- 1Division of Endocrinology and Metabolism, Department of Internal Medicine, Carver College of Medicine, University of Iowa, Iowa City, IA, United States
- 2Fraternal Order of Eagles Diabetes Research Centre, Carver College of Medicine, University of Iowa, Iowa City, IA, United States
Peripheral artery disease (PAD) is one of the major cardiovascular diseases that afflicts a large population worldwide. PAD results from occlusion of the peripheral arteries of the lower extremities. Although diabetes is a major risk factor for developing PAD, coexistence of PAD and diabetes poses significantly greater risk of developing critical limb threatening ischemia (CLTI) with poor prognosis for limb amputation and high mortality. Despite the prevalence of PAD, there are no effective therapeutic interventions as the molecular mechanism of how diabetes worsens PAD is not understood. With increasing cases of diabetes worldwide, the risk of complications in PAD have greatly increased. PAD and diabetes affect a complex web of multiple cellular, biochemical and molecular pathways. Therefore, it is important to understand the molecular components that can be targeted for therapeutic purposes. In this review, we describe some major developments in enhancing the understanding of the interactions of PAD and diabetes. We also provide results from our laboratory in this context.
Introduction
Peripheral artery disease (PAD) is the atherosclerotic occlusion of the peripheral arteries of the lower extremities. It is the third largest cardiovascular disease that afflicts more than 200 million people worldwide (1). A large number of cases go undiagnosed because often the patients have no complaint, and the symptoms and signs of the disease may appear only at an advanced stage of the disease. These symptoms primarily originate from diminished blood flow to the muscular tissue and range from intermittent claudication or pain with ambulation. Individuals may also present with rest pain, non-healing wounds, ulceration, and gangrene which are hallmarks of the more severe presentation of the disease termed critical limb threatening ischemia (CLTI). CTLI often leads to amputation and is associated with high mortality rates (2).
PAD has similar risk factors as coronary atherosclerosis, which includes old age, smoking, hypertension and diabetes. Unlike Coronary artery disease (CAD), diabetes and smoking accounts for most of the risk for developing PAD (3). Cardiovascular diseases including PAD are the most common cause of morbidity and mortality among adults with diabetes (4). With the global prevalence of diabetes mellitus rising from 171 million (Year 2000) to 366 million in 2030 (5), the prevalence of PAD and its severity also are expected to increase. However, beyond being a risk factor, the presence of diabetes worsens outcomes in individuals with PAD (6, 7). Among the risk factors for developing CTLI in PAD diabetes has the strongest effect (8). Both clinical data and preclinical studies with experimental models of PAD show that post-ischemic recovery is poor under diabetic conditions (6). Although diabetes in general dramatically increases the risk of developing CTLI and limb amputation (9–13), clinical data show individuals with T1D and PAD are more likely to undergo limb amputations compared to those with T2D (9). Moreover, the cohort with T1D and PAD had amputations at a younger age than those with T2D and PAD. Interestingly, although poor glycemic control has been associated with increased prevalence of PAD (14) and tight glycemic control has been shown to improve microvascular and macrovascular outcomes in type 1 and type 2 DM (15) it is unclear whether glycemic control improves PAD outcomes in individuals with advanced disease such as CTLI. In a mouse model of PAD and DM, glycemic control does improve PAD outcomes (6). These findings are consistent with hyperglycemia in diabetes being a major driver of the worse PAD outcomes in diabetes. However, lack of insulin or duration of hyperglycemia and resulting metabolic consequences of diabetes may also contribute to the observed severity of PAD among individuals with diabetes. To understand the impact of diabetes on the outcomes of PAD, it would be important to first understand the molecular mechanisms and genetic factors driving normal post-ischemic adaptations. Investigation of the impact of diabetes and its associated metabolic abnormalities on the identified molecular mechanisms and genetic factors will likely provide deeper insight into how diabetes exacerbates the outcomes of PAD.
In preclinical studies, several different models of T1D and T2D have been described each with its strengths and weaknesses, modeling different aspects of the human disease. A review of these models is beyond the scope of this review and has been discussed elsewhere (16–18). It is however possible to simulate aspects of PAD in T1D and T2D using well-established mouse models such as Akita mice, (simulates effect of hyperglycemia), high fat diet feeding (HFD, simulated effect of insulin resistance) or a combination of HFD and streptozotocin, STZ, (simulates effect of both insulin resistance and hyperglycemia), in conjunction with hind limb ischemia (HLI) surgery. Although DM is a complex disease with multiple clinical variables, hyperglycemia, insulin resistance, and associated metabolic disorders are common threads that have been studied in preclinical research to gain deeper insight into cardiovascular diseases including PAD. The mouse model, with rich resources of genetic tools, provides opportunities to understand aspects of the molecular, cellular and structural bases of the effects of diabetes on PAD.
Adaptations associated with PAD
Following vessel occlusion or narrowing in PAD, the poor perfusion leads to reduced oxygen and nutrients to the limb tissues. This results in inability to meet the metabolic demand of the affected tissue and may result in tissue necrosis and loss of function. The main affected tissue in PAD are the lower extremities where the ischemic condition may result in the death of muscle and vascular cells. Nevertheless, following vessel occlusion three major adaptive processes appear to protect the affected limb from limbs loss and this includes: (1) development of collateral blood flow through arteriogenesis, (2) growth of new blood vessels from existing vessels through angiogenesis and (3) skeletal muscle survival and repair processes.
The impact of diabetes on vascular adaptation in PAD
One of the key early compensatory responses following vessel occlusion is arteriogenesis whereby remodeling of existing arteries results from shear stress. This process is extensively described in a number of reviews (19–22). Vascular remodeling in arteriogenesis involves nitric oxide, recruitment of monocytes, extracellular matrix remodeling, growth factor and chemokine mediated alteration of existing vessels (23–25). Arteriogenesis may be followed by angiogenesis that entails formation of new capillaries in response to hypoxia-related factors and may involve homing of bone marrow derived progenitor cells in the ischemic tissue (26–28). This process involves the action of cytokines and growth factors including vascular endothelial growth factor (VEGF), fibroblast growth factor-1 (aFGF), hepatocyte growth factor (HGF), hypoxia-inducible factor-1a (HIF-1 a), developmental endothelial locus-1 (Del-1), and stroll cell-derived factor-1 (SDF-1) that are pro-angiogenic (29–31).
There is evidence that diabetes impairs arteriogenesis, but the molecular mechanisms involved are poorly understood (32, 33). The metabolic abnormalities associated with diabetes may affect many of the known processes involved in arteriogenesis. One of the first steps in arteriogenesis is the sensing of shear stress and has been shown to be a major trigger for the process. In diabetes shear stress sensing appear to be impaired but the mechanism has not been well described, however a possible role of production of advance glycation end products has been suggested (33–35). Moreover, another essential factor in collateral formation is endothelial nitric oxide synthase (eNOS) and its expression is increased significantly in collateral vessels during arteriogenesis. eNOS mediated NO release has been shown to be impaired in a mouse model of type 1 DM (STZ) and type 2 DM (Leprdb/db mice) (36–38).
Diabetes has profound negative effects on ischemic neovascularization of the limb in PAD (33, 39–42). However, the molecular mechanisms of these effects are not well understood. Clearly, the low-grade proinflammatory changes and increased reactive oxygen species (ROS) from hyperglycemia must contribute to this disarray, but additional mechanisms must also be involved. In streptozotocin (STZ)-induced diabetes of NOX2−/− mice, post-HLI vascularization was improved, suggesting a role of oxidative stress in impaired post ischemic angiogenesis in diabetes (43). One of the best investigated aspects of impaired angiogenesis in diabetes is the impact of diabetes on VEGF ligand/receptor expression. The role of VEGF and its receptors as critical signaling pathways in post-ischemic angiogenesis is well established (44–47). In mouse models of type 1 and type 2 diabetes investigators have shown impaired expression of VEGFA protein and mRNA in ischemic mouse hind limbs following experimental induction of PAD (6, 40, 48, 49). Thangarajah et al. showed this was due at least in part to decreased HIF-1alpha activity resulting from impaired HIF-1alpha binding to the coactivator p300 (50). Moreover, Ebrahimian et al. showed normal VEGFA expression was restored in ischemic diabetic limbs by inhibition of ROS formation or by ROS scavenging by the anti-oxidant N-acetyl-l-cysteine (NAC) (43) suggesting impaired VEGF expression was driven by ROS. Studies by our group evaluated the effect of diabetes on expression of VEGF receptors (VEGFR1 and VEGFR2). We found increased expression of soluble VEGFR1 thought to be an inhibitor of VEGFA signaling in ischemic diabetic limbs (40). Additionally, our analysis of VEGFR2 the primary receptor for VEGFA signaling showed decreased expression is ischemic diabetic skeletal muscle. We further demonstrated that this was due to hyperglycemia mediated increased ubiquitination and degradation of the receptor (6). Taken together these studies demonstrate how metabolic changes in diabetes can alter expression and signaling pathways that play important roles in post-ischemic adaptation (Figure 1).
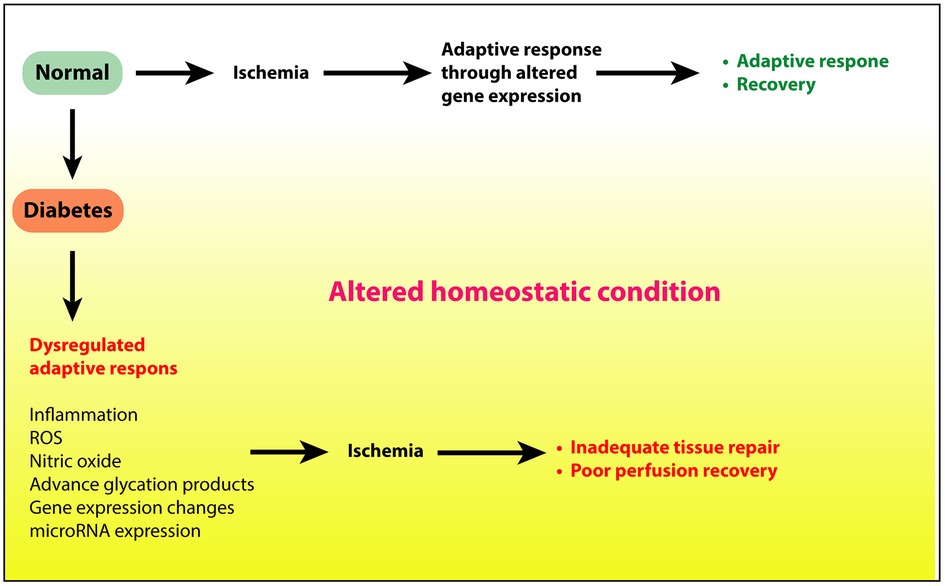
Figure 1. Ischemic injury to limbs under normal conditions elicits adequate adaptive response to restore tissue health and perfusion. However, under diabetic conditions, hyperglycemia, insulin resistance, or a combination of these, alters the tissue homeostatsis through increased inflammation, reactive oxygen species, and other proinflammatory processes. This altered state in turn delays or prevents the adaptive response to repair the tissue.
Beyond hyperglycemia, formation of AGEs and ROS activation of protein kinase C (PKC) is another well described finding in diabetic tissues (51, 52). Studies from our lab have shown that persistent hyperglycemia results in impairment of the canonical NF-kB pathway but promotes the non-canonical pathway. This misbalance in NF-kB pathway results from increased PKCb-S664 phosphorylation. Accordingly, in diabetic mice (T1DM, Akita mice), inhibition of PKCb activity by ruboxistaurin improved blood perfusion after hind limb ischemia (51).
The effects of limb ischemia are not limited to the vasculature; the ability of musculature to withstand the ischemic assault and recover from it is also important but remains a less studied area. It is conceivable that without improving the viability and function of the skeletal muscles attempts at revascularization may not suffice for limb preservation in PAD.
Ischemic limb skeletal muscle effects
Whether the evolution of limb ischemia is chronic or acute, the resulting loss of blood flow adversely affects the perfused tissue. Whereas in chronic ischemia collaterals may be formed as an adaptation to repeated near ischemic episodes, embolic acute ischemia causes more severe damage to the tissue that, if not treated, may lead to infarction and loss of limb. Although several limb muscle groups are affected by ischemia in HLI including the gastrocnemius (GA), the tibialis anterior (TA) and solius (53, 54), owing to its size and volume, the gastrocnemius muscle in mouse model is one of the most studied muscle that is affected by PAD showing both structural changes in the form of denervation, cell death (55), decreased muscle mass, smaller fiber size, fatty deposition, increased number of muscle fibers with central nuclei and biochemical changes (56–58). Centralized nuclei in muscle fibers were originally shown to be in myopathy (59, 60) and also during the repair process in injured muscle. Indeed, a muscle injury entails infiltration of the the tissue by immune cells. However, the size of these cells is usually much smaller than muscle fibers in the cross-sections. Thus, in the absence of a central-nuclear myopathy (CNM), centralized nuclei in muscle fibers are widely considered to be a reflection of the process of repair (61). Moreover, the GA muscle in PAD also show greater mitochondrial impairment, impaired oxidative metabolism, increased reactive oxygen species, and increased fibrosis (58, 62, 63).
Similar to what has been observed in humans, histopathological changes have also been observed in mouse models of PAD (59, 60). The use of mouse models and ex vivo studies have provided the tools to better understand the effects of ischemia on the skeletal muscle. The alignment of nuclei in the center of myofibers is a diagnostic feature of centronuclear myopathy, however, in the context of injury, it is a transitionary process of re-localization during repair Accordingly, we also have observed that centralized nuclei in the cross sections of myofibers of ischemic limbs of DM mice persist much longer and are present in higher numbers of fibers than in sections from control non-diabetic ischemic limbs (64).
Effect of diabetes on ischemic limb skeletal muscle
Reduced blood flow and intermittent ischemia adversely affect the metabolism of the lower limb musculature causing structural damage to the calf muscle area by reducing muscles mass and increased fatty deposits and fibrosis (55). In addition, decreased perfusion leads to impaired mitochondrial function and development of smaller muscle fibers (65). In diabetic patients with PAD, insulin resistance and hyperglycemia may result in adverse metabolic changes in the skeletal muscles causing greater damage and loss of recovery from injury. The metabolic abnormalities in diabetes may also hamper skeletal muscle repair. However, the role of diabetes on skeletal muscle recovery from ischemic injury is relatively less studied. A study in diabetic db/db mice suggests that factors produced in myofibers act upon endothelial cells to modulate their gene expression and function (66). In high fat diet-fed mice with impaired glucose tolerance and HLI surgery, exogenous expression of hepatocyte growth factor and vascular endothelial growth factor 165 improved muscle regeneration by improving innervation and muscle regeneration (67). Studies in our laboratory have shown that following HLI the extent of skeletal muscle injury is more severe in diabetic mice (HFD) compared to non-diabetic and this was associated with a higher rates of limb amputation and poor skeletal muscle function (64). These poorer outcomes may be at least in part due to impaired upregulation of genes important to skeletal muscle adaptation to ischemia (68).
Effects of diabetes on molecular adaptations in ischemia
In accordance with the ischemic injury, post-ischemic tissue shows marked changes in gene expression. Using microarray-based global gene expression analyses, we have shown that in both type 1 diabetes (Akita mice) and in high fat diet fed type 2 diabetes models there are significantly differences in gene expression in ischemic GA's when compared to expression in non-diabetic mice (69, 70). In both cases, significant changes were observed in the expression of genes involved in metabolic pathways, underlining the role of diabetes on metabolism under ischemic conditions. However, diabetic condition itself alters gene expression (71–73). Diabetic hyperglycemia affects multiple cellular and molecular processes through production of advance glycation end products (AGEs), imbalance in nitric oxide levels, and reactive oxygen species (ROS) through the NF-kB pathways (51, 74–78). Thus, hyperglycemia induces low grade chronic inflammation leading to an altered homeostatic state. In contrast to the non-diabetic state, in diabetes the adaptive response to ischemic injury commences from an altered state, which may not follow the response of the normal state.
microRNAs in diabetic PAD
MicroRNAs are short RNAs (approximately 22 nucleotides in length) that bind to near complementary seed sequences to their target mRNAs to repress their expression. Although miRNAs primarily target sequences in the 3’UTRs of mRNA, these targets may exist in the coding sequence or in 5’-UTRs. As a large collection of more than 2,000 identified miRNAs in humans, they can potentially regulate one-third of all genes in the human genome. Therefore, it is not surprising that miRNAs would also affect gene expression in PAD. Although a number of studies have looked at the role of miRNAs in PAD as biomarkers or for their regulatory potential for post-ischemic outcomes (79–85), relatively few studies have examined their role in regulating the outcome of PAD in diabetes (64, 86, 87).
T2DM itself can alter the expression of miRNAs. A meta-analysis of 39 case-control studies showed that compared to non-diabetic controls, 494 miRNAs were identified to have differential expression in T2DM patients (88). An interesting feature of studying miRNA is that by targeting a transcription factor they may affect a nodal point and related pathways regulated by the transcription factor (86, 89). In this vein, Feinberg and colleagues (90) identified endothelium-specific miR-375 whose expression was upregulated in the gastrocnemius muscle of non-diabetic mice (db/+) after hind limb ischemia but not in db/db mice. Moreover, miR-375 targets pro-angiogenic pathways through KLF5 transcription factor that is known to play a role in regulation of angiogenesis and skeletal muscle repair. In another study, Caporali et al. showed that expression of miR-503 was upregulated in endothelial cells both in vitro in simulated hyperglycemia or ischemia and in vivo in ischemic limbs of mice and in PAD patients (89). Inhibition of miR-503 in ischemic limbs of mice improved angiogenesis and blood flow recovery. Mechanistically, miR-503 expression is induced by NF-kB transcription factor and in turn regulates interaction between endothelial cells and pericytes to affect angiogenesis (86).
In our own studies, we have demonstrated that miR-29a influences the recovery from hind limb ischemia in diabetic mice by targeting ADAM12, an extracellular proteolytic enzyme that plays a role in extracellular matrix remodeling and post ischemic angiogenesis. In non-diabetic mice, the expression of miR-29a is significantly decreased after HLI, whereas in diabetic mice, its expression is increased. Using bioinformatics tools, we identified ADAM12 to be one of the targets of miR-29a. The expression of ADAM12 is increased in post-HLI ischemic limbs of non-diabetic mice, which would be expected if miR-29a targets ADAM12 transcript to repress its expression. On the other hand, in diabetic mice, the post-HLI expression of ADAM12 was significantly lower than the non-diabetic mice. This is in accord with the higher expression of miR-29a in these tissues. Interestingly, inhibition of miR-29a in ischemic limbs of diabetic mice by antagomir, increased ADAM12 expression and improved the post-ischemic tissue repair and blood perfusion. Moreover, we have identified several other miRNAs in these experiments whose expression is modulated in the ischemic limbs of diabetic mice (unpublished results). These results strongly suggest that altered miRNA expression in ischemic diabetic tissues are likely potent contributors to poor PAD outcomes in diabetes. Identifying the target of miRNAs with altered expression in ischemic diabetic tissues may provide novel insights into the molecular mechanisms by with diabetes contributes to poor PAD outcomes. It will be interesting to know whether these miRNAs act in concert with one another to affect the genes involved in the same pathway or they target genes involved in additional pathways.
To this end, a combination of miRNA expression by global miRNA sequencing (miRseq) and mRNA sequencing (RNAseq) or mRNA array analyses will be highly informative in identifying differences in miRNA and their target genes that are critical in diabetes-induced pathology. Using such approach endothelial-enriched miRNAs miR-615-5p was identified to be downregulated under pro-angiogenic conditions and upregulated in wounds including in diabetic mice (db/db mice). Inhibition of miR-615-5p by synthetic antagomir improved blood perfusion and angiogenesis after surgical hind limb ischemia in diabetic db/db mice (91).
In addition to identifying the miRNAs that shape the outcomes of diabetic ischemic limb injury, it is also important to understand the regulation of expression of these miRNAs. A number of miRNAs are embedded in protein-coding genes and their expression is closely synchronized with the host genes. However, miRNAs also exist independently in the genome, and little is known of the regulation of their expression. In our studies, the miR-29a location has been known to exist independently. The information on its expression and regulatory cis-elements or transcriptional factors is not known. It will be important to understand how the expression of this miRNA is regulated under ischemic conditions and how the expression is influenced by the metabolic abnormalities in diabetes leading to altered expression of its target genes.
Genetic mapping and role of limb salvage QTL-1 (LSQ-1) genes on diabetic PAD outcomes
Our laboratory's interest has been to understand the molecular mechanisms of recovery from ischemic limb injury in PAD, and how diabetes adversely affects the outcomes of post-ischemic recovery. Using a well-established mouse hind limb ischemia (HLI) model of PAD, we have taken a genetic mapping approach to identify genes that have major impact on post-ischemic recovery, explore their mechanism of action followed by investigations of the effect of diabetes on the expression and function of these genes. We identified a genetic locus on C57Bl/6 mouse chromosome 7 termed LSQ-1 that determines good outcomes following ischemic limb injury (92). This approach led us to map a variant Bag3 gene that functions as a key co-chaperone in autophagy, and also an anti-apoptotic protein (93). We have shown that BAG3 may be a key determinant of post-ischemic recovery in mouse HLI. In diabetic mice (high fat diet), we observed high rates of limb loss compared to non-diabetic mice, likely due to poorer perfusion, lesser degree of angiogenesis, and a more severe post ischemic skeletal muscle injury. These findings suggest that despite the adequate post-HLI adaptation and recovery of C57BL/6 mice, high fat diet or hyperglycemia (HFD + STZ, or Akita mice) alters the expression of a key molecule involved in post-injury repair of limb tissue. Interestingly the ischemic hind limb skeletal muscles of diabetic C57BL/6J mice showed significantly lower expression of two genes mapped on Lsq-1 locus, i.e., BAG3 and ADAM12, compared to that in non-diabetic mouse limbs (64, 68). Exogenous expression of BAG3 in the limbs of diabetic mice prior to HLI significantly reduced limb loss, improved perfusion recovery and decreased extent of skeletal muscle injury (68). The repression of gene expression in diabetes could result from either transcriptional regulation or from post-transcriptional regulatory mechanisms, such as microRNAs. At least in the case of ADAM12, we have identified a regulatory mechanism whereby increased expression of miRNA-29a in post-HLI limb tissue results in decreased ADAM12 expression. In contrast, neutralization of miR-29a by exogenous antagomir prevents the loss of ADAM12 in post-HLI limbs and improves perfusion recovery and tissue damage (64). In the future studies, it will be important to understand these mechanisms that may identify transcription factors, Bag3 promoter regulatory sequences, and specific miRNAs of potential therapeutic use. It also remains to be determined whether poor post-HLI recovery resulting from BAG3 deficiency is cell type specific. Genetically modified mice to either knockout Bag3 in cell type-specific manner or to selectively express BAG3 protein in specific cell types is likely to be very informative.
Conclusion
While it is well established that diabetes contributes to the development and progression of arteriosclerosis, the primary cause of vessel occlusion in PAD, the effect of diabetes on PAD goes beyond development of the disease. Diabetes is a major driver of disease severity likely through its effect on cellular and molecular processes involved in vascular and skeletal muscle adaption to ischemia. The precise mechanism of how diabetes has this profound effect on PAD outcomes is poorly understood and remain an area of great need for investigation.
Author contributions
Conceptualization, AD; literature search and analysis, MS, writing—original draft preparation MS and AD; writing—revision, review and editing, MS and AD. All authors contributed to the article and approved the submitted version.
Funding
This research was funded by National Heart, Lung, and Blood Institute (R01 HL130399) to AD.
Conflict of interest
The authors declare that the research was conducted in the absence of any commercial or financial relationships that could be construed as a potential conflict of interest.
Publisher's note
All claims expressed in this article are solely those of the authors and do not necessarily represent those of their affiliated organizations, or those of the publisher, the editors and the reviewers. Any product that may be evaluated in this article, or claim that may be made by its manufacturer, is not guaranteed or endorsed by the publisher.
References
1. Fowkes FGR, Rudan D, Rudan I, Aboyans V, Denenberg JO, McDermott MM, et al. Comparison of global estimates of prevalence and risk factors for peripheral artery disease in 2000 and 2010: a systematic review and analysis. Lancet. (2013) 382:1329–40. doi: 10.1016/S0140-6736(13)61249-0
2. Conte MS, Bradbury AW, Kolh P, White JV, Dick F, Fitridge R, et al. Global vascular guidelines on the management of chronic limb-threatening ischemia. J Vasc Surg. (2019) 69:3S–125S.e40. doi: 10.1016/j.jvs.2019.02.016
3. Criqui MH, Aboyans V. Epidemiology of peripheral artery disease. Circ Res. (2015) 116:1509–26. doi: 10.1161/CIRCRESAHA.116.303849
4. Fox CS, Golden SH, Anderson C, Bray GA, Burke LE, de Boer IH, et al. Update on prevention of cardiovascular disease in adults with type 2 diabetes mellitus in light of recent evidence: a scientific statement from the American heart association and the American diabetes association. Diabetes Care. (2015) 38:1777–803. doi: 10.2337/dci15-0012
5. Wild S, Roglic G, Green A, Sicree R, King H. Global prevalence of diabetes: estimates for the year 2000 and projections for 2030. Diabetes Care. (2004) 27:1047–53. doi: 10.2337/diacare.27.5.1047
6. Dokun AO, Chen L, Lanjewar SS, Lye RJ, Annex BH. Glycaemic control improves perfusion recovery and VEGFR2 protein expression in diabetic mice following experimental PAD. Cardiovasc Res. (2014) 101:364–72. doi: 10.1093/cvr/cvt342
7. Fadini GP, Spinetti G, Santopaolo M, Madeddu P. Impaired regeneration contributes to poor outcomes in diabetic peripheral artery disease. Arterioscler Thromb Vasc Biol. (2020) 40:34–44. doi: 10.1161/ATVBAHA.119.312863
8. Fitridge R, Pena G, Mills JL. The patient presenting with chronic limb-threatening ischaemia. Does diabetes influence presentation, limb outcomes and survival? Diabetes Metab Res Rev. (2020) 36(Suppl 1):e3242. doi: 10.1002/dmrr.3242
9. Jain N, Agarwal MA, Jalal D, Dokun AO. Individuals with peripheral artery disease (PAD) and type 1 diabetes are more likely to undergo limb amputation than those with PAD and type 2 diabetes. J Clin Med. (2020) 9(9):2809. doi: 10.3390/jcm9092809
10. Trautner C, Haastert B, Giani G, Berger M. Incidence of lower limb amputations and diabetes. Diabetes Care. (1996) 19:1006–9. doi: 10.2337/diacare.19.9.1006
11. Siitonen OI, Niskanen LK, Laakso M, Siitonen JT, Pyorala K. Lower-extremity amputations in diabetic and nondiabetic patients. A population-based study in eastern Finland. Diabetes Care. (1993) 16:16–20. doi: 10.2337/diacare.16.1.16
12. Pecoraro RE, Reiber GE, Burgess EM. Pathways to diabetic limb amputation. Basis for prevention. Diabetes Care. (1990) 13:513–21. doi: 10.2337/diacare.13.5.513
13. Beckman JA, Schneider PA, Conte MS. Advances in revascularization for peripheral artery disease: revascularization in PAD. Circ Res. (2021) 128:1885–912. doi: 10.1161/CIRCRESAHA.121.318261
14. Singh S, Armstrong EJ, Sherif W, Alvandi B, Westin GG, Singh GD, et al. Association of elevated fasting glucose with lower patency and increased major adverse limb events among patients with diabetes undergoing infrapopliteal balloon angioplasty. Vasc Med. (2014) 19:307–14. doi: 10.1177/1358863X14538330
15. Dokun AO. Lessons learned from glycemia control studies. Curr Diab Rep. (2010) 10:133–8. doi: 10.1007/s11892-010-0094-6
16. King A, Bowe J. Animal models for diabetes: understanding the pathogenesis and finding new treatments. Biochem Pharmacol. (2016) 99:1–10. doi: 10.1016/j.bcp.2015.08.108
17. Kleinert M, Clemmensen C, Hofmann SM, Moore MC, Renner S, Woods SC, et al. Animal models of obesity and diabetes mellitus. Nat Rev Endocrinol. (2018) 14:140–62. doi: 10.1038/nrendo.2017.161
18. Kottaisamy CPD, Raj DS, Prasanth Kumar V, Sankaran U. Experimental animal models for diabetes and its related complications-a review. Lab Anim Res. (2021) 37:23. doi: 10.1186/s42826-021-00101-4
19. Annex BH, Cooke JP. New directions in therapeutic angiogenesis and arteriogenesis in peripheral arterial disease. Circ Res. (2021) 128:1944–57. doi: 10.1161/CIRCRESAHA.121.318266
20. Buschmann I, Schaper W. Arteriogenesis versus angiogenesis: two mechanisms of vessel growth. News Physiol Sci. (1999) 14:121–5. doi: 10.1152/physiologyonline.1999.14.3.121
21. Deindl E, Quax PHA. Arteriogenesis and therapeutic angiogenesis in its multiple aspects. Cells. (2020) 9(6):1439. doi: 10.3390/cells9061439
22. van Royen N, Piek JJ, Schaper W, Fulton WF. A critical review of clinical arteriogenesis research. J Am Coll Cardiol. (2009) 55:17–25. doi: 10.1016/j.jacc.2009.06.058
23. Carmeliet P. Mechanisms of angiogenesis and arteriogenesis. Nat Med. (2000) 6:389–95. doi: 10.1038/74651
24. Gatzke N, Hillmeister P, Dulsner A, Guc N, Dawid R, Smith KH, et al. Nitroglycerin application and coronary arteriogenesis. PLoS One. (2018) 13:e0201597. doi: 10.1371/journal.pone.0201597
25. Kulkarni R, Andraska E, McEnaney R. Structural remodeling of the extracellular matrix in arteriogenesis: a review. Front Cardiovasc Med. (2021) 8:761007. doi: 10.3389/fcvm.2021.761007
26. Golledge J, Van Campenhout A, Pal S, Rush C. Bone marrow-derived cells and arterial disease. J Vasc Surg. (2007) 46:590–600. doi: 10.1016/j.jvs.2007.04.031
27. Meisner JK, Price RJ. Spatial and temporal coordination of bone marrow-derived cell activity during arteriogenesis: regulation of the endogenous response and therapeutic implications. Microcirculation. (2010) 17:583–99. doi: 10.1111/j.1549-8719.2010.00051.x
