- 1Center of Basic Research, Biomedical Research Foundation of the Academy of Athens, Athens, Greece
- 2Center of Clinical, Experimental Surgery & Translational Research, Biomedical Research Foundation of the Academy of Athens, Athens, Greece
Following an insult by both intrinsic and extrinsic pathways, complex cellular, and molecular interactions determine a successful recovery or inadequate repair of damaged tissue. The efficiency of this process is particularly important in the heart, an organ characterized by very limited regenerative and repair capacity in higher adult vertebrates. Cardiac insult is characteristically associated with fibrosis and heart failure, as a result of cardiomyocyte death, myocardial degeneration, and adverse remodeling. Recent evidence implies that resident non-cardiomyocytes, fibroblasts but also macrophages -pillars of the innate immunity- form part of the inflammatory response and decisively affect the repair process following a cardiac insult. Multiple studies in model organisms (mouse, zebrafish) of various developmental stages (adult and neonatal) combined with genetically engineered cell plasticity and differentiation intervention protocols -mainly targeting cardiac fibroblasts or progenitor cells-reveal particular roles of resident and recruited innate immune cells and their secretome in the coordination of cardiac repair. The interplay of innate immune cells with cardiac fibroblasts and cardiomyocytes is emerging as a crucial platform to help our understanding and, importantly, to allow the development of effective interventions sufficient to minimize cardiac damage and dysfunction after injury.
Tissue Repair and Regeneration: The Crucial Cardiac Inabilities, Consequences, and Exceptions
The harmonious homeostatic balance of cellular and molecular interactions in a given tissue may be dramatically disturbed upon insult. The tissue and organ response, coordinated by local cellular and molecular players and gradually by systemic reactions, will either lead to sealing of a wound, isolation, or extinction of an infectious or toxic agent, manipulation of a stress condition etc. However, the achievement of an efficient homeostatic repair requires the ability of the parenchyma to regenerate. In humans and mammals, liver, blood, skeletal muscle and intestinal epithelium have a good regenerative ability, whereas nervous system and heart fail to do so (1, 2). This is disastrous for the cardiac tissue, particularly following a major yet common injury event such as myocardial infarction (MI). Danger signals (DAMPs) released by dying cardiomyocytes, elicit inflammatory reactions and tissue remodeling to stabilize the damaged myocardium (3). Unfortunately, the myocardium has very weak regenerative capacity because the survived cardiomyocytes practically cannot proliferate and the heart contains very few cardiomyocyte progenitors (4, 5). As a result, the primary scar may get extended and adjacent and remote regions are affected with fibrosis, a major hallmark of adverse tissue remodeling events, which influence the entire organ, leading eventually to heart failure (HF), a currently incurable public health problem. Ischemic conditions are responsible for the major part of HF cases. However, similar pathways and sequence of events may characterize the inability of the heart to cope with the rest 16 known HF etiologies (6), including cardiomyopathies developed due to cardiomyocyte intrinsic defects or extrinsic pathologies and non-ischemic insults. Notably, mitochondrial defects comprise a hallmark of cardiomyocyte intrinsic abnormalities and are often linked to necrotic cell death, thus being an important target in HF (7, 8).
The mechanisms of cardiac pathophysiology and HF are conveniently studied in the mice with induced models of coronary ligation and aortic constriction, recapitulating, respectively, aspects of the initiation of ischemic and hypertensive cardiomyopathy and progression to HF. On the other hand, the availability of various genetic models of HF offers a plethora of additional perspectives, time points, and combinations of conditions for more proper investigation of cardiomyopathy and HF. Among them, the desmin-null mouse lacks one of the earliest expressing genes in cardiomyocytes and myocyte progenitors, which encodes the muscle-specific intermediate filament protein desmin [recently reviewed in (9, 10)]. Its importance for cardiac homeostasis has been clearly manifested by the development of mitochondrial defects, oxidative stress, myocardial remodeling, dilated cardiomyopathy, and HF in desmin-deficient mice (11–16). Actually, these mice combine features of multiple cardiomyopathies (12, 17, 18) and also serve as a model of cardiac injury with cardiomyocyte death, leading to a macrophage-driven inflammatory response, extended cardiac fibrosis and finally myocardial degeneration (17).
Both induced and genetic HF models readily manifest the disastrous effects of ineffective cardiomyocyte renewal and cardiac regeneration upon injury. As a hopeful exception to the above, the hearts of two widely used experimental animals, the zebrafish and the neonatal mouse (19–21), show a robust regenerative capacity and repair following an MI or other injuries. Studying the species-and developmental stage-specific differences that mechanistically support this success story may dictate novel routes of intervention in the failing human heart. In the present review, cardiomyocytes will be the main focus; however, cardiac fibroblasts and macrophages are increasingly recognized as major partners of cardiomyocytes and important regulators of cardiac homeostasis (22, 23). Therefore, understanding in detail the interactions of this “ménage a trois” may help us to decisively change their “rules of engagement” following myocardial damage. We enlighten here the most important points, focusing on mechanistic insights deduced from basic research and preclinical studies.
Cardiac Subpopulations and the Contribution of Innate Immunity
Cardiomyocytes and Their Progenitors
In the mouse embryo, the first differentiated myocardial cells appear in cardiac crescent at embryonic day E7.5. Two distinct cell lineages appearing by E8.5 segregate leading later to the formation of left and right ventricle, the atria and outflow tract. Myocardial sub-lineages have also been identified contributing to the arterial and venous poles [reviewed in (24)]. Adult mammalian myocardium consists of 30% cardiomyocytes (25) and ~1% cardiomyocyte progenitor or stem cells (CPCs, CSCs). Despite the lower estimations that occasionally appeared (26), cardiomyocytes occupy 90% of the adult heart's mass (27) and drive its principal function that is, to rhythmically contract and pumping blood to supply the body.
The majority of adult cardiomyocytes are quiescent, and although some studies suggest that they have a measurable capacity for turnover, this is very low (~1% per year) and insufficient to allow recovery to a functional myocardium, after a significant cellular loss (28–30). In the neonatal mouse, resident cardiomyocytes maintain a regenerative force and 1 day-old injured hearts can partially replace their parenchyma, an ability that will be soon lost, e.g., in animals older than 1 week (19). Recent studies showed that neonatal regeneration can also occur in pigs (31, 32). A similar situation in humans has been sporadically reported (33), conforming to a broader scar-less repair ability lost during development (34). This ability, however, is not limitless given that apical resection exceeding 15% of neonatal mouse tissue fails to regenerate (35). In addition, in other injuries such as MI it is debatable whether the neonatal mouse heart can successfully achieve scarless repair and regeneration (33, 36). Moreover, some groups reported absence of regeneration following resection (37). Recent studies in the mouse confirmed that there is, indeed, a time window allowing efficient postnatal cardiac regeneration but this is very short and the ability vanishes in 2 day-old animals (38). Similarly, neonatal swine hearts are able to regenerate following MI injury and this ability declines within 48 h (31, 32). Notably, fate mapping tools used in mice suggested that it is the resident cardiomyocyte populations and not CPCs or CSCs that give rise to the novel cells 3 weeks after an injury occurred at day 1 (19). Moreover, recent data based on the expression of a reliable marker for all cycling cells (ki67) and single cell transcriptomic analysis, revealed that a majority among cycling cells (about 10% of myocardial cells) in 1 day-old mice are cardiomyocytes and not CPCs or CSCs (39). The overall cycling activity drops to 0.05% in the adult murine heart and restarts reaching higher levels (about 1%) in an infarct after MI. However, in that case, the proliferating cells are mostly endothelial cells, fibroblasts, and macrophages, not cardiomyocytes. Importantly, ki67 lineage tracing followed up to 1.5 years in the same experimental settings failed to identify CPCs or CSCs among the sources of any cycling population, in contrast to a limited yet existing fraction of cycling cardiomyocytes (0.16% of cycling myocardial cells) emerged from pre-existing cardiomyocytes (39). Although previous studies suggested that during preadolescence a native cardiomyocyte number expansion occurs (40, 41), this is under debate (42, 43), and it is believed that the dramatic increase in mammalian cardiac size during this period is due to cardiomyocyte hypertrophy rather than proliferation (44).
An obvious question is whether there are intrinsic pathways that gradually block the ability of neonatal mammalian cardiomyocytes to proliferate. Which are the pivotal differences compared to cardiomyocytes of zebrafish, newts, and axolotls that retain this ability throughout lifespan? A particular cardiomyocyte characteristic that attracted attention is bi-nucleation, thought to be generated because of incomplete cytokinesis failing to capitalize persistent cardiomyocyte karyokinesis postnatally. In contrast to fetal heart that is comprised by mononuclear cardiomyocytes, the majority of the cardiomyocytes in the adult mouse are bi-nucleated (26) and the extent of bi-nucleation negatively correlates with cardiomyocyte's ability to proliferate and recover cardiac tissue following an injury (45). Accordingly, deletion of Tnni3k, a gene promoting bi-nucleation, enhanced adult mouse cardiomyocyte proliferation after injury (45). On the other hand, regenerating adult zebrafish hearts contain mononuclear diploid cardiomyocytes and when these were modified to achieve a degree of polyploidy either by Tnni3k overexpression (45) or ect2 mosaic expression (46), their regenerative capacity was compromised. However, cardiomyocyte bi-nucleation represents a minor status in human hearts (29), thus failing to explain the lack of regeneration in our species. Moreover, in pigs, bi-nucleated cardiomyocytes increase from 10% at birth to only 30% in adulthood (47), again not explaining the switch of the regenerating neonatal heart. A possibly relevant, but not well-studied yet parameter, may be polyploidy (48), which is readily observed in adult swine and human hearts and to a much lesser extent in rodents, whereas zebrafish hearts contain only diploid cardiomyocytes.
On the other hand, the inability of cardiomyocytes to reenter the cell cycle has been linked to premature telomere dysfunction (49), nuclear interactions of the Hippo and Wnt signaling pathways (50), as well as to contribution of additional pathways including those of Notch (51) and neuregulin-ErbB (52, 53), albeit administration of neuregulin appeared inefficient in some settings (54). Forced overexpression of single or combinations of cell cycle regulators (cyclins and cyclin-dependent regulators) in mice had impressive beneficial effects in MI (55) and pressure overload [thoracic aortic banding (TAC) model] (56). However, in a setting of volume overload (aortocaval shunt), cyclin D forced expression failed to confer improved survival, cardiac function, and remodeling features (56). Nevertheless, there are obvious limits and risks in human therapeutic approaches when cell cycle reinforcing agents are used.
Moreover, cardiac regeneration and proliferation of cardiomyocytes may be regulated by their metabolic and oxidative status and hypoxia (57–59), as well as genes involved in mitochondrial quality control (60). Importantly, extrinsic cues such as physical interactions with extracellular space and matrix (61, 62) and even the innervation of the cardiac tissue (63) are crucial determinants. As discussed above, the native cardiomyocyte turnover in adult mammals, including humans (28, 64) is not enough to sustain cardiac integrity during injury, such as an MI, where millions of cardiomyocytes may be lost. As a consequence, replacement of myocytes by a fibrotic, non-contractile scar tissue occurs that might be initially helpful, but eventually compromises cardiac function, ultimately leading to HF (65). Even in the absence of injury, changes in the stiffness of the extracellular matrix surrounding the cardiomyocytes that occur during the first days of life, may impede the ability of cardiomyocytes to proliferate and consequently the capacity of the cardiac tissue to repair following an insult (38). Accordingly, cardiac stromal cells and macrophages, pivotal cellular determinants of the myocardial extracellular milieu, and their interactions with cardiomyocytes have lately attracted much attention as potential targets of intervention to improve cardiac repair.
Cardiac Fibroblasts and Other Non-cardiomyocytes
Fibroblasts constitute a dynamic and versatile population of cells of mesenchymal origin that secrete collagen and other ECM components providing to neighboring cells a physical support to migrate, proliferate, differentiate, and properly function (23), thus being implicated in both regenerative processes and pathological conditions. Even though they have been commonly associated with disease, particularly through the development of fibrotic tissue, fibroblasts also produce mediators like growth factors, cytokines, and proteases and are involved not only in tissue homeostasis but also in repair and regeneration (23, 66, 67). Currently, there is no specific molecular signature able to accurately identify fibroblasts and since they exist in virtually any organ, they can express distinct phenotypic markers depending on their location (68). However, the combinatorial use of transgenic mouse lines expressing cell tracing markers under cardiac fibroblast specific promoters is a reliable manner of cardiac fibroblast tracking (69). Such markers include the ECM component Collagen1, the transcription factor Tcf21, the membrane receptor PDGFRα, as well as the matricellular periostin, the latter being expressed particularly by activated cardiac fibroblasts (70, 71).
In steady-state conditions, cardiac fibroblasts are responsible for maintaining proper ECM through a dynamic process of synthesis and remodeling, especially during the first week after birth. They contribute to modulation of physiological events, including the homogeneous distribution of mechanical stress and electrical insulation of the ventricles from the atria (19, 72, 73). On the other hand, by their biophysical interactions with cardiomyocytes through mechanical or electrical junctions, fibroblasts can facilitate electro-mechanical transduction important for the proper maintenance of the conduction system (74, 75). Upon insult, fibroblasts initiate production of ECM components for tissue reconstruction (76) and transmit signals to surrounding cardiomyocytes, but also to other stromal cells for initiation of the healing process (23, 77–79). However, alterations of fibroblast behavior in response to extrinsic factors, such as inflammatory cytokines, and persistent activation lead to deregulated matrix deposition and consequently to the formation of fibrotic tissue, underlining the pivotal role of fibroblasts in regulating the equilibrium between disease development and tissue repair and regeneration in the heart (70, 80–82) and other tissues (83–85).
Similarly to the stroma of other tissues (85, 86), myocardial fibroblasts are a heterogeneous population (87, 88). Most cardiac fibroblasts, including those of the annulus fibrosus and the leaflets of some valves, originate from the epicardium. Moreover, endocardium provides fibroblasts found in the atrioventricular junction of the septum, whereas a smaller population in the right atrium originates from neural crest. As highly specific markers and tools were lacking, estimations fluctuated during the last decades, but according to recent updates fibroblasts may account for <15% of the total myocardial cell population (25). This, however, changes both quantitatively and qualitatively upon injury.
In MI, fibroblast numbers increase during the proliferative phase. Fibroblast proliferation rates were previously reported to exceed that of endothelial cells following mouse MI injury (89). Within a newly formed infarct, proliferating fibroblasts peak 4–5 days after MI, whereas endothelial cells are dramatically reduced (69). Two to three weeks after MI the fibroblast proliferation rates return to basal levels, representing only 2% of proliferating myocardial cells. In other types of injury, expanding fibroblast populations occupy different locations, as for instance the arterial adventitia and perivascular areas in pressure overload and endocardial and septum areas after exposure to isoproterenol (simulating β-adrenergic cardiac stress). Nevertheless, they follow more or less similar expansion time courses (69).
Differentiation into myofibroblasts, a feature common to most fibroproliferative diseases (83), also occurs and is responsible for the production of high levels of collagen type I, and other cardiac ECM components. Cardiac myofibroblasts originate mostly from resident fibroblasts (87, 90) and may persist for months or longer, following MI or other injuries, whereas their ineffective removal has been correlated with the propagation of fibrosis and dysfunction in many organs including the heart (78, 83). However, following a cardiac insult, not all expanding fibroblasts are myofibroblasts (69, 87, 90). On the other hand, certain myofibroblast populations secrete MFG-E8 (milk fat globule epidermal growth factor 8) to remove dead cells from infarcted hearts, thus suppressing inflammation and restoring cardiac function (91). Accordingly, detrimental fibrosis per se (92), activated fibroblasts and associated molecules are being widely recognized as therapeutic targets in HF (81). In addition to the well-known TGF-β contribution to cardiac fibrosis (93), recent important advances include IL-11 that induces fibrosis acting downstream of TGF-β (94) and the small proline-rich protein 2b that induces proliferation of mouse and human cardiac fibroblasts (95). Additional specialized fibroblast populations have been identified in the infarcted heart that may sustain ECM changes and pathology (96), again by analogy to what happens in other tissues. Upon cardiac injury, fibroblasts may even adopt osteoblastic cell-like phenotypes, thus promoting detrimental cardiac muscle calcification (97). More recent transcriptomics data show that, at least in MI, cardiac fibroblasts sequentially assume different phenotypes which are important for cardiac repair (98). In particular, 1 day after injury fibroblasts become pro-inflammatory, they are highly proliferative and promote both fibrosis and angiogenesis 3 days later, whereas they inhibit angiogenesis after a week. Identification of molecular players regulating fibroblast polarization toward fibrotic or inflammatory phenotypes (99) is of obvious importance.
In recent years, an increasing amount of data has shown the great versatility of fibroblasts and the plasticity that characterizes them. A cardiogenic transcription profile and characteristics of cardiac mesenchymal stromal cells (MSCs), and pericytes has been revealed (100–103). Current engineering approaches try to take advantage of this plasticity and force their beneficial transformation into cardiomyocytes (see subsection on Engineering the fibroblast plasticity).
Apart from fibroblasts, in the complex cellular-extracellular milieu of the heart, additional non-myocytes forming part of the epicardial and endocardial layers and the vascular compartment interact with cardiomyocytes and fibroblasts and play important roles in cardiac repair and regeneration thus determining the final cardiac output in disease and HF. Due to space limitations however, we will only briefly describe selected regeneration-related aspects of vascular endothelial and smooth muscle cells, pericytes, epicardial and endocardial cells, and other less well-characterized populations [for specific reviews see (104–107)].
Recent estimations suggest that actually, endothelial cells comprise the most abundant myocardial population (25). Resident endothelial cells and not fibroblasts are the source of newly formed coronary vessels after injury (108), a process of great importance for regeneration (109, 110) and maintenance of heart function (104). Other vessel-associated cell types are important as well: Perivascular Gli1+ mesenchymal stem-like cells may differentiate into myofibroblasts upon aortic banding injury in the mouse, contributing to fibrosis (111) and pericytes that surround endothelial microvasculature are involved in perivascular inflammation and fibrosis promoting angiogenesis and regeneration (106). The outer epithelial layer (epicardium) serves as a major source of smooth muscle cells of coronary vasculature and cardiac fibroblasts in development and disease. Furthermore, it contributes to cardiac repair and regeneration by paracrinely inducing angiogenesis and cardiomyocyte division (112, 113) as demonstrated in zebrafish hearts (see respective section below). Endocardium, the inner cardiac endothelial layer, signals to the myocardium in development and endocardial Notch signaling activation may promote mammalian heart regeneration [reviewed in (114)]. Valve cells comprise distinct interstitial cell populations generated by endocardial cells through endothelial-to-mesenchymal transition (EMT), forming initially cardiac cushions at E9.5 and later the valves [reviewed in (24)]. Upon inflammatory signals, valve interstitial cells may respond in a manner similar to extra-cardiac fibroblast species leading to valve thickening and cardiac comorbidities, as in rheumatoid arthritis (115). Finally, telocytes comprise a fibroblast-like population with distinct morphological characteristics. Their position near potential cardiac stem cells and intense secretory identities may explain their anti-fibrotic and pro-regenerative effects which are used to improve heart pathology (116, 117).
Notably, many of the above cardiac populations share characteristics and markers with fibroblasts (118). Given the pronounced plasticity of the non-myocytes in cardiac development and disease, accurate definition of their molecular signature might be difficult to be achieved therefore many efforts focus on their beneficial manipulation.
Cardiac Macrophages and Other Innate Immune Cells
Macrophages comprise 5–10% of total myocardial cells and are the most abundant leukocyte species in the heart (25, 119). Their identification is based on “classical” surface (F4/80) or intracellular (CD68) molecules, as well as several additional markers (CD11b, CCR2, Ly6C, MHC-II, MerTK, CD64, CX3CR1 being the most important in the mouse) used in various combinations in flow cytometry gating strategies or immunohistochemical studies. Some of these markers show various levels of expression (high, low) among macrophage subtypes and may be partially shared with other cardiac cells and immunocytes, including monocytes and dendritic cells. Recently CCR2 (the receptor of the MCP1/CCL2 chemokine) has been used to identify macrophages of monocyte origin.
During embryogenesis, macrophages firstly arise before definitive hematopoiesis, representing in the mouse at least four different subpopulations (120). The ontogeny of cardiac subpopulations includes primitive yolk-sac derived, fetal monocyte-derived, and adult monocyte-derived macrophages (121, 122). Among them, the yolk sac-derived CCR2− macrophages populate the subepicardial space as early as E12.5 and are mostly located near the coronary vasculature, being also essential to its maturation (123). With the exception of the CCR2+ minor subpopulation, cardiac macrophages may follow monocyte-independent renewal in a homeostatic postnatal period. They maintain crucial functions, such as cardiac rhythm, by forming connexin 43-based gap junctions to electrically polarize cardiomyocytes in the cardiac conduction system (124). It was recently found that similar distinct subsets (CCR2−, CCR2+) constitute the macrophage compartment of the human heart (125), with CCR2− macrophage renewal to be exclusively based on local proliferation, whereas the CCR2− expressing species are monocyte-dependent. In cardiomyopathy patients, CCR2− macrophages seem to locate near the coronary vasculature, similarly to what has been reported for mice, whereas CCR2+ macrophages occupy fibrotic areas (125).
Macrophages, in general, are highly plastic cells, adopting different, tissue- and milieu-dependent activation status (126). Their division into classically (M1) and alternatively (M2) activated, even if over-simplistic in some cases (127), has been helpful to describe macrophage populations that orchestrate inflammation (M1) or healing (M2), respectively (128, 129). A number of characteristic cell surface, cytoplasmic or nuclear markers were identified mostly from in vitro studies during the last years, whose presence or expression was considered to reflect M1 activation status or M2 and its variations (M2a, M2b, M2c). Accordingly, in a healthy and adult murine heart, the characteristics of resident macrophages would conform better to that of a polarized, alternative activation (130). However, timely emerging subtypes following a cardiac injury may be tissue-and case-sensitive, largely failing to be categorized according to the M1/M2 polarization paradigm (131), whose accuracy in representing the in vivo state is debatable (127, 132, 133). Recent pooled-cell transcriptomic analysis in the mouse MI model revealed that cardiac macrophages show high plasticity and assume diverse activation states, ranging from proinflammatory (resembling M1) to proreparative (resembling M2) within a short time window (7 days) after an injury. However, such states do not conform to the M1 and M2-states, in accordance to what is shown by independent single-cell transcriptomic analyses (134, 135), including the absence of the characteristic differential expression of iconic M1 or M2 markers, such as nitric oxide synthase 2 (Nos2) and arginase 1 (Arg1), respectively (136). However, it should be noted that gene expression and molecular marker distribution changes induced in cultured macrophages are indeed biased toward a “classical” or “alternative” activation state as described by M1 and M2 [also suggested to be replaced by terms denoting the polarization stimulus, e.g., M(IL4) and M(IFN-γ) (132)]. Although these may not coincide to the in vivo states, some markers and cytokines are still used to describe polarization and the paradigm has not been fully abandoned in the literature, including the characterization of cardiac pathology [e.g., (136, 137)]. Interestingly, macrophages can also mimic other cardiac cell types. Thus, the reparatory macrophages enriching an infarct region 1 week post-MI appear to overexpress collagen and periostin, both being acknowledged as cardiac fibroblast markers (136), underlining the need for detailed expression analysis of these cell populations of high plasticity at various time points following an injury, including longer-term conditions. What is happening, for instance, several months after an MI, where remaining fibrosis may sustain the course of the tissue toward heart failure?
During inflammation, such as after MI, inflammatory chemokines and cytokines released by the activated resident cardiac cells, including macrophages, stimulate bone marrow, and splenic reservoirs of stem cells and progenitors to produce in the blood and recruit in the cardiac tissue neutrophils and monocytes. These cells, in addition to digesting dead cells and removing debris, also release additional chemokines, cytokines, and enzymes sustaining inflammation. Distinct macrophage sub-populations may also emerge at early time points following an injury, such as MI, as for instance the blood-borne IFNIC (interferon-inducible cell) macrophage subset in which the type I interferon signaling pathway is activated (134). Actually, such cells may mediate detrimental MI inflammation. In the mouse, they sense as DAMP the DNA released by dying cardiomyocytes and respond through activation of the transcription factor IRF3 and associated type I interferon and interferon-stimulated gene expression in order to propagate inflammation.
Monocytes, on the other hand, originated from bone marrow, as well as splenic reservoirs (138), appear rapidly as Ly6Chi (a surface marker) fraction and then, coinciding with neutrophil disappearance, a pro-repairing Ly6Clo fraction, populating the post-MI heart (139, 140). Recently, a detailed analysis of the murine cardiac macrophage population, and the monocyte contribution to it in steady state and post-MI, was performed. This analysis was based on single-cell RNA-seq and the presence of known (MHCII and CCR2) and novel (LYVE1, a type I membrane glycoprotein binding hyaluronan, and TIMD4, a phosphatidylserine receptor) macrophage markers. Among the four macrophage populations identified as clusters with distinct expression profiles in the unbiased transcriptomic analysis, those expressing TIMD4 appear to be maintained independently of monocytes, whereas the rest are partially or fully replenished by monocytes (135). Notably, a monocyte population was also identified. Early after an infarct (day 2), resident cardiac macrophage levels are considerably reduced but they subsequently (days 4–28) slowly expand by proliferation. Importantly, in addition to the steady-state species, up to seven new myeloid cell subtypes, including four macrophage populations, can be identified following MI. TIMD4 expression appears to be a marker able to discriminate resident from CCR2+ monocyte-derived cardiac macrophages. Selective depletion of resident macrophages leads to exaggerated adverse remodeling, particularly in the peri-infarct zone. These results complement independent single-cell RNA-seq based studies described above (134) and suggest that in contrast to recruited macrophages, resident cardiac macrophages may actually exert a protective role in MI (135), at least in the particular time frames examined so far. The complete functional characterization of the multiple macrophage subtypes populating the mouse heart under steady-state conditions and injury (134, 135) will reveal the relative importance of each one of these populations as regards intervention targets in cardiac repair.
It is of interest that, among the similar sub-populations corresponding to the resident (CCR2−MHChi) or recruited (CCR2+) species, also identifiable in human cardiomyopathy samples (135), the monocyte-dependent CCR2+ population shows proinflammatory features and its abundance is associated with persistent systolic dysfunction in patients undergoing LV-assisted device unloading (125). Moreover, the resident CCR2+ cardiac macrophages are responsible for the recruitment of monocytes in injured murine hearts, and, furthermore, they promote the differentiation of the recruited monocytes into inflammatory macrophage subsets. This occurs not only in MI but also following reperfusion injury or in a diphtheria toxin-based cardiomyocyte ablation setting (141). Another recent analysis identified three macrophage subpopulations dynamically expanding in somewhat distinct courses within the first 2 weeks after an MI (142). Among these populations, MHC-IIhi/Ly6Clo and MHC-IIlo/Ly6Clo expanding macrophages are of a proinflammatory phenotype (e.g., showing up-regulated expression of TNF family members), whereas Ly6Chi macrophages assume a pro-reparative task by overexpressing genes promoting neoangiogenesis. Thus, distinct macrophage subsets in the mammalian heart respond to injury and differentially regulate repair processes following cardiomyocyte death-associated insults, suggesting that specific populations should be targeted in a time-dependent manner to achieve beneficial results.
As another example demonstrating a multifunctional role of macrophages and innate immunity, restriction of monocyte recruitment following viral myocarditis by IRAK-4 activation may actually lead to disease exacerbation exactly because interferon production will be suppressed and consequently viral survival and associated damage will be augmented (143). Reversely, mice deficient in interferon regulatory factor 3 or type I interferon receptor are protected from fatal MI. In that case, a reduced production of type I interferon by the DAMP-activated cardiac macrophages limits detrimental inflammation (134).
More changes may occur in cardiac macrophages following specific types of insult; over time, less IL-6, TNF, and MMP9 are produced in favor of higher levels of TGF-β and VEGF. This profibrotic and proangiogenic polarization, however, may have different impacts, as VEGF and angiogenesis appear to support regeneration, whereas a profibrotic response will harm the tissue in a longer term, leading to HF. Accordingly, in chronic rather than acute inflammatory injuries—such as TAC or angiotensin 2/aldosterone-induced pressure overload models in the mouse, roughly corresponding to HF with preserved ejection fraction (HFpEF) in humans—cardiac macrophage numbers are increased and mediate diastolic dysfunction by the production of profibrotic IL-10 (144). In particular, an overcrowding MHC-IIhi subpopulation seems to produce less MMPs and more osteopontin, thus promoting fibrosis. Similarly, we have found that osteopontin produced by macrophage-rich infiltrates in the desmin-null HF model, promotes over time cardiac fibrosis and dysfunction, mediated by an osteopontin-dependent galectin-3 secretion by macrophages (17). The temporally biphasic manner of macrophage contribution in the cardiac remodeling and potentially in the regeneration programs was shown in the TAC model of pressure overload, where macrophages initially proliferate and support angiogenesis in a KLF4-dependent manner (145). On the other hand, blockade of recruitment of macrophages that are linked to detrimental effects during late phase-pressure overload hypertrophy sustains cardiac angiogenesis and protects the heart from dysfunction (145). Alternatively, the expansion of CCR2+ macrophages in the left ventricle during the early compensatory phase following pressure overload in the mouse due to local up-regulation of CCR2 ligands is responsible for the subsequent CD4+ and CD8+ T-cell expansion in the mediastinal lymph nodes and the heart (146). These T-cell subsets actually mediate the late transition from hypertrophy to heart failure in this model (147, 148). Thus, early macrophage recruitment/expansion events may also trigger antigen presentation and T cell expansion leading to fibrosis and systolic dysfunction.
Changes in macrophage abundance and status occur also during aging (119, 144), sustaining a fibrotic cardiac phenotype. Aging-associated fibrosis, however, has also been attributed to events involving other cardiac populations such as accumulation of activated CD4+ T-cells (119) or differentiation of resident mesenchymal stem cells into fibroblasts that aberrantly secrete inflammatory cytokines, such as IL-6 and MCP-1 (82).
Additional cells of innate immunity regulate both the status and action of macrophages, as well as cardiac response to repair. Neutrophils, despite their short stay (e.g., up to 7 days in MI), affect macrophage polarization and promote cardiac post-MI repair, protecting from fibrosis and dysfunction (149). They can also adopt themselves different polarization states, analogous to those exemplified by macrophages (150). Therefore, their presence should not be considered anymore as plain pro-inflammatory but may have an important impact on remodeling and presumably on myocardial regeneration programs. The importance of macrophages in this interplay was underlined by recent findings showing that following cardiac injury the CCR2− macrophages resident in the mouse heart promote the recruitment of neutrophils through TLR9/MyD88 signaling (151). Moreover, mast cells are also infiltrating the injured or stressed hearts and get activated to release enzyme and cytokines (for instance TNFα) important for adverse myocardial remodeling (152). Although a minor one, this innate immune cell population may both activate cardiac fibroblasts promoting detrimental fibrosis (153), and positively affect cardiomyocyte contractility following MI (154).
Another mononuclear phagocyte species, the antigen-presentation specialized dendritic cells (DCs), represents a minority among CD45+ cells in murine myocardium. Cardiac DCs are comprised by at least three subsets (135), among them two conventional DCs populations, cDC1, and cDC2 (155). In viral myocarditis, DAMPs secreted by dying infected cardiomyocytes lead to recruitment of phagocytes, such as monocytes and DCs. This allows for the generation of effector lymphocytes by viral antigen presentation of DCs populating the draining lymph nodes. CD103+ cDCs actually protect the murine heart from heart failure development following viral myocarditis, via cDC-mediated generation of antigen-specific CD8+ T-cells that promote viral clearance and resolution of cardiac inflammation (156). In steady state, cDC1s promote the development of regulatory T cell (Treg) (155), an immunosuppressive cell type of the adaptive immunity system, known to also restrict cardiac inflammation and MI fibrosis (157). During MI, DC infiltration and cDC2s activation induce the priming of autoreactive CD4+ T-cells specific to cardiac α-myosin that leads to the production of detrimental IFN-γ and IL-17 (155). Furthermore, it appears that depletion of cDCs from MI heart reduces IFN-γ and IL-1β expression and inflammatory infiltration of neutrophils and macrophages and improves cardiac fibrosis and function (158). Thus, despite earlier reports pointing out to a beneficial role of DCs in MI (159), a rather detrimental action of cDCs is currently described at least in the murine models of cardiac insult and repair examined so far. Further consequences of the adaptive immunity arm of DC activation in cardiac repair, however, are beyond the scope of the present review and the readers could refer to recent excellent reviews (22, 160).
Overall, macrophages and other innate immune cell populations undergo significant functional changes following cardiac injury and may exert both beneficial and detrimental actions in cardiac repair, in a case-specific and time-dependent manner. Accordingly, they have been the subject of intense research approaches aiming to precisely define their ontogeny, polarization, and contribution to cardiac remodeling and regeneration (22, 161–163).
The macrophages, in particular, being the major cardiac innate immune population, may exert detrimental excessive inflammation at early time points after an acute injury mediated by its monocyte-derived arm, whereas the resident macrophage subset mediates rather cardioprotective actions. Their subsequently emerging reparatory phenotypes further induce cardiac repair but can be also detrimental in the longer term by sustaining fibrosis, particularly under chronic stress conditions or aging (Figure 1).
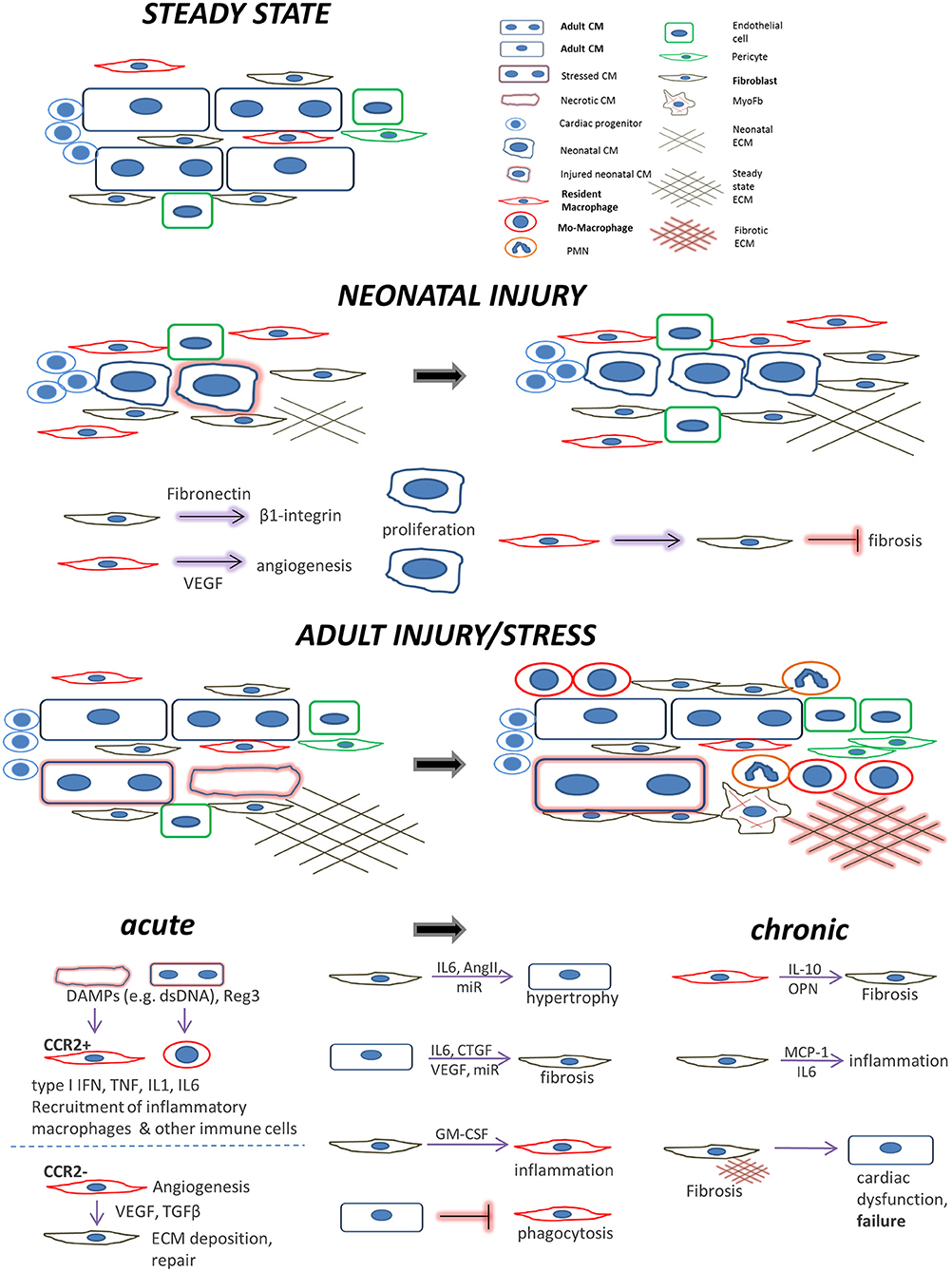
Figure 1. Main cell players during cardiac repair and regeneration: the interplay between cardiomyocytes, fibroblasts, and macrophages. Healthy adult steady state: Quiescent cardiomyocytes are in a homeostatic balance with interspersed cardiac fibroblasts, resident macrophages, and endothelial cells. Neonatal injury: cardiac regeneration happens only within a very short window postpartum. If injured, neonatal mammalian cardiomyocytes can proliferate in a permissive environment of appropriate stiffness. This is achieved with the help of (a) neonatal cardiac fibroblasts (which secrete factors to induce cardiomyocyte proliferation via integrin signaling) and (b), resident, yolk-sac derived, CCR2− macrophages which secrete proangiogenic factors or instruct fibroblasts to assume alternative, less fibrogenic states following an injury. Adult injury: almost no regeneration occurs in the adult myocardium. Under acute injury conditions (myocardial infarction, infection, intrinsic cardiomyocyte defects, etc.) dying cardiomyocytes release danger signals (DAMPs) leading to mobilization and recruitment of monocytes and generation of monocyte-derived macrophages. Additional cardiomyocyte derived mediators such as the Reg3 proteins contribute to inflammatory macrophage expansion. CCR2+ resident macrophages recruit more monocyte-derived inflammatory macrophages and secrete agents (e.g., type I IFN, IL-1, TNF) propagating inflammation, whereas they can also recruit detrimental T-cells (not shown). On the other hand, CCR2− resident macrophages and macrophage subsets emerging at later time points following injury, assume a pro-reparatory phenotype by expressing and releasing pro-fibrotic and proangiogenic factors, mainly through activation of fibroblasts and ECM deposition, to promote cardiac repair, and also by recruiting pro-reparative neutrophils (PMN). In addition, macrophage phagocytosis action promotes cardiac repair and is probably prevented by cardiomyocyte signals. Moreover, fibroblasts interact with cardiomyocytes in a paracrine manner to promote hypertrophy and fibrosis and instruct macrophages to mediate inflammation by secreting agents such as GM-CSF. Chronic stress and injury: macrophage activation in early time points affects the outcome. The inflammatory macrophage expansion in early pressure overload injury, for instance, leads to fibrotic ECM and heart failure in later time points triggering detrimental T-cell expansion (not shown). Under chronic activation conditions (pressure overload, cardiomyopathies, aging) macrophages secrete factors promoting fibrosis (e.g., IL-10, osteopontin) and fibrosis mediated by activated fibroblasts (including myofibroblasts) stimulates additional pathways affecting cardiomyocyte integrity and function. This propagates the detrimental effects of inefficient regeneration and repair, leading to heart failure. Fibroblasts can also mediate inflammation by releasing inflammatory cytokines such as MCP1 and IL6.
Particular characteristics of neonatal macrophages may decisively contribute to the maintenance of cardiomyocyte regenerative capacity in a mammalian neonatal heart. In the pro-regenerative environment of the heart during the first week of postnatal life the yolk sac-derived CCR2− macrophages expand by proliferation, whereas the newcomer CCR2− population of hematopoietic origin enters the regeneration-hostile heart by the 2nd week of life (120, 122). Deletion of CCR2− derived macrophages from the neonatal murine heart blocks its transient regenerative response to MI, leading to fibrotic scar and regenerating hearts of 1 day old mice are enriched in macrophages in contrast to the non-regenerating fibrotic hearts of infarcted 14 day-old mice (164). Further analysis revealed that although cardiomyocyte proliferation was not directly affected in the macrophage-depleted infarcted hearts, angiogenesis was severely compromised. In line with the higher expression of pro-angiogenic genes observed in macrophages from regenerating hearts, it was deduced from these data that neonatal cardiac macrophages support neoangiogenesis instead of fibrosis, thus promoting cardiac regeneration (164).
We will next summarize the contribution of macrophage interactions with cardiomyocytes and cardiac fibroblasts in the regulation of cardiac injury, regeneration, and repair (Figure 1), including attempts to beneficially manipulate these interactions to decrease the negative consequences of myocardial injury.
Cardiomyocyte-Cardiac Fibroblast-Macrophage Interactions
Cardiomyocytes and interspersed interstitial cells such as fibroblasts are adjacently situated in the heart and can interact via the release of paracrine factors, direct cell-cell interactions and indirectly through ECM (165). Cardiomyocytes and interstitial cells, including resident macrophages, possess specialized structures to interconnect and communicate (77, 124). Gap junctions (formed mostly by connexin 43 and 40) and adherens junctions mediate electrical and mechanical conduction and are important for the development of arrhythmias and fibrosis in a diseased heart. In addition, long thin membrane nanotubular structures have been observed between cardiomyocytes and fibroblasts in vitro and in vivo (166), and it was suggested that they might allow mitochondria exchange and calcium signal propagation. We will mainly focus on the interactions mediated in a paracrine manner among these three cell populations and their consequences for cardiac repair and regeneration (Figure 1).
Several in vitro approaches using co-cultures or exposure of one cardiac cell type to the secretome of the other have revealed effects of communication and interaction primarily between cardiomyocytes and fibroblasts. Some of their conclusions were supported by or translated to successful preclinical interventions.
Thus, in a paracrine fashion, fibroblasts may trigger cardiomyocyte hypertrophy via secreted angiotensin II or microRNA species, whereas cardiomyocytes can induce fibroblast proliferation by secreting IL-6 (167, 168). In contrast to adult fibroblasts that induce hypertrophy, embryonic fibroblast-derived signals promote cardiomyocyte DNA synthesis through β1-integrin signaling (169). Similarly, macrophages derived from neonatal -but not adult- mouse hearts secrete angiogenic factors such as VEGF and instruct cardiomyocytes to proliferate (164). Vice versa, in hypertrophic cardiomyopathy, mutations in sarcomeric proteins in cardiomyocytes can lead to increased profibrotic gene expression and proliferation of non-myocytes (170). This vicious cell interaction circle was recently confirmed by demonstrating that activation of p38α in adult cardiac fibroblasts, under β-adrenergic receptor stimulation, leads to IL-6 secretion that paracrinely causes cardiomyocyte hypertrophy (171). On the other hand, stimulation of protein kinase A in cardiomyocytes leads to CTGF and VEGF secretion that paracrinely induce collagen production and fibroblast proliferation leading to fibrosis (172).
Apart from hypertrophic signaling, cardiomyocytes may also secrete molecules, such as micro-RNAs to paracrinely stimulate collagen production via activation of the TGF-β1 pathway in co-cultured cardiac fibroblasts (173). However, the relative contribution of TGF-β1 pathway activation to fibrosis differs among the two cardiac populations. Whereas, cardiomyocyte-specific deletion of Smad3, a pivotal downstream effector in the TGF-β1 pathway, protects them from apoptosis in MI and restricts adverse remodeling, fibroblast-specific deletion leads to their hyperproliferation with a concomitant reduction of collagen synthesis leading to post-MI cardiac rupture (174).
Upon cardiac injury, factors such as GM-CSF secreted by activated fibroblasts can instruct resident macrophages to recruit inflammatory monocytes and neutrophils and propagate MI inflammation (175). Similarly, in a mouse autoimmune myocarditis model simulating inflammatory dilated cardiomyopathy, IL-17A, an inflammatory cytokine, induces GM-CSF production by cardiac fibroblasts, which in turn paracrinely instruct proinflammatory differentiation of monocytes in vitro and cardiac infiltration of Ly6Chi monocytes/macrophages (176). Interestingly, cardiac infiltrating Ly6Chi macrophages mediated pathology in this case by overexpressing both proinflammatory and profibrotic genes. On the other hand, the activation status of macrophages appears to play a role in cardiac fibroblast behavior. Accordingly, infusion of anti-inflammatory IL-10 into infarcted hearts conferred alternative type polarization to cardiac macrophages, which in turn stimulated fibroblast proliferation and collagen production, thus protecting against MI damage in the short term (177). Moreover, MI in mice lacking TRIB1 -a member of the Ca+2/calmodulin-dependent protein kinase (CAMK) of the Ser/Thr protein kinase family- that show selective depletion of alternatively activated macrophages led to the suppression of fibroblast activation, reduced collagen deposition and fatal cardiac rupture. These events were reversed by IL-4 or re-administration of alternatively activated macrophages themselves, which seem to beneficially activate fibroblasts in this setting by secreting osteopontin and IL-1α (178). However, as we noted above, macrophage expansion may be detrimental in other time points and types of injury as occurs for instance under conditions of pressure overload or in the desmin-null mouse. Another important aspect of macrophage-fibroblast interaction in the heart comes from studying cardiac regeneration in another model than zebrafish, the urodele amphibian salamander. In this regenerating cardiac milieu, macrophage depletion results in a permanent and highly cross-linked fibrotic scar and cease of regeneration (179). This, however, is not directly owing to affected cardiomyocyte proliferation and is rather a result of macrophage-mediated altered fibroblast proliferation counteracting efficient cardiomyocyte proliferation per se.
Cardiomyocyte-macrophage interactions are also important for cardiac repair [recently reviewed in (180)]. As already described, cardiomyocytes releasing DAMPs under stress or injury recruit inflammatory monocytes and monocyte-derived macrophages (134). Inhibition of the responsive pathways by deletion of pattern recognition receptors (PRR) in mice (e.g., the cyclic GMP-AMP synthase—a stimulator of interferon gene pathway) results in polarization of macrophages toward a reparative phenotype and protects against MI adverse remodeling and rupture (181).
The release of DAMPs is a characteristic of necrotic cell death and pyroptosis (182). In that case, activation of RIPK1 and RIPK3 protein kinases and necrosome formation (183) or inflammasome formation and caspase-1 activation (184) lead to loss of plasma membrane integrity and cell lysis, releasing DAMPs that mediate inflammation upon tissue injury, including cardiac injury (3, 185, 186). In contrast, apoptotic cell death, mediated either by caspase 3, 6, or 7 activation via the cell death receptor (extrinsic) or mitochondrial (intrinsic) pathways, maintains membrane integrity but leads to the release of “eat me” signals to trigger phagocytosis of apoptotic cells (182). These events (called efferocytosis) actually activate the generation of anti-inflammatory signals, such as the production of TGF-β and IL-10, by the phagocytosing macrophages (187), to terminate inflammation. Macrophage efferocytosis of apoptotic cardiomyocytes is regulated by both myeloid-epithelial-reproductive tyrosine kinase (MerTK) and MFG-E8 and when compromised, the acute inflammation following for instance an MI fails to resolve, leading to enhanced adverse remodeling (188, 189). Moreover, resident reparatory CCR2− macrophages express high levels of MerTK and release TGF-β upon cardiomyocyte engulfment (190). On the other hand, dying cardiomyocytes in MI may hinder their phagocytic removal by inducing shedding of macrophage MerTK (191). Both apoptosis and necrosis characterize cardiac cell death in MI and heart failure (192, 193), differentially contributing to pathophysiology. These forms of cell death may prevail at different time points (194) and cell populations (195, 196), with apoptosis being mostly responsible for non-myocyte death, including macrophages themselves. Nonetheless, cardiomyocyte apoptosis, even if limited, is considered important for the long term development of heart failure pathophysiology, given the inability of the cardiomyocyte population to regenerate.
Apart from such interactions, cardiomyocytes can also more directly instruct macrophage polarization and activities. Thus, extracellular vesicles isolated from mouse neonatal cardiomyocytes, when applied on macrophages induce p38MAPK activation and inflammatory gene expression (197). Moreover, early after MI, cardiomyocytes transiently overexpress and release several Reg3 proteins, members of the C-type lectin family (142). Local administration of Reg3 protein paralogues in mice promoted recruitment of pro-inflammatory macrophage subsets (MHC-IIhi/Ly6Clo and MHC-IIlo/Ly6Clo), whereas the pro-angiogenic and reparative Ly6Chi subpopulation was suppressed. Moreover, under ischemic conditions, cardiomyocytes seem to induce via secreted vesicles the adhesion of macrophages to ECM and lead to the reduction of their ability to phagocytose, suggesting a paracrine cardiomyocyte-macrophage crosstalk (197) that impairs debris clearance in MI. The debridement of damaged cardiac tissue is an important parameter, as its impairment by depletion of macrophages following cryo-injury in the mouse leads to diminished myofibroblast infiltration and vascularization and a thinner free wall accompanied by increased dilatation and mortality rate (198).
Most of our current knowledge on paracrine cardiac cell interactions stems from conventional two dimensional (2D) cell culture experimentation, mainly analyzing the effects caused by co-culturing two distinct isolated cardiac populations or exposure of one cell type to the secretome of another. Although informative, such approaches ignore important parameters such as specific topological arrangements in a healthy or diseased heart. Thus, in a fibrotic heart, fibroblasts may form aggregates at certain time points. Mimicking this condition in vitro, by spherical rearrangement of cardiac fibroblasts from a 2D monolayer to a 3D cluster, revealed that fibroblast expression profiles and secretory activity better conform to those prevailing in a remodeling heart (199). These 3D culture signatures are characterized by reduced proliferation, ECM and contractile protein synthesis and increased ECM degradation activity (with a pronounced up-regulation of MMP-11), chemotaxis and inflammation. Importantly, conditioned media from the 3D fibroblast cultures induce a stronger hypertrophic response of neonatal cardiomyocytes than the 2D counterparts (199). Moreover, 3D spheroids of mixed populations (e.g., cardiomyocytes and fibroblasts) with the use of directed fusion allow the build-up of heterocellular microtissues recapitulating the spatial distribution of cardiac cells and provide new tools to study parameters of arrhythmias and fibrosis (200). Going a step forward, scaffold-based 3D cultures include ECM components and may be game changers in understanding the real in vivo relations and effects under altered stiffness conditions, as those occurring for instance in a fibrotic MI scar. These so-called cardiovascular organs-on-a-chip approaches (201, 202), also serve as tools to understand the molecular basis of cardiac diseases and test drug efficiency, approaching the precision medicine level. Moreover, heart slices may represent a faithful tissue replica (77, 165).
Many of the known effects of cardiomyocytes on cardiac fibroblasts and vice versa should be re-examined using similar novel approaches to achieve evaluations a step closer to the in vivo state. Importantly, the introduction of resident macrophages in these systems will greatly precipitate our understanding of cardiac repair and regeneration mechanisms.
Exploitation of Cardiac and Modified Extracardiac Cells and Their Interactions to Orchestrate Cardiac Repair
The pronounced inability of the adult human heart to regenerate motivated numerous interventional approaches, mostly based on previous mechanistic studies in mouse and zebrafish models (4, 105, 118). These efforts targeted the cardiomyocytes themselves, their potential progenitors, extracardiac stem cells, cardiac fibroblasts, and immune components. Despite acknowledged limits and some disappointments (203), a vast amount of knowledge was gained pointing out immune response manipulation as well as engineering of fibroblasts, stem cells and their tissue environment as the most promising therapeutic targets, some already being the subject of ongoing clinical trials (204). In the following four subsections, we briefly summarize the main goals and achievements of such approaches, particularly focusing on the importance of the interactions among the main cell players and their exploitation to orchestrate cardiac repair.
Lessons From the Zebrafish Models
Zebrafish is a unique vertebrate animal model that combines the ability to regenerate its organs throughout its life, as well as allowing non-invasive in vivo imaging and genetic manipulations (205). Tail fin amputation (206), central neural system injury (207), retina (208) and heart injury models have been developed to explore the remarkable regenerative potential of zebrafish. In particular, concerning the heart, several injury models have been established: resection (20), cryoinjury (21, 209, 210), and chemogenetic ablation (211, 212). The latter refers to the use of metronidazole/nitroreductase combination or the tamoxifen-inducible tissue-specific expression of Diphtheria toxin A. Both approaches allow inducible tissue-specific damage and offer the possibility to study regeneration in different organs and developmental stages that are not surgically amenable. This approach allowed following in vivo the regeneration of pancreatic beta cells (211, 213), cardiomyocytes, hepatocytes, macrophages, podocytes, retinal neurons subtypes, etc. [reviewed in (214)]. These studies revealed that the reactivation of developmental pathways seems to be a recurrent theme during regeneration, as well as that an early stage acute inflammatory phase is beneficial for the regenerative response.
Inflammation is required and sufficient for enhancing the proliferation of both neural progenitors (207) and zebrafish cardiomyocytes (215). Medaka (Oryzia latipes) failed to activate a robust inflammatory response and recruit enough macrophages, upon cardiac cryoinjury, and subsequently showed impaired regeneration potential (216). In this context, activating Toll-like receptor signaling enhanced the immune response and neutrophil clearance, promoting regeneration also in medaka. An inflammatory response leads to fibrosis, but zebrafish provides a paradigm where fibrosis regresses, implying that fibrosis, and regeneration after cardiac injury are not mutually exclusive. Zebrafish resected hearts form a fibrin clot, or myocardial cells undergo necrosis after application of a liquid nitrogen-cooled probe, and collagen scars or fibrin deposits are primarily formed. However, and in contrast to mammals, they are subsequently replaced by new cardiomyocytes within 60–90 days (20). Upon cryoinjury and cardiomyocyte damage, leukocytes are attracted to and populate the damaged area. ECM proteins accumulate at the injury site, and a transient fibrotic tissue is formed. However, in contrast to the situation in mammals, fibrosis regresses and eventually is resolved. Fibroblasts remain at the damaged area but are inactivated, shutting down their profibrotic program (217). Identifying, therefore, the signaling pathways that drive fibroblast inactivation in zebrafish to enhance the regenerative potential of mammals is an intriguing hypothesis.
Three major regeneration events occur in the zebrafish heart following damage. The epicardium is activated by re-expressing embryonic markers and contributes to new vascularization of the regenerating myocardium. Cardiomyocytes start to proliferate to replace lost myocardium. The transient fibrotic tissue regresses. The signaling molecules guiding this regenerative response in fish remain largely unknown. In vivo high-throughput chemical screens of a fluorescent cell-cycle indicator transgenic line already identified several molecules that could induce cardiomyocyte proliferation at the early embryonic stages. These molecules have been shown to also facilitate heart regeneration in adult zebrafish (218). The endocardium and epicardium are activated to produce retinoic acid that supports cardiomyocyte proliferation as a paracrine factor (219). The epicardium is a key tissue for regeneration that secretes several essential factors for the proliferation and survival of cardiomyocytes. Additional signaling pathways identified to be important for heart regeneration are the Hedgehog (220), Notch (221), Sdf1a (222), Pdgf (223), and Vegfaa (224). However, Vegfaa exhibited a dual role in the context of cardiac injury enabling ectopic cardiomyogenesis but inhibiting regeneration at the site of the injury. Recently published studies of the Mexican cavefishes that fail to regenerate their hearts when compared to their surface fish relatives unraveled several quantitative trait loci that are linked to this ability. The leucine-rich repeat containing 10 gene (lrrc10) was already verified as necessary for proper heart regeneration whereas several more candidates may prove to be pivotal (225).
Preconditioning zebrafish hearts by several means, including remote stimulation by peritoneal sterile inflammation, also induces cardiomyocyte re-entry into the cell-cycle and boosts their regeneration potential (226). Inflammation is thought of as a double-edged sword having both detrimental and beneficial consequences. It is becoming therefore evident that instructive factors for heart regeneration, as well as inflammation, require tight spatiotemporal control for efficacy. A major challenge becomes to regulate this spatiotemporal response and identify the beneficial signals to promote regeneration while maintaining the ability to resolve fibrosis.
Using Cardiac Progenitors and Induced Progenitor Cells
Endogenous cardiac progenitor cell (CPC) populations that reside at small clusters in niches within the postnatal myocardium raised new possibilities for cardiac regeneration. Despite recent evidence suggesting that most probably there is no true stem cell in the human and experimental rodent heart and that any newly formed cardiomyocytes in steady state or after injury are actually the descendants of pre-existing mature cardiomyocytes (39, 227), mobilization or administration of native or ex vivo modified CPCs or their products has been proven beneficial in preclinical protocols and deserve further exploitation. A variety of resident CPCs have been identified in the adult mammalian heart according to their expression of the cell surface markers c-kit (228) and Sca-1(229), the transcription factor Islet-1 (230) or according to their ability to form Cardiospheres (CS) in vitro (231). All the above were shown to have proliferative properties and differentiation capacity toward cardiomyocytes, endothelial and smooth muscle cells. A novel population expressing the transcription factor twist-2 was recently demonstrated to contribute to cardiomyocytes, endothelial cells and fibroblasts in the adult murine heart as well (232). Resident cardiac Side Population (CSP) cells that are defined by their ability to efflux the dye Hoechst33342 from the cytoplasm (233, 234) are another source of progenitor cells for heart repair, as they can differentiate toward all cardiac lineages (235–238). Both CSP and CS-derived cells represent more heterogeneous populations (231, 239), expressing mostly the markers Sca-1 and c-kit, respectively, among others, while Islet-1+ cells represent remnants of an embryonic progenitor population that contributes to the formation of the second heart field during development (240). A significant contribution to cardiac regeneration may also arise from epicardium, as priming with thymosin β4 before injury resulted in re-expression of the key embryonic marker Wt1 in epicardial progenitor cells, causing them to invade the myocardium and to differentiate into cardiomyocytes (241). Notably, c-kit+ (242) and CS-derived cells (243) have undergone clinical trials to treat ischemic myocardium. Despite the reduction of the infarct size, the overall improvement of cardiac function was moderate with minimal engraftment of the cells within the myocardium, thus entering their cardiomyogenic potential under debate (244–246). Indeed, recent genetic lineage tracing approaches for the c-kit and Sca-1 markers showed that these CPCs do not contribute significantly to new cardiomyocyte formation during development, with aging or after acute injury, in contrast to what was believed before, but rather differentiate to vascular lineages (244–247).
Although c-kit+ and Sca-1+ CPCs are not considered anymore as a native source of cardiomyocyte producing progenitors, nonetheless they augmented the function of injured heart upon transplantation, which was also reported for CSP and CS-derived cells in animal models, likely through a paracrine manner (248–252). The latter implies that the regenerative potential of the heart is not only determined by the characteristics of CPC but is also influenced by the various cellular interactions and the microenvironment. Indeed, a great body of studies indicate that the constitution of the ECM, secreted molecules such as pro-inflammatory cytokines and chemokines, mechanical forces, the cellular interactions of CPCs with the stromal cells or the immune components of the myocardium, as well as the disease conditions that possibly predominate the cardiac tissue, may impact the proliferation, aging and differentiation capacity of CPCs and, consequently, positively or negatively regulate heart regeneration (61, 249, 253–258) [also reviewed by (259)].
Interestingly, immune cells seem to play a profound role in muscle regeneration either by regulating cardiac remodeling or/and cardiomyocyte proliferation (164, 260). Moreover, an intact immune response is essential to achieve any improvement observed in cardiac function when injecting bone marrow derived progenitor cells into infarcted myocardium (261). Furthermore, bone marrow-derived MSCs achieve better improvement in cardiac function, fibrosis, and angiogenesis when administered in combination with co-cultured macrophages in rat MI, the improvements being associated with elevated anti-inflammatory macrophages and gene expression (262). Finally, the inflammatory response per se elicited during and owing to a stem cell-based therapy may be of paramount importance for the beneficial effects of the latter in a diseased heart (263). Indeed, the amelioration of cardiac dysfunction observed when c-kit+ CPCs or fractionated bone marrow mononuclear cells are injected in mouse hearts undergoing ischemia/reperfusion is not owing to newly generated cardiomyocytes, but rather to the associated inflammatory response that leads to improved mechanical properties of the infarcted area (264). In particular, direct injection of these cells in the heart triggers a local accumulation of CCR2+ and CX3CR1+ macrophages that alter fibroblast activity and ECM content in the border zone of the infarct improving cardiac output. Notably, similar improvements can be achieved by the injection of non-cellular activators of innate immune response such as zymosan (264), further underlining the pivotal role of inflammation in cardiac fate after an injury.
Serving as an important alternative to CPCs, the induced Pluripotent Stem Cells (iPSCs) are extracardiac stem cells that originate from the reprogramming of somatic cells via the ectopic expression of the transcription factors Oct3/4, Sox2, Klf4, and c-Myc and have wide differentiation ability, giving rise to all cell types of the three germ layers (265). Their cardiovascular differentiation capacity has been studied in vitro and in vivo with beneficial results regarding cardiac function after MI in mice (266–269). The use of human iPSCs for myocardial regeneration circumvents the low available number of resident CPCs, as well as suboptimal cell engraftment upon transplantation, two additional hurdles with CPC therapy. However, the disadvantage of teratoma formation by iPSC still persists (270, 271). iPSC-derived cardiomyocytes (iPSC-CMs), representing a source of differentiated/mature cells, overcome this limitation and their use to heal the diseased myocardium of small and primate animal models showed improvement of cardiac function after transplantation (272–274). Moreover, human iPSC-CMs can serve as an alternative to animals for modeling human diseases, drug testing, and development of personalized therapies, since animal models often do not accurately reproduce human pathophysiology. Over the past years, more than 70 human diseases and cardiomyopathies have been modeled using iPSC-derived cells, including cardiac sodium channel diseases, the long QT syndrome, a desmin-related dilated cardiomyopathy, Barth syndrome and Duchenne muscular dystrophy (201, 275–277).
Future cardiac regeneration efforts are focused on taking advantage of gaining knowledge from trial and error so far, in combination with technological advances. Toward this direction, a lot of work is done on increasing CPC and iPSC-CM engraftment and survival after transplantation to the heart using scaffold-based approaches, such as hydrogels or biomaterial cardiac patches on which cells are seeded and expanded in vitro prior to transplantation, or scaffold-free cell sheets (278–281). Importantly, these approaches take into account the spatial interactions among cardiac cells and their surrounding matrix that are of most importance in the complex myocardial tissue, as also repeatedly underlined in the previous sections. Introduction of CPCs and iPSC-CMs after injury via these methods resulted in increased cell survival, long term retention to the host myocardium and increased cardiac function (278, 279). The advantage of a patch-based approach over intra-myocardial cell injection for cardiac repair may rely on simultaneously acting as a substrate that strengthens the injured myocardium and prevents adverse remodeling, and as a template for cells to survive and proliferate. In addition, the use of stem cell-derived exosomes, as a cell-free alternative for stem cell-based regenerative approach, induced heart regeneration and augmented cardiac function when administrated after injury (281, 282). Their beneficial effect relies on their composition of soluble factors and macromolecules, mostly specific microRNAs that are transferred by extracellular vesicles to cardiomyocytes (282–284).
It is important to note that the integration of cardiac cell-cell and cell-ECM interactions in all these approaches is crucial. Accordingly, the particular cell and ECM composition and interactions in the pro-regenerative milieu of rodent neonatal hearts decisively promote the maturation of mouse stem cell-CM and human iPC-CM preparations toward adult cardiomyocytes (285). Moreover, mouse embryonic stem cell-derived CMs cultured in microtissues and exposed to conditioned medium from fibroblasts obtained from pro-regenerative neonatal hearts, assumed better spreading and contractile activity compared to those exposed to medium from adult fibroblasts (286). Thus, cardiac cell interactions from a pro-regenerative environment can be exploited to induce maturation of engineered cardiomyocytes, expected to allow better regeneration properties of future intervention protocols. Additional aspects regarding the particular contribution of innate immune cells are discussed under the Manipulating the Immune Response section underneath.
Engineering the Fibroblast Plasticity: Transdifferentiation to Cardiomyocytes
Fibroblasts have been extensively used in order to uncover new potential applications in regenerative medicine. Despite their increased use as a source of iPSCs the immunological side effects, the oncogenic potential of the remaining undifferentiated cells and the low engraftment of transplanted cells, potential hurdles of iPSC technology application, urgently called for an alternative approach.
These challenges can be bypassed by the direct reprogramming of differentiated somatic cells into a different, distinct somatic fate without passing first through a pluripotent state. Indeed, an alternative to iPSC protocol using the ectopic expression of a combination of three genes encoding the cardiac transcription factors, Gata4, Mef2c, and Tbx5 (GMT), successfully induced reprogramming of murine fibroblasts into cardiomyocyte-like (iCM) cells in vitro (287). This promising achievement proved later to be also transferrable in vivo [reviewed in (4)], thus constituting an alternative route to support cardiac regeneration using an abundant cardiac cell population.
iCMs express major cardiac genes and exhibit cardiomyocyte characteristics, such as sarcomere structure, spontaneous intracellular calcium oscillations, and beating contractions (287). Other studies showed that the addition of other transcription factors, such as HAND2 (GHMT cocktail) (288), transcription repressor molecules (ZNF281) (289), miRNAs, and growth factors can enhance the reprogramming efficiency (290–294). Suppression of certain genes encoding factors such as the Polycomb complex protein BMI1 and the splicing factor polypyrimidine tract-binding protein 1 (PTB) (88, 295) or manipulation of major signaling pathways such as those of Wnt, Notch, TGF-β, and the serine/threonine-protein kinase, protein Kinase B (AKT1) pathway also enhanced cardiac reprogramming (296–299). In addition to direct reprogramming, there has been partial reprogramming of fibroblasts into CPCs by using a cocktail of five genes encoding early cardiac factors -Mesp1, Gata4, Tbx5, Nkx2-5, and Baf60c (300). These progenitors are an expandable multipotent -and not a pluripotent- cell population, aiming to increase in numbers the iCMs, and be able to replace the lost cardiomyocytes after insult (300). In both cases of direct and partial cardiac reprogramming, transplantation of reprogrammed fibroblasts into immunodeficient murine hearts after MI, resulted into (i) differentiated cardiomyocytes, in actually higher numbers than in previous in vitro studies, suggesting also a paracrine favorable factor, (ii) endothelial cells, and (iii) smooth muscle cells, as well as improved survival (287, 300). The next step in in vitro direct reprogramming is the use of human fibroblasts to generate functional iCMs. Whereas this was proven to be more challenging, it was achieved with the addition to the original GMT or GHMT viral cocktail of factors such as myocardin (MYOCD), MESP1, estrogen-related receptor-γ (ESRRγ), and zinc-finger protein ZFPM2, or miR-1 and/or miR-133, that resulted in human iCMs with gene expression profile, sarcomere structure, and spontaneous calcium transients reminiscent of cardiomyocytes (301–303). However, the use of viral vectors to ectopically express genes of interest finds a lot of skepticism. To eliminate this drawback, a virus-free method using a combination of nine chemicals was used to transdifferentiate human fibroblasts to cardiomyocyte-like cells that expressed cardiac genes and had a well-organized sarcomere structure. Even though it is not clear if the chemically reprogrammed cardiomyocyte-like cells underwent through a progenitor state, when they were transplanted into a murine heart after MI, they obtained a cardiomyocyte signature and were spontaneously beating (304). To eliminate the transplantation side effects, another strategy aiming to direct reprogramming in vivo was performed using the GMT and GHMT retroviral cocktails. Administration of the viral cocktail was performed directly in murine hearts after MI and lineage tracing of fibroblasts revealed that transduced resident fibroblasts were reprogrammed into cardiomyocyte-like cells. The reprogramming efficiency was lower than the one achieved in vitro. However, the de novo cardiomyocytes revealed similar behavior to endogenous cardiomyocytes which was better than that in vitro and resulted in reduced size of fibrotic tissue, implying the importance of the resident cardiac microenvironment (288, 294, 303, 305).
Obviously, the reprogrammed cells are new subjects in the cellular interactions governing the cardiac milieu. Thus, the better than expected in vivo performance of the reprogramming protocols may be owing to a paracrine action inhibiting resident cardiac fibroblast proliferation and associated fibrosis, promoting cardiac endothelial cell expansion (neoangiogenesis) or mediating cardioprotection by improving cardiomyocyte survival. In addition, as mentioned the inflammatory milieu of an injured heart may positively affect their engraftment, survival, and activity. However, inflammatory gene activation is not always beneficial as it appears to counteract adult cardiac fibroblast reprogramming (289).
Manipulating the Immune Response
Despite earlier failures [reviewed in (306, 307)], a recent large scale (>10,000 participants) clinical trial targeting IL-1β secured that manipulating inflammation is a valid target in ischemic heart failure (308, 309). Based on their abundance, interaction with cardiomyocytes, and fibroblasts and their potent reparatory phenotypes assumed during ischemic HF progress (125, 131, 178), macrophages can play a leading role. Accordingly, liposome-mediated induction of anti-inflammatory properties of macrophages promoted angiogenesis and preserved cardiac structure and function in a rat model of acute MI (310). Macrophage status and maturity may also affect cardiac regeneration as deduced from comparisons between neonatal and adult mouse injury models (120, 164, 311), as well as the regenerating newt and zebrafish, but not medaka, teleost hearts (179, 216). The potential pro-and anti-regeneration abilities of the particular macrophage subsets populating the mammalian and, more importantly, human heart (125) in steady state and upon injury have not been presently conclusively mapped, but this is an obvious task.
Moreover, innate immunity activation can be used as a driving force to mobilize stem cells and regeneration [reviewed in (312)] and is actually required for successful nuclear reprogramming, at least in the iPSC protocols (313). In this context, in a recent cell therapy trial combined macrophage and mesenchymal stem cell cardiac delivery into ischemic HF patients achieved 37% reduction in adverse cardiac events (including deaths and hospitalization) (314). Furthermore, when macrophages were depleted from rats undergoing MI, the cardioprotective effect of cardiospheres, administered after the infarct formation, was abolished (315). In the same setting, the cardiospheres paracrinely modified macrophages to confer in turn antiapoptotic protection to cardiomyocytes.
Whether signals released by the stressed cardiomyocytes upon various insults that recruit macrophages and other inflammatory cells [reviewed in (316)] can be artificially oriented toward a beneficial outcome is of great importance, but such attempts should be planned with caution for several reasons. First of all, some of the native signals are actually beneficial. Upon MI, border zone cardiomyocytes express early cardiac transcription factors in a process reminiscent of dedifferentiation. They also secrete the small Reg3β protein to recruit reparatory macrophages, thus protecting the intact remote myocardium from damage by inflammatory neutrophils (317). Second, resident and recruited macrophages may considerably differ in their contribution to cardiac repair and regeneration in a time-dependent manner. For instance, in an animal model of non-ischemic HF resident macrophages initially support angiogenesis and repair but macrophages recruited subsequently are anti-angiogenic and detrimental (145). Furthermore, immunomodulation of cardiac repair and regeneration should be feasible, but must be timely and properly applied, as well as in harmonious conjunction to the parenchymal and stromal cardiac cells (4, 22, 118, 204). Apart from redirecting immune response by modifying additional pathways and populations such as that of mast cells, DCs, as well as components of the adaptive immune response [elaborated in (118)], beneficial outcomes may also take advantage of the cardiac lymphatic endothelium that seems to moderate MI inflammation (318).
Conclusions-Perspectives
Recent advances enlightened important characteristics of cardiac cell populations and their mutual interactions, as well as the emerging opportunities to exploit these interactions toward efficient repair and regeneration following heart injury. In mammalian hearts, resident cardiac fibroblasts and macrophages and a permissive extracellular matrix sustain cardiomyocyte regeneration during a very short time window after birth. This opportunity is, however, lost later in life and minimal cardiomyocyte proliferation cannot support the loss of cardiac tissue after an injury. Whereas, resident macrophage subsets may sustain repair, mononuclear cell-derived macrophages, recruited in the heart by injured cardiomyocyte signals, may exert detrimental inflammation and polarize fibroblasts to promote cardiac fibrosis, hypertrophy, and further inflammation, building up a regeneration-hostile environment. Along with macrophages, additional immune cells become activated and involved mediating cardiac fibrosis and dysfunction in both acute and chronic injury and stress conditions. However, parts of the inflammatory response and its interactions with cardiac parenchymal and stromal cells are actually beneficial and necessary to sustain cardiac repair and regeneration following an insult or in interventional approaches.
Despite the tremendous research efforts and progress, human hearts remain unprotected after injury and insult. Any forced cardiomyocyte and progenitor mobilization attempts are of very limited efficacy and HF is still incurable with main medications targeting symptomatic neurohumoral changes and organ transplantation being the ultimate salvation tool. What is needed to achieve human cardiac repair and regeneration in the future? We hope it is obvious from the above that the answers to this central question will be multifaceted.
Basic research approaches combining Cre recombinase-based-or other genetic lineage tracing with single-cell transcriptomic and advanced proteomic and metabolomic analyses, should clarify the identities and qualities of the major cardiac cell populations and their timely changes following particular insults leading to HF. This is important and challenging given the plasticity of fibroblasts and macrophages and a certain degree of overlapping identities among cardiac cells (88, 100, 319). It is also expected to increase the probability of revealing novel target pathways and molecules for modifiable pro-regenerative protocols. It will be very crucial to find efficient ways to guide in vivo resident cardiac cells such as fibroblasts to transdifferentiate to a cardiogenic fate instead of a fibrotic one, thus accomplishing both decrease in fibrosis and more sufficient regeneration. Interventions targeting ECM appear to be particularly important for cardiac regeneration and repair. At the preclinical level, the new technologies using ECM scaffolds and injectable hydrogels are expected to further develop, aiming to improve the tissue reception of exogenous stem-or cardiac-committed cells. The latter appear to contribute more in a paracrine manner rather than by getting transformed to cardiomyocytes, thus analysis of their secretomes and efficient delivery are expected to improve repair. In addition, cross-species approaches such as administration of zebrafish ECM to murine hearts (320) provide useful links to successful pro-regenerative recipes. Furthermore, when manipulating fibrosis one should always keep an eye on its beneficial roles even for regeneration (217). Finally, exosome-, liposome- and nanoparticle-based advances in cell-free delivery technologies of therapeutic molecules (ranging from biologicals, antibodies, and antagonists to microRNAs, proteins, and even whole cell secretomes) are underway to more efficiently target myocardial tissue (4, 118, 204). Ideally, such approaches would combine pro-regenerative identities from all cell players, that is, native or engineered cardiomyocytes, fibroblasts, and cardiac macrophages, simultaneously excluding any of their counter actions that are known or will be revealed in the near future to inhibit regeneration. Furthermore, the mutual interactions of cardiomyocytes and the non-myocytes during HF progress should be carefully taken into account, to maximize the benefits of timely planned interventions. The success will probably lie in therapies simultaneously manipulating more than one cardiac or extracardiac population.
All these efforts attempt to either directly replenish the failing cardiomyocyte population or motivate endogenous pro-repair and pro-regenerative pathways in the human heart. The precise understanding of cardiomyocyte, stromal, and immune cell identities and interactions in steady state and at particular time points during the course of cardiac injury will allow our success in a currently unresolved health issue.
Author Contributions
All authors listed have made a substantial, direct and intellectual contribution to the work, and approved it for publication.
Conflict of Interest Statement
The authors declare that the research was conducted in the absence of any commercial or financial relationships that could be construed as a potential conflict of interest.
Abbreviations
CF, Cardiac Fibroblasts; CS, Cardiospheres; CPC, Cardiomyocyte Progenitor Cell; CSC, Cardiomyocyte Stem Cell; CSP, Cardiac Side Population Cell; DAMP, damage-associated molecular pattern; DC, dendritic cell; ECM, Extracellular Matrix; EMT, endothelial-to-mesenchymal transition; HF, Heart Failure; HFpEF, HF with preserved in ejection fraction; iCM, Induced Cardiomyocyte-like Cell; iPSC, Induced Pluripotent Stem Cell; iPSC-CM, iPSC-derived cardiomyocytes; MI, Myocardial Infarction; MSC, Mesenchymal Stromal Cell; TAC, Thoracic Aortic Constriction.
References
1. Wells JM, Watt FM. Diverse mechanisms for endogenous regeneration and repair in mammalian organs. Nature. (2018) 557:322–8. doi: 10.1038/s41586-018-0073-7
2. Karin M, Clevers H. Reparative inflammation takes charge of tissue regeneration. Nature. (2016) 529:307–14. doi: 10.1038/nature17039
3. Frangogiannis NG. The inflammatory response in myocardial injury, repair, and remodelling. Nat Rev Cardiol. (2014) 11:255–65. doi: 10.1038/nrcardio.2014.28
4. Hashimoto H, Olson EN, Bassel-Duby R. Therapeutic approaches for cardiac regeneration and repair. Nat Rev Cardiol. (2018) 15:585–600. doi: 10.1038/s41569-018-0036-6
5. Cahill TJ, Choudhury RP, Riley PR. Heart regeneration and repair after myocardial infarction: translational opportunities for novel therapeutics. Nat Rev Drug Discov. (2017) 16:699–717. doi: 10.1038/nrd.2017.106
6. Ziaeian B, Fonarow GC. Epidemiology and aetiology of heart failure. Nat Rev Cardiol. (2016) 13:368–78. doi: 10.1038/nrcardio.2016.25
7. Orogo AM, Gustafsson ÅB. Cell death in the myocardium: my heart won't go on. IUBMB Life. (2013) 65:651–6. doi: 10.1002/iub.1180
8. Brown DA, Perry JB, Allen ME, Sabbah HN, Stauffer BL, Shaikh SR, et al. Expert consensus document: mitochondrial function as a therapeutic target in heart failure. Nat Rev Cardiol. (2017) 14:238–50. doi: 10.1038/nrcardio.2016.203
9. Tsikitis M, Galata Z, Mavroidis M, Psarras S, Capetanaki Y. Intermediate filaments in cardiomyopathy. Biophys Rev. (2018) 10:1007–31. doi: 10.1007/s12551-018-0443-2
10. Capetanaki Y, Papathanasiou S, Diokmetzidou A, Vatsellas G, Tsikitis M. Desmin related disease: a matter of cell survival failure. Curr Opin Cell Biol. (2015) 32:113–20. doi: 10.1016/j.ceb.2015.01.004
11. Milner DJ, Weitzer G, Tran D, Bradley A, Capetanaki Y. Disruption of muscle architecture and myocardial degeneration in mice lacking desmin. J Cell Biol. (1996) 134:1255–70. doi: 10.1083/jcb.134.5.1255
12. Milner DJ, Taffet GE, Wang X, Pham T, Tamura T, Hartley C, et al. The absence of desmin leads to cardiomyocyte hypertrophy and cardiac dilation with compromised systolic function. J Mol Cell Cardiol. (1999) 31:2063–76. doi: 10.1006/jmcc.1999.1037
13. Milner DJ, Mavroidis M, Weisleder N, Capetanaki Y. Desmin cytoskeleton linked to muscle mitochondrial distribution and respiratory function. J Cell Biol. (2000) 150:1283–97. doi: 10.1083/jcb.150.6.1283
14. Papathanasiou S, Rickelt S, Soriano ME, Schips TG, Maier HJ, Davos CH, et al. Tumor necrosis factor-α confers cardioprotection through ectopic expression of keratins K8 and K18. Nat Med. (2015) 21:1076–84. doi: 10.1038/nm.3925
15. Diokmetzidou A, Soumaka E, Kloukina I, Tsikitis M, Makridakis M, Varela A, et al. Desmin and αB-crystallin interplay in the maintenance of mitochondrial homeostasis and cardiomyocyte survival. J Cell Sci. (2016) 129:3705–20. doi: 10.1242/jcs.192203
16. Rapti K, Diokmetzidou A, Kloukina I, Milner DJ, Varela A, Davos CH, et al. Opposite effects of catalase and MnSOD ectopic expression on stress induced defects and mortality in the desmin deficient cardiomyopathy model. Free Radic Biol Med. (2017) 110:206–18. doi: 10.1016/j.freeradbiomed.2017.06.010
17. Psarras S, Mavroidis M, Sanoudou D, Davos CH, Xanthou G, Varela AE, et al. Regulation of adverse remodelling by osteopontin in a genetic heart failure model. Eur Heart J. (2012) 33:1954–63. doi: 10.1093/eurheartj/ehr119
18. Mavroidis M, Davos CH, Psarras S, Varela A.C., Athanasiadis N, Katsimpoulas M, et al. Complement system modulation as a target for treatment of arrhythmogenic cardiomyopathy. Basic Res Cardiol. (2015) 110:27. doi: 10.1007/s00395-015-0485-6
19. Porrello ER, Olson EN, Sadek HA. Transient regenerative potential of the neonatal mouse heart. Science. (2011) 331:1078–80. doi: 10.1126/science.1200708
20. Poss KD, Wilson LG, Keating MT. Heart regeneration in zebrafish. Science. (2002) 298:2188–90. doi: 10.1126/science.1077857
21. Gonzalez-Rosa JM, Martin V, Peralta M, Torres M, Mercader N. Extensive scar formation and regression during heart regeneration after cryoinjury in zebrafish. Development. (2011) 138:1663–74. doi: 10.1242/dev.060897
22. Swirski FK, Nahrendorf M. Cardioimmunology: the immune system in cardiac homeostasis and disease. Nat Rev Immunol. (2018) 18:733–44. doi: 10.1038/s41577-018-0065-8
23. Furtado MB, Nim HT, Boyd SE, Rosenthal NA. View from the heart: cardiac fibroblasts in development, scarring and regeneration. Development. (2016) 143:387–97. doi: 10.1242/dev.120576
24. Meilhac SM, Buckingham ME. The deployment of cell lineages that form the mammalian heart. Nat Rev Cardiol. (2018) 15:705–24. doi: 10.1038/s41569-018-0086-9
25. Pinto AR, Ilinykh A, Ivey MJ, Kuwabara JT, D'antoni ML, Debuque R, et al. Revisiting cardiac cellular composition. Circ Res. (2016) 118:400–9. doi: 10.1161/CIRCRESAHA.115.307778
26. Soonpaa MH, Kim KK, Pajak L, Franklin M, Field LJ. Cardiomyocyte DNA synthesis and binucleation during murine development. Am J Physiol. (1996) 271:H2183–9.
27. Reiss K, Cheng W, Ferber A, Kajstura J, Li P, Li B, et al. Overexpression of insulin-like growth factor-1 in the heart is coupled with myocyte proliferation in transgenic mice. Proc Natl Acad Sci USA. (1996) 93:8630–5. doi: 10.1073/pnas.93.16.8630
28. Bergmann O, Bhardwaj RD, Bernard S, Zdunek S, Barnabé-Heide F, Walsh S, et al. Evidence for cardiomyocyte renewal in humans. Science. (2009) 324:98–102. doi: 10.1126/science.1164680
29. Bergmann O, Zdunek S, Felker A, Salehpour M, Alkass K, Bernard S, et al. Dynamics of cell generation and turnover in the human heart. Cell. (2015) 161:1566–75. doi: 10.1016/j.cell.2015.05.026
30. Senyo SE, Steinhauser ML, Pizzimenti CL, Yang VK, Cai L, Wang M, et al. Mammalian heart renewal by pre-existing cardiomyocytes. Nature. (2013) 493:433–6. doi: 10.1038/nature11682
31. Ye L, D'Agostino G, Loo S, Wang C, Su L, Tan S, et al. Early regenerative capacity in the porcine heart. Circulation. (2018) 138:2798–808. doi: 10.1161/CIRCULATIONAHA.117.031542
32. Zhu W, Zhang E, Zhao M, Chong Z, Fan C, Tang Y, et al. Regenerative potential of neonatal porcine hearts. Circulation. (2018) 138:2809–16. doi: 10.1161/CIRCULATIONAHA.118.034886
33. Haubner BJ, Schneider J, Schweigmann U, Schuetz T, Dichtl W, Velik-Salchner C, et al. Functional recovery of a human neonatal heart after severe myocardial infarction. Circ Res. (2016) 118:216–21. doi: 10.1161/CIRCRESAHA.115.307017
34. Larson BJ, Longaker MT, Lorenz HP. Scarless fetal wound healing: a basic science review. Plast Reconstr Surg. (2010) 126:1172–80. doi: 10.1097/PRS.0b013e3181eae781
35. Bryant DM, O'Meara CC, Ho NN, Gannon J, Cai L, Lee RT. A systematic analysis of neonatal mouse heart regeneration after apical resection. J Mol Cell Cardiol. (2015) 79:315–8. doi: 10.1016/j.yjmcc.2014.12.011
36. Konfino T, Landa N, Ben-Mordechai T, Leor J. The type of injury dictates the mode of repair in neonatal and adult heart. J Am Heart Assoc. (2015) 4:e001320. doi: 10.1161/JAHA.114.001320
37. Andersen DC, Ganesalingam S, Jensen CH, Sheikh SP. Do neonatal mouse hearts regenerate following heart apex resection? Stem Cell Rep. (2014) 2:406–13. doi: 10.1016/j.stemcr.2014.02.008
38. Notari M, Ventura-Rubio A, Bedford-Guaus SJ, Jorba I, Mulero L, Navajas D, et al. The local microenvironment limits the regenerative potential of the mouse neonatal heart. Sci Adv. (2018) 4:eaao5553. doi: 10.1126/sciadv.aao5553
39. Kretzschmar K, Post Y, Bannier-Hélaouët M, Mattiotti A, Drost J, Basak O, et al. Profiling proliferative cells and their progeny in damaged murine hearts. Proc Natl Acad Sci USA. (2018) 115:E12245–54. doi: 10.1073/pnas.1805829115
40. Naqvi N, Li M, Calvert JW, Tejada T, Lambert JP, Wu J, et al. A proliferative burst during preadolescence establishes the final cardiomyocyte number. Cell. (2014) 157:795–807. doi: 10.1016/j.cell.2014.03.035
41. Mollova M, Bersell K, Walsh S, Savla J, Das LT, Park S-Y, et al. Cardiomyocyte proliferation contributes to heart growth in young humans. Proc Natl Acad Sci USA. (2013) 110:1446–51. doi: 10.1073/pnas.1214608110
42. Naqvi N, Singh R, Iismaa SE, Li M, Calvert JW, Martin DIK, et al. Cardiomyocytes replicate and their numbers increase in young hearts. Cell. (2015) 163:783–4. doi: 10.1016/j.cell.2015.10.038
43. Soonpaa MH, Zebrowski DC, Platt C, Rosenzweig A, Engel FB, Field LJ. Cardiomyocyte cell-cycle activity during preadolescence. Cell. (2015) 163:781–2. doi: 10.1016/j.cell.2015.10.037
44. Alkass K, Panula J, Westman M, Wu T Di, Guerquin-Kern JL, Bergmann O. No evidence for cardiomyocyte number expansion in preadolescent mice. Cell. (2015) 163:1026–36. doi: 10.1016/j.cell.2015.10.035
45. Patterson M, Barske L, Van Handel B, Rau CD, Gan P, Sharma A, et al. Frequency of mononuclear diploid cardiomyocytes underlies natural variation in heart regeneration. Nat Genet. (2017) 49:1346–53. doi: 10.1038/ng.3929
46. González-Rosa JM, Sharpe M, Field D, Soonpaa MH, Field LJ, Burns CE, et al. Myocardial polyploidization creates a barrier to heart regeneration in zebrafish. Dev Cell. (2018) 44:433–46.e7. doi: 10.1016/j.devcel.2018.01.021
47. Kim MY, Eiby YA, Lumbers ER, Wright LL, Gibson KJ, Barnett AC, et al. Effects of glucocorticoid exposure on growth and structural maturation of the heart of the preterm piglet. PLoS ONE. (2014) 9:e93407. doi: 10.1371/journal.pone.0093407
48. Machmoud A i, Porrello ER. Neonatal heart regeneration. Circulation. (2018) 138:2817–9. doi: 10.1161/CIRCULATIONAHA.118.037333
49. Aix E, Gutiérrez-Gutiérrez Ó, Sánchez-Ferrer C, Aguado T, Flores I. Postnatal telomere dysfunction induces cardiomyocyte cell-cycle arrest through p21 activation. J Cell Biol. (2016) 213:571–83. doi: 10.1083/jcb.201510091
50. Heallen T, Zhang M, Wang J, Bonilla-Claudio M, Klysik E, Johnson RL, et al. Proliferation and heart size hippo pathway inhibits Wnt signaling to restrain cardiomyocyte. Science. (2011) 332:458–61. doi: 10.1126/science.1199010
51. Nemir M, Metrich M, Plaisance I, Lepore M, Cruchet S, Berthonneche C, et al. The Notch pathway controls fibrotic and regenerative repair in the adult heart. Eur Heart J. (2014) 35:2174–85. doi: 10.1093/eurheartj/ehs269
52. D'Uva G, Aharonov A, Lauriola M, Kain D, Yahalom-Ronen Y, Carvalho S, et al. ERBB2 triggers mammalian heart regeneration by promoting cardiomyocyte dedifferentiation and proliferation. Nat Cell Biol. (2015) 17:627–38. doi: 10.1038/ncb3149
53. Polizzotti BD, Ganapathy B, Walsh S, Choudhury S, Ammanamanchi N, Bennett DG, et al. Neuregulin stimulation of cardiomyocyte regeneration in mice and human myocardium reveals a therapeutic window. Sci Transl Med. (2015) 7:281ra45. doi: 10.1126/scitranslmed.aaa5171
54. Reuter S, Soonpaa MH, Firulli AB, Chang AN, Field LJ. Recombinant neuregulin 1 does not activate cardiomyocyte DNA synthesis in normal or infarcted adult mice. PLoS ONE. (2014) 9:e115871. doi: 10.1371/journal.pone.0115871
55. Mohamed TMA, Ang YS, Radzinsky E, Zhou P, Huang Y, Elfenbein A, et al. Regulation of cell cycle to stimulate adult cardiomyocyte proliferation and cardiac regeneration. Cell. (2018) 173:104–16.e12. doi: 10.1016/j.cell.2018.02.014
56. Toischer K, Zhu W, Hünlich M, Mohamed BA, Khadjeh S, Reuter SP, et al. Cardiomyocyte proliferation prevents failure in pressure overload but not volume overload. J Clin Invest. (2017) 127:4285–96. doi: 10.1172/JCI81870
57. Talman V, Teppo J, Pöhö P, Movahedi P, Vaikkinen A, Karhu ST, et al. Molecular atlas of postnatal mouse heart development. J Am Heart Assoc. (2018) 7:e010378. doi: 10.1161/JAHA.118.010378
58. Puente BN, Kimura W, Muralidhar SA, Moon J, Amatruda JF, Phelps KL, et al. The oxygen-rich postnatal environment induces cardiomyocyte cell-cycle arrest through DNA damage response. Cell. (2014) 157:565–79. doi: 10.1016/j.cell.2014.03.032
59. Kimura W, Xiao F, Canseco DC, Muralidhar S, Thet S, Zhang HM, et al. Hypoxia fate mapping identifies cycling cardiomyocytes in the adult heart. Nature. (2015) 523:226–30. doi: 10.1038/nature14582
60. Leach JP, Heallen T, Zhang M, Rahmani M, Morikawa Y, Hill MC, et al. Hippo pathway deficiency reverses systolic heart failure after infarction. Nature. (2017) 550:260–4. doi: 10.1038/nature24045
61. Bassat E, Mutlak YE, Genzelinakh A, Shadrin IY, Baruch Umansky K, Yifa O, et al. The extracellular matrix protein agrin promotes heart regeneration in mice. Nature. (2017) 547:179–84. doi: 10.1038/nature22978
62. Morikawa Y, Heallen T, Leach J, Xiao Y, Martin JF. Dystrophin-glycoprotein complex sequesters Yap to inhibit cardiomyocyte proliferation. Nature. (2017) 547:227–31. doi: 10.1038/nature22979
63. Mahmoud AI, O'Meara CC, Gemberling M, Zhao L, Bryant DM, Zheng R, et al. Nerves regulate cardiomyocyte proliferation and heart regeneration. Dev Cell. (2015) 34:387–99. doi: 10.1016/j.devcel.2015.06.017
64. Graham E, Bergmann O. Dating the heart: exploring cardiomyocyte renewal in humans. Physiology. (2017) 32:33–41. doi: 10.1152/physiol.00015.2016
65. Frangogiannis NG. The immune system and cardiac repair. Pharmacol Res. (2008) 58:88–111. doi: 10.1016/j.phrs.2008.06.007
66. Nolte SV, Xu W, Rennekampff HO, Rodemann HP. Diversity of fibroblasts - A review on implications for skin tissue engineering. Cells Tissues Organs. (2008) 187:165–76. doi: 10.1159/000111805
67. Desjardins-Park HE, Foster DS, Longaker MT. Fibroblasts and wound healing: an update " Increased comprehension of dermal fibroblast heterogeneity may yield both mechanistic insights into existing therapies and inspiration for novel therapeutics targeting specific cell populations in wound heal. Regen Med. (2018) 13:491–5. doi: 10.2217/rme-2018-0073
68. Chang HY, Chi J-T, Dudoit S, Bondre C, van de Rijn M, Botstein D, et al. Diversity, topographic differentiation, and positional memory in human fibroblasts. Proc Natl Acad Sci USA. (2002) 99:12877–82. doi: 10.1073/pnas.162488599
69. Ivey MJ, Kuwabara JT, Pai JT, Moore RE, Sun Z, Tallquist MD. Resident fibroblast expansion during cardiac growth and remodeling. J Mol Cell Cardiol. (2018) 114:161–74. doi: 10.1016/j.yjmcc.2017.11.012
70. Tallquist MD. Cardiac fibroblasts: from origin to injury. Curr Opin Physiol. (2018) 1:75–9. doi: 10.1016/j.cophys.2017.08.002
71. Tallquist MD, Molkentin JD. Redefining the identity of cardiac fibroblasts. Nat Rev Cardiol. (2017) 14:484–91. doi: 10.1038/nrcardio.2017.57
72. Coulombe KLK, Bajpai VK, Andreadis ST, Murry CE. Heart regeneration with engineered myocardial tissue. Annu Rev Biomed Eng. (2014) 16:1–28. doi: 10.1146/annurev-bioeng-071812-152344
73. Lajiness JD, Conway SJ. The dynamic role of cardiac fibroblasts in development and disease. J Cardiovasc Transl Res. (2012) 5:739–48. doi: 10.1007/s12265-012-9394-3
74. Kohl P, Kamkin A, Kiseleva I, Noble D. Mechanosensitive fibroblasts in the sino-atrial node region of rat heart: interaction with cardiomyocytes and possible role. Exp Physiol. (1994) 79:943–56. doi: 10.1113/expphysiol.1994.sp003819
75. Ongstad E, Kohl P. Fibroblast-myocyte coupling in the heart: potential relevance for therapeutic interventions. J Mol Cell Cardiol. (2016) 91:238–46. doi: 10.1016/j.yjmcc.2016.01.010
76. Furtado M, Hasham M. Properties and immune function of cardiac fibroblasts. Adv Med Exp Biol. (2017) 1003:35–70. doi: 10.1007/978-3-319-57613-8_3
77. Pellman J, Zhang J, Sheikh F. Myocyte-fibroblast communication in cardiac fibrosis and arrhythmias: mechanisms and model systems. J Mol Cell Cardiol. (2016) 94:22–31. doi: 10.1016/j.yjmcc.2016.03.005
78. Shinde A V, Frangogiannis NG. Fibroblasts in myocardial infarction : a role in inflammation and repair. J Mol Cell Cardiol. (2014) 70:74–82. doi: 10.1016/j.yjmcc.2013.11.015
79. Frangogiannis NG. Fibroblasts and the extracellular matrix in right ventricular disease. Cardiovasc Res. (2017) 113:1453–64. doi: 10.1093/cvr/cvx146
80. Travers JG, Kamal FA, Robbins J, Yutzey KE, Burns C. Cardiac fibrosis : the fibroblast awakens. Circ Res. (2017) 118:1021–40. doi: 10.1161/CIRCRESAHA.115.306565
81. Gourdie RG, Dimmeler S, Kohl P. Novel therapeutic strategies targeting fibroblasts and fibrosis in heart disease. Nat Rev Drug Discov. (2016) 15:620–38. doi: 10.1038/nrd.2016.89
82. Trial JA, Entman ML, Cieslik KA. Mesenchymal stem cell-derived inflammatory fibroblasts mediate interstitial fibrosis in the aging heart. J Mol Cell Cardiol. (2016) 91:28–34. doi: 10.1016/j.yjmcc.2015.12.017
83. Wynn TA. Common and unique mechanisms regulate fibrosis in various fibroproliferative diseases. J Clin Invest. (2007) 117:524–9. doi: 10.1172/JCI31487
84. Klingberg F, Hinz B, White ES. The myofibroblast matrix: Implications for tissue repair and fibrosis. J Pathol. (2013) 229:298–309. doi: 10.1002/path.4104
85. Rinkevich Y, Walmsley GG, Hu MS, Maan ZN, Newman AM, Drukker M, et al. Identification and isolation of a dermal lineage with intrinsic fibrogenic potential. Science. (2014) 348:aaa2154. doi: 10.1126/science.aaa2151
86. Driskell RR, Lichtenberger BM, Hoste E, Kretzschmar K, Simons BD, Charalambous M, et al. Distinct fibroblast lineages determine dermal architecture in skin development and repair. Nature. (2013) 504:277–81. doi: 10.1038/nature12783
87. Moore-Morris T, Guimarães-camboa N, Banerjee I, Zambon AC, Kisseleva T, Velayoudon A, et al. Resident fibroblast lineages mediate pressure overload – induced cardiac fibrosis. J Clin Invest. (2014) 124:2921–34. doi: 10.1172/JCI74783
88. Liu Z, Wang L, Welch JD, Ma H, Zhou Y, Vaseghi HR, et al. Single-cell transcriptomics reconstructs fate conversion from fibroblast to cardiomyocyte. Nature. (2017) 551:100–4. doi: 10.1038/nature24454
89. Virag JI, Murry CE. Myofibroblast and endothelial cell proliferation during murine myocardial infarct repair. Am J Pathol. (2003) 163:2433–40. doi: 10.1016/S0002-9440(10)63598-5
90. Kanisicak O, Khalil H, Ivey MJ, Karch J, Maliken BD, Correll RN, et al. Genetic lineage tracing defines myofibroblast origin and function in the injured heart. Nat Commun. (2016) 7:1–14. doi: 10.1038/ncomms12260
91. Nakaya M, Watari K, Tajima M, Nakaya T, Matsuda S, Ohara H, et al. Cardiac myofibroblast engulfment of dead cells facilitates recovery after myocardial infarction. J Clin Invest. (2017) 127:383–401. doi: 10.1172/JCI83822
92. Gulati A, Jabbour A, Ismail TF, Guha K, Khwaja J, Raza S, et al. Association of fibrosis with mortality and sudden cardiac death in patients with nonischemic dilated cardiomyopathy. J Am Med Assoc. (2013) 309:896–908. doi: 10.1001/jama.2013.1363
93. Khalil H, Kanisicak O, Prasad V, Correll RN, Fu X, Schips T, et al. Fibroblast-specific TGF-β-Smad2/3 signaling underlies cardiac fibrosis. J Clin Invest. (2017) 127:3770–83. doi: 10.1172/JCI94753
94. Schafer S, Viswanathan S, Widjaja AA, Lim WW, Moreno-Moral A, DeLaughter DM, et al. IL-11 is a crucial determinant of cardiovascular fibrosis. Nature. (2017) 552:110–5. doi: 10.1038/nature24676
95. Burke RM, Lighthouse JK, Quijada P, Dirkx RA, Rosenberg A, Moravec CS, et al. Small proline-rich protein 2B drives stress-dependent p53 degradation and fibroblast proliferation in heart failure. Proc Natl Acad Sci USA. (2018) 115:E3436–45. doi: 10.1073/pnas.1717423115
96. Fu X, Blaxall BC, Molkentin JD, Fu X, Khalil H, Kanisicak O, et al. Specialized fibroblast differentiated states underlie scar formation in the infarcted mouse heart. J Clin Invest. (2018) 128:2127–43. doi: 10.1172/JCI98215
97. Pillai ICL, Li S, Romay M, Lam L, Lu Y, Huang J, et al. Cardiac fibroblasts adopt osteogenic fates and can be targeted to attenuate pathological heart calcification. Cell Stem Cell. (2017) 20:218–232.e5. doi: 10.1016/j.stem.2016.10.005
98. Mouton AJ, Ma Y, Rivera Gonzalez OJ, Daseke MJ, Flynn ER, Freeman TC, et al. Fibroblast polarization over the myocardial infarction time continuum shifts roles from inflammation to angiogenesis. Basic Res Cardiol. (2019) 114:6. doi: 10.1007/s00395-019-0715-4
99. Wohlfahrt T, Rauber S, Uebe S, Luber M, Soare A, Ekici A, et al. PU.1 controls fibroblast polarization and tissue fibrosis. Nature. (2019) 566:344–9. doi: 10.1038/s41586-019-0896-x
100. Furtado MB, Costa MW, Pranoto EA, Salimova E, Pinto AR, Lam NT, et al. Cardiogenic genes expressed in cardiac fibroblasts contribute to heart development and repair. Circ Res. (2014) 114:1422–34. doi: 10.1161/CIRCRESAHA.114.302530
101. Ivey MJ, Tallquist MD. Defining the cardiac fibroblast. Circ J. (2016) 80:2269–76. doi: 10.1253/circj.CJ-16-1003
102. Denu RA, Nemcek S, Bloom DD, Goodrich AD, Kim J, Mosher DF, et al. Fibroblasts and mesenchymal stromal/stem cells are phenotypically indistinguishable. Acta Haematol. (2016) 136:85–97. doi: 10.1159/000445096
103. Haniffa MA, Collin MP, Buckley CD, Dazzi F. Mesenchymal stem cells: the fibroblasts' new clothes? Haematologica. (2009) 94:258–63. doi: 10.3324/haematol.13699
104. Oka T, Akazawa H, Atsuhiko TN, Issei K. Angiogenesis and cardiac hypertrophy. Circ Res. (2014) 114:565–72. doi: 10.1161/CIRCRESAHA.114.300507
105. Cao J, Poss KD. The epicardium as a hub for heart regeneration. Nat Rev Cardiol. (2018) 15:631–47. doi: 10.1038/s41569-018-0046-4
106. Murray IR, Bally JE, Chen WCW, Dar A, Gonzalez ZN, Jensen AR, et al. Skeletal and cardiac muscle pericytes: Functions and therapeutic potential. Pharmacol Ther. (2017) 171:65–74. doi: 10.1016/j.pharmthera.2016.09.005
107. Kostin S. Cardiac telocytes in normal and diseased hearts. Semin Cell Dev Biol. (2016) 55:22–30. doi: 10.1016/j.semcdb.2016.02.023
108. He L, Huang X, Kanisicak O, Li Y, Wang Y, Li Y, et al. Preexisting endothelial cells mediate cardiac neovascularization after injury. J Clin Invest. (2017) 127:2968–81. doi: 10.1172/JCI93868
109. Banquet S, Gomez E, Nicol L, Edwards-Lévy F, Henry JP, Cao R, et al. Arteriogenic therapy by intramyocardial sustained delivery of a novel growth factor combination prevents chronic heart failure. Circulation. (2011) 124:1059–69. doi: 10.1161/CIRCULATIONAHA.110.010264
110. Marín-juez R, Marass M, Gauvrit S, Rossi A, Lai S, Materna SC. Fast revascularization of the injured area is essential to support zebrafish heart regeneration. Proc Natl Acad Sci USA. (2016) 113:11237–42. doi: 10.1073/pnas.1605431113
111. Kramann R, Schneider RK, Dirocco DP, Machado F, Fleig S, Bondzie PA, et al. Perivascular Gli1+progenitors are key contributors to injury-induced organ fibrosis. Cell Stem Cell. (2015) 16:51–66. doi: 10.1016/j.stem.2014.11.004
112. Zhou B, Honor LB, He H, Qing M, Oh JH, Butterfield C, et al. Adult mouse epicardium modulates myocardial injury by secreting paracrine factors. J Clin Invest. (2011) 121:1894–904. doi: 10.1172/JCI45529
113. Wei K, Serpooshan V, Hurtado C, Diez-Cunado M, Zhao M, Maruyama S, et al. Epicardial FSTL1 reconstitution regenerates the adult mammalian heart. Nature. (2015) 525:479–85. doi: 10.1038/nature15372
114. MacGrogan D, Münch J, de la Pompa JL. Notch and interacting signalling pathways in cardiac development, disease, and regeneration. Nat Rev Cardiol. (2018) 15:685–704. doi: 10.1038/s41569-018-0100-2
115. Ntari L, Sakkou M, Chouvardas P, Mourouzis I, Prados A, Denis MC, et al. Comorbid TNF-mediated heart valve disease and chronic polyarthritis share common mesenchymal cell-mediated aetiopathogenesis. Ann Rheum Dis. (2018) 77:926–34. doi: 10.1136/annrheumdis-2017-212597
116. Zhao B, Liao Z, Chen S, Yuan Z, Yilin C, Lee KKH, et al. Intramyocardial transplantation of cardiac telocytes decreases myocardial infarction and improves post-infarcted cardiac function in rats. J Cell Mol Med. (2014) 18:780–9. doi: 10.1111/jcmm.12259
117. Bei Y, Zhou Q, Sun Q, Xiao J. Telocytes in cardiac regeneration and repair. Semin Cell Dev Biol. (2016) 55:14–21. doi: 10.1016/j.semcdb.2016.01.037
118. Forte E, Furtado MB, Rosenthal N. The interstitium in cardiac repair: role of the immune–stromal cell interplay. Nat Rev Cardiol. (2018) 15:601–16. doi: 10.1038/s41569-018-0077-x
119. Ramos GC, van den Berg A, Nunes-Silva V, Weirather J, Peters L, Burkard M, et al. Myocardial aging as a T-cell–mediated phenomenon. Proc Natl Acad Sci USA. (2017) 114:E2420–9. doi: 10.1073/pnas.1621047114
120. Lavine KJ, Epelman S, Uchida K, Weber KJ, Nichols CG, Schilling JD, et al. Distinct macrophage lineages contribute to disparate patterns of cardiac recovery and remodeling in the neonatal and adult heart. Proc Natl Acad Sci USA. (2014) 111:16029–34. doi: 10.1073/pnas.1406508111
121. Heidt T, Courties G, Dutta P, Sager HB, Sebas M, Iwamoto Y, et al. Differential contribution of monocytes to heart macrophages in steady-state and after myocardial infarction. Circ Res. (2014) 115:284–95. doi: 10.1161/CIRCRESAHA.115.303567
122. Epelman S, Lavine KJ, Beaudin AE, Sojka DK, Carrero JA, Calderon B, et al. Embryonic and adult-derived resident cardiac macrophages are maintained through distinct mechanisms at steady state and during inflammation. Immunity. (2014) 40:91–104. doi: 10.1016/j.immuni.2013.11.019
123. Leid J, Carrelha J, Boukarabila H, Epelman S, Jacobsen SEW, Lavine KJ. Primitive embryonic macrophages are required for coronary development and maturation. Circ Res. (2016) 118:1498–511. doi: 10.1161/CIRCRESAHA.115.308270
124. Hulsmans M, Clauss S, Xiao L, Aguirre AD, King KR, Hanley A, et al. Macrophages facilitate electrical conduction in the heart. Cell. (2017) 169:510–22.e20. doi: 10.1016/j.cell.2017.03.050
125. Bajpai G, Schneider C, Wong N, Bredemeyer A, Hulsmans M, Nahrendorf M, et al. The human heart contains distinct macrophage subsets with divergent origins and functions. Nat Med. (2018) 24:1234–45. doi: 10.1038/s41591-018-0059-x
126. Amit I, Winter DR, Jung S. The role of the local environment and epigenetics in shaping macrophage identity and their effect on tissue homeostasis. Nat Immunol. (2016) 17:18–25. doi: 10.1038/ni.3325
127. Nahrendorf M, Swirski FK. Abandoning M1/M2 for a network model of macrophage function. Circ Res. (2016) 119:414–7. doi: 10.1161/CIRCRESAHA.116.309194
128. Ley K. M1 means kill; M2 means heal. J Immunol. (2017) 199:2191–3. doi: 10.4049/jimmunol.1701135
129. Murray PJ. Macrophage Polarization. Annu Rev Physiol. (2017) 79:541–66. doi: 10.1146/annurev-physiol-022516-034339
130. Pinto AR, Paolicelli R, Salimova E, Gospocic J, Slonimsky E, Bilbao-Cortes D, et al. An abundant tissue macrophage population in the adult murine heart with a distinct alternatively-activated macrophage profile. PLoS ONE. (2012) 7:e36814. doi: 10.1371/journal.pone.0036814
131. Sager HB, Hulsmans M, Lavine KJ, Moreira MB, Heidt T, Courties G, et al. Proliferation and recruitment contribute to myocardial macrophage expansion in chronic heart failure. Circ Res. (2016) 119:853–64. doi: 10.1161/CIRCRESAHA.116.309001
132. Murray PJ, Allen JE, Biswas SK, Fisher EA, Gilroy DW, Goerdt S, et al. Macrophage activation and polarization: nomenclature and experimental guidelines. Immunity. (2014) 41:14–20. doi: 10.1016/j.immuni.2014.06.008
133. Mosser DM, Edwards JP. Exploring the full spectrum of macrophage activation. Nat Rev Immunol. (2008) 8:958–69. doi: 10.1038/nri2448
134. King KR, Aguirre AD, Ye YX, Sun Y, Roh JD, Ng RP, et al. IRF3 and type i interferons fuel a fatal response to myocardial infarction. Nat Med. (2017) 23:1481–7. doi: 10.1038/nm.4428
135. Dick SA, Macklin JA, Nejat S, Momen A, Clemente-Casares X, Althagafi MG, et al. Self-renewing resident cardiac macrophages limit adverse remodeling following myocardial infarction. Nat Immunol. (2019) 20:29–39. doi: 10.1038/s41590-018-0272-2
136. Mouton AJ, DeLeon-Pennell KY, Rivera Gonzalez OJ, Flynn ER, Freeman TC, Saucerman JJ, et al. Mapping macrophage polarization over the myocardial infarction time continuum. Basic Res Cardiol. (2018) 113:26. doi: 10.1007/s00395-018-0686-x
137. Kimbrough D, Wang SH, Wright LH, Mani SK, Kasiganesan H, LaRue AC, et al. HDAC inhibition helps post-MI healing by modulating macrophage polarization. J Mol Cell Cardiol. (2018) 119:51–63. doi: 10.1016/j.yjmcc.2018.04.011
138. Swirski FK, Nahrendorf M, Etzrodt M, Wildgruber M, Cortez-Retamozo V, Panizzi P, et al. Identification of splenic reservoir monocytes and their deployment to inflammatory sites. Science. (2009) 325:612–6. doi: 10.1126/science.1175202
139. Nahrendorf M, Swirski FK, Aikawa E, Stangenberg L, Wurdinger T, Figueiredo J-L, et al. The healing myocardium sequentially mobilizes two monocyte subsets with divergent and complementary functions. J Exp Med. (2007) 204:3037–47. doi: 10.1084/jem.20070885
140. Leuschner F, Rauch PJ, Ueno T, Gorbatov R, Marinelli B, Lee WW, et al. Rapid monocyte kinetics in acute myocardial infarction are sustained by extramedullary monocytopoiesis. J Exp Med. (2012) 209:123–37. doi: 10.1084/jem.20111009
141. Bajpai G, Bredemeyer A, Li W, Zaitsev K, Koenig AL, Lokshina I, et al. Tissue resident CCR2- and CCR2+ cardiac macrophages differentially orchestrate monocyte recruitment and fate specification following myocardial injury. Circ Res. (2018) 124:263–78. doi: 10.1161/CIRCRESAHA.118.314028
142. Lörchner H, Hou Y, Adrian-Segarra JM, Kulhei J, Detzer J, Günther S, et al. Reg proteins direct accumulation of functionally distinct macrophage subsets after myocardial infarction. Cardiovasc Res. (2018) 114:1667–79. doi: 10.1093/cvr/cvy126
143. Valaperti A, Nishii M, Liu Y, Naito K, Chan M, Zhang L, et al. Innate immune interleukin-1 receptor-associated kinase 4 exacerbates viral myocarditis by reducing CCR5+CD11b+ monocyte migration and impairing interferon production. Circulation. (2013) 128:1542–54. doi: 10.1161/CIRCULATIONAHA.113.002275
144. Hulsmans M, Sager HB, Roh JD, Valero-Muñoz M, Houstis NE, Iwamoto Y, et al. Cardiac macrophages promote diastolic dysfunction. J Exp Med. (2018) 215:423–40. doi: 10.1084/jem.20171274
145. Liao X, Shen Y, Zhang R, Sugi K, Vasudevan NT, Alaiti MA, et al. Distinct roles of resident and nonresident macrophages in nonischemic cardiomyopathy. Proc Natl Acad Sci USA. (2018) 115:E4661–9. doi: 10.1073/pnas.1720065115
146. Patel B, Bansal SS, Ismahil MA, Hamid T, Rokosh G, Mack M, et al. CCR2+ monocyte-derived infiltrating macrophages are required for adverse cardiac remodeling during pressure overload. JACC Basic to Transl Sci. (2018) 3:230–44. doi: 10.1016/j.jacbts.2017.12.006
147. Laroumanie F, Douin-Echinard V, Pozzo J, Lairez O, Tortosa F, Vinel C, et al. CD4+ T cells promote the transition from hypertrophy to heart failure during chronic pressure overload. Circulation. (2014) 129:2111–24. doi: 10.1161/CIRCULATIONAHA.113.007101
148. Kallikourdis M, Martini E, Carullo P, Sardi C, Roselli G, Greco CM, et al. T cell costimulation blockade blunts pressure overload-induced heart failure. Nat Commun. (2017) 8:14680. doi: 10.1038/ncomms14680
149. Horckmans M, Ring L, Duchene J, Santovito D, Schloss MJ, Drechsler M, et al. Neutrophils orchestrate post-myocardial infarction healing by polarizing macrophages towards a reparative phenotype. Eur Heart J. (2017) 38:187–97. doi: 10.1093/eurheartj/ehw002
150. Ma Y, Yabluchanskiy A, Iyer RP, Cannon PL, Flynn ER, Jung M, et al. Temporal neutrophil polarization following myocardial infarction. Cariovasc Res. (2016) 110:51–61. doi: 10.1093/cvr/cvw024
151. Li W, Hsiao H-M, Higashikubo R, Saunders BT, Bharat A, Goldstein DR, et al. Heart-resident CCR2+ macrophages promote neutrophil extravasation through TLR9/MyD88/CXCL5 signaling. JCI Insight. (2016) 1:e87315. doi: 10.1172/jci.insight.87315
152. Levick SP, Melndez GC, Plante E, McLarty JL, Brower GL, Janicki JS. Cardiac mast cells: the centrepiece in adverse myocardial remodelling. Cardiovasc Res. (2011) 89:12–9. doi: 10.1093/cvr/cvq272
153. Zhang W, Chancey AL, Tzeng HP, Zhou Z, Lavine KJ, Gao F, et al. The development of myocardial fibrosis in transgenic mice with targeted overexpression of tumor necrosis factor requires mast cell-fibroblast interactions. Circulation. (2011) 124:2106–16. doi: 10.1161/CIRCULATIONAHA.111.052399
154. Ngkelo A, Richart A, Kirk JA, Bonnin P, Vilar J, Lemitre M, et al. Mast cells regulate myofilament calcium sensitization and heart function after myocardial infarction. J Exp Med. (2016) 213:1353–74. doi: 10.1084/jem.20160081
155. Van der Borght K, Scott CL, Nindl V, Bouché A, Martens L, Sichien D, et al. Myocardial infarction primes autoreactive T cells through activation of dendritic cells. Cell Rep. (2017) 18:3005–17. doi: 10.1016/j.celrep.2017.02.079
156. Clemente-Casares X, Hosseinzadeh S, Barbu I, Dick SA, Macklin JA, Wang Y, et al. A CD103+ conventional dendritic cell surveillance system prevents development of overt heart failure during subclinical viral myocarditis. Immunity. (2017) 47:974–989.e8. doi: 10.1016/j.immuni.2017.10.011
157. Ramjee V, Li D, Manderfield LJ, Liu F, Engleka KA, Aghajanian H, et al. Epicardial YAP/TAZ orchestrate an immunosuppressive response following myocardial infarction. J Clin Invest. (2017) 127:899–911. doi: 10.1172/JCI88759
158. Lee JS, Jeong S-J, Kim S, Chalifour L, Yun TJ, Miah MA, et al. Conventional dendritic cells impair recovery after myocardial infarction. J Immunol. (2018) 20:1784–98. doi: 10.4049/jimmunol.1800322
159. Anzai A, Anzai T, Nagai S, Maekawa Y, Naito K, Kaneko H, et al. Regulatory role of dendritic cells in postinfarction healing and left ventricular remodeling. Circulation. (2012) 125:1234–45. doi: 10.1161/CIRCULATIONAHA.111.052126
160. Ramos G, Hofmann U, Frantz S. Myocardial fibrosis seen through the lenses of T-cell biology. J Mol Cell Cardiol. (2016) 92:41–5. doi: 10.1016/j.yjmcc.2016.01.018
161. Ma Y, Mouton AJ, Lindsey ML. Cardiac macrophage biology in the steady-state heart, the aging heart, and following myocardial infarction. Transl Res. (2018) 191:15–28. doi: 10.1016/j.trsl.2017.10.001
162. Leor J, Palevski D, Amit U, Konfino T. Macrophages and regeneration: lessons from the heart. Semin Cell Dev Biol. (2016) 58:26–33. doi: 10.1016/j.semcdb.2016.04.012
163. Lavine KJ, Pinto AR, Epelman S, Kopecky BJ, Clemente-Casares X, Godwin J, et al. The macrophage in cardiac homeostasis and disease: JACC macrophage in CVD series (Part 4). J Am Coll Cardiol. (2018) 72:2213–30. doi: 10.1016/j.jacc.2018.08.2149
164. Aurora AB, Porrello ER, Tan W, Mahmoud AI, Hill JA, Bassel-duby R, et al. Macrophages are required for neonatal heart regeneration. J Clin Invest. (2014) 124:1382–92. doi: 10.1172/JCI72181
165. Zhang P, Su J, Mende U. Cross talk between cardiac myocytes and fibroblasts: from multiscale investigative approaches to mechanisms and functional consequences. AJP Hear Circ Physiol. (2012) 303:H1385–96. doi: 10.1152/ajpheart.01167.2011
166. He K, Shi X, Zhang X, Dang S, Ma X, Liu F, et al. Long-distance intercellular connectivity between cardiomyocytes and cardiofibroblasts mediated by membrane nanotubes. Cardiovasc Res. (2011) 92:39–47. doi: 10.1093/cvr/cvr189
167. Bang C, Batkai S, Dangwal S, Gupta SK, Foinquinos A, Holzmann A, et al. Cardiac fibroblast-derived microRNA passenger strand-enriched exosomes mediate cardiomyocyte hypertrophy. J Clin Invest. (2014) 124:2136–46. doi: 10.1172/JCI70577
168. Fredj S, Bescond J, Louault C, Delwail A, Lecron JC, Potreau D. Role of interleukin-6 in cardiomyocyte/cardiac fibroblast interactions during myocyte hypertrophy and fibroblast proliferation. J Cell Physiol. (2005) 204:428–36. doi: 10.1002/jcp.20307
169. Ieda M, Tsuchihashi T, Ivey KN, Ross RS, Hong T, Shaw RM. Cardiac fibroblasts regulate myocardial proliferation through b 1 integrin signaling. Dev Cell. (2009) 16:233–44. doi: 10.1016/j.devcel.2008.12.007
170. Teekakirikul P, Eminaga S, Toka O, Alcalai R, Wang L, Wakimoto H, et al. Cardiac fibrosis in mice with hypertrophic cardiomyopathy is mediated by non-myocyte proliferation and requires Tgf-β. J Clin Invest. (2010) 120:3520–9. doi: 10.1172/JCI42028
171. Bageghni SA, Hemmings KE, Zava N, Denton CP, Porter KE, Ainscough JFX, et al. Cardiac fibroblast-specific p38a map kinase promotes cardiac hypertrophy via a putative paracrine interleukin-6 signaling mechanism. FASEB J. (2018) 32:4941–54. doi: 10.1096/fj.201701455RR
172. Nuamnaichati N, Sato VH, Moongkarndi P, Parichatikanond W, Mangmool S. Sustained β-AR stimulation induces synthesis and secretion of growth factors in cardiac myocytes that affect on cardiac fibroblast activation. Life Sci. (2018) 193:257–69. doi: 10.1016/j.lfs.2017.10.034
173. Zhao D, Li C, Yan H, Li T, Qian M, Zheng N, et al. Cardiomyocyte derived miR-328 promotes cardiac fibrosis by paracrinely regulating adjacent fibroblasts. Cell Physiol Biochem. (2018) 46:1555–65. doi: 10.1159/000489201
174. Kong P, Shinde AV, Su Y, Russo I, Chen B, Saxena A, et al. Opposing actions of fibroblast and cardiomyocyte smad3 signaling in the infarcted myocardium. Circulation. (2018) 137:707–24. doi: 10.1161/CIRCULATIONAHA.117.029622
175. Anzai A, Choi JL, He S, Fenn AM, Nairz M, Rattik S, et al. The infarcted myocardium solicits GM-CSF for the detrimental oversupply of inflammatory leukocytes. J Exp Med. (2017) 214:3293–310. doi: 10.1084/jem.20170689
176. Wu L, Ong S, Talor MV, Barin JG, Baldeviano GC, Kass DA, et al. Cardiac fibroblasts mediate IL-17A–driven inflammatory dilated cardiomyopathy. J Exp Med. (2014) 211:1449–64. doi: 10.1084/jem.20132126
177. Jung M, Ma Y, Iyer RP, DeLeon-Pennell KY, Yabluchanskiy A, Garrett MR, et al. IL-10 improves cardiac remodeling after myocardial infarction by stimulating M2 macrophage polarization and fibroblast activation. Basic Res Cardiol. (2017) 112:33. doi: 10.1007/s00395-017-0622-5
178. Shiraishi M, Shintani Y, Shintani Y, Ishida H, Saba R, Yamaguchi A, et al. Alternatively activated macrophages determine repair of the infarcted adult murine heart. J Clin Invest. (2016) 126:2151–66. doi: 10.1172/JCI85782
179. Godwin JW, Debuque R, Salimova E, Rosenthal NA. Heart regeneration in the salamander relies on macrophage-mediated control of fibroblast activation and the extracellular landscape. npj Regen Med. (2017) 2:22. doi: 10.1038/s41536-017-0027-y
180. Gomez I, Duval V, Silvestre J-S. Cardiomyocytes and macrophages discourse on the method to govern cardiac repair. Front Cardiovasc Med. (2018) 5:134. doi: 10.3389/fcvm.2018.00134
181. Cao DJ, Schiattarella GG, Villalobos E, Jiang N, May HI, Li T, et al. Cytosolic DNA sensing promotes macrophage transformation and governs myocardial ischemic injury. Circulation. (2018) 137:2613–34. doi: 10.1161/CIRCULATIONAHA.117.031046
182. Wallach D, Kang TB, Kovalenko A. Concepts of tissue injury and cell death in inflammation: a historical perspective. Nat Rev Immunol. (2014) 14:51–9. doi: 10.1038/nri3561
183. Duprez L, Takahashi N, Van Hauwermeiren F, Vandendriessche B, Goossens V, Vanden Berghe T, et al. RIP kinase-dependent necrosis drives lethal systemic inflammatory response syndrome. Immunity. (2011) 35:908–18. doi: 10.1016/j.immuni.2011.09.020
184. Sharma D, Kanneganti TD. The cell biology of inflammasomes: Mechanisms of inflammasome activation and regulation. J Cell Biol. (2016) 213:617–29. doi: 10.1083/jcb.201602089
185. Kung G, Konstantinidis K, Kitsis RN. Programmed necrosis, not apoptosis, in the heart. Circ Res. (2011) 108:1017–36. doi: 10.1161/CIRCRESAHA.110.225730
186. Mezzaroma E, Toldo S, Farkas D, Seropian IM, Van Tassell BW, Salloum FN, et al. The inflammasome promotes adverse cardiac remodeling following acute myocardial infarction in the mouse. Proc Natl Acad Sci USA. (2011) 108:19725–30. doi: 10.1073/pnas.1108586108
187. Fadok VA, Bratton DL, Konowal A, Freed PW, Westcott JY, Henson PM. Macrophages that have ingested apoptotic cells in vitro inhibit proinflammatory cytokine production through autocrine/paracrine mechanisms involving TGF-β, PGE2, and PAF. J Clin Invest. (1998) 101:890–8. doi: 10.1172/JCI1112
188. Wan E, Yeap XY, Dehn S, Terry R, Novak M, Zhang S, et al. Enhanced efferocytosis of apoptotic cardiomyocytes through myeloid-epithelial-reproductive tyrosine kinase links acute inflammation resolution to cardiac repair after infarction. Circ Res. (2013) 113:1004–12. doi: 10.1161/CIRCRESAHA.113.301198
189. Howangyin KY, Zlatanova I, Pinto C, Ngkelo A, Cochain C, Rouanet M, et al. Myeloid-epithelial-reproductive receptor tyrosine kinase and milk fat globule epidermal growth factor 8 coordinately improve remodeling after myocardial infarction via local delivery of vascular endothelial growth factor. Circulation. (2016) 133:826–39. doi: 10.1161/CIRCULATIONAHA.115.020857
190. DeBerge M, Yeap XY, Dehn S, Zhang S, Grigoryeva L, Misener S, et al. MerTK cleavage on resident cardiac macrophages compromises repair after myocardial ischemia reperfusion injury. Circ Res. (2017) 121:930–40. doi: 10.1161/CIRCRESAHA.117.311327
191. Zhang S, Yeap XY, Grigoryeva L, Dehn S, DeBerge M, Tye M, et al. Cardiomyocytes induce macrophage receptor shedding to suppress phagocytosis. J Mol Cell Cardiol. (2015) 87:171–9. doi: 10.1016/j.yjmcc.2015.08.009
192. Whelan RS, Kaplinskiy V, Kitsis RN. Cell death in the pathogenesis of heart disease: mechanisms and significance. Annu Rev Physiol. (2010) 72:19–44. doi: 10.1146/annurev.physiol.010908.163111
193. Chiong M, Wang ZV, Pedrozo Z, Cao DJ, Troncoso R, Ibacache M, et al. Cardiomyocyte death: mechanisms and translational implications. Cell Death Dis. (2011) 2:e244–11. doi: 10.1038/cddis.2011.130
194. McCully JD, Wakiyama H, Hsieh YJ, Jones M, Levitsky S. Differential contribution of necrosis and apoptosis in myocardial ischemia-reperfusion injury. AJP Hear Circ Physiol. (2004) 286:H1923–35. doi: 10.1152/ajpheart.00935.2003
195. Gelpi RJ, Park M, Gao S, Dhar S, Vatner DE, Vatner SF. Apoptosis in severe, compensated pressure overload predominates in nonmyocytes and is related to the hypertrophy but not function. Am J Physiol Hear Circ Physiol. (2011) 300:1062–8. doi: 10.1152/ajpheart.00998.2010
196. Park M, Shen Y-T, Gaussin V, Heyndrickx GR, Bartunek J, Resuello RRG, et al. Apoptosis predominates in nonmyocytes in heart failure. Am J Physiol Circ Physiol. (2009) 297:H785–791. doi: 10.1152/ajpheart.00310.2009
197. Almeida Paiva R, Martins-Marques T, Jesus K, Ribeiro-Rodrigues T, Zuzarte M, Silva A, et al. Ischaemia alters the effects of cardiomyocyte-derived extracellular vesicles on macrophage activation. J Cell Mol Med. (2018) 23:1137–51. doi: 10.1111/jcmm.14014
198. Van Amerongen MJ, Harmsen MC, Van Rooijen N, Petersen AH, Van Luyn MJA. Macrophage depletion impairs wound healing and increases left ventricular remodeling after myocardial injury in mice. Am J Pathol. (2007) 170:818–29. doi: 10.2353/ajpath.2007.060547
199. Yu J, Seldin MM, Fu K, Li S, Lam L, Wang P, et al. Topological arrangement of cardiac fibroblasts regulates cellular plasticity novelty and significance. Circ Res. (2018) 123:73–85. doi: 10.1161/CIRCRESAHA.118.312589
200. Kim TY, Kofron CM, King ME, Markes AR, Okundaye AO, Qu Z, et al. Directed fusion of cardiac spheroids into larger heterocellular microtissues enables investigation of cardiac action potential propagation via cardiac fibroblasts. PLoS ONE. (2018) 13:e0196714. doi: 10.1371/journal.pone.0196714
201. Wang Y, Liang P, Lan F, Wu H, Lisowski L, Gu M, et al. Genome editing of isogenic human induced pluripotent stem cells recapitulates long QT phenotype for drug testing. J Am Coll Cardiol. (2014) 64:451–9. doi: 10.1016/j.jacc.2014.04.057
202. Hinson JT, Chopra A, Nafissi N, Polacheck WJ, Benson CC, Swist S, et al. Titin mutations in iPS cells define sarcomere insufficiency as a cause of dilated cardiomyopathy. Science. (2015) 349:982–6. doi: 10.1126/science.aaa5458
203. Vagnozzi RJ, Molkentin JD, Houser SR. New myocyte formation in the adult heart. Circ Res. (2018) 123:159–76. doi: 10.1161/CIRCRESAHA.118.311208
204. Menasché P. Cell therapy trials for heart regeneration — lessons learned and future directions. Nat Rev Cardiol. (2018) 15:659–71. doi: 10.1038/s41569-018-0013-0
205. Bournele D, Beis D. Zebrafish models of cardiovascular disease. Review. Hear Fail Rev. (2016) 21:803–13. doi: 10.1007/s10741-016-9579-y
206. Petrie TA, Strand NS, Yang C-T, Rabinowitz JS, Moon RT. Macrophages modulate adult zebrafish tail fin regeneration. Development. (2015) 142:2581–91. doi: 10.1242/dev.120642
207. Kyritsis N, Kizil C, Zocher S, Kroehne V, Kaslin J, Freudenreich D, et al. Acute inflammation initiates the regenerative response in the adult zebrafish brain. Science. (2012) 338:1353–6. doi: 10.1126/science.1228773
208. Mitchell DM, Lovel AG, Stenkamp DL. Dynamic changes in microglial and macrophage characteristics during degeneration and regeneration of the zebrafish retina. J Neuroinflamm. (2018) 15:163. doi: 10.1186/s12974-018-1185-6
209. Chablais F, Veit J, Rainer G, Jawiska A. The zebrafish heart regenerates after cryoinjury-induced myocardial infarction. BMC Dev Biol. (2011) 11:21. doi: 10.1186/1471-213X-11-21
210. Schnabel K, Wu CC, Kurth T, Weidinger G. Regeneration of cryoinjury induced necrotic heart lesions in zebrafish is associated with epicardial activation and cardiomyocyte proliferation. PLoS ONE. (2011) 6:e18503. doi: 10.1371/journal.pone.0018503
211. Curado S, Anderson RM, Jungblut B, Mumm J, Schroeter E, Stainier DYR. Conditional targeted cell ablation in zebrafish: A new tool for regeneration studies. Dev Dyn. (2007) 236:1025–35. doi: 10.1002/dvdy.21100
212. Wang J, Panáková D, Kikuchi K, Holdway JE, Gemberling M, Burris JS, et al. The regenerative capacity of zebrafish reverses cardiac failure caused by genetic cardiomyocyte depletion. Development. (2011) 138:3421–30. doi: 10.1242/dev.068601
213. Pisharath H, Rhee JM, Swanson MA, Leach SD, Parsons MJ. Targeted ablation of beta cells in the embryonic zebrafish pancreas using E.coli nitroreductase. Mech Dev. (2008) 124:218–29. doi: 10.1016/j.mod.2006.11.005
214. White DT, Mumm JS. The nitroreductase system of inducible targeted ablation facilitates cell-specific regenerative studies in zebrafish. Methods. (2013) 62:232–40. doi: 10.1016/j.ymeth.2013.03.017
215. De Preux Charles AS, Bise T, Baier F, Marro J, Jazwinska A. Distinct effects of inflammation on preconditioning and regeneration of the adult zebrafish heart. Open Biol. (2016) 6:160102. doi: 10.1098/rsob.160102
216. Lai SL, Marín-Juez R, Moura PL, Kuenne C, Lai JKH, Tsedeke AT, et al. Reciprocal analyses in zebrafish and medaka reveal that harnessing the immune response promotes cardiac regeneration. Elife. (2017) 6:e25605. doi: 10.7554/eLife.25605
217. Sánchez-iranzo H, Galardi-castilla M, Minguillón C, Sanz-morejón A, González-rosa JM, Felker A, et al. Transient fibrosis resolves via fibroblast inactivation in the regenerating zebrafish heart. Proc Natl Acad Sci USA. (2018) 115:4188–93. doi: 10.1073/pnas.1716713115
218. Choi WW-Y, Gemberling M, Wang J, Holdway JE, Shen M-CM, Karlstrom RO, et al. In vivo monitoring of cardiomyocyte proliferation to identify chemical modifiers of heart regeneration. Development. (2013) 666:660–6. doi: 10.1242/dev.088526
219. Kikuchi K, Holdway JE, Major RJ, Blum N, Dahn RD, Begemann G, et al. Retinoic acid production by endocardium and epicardium is an injury response essential for zebrafish heart regeneration. Dev Cell. (2011) 20:397–404. doi: 10.1016/j.devcel.2011.01.010
220. Wang J, Cao J, Dickson AL, Poss KD. Epicardial regeneration is guided by cardiac outflow tract and Hedgehog signalling. Nature. (2015) 522:226–30. doi: 10.1038/nature14325
221. Zhao L, Borikova AL, Ben-Yair R, Guner-Ataman B, MacRae CA, Lee RT, et al. Notch signaling regulates cardiomyocyte proliferation during zebrafish heart regeneration. Proc Natl Acad Sci USA. (2014) 111:1403–8. doi: 10.1073/pnas.1311705111
222. Itou J, Oishi I, Kawakami H, Glass TJ, Richter J, Johnson A, et al. Migration of cardiomyocytes is essential for heart regeneration in zebrafish. Development. (2012) 139:4133–42. doi: 10.1242/dev.079756
223. Kim J, Wu Q, Zhang Y, Wiens KM, Huang Y, Rubin N, et al. PDGF signaling is required for epicardial function and blood vessel formation in regenerating zebrafish hearts. Proc Natl Acad Sci USA. (2010) 107:17206–10. doi: 10.1073/pnas.0915016107
224. Karra R, Foglia MJ, Choi W-Y, Belliveau C, DeBenedittis P, Poss KD. Vegfaa instructs cardiac muscle hyperplasia in adult zebrafish. Proc Natl Acad Sci USA. (2018) 115:8805–10. doi: 10.1073/pnas.1722594115
225. Stockdale WT, Lemieux ME, Killen AC, Zhao J, Hu Z, Riepsaame J, et al. Heart regeneration in the Mexican cavefish. Cell Rep. (2018) 25:1997–2007. doi: 10.1016/j.celrep.2018.10.072
226. De Preux Charles AS, Bise T, Baier F, Sallin P, Jazwinska A. Preconditioning boosts regenerative programmes in the adult zebrafish heart. Open Biol. (2016) 6:160101. doi: 10.1098/rsob.160101
227. Cai CL, Molkentin JD. The elusive progenitor cell in cardiac regeneration: slip Slidin' away. Circ Res. (2017) 120:400–6. doi: 10.1161/CIRCRESAHA.116.309710
228. Beltrami AP, Barlucchi L, Torella D, Baker M, Limana F, Chimenti S, et al. Adult cardiac stem cells are multipotent and support myocardial regeneration. Cell. (2003) 114:763–76. doi: 10.1016/S0092-8674(03)00687-1
229. Oh H, Bradfute SB, Gallardo TD, Nakamura T, Gaussin V, Mishina Y, et al. Cardiac progenitor cells from adult myocardium: Homing, differentiation, and fusion after infarction. Proc Natl Acad Sci USA. (2003) 100:12313–8. doi: 10.1073/pnas.2132126100
230. Laugwitz KL, Moretti A, Lam J, Gruber P, Chen Y, Woodard S, et al. Postnatal isl1+cardioblasts enter fully differentiated cardiomyocyte lineages. Nature. (2005) 433:647–53. doi: 10.1038/nature03215
231. Messina E, De Angelis L, Frati G, Morrone S, Chimenti S, Fiordaliso F, et al. Isolation and expansion of adult cardiac stem cells from human and murine heart. Circ Res. (2004) 95:911–21. doi: 10.1161/01.RES.0000147315.71699.51
232. Yi-Li M, Jaichander P, Sanchez-Ortiz E, Bezprozvannaya S, Malladi VS, Bassel-duby R, et al. Identification of a multipotent Twist2-expressing cell population in the adult heart. Proc Natl Acad Sci USA. (2018) 115:E8430–8439. doi: 10.1073/pnas.1800526115
233. Goodell MA, Brose K, Paradis G, Conner AS, Mulligan RC. Isolation and functional properties of murine hematopoietic stem cells that are replicating in vivo. J Exp Med. (1996) 183:1797–806. doi: 10.1084/jem.183.4.1797
234. Hierlihy AM, Seale P, Lobe CG, Rudnicki MA, Megeney LA. The post-natal heart contains a myocardial stem cell population. FEBS Lett. (2002) 530:239–43. doi: 10.1016/S0014-5793(02)03477-4
235. Pfister O. CD31- but not CD31+ cardiac side population cells exhibit functional cardiomyogenic differentiation. Circ Res. (2005) 97:52–61. doi: 10.1161/01.RES.0000173297.53793.fa
236. Mouquet F, Pfister O, Jain M, Oikonomopoulos A, Ngoy S, Summer R, et al. Restoration of cardiac progenitor cells after myocardial infarction by self-proliferation and selective homing of bone marrow-derived stem cells. Circ Res. (2005) 97:1090–2. doi: 10.1161/01.RES.0000194330.66545.f5
237. Oyama T, Nagai T, Wada H, Naito AT, Matsuura K, Iwanaga K, et al. Cardiac side population cells have a potential to migrate and differentiate into cardiomyocytes in vitro and in vivo. J Cell Biol. (2007) 176:329–41. doi: 10.1083/jcb.200603014
238. Liang SX, Tan TYL, Gaudry L, Chong B. Differentiation and migration of Sca1+/CD31- cardiac side population cells in a murine myocardial ischemic model. Int J Cardiol. (2010) 138:40–9. doi: 10.1016/j.ijcard.2008.08.032
239. Yamahara K, Fukushima S, Coppen SR, Felkin LE, Varela-Carver A, Barton PJR, et al. Heterogeneic nature of adult cardiac side population cells. Biochem Biophys Res Commun. (2008) 371:615–20. doi: 10.1016/j.bbrc.2008.04.021
240. Cai CL, Liang X, Shi Y, Chu PH, Pfaff SL, Chen J, et al. Isl1 identifies a cardiac progenitor population that proliferates prior to differentiation and contributes a majority of cells to the heart. Dev Cell. (2003) 5:877–89. doi: 10.1016/S1534-5807(03)00363-0
241. Smart N, Bollini S, Dubé KN, Vieira JM, Zhou B, Davidson S, et al. De novo cardiomyocytes from within the activated adult heart after injury. Nature. (2011) 474:640–4. doi: 10.1038/nature10188
242. Bolli R, Chugh AR, D'Amario D, Loughran JH, Stoddard MF, Ikram S, et al. Cardiac stem cells in patients with ischaemic cardiomyopathy (SCIPIO): initial results of a randomised phase 1 trial. Lancet. (2011) 378:1847–57. doi: 10.1016/S0140-6736(11)61590-0
243. Makkar RR, Smith RR, Cheng K, Malliaras K, Thomson LE, Berman D, et al. Intracoronary cardiosphere-derived cells for heart regeneration after myocardial infarction (CADUCEUS): a prospective, randomised phase 1 trial. Lancet. (2012) 379:895–904. doi: 10.1016/S0140-6736(12)60195-0
244. Ellison GM, Vicinanza C, Smith AJ, Aquila I, Leone A, Waring CD, et al. Adult c-kitpos cardiac stem cells are necessary and sufficient for functional cardiac regeneration and repair. Cell. (2013) 154:827–42. doi: 10.1016/j.cell.2013.07.039
245. Sultana N, Zhang L, Yan J, Chen J, Cai W, Razzaque S, et al. Resident c-kit + cells in the heart are not cardiac stem cells. Nat Commun. (2015) 6:8701. doi: 10.1038/ncomms9701
246. Van Berlo JH, Kanisicak O, Maillet M, Vagnozzi RJ, Karch J, Lin SCJ, et al. C-kit+cells minimally contribute cardiomyocytes to the heart. Nature. (2014) 509:337–41. doi: 10.1038/nature13309
247. Vagnozzi R, Sargent M, Lin S, Palpant N, Murry C, Molkentin JD. Tracing of Sca-1+ cells reveals endothelial but not myogenic contribution to the murine heart. Circulation. (2018) 138:2931–9. doi: 10.1161/CIRCULATIONAHA.118.035210
248. Matsuura K, Honda A, Nagai T, Fukushima N, Iwanaga K, Tokunaga M, et al. Transplantation of cardiac progenitor cells ameliorates cardiac dysfunction after myocardial infarction in mice. J Clin Invest. (2009) 119:2204–17. doi: 10.1172/JCI37456
249. Huang C, Gu H, Yu Q, Manukyan MC, Poynter JA, Wang M. Sca-1+ cardiac stem cells mediate acute cardioprotection via paracrine factor SDF-1 following myocardial ischemia/reperfusion. PLoS ONE. (2011) 6:e29246. doi: 10.1371/journal.pone.0029246
250. Lee ST, White AJ, Matsushita S, Malliaras K, Steenbergen C, Zhang Y, et al. Intramyocardial injection of autologous cardiospheres or cardiosphere-derived cells preserves function and minimizes adverse ventricular remodeling in pigs with heart failure post-myocardial infarction. J Am Coll Cardiol. (2011) 57:455–65. doi: 10.1016/j.jacc.2010.07.049
251. Cheng K, Ibrahim A, Hensley MT, Shen D, Sun B, Middleton R, et al. Relative roles of CD90 and c-Kit to the regenerative efficacy of cardiosphere-derived cells in humans and in a mouse model of myocardial infarction. J Am Heart Assoc. (2014) 3:e001260. doi: 10.1161/JAHA.114.001260
252. Noseda M, Harada M, McSweeney S, Leja T, Belian E, Stuckey DJ, et al. PDGFRα demarcates the cardiogenic clonogenic Sca1+ stem/progenitor cell in adult murine myocardium. Nat Commun. (2015) 6:6930. doi: 10.1038/ncomms7930
253. Boni A, Urbanek K, Nascimbene A, Hosoda T, Zheng H, Delucchi F, et al. Notch1 regulates the fate of cardiac progenitor cells. Proc Natl Acad Sci USA. (2008) 105:15529–34. doi: 10.1073/pnas.0808357105
254. Mohri T, Fujio Y, Maeda M, Ito T, Iwakura T, Oshima Y, et al. Leukemia inhibitory factor induces endothelial differentiation in cardiac stem cells. J Biol Chem. (2006) 281:6442–7. doi: 10.1074/jbc.M508969200
255. Hamid T, Xu Y, Ismahil MA, Li Q, Jones SP, Bhatnagar A, et al. TNF receptor signaling inhibits cardiomyogenic differentiation of cardiac stem cells and promotes a neuroadrenergic-like fate. Am J Physiol Hear Circ Physiol. (2016) 311:H1189–201. doi: 10.1152/ajpheart.00904.2015
256. Dimova N, Wysoczynski M, Rokosh G. Stromal cell derived factor-1α promotes C-Kit+ cardiac stem/progenitor cell quiescence through casein kinase 1α and GSK3β. Stem Cells. (2014) 32:487–99. doi: 10.1002/stem.1534
257. Baghdadi MB, Castel D, Machado L, Fukada S, Birk DE, Relaix F, et al. Reciprocal signalling by Notch–Collagen V–CALCR retains muscle stem cells in their niche. Nature. (2018) 557:714–8. doi: 10.1038/s41586-018-0144-9
258. Qiu Y, Bayomy AF, Gomez M V, Bauer M, Du P, Yang Y, et al. A role for matrix stiffness in the regulation of cardiac side population cell function. Am J Physiol Heart Circ Physiol. (2015) 308:H990–7. doi: 10.1152/ajpheart.00935.2014
259. Mauretti A, Spaans S, Bax NAM, Sahlgren C, Bouten CVC. Cardiac progenitor cells and the interplay with their microenvironment. Stem Cells Int. (2017) 2017:7471582. doi: 10.1155/2017/7471582
260. Han C, Nie Y, Lian H, Liu R, He F, Huang H, et al. Acute inflammation stimulates a regenerative response in the neonatal mouse heart. Cell Res. (2015) 25:1137–51. doi: 10.1038/cr.2015.110
261. Tolar J, Wang X, Braunlin E, McElmurry RT, Nakamura Y, Bell S, et al. The host immune response is essential for the beneficial effect of adult stem cells after myocardial ischemia. Exp Hematol. (2007) 35:682–90. doi: 10.1016/j.exphem.2006.12.005
262. Lim S yeon, Cho DI, Jeong H, Kang H, Kim MR, Cho M, et al. Adjuvant role of macrophages in stem cell-induced cardiac repair in rats. Exp Mol Med. (2018) 50:143. doi: 10.1038/s12276-018-0171-5
263. Thum T, Bauersachs J, Poole-Wilson PA, Volk HD, Anker SD. The dying stem cell hypothesis: immune modulation as a novel mechanism for progenitor cell therapy in cardiac muscle. J Am Coll Cardiol. (2005) 46:1799–802. doi: 10.1016/j.jacc.2005.07.053
264. Vagnozzi RJ, Maillet M, Sargent MA, Khalil H, Johansen AK, Schwanekamp JA, et al. An acute immune response underlies the benefit of cardiac adult stem cell therapy. bioRxiv [Preprint]. Available online at: https://www.biorxiv.org/content/10.1101/506626v1 (Posted December 26, 2018; Accessed January 29, 2019).
265. Takahashi K, Yamanaka S. Induction of pluripotent stem cells from mouse embryonic and adult fibroblast cultures by defined factors. Cell. (2006) 126:663–76. doi: 10.1016/j.cell.2006.07.024
266. Mauritz C, Schwanke K, Reppel M, Neef S, Katsirntaki K, Maier LS, et al. Generation of functional murine cardiac myocytes from induced pluripotent stem cells. Circulation. (2008) 118:507–17. doi: 10.1161/CIRCULATIONAHA.108.778795
267. Mauritz C, Martens A, Rojas SV, Schnick T, Rathert C, Schecker N, et al. Induced pluripotent stem cell (iPSC)-derived Flk-1 progenitor cells engraft, differentiate, and improve heart function in a mouse model of acute myocardial infarction. Eur Heart J. (2011) 32:2634–41. doi: 10.1093/eurheartj/ehr166
268. Zhang J, Wilson GF, Soerens AG, Koonce CH, Yu J, Palecek SP, et al. Functional cardiomyocytes derived from human induced pluripotent stem cells. Circ Res. (2009) 104:e30–41. doi: 10.1161/CIRCRESAHA.108.192237
269. Nelson TJ, Martinez-Fernandez A, Yamada S, Perez-Terzic C, Ikeda Y, Terzic A. Repair of acute myocardial infarction by human stemness factors induced pluripotent stem cells. Circulation. (2009) 120:408–16. doi: 10.1161/CIRCULATIONAHA.109.865154
270. Ahmed RPH, Ashraf M, Buccini S, Shujia J, Haider HK. Cardiac tumorigenic potential of induced pluripotent stem cells in an immunocompetent host with myocardial infarction. Regen Med. (2011) 6:171–8. doi: 10.2217/rme.10.103
271. Zhang Y, Wang D, Chen M, Yang B, Zhang F, Cao K. Intramyocardial transplantation of undifferentiated rat induced pluripotent stem cells causes tumorigenesis in the heart. PLoS ONE. (2011) 6:e19012. doi: 10.1371/journal.pone.0019012
272. Dai B, Huang W, Xu M, Millard RW, Gao MH, Hammond HK, et al. Reduced collagen deposition in infarcted myocardium facilitates induced pluripotent stem cell engraftment and angiomyogenesis for improvement of left ventricular function. J Am Coll Cardiol. (2011) 58:2118–27. doi: 10.1016/j.jacc.2011.06.062
273. Kawamura M, Miyagawa S, Miki K, Saito A, Fukushima S, Higuchi T, et al. Feasibility, safety, and therapeutic efficacy of human induced pluripotent stem cell-derived cardiomyocyte sheets in a porcine ischemic cardiomyopathy model. Circulation. (2012) 126:S29–37. doi: 10.1161/CIRCULATIONAHA.111.084343
274. Chong JJH, Yang X, Don CW, Minami E, Liu YW, Weyers JJ, et al. Human embryonic-stem-cell-derived cardiomyocytes regenerate non-human primate hearts. Nature. (2014) 510:273–7. doi: 10.1038/nature13233
275. Davis RP, Casini S, Van Den Berg CW, Hoekstra M, Remme CA, Dambrot C, et al. Cardiomyocytes derived from pluripotent stem cells recapitulate electrophysiological characteristics of an overlap syndrome of cardiac sodium channel disease. Circulation. (2012) 125:3079–91. doi: 10.1161/CIRCULATIONAHA.111.066092
276. Wang G, McCain ML, Yang L, He A, Pasqualini FS, Agarwal A, et al. Modeling the mitochondrial cardiomyopathy of Barth syndrome with induced pluripotent stem cell and heart-on-chip technologies. Nat Med. (2014) 20:616–23. doi: 10.1038/nm.3545
277. Tse HF, Ho JCY, Choi SW, Lee YK, Butler AW, Ng KM, et al. Patient-specific induced-pluripotent stem cells-derived cardiomyocytes recapitulate the pathogenic phenotypes of dilated cardiomyopathy due to a novel DES mutation identified by whole exome sequencing. Hum Mol Genet. (2013) 22:1395–403. doi: 10.1093/hmg/dds556
278. Mayfield AE, Tilokee EL, Latham N, McNeill B, Lam BK, Ruel M, et al. The effect of encapsulation of cardiac stem cells within matrix-enriched hydrogel capsules on cell survival, post-ischemic cell retention and cardiac function. Biomaterials. (2014) 35:133–42. doi: 10.1016/j.biomaterials.2013.09.085
279. Ye L, Chang Y-H, Xiong Q, Zhang P, Zhang L, Somasundaram P, et al. Cardiac repair in a porcine model of acute myocardial infarction with human induced pluripotent stem cell-derived cardiovascular cells. Cell Stem Cell. (2014) 15:750–61. doi: 10.1016/j.stem.2014.11.009
280. Gaetani R, Yin C, Srikumar N, Braden R, Doevendans PA, Sluijter JPG, et al. Cardiac derived extracellular matrix enhances cardiogenic properties of human cardiac progenitor cells. Cell Transplant. (2015) 25:1653–63. doi: 10.3727/096368915X689794
281. Dergilev K, Tsokolaeva Z, Makarevich P, Beloglazova I, Zubkova E, Boldyreva M, et al. C-Kit cardiac progenitor cell based cell sheet improves vascularization and attenuates cardiac remodeling following myocardial infarction in rats. Biomed Res Int. (2018) 2018:3536854. doi: 10.1155/2018/3536854
282. Ibrahim AGE, Cheng K, Marbán E. Exosomes as critical agents of cardiac regeneration triggered by cell therapy. Stem Cell Rep. (2014) 2:606–19. doi: 10.1016/j.stemcr.2014.04.006
283. Khan M, Nickoloff E, Abramova T, Johnson J, Verma SK, Krishnamurthy P, et al. Embryonic stem cell-derived exosomes promote endogenous repair mechanisms and enhance cardiac function following myocardial infarction. Circ Res. (2015) 117:52–64. doi: 10.1161/CIRCRESAHA.117.305990
284. El Harane N, Kervadec A, Bellamy V, Pidial L, Neametalla HJ, Perier MC, et al. Acellular therapeutic approach for heart failure: in vitro production of extracellular vesicles from human cardiovascular progenitors. Eur Heart J. (2018) 39:1835–47. doi: 10.1093/eurheartj/ehy012
285. Cho GS, Lee DI, Tampakakis E, Murphy S, Andersen P, Uosaki H, et al. Neonatal transplantation confers maturation of PSC-derived cardiomyocytes conducive to modeling cardiomyopathy. Cell Rep. (2017) 18:571–82. doi: 10.1016/j.celrep.2016.12.040
286. Liau B, Jackman CP, Li Y, Bursac N. Developmental stage-dependent effects of cardiac fibroblasts on function of stem cell-derived engineered cardiac tissues. Sci Rep. (2017) 7:1–11. doi: 10.1038/srep42290
287. Ieda M, Fu JD, Delgado-Olguin P, Vedantham V, Hayashi Y, Bruneau BG, et al. Direct reprogramming of fibroblasts into functional cardiomyocytes by defined factors. Cell. (2010) 142:375–86. doi: 10.1016/j.cell.2010.07.002
288. Song K, Nam YJ, Luo X, Qi X, Tan W, Huang GN, et al. Heart repair by reprogramming non-myocytes with cardiac transcription factors. Nature. (2012) 485:599–604. doi: 10.1038/nature11139
289. Zhou H, Morales MG, Hashimoto H, Dickson ME, Song K, Ye W, et al. ZNF281 enhances cardiac reprogramming by modulating cardiac and inflammatory gene expression. Genes Dev. (2017) 31:1770–83. doi: 10.1101/gad.305482.117
290. Efe JA, Hilcove S, Kim J, Zhou H, Ouyang K, Wang G, et al. Conversion of mouse fibroblasts into cardiomyocytes using a direct reprogramming strategy. Nat Cell Biol. (2011) 13:215–22. doi: 10.1038/ncb2164
291. Wang H, Cao N, Spencer CI, Nie B, Ma T, Xu T, et al. Small molecules enable cardiac reprogramming of mouse fibroblasts with a single factor, oct4. Cell Rep. (2014) 6:951–60. doi: 10.1016/j.celrep.2014.01.038
292. Muraoka N, Yamakawa H, Miyamoto K, Sadahiro T, Umei T, Isomi M, et al. MiR-133 promotes cardiac reprogramming by directly repressing Snai1 and silencing fibroblast signatures. EMBO J. (2014) 33:1565–81. doi: 10.15252/embj.201387605
293. Yamakawa H, Muraoka N, Miyamoto K, Sadahiro T, Isomi M, Haginiwa S, et al. Fibroblast growth factors and vascular endothelial growth factor promote cardiac reprogramming under defined conditions. Stem Cell Rep. (2015) 5:1128–42. doi: 10.1016/j.stemcr.2015.10.019
294. Jayawardena TM, Egemnazarov B, Finch EA, Zhang L, Alan Payne J, Pandya K, et al. MicroRNA-mediated in vitro and In vivo direct reprogramming of cardiac fibroblasts to cardiomyocytes. Circ Res. (2012) 110:1465–73. doi: 10.1161/CIRCRESAHA.112.269035
295. Zhou Y, Wang L, Vaseghi HR, Liu Z, Lu R, Alimohamadi S, et al. Bmi1 is a key epigenetic barrier to direct cardiac reprogramming. Cell Stem Cell. (2016) 18:382–95. doi: 10.1016/j.stem.2016.02.003
296. Abad M, Hashimoto H, Zhou H, Morales MG, Chen B, Bassel-Duby R, et al. Notch inhibition enhances cardiac reprogramming by increasing MEF2C transcriptional activity. Stem Cell Rep. (2017) 8:548–60. doi: 10.1016/j.stemcr.2017.01.025
297. Mohamed TMA, Stone NR, Berry EC, Radzinsky E, Huang Y, Pratt K, et al. Chemical enhancement of in vitro and In vivo direct cardiac reprogramming. Circulation. (2017) 135:978–95. doi: 10.1161/CIRCULATIONAHA.116.024692
298. Zhao Y, Londono P, Cao Y, Sharpe EJ, Proenza C, O'Rourke R, et al. High-efficiency reprogramming of fibroblasts into cardiomyocytes requires suppression of pro-fibrotic signalling. Nat Commun. (2015) 6:8243. doi: 10.1038/ncomms9243
299. Zhou H, Dickson ME, Kim MS, Bassel-Duby R, Olson EN. Akt1/protein kinase B enhances transcriptional reprogramming of fibroblasts to functional cardiomyocytes. Proc Natl Acad Sci USA. (2015) 112:11864–9. doi: 10.1073/pnas.1516237112
300. Lalit PA, Salick MR, Nelson DO, Squirrell JM, Shafer CM, Patel NG, et al. Lineage reprogramming of fibroblasts into proliferative induced cardiac progenitor cells by defined factors. Cell Stem Cell. (2016) 18:354–67. doi: 10.1016/j.stem.2015.12.001
301. Fu JD, Stone NR, Liu L, Spencer CI, Qian L, Hayashi Y, et al. Direct reprogramming of human fibroblasts toward a cardiomyocyte-like state. Stem Cell Rep. (2013) 22:235–47. doi: 10.1016/j.stemcr.2013.07.005
302. Nam Y-J, Song K, Luo X, Daniel E, Lambeth K, West K, et al. Reprogramming of human fibroblasts toward a cardiac fate. Proc Natl Acad Sci USA. (2013) 110:5588–93. doi: 10.1073/pnas.1301019110
303. Wada R, Muraoka N, Inagawa K, Yamakawa H, Miyamoto K, Sadahiro T, et al. Induction of human cardiomyocyte-like cells from fibroblasts by defined factors. Proc Natl Acad Sci USA. (2013) 110:12667–72. doi: 10.1073/pnas.1304053110
304. Cao N, Huang Y, Zheng J, Spencer CI, Zhang Y, Fu JD, et al. Conversion of human fibroblasts into functional cardiomyocytes by small molecules. Science. (2016) 352:1216–20. doi: 10.1126/science.aaf1502
305. Qian L, Huang Y, Spencer CI, Foley A, Vedantham V, Liu L, et al. In vivo reprogramming of murine cardiac fibroblasts into induced cardiomyocytes. Nature. (2012) 485:593–8. doi: 10.1038/nature11044
306. Christia P, Frangogiannis NG. Targeting inflammatory pathways in myocardial infarction. Eur J Clin Invest. (2013) 43:986–95. doi: 10.1111/eci.12118
307. Mann DL. Innate immunity and the failing heart: the cytokine hypothesis revisited. Circ Res. (2015) 116:1254–68. doi: 10.1161/CIRCRESAHA.116.302317
308. Ridker PM, Everett BM, Thuren T, MacFadyen JG, Chang WH, Ballantyne C, et al. Antiinflammatory therapy with canakinumab for atherosclerotic disease. N Engl J Med. (2017) 377:1119–31. doi: 10.1056/NEJMoa1707914
309. Ridker PM, MacFadyen JG, Everett BM, Libby P, Thuren T, Glynn RJ. Relationship of C-reactive protein reduction to cardiovascular event reduction following treatment with canakinumab: a secondary analysis from the CANTOS randomised controlled trial. Lancet. (2017) 391:319–28. doi: 10.1016/S0140-6736(17)32814-3
310. Harel-Adar T, Mordechai T Ben, Amsalem Y, Feinberg MS, Leor J, Cohen S. Modulation of cardiac macrophages by phosphatidylserine-presenting liposomes improves infarct repair. Proc Natl Acad Sci USA. (2011) 108:1827–32. doi: 10.1073/pnas.1015623108
311. Riley PR. Fanning the flames to regenerate the heart. J Clin Invest. (2014) 124:961–4. doi: 10.1172/JCI74418
312. Aurora AB, Olson EN. Immune modulation of stem cells and regeneration. Stem Cell. (2014) 15:14–25. doi: 10.1016/j.stem.2014.06.009
313. Lee J, Sayed N, Hunter A, Au KF, Wong WH, Mocarski ES, et al. Activation of innate immunity is required for efficient nuclear reprogramming. Cell. (2012) 151:547–58. doi: 10.1016/j.cell.2012.09.034
314. Patel AN, Henry TD, Quyyumi AA, Schaer GL, Anderson RD, Toma C, et al. Ixmyelocel-T for patients with ischaemic heart failure: a prospective randomised double-blind trial. Lancet. (2016) 387:2412–21. doi: 10.1016/S0140-6736(16)30137-4
315. De Couto G, Liu W, Tseliou E, Sun B, Makkar N, Kanazawa H, et al. Macrophages mediate cardioprotective cellular postconditioning in acute myocardial infarction. J Clin Invest. (2015) 125:3147–62. doi: 10.1172/JCI81321
316. Ghigo A, Franco I, Morello F, Hirsch E. Myocyte signalling in leucocyte recruitment to the heart. Cardiovasc Res. (2014) 102:270–80. doi: 10.1093/cvr/cvu030
317. Lörchner H, Pöling J, Gajawada P, Hou Y, Polyakova V, Kostin S, et al. Myocardial healing requires Reg3β-dependent accumulation of macrophages in the ischemic heart. Nat Med. (2015) 21:353–62. doi: 10.1038/nm.3816
318. Vieira JM, Norman S, Del Campo CV, Cahill TJ, Barnette DN, Gunadasa-Rohling M, et al. The cardiac lymphatic system stimulates resolution of inflammation following myocardial infarction. J Clin Invest. (2018) 128:3402–12. doi: 10.1172/JCI97192
319. Quaife-Ryan GA, Sim CB, Ziemann M, Kaspi A, Rafehi H, Ramialison M, et al. Multicellular transcriptional analysis of mammalian heart regeneration. Circulation. (2017) 136:1123–39. doi: 10.1161/CIRCULATIONAHA.117.028252
Keywords: cardiac regeneration, heart failure, innate immune cells, cardiac repair, cardiomyocytes, cardiac macrophages, cardiac fibroblasts, fibrosis
Citation: Psarras S, Beis D, Nikouli S, Tsikitis M and Capetanaki Y (2019) Three in a Box: Understanding Cardiomyocyte, Fibroblast, and Innate Immune Cell Interactions to Orchestrate Cardiac Repair Processes. Front. Cardiovasc. Med. 6:32. doi: 10.3389/fcvm.2019.00032
Received: 16 November 2018; Accepted: 11 March 2019;
Published: 02 April 2019.
Edited by:
JeanSébastien Silvestre, Institut National de la Santé et de la Recherche Médicale (INSERM), FranceReviewed by:
Federico Quaini, University of Parma, ItalyClaudia Bearzi, Italian National Research Council (CNR), Italy
Clement Cochain, Universitätsklinikum Würzburg, Germany
Copyright © 2019 Psarras, Beis, Nikouli, Tsikitis and Capetanaki. This is an open-access article distributed under the terms of the Creative Commons Attribution License (CC BY). The use, distribution or reproduction in other forums is permitted, provided the original author(s) and the copyright owner(s) are credited and that the original publication in this journal is cited, in accordance with accepted academic practice. No use, distribution or reproduction is permitted which does not comply with these terms.
*Correspondence: Stelios Psarras, c3BzYXJyYXNAYmlvYWNhZGVteS5ncg==