrogel delivery system based on methacrylic anhydride-modified carboxymethyl chitosan (MA-CMCS) (Wang C.-Y. et al., 2022). The system successfully loaded and delivered imatinib (IMT) by combining the osmotic accelerator sodium deoxycholate (SD). This delivery system not only has enzyme-responsive long-acting drug release properties, but also synchronously regulates epithelial tight junctions and significantly improves intestinal permeability. This innovative research provides a new strategy and theoretical basis for improving the oral bioavailability of hydrophobic anticancer chemotherapy drugs.
The carboxyl group on the alginate molecular chain can electrostatically interact with the positively charged protein in the colon mucosa to form a hydrophilic adsorption layer (Szekalska et al., 2023). Alginate can form gel-protected loaded active molecules in an acidic environment and is specifically degraded under the action of reduction to solution in the colonic environment, such as glucuronidase, which makes it an ideal colon-targeting carrier material (Lee et al., 2025). Studies have shown that the mucosal adhesion properties of alginate can significantly prolong the retention time of the drug at the absorption site, thereby effectively improving the bioavailability of the drug (Kolawole et al., 2023). In recent years, researchers have made breakthroughs in the development of alginate-based delivery systems. The Abbasi research team has innovatively designed a folate-functionalized alginate nanoparticle-hydrogel composite delivery system for carrying the hydrophobic anti-tumor drug diferuloylmethane (Abbasi et al., 2023). The delivery system skillfully integrates the advantages of a high drug load of nanoparticles, the slow-release property of hydrogel, and the active targeting function of the folic acid receptor, successfully overcoming the key problem of low bioavailability of diferuloylmethane in the treatment of CRC, and provides a new technical strategy for improving its therapeutic effect. In addition, Li and other researchers adopted advanced layer-by-layer assembly technology (Li et al., 2024) (Figure 2B). A probiotic microgel delivery system ((L.r@ (SA-CS)2) was constructed based on sodium alginate (SA) and chitosan (CS). This system not only significantly improves the survival rate of Lactobacillus reuteri (L.r) in the harsh gastrointestinal environment, but also achieves the controlled release of probiotics in the gut by precisely regulating the swelling and degradation behavior of the hydrogel. In vitro and in vivo experiments confirmed that the delivery system can effectively regulate the intestinal microecological balance, and showed a significant synergistic effect in anti-tumor therapy, providing a new idea for tumor microecological therapy.
2.2 Targeted NDDS
In recent years, NDDS, as an emerging therapeutic strategy, has shown great potential in tumor-targeted therapy (Batool et al., 2023). Excellent targeted delivery capabilities enable the precise delivery of drugs packaged in nanocarriers to the site of action, which can enhance therapeutic effectiveness and reduce side effects. The application of nanomedicine delivery systems in the treatment of CRC primarily employs two strategies: passive targeting and active targeting.
2.2.1 Passive targeting
It is generally accepted that solid tumors are usually highly heterogeneous, with significant differences in the vascular system depending on tumor type and stage of growth (Pe’er et al., 2021). It is worth noting that tumor tissues generally lack functional lymphatic vessels and there is a large gap between endothelial cells, which leads to the easy exosmosis or retention of macromolecules. This unique pathophysiological property promotes the selective accumulation of nanoparticles in tumor tissues, a phenomenon defined as enhanced permeability and retention (EPR) (Maeda et al., 2000). The passive targeting strategy based on the EPR effect can significantly improve the specific distribution of drugs in tumor tissues and minimize the toxic effect on normal tissues through the rational design of nanocarriers (such as liposomes, polymer nanoparticles, etc.).
Yuan et al. adopted silk fibroin (SF) as the carrier material of the drug delivery system, and successfully constructed a dual-drug co-loaded hydrogel system (Yuan et al., 2025). The team combined 5-FU in SF solution with curcumin dissolved in acetone solvent through precise process control to produce a nanoparticle drug delivery system with a particle size distribution ranging from 77.87 nm to 299.22 nm. It is worth noting that this composite nanosphere exhibits a significant EPR effect, which enables it to effectively enrich solid tumor tissue through a passive targeting mechanism, thus enhancing tumor therapy.
In view of the complex variability and potential toxicity of nanocarriers, Ji and other researchers have carried out innovative research based on natural small molecules (Ji et al., 2022) (Figure 3A). They found that honokiol (HK) can self-assemble through intermolecular hydrogen bonds and hydrophobic interactions to form stable NPs. This self-assembled system based on natural ingredients exhibits unique tumor-targeting properties: First, HK NPs can selectively accumulate at the tumor site thanks to the EPR effect; Subsequently, they enter the cell through endocytosis of tumor cells. Finally, under the weakly acidic condition characteristic of the TME, HK molecules are released from the NPs in a controlled manner, thereby maintaining a sustained drug concentration within the tumor cells and exerting its cytotoxic effects.
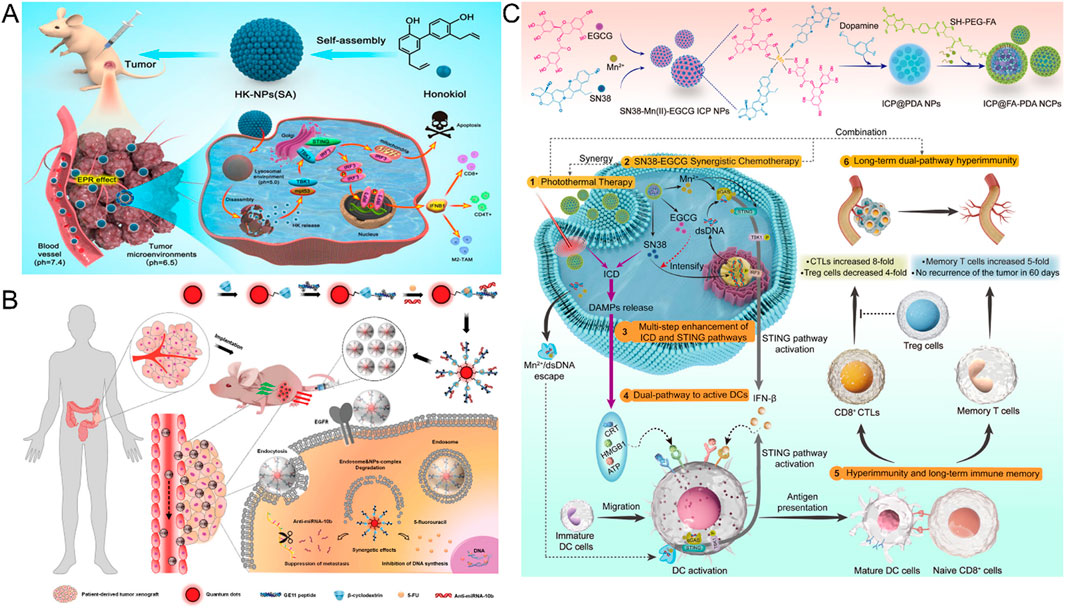
Figure 3. (A) With the EPR effect, the accumulation of HK NPs at the tumor site for the treatment of colorectal cancer (Reproduced with permission from the reference: Natural Small Molecules Enabled Efficient Immunotherapy through Supramolecular Self-Assembly in P53-Mutated Colorectal Cancer). Adapted from Ji et al., ACS Applied Materials and Interfaces, 2022, under a Creative Commons CC BY license. (B) Mechanism of GCD-targeted therapy in EGFR-overexpressing colorectal cancer (Reproduced with permission from the reference: Enhancing the Management of Metastatic Tumors by Robust Co-Delivery of 5-Fluorouracil/MicroRNA-10b Inhibitor Using EGFR-Targeted Nanovehicles). Adapted from Wang et al., Advanced Healthcare Materials, 2023, under a Creative Commons CC BY license. (C) Schematic synthesis and antitumor mechanism of ICP@FA-PDA (Reproduced with permission from the reference: Infinite Coordination Polymer Polydopamine Nanocomposites Dual-Pathway Multistep Induction of Long-Term Hyperimmunity Combined with Photothermal-Chemo Synergistic Therapy Colorectal Cancer). Adapted from Zhang et al., Aggregate, 2025, under a Creative Commons CC BY license.
However, the limitation of passive targeting is that its targeting is relatively weak, and it is greatly affected by tumor heterogeneity and individual differences (Ojha et al., 2017). Therefore, it is necessary to combine EPR-based NDDS with other mechanisms to enhance their targeting.
2.2.2 Active targeting
Active targeting, by contrast, modifies specific targeting molecules (such as antibodies, peptides, aptamers, etc.) on the surface of the nanocarriers so that they can accurately recognize and bind to specific antigens or receptors on the surface of tumor cells, thus achieving efficient drug delivery. Active targeting strategy not only significantly improves the targeting and therapeutic effect of drugs, but also further reduces systemic toxicity, providing a new idea for the precise treatment of CRC. In recent years, the design concept of a nano-drug-targeted delivery system for CRC is mainly based on the “ligand-receptor” specific binding principle (Xu et al., 2020). By screening specific receptors that are highly expressed in CRC cells and selecting the corresponding ligands for surface modification of the nanoparticles, the researchers realized the active targeted therapy of the drug on the tumor cells. Among many highly expressed tumor-related receptors, CD44, epidermal growth factor receptor (EGFR), folate receptor (FR) and transferrin receptor (TfR) are the most widely studied targets (Rao et al., 2013; Napolitano et al., 2024; Zhen et al., 2023b; Soe et al., 2019; Kim et al., 2023).
As a key regulatory molecule in tumor progression and metastasis, overexpression of CD44 has been shown to be significantly associated with poor prognosis in patients with rectal cancer (Du et al., 2008). In view of the important regulatory role of CD44 in tumor-targeted therapy, researchers have developed a variety of nano-delivery systems targeting CD44. Among them, Gonzalez-Valdivieso et al. innovatively designed a multifunctional nanohybrid system that integrates Akt inhibitors with DTX through chemical coupling and specifically binds to CD44 DNA aptamers (Gonzalez-Valdivieso et al., 2023). The experimental results showed that the nano-system had a significant selective inhibitory effect on CRC cells, reducing cell survival to 2.5%. It is worth noting that the specific recognition ability of hyaluronic acid (HA) and CD44 on the surface of tumor cells provides an important way to achieve active targeting. On this basis, Phatak et al. developed a novel dual-polymer coating system, which encapsulated sorafenib in an HA-modified poly-lactic-co-glycolic acid (PLGA) - polysarcosine (PSAR) dual-polymer shell (Phatak et al., 2023). The specific delivery of the drug to CD44 overexpressed CRC cells was successfully achieved. In addition, Hu et al. built NDDS by integrating HA with pheophorbide A (PPa) and NLG919 heterodimers (Hu et al., 2020). This system not only makes full use of the active targeting properties of HA, but also realizes the precise release of drugs at the target through the cleavage of NSP disulfide bond triggered by glutathione in the tumor microenvironment and HA degradation mediated by hyaluronidase. The introduction of this dual response mechanism significantly improves the tumor targeting and therapeutic efficacy of drugs, providing a new strategy for targeted therapy of CRC.
Activation of the EGFR and its downstream signaling pathway plays a key role in the onset and progression of CRC (Catalano and Trusolino, 2019). Monoclonal antibodies (MoAbs) targeting EGFR, such as cetuximab and panizumab, have made significant progress in the treatment of metastatic CRC (mCRC) (Stroh et al., 2010). To enhance the therapeutic effect, the researchers developed anti-EGFR antibodies or ligand-modified nanoparticles that are able to specifically target cancer cells with high EGFR expression. For example, Fang and his team synthesized mixed NPs “Cet-CINPs” and coupled their surfaces to cetuximab to achieve precise targeting of tumors (Fang et al., 2023). In addition, by introducing a photosensitizer into the NPs, the NDDS showed enhanced cell uptake and significant cytotoxic activity under near-infrared light irradiation, thus combining targeted therapy with chemical photothermal therapy and providing a new strategy for CRC treatment. In 2023, Wang et al. developed a nanocarrier called GCD (Wang D. et al., 2023) (Figure 3B). The vector is composed of GE11 peptide, bioprobe quantum dots (QD), and β-cyclodextrin (β-CD), which has EGFR targeting properties. GCD nanocarriers can simultaneously deliver different targets of 5-FU and anti-Mir-10b to CRC, especially in anti-CRC metastasis, showing potential application value. This study provides new ideas and tools for the precise treatment of CRC.
FR, as a target that is overexpressed on the surface of a variety of cancer cells but underexpressed in normal cells, has attracted much attention in the development of tumor-targeting nano-drug delivery systems (Frigerio et al., 2019). The specific binding of the folate-drug complex to the folate receptor can not only enhance the retention of the drug at the target site, but also effectively reduce the drug resistance of colon cancer cells (Mallik et al., 2024). Based on this principle, Ge et al. innovatively designed a F2-C-5-FU-FA constructed by the covalent connection of Angelica gigas polysaccharide (F2), carboxymethyl-5-fluorouracil (C-5-FU) and FA (Ge et al., 2024). The results of in vivo and in vitro experiments showed that the nano-conjugated compound showed excellent selectivity to cancer cells with high expression of folic acid receptor, and significantly reduced the non-specific toxicity of the drug to immune organs. Similarly, the Ma research team used the same strategy to prepare CFP2-C-5-FU-FA NPs, and confirmed through in vivo fluorescence imaging technology that the fluorescence signal intensity of the targeted nanoparticles in the tumor region was significantly higher than that of the non-targeted control group, and had lower cardiac toxicity (Ma et al., 2023). Zhang et al. developed a folate-modified polydopamine (PDA) infinite coordination polymer nanocomposite (ICP@FA-PDA) (Zhang X. et al., 2025) (Figure 3C). The system not only realizes the efficient utilization of drug components, but also has the ability of strong immune response induced by dual pathways and precise targeting mediated by folic acid. In vitro experimental data showed that the nanocomposite material had a 100% inhibition rate on primary tumors in mice, and no tumor recurrence was observed during the observation period of 60 days. In recent years, magnetic nanoparticles with superparamagnetic properties have been widely used in the biomedical field because of their ability to be regulated by external magnetic fields. Under this research background, Alnasraui et al. successfully designed and synthesized Fe3O4@Au-DEX-CP-FA multifunctional nanoparticles (Alnasraui et al., 2023). The system adopts the structure design of a gold shell-coated ferric oxide core, which not only enhances the stability of the nanoparticles, but also prevents the oxidation of the core. They integrated dexamethasone (DEX) and cyclophosphamide (CP) as active molecules into the nano-system, and functionalized folic acid in the outermost layer, thus achieving precise targeting of tumor sites.
Transferrin receptor (TfR) is highly expressed on the surface of various cancer cells, which provides a theoretical basis for TfR-mediated targeted drug delivery by Tf-modified nanoparticles (Trabolsi et al., 2022). In a related study, Cheng et al. innovationally used genetic engineering technology to construct recombinant mouse heavy chain ferritin (mHFn), which not only has a high affinity for TfR, but also contains a unique heat-sensitive channel structure (Cheng et al., 2024). Mitoxantrone (MTO) was successfully encapsulated in the internal cavity of mHFn (mHFn@MTO). Under the irradiation of 660 nm laser, the photothermal effect of MTO can rapidly increase the local temperature and trigger the opening of heat-sensitive channels, thus achieving rapid release of MTO in a heat-responsive manner and significantly inducing apoptosis of tumor cells. In addition, Wei et al. developed a transferrin binding peptide (TBP) functionalized polymer carrier system that specifically binds Tf and mediates the targeted delivery of doxorubicin (Dox) to TfR-overexpressed CRC cells (Wei et al., 2020). The experimental results showed that, compared with common polymer-doxorubicin complex (Ps-Dox), Tf@TBP-Ps-Dox had a longer blood circulation half-life and significantly enhanced tumor inhibition effect on HCT-116. It is worth noting that nanoparticles are easy to form protein corona (PC) in the biological fluid environment, and this surface-adsorbed biomolecular layer may mask the targeting ligand, resulting in loss of targeting function. To address this challenge, Zhang et al. designed a Tf functionalized superparamagnetic iron oxide nanoparticle (SPIO-Tf) based on remote magnetothermal stimulation, which was modified by polyethylene glycol and grafted with Tf on the surface (Zhang T. et al., 2025). It was found that after the application of alternating magnetic field (AMF) stimulation, the local heat generated by SPIO-Tf can induce PC recombination, so that the shielded Tf can be re-exposed to the surface of the nanoparticles, thus significantly improving its tumor-targeting efficiency.
In addition, mannose receptors (MR) and EpCAM (epithelial cell adhesion molecules) are also effective targets for CRC treatment (Dalle Vedove et al., 2018; Gires and Bauerle, 2010). Bai et al. constructed a new nanomedicine delivery system, RG@M- γ-CD CNPs (Bai et al., 2021). The core structure of the system is γ-cyclodextrin (γ-CD), and its hydrophobic cavity can effectively contain the antitumor drug regorafenib (RG). At the same time, mannose modification in the outer layer gives the nanosystem the ability to specifically target tumor cells. Ge et al. developed a bioconjugate consisting of EpCAM aptamers, polyethylene glycol, and dendrimers for specific delivery of celastrol to EPCAM-rich tumors to improve anti-tumor efficacy and mitigate toxicity (Ge et al., 2020). Due to the modification of EpCAM aptamer, the NDDS can actively target tumors, which greatly reduces the acute toxicity of celastrol. Since the particle size is less than 40 nm and has a high negative surface charge, it can efficiently penetrate the tumor upon reaching the tumor site.
The surface of NDDS can be conjugated with targeting moieties such as antibodies or ligands to specifically bind overexpressed receptors on target cell surfaces, thereby facilitating drug internalization and avoiding off-target effects. Different receptor-targeted NDDS exhibit unique advantages and limitations in CRC treatment, with performance disparities primarily stemming from the complexity of target biology, carrier design, and TME interactions.
CD44, as a hyaluronic acid receptor highly expressed on CRC stem cells, enables hyaluronic acid-based nanocarriers to effectively penetrate the tumor extracellular matrix and eliminate cancer stem cells, reducing recurrence risk (Wang K. et al., 2022). However, its widespread expression in normal tissues may induce off-target toxicity, while CD44 isoform heterogeneity limits targeting precision (Cisterna et al., 2016). EGFR-targeted systems achieve efficient drug delivery via receptor-mediated endocytosis, demonstrating significant efficacy in EGFR-overexpressing wild-type tumors. Nevertheless, drug resistance in KRAS-mutated CRC and EGFR signaling-induced immunosuppressive TME restrict therapeutic outcomes (Parseghian et al., 2019; Woolston et al., 2019). FR-targeted systems leverage the high-affinity interaction between folate and FR-α to precisely recognize FR-positive tumor cells, with the potential to cross the blood-brain barrier (BBB) for brain metastasis treatment. However, competition with plasma folate may reduce tumor accumulation (Wang K. et al., 2022). TfR-targeted systems selectively deliver drugs to highly proliferative tumor cells via iron-dependent receptor-mediated endocytosis, however, TfR expression in normal tissue cells limits safety (Bajracharya et al., 2022).
Collectively, these systems face challenges including TME heterogeneity, immune clearance, and scalability. Future development requires integrating multitargeted strategies to overcome heterogeneity, designing smart responsive carriers for TME-specific drug release, and optimizing patient stratification via personalized medicine. Despite technical bottlenecks, receptor-targeted nano-systems represent a promising innovation in CRC therapy, with clinical translation dependent on deep interdisciplinary integration of materials science, molecular biology, and clinical research.
2.3 NDDS based on TME
TME is a complex ecosystem composed of malignant and non-malignant cells, an extracellular matrix, and various biomolecules (Li Y. et al., 2022), playing a crucial role in tumor progression and therapy resistance. TME is not only a key driver of tumor progression but also an important basis for tumor resistance therapy and immune escape (Xiao and Yu, 2021). Typical features of TME include moderate acidity (pH 6.5–7.0), excessive secretion of reactive oxygen species (such as H2O2), and reducing molecules (such as glutathione and GSH) (Chen et al., 2024). Together, these features create a highly dynamic and heterogeneous environment that promotes tumor cell proliferation, invasion, and metastasis. Overall, the dynamic changes in acidity, redox status, and specific metabolites of TME not only reflect the specific physiological characteristics of tumors, but also provide potential targets for the development of therapeutic strategies against TME.
2.3.1 pH-responsive NDDS
Even when oxygen is plentiful, tumor cells tend to metabolize glucose through glycolysis rather than efficient oxidative phosphorylation. Glycolysis produces large amounts of lactic acid, resulting in a decrease in intracellular and extracellular pH (Warburg, 1925). At the same time, the enhancement of proton pump activity, abnormal blood vessels, overexpression of carbonic anhydrase, and regulation of hypoxia-inducing factors all work together to cause significant acidification of TME (Dorai et al., 2005).
Song has developed Cet-coupled DMAKO-20/PCL-PEOz micelles (DMAKO@PCL-PEOz-Cet) in which DMAKO-20 has been shown to have specific antitumor activity against CRC, but its hydrophobicity has been a puzzle (Song et al., 2024). Amphiphilic PCL-PEOZ solves this problem well and has been shown to promote the release of encapsulated drugs in acidic tumor environments due to the crystallinity of PCL and the pH sensitivity of PEOz. Similarly, Bu and Li et al. also demonstrated that using PCL-PEOz as a carrier to deliver DOX has a good cell-killing effect on tumor cells (Bu et al., 2019; Li et al., 2017).
Jia et al. developed a bifocal nano-delivery system (FCNP) based on DSPE-Hyd-mPEG for the co-delivery of fruquintinib (Fru) and siCCAT1 (Jia et al., 2023). The nanocomplex achieves specific accumulation at the tumor site through the EPR effect. Under acidic conditions in the tumor microenvironment, DSPE-Hyd-mPEG degrades in a pH response, resulting in the release of FCNP. Among them, Fru first acts on the tumor vascular system and exerts its rapid anti-angiogenesis effect. Subsequently, SICCAT1-loaded nanoparticles (CNP) were specifically taken up by tumor cells through receptor-mediated endocytosis, significantly improving the bioavailability of therapeutic molecules in tumor cells.
Malignant cells typically exhibit higher levels of reactive oxygen species (ROS) accumulation compared to normal cells. Based on this property, Su and his team developed an innovative pH and ROS dual-response drug delivery system (TPDM/PGA) (Su et al., 2024) (Figure 4A). The system specifically delivers DHA and MTO into tumor cells by the following mechanism: In the acidic TME, the carboxyl group in PGA undergoes deprotonation, resulting in the dissociation of PGA from the complex, exposing TPDM. Because TPDM is able to break down in response to the high concentration of ROS in cancer cells, DHA and MTO are released. These drugs further induce the production of more ROS, forming a positive feedback loop that accelerates drug release and exacerbates oxidative stress. This process not only enhances the immunogenicity of the tumor, but also significantly inhibits the growth of the tumor.
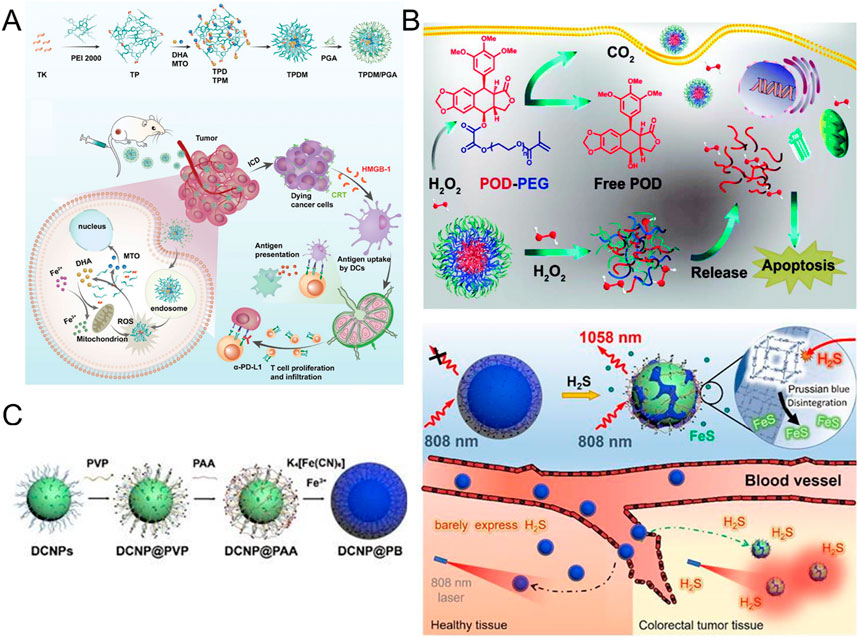
Figure 4. (A) Synthesis and tumor immunotherapy mechanisms of TPDM/PGA (Reproduced with permission from the reference: pH and ROS Dual-Responsive Autocatalytic Release System Potentiates Immunotherapy of Colorectal Cancer). Adapted from Su et al., Advanced Healthcare Materials, 2024, under a Creative Commons CC BY license. (B) Mechanism of action of H2O2-responsive POD-PEG NPs for cancer treatment (Reproduced with permission from the reference: H2O2-responsive nano-prodrug for podophyllotoxin delivery). Adapted from Ou et al., Biomaterials Science, 2019, under a Creative Commons CC BY license. (C) Preparation of DCNP@PB and the mechanism for the specific detection of H2S (Reproduced with permission from the reference: A H2S-activated NIR-II imaging probe for precise diagnosis and pathological evaluation of colorectal tumor). Adapted from Ji et al., Theranostics, 2025, under a Creative Commons CC BY license.
2.3.2 GSH-responsive NDDS
GSH, as an important antioxidant, plays a key role in TME. Tumor cells promote the production and accumulation of GSH through the upregulation of key enzymes of GSH synthesis and activation of the Nrf2-Keap1 antioxidant signaling pathway (Zhang, 2010). In addition, hypoxia and acidic conditions also induce excessive secretion of GSH. On the one hand, high levels of GSH can neutralize ROS in tumor cells, protect tumor cells from oxidative damage, and inhibit anti-tumor immune response by regulating the function of immune cells (Niu et al., 2021). Therefore, the development of GSH consumables and GSH-responsive nanomedicines is a new direction in the treatment of tumors (Li Y. et al., 2023).
Li et al. developed an anticancer nano-delivery system Cu-Olsa@HA, and HA enhances its targeting and EPR effects (Li J. et al., 2022). In addition, the system takes advantage of the high concentration of GSH in the tumor microenvironment to achieve a valence transition between Cu2+ and Cu+. This transformation process not only effectively promotes the conversion of H2O2 to molecular oxygen O2, but also generates singlet oxygen 1O2 and hydroxyl radical OH with high cytotoxicity. This cascade eventually leads to the imbalance of redox homeostasis in tumor cells, thereby exerting its antitumor effect.
Yang et al. successfully reported a new nanoparticle pHCT74/MOF-5@DHA&CORM-401 in 2024. The nanoparticle design cleverly combines the specific targeting function of pHCT74 with the pH/GSH dual-responsive properties of metal-organic Framework 5 (MOF-5) (Yang et al., 2024a). pHCT74 precisely directs nanoparticle enrichment at tumor sites, while MOF-5 ensures the rapid release of active ingredients such as DHA and carbon monoxide-releasing molecule 401 (CORM-401) under acidic conditions in the tumor microenvironment. It not only promoted ROS-mediated ferroptosis and apoptosis, but also triggered immunogenic cell death (ICD).
2.3.3 H2O2-responsive NDDS
In TME, the generation and metabolism imbalance of ROS is one of the important characteristics of tumorigenesis and development. H2O2, one of the main members of ROS, is often secreted in excess in the tumor microenvironment, and this phenomenon has been reported in multiple cancer types (Zaher et al., 2024). Excess H2O2 causes oxidative stress, DNA damage, protein oxidation, and lipid peroxidation, which induces apoptosis or necrosis (Rani et al., 2016). However, tumor cells often resist this oxidative damage by upregulating antioxidant defense mechanisms, thus surviving the harsh microenvironment. The excessive secretion of H2O2 in the tumor microenvironment is one of the important mechanisms for tumor cells to adapt to the harsh environment, promote their own growth, and evade immune surveillance (Wu, 2006). Therefore, nanomedicine targeting H2O2-responsive forms may become a new strategy for cancer treatment in the future.
Ou et al. used the H2O2-sensitive oxalate bond as the linking group to covalently link PEGMA to podophyllotoxin (POD) molecules and successfully constructed an amphiphilic prodrug molecule (POD-PEG) with H2O2 response characteristics (Ou et al., 2019) (Figure 4B). The prodrug molecules can form stable nanoparticles (POD-PEG-NPs) by self-assembly in aqueous solution. Benefit from EPR effect This NP accumulates specifically in tumor tissue. More importantly, based on the high H2O2 of TME, the oxalate bond in the prodrug molecule is broken, thus the POD molecule with anti-tumor activity is controllably released, and tumor-specific treatment is realized.
2.3.4 H2S-responsive NDDS
In CRC, a significant physiological feature of TME is the high expression of endogenous hydrogen sulfide (H2S) (0.3–3.4 mM) (An et al., 2018). This phenomenon is mainly attributed to the selective upregulation of Cystathionine β-synthase (CBS) (Szabo et al., 2013). CBS is a key enzyme in the H2S biosynthetic pathway, and its overexpression leads to the accumulation of H2S in tumor tissues (Hellmich et al., 2015). This makes therapeutic intervention targeting the CBS-H2S axis likely to become an important direction of precision medicine in the future.
Chang et al. first used advanced nanomaterials synthesis technology to successfully prepare Cu2O nanoparticles with hollow mesoporous structures (Chang et al., 2020). A core-shell Cu2O@CaCO3 composite nanomaterial is formed by coating a CaCO3 shell uniformly on the Cu2O surface. Subsequently, HA was used for surface modification to enhance the tumor targeting of the material. The CaCO3 shell decomposes in the acidic microenvironment of tumor tissue, and Cu2O is converted to Cu2-xS with high activity under the action of endogenous H2S. This conversion not only gives the material excellent photothermal conversion performance, but also significantly enhances its photocatalytic activity, enabling efficient treatment of CRC.
Recently, Ji et al. made an important breakthrough in the field of accurate diagnosis and pathological evaluation of colorectal tumors (Ji et al., 2025) (Figure 4C). They successfully developed an H2S-activated NIR-II imaging probe consisting of Nd3+ doped down-conversion nanoparticles (DCNPs) coated with a Prussian blue (PB) layer, DCNP@PB. In this innovative design, PB not only acts as a response group for H2S, but also plays a key role in regulating the emission characteristics of DCNP@PB. Specifically, the coating of PB leads to a significant quenching of the luminescence of DCNP, which is mainly due to the overlapping effect between the absorption spectrum of PB and the excitation/emission spectrum of DCNP. However, when the probe arrived at the tumor site, Fe (III) in PB would react with the highly expressed H2S at the tumor site, thereby triggering the decomposition of the PB layer, resulting in a significant decrease in PB absorbance. This process allows the luminescence of DCNPs to be restored, thereby illuminating colorectal tumors with high sensitivity and specificity. It is particularly worth mentioning that the H2S response range of DCNP@PB is highly matched with the H2S concentration at the tumor site, which provides a strong guarantee for the specific detection of colorectal tumors in a complex physiological environment (Table 1).
3 Discussion
As a malignant tumor with high morbidity and mortality, the treatment strategy of CRC is undergoing a transformation from traditional chemotherapy and radiotherapy to targeted therapy and immunotherapy. In recent years, nanomedicine delivery systems, as an emerging therapeutic approach, have shown great potential in the field of CRC treatment. By encapsulating chemotherapeutic agents, targeted drugs, or immunomodulators in nanocarriers, NDDS can effectively improve drug targeting, minimize toxic side effects, enhance therapeutic efficacy, and overcome tumor resistance. Novel NDDS are also being progressively developed. Extracellular vesicles (EVs), as emerging nanomaterials, have garnered extensive attention. Endogenous EVs can reduce immune clearance, thereby demonstrating natural biocompatibility. Meanwhile, their high stability and affinity for tumor cells enable them to carry drugs to tumor sites for therapeutic effects (Yang et al., 2024b). Viral nanoparticles (VNPs) hold unique application prospects in gene therapy due to their efficient gene delivery capability and specific cell recognition. They can self-assemble into capsids to encapsulate therapeutic agents while retaining antigenic properties to induce immune responses, thus being utilized in vaccine development (Sun et al., 2024).
Although NDDS enhance drug efficacy through targeted delivery, microenvironment-responsive strategies, and multimodal synergies, their clinical translation remains challenging. The tumor microenvironment restricts precise drug delivery to all tumor regions, contributing to suboptimal therapeutic outcomes. While immune cells can target tumor cells, augmented immunosuppression weakens their infiltration and targeting capabilities. Additionally, due to limitations in preclinical research, insufficient comparative data on the efficacy and safety of different nanodrug types exist, making comprehensive evaluations unattainable. NDDS also face challenges in large-scale production due to inadequate production processes, difficult cost control, and susceptibility to stability issues during storage and transportation.
Therefore, based on this review, future NDDS development should prioritize safety and efficacy by developing advanced nanocarrier materials with enhanced biocompatibility, biodegradability, and targeting capability. Concurrently, tumor site-specific intelligent responsive, and dynamically regulated nano-systems must be designed to achieve precise drug release in the TME and minimize nonspecific toxic effects. Nano-drug design should be optimized through surface modification and drug co-loading strategies to explore synergistic mechanisms with immune checkpoint inhibitors, oncolytic viruses, etc., thereby further enhancing targeting specificity and therapeutic efficacy. In-depth investigations into NDDS-TME interactions are critical to elucidate distribution, metabolism, and clearance mechanisms within tumor tissues, providing a theoretical foundation for optimizing nanodrug design. Personalized nano-drug combinations should be customized based on CRC molecular typing and gut microbiota characteristics to achieve precision therapy. Simultaneously, innovative production processes are needed to reduce costs and improve batch consistency. Strengthening the interface between basic research and clinical translation will facilitate the transition of nanodrugs from bench to bedside. In summary, NDDS represent a promising strategy for CRC treatment. With continuous advancements in nanotechnology, NDDS are poised to play an increasingly pivotal role in improving therapeutic outcomes for colorectal cancer patients.
Author contributions
MC: Data curation, Funding acquisition, Investigation, Methodology, Writing – original draft. SW: Resources, Writing – original draft. YC: Resources, Writing – original draft. XP: Data curation, Funding acquisition, Validation, Writing – review and editing. XZ: Conceptualization, Supervision, Writing – review and editing.
Funding
The author(s) declare that financial support was received for the research and/or publication of this article. This work was supported by the Natural Science Basic Research Program of Shaanxi (Program No. 2024JC-YBQN-0929), Xi’an Science and Technology Plan Project (grant No. 23YXYJ0067), Xi’an Administration of Traditional Chinese Medicine Project (grant No. SZZ202402). This work was also supported by the National Natural Science Foundation of China (No. 82300336).
Conflict of interest
The authors declare that the research was conducted in the absence of any commercial or financial relationships that could be construed as a potential conflict of interest.
The reviewers WT and YJ declared a shared affiliation with one of the authors XZ to the handling editor at the time of the review.
Generative AI statement
The author(s) declare that no Generative AI was used in the creation of this manuscript.
Publisher’s note
All claims expressed in this article are solely those of the authors and do not necessarily represent those of their affiliated organizations, or those of the publisher, the editors and the reviewers. Any product that may be evaluated in this article, or claim that may be made by its manufacturer, is not guaranteed or endorsed by the publisher.
References
Abbasi, M., Sohail, M., Minhas, M. U., Mahmood, A., Shah, S. A., Munir, A., et al. (2023). Folic acid-decorated alginate nanoparticles loaded hydrogel for the oral delivery of diferourylmethane in colorectal cancer. Int. J. Biol. Macromol. 233, 123585. doi:10.1016/j.ijbiomac.2023.123585
Adami, H.-O., Bretthauer, M., Emilsson, L., Hernán, M. A., Kalager, M., Ludvigsson, J. F., et al. (2016). The continuing uncertainty about cancer risk in inflammatory bowel disease. Gut 65 (6), 889–893. doi:10.1136/gutjnl-2015-311003
Alnasraui, A. H. F., Joe, I. H., and Al-Musawi, S. (2023). Design and synthesize of folate decorated Fe3O4@Au-DEX-CP nano formulation for targeted drug delivery in colorectal cancer therapy: in vitro and in vivo studies. J. Drug Deliv. Sci. Technol. 87, 104798. doi:10.1016/j.jddst.2023.104798
An, L., Wang, X., Rui, X., Lin, J., Yang, H., Tian, Q., et al. (2018). The in situ sulfidation of Cu2O by endogenous H2S for colon cancer theranostics. Angew. Chem. Int. Ed. 57 (48), 15782–15786. doi:10.1002/anie.201810082
Bai, H., Wang, J., Phan, C. U., Chen, Q., Hu, X., Shao, G., et al. (2021). Cyclodextrin-based host-guest complexes loaded with regorafenib for colorectal cancer treatment. Nat. Commun. 12 (1), 759. doi:10.1038/s41467-021-21071-0
Bajracharya, R., Baral, K., Lee, S., Song, J., and Han, H.-K. (2023). Organometallic phyllosilicate-gold nanocomplex: an effective oral delivery system of methotrexate for enhanced in vivo efficacy against colorectal cancer. Int. J. Nanomedicine 18, 7257–7266. doi:10.2147/ijn.S437860
Bajracharya, R., Song, J. G., Patil, B. R., Lee, S. H., Noh, H. M., Kim, D. H., et al. (2022). Functional ligands for improving anticancer drug therapy: current status and applications to drug delivery systems. Drug Deliv. 29 (1), 1959–1970. doi:10.1080/10717544.2022.2089296
Bano, R., Soleja, N., and Mohsin, M. (2023). Genetically encoded FRET-based nanosensor for real-time monitoring of A549 exosomes: early diagnosis of cancer. Anal. Chem. 95 (13), 5738–5746. doi:10.1021/acs.analchem.2c05774
Bashiardes, S., and Christodoulou, C. (2024). Orally administered drugs and their complicated relationship with our gastrointestinal tract. Microorganisms 12 (2), 242. doi:10.3390/microorganisms12020242
Batool, S., Sohail, S., ud Din, F., Alamri, A. H., Alqahtani, A. S., Alshahrani, M. A., et al. (2023). A detailed insight of the tumor targeting using nanocarrier drug delivery system. Drug Deliv. 30 (1), 2183815. doi:10.1080/10717544.2023.2183815
Bu, L., Zhang, H., Xu, K., Du, B., Zhu, C., and Li, Y. (2019). pH and reduction dual-responsive micelles based on novel polyurethanes with detachable poly(2-ethyl-2-oxazoline) shell for controlled release of doxorubicin. Drug Deliv. 26 (1), 300–308. doi:10.1080/10717544.2019.1580323
Catalano, I., and Trusolino, L. (2019). The stromal and immune landscape of colorectal cancer progression during anti-EGFR therapy. Cancer Cell. 36 (1), 1–3. doi:10.1016/j.ccell.2019.06.001
Chai, M., Gao, B., Wang, S., Zhang, L., Pei, X., Yue, B., et al. (2025). Leveraging plant-derived nanovesicles for advanced nucleic acid-based gene therapy. Theranostics 15 (1), 324–339. doi:10.7150/thno.104507
Chang, M., Hou, Z., Jin, D., Zhou, J., Wang, M., Wang, M., et al. (2020). Colorectal tumor microenvironment-activated bio-decomposable and metabolizable Cu2O@CaCO3 nanocomposites for synergistic oncotherapy. Adv. Mater. 32 (43), 2004647. doi:10.1002/adma.202004647
Chehelgerdi, M., Chehelgerdi, M., Allela, O. Q. B., Pecho, R. D. C., Jayasankar, N., Rao, D. P., et al. (2023). Progressing nanotechnology to improve targeted cancer treatment: overcoming hurdles in its clinical implementation. Mol. Cancer 22 (1), 169. doi:10.1186/s12943-023-01865-0
Chen, C., Beloqui, A., and Xu, Y. (2023). Oral nanomedicine biointeractions in the gastrointestinal tract in health and disease. Adv. Drug Deliv. Rev. 203, 115117. doi:10.1016/j.addr.2023.115117
Chen, W., Hu, F., Gao, Q., Zheng, C., Bai, Q., Liu, J., et al. (2024). Tumor acidification and GSH depletion by bimetallic composite nanoparticles for enhanced chemodynamic therapy of TNBC. J. Nanobiotech. 22 (1), 98. doi:10.1186/s12951-024-02308-8
Cheng, J., Li, J., Yu, Q., Li, P., Huang, J., Li, J., et al. (2024). Laser-activable murine ferritin nanocage for chemo-photothermal therapy of colorectal cancer. J. Nanobiotech. 22 (1), 297. doi:10.1186/s12951-024-02566-6
Chu, A. H. Y., Lin, K., Croker, H., Kefyalew, S., Markozannes, G., Tsilidis, K. K., et al. (2025). Dietary-lifestyle patterns and colorectal cancer risk: global cancer update programme (CUP global) systematic literature review. Am. J. Clin. Nutr. S0002-9165 (25), 00014–20. doi:10.1016/j.ajcnut.2025.01.014
Chuang, S. T., Conklin, B., Stein, J. B., Pan, G., and Lee, K.-B. (2022). Nanotechnology-enabled immunoengineering approaches to advance therapeutic applications. Nano Converg. 9 (1), 19. doi:10.1186/s40580-022-00310-0
Cisterna, B. A., Kamaly, N., Choi, W. I., Tavakkoli, A., Farokhzad, O. C., and Vilos, C. (2016). Targeted nanoparticles for colorectal cancer. Nanomedicine (Lond) 11 (18), 2443–2456. doi:10.2217/nnm-2016-0194
Cole, H., Bryan, D., Lancaster, L., Mawas, F., and Vllasaliu, D. (2018). Chitosan nanoparticle antigen uptake in epithelial monolayers can predict mucosal but not systemic in vivo immune response by oral delivery. Carbohydr. Polym. 190, 248–254. doi:10.1016/j.carbpol.2018.02.084
Collnot, E.-M., Ali, H., and Lehr, C.-M. (2012). Nano- and microparticulate drug carriers for targeting of the inflamed intestinal mucosa. J. Control. Release 161 (2), 235–246. doi:10.1016/j.jconrel.2012.01.028
Dalle Vedove, E., Costabile, G., and Merkel, O. M. (2018). Mannose and mannose-6-phosphate receptor–targeted drug delivery systems and their application in cancer therapy. Adv. Healthc. Mater. 7 (14), 1701398. doi:10.1002/adhm.201701398
Damianos, J., Abdelnaem, N., and Camilleri, M. (2025). Gut goo: physiology, diet, and therapy of intestinal mucus and biofilms in gastrointestinal health and disease. Clin. Gastro. Hepato. 23 (2), 205–215. doi:10.1016/j.cgh.2024.09.007
das Neves, J., Sverdlov Arzi, R., and Sosnik, A. (2020). Molecular and cellular cues governing nanomaterial–mucosae interactions: from nanomedicine to nanotoxicology. Chem. Soc. Rev. 49 (14), 5058–5100. doi:10.1039/C8CS00948A
Di, J., Xie, F., and Xu, Y. (2020). When liposomes met antibodies: drug delivery and beyond. Adv. Drug Deliv. Rev. 154-155, 151–162. doi:10.1016/j.addr.2020.09.003
Dorai, T., Sawczuk, I. S., Pastorek, J., Wiernik, P. H., and Dutcher, J. P. (2005). The role of carbonic anhydrase IX overexpression in kidney cancer. Eur. J. Cancer 41 (18), 2935–2947. doi:10.1016/j.ejca.2005.09.011
Du, L., Wang, H., He, L., Zhang, J., Ni, B., Wang, X., et al. (2008). CD44 is of functional importance for colorectal cancer stem cells. Clin. Cancer Res. 14 (21), 6751–6760. doi:10.1158/1078-0432.Ccr-08-1034
Ejazi, S. A., Louisthelmy, R., and Maisel, K. (2023). Mechanisms of nanoparticle transport across intestinal tissue: an oral delivery perspective. ACS Nano 17 (14), 13044–13061. doi:10.1021/acsnano.3c02403
Elmorshedy, Y. M., Teleb, M., Sallam, M. A., Elkhodairy, K. A., Bahey-El-Din, M., Ghareeb, D. A., et al. (2023). Engineered microencapsulated lactoferrin nanoconjugates for oral targeted treatment of colon cancer. Biomacromolecules 24 (5), 2149–2163. doi:10.1021/acs.biomac.3c00037
Fang, F., Zhang, X., Tang, J., Wang, Y., Xu, J., and Sun, Y. (2023). EGFR-targeted hybrid lipid nanoparticles for chemo-photothermal therapy against colorectal cancer cells. Chem. Phys. Lipids 251, 105280. doi:10.1016/j.chemphyslip.2023.105280
Farooqui, M. W., Gustafson, N., Patel, N., Danelski, E., Patel, T., Srinivasan, K., et al. (2020). Bevacizumab induced proteinuria and hypertension: a comparative analysis of anti-hypertensives utilized within a single institution. J. Clin. Oncol. 38 (15), e15631. doi:10.1200/JCO.2020.38.15_suppl.e15631
Frigerio, B., Bizzoni, C., Jansen, G., Leamon, C. P., Peters, G. J., Low, P. S., et al. (2019). Folate receptors and transporters: biological role and diagnostic/therapeutic targets in cancer and other diseases. J. Exp. and Clin. Cancer Res. 38 (1), 125. doi:10.1186/s13046-019-1123-1
Ge, P., Niu, B., Wu, Y., Xu, W., Li, M., Sun, H., et al. (2020). Enhanced cancer therapy of celastrol in vitro and in vivo by smart dendrimers delivery with specificity and biosafety. Chem. Eng. J. 383, 123228. doi:10.1016/j.cej.2019.123228
Ge, Y., Kwon, M.-H., Kou, F., Uthamapriya, R. A., Zhang, P., Lee, D.-J., et al. (2024). Folic-acid-targeted drug delivery system implementing Angelica gigas polysaccharide: a potential strategy for colorectal cancer treatment. Int. J. Biol. Macromol. 283, 137653. doi:10.1016/j.ijbiomac.2024.137653
Gerweck, L. E., and Seetharaman, K. (1996). Cellular pH gradient in tumor versus normal tissue: potential exploitation for the treatment of cancer. Cancer Res. 56 (6), 1194–1198.
Gires, O., and Bauerle, P. A. (2010). EpCAM as a target in cancer therapy. J. Clin. Oncol. 28 (15), e239–e240. doi:10.1200/jco.2009.26.8540
Gonzalez-Valdivieso, J., Vallejo, R., Rodriguez-Rojo, S., Santos, M., Schneider, J., Arias, F. J., et al. (2023). CD44-targeted nanoparticles for co-delivery of docetaxel and an Akt inhibitor against colorectal cancer. Biomater. Adv. 154, 213595. doi:10.1016/j.bioadv.2023.213595
Gupta, A., Dhiman, A., Sood, A., Bharadwaj, R., Silverman, N., and Agrawal, G. (2023). Dextran/eudragit S-100 based redox sensitive nanoparticles for colorectal cancer therapy. Nanoscale 15 (7), 3273–3283. doi:10.1039/D3NR00248A
Hellmich, M. R., Coletta, C., Chao, C., and Szabo, C. (2015). The therapeutic potential of cystathionine β-synthetase/hydrogen sulfide inhibition in cancer. Antioxidants and Redox Signal. 22 (5), 424–448. doi:10.1089/ars.2014.5933
Hu, X., Hou, B., Xu, Z., Saeed, M., Sun, F., Gao, Z., et al. (2020). Supramolecular prodrug nanovectors for active tumor targeting and combination immunotherapy of colorectal cancer. Adv. Sci. 7 (8), 1903332. doi:10.1002/advs.201903332
Hultcrantz, R. (2021). Aspects of colorectal cancer screening, methods, age and gender. J. Intern. Med. 289 (4), 493–507. doi:10.1111/joim.13171
Ji, H., Wang, W., Li, X., Han, X., Zhang, X., Wang, J., et al. (2022). Natural small molecules enabled efficient immunotherapy through supramolecular self-assembly in P53-mutated colorectal cancer. ACS Appl. Mater. and Interfaces 14 (2), 2464–2477. doi:10.1021/acsami.1c16737
Ji, Y., Huang, Q., Jia, Q., Yan, H., Chi, Y., Jia, Y., et al. (2025). A H2S-activated NIR-II imaging probe for precise diagnosis and pathological evaluation of colorectal tumor. Theranostics 15 (1), 189–201. doi:10.7150/thno.103999
Jia, F., Li, Y., Gao, Y., Wang, X., Lu, J., Cui, X., et al. (2023). Sequential-delivery nanocomplex for combined anti-angiogenesis and gene therapy against colorectal cancer. Int. J. Pharm. 637, 122850. doi:10.1016/j.ijpharm.2023.122850
Kassem, A. M., Almukainzi, M., Faris, T. M., Ibrahim, A. H., Anwar, W., Elbahwy, I. A., et al. (2024). A pH-sensitive silica nanoparticles for colon-specific delivery and controlled release of catechin: optimization of loading efficiency and in vitro release kinetics. Eur. J. Pharm. Sci. 192, 106652. doi:10.1016/j.ejps.2023.106652
Kendall, R. A., Alhnan, M. A., Nilkumhang, S., Murdan, S., and Basit, A. W. (2009). Fabrication and in vivo evaluation of highly pH-responsive acrylic microparticles for targeted gastrointestinal delivery. Eur. J. Pharm. Sci. 37 (3), 284–290. doi:10.1016/j.ejps.2009.02.015
Kim, H., Villareal, L. B., Liu, Z., Haneef, M., Falcon, D. M., Martin, D. R., et al. (2023). Transferrin receptor-mediated iron uptake promotes colon tumorigenesis. Adv. Sci. 10 (10), 2207693. doi:10.1002/advs.202207693
Kolawole, O. M., Ifeanafor, A. R., Ifade, W. A., Akinleye, M. O., Patrojanasophon, P., Silva, B. O., et al. (2023). Formulation and evaluation of paclitaxel-loaded boronated chitosan/alginate nanoparticles as a mucoadhesive system for localized cervical cancer drug delivery. J. Drug Deliv. Sci. Technol. 87, 104810. doi:10.1016/j.jddst.2023.104810
Koziolek, M., Grimm, M., Becker, D., Iordanov, V., Zou, H., Shimizu, J., et al. (2015). Investigation of pH and temperature profiles in the GI tract of fasted human subjects using the Intellicap® system. J. Pharm. Sci. 104 (9), 2855–2863. doi:10.1002/jps.24274
Lee, J., Kim, J., Kwak, D., Kim, H., Ullah, M., Kim, M. C., et al. (2025). On-site sol-gel-sol transition of alginate enables reversible shielding/deshielding of tumor cell-activated nanoconjugates for precise local colorectal cancer therapy. Chem. Eng. J. 505, 158935. doi:10.1016/j.cej.2024.158935
Li, J., Zhang, Z., Li, J., Cun, J.-E., Pan, Q., Gao, W., et al. (2022a). Copper-olsalazine metal-organic frameworks as a nanocatalyst and epigenetic modulator for efficient inhibition of colorectal cancer growth and metastasis. Acta Biomater. 152, 495–506. doi:10.1016/j.actbio.2022.08.076
Li, N., Niu, L., Liu, Y., Wang, Y., Su, X., Xu, C., et al. (2024). Taking SCFAs produced by Lactobacillus reuteri orally reshapes gut microbiota and elicits antitumor responses. J. Nanobiotech. 22 (1), 241. doi:10.1186/s12951-024-02506-4
Li, X., Ma, Y., Xin, Y., Ma, F., and Gao, H. (2023a). Tumor-targeting nanoassembly for enhanced colorectal cancer therapy by eliminating intratumoral fusobacterium nucleatum. ACS Appl. Mater. and Interfaces 15 (11), 14164–14172. doi:10.1021/acsami.3c01210
Li, X., Zhang, S., Sheng, H., Zhen, Y., Wu, B., Li, Z., et al. (2025). Oral Fusobacterium nucleatum resists the acidic pH of the stomach due to membrane erucic acid synthesized via enoyl-CoA hydratase-related protein FnFabM. J. Oral Microbiol. 17 (1), 2453964. doi:10.1080/20002297.2025.2453964
Li, Y., Baiyang, L., Leran, B., Zhen, W., Yandong, X., Baixiang, D., et al. (2017). Reduction-responsive PEtOz-SS-PCL micelle with tailored size to overcome blood–brain barrier and enhance doxorubicin antiglioma effect. Drug Deliv. 24 (1), 1782–1790. doi:10.1080/10717544.2017.1402218
Li, Y., Chen, W., Kang, Y., Zhen, X., Zhou, Z., Liu, C., et al. (2023b). Nanosensitizer-mediated augmentation of sonodynamic therapy efficacy and antitumor immunity. Nat. Commun. 14 (1), 6973. doi:10.1038/s41467-023-42509-7
Li, Y., Chen, Z., Gu, L., Duan, Z., Pan, D., Xu, Z., et al. (2022b). Anticancer nanomedicines harnessing tumor microenvironmental components. Expert Opin. Drug Deliv. 19 (4), 337–354. doi:10.1080/17425247.2022.2050211
Liu, G., Franssen, E., Fitch, M. I., and Warner, E. (1997). Patient preferences for oral versus intravenous palliative chemotherapy. J. Clin. Oncol. 15 (1), 110–115. doi:10.1200/jco.1997.15.1.110
Liu, Z., Hu, Y., Xie, H., Chen, K., Wen, L., Fu, W., et al. (2024). Single-cell chromatin accessibility analysis reveals the epigenetic basis and signature transcription factors for the molecular subtypes of colorectal cancers. Cancer Discov. 14 (6), 1082–1105. doi:10.1158/2159-8290.Cd-23-1445
Lu, H., Yang, G., Ran, F., Gao, T., Sun, C., Zhao, Q., et al. (2020). Polymer-functionalized mesoporous carbon nanoparticles on overcoming multiple barriers and improving oral bioavailability of Probucol. Carbohydr. Polym. 229, 115508. doi:10.1016/j.carbpol.2019.115508
Ma, N., Kwon, M.-H., Palanisamy, S., Ge, Y., Zhang, Y., Kou, F., et al. (2023). A novel sulfated mannan-carboxymethyl-5-fluorouracil-folic acid conjugates for targeted anticancer drug delivery. Carbohydr. Polym. 304, 120454. doi:10.1016/j.carbpol.2022.120454
Maeda, H., Wu, J., Sawa, T., Matsumura, Y., and Hori, K. (2000). Tumor vascular permeability and the EPR effect in macromolecular therapeutics: a review. J. Control. Release 65 (1), 271–284. doi:10.1016/S0168-3659(99)00248-5
Mallik, R., Saha, M., Ghosh, B., Chauhan, N., Mohan, H., Kumaran, S. S., et al. (2024). Folate receptor targeting Mn(II) complex encapsulated porous silica nanoparticle as an MRI contrast agent for early-state detection of cancer. Small 21 (7), e2401787. doi:10.1002/smll.202401787
Mangone, L., Zizzo, M., Nardecchia, M., Marinelli, F., Bisceglia, I., Braghiroli, M. B., et al. (2024). Impact of multidisciplinary team management on survival and recurrence in stage I–III colorectal cancer: a population-based study in Northern Italy. Biology 13 (11), 928. doi:10.3390/biology13110928
Napolitano, S., Martini, G., Ciardiello, D., Del Tufo, S., Martinelli, E., Troiani, T., et al. (2024). Targeting the EGFR signalling pathway in metastatic colorectal cancer. Lancet Gastro. and Hepato. 9 (7), 664–676. doi:10.1016/S2468-1253(23)00479-X
Ni, G., Yang, G., He, Y., Li, X., Du, T., Xu, L., et al. (2020). Uniformly sized hollow microspheres loaded with polydopamine nanoparticles and doxorubicin for local chemo-photothermal combination therapy. Chem. Eng. J. 379, 122317. doi:10.1016/j.cej.2019.122317
Nikam, A., Sahoo, P. R., Musale, S., Pagar, R. R., Paiva-Santos, A. C., and Giram, P. S. (2023). A systematic overview of Eudragit® based copolymer for smart Healthcare. Pharmaceutics 15 (2), 587. doi:10.3390/pharmaceutics15020587
Niu, B., Liao, K., Zhou, Y., Wen, T., Quan, G., Pan, X., et al. (2021). Application of glutathione depletion in cancer therapy: enhanced ROS-based therapy, ferroptosis, and chemotherapy. Biomaterials 277, 121110. doi:10.1016/j.biomaterials.2021.121110
Nolano, A., Medugno, A., Trombetti, S., Liccardo, R., De Rosa, M., Izzo, P., et al. (2023). Hereditary colorectal cancer: state of the art in Lynch syndrome. Cancers 15 (1), 75. doi:10.3390/cancers15010075
Noreen, S., Pervaiz, F., Ijaz, M., Hanif, M. F., Hamza, J. R., Mahmood, H., et al. (2024). Ph-sensitive docetaxel-loaded chitosan/thiolated hyaluronic acid polymeric nanoparticles for colorectal cancer. Nanomedicine 19 (9), 755–777. doi:10.2217/nnm-2023-0318
Ojha, T., Pathak, V., Shi, Y., Hennink, W. E., Moonen, C. T. W., Storm, G., et al. (2017). Pharmacological and physical vessel modulation strategies to improve EPR-mediated drug targeting to tumors. Adv. Drug Deliv. Rev. 119, 44–60. doi:10.1016/j.addr.2017.07.007
Ou, K., Kang, Y., Chen, L., Zhang, X., Chen, X., Zheng, Y., et al. (2019). H2O2-responsive nano-prodrug for podophyllotoxin delivery. Biomaterials Sci. 7 (6), 2491–2498. doi:10.1039/c9bm00344d
Öztürk, K., Kaplan, M., and Çalış, S. (2024). Effects of nanoparticle size, shape, and zeta potential on drug delivery. Int. J. Pharm. 666, 124799. doi:10.1016/j.ijpharm.2024.124799
Parseghian, C. M., Napolitano, S., Loree, J. M., and Kopetz, S. (2019). Mechanisms of innate and acquired resistance to anti-EGFR therapy: a review of current knowledge with a focus on rechallenge therapies. Clin. Cancer Res. 25 (23), 6899–6908. doi:10.1158/1078-0432.CCR-19-0823
Pe’er, D., Ogawa, S., Elhanani, O., Keren, L., Oliver, T. G., and Wedge, D. (2021). Tumor heterogeneity. Cancer Cell. 39 (8), 1015–1017. doi:10.1016/j.ccell.2021.07.009
Phatak, N., Bhattacharya, S., Shah, D., Manthalkar, L., Sreelaya, P., and Jain, A. (2023). Cd44 targeted delivery of hyaluronic acid-coated polymeric nanoparticles against colorectal cancer. Nanomedicine 18 (23), 1613–1634. doi:10.2217/nnm-2023-0145
Rani, V., Deep, G., Singh, R. K., Palle, K., and Yadav, U. C. S. (2016). Oxidative stress and metabolic disorders: pathogenesis and therapeutic strategies. Life Sci. 148, 183–193. doi:10.1016/j.lfs.2016.02.002
Rao, G., Wang, H., Li, B., Huang, L., Xue, D., Wang, X., et al. (2013). Reciprocal interactions between tumor-associated macrophages and CD44-positive cancer cells via osteopontin/CD44 promote tumorigenicity in colorectal cancer. Clin. Cancer Res. 19 (4), 785–797. doi:10.1158/1078-0432.Ccr-12-2788
Ray, S. (2019). “25 - advanced colon-specific delivery systems for treating local disorders,” in Polysaccharide carriers for drug delivery. Editors S. Maiti, and S. Jana (Delhi, India: Woodhead Publishing), 737–762.
Rivera del Rio, A., Keppler, J. K., Boom, R. M., and Janssen, A. E. M. (2021). Protein acidification and hydrolysis by pepsin ensure efficient trypsin-catalyzed hydrolysis. Food and Funct. 12 (10), 4570–4581. doi:10.1039/D1FO00413A
Safari, J., and Zarnegar, Z. (2014). Advanced drug delivery systems: nanotechnology of health design: a review. J. Saudi Chem. Soc. 18 (2), 85–99. doi:10.1016/j.jscs.2012.12.009
Salyers, A. A., Palmer, J. K., and Wilkins, T. D. (1978). Degradation of polysaccharides by intestinal bacterial enzymes. Am. J. Clin. Nutr. 31 (10), S128–S130. doi:10.1093/ajcn/31.10.S128
Sandha, K. K., Kaur, S., Sharma, K., Ali, S. M., Ramajayan, P., Kumar, A., et al. (2025). Autophagy inhibition alleviates tumor desmoplasia and improves the efficacy of locally and systemically administered liposomal doxorubicin. J. Control. Release 378, 1030–1044. doi:10.1016/j.jconrel.2024.12.078
Seyfoori, A., Seyyed Ebrahimi, S. A., Samandari, M., Samiei, E., Stefanek, E., Garnis, C., et al. (2023). Microfluidic-assisted CTC isolation and in situ monitoring using smart magnetic microgels. Small 19 (16), 2205320. doi:10.1002/smll.202205320
Shahdadi Sardou, H., Akhgari, A., Afrasiabi Garekani, H., and Sadeghi, F. (2019). Screening of different polysaccharides in a composite film based on Eudragit RS for subsequent use as a coating for delivery of 5-ASA to colon. Int. J. Pharm. 568, 118527. doi:10.1016/j.ijpharm.2019.118527
Shao, H., Liu, M., Jiang, H., and Zhang, Y. (2025). Polysaccharide-based drug delivery targeted approach for colon cancer treatment: a comprehensive review. Int. J. Biol. Macromol. 302, 139177. doi:10.1016/j.ijbiomac.2024.139177
Shimomura, K., Hattori, N., Iida, N., Muranaka, Y., Sato, K., Shiraishi, Y., et al. (2023). Sleeping Beauty transposon mutagenesis identified genes and pathways involved in inflammation-associated colon tumor development. Nat. Commun. 14 (1), 6514. doi:10.1038/s41467-023-42228-z
Si, X., Ji, G., Ma, S., Xu, Y., Zhao, J., Zhang, Y., et al. (2021). In–situ-sprayed dual-functional immunotherapeutic gel for colorectal cancer postsurgical treatment. Adv. Healthc. Mater. 10 (20), 2100862. doi:10.1002/adhm.202100862
Sobhani, I., Rotkopf, H., and Khazaie, K. (2020). Bacteria-related changes in host DNA methylation and the risk for CRC. Gut Microbes 12 (1), 1800898. doi:10.1080/19490976.2020.1800898
Soe, Z. C., Poudel, B. K., Nguyen, H. T., Thapa, R. K., Ou, W., Gautam, M., et al. (2019). Folate-targeted nanostructured chitosan/chondroitin sulfate complex carriers for enhanced delivery of bortezomib to colorectal cancer cells. Asian J. Pharm. Sci. 14 (1), 40–51. doi:10.1016/j.ajps.2018.09.004
Song, H., Li, H., Shen, X., Liu, K., Feng, H., Cui, J., et al. (2024). A pH-responsive cetuximab-conjugated DMAKO-20 nano-delivery system for overcoming K-ras mutations and drug resistance in colorectal carcinoma. Acta Biomater. 177, 456–471. doi:10.1016/j.actbio.2024.01.047
Stroh, C., Reusch, C., Schmidt, J., Splittgerber, J., Wesolowski, J. S., and Blaukat, A. (2010). Pharmacological and immunological characteristics of the therapeutic anti-EGFR antibodies cetuximab, panitumumab, and nimotuzumab. J. Clin. Oncol. 28 (15), e13025. doi:10.1200/jco.2010.28.15_suppl.e13025
Su, Q., Wang, Z., Li, P., Wei, X., Xiao, J., and Duan, X. (2024). pH and ROS dual-responsive autocatalytic release system Potentiates immunotherapy of colorectal cancer. Adv. Healthc. Mater. 13 (29), e2401126. doi:10.1002/adhm.202401126
Subramanian, D. A., Langer, R., and Traverso, G. (2022). Mucus interaction to improve gastrointestinal retention and pharmacokinetics of orally administered nano-drug delivery systems. J. Nanobiotechnology 20 (1), 362. doi:10.1186/s12951-022-01539-x
Sun, L., Liu, H., Ye, Y., Lei, Y., Islam, R., Tan, S., et al. (2023). Smart nanoparticles for cancer therapy. Signal Transduct. Target. Ther. 8 (1), 418. doi:10.1038/s41392-023-01642-x
Sun, X., Tian, T., Lian, Y., and Cui, Z. (2024). Current advances in viral nanoparticles for biomedicine. ACS Nano 18 (50), 33827–33863. doi:10.1021/acsnano.4c13146
Szabo, C., Coletta, C., Chao, C., Módis, K., Szczesny, B., Papapetropoulos, A., et al. (2013). Tumor-derived hydrogen sulfide, produced by cystathionine-β-synthase, stimulates bioenergetics, cell proliferation, and angiogenesis in colon cancer. Proc. Natl. Acad. Sci. 110 (30), 12474–12479. doi:10.1073/pnas.1306241110
Szekalska, M., Czajkowska-Kośnik, A., Maciejewski, B., Misztalewska-Turkowicz, I., Wilczewska, A. Z., Bernatoniene, J., et al. (2023). Mucoadhesive alginate/pectin films crosslinked by calcium carbonate as carriers of a model antifungal drug—posaconazole. Pharmaceutics 15 (10), 2415. doi:10.3390/pharmaceutics15102415
Taipaleenmäki, E., Christensen, G., Brodszkij, E., Mouritzen, S. A., Gal, N., Madsen, S., et al. (2020). Mucopenetrating polymer – lipid hybrid nanovesicles as subunits in alginate beads as an oral formulation. J. Control. Release 322, 470–485. doi:10.1016/j.jconrel.2020.03.047
Tang, K., Xue, J., Zhu, Y., and Wu, C. (2024). Design and synthesis of bioinspired nanomaterials for biomedical application. WIREs Nanomed. Nanobiotech. 16 (1), e1914. doi:10.1002/wnan.1914
Trabolsi, A., Arumov, A., Yin, J., Halmos, B., Brodskiy, P., Oberley, M. J., et al. (2022). Pan-cancer association between increased iron utilization and poor prognosis highlights potential of transferrin receptor-targeting therapies in multiple tumor types. J. Clin. Oncol. 40 (16), 3120. doi:10.1200/JCO.2022.40.16_suppl.3120
Tramontano, C., Martins, J. P., De Stefano, L., Kemell, M., Correia, A., Terracciano, M., et al. (2022). Microfluidic-assisted production of gastro-resistant active-targeted diatomite nanoparticles for the local release of galunisertib in metastatic colorectal cancer cells. Adv. Healthc. Mater. 12 (6), e2202672. doi:10.1002/adhm.202202672
Turanlı, Y., and Acartürk, F. (2022). Preparation and characterization of colon-targeted pH/Time-dependent nanoparticles using anionic and cationic polymethacrylate polymers. Eur. J. Pharm. Sci. 171, 106122. doi:10.1016/j.ejps.2022.106122
Udaipuria, N., and Bhattacharya, S. (2025). Novel carbohydrate polymer-based systems for precise drug delivery in colon cancer: improving treatment effectiveness with intelligent biodegradable materials. Biopolymers 116 (1), e23632. doi:10.1002/bip.23632
Udenigwe, C. C., Abioye, R. O., Okagu, I. U., and Obeme-Nmom, J. I. (2021). Bioaccessibility of bioactive peptides: recent advances and perspectives. Curr. Opin. Food Sci. 39, 182–189. doi:10.1016/j.cofs.2021.03.005
van Erning, F. N., Creemers, G. J., De Hingh, I. H. J. T., Loosveld, O. J. L., Goey, S. H., and Lemmens, V. E. P. P. (2013). Reduced risk of distant recurrence after adjuvant chemotherapy in patients with stage III colon cancer aged 75 years or older. Ann. Oncol. 24 (11), 2839–2844. doi:10.1093/annonc/mdt334
Vieira, A., Martins, F., and Machado, A. S. (2024). Alexithymia, emotion regulation and autistic traits in Familial adenomatous polyposis. Eur. Psychiatry 67 (S1), S367–S368. doi:10.1192/j.eurpsy.2024.755
Wang, C.-Y., Sun, M., Fan, Z., and Du, J.-Z. (2022a). Intestine enzyme-responsive polysaccharide-based hydrogel to open epithelial tight junctions for oral delivery of imatinib against colon cancer. Chin. J. Polym. Sci. 40 (10), 1154–1164. doi:10.1007/s10118-022-2726-0
Wang, D., Wang, L., Zheng, L., Chen, J., Zhang, W., Zhou, W., et al. (2023a). Enhancing the management of metastatic tumors by robust Co-delivery of 5-fluorouracil/MicroRNA-10b inhibitor using EGFR-targeted nanovehicles. Adv. Healthc. Mater. 12 (15), e2202989. doi:10.1002/adhm.202202989
Wang, H., Cai, Y., Jin, M., Huang, C. Q., Ning, C., Niu, S., et al. (2023b). Identification of specific susceptibility loci for the early-onset colorectal cancer. Genome Med. 15 (1), 13. doi:10.1186/s13073-023-01163-w
Wang, K., Shen, R., Meng, T., Hu, F., and Yuan, H. (2022b). Nano-drug delivery systems based on different targeting mechanisms in the targeted therapy of colorectal cancer. Molecules 27 (9), 2981. doi:10.3390/molecules27092981
Wang, L., Xia, J., Fan, H., Hou, M., Wang, H., Wang, X., et al. (2021). A tumor microenvironment responsive nanosystem for chemodynamic/chemical synergistic theranostics of colorectal cancer. Theranostics 11 (18), 8909–8925. doi:10.7150/thno.61651
Wang, T., Wang, F., Sun, M., Tian, M., Mu, Y., Chen, X., et al. (2019a). Gastric environment-stable oral nanocarriers for in situ colorectal cancer therapy. Int. J. Biol. Macromol. 139, 1035–1045. doi:10.1016/j.ijbiomac.2019.08.088
Wang, Z.-H., Liu, J.-M., Li, C.-Y., Wang, D., Lv, H., Lv, S.-W., et al. (2019b). Bacterial biofilm bioinspired persistent luminescence nanoparticles with gut-oriented drug delivery for colorectal cancer imaging and chemotherapy. ACS Appl. Mater. and Interfaces 11 (40), 36409–36419. doi:10.1021/acsami.9b12853
Warburg, O. (1925). The metabolism of carcinoma cells. J. Cancer Res. 9 (1), 148–163. doi:10.1158/jcr.1925.148
Was, H., Borkowska, A., Bagues, A., Tu, L., Liu, J. Y. H., Lu, Z., et al. (2022). Mechanisms of chemotherapy-induced neurotoxicity. Front. Pharmacol. 13, 750507. doi:10.3389/fphar.2022.750507
Wathoni, N., Nguyen, A. N., Rusdin, A., Umar, A. K., Mohammed, A. F. A., Motoyama, K., et al. (2020). Enteric-coated strategies in colorectal cancer nanoparticle drug delivery system. Drug Des. Dev. Ther. 14, 4387–4405. doi:10.2147/DDDT.S273612
Wei, Y., Gu, X., Sun, Y., Meng, F., Storm, G., and Zhong, Z. (2020). Transferrin-binding peptide functionalized polymersomes mediate targeted doxorubicin delivery to colorectal cancer in vivo. J. Control. Release 319, 407–415. doi:10.1016/j.jconrel.2020.01.012
Woolston, A., Khan, K., Spain, G., Barber, L. J., Griffiths, B., Gonzalez-Exposito, R., et al. (2019). Genomic and transcriptomic determinants of therapy resistance and immune landscape evolution during anti-EGFR treatment in colorectal cancer. Cancer Cell. 36 (1), 35–50.e9. doi:10.1016/j.ccell.2019.05.013
Wu, W. S. (2006). The signaling mechanism of ROS in tumor progression. Cancer Metastasis Rev. 25 (4), 695–705. doi:10.1007/s10555-006-9037-8
Xiao, Y., and Yu, D. (2021). Tumor microenvironment as a therapeutic target in cancer. Pharmacol. and Ther. 221, 107753. doi:10.1016/j.pharmthera.2020.107753
Xiong, S., Qin, B., Liu, C., and Pan, Y. (2024a). Editorial: immunosuppression mechanisms and immunotherapy strategies in glioblastoma. Front. Cell. Neurosci. 18, 1411330. doi:10.3389/fncel.2024.1411330
Xiong, S., Shah, K., and Liu, C. (2024b). A zwitterionic twist. Nat. Rev. Chem. 8 (10), 721. doi:10.1038/s41570-024-00654-7
Xu, Y., Wu, H., Huang, J., Qian, W., Martinson, D. E., Ji, B., et al. (2020). Probing and enhancing ligand-mediated active targeting of tumors using sub-5 nm ultrafine iron oxide nanoparticles. Theranostics 10 (6), 2479–2494. doi:10.7150/thno.39560
Yang, C., Ming, H., Li, B., Liu, S., Chen, L., Zhang, T., et al. (2024a). A pH and glutathione-responsive carbon monoxide-driven nano-herb delivery system for enhanced immunotherapy in colorectal cancer. J. Control. Release 376, 659–677. doi:10.1016/j.jconrel.2024.10.043
Yang, C., Xue, Y., Duan, Y., Mao, C., and Wan, M. (2024b). Extracellular vesicles and their engineering strategies, delivery systems, and biomedical applications. J. Control. Release 365, 1089–1123. doi:10.1016/j.jconrel.2023.11.057
Yuan, J., Sun, W., Zhang, Z., Wang, Y., Huang, D., Ren, D., et al. (2025). 5-Fluorouracil/curcumin loaded silk fibroin hydrogel for the adjuvant therapy in colorectal cancer. Biomater. Adv. 168, 214108. doi:10.1016/j.bioadv.2024.214108
Yue, F., Zeng, X., Wang, Y., Fang, Y., Yue, M., Zhao, X., et al. (2024). Bifidobacterium longum SX-1326 ameliorates gastrointestinal toxicity after irinotecan chemotherapy via modulating the P53 signaling pathway and brain-gut axis. BMC Microbiol. 24 (1), 8. doi:10.1186/s12866-023-03152-w
Zaher, A., Petronek, M. S., Allen, B. G., and Mapuskar, K. A. (2024). Balanced duality: H2O2-based therapy in cancer and its protective effects on non-malignant tissues. Int. J. Mol. Sci. 25 (16), 8885. doi:10.3390/ijms25168885
Zamboulis, A., Nanaki, S., Michailidou, G., Koumentakou, I., Lazaridou, M., Ainali, N. M., et al. (2020). Chitosan and its derivatives for ocular delivery formulations: recent advances and developments. Polymers 12 (7), 1519. doi:10.3390/polym12071519
Zhang, D. D. (2010). The Nrf2-Keap1-ARE signaling pathway: the regulation and dual function of Nrf2 in cancer. Antioxidants and Redox Signal. 13 (11), 1623–1626. doi:10.1089/ars.2010.3301
Zhang, T., Li, J., Lu, J., Li, J., Zhang, H., Miao, Y., et al. (2025a). Enhanced tumor-targeting ability of transferrin-functionalized magnetic nanoparticles by in vivo AMF stimulation. Biomaterials 315, 122925. doi:10.1016/j.biomaterials.2024.122925
Zhang, X., Zhang, Y., Wang, P., Shi, F., Du, S., Zhang, Z., et al. (2025b). Infinite coordination polymer polydopamine nanocomposites dual-pathway Multistep induction of long-term hyperimmunity combined with photothermal-chemo synergistic therapy colorectal cancer. Aggregate e730. doi:10.1002/agt2.730
Zhang, Y., Ma, R., You, C., Leng, X., Wang, D., Deng, S., et al. (2023a). Hyaluronic acid modified oral drug delivery system with mucoadhesiveness and macrophage-targeting for colitis treatment. Carbohydr. Polym. 313, 120884. doi:10.1016/j.carbpol.2023.120884
Zhang, Y., Zhao, Z., Li, J., Wang, Q., Fan, Z., Yuan, Z., et al. (2023b). Treatment of colorectal cancer by anticancer and antibacterial effects of hemiprotonic phenanthroline-phenanthroline+ with nanomicelle delivery. Asian J. Pharm. Sci. 18 (3), 100801. doi:10.1016/j.ajps.2023.100801
Zhen, X., Jia, L., Tang, Q., Zhao, Y., Li, P., Li, J., et al. (2023b). Hybrid biointerface engineering nanoplatform for dual-targeted tumor hypoxia relief and enhanced photodynamic therapy. J. Colloid Interface Sci. 647, 211–223. doi:10.1016/j.jcis.2023.05.114
Zhen, X., Li, L., Jia, L., Zhu, A., Yang, Y., Wang, S., et al. (2023a). Biointerface engineering of self-protective bionic nanomissiles for targeted synergistic chemotherapy. Chin. Chem. Lett. 34 (4), 107680. doi:10.1016/j.cclet.2022.07.023
Zhen, X., Li, Y., Yuan, W., Zhang, T., Li, M., Huang, J., et al. (2024). Biointerface-engineered hybrid nanovesicles for targeted reprogramming of tumor microenvironment. Adv. Mater. 36 (41), e2401495. doi:10.1002/adma.202401495
Keywords: colorectal cancer, nano-drug delivery systems, stimuli-responsive nanocarriers, oral drug delivery systems, targeted delivery, tumor microenvironment
Citation: Chai M, Wang S, Chen Y, Pei X and Zhen X (2025) Targeted and intelligent nano-drug delivery systems for colorectal cancer treatment. Front. Bioeng. Biotechnol. 13:1582659. doi: 10.3389/fbioe.2025.1582659
Received: 24 February 2025; Accepted: 14 April 2025;
Published: 25 April 2025.
Edited by:
Merlis Alvarez-Berrios, Inter American University of Puerto Rico, Puerto RicoReviewed by:
Weimin Tang, Boston Children’s Hospital and Harvard Medical School, United StatesOpeoluwa Iwaloye, University of Florida, United States
Olamilekan Ibukun, Washington University in St. Louis, United States
Yibo Jia, Brigham and Women’s Hospital and Harvard Medical School, United States
Copyright © 2025 Chai, Wang, Chen, Pei and Zhen. This is an open-access article distributed under the terms of the Creative Commons Attribution License (CC BY). The use, distribution or reproduction in other forums is permitted, provided the original author(s) and the copyright owner(s) are credited and that the original publication in this journal is cited, in accordance with accepted academic practice. No use, distribution or reproduction is permitted which does not comply with these terms.
*Correspondence: Xueyan Zhen, eHpoZW5oYXJ2YXJkQGdtYWlsLmNvbQ==, eHpoZW5AYndoLmhhcnZhcmQuZWR1