28. Tongers J, Roncalli JG, Losordo DW. Role of endothelial progenitor cells during ischemia-induced vasculogenesis and collateral formation. Microvasc Res. (2010) 79:200–6. doi: 10.1016/j.mvr.2010.01.012
29. Forster R, Liew A, Bhattacharya V, Shaw J, Stansby G. Gene therapy for peripheral arterial disease. Cochrane Database Syst Rev. (2018) 10:CD012058. doi: 10.1002/14651858.CD012058.pub2
30. Han SW, Vergani CA, Reis PEO. Is gene therapy for limb ischemia a reality? J Vasc Bras. (2020) 19:e20190059. doi: 10.1590/1677-5449.190059
31. Ganta VC, Annex BH. Peripheral vascular disease: preclinical models and emerging therapeutic targeting of the vascular endothelial growth factor ligand-receptor system. Expert Opin Ther Targets. (2021) 25:381–91. doi: 10.1080/14728222.2021.1940139
32. Kolluru GK, Bir SC, Kevil CG. Endothelial dysfunction and diabetes: effects on angiogenesis, vascular remodeling, and wound healing. Int J Vasc Med. (2012) 2012:918267. doi: 10.1155/2012/918267
33. Ruiter MS, van Golde JM, Schaper NC, Stehouwer CD, Huijberts MS. Diabetes impairs arteriogenesis in the peripheral circulation: review of molecular mechanisms. Clin Sci. (2010) 119:225–38. doi: 10.1042/CS20100082
34. Schiekofer S, Galasso G, Sato K, Kraus BJ, Walsh K. Impaired revascularization in a mouse model of type 2 diabetes is associated with dysregulation of a complex angiogenic-regulatory network. Arterioscler Thromb Vasc Biol. (2005) 25:1603–9. doi: 10.1161/01.ATV.0000171994.89106.ca
35. Yan J, Tie G, Park B, Yan Y, Nowicki PT, Messina LM. Recovery from hind limb ischemia is less effective in type 2 than in type 1 diabetic mice: roles of endothelial nitric oxide synthase and endothelial progenitor cells. J Vasc Surg. (2009) 50:1412–22. doi: 10.1016/j.jvs.2009.08.007
36. Becerril S, Rodriguez A, Catalan V, Ramirez B, Unamuno X, Portincasa P, et al. Functional relationship between leptin and nitric oxide in metabolism. Nutrients. (2019) 11(9):2129. doi: 10.3390/nu11092129
37. Mohan S, Reddick RL, Musi N, Horn DA, Yan B, Prihoda TJ, et al. Diabetic eNOS knockout mice develop distinct macro- and microvascular complications. Lab Invest. (2008) 88:515–28. doi: 10.1038/labinvest.2008.23
38. Namba T, Koike H, Murakami K, Aoki M, Makino H, Hashiya N, et al. Angiogenesis induced by endothelial nitric oxide synthase gene through vascular endothelial growth factor expression in a rat hindlimb ischemia model. Circulation. (2003) 108:2250–7. doi: 10.1161/01.CIR.0000093190.53478.78
39. Costa PZ, Soares R. Neovascularization in diabetes and its complications. Unraveling the angiogenic paradox. Life Sci. (2013) 92:1037–45. doi: 10.1016/j.lfs.2013.04.001
40. Hazarika S, Dokun AO, Li Y, Popel AS, Kontos CD, Annex BH. Impaired angiogenesis after hindlimb ischemia in type 2 diabetes mellitus: differential regulation of vascular endothelial growth factor receptor 1 and soluble vascular endothelial growth factor receptor 1. Circ Res. (2007) 101:948–56. doi: 10.1161/CIRCRESAHA.107.160630
41. Li Y, Hazarika S, Xie D, Pippen AM, Kontos CD, Annex BH. In mice with type 2 diabetes, a vascular endothelial growth factor (VEGF)-activating transcription factor modulates VEGF signaling and induces therapeutic angiogenesis after hindlimb ischemia. Diabetes. (2007) 56:656–65. doi: 10.2337/db06-0999
42. Tamarat R, Silvestre J-SB, Huijberts M, Benessiano J, Ebrahimian TG, Duriez M, et al. Blockade of advanced glycation end-product formation restores ischemia-induced angiogenesis in diabetic mice. Proc Natl Acad Sci USA. (2003) 100:8555–60. doi: 10.1073/pnas.1236929100
43. Ebrahimian TG, Heymes C, You D, Blanc-Brude O, Mees B, Waeckel L, et al. NADPH oxidase-derived overproduction of reactive oxygen species impairs postischemic neovascularization in mice with type 1 diabetes. Am J Pathol. (2006) 169:719–28. doi: 10.2353/ajpath.2006.060042
44. Ahmad A, Nawaz MI. Molecular mechanism of VEGF and its role in pathological angiogenesis. J Cell Biochem. (2022) 123:1938–65. doi: 10.1002/jcb.30344
45. Ferrara N. Vascular endothelial growth factor: basic science and clinical progress. Endocr Rev. (2004) 25:581–611. doi: 10.1210/er.2003-0027
46. Hoeben A, Landuyt B, Highley MS, Wildiers H, Van Oosterom AT, De Bruijn EA. Vascular endothelial growth factor and angiogenesis. Pharmacol Rev. (2004) 56:549–80. doi: 10.1124/pr.56.4.3
47. Melincovici CS, Bosca AB, Susman S, Marginean M, Mihu C, Istrate M, et al. Vascular endothelial growth factor (VEGF) - key factor in normal and pathological angiogenesis. Rom J Morphol Embryol. (2018) 59:455–67.30173249
48. Rivard A, Silver M, Chen D, Kearney M, Magner M, Annex B, et al. Rescue of diabetes-related impairment of angiogenesis by intramuscular gene therapy with adeno-VEGF. Am J Pathol. (1999) 154:355–63. doi: 10.1016/S0002-9440(10)65282-0
49. Wang K, Dai X, He J, Yan X, Yang C, Fan X, et al. Endothelial overexpression of metallothionein prevents diabetes-induced impairment in ischemia angiogenesis through preservation of HIF-1alpha/SDF-1/VEGF signaling in endothelial progenitor cells. Diabetes. (2020) 69:1779–92. doi: 10.2337/db19-0829
50. Thangarajah H, Yao D, Chang EI, Shi Y, Jazayeri L, Vial IN, et al. The molecular basis for impaired hypoxia-induced VEGF expression in diabetic tissues. Proc Natl Acad Sci USA. (2009) 106:13505–10. doi: 10.1073/pnas.0906670106
51. Alleboina S, Wong T, Singh MV, Dokun AO. Feature article: inhibition of protein kinase C beta phosphorylation activates nuclear factor-kappa B and improves postischemic recovery in type 1 diabetes. Exp Biol Med. (2020) 245:785–96. doi: 10.1177/1535370220920832
52. Inoguchi T, Li P, Umeda F, Yu HY, Kakimoto M, Imamura M, et al. High glucose level and free fatty acid stimulate reactive oxygen species production through protein kinase C–dependent activation of NAD(P)H oxidase in cultured vascular cells. Diabetes. (2000) 49:1939–45. doi: 10.2337/diabetes.49.11.1939
53. Goldberg EJ, Schmidt CA, Green TD, Karnekar R, Yamaguchi DJ, Spangenberg EE, et al. Temporal association between ischemic muscle perfusion recovery and the restoration of muscle contractile function after hindlimb ischemia. Front Physiol. (2019) 10:804. doi: 10.3389/fphys.2019.00804
54. Schmidt CA, Amorese AJ, Ryan TE, Goldberg EJ, Tarpey MD, Green TD, et al. Strain-dependent variation in acute ischemic muscle injury. Am J Pathol. (2018) 188:1246–62. doi: 10.1016/j.ajpath.2018.01.008
55. McDermott MM, Ferrucci L, Gonzalez-Freire M, Kosmac K, Leeuwenburgh C, Peterson CA, et al. Skeletal muscle pathology in peripheral artery disease. Arterioscler Thromb Vasc Biol. (2020) 40:2577–85. doi: 10.1161/ATVBAHA.120.313831
56. Pipinos II, Sharov VG, Shepard AD, Anagnostopoulos PV, Katsamouris A, Todor A, et al. Abnormal mitochondrial respiration in skeletal muscle in patients with peripheral arterial disease. J Vasc Surg. (2003) 38:827–32. doi: 10.1016/S0741-5214(03)00602-5
57. Weiss DJ, Casale GP, Koutakis P, Nella AA, Swanson SA, Zhu Z, et al. Oxidative damage and myofiber degeneration in the gastrocnemius of patients with peripheral arterial disease. J Transl Med. (2013) 11:230. doi: 10.1186/1479-5876-11-230
58. McDermott MM, Dayanidhi S, Kosmac K, Saini S, Slysz J, Leeuwenburgh C, et al. Walking exercise therapy effects on lower extremity skeletal muscle in peripheral artery disease. Circ Res. (2021) 128:1851–67. doi: 10.1161/CIRCRESAHA.121.318242
59. Mohiuddin M, Lee NH, Moon JY, Han WM, Anderson SE, Choi JJ, et al. Critical limb ischemia induces remodeling of skeletal muscle motor unit, myonuclear-, and mitochondrial-domains. Sci Rep. (2019) 9:9551. doi: 10.1038/s41598-019-45923-4
60. Roman W, Pinheiro H, Pimentel MR, Segales J, Oliveira LM, Garcia-Dominguez E, et al. Muscle repair after physiological damage relies on nuclear migration for cellular reconstruction. Science. (2021) 374:355–9. doi: 10.1126/science.abe5620
61. Folker ES, Baylies MK. Nuclear positioning in muscle development and disease. Front Physiol. (2013) 4:363. doi: 10.3389/fphys.2013.00363
62. Ha DM, Carpenter LC, Koutakis P, Swanson SA, Zhu Z, Hanna M, et al. Transforming growth factor-beta 1 produced by vascular smooth muscle cells predicts fibrosis in the gastrocnemius of patients with peripheral artery disease. J Transl Med. (2016) 14:39. doi: 10.1186/s12967-016-0790-3
63. Hiatt WR, Wolfel EE, Regensteiner JG, Brass EP. Skeletal muscle carnitine metabolism in patients with unilateral peripheral arterial disease. J Appl Physiol. (1992) 73:346–53. doi: 10.1152/jappl.1992.73.1.346
64. Lamin V, Verry J, Eigner-Bybee I, Fuqua JD, Wong T, Lira VA, et al. Modulation of miR-29a and ADAM12 reduces post-ischemic skeletal muscle injury and improves perfusion recovery and skeletal muscle function in a mouse model of type 2 diabetes and peripheral artery disease. Int J Mol Sci. (2021) 23(1):429. doi: 10.3390/ijms23010429
65. Ryan TE, Schmidt CA, Green TD, Spangenburg EE, Neufer PD, McClung JM. Targeted expression of catalase to mitochondria protects against ischemic myopathy in high-fat diet-fed mice. Diabetes. (2016) 65:2553–68. doi: 10.2337/db16-0387
66. Foussard N, Rouault P, Cornuault L, Reynaud A, Buys ES, Chapouly C, et al. Praliciguat promotes ischemic leg reperfusion on leptin receptor-deficient mice. Circ Res. (2022) 132(1):34–48. doi: 10.1161/CIRCRESAHA.122.322033
67. Stafeev II, Boldyreva MA, Michurina SS, Agareva MY, Radnaeva AV, Menshikov MY, et al. The efficacy of HGF/VEGF gene therapy for limb ischemia in mice with impaired glucose tolerance: shift from angiogenesis to axonal growth and oxidative potential in skeletal muscle. Cells. (2022) 11(23):3824. doi: 10.3390/cells11233824
68. Mani AM, Dhanabalan K, Lamin V, Wong T, Singh MV, Dokun AO. BAG3 attenuates ischemia-induced skeletal muscle necroptosis in diabetic experimental peripheral artery disease. Int J Mol Sci. (2022) 23(18):10715. doi: 10.3390/ijms231810715
69. Peravali R, Gunnels L, Alleboina S, Gerling IC, Dokun AO. Type 1 diabetes alters ischemia-induced gene expression. J Clin Transl Endocrinol. (2019) 15:19–24. doi: 10.1016/j.jcte.2018.11.003
70. Peravali R, Gunnels L, Dhanabalan K, Ariganjoye F, Gerling IC, Dokun AO. In experimental peripheral arterial disease, type 2 diabetes alters post-ischemic gene expression. J Clin Transl Endocrinol. (2019) 17:100199. doi: 10.1016/j.jcte.2019.100199
71. Moller AB, Kampmann U, Hedegaard J, Thorsen K, Nordentoft I, Vendelbo MH, et al. Altered gene expression and repressed markers of autophagy in skeletal muscle of insulin resistant patients with type 2 diabetes. Sci Rep. (2017) 7:43775. doi: 10.1038/srep43775
72. Palsgaard J, Brons C, Friedrichsen M, Dominguez H, Jensen M, Storgaard H, et al. Gene expression in skeletal muscle biopsies from people with type 2 diabetes and relatives: differential regulation of insulin signaling pathways. PLoS One. (2009) 4:e6575. doi: 10.1371/journal.pone.0006575
73. Willsky GR, Chi LH, Liang Y, Gaile DP, Hu Z, Crans DC. Diabetes-altered gene expression in rat skeletal muscle corrected by oral administration of vanadyl sulfate. Physiol Genomics. (2006) 26:192–201. doi: 10.1152/physiolgenomics.00196.2005
74. Asmat U, Abad K, Ismail K. Diabetes mellitus and oxidative stress-a concise review. Saudi Pharm J. (2016) 24:547–53. doi: 10.1016/j.jsps.2015.03.013
75. Burgos-Moron E, Abad-Jimenez Z, Maranon AM, Iannantuoni F, Escribano-Lopez I, Lopez-Domenech S, et al. Relationship between oxidative stress, ER stress, and inflammation in type 2 diabetes: the battle continues. J Clin Med. (2019) 8(9):1385. doi: 10.3390/jcm8091385
76. Lin KY, Ito A, Asagami T, Tsao PS, Adimoolam S, Kimoto M, et al. Impaired nitric oxide synthase pathway in diabetes mellitus: role of asymmetric dimethylarginine and dimethylarginine dimethylaminohydrolase. Circulation. (2002) 106:987–92. doi: 10.1161/01.CIR.0000027109.14149.67
77. Meyerovich K, Ortis F, Cardozo AK. The non-canonical NF-kappaB pathway and its contribution to beta-cell failure in diabetes. J Mol Endocrinol. (2018) 61:F1–6. doi: 10.1530/JME-16-0183
78. Yamamoto Y, Yamamoto H. RAGE-mediated inflammation, type 2 diabetes, and diabetic vascular complication. Front Endocrinol. (2013) 4:105. doi: 10.3389/fendo.2013.00105
79. Ganta VC, Choi MH, Kutateladze A, Fox TE, Farber CR, Annex BH. A MicroRNA93-interferon regulatory factor-9-immunoresponsive gene-1-itaconic acid pathway modulates M2-like macrophage polarization to revascularize ischemic muscle. Circulation. (2017) 135:2403–25. doi: 10.1161/CIRCULATIONAHA.116.025490
80. Hazarika S, Farber CR, Dokun AO, Pitsillides AN, Wang T, Lye RJ, et al. MicroRNA-93 controls perfusion recovery after hindlimb ischemia by modulating expression of multiple genes in the cell cycle pathway. Circulation. (2013) 127:1818–28. doi: 10.1161/CIRCULATIONAHA.112.000860
81. Hsu PY, Hsi E, Wang TM, Lin RT, Liao YC, Juo SH. MicroRNA let-7 g possesses a therapeutic potential for peripheral artery disease. J Cell Mol Med. (2017) 21:519–29. doi: 10.1111/jcmm.12997
82. Lee CY, Lin SJ, Wu TC. miR-548j-5p regulates angiogenesis in peripheral artery disease. Sci Rep. (2022) 12:838. doi: 10.1038/s41598-022-04770-6
83. Shu X, Mao Y, Li Z, Wang W, Chang Y, Liu S, et al. MicroRNA-93 regulates angiogenesis in peripheral arterial disease by targeting CDKN1A. Mol Med Rep. (2019) 19:5195–202. doi: 10.3892/mmr.2019.10196
84. Saenz-Pipaon G, Martinez-Aguilar E, Orbe J, Gonzalez Miqueo A, Fernandez-Alonso L, Paramo JA, et al. The role of circulating biomarkers in peripheral arterial disease. Int J Mol Sci. (2021) 22(7):3601. doi: 10.3390/ijms22073601
85. Wang T, Yang L, Yuan M, Farber CR, Spolski R, Leonard WJ, et al. MicroRNA-30b is both necessary and sufficient for interleukin-21 receptor-mediated angiogenesis in experimental peripheral arterial disease. Int J Mol Sci. (2021) 23(1):271. doi: 10.3390/ijms23010271
86. Caporali A, Meloni M, Nailor A, Mitić T, Shantikumar S, Riu F, et al. p75NTR-dependent activation of NF-κB regulates microRNA-503 transcription and pericyte–endothelial crosstalk in diabetes after limb ischaemia. Nat Commun. (2015) 6:8024. doi: 10.1038/ncomms9024
87. Chen L, Okeke E, Ayalew D, Wang D, Shahid L, Dokun AO. Modulation of miR29a improves impaired post-ischemic angiogenesis in hyperglycemia. Exp Biol Med. (2017) 242:1432–43. doi: 10.1177/1535370217716424
88. Liang YZ, Li JJ, Xiao HB, He Y, Zhang L, Yan YX. Identification of stress-related microRNA biomarkers in type 2 diabetes mellitus: a systematic review and meta-analysis. J Diabetes. (2020) 12:633–44. doi: 10.1111/1753-0407.12643
89. Caporali A, Meloni M, Vollenkle C, Bonci D, Sala-Newby GB, Addis R, et al. Deregulation of microRNA-503 contributes to diabetes mellitus-induced impairment of endothelial function and reparative angiogenesis after limb ischemia. Circulation. (2011) 123:282–91. doi: 10.1161/CIRCULATIONAHA.110.952325
90. McCoy MG, Jamaiyar A, Sausen G, Cheng HS, Pérez-Cremades D, Zhuang R, et al. MicroRNA-375 repression of kruppel-like factor 5 improves angiogenesis in diabetic critical limb ischemia. Angiogenesis. (2022) 26(1):107–27. doi: 10.1007/s10456-022-09856-3
91. Icli B, Wu W, Ozdemir D, Li H, Cheng HS, Haemmig S, et al. MicroRNA-615-5p regulates angiogenesis and tissue repair by targeting AKT/eNOS (protein kinase B/endothelial nitric oxide synthase) signaling in endothelial cells. Arterioscler Thromb Vasc Biol. (2019) 39:1458–74. doi: 10.1161/ATVBAHA.119.312726
92. Okeke E, Dokun AO. Role of genetics in peripheral arterial disease outcomes; significance of limb-salvage quantitative locus-1 genes. Exp Biol Med. (2018) 243:190–7. doi: 10.1177/1535370217743460
Keywords: peripheral artery disease, diabetes, ischemia, vascular, gene expression, microRNA, angiogenesis
Citation: Singh MV and Dokun AO (2023) Diabetes mellitus in peripheral artery disease: Beyond a risk factor. Front. Cardiovasc. Med. 10:1148040. doi: 10.3389/fcvm.2023.1148040
Received: 19 January 2023; Accepted: 22 March 2023;
Published: 17 April 2023.
Edited by:
Brian Annex, Augusta University, United StatesReviewed by:
Joseph M. McClung, East Carolina University, United States© 2023 Singh and Dokun. This is an open-access article distributed under the terms of the Creative Commons Attribution License (CC BY). The use, distribution or reproduction in other forums is permitted, provided the original author(s) and the copyright owner(s) are credited and that the original publication in this journal is cited, in accordance with accepted academic practice. No use, distribution or reproduction is permitted which does not comply with these terms.
*Correspondence: Ayotunde O. Dokun YXlvdHVuZGUtZG9rdW5AdWlvd2EuZWR1
Specialty Section: This article was submitted to Atherosclerosis and Vascular Medicine, a section of the journal Frontiers in Cardiovascular Medicine