- 1Laboratorio de Embriología Comparada, Programa de Biología Integrativa, Instituto de Ciencias Biomédicas, Facultad de Medicina, Universidad de Chile, Santiago, Chile
- 2Programa de Doctorado en Ciencias Morfológicas, Facultad de Medicina, Universidad de La Frontera, Temuco, Chile
- 3Escuela de Tecnología Médica, Facultad de Salud, Universidad Santo Tomás, Los Carrera, Osorno, Chile
- 4Departamento de Medicina Interna, Facultad de Medicina, Universidad de La Frontera, Temuco, Chile
- 5Laboratory of Morphometry, Metabolism and Cardiovascular Diseases, Biomedical Center, Institute of Biology, Universidade do Estado do Rio de Janeiro, Rio de Janeiro, Brazil
Introduction: Hypoxia is a recurring problem in the fish farming industry. Currently, it is known that the exposure of fish and fry to a hypoxic environment induces important changes in their metabolism, compromising not only their development but also their reproduction and mortality rates. Our hypothesis is that hypoxia constitutes one of the etiological factors causing deformation of the body and caudal fin in this species, as well as affecting its growth.
Methods: We analyzed two hundred forty Salmo salar salmon fry, differentially cultured at 100% saturation (normoxia condition) and 60% (hypoxia condition) for 2, 4, 6, and 8 days, including a group under continuous hypoxia. We performed diaphanization and Alcian blue staining, along with standard histological techniques. The polyclonal anti-HIF-1a antibody was used as a marker of hypoxia in Salmo salar, and hypoxia in these fish was associated with the immunopositivity of this antibody.
Results and discussion: The results indicate that there is an association between exposure to hypoxia and the deformation of the body and fin, as well as an agreement between hypoxia and the total length of the fry and fin. Several months after the event occurred, we were able to find and describe angiogenesis, blood vessel disorganization, and vasodilation histologically. Finally, hypoxic cells in the fry (HIF-1a) could be recognized and confirmed as hypoxia sensors. All of this indicates that hypoxia not only affects the fry during the development phase of the event, but that its results can be evident much later and affect the fry throughout their entire ontogeny.
1 Introduction
There are various factors capable of causing deformities in fish, including increases in temperature (Rojas et al., 2020), deficiency of nutrients such as phosphorus (Venegas et al., 2003), hypoxia (Shang and Wu, 2004; Zhan et al., 2023), as well as the presence of heavy metals (Sfakianakis et al., 2015) and wastewater discharges (Quesada et al., 2023). The effects of these factors can be devastating, given that they can compromise the development and physiology of fish, affecting, for example, the caudal fin as well as other structures (Rojas et al., 2020; Pellón et al., 2022), compromising swimming, escape efficiency, feeding, and/or generating susceptibility to bacterial and fungal infections (Böckelmann et al., 2010).
Hypoxia is one of the most important environmental stressors affecting animal welfare. During their early lives, most fish are sensitive to low levels of dissolved oxygen in the aquatic environment (hypoxia), a sensitivity that varies depending on the stage of development (Shang and Wu, 2004; Jonz and Nurse, 2006; Podrabsky et al., 2007). Research has widely demonstrated that hypoxia in fish slows down growth, triggers apoptosis, and reduces fry survival. Studies have also reported that sublethal levels of hypoxia can increase the appearance of malformations during fish embryonic development by more than 77% (Shang and Wu, 2004). In this context, it’s crucial to acknowledge the significance of certain markers such as neuroepithelial cells (NECs), which are oxygen-sensing cells and are located at the end of the primary epithelium in each gill arch (Dunel-Erb et al., 1982; Jonz and Nurse, 2003; Saltys et al., 2006; Zaccone et al., 2006; Reed and Jonz, 2016; Zaccone et al., 2017; Lauriano et al., 2021).
Juvenile and adult fish use a dermal skeleton with lepidotrichia, or bony rays, connected by interrays to form their fins. Every ray comprises two hemirays (Becerra et al., 1983; Becerra et al., 1996). The situation is very different in post-hatching fry. At this stage of development, lepidotrichia are not easily detectable in the caudal fin because they are not yet ossified or calcified, do not present segmentations or bifurcations, and the lobes or the groove between lobes are not recognized (Rojas et al., 2015). A groove separates two lobes of similar size when the fry reaches 30 mm in length and 950 accumulated temperature units (ATUs). Each lepidotrichia is made up of two hemirays that are symmetrical and parallel. Each has a few short segments that are separated by joints and joined together by collagen ligaments. Each ray forks in the most distal region of the fin (Rolland-Lagan et al., 2012).
During fish growth, lepidotrichiae lengthen as a consequence of the addition of new segments at their distal margin, but the length of each segment remains constant after their formation (Christou et al., 2018). It has been reported that the segmentation pattern of the caudal fin of zebrafish is affected by temperature, and, as a consequence, multiple fusions between segments occur (Christou et al., 2018).
Fish have developed various adaptation mechanisms for hypoxic environments. The Hypoxia Inducible Factor 1 (HIF-1) pathway controls gene expression based on oxygen levels (Semenza, 2000; Vuori et al., 2004; Rojas et al., 2007; Rytkönen et al., 2007). This system helps the body adapt to low oxygen levels by turning on genes that make erythropoietin and vascular endothelial growth factor (VEGF). This makes the body’s oxygen transport system work better and increases the growth of blood vessels in specific areas (Nikinmaa and Rees, 2005; Zarember and Malech, 2005; Nanka et al., 2006).
HIF-1 is a heterodimer composed of two protein subunits, HIF-1α and HIF-1β. The ubiquitin-proteasome system quickly degrades HIF-1, which results in its inactivation, while the subunit’s expression is constitutive. Under normoxia conditions, HIF-1 undergoes an oxygen-dependent mechanism of hydroxylation on proline and asparagine residues. Hypoxic conditions, on the other hand, cause more HIF-1α to build up in the nucleus of cells, which controls how HIF-1 works (Hochachka and Lutz, 2001; Zarember and Malech, 2005; Rojas et al., 2007). Based on the aforementioned, we can infer that various HIF-1 detection systems serve as reliable indicators for identifying whether the studied tissues have experienced low oxygen concentrations.
Our hypothesis is that hypoxia is one of the etiological factors in the deformation of the body of the fish and the caudal fin. So, the first goal was to find out if there is a link between being exposed to hypoxia and body deformities, including deformities of the caudal fin, as well as if there is a link between hypoxia and changes in the fry’s total length and caudal fin length. The second objective was to histologically recognize the effects of different periods of hypoxia on the caudal fin of fry (angiogenesis). The third goal was to use immunocytochemistry to find hypoxic cells in the fry (HIF-1α) and see if it was possible to use the anti-HIF-1 antibody as a hypoxia sensor.
2 Materials and methods
2.1 Animals
Around a thousand Salmo salar salmon eggs were used, which were grown at the Aquaculture Center of the Universidad de Chile in the city of Castro, Chiloé, Chile. An Oxyguard® monitor controlled the culture at a constant temperature of 9°C and 100% oxygen saturation.
We measured the fry’s ages in ATUs, or accumulated thermal units, which represent the number of days of fry development multiplied by the water temperature. It is equal to multiplying the fry’s development days by the temperature of the water, where 950 ATU corresponds to a larva or fry without a yolk sac, and that is at the beginning of the first feeding. A hatching of 495 UTAs results in a fry of 950 UTAs, which corresponds to an alevin without a vitelline sac and marks the beginning of the first feeding.
At the time of hatching (495 ATUs), a total of two hundred and forty fry were randomly selected and assigned to six groups of forty individuals each (Table 1). Group H0 maintained its fry under normoxia conditions, with 100% O2 saturation. H2, H4, H6, H8, and CH were exposed to different periods of hypoxia (60% O2 saturation, a condition obtained by slowing the water flow).
We transferred the fry from groups H2, H4, H6 and H8 from hypoxia to normoxia on days 2, 4, 6, and 8 post-hatching (corresponding to 513, 531, 549, and 567 ATUs, respectively). We maintained the CH group in hypoxic conditions from hatching until 950 ATUs. We used 5% benzocaine (BZ-20®, Veterquimica, Chile) to euthanize the fry after 950 ATUs, or roughly 3 months of culture. The Scientific Ethics Committee of the Universidad de La Frontera has approved the experimental protocol (N°061_20).
We randomly separated ten fry from each group and subjected them to various types of anatomical and morphological studies. These fry were fixed by immersion for 24 h in 10% neutral buffered formalin (4% w/v) in 0.1M PBS pH 7.0 at room temperature.
2.2 Anatomical evaluation
The protocol of Hanken and Wassersug (1981) guided the diaphanization process. We randomly selected eight fry from each group (a total of 48), subjected them to protein digestion using trypsin, and then treated them with Alcian Blue stain to detect the glycosaminoglycans of the cartilage tissue. We evaluated the specimens using a stereoscopic microscope (Stemi DV4, Zeiss®, Oberkochen, Germany) and took photographs with a digital camera (Powershot Megapixeles, Canon Inc., Tokyo, Japan). We determined the body length of each individual (Figure 1A), and the present deformations were described, using as a criterion the existence of one or more visible alterations in the anatomical planes. We analyzed the caudal fin by quantifying the number and length of the rays.
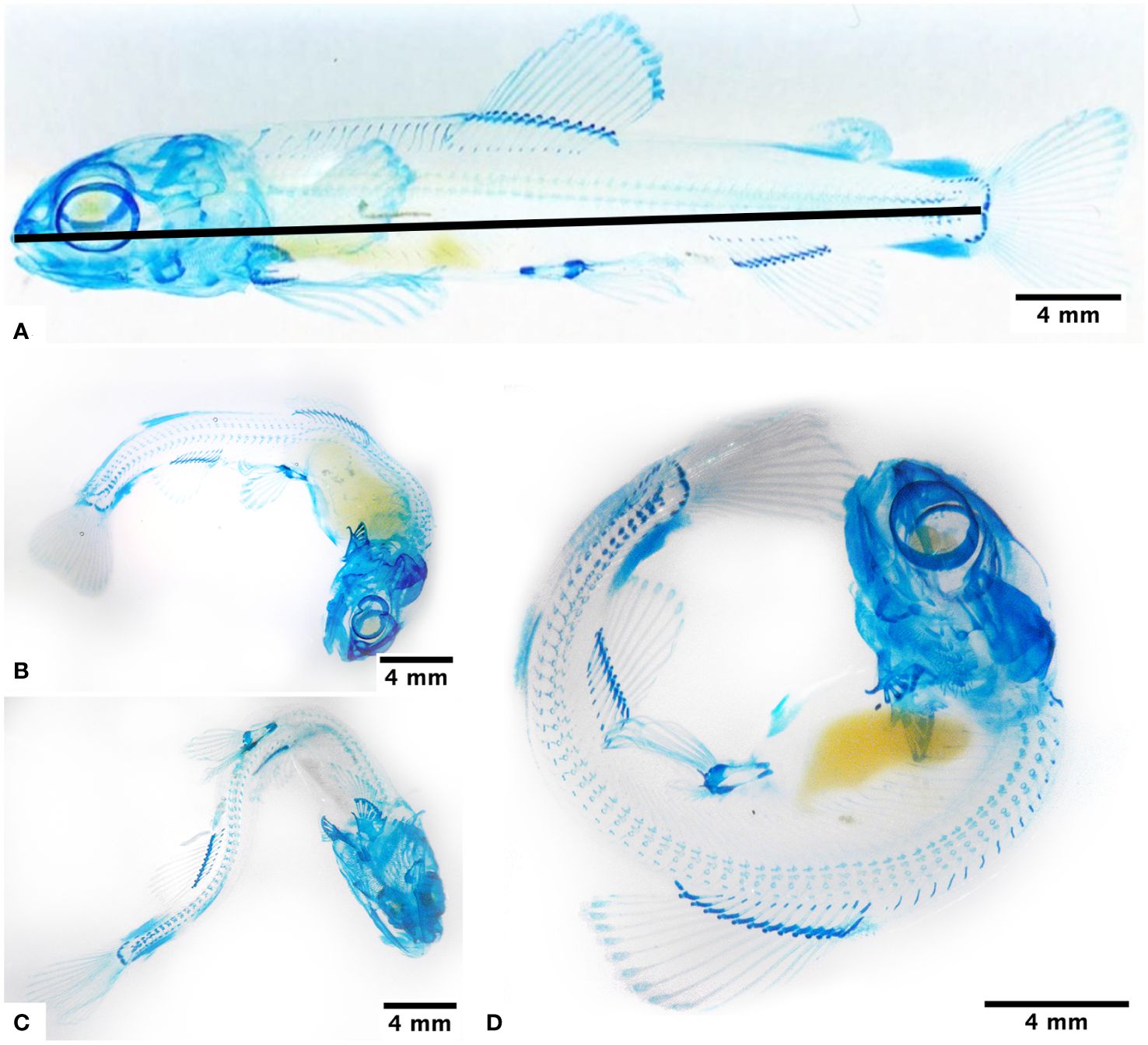
Figure 1 Association between exposure to hypoxia and the presentation of body deformities, observed at 950 ATUs in fry under normoxia conditions (A), subjected to 4 days in hypoxia (B), and subjected to 8 days in hypoxia (C, D). Alcian blue staining highlights glycosaminoglycans mainly in the hyaline cartilage of the skull, spine, and fins, as well as the blastema of the lepidotrichiae. The continuous line indicates where the measures were performed.
2.3 Histological evaluation
For histological evaluation, 20 samples of fry were selected from each group, which were processed and included in Paraplast. A microtome (Microm HM315R, Thermo Fisher Scientific, Waltham, MA, USA) was used to cut the tissue into 5 μm slices. These slices were then stained with Hematoxylin-Eosin-Alcian Blue at pH 2.5 to identify the presence of glycosaminoglycan deposits, check the general structure, and identify areas of vascular density. Images were obtained using a light microscope (Axiostar Plus, Zeiss®, Oberkochen, Germany) with an attached digital camera (Powershot Megapixeles, Canon Inc., Tokyo, Japan).
2.4 Immunohistochemical evaluation
Rabbit polyclonal antibodies HIF-1α (H-206, Santa Cruz Biotechnology, Dallas, TX, USA) were used. Incubations were performed on 5 μm-thick sections obtained on a microtome (Microm HM315R, Thermo Fisher Scientific, Waltham, MA, USA), which were collected on silanized coverslips. Antigen retrieval was carried out in a steamer using an antigen unmasking solution (Vector Laboratories, Newark, CA, USA). We blocked nonspecific proteins with PBS+BSA and then incubated the primary antibodies in PBS at a 1:100 dilution. Subsequently, the sections were incubated with conjugated polymer for 10 to 15 min. The enzymatic reaction was revealed with diaminobenzidine (Vector Laboratories, Newark, CA, USA). The negative control contemplated the development of the complete immunohistochemical technique but omitted the primary antibodies. Blood vessels were used as a positive control for the anti-HIF-1α antibody. In each sample, 100 cells were counted, and the number of them that showed positive immunostaining was indicated.
2.5 Statistical analysis
The values obtained for the quantitative variables were described through the arithmetic mean and standard deviation. The Kurtosis Coefficient Test was carried out with the purpose of analyzing the degree of concentration of the values present around the central area of the distribution. The ANOVA test was used to evaluate the equality of the group means. In order to establish whether the differences between the means had statistical significance, the Tukey test was used. Finally, Pearson correlation coefficients (r) were calculated to analyze the relationship between those variables considered most relevant. An α error of 5% was considered in all statistical tests used. The data obtained were processed with the GraphPad Prism 5.0 statistical program (GraphPad Software, San Diego, CA, USA).
3 Results
3.1 Association between exposure to hypoxia and the presence of deformities in Salmo salar fry
The group of fry maintained in normoxia conditions (H0) and for two days in hypoxia (H2) did not present body malformations (Figure 1A). Groups H4 and H6 presented a notable curvature in the dorsal region (Figure 1B), and fry H8 and CH presented different curvatures of the body (Figure 1C); some fry even appears curled in the shape of a C (Figure 1D).
3.2 Relationship between exposure to hypoxia and body length measured at 950 ATUs
In relation to body length, significant differences (p<0.025) were observed in body length between groups H0, H2, and H6 compared to group CH (continuous hypoxia). Likewise, it showed significant differences (p<0.05) between groups H8 and CH (Table 2). Consistently, fry exposed to continuous hypoxia achieved shorter body lengths compared to all other groups.
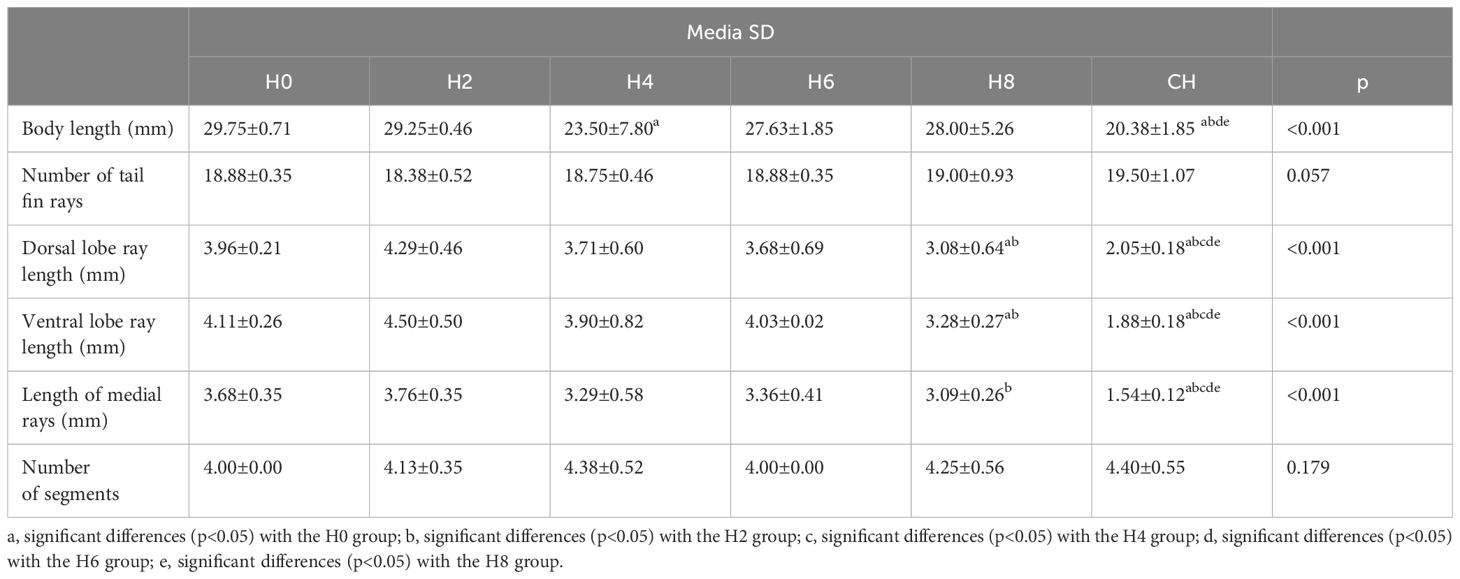
Table 2 Statistical analysis of morphological characteristics of the body of the fry and the caudal fin of the Atlantic Salmon (Salmo salar) subjected to hypoxia.
3.3 Morphology of the normal caudal fin of a fry at 950 ATUs
The caudal fin of the normal fry of 950 ATUs has two lobes, one dorsal and one ventral, both approximately symmetrical in shape. On average, 18.9 ± 0.35 lepidotrichias were observed, arranged in a fan shape, with those located in the central region of the fin being shorter. Each lepidotrichia is made up of two hemirays (right and left). Additionally, the presence of 4–5 segments and the interrays of mesenchymal tissue can be distinguished. Figure 2A illustrates these anatomical features. Externally, the interrays are covered by the epidermis. In general, in teleost fish, ns of the lepidotrichia of the fins can be evident in their most distal area. The segmentations are almost imperceptible. The lepidotrichia of the caudal fin of fry are made up of a blastema of mesenchymal tissue very rich in glycosaminoglycans, which was evidenced by staining with Alcian Blue (Figure 2A).
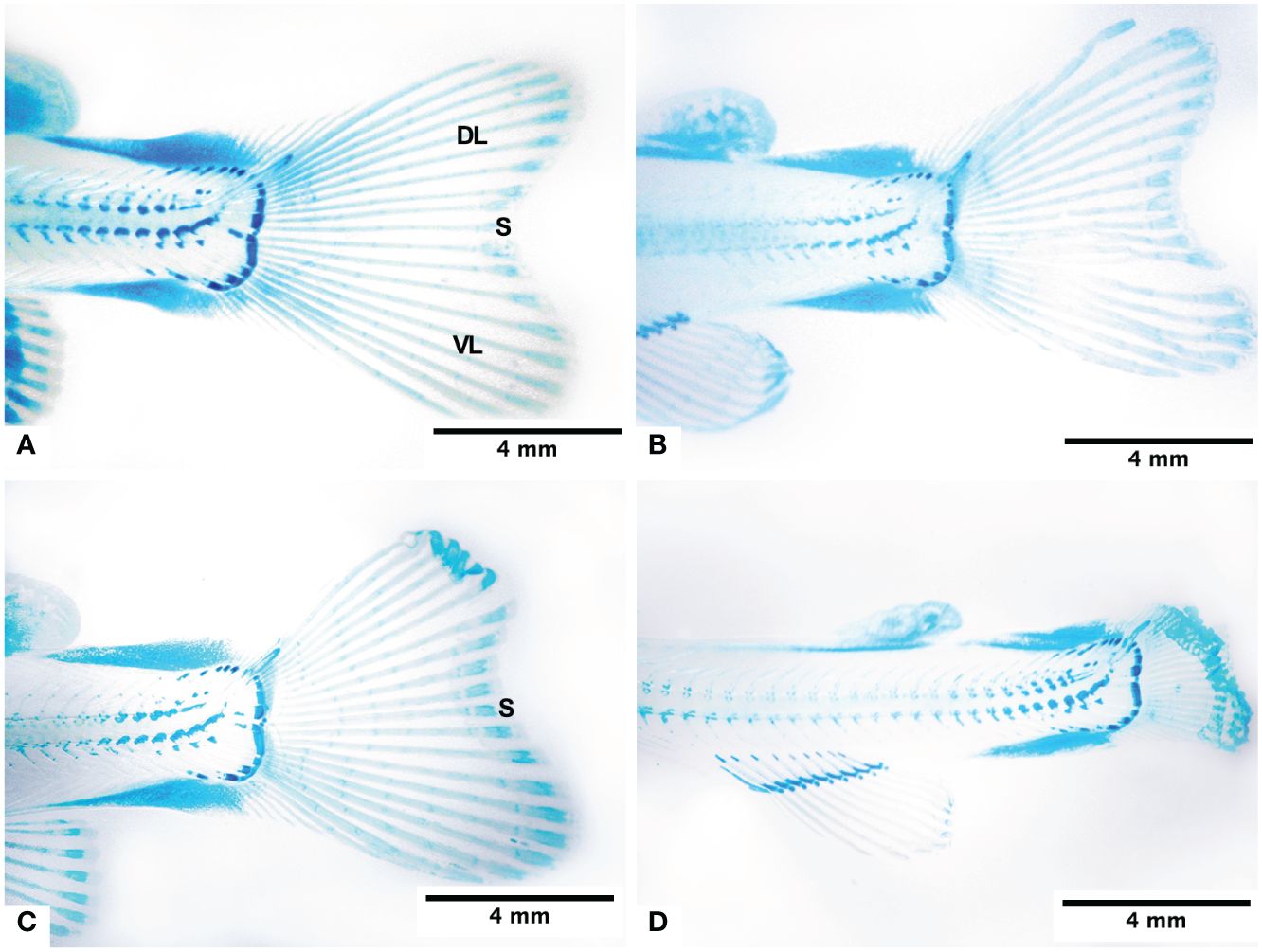
Figure 2 Morphology of the caudal fin of the fry at 950 ATUs and its changes under normoxia conditions (A), subjected to 4 days in hypoxia (B), subjected to 8 days in hypoxia (C), and subjected to continous hypoxia (D). Dorsal lobe (DL), ventral lobe (VL) and sulcus (S).
3.4 Morphology of the caudal fin of a fry subjected to hypoxia
The fry from groups H2 to H8, subjected to varying times of hypoxia, displayed very similar morphological characteristics (Figures 2B, C). The dorsal and ventral lobes are similar in size. The CH group fry had a very short caudal fin, and its hemirays were spread out and folded in the farthest part (Figure 2D). There was a big difference in the length of the dorsal lobe rays between groups H0, H2, H4, and H6 and those who were always lacking oxygen (Table 2).
The length of the rays of the ventral lobe presented significant differences between groups H0, H2, H4, and H6 (4 mm), group H8 (eight days in hypoxia), and group CH. We also found significant differences between groups H6 and CH. The lepidotrichiae on the middle part of the fin of groups H0 to H8 are significantly longer than those of group CH. The length of the lepidotrichiae of groups H0 and H2 is significantly greater than that of group H8.
3.5 Histology of the caudal fin of fry from 950 ATUs kept in hypoxia
The fish were exposed to hypoxia for two to eight days. It was found that the interray blastema was made up of mesenchymal tissue with blood vessels, some episodes of angiogenesis, and melanomacrophages (Figures 3A, B). The fish arranged their blood vessels parallel to the rays and interrays (Figure 3C). New capillaries emerged perpendicularly from these blood vessels (Figure 3D).
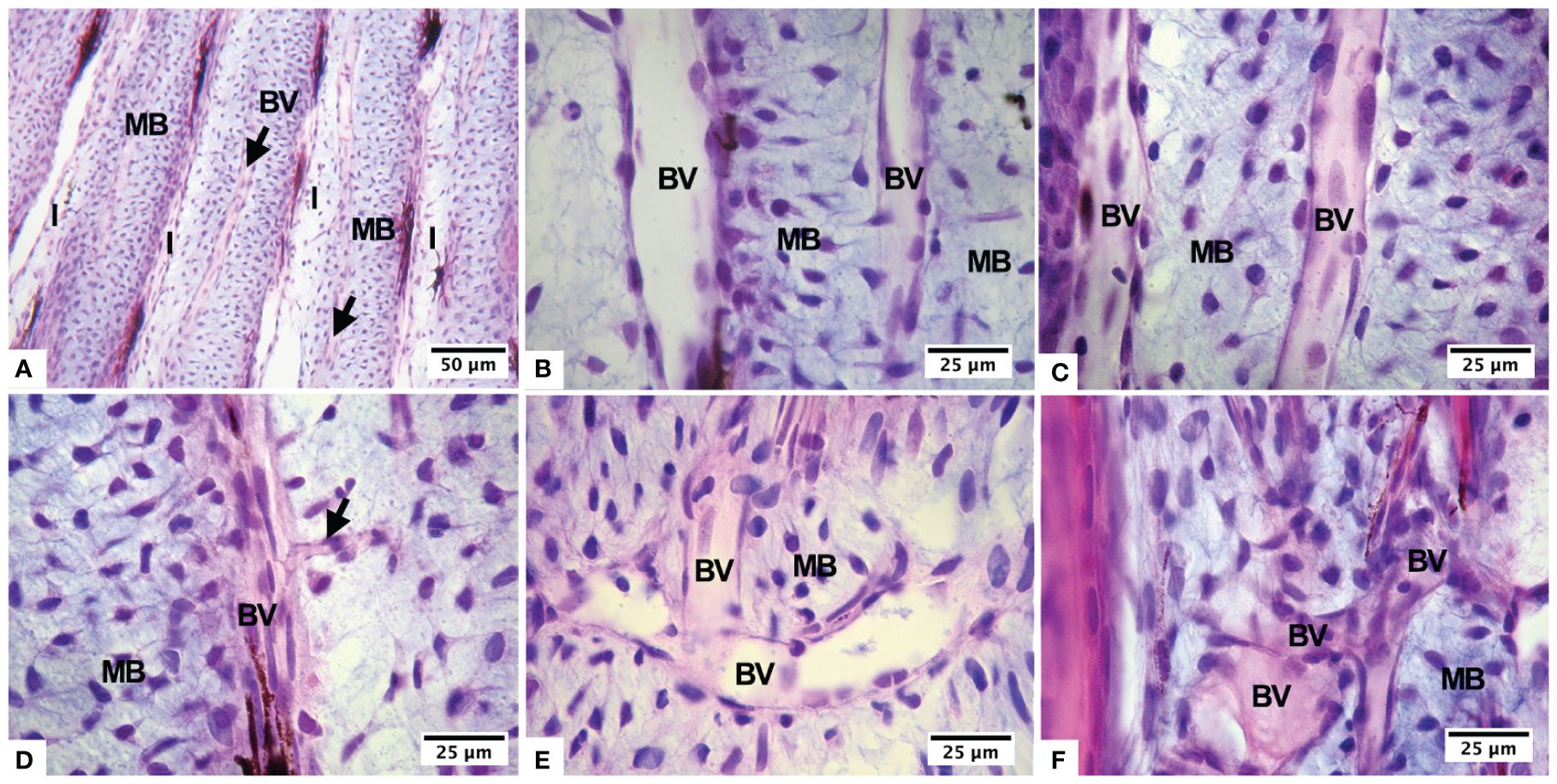
Figure 3 Histological changes of the lepidotrichia of the caudal fin usually formed by a mesenchymal blastema that includes a blood vessel within and interlepidotrichial areas (A), during normoxia (B), hypoxia for 4 days (C, D), hypoxia for 8 days (E), and continuous hypoxia (F). Blood vessels in longitudinal arrangement (BV), mesenchymal blastema (MB), and inter-lepidotrichial areas (I).
Advanced angiogenesis and disorganization of the blood capillaries, arranged in different directions and overlapping each other, were evident in fish kept in hypoxia for 8 days and in continuous hypoxia. Furthermore, we observed an interray blastema, characterized by an extracellular matrix rich in glycosaminoglycans and blood vessels of varying caliber. These vessels emit sprouts in a disorganized manner, and both the rays and interrays exhibit neoformation of blood vessels (Figures 3E, F). In some lepidotrichias, the beginning of the process of ossification and peripheral calcification occurs, evidencing the presence of flattened osteoblastic cells. We observed an internal blastema of mesenchymal cells.
3.6 Immunohistochemical evaluation
We observed no immunopositive cells in fish maintained under normoxia conditions (Figure 4B), nor in groups H2 and H4 under hypoxic conditions.
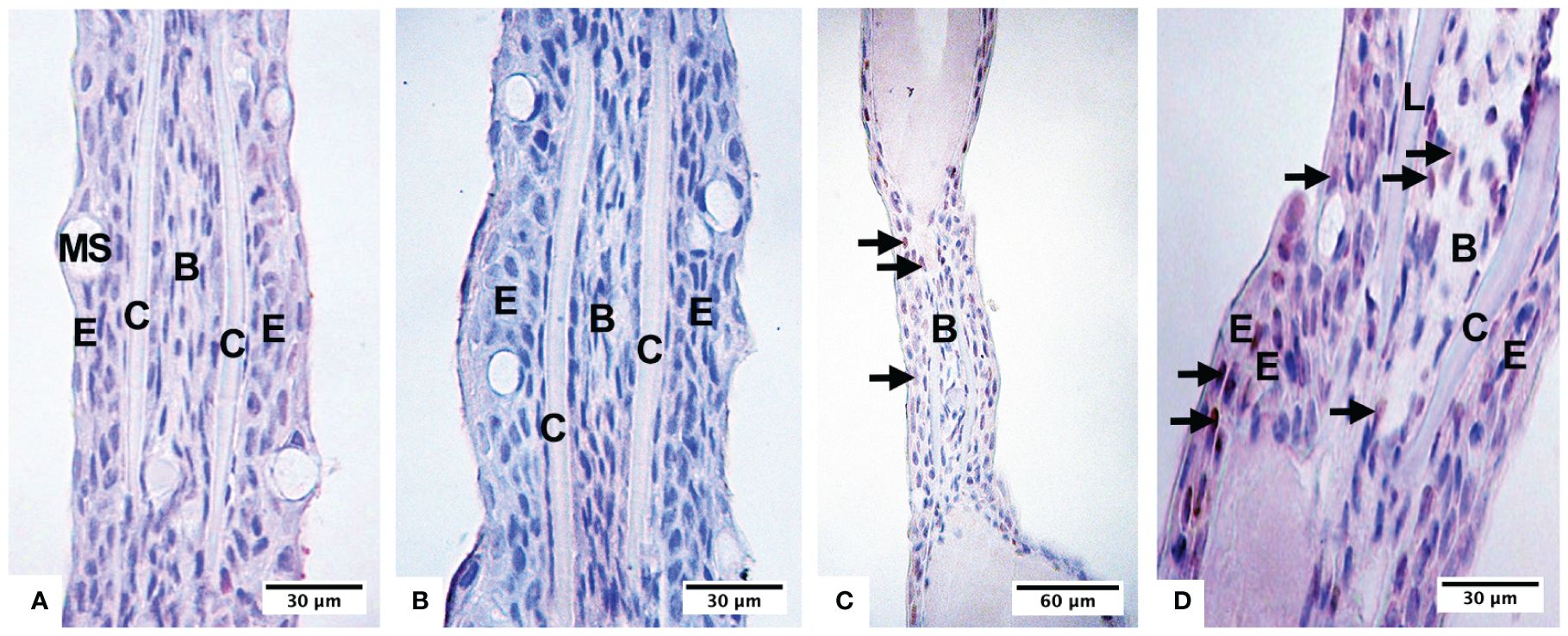
Figure 4 Presence of hypoxic cells in fry, recognizable by immunohistochemistry with the anti-HIF-1a antibody in the negative control (A), under normoxia conditions (B), subjected to 6 days in hypoxia (C), and subjected to 8 days in hypoxia (D). Epidermis (E), calcified area of the lepidotrichia (C), blastema of the lepidotrichia (B), mucus-secreting cells (MS).
The presence of nuclei of cells immunopositive for the antiHIF-1α antibody was recognized after 6 days of being maintained in hypoxia (Figure 4C); thus, in the epidermis, 10% of positive cells were quantified, and in the intralepidotrichia mesenchymal tissue, 6% of positive cells. After 8 days, we found 37% of the labeled cells in the epidermis and 10% in the blastema (Figure 4D). The fry, kept in continuous hypoxia, had 80% of the labeled cells in the epidermis and 20% in the intralepidotrichia blastema (Table 2). The negative control of the technique is indicated in Figure 4A.
4 Discussion
4.1 Association between exposure to hypoxia and the presentation of deformities, especially of caudal fins
We have presented evidence that points to an association between continuous hypoxia and caudal fin deformities in S. salar fry. There are significant differences between exposure to hypoxia and the presentation of caudal fin deformities between the groups with 2, 4, 6, and 8 days of exposure to hypoxia and the group subjected to continuous hypoxia. However, when compared to the control group under normoxia, these differences did not reach statistical significance.
Other published studies have not examined the caudal fin of S. salar fry, which has not been the subject of any other published studies. Regarding spinal column deformation, the findings of the current work are consistent with those of Castro et al. (2011). It has also been reported that continuous hypoxia, induced by culture density, affects skeletal development by altering the expression of genes related to bone metabolism in Atlantic salmon (Pellón et al., 2015). Our results point in the same direction as the findings reported by Shang and Wu (2004), who point out that aquatic hypoxia in embryos generates congenital malformations.
4.2 Association between hypoxia, body length, and caudal fin
The findings from studying fry that were kept in hypoxic conditions for 2, 4, 6, and 8 days, but mostly after being there for 6 or more days, show that this is a major environmental stressor that hinders the normal growth of both the body length and the caudal fin. Peñailillo (2011), who observed lower growth in fry kept in a hypoxic environment compared to control individuals kept in normoxia, reported a similar finding. From day 5 onwards, the fry in the group subjected to more intense hypoxia significantly reduced their body length compared to the control group, which, according to the author’s work, corresponded to 60% O2 saturation.
It has been found that fibroblast growth factors (Fgfs), Sonic hedgehog a (Shha), and bone morphogenetic protein 2b (Bmp2b) all play a role in the growth of lepidotrichia (Quint et al., 2002; Lee et al., 2005). During ontogeny, Fgfs control cell proliferation rates in the caudal fin (Wills et al., 2008). Zebrafish also exhibit varying segment lengths within and between rays (Iovine and Johnson, 2000; Jain et al., 2007). In particular, within a lepidotrichia, segments are longer in proximal positions than in distal positions (Iovine and Johnson, 2000; Sims et al., 2009). However, similar research has reported that lobar lepidotrichiae have longer segments (Haas, 1962) and more segments than central ones (Goldsmith et al., 2006). In the present work, we have presented evidence that shows that in cases of hypoxia, the caudal fins grow less and their apical edges bend and roll.
4.3 Histology of the caudal fin of fry from 950 ATUs in hypoxia
We kept salmon fry under hypoxic conditions for 2, 4, 6, and 8 days in our study. After that, we did a histological analysis at 950 ATUs (about 3 months) and saw that hypoxia caused more new blood vessels to form (angiogenesis), which could be seen as a response to get more oxygen to the tissues.
Rojas et al. (2015) have previously described both the histology and anatomy of the salmon caudal fin at different stages of development, making the diagnosis of early pathologies in said structure more plausible. According to Griffioen and Molema (2000) and Distler et al. (2003), hypoxia constitutes the main stimulus for the formation of new blood vessels by inducing, by endothelial, tumor, inflammatory cells, and macrophages, the production of pro-angiogenic factors such as vascular endothelial growth factor (VEGF). These factors, in turn, generate effects such as increased vascular permeability and vasodilation. This would explain the increase in the diameter of blood vessels observed both at eight days under hypoxia and in continuous hypoxia, a finding similar to that reported by Peñailillo (2011) in the gills. Taking into consideration that HIF mediates the expression of the genes that transcribe VEGF (Nikinmaa and Rees, 2005; Zarember and Malech, 2005; Nanka et al., 2006), it is reasonable to propose that this factor could be responsible for the observed increase in local vascularization.
4.4 Anti-HIF1α antibody as a sensor of hypoxic cells
After being exposed to hypoxia for 6 days, fry that were constantly exposed to it had HIF-1α-positive nuclei in cells of the epidermis’s epithelial tissue, the mesenchyme, and the blastema. On the contrary, fry that remained in normoxia conditions did not show positive labeling in such structures. This finding suggests that we can use the anti-HIF antibody as a sensor of hypoxic cells in the same tissues. It is worth mentioning that HIF-1α participates in several developmental processes, including angiogenesis, vasculogenesis, and the development of the heart and central nervous system (Nikinmaa and Rees, 2005). For instance, studies have demonstrated the essential role of HIF-1 in the normal development of the heart and blood vessels in zebrafish, as well as in ensuring the survival of embryos. HIF-1 activates the expression of genes that produce growth factors such as VEGF and platelet-derived growth factor (PDGF) in Atlantic salmon. These factors help create new blood vessels and blood vessels in areas that do not get enough oxygen. However, more research needs to be done on oxygen sensing, hypoxia adaptation, chemosensory NECs that start reflex responses to hypoxia, and how these cells connect with the growth of filaments and lamellae in salmon fry.
According to the results obtained, it is feasible to indicate that there is a clear association between i) exposure to hypoxia and the presentation of deformities in salmon fry and ii) hypoxia and changes in the body length of the fry and its caudal fin.
5 Conclusions
The present study observed that fry exposed to continuous hypoxia achieved a shorter body length compared to all other groups. This fact alone points to the importance of monitoring and controlling oxygen conditions in culture systems and also in fish tissues.
Histologically, angiogenesis, disorganization of blood vessels, and vasodilation can be recognized, which was possible to verify and describe several months after the hypoxia exposure event occurred. Finally, hypoxic cells in the fry could be recognized by labeling with the anti-HIF-1α antibody, thus confirming its use as a hypoxia sensor. Taken together, the evidence reported here indicates that hypoxia not only affects the fry during the phase of development directly exposed to the event, but that its effects persist long afterward and affect the fry during their ontogeny.
Data availability statement
The original contributions presented in the study are included in the article/supplementary material. Further inquiries can be directed to the corresponding author/s.
Ethics statement
The animal study was approved by Scientific Ethics Committee of the Universidad de La Frontera (N°061_20). The study was conducted in accordance with the local legislation and institutional requirements.
Author contributions
MR: Conceptualization, Formal analysis, Methodology, Writing – original draft, Writing – review & editing. RS: Investigation, Writing – original draft, Writing – review & editing. CaS: Investigation, Methodology, Writing – original draft, Writing – review & editing. CrS: Formal analysis, Methodology, Writing – original draft, Writing – review & editing. VS-M: Methodology, Writing – original draft, Writing – review & editing. MD: Conceptualization, Supervision, Writing – original draft, Writing – review & editing.
Funding
The author(s) declare that no financial support was received for the research, authorship, and/or publication of this article.
Conflict of interest
The authors declare that the research was conducted in the absence of any commercial or financial relationships that could be construed as a potential conflict of interest.
Publisher’s note
All claims expressed in this article are solely those of the authors and do not necessarily represent those of their affiliated organizations, or those of the publisher, the editors and the reviewers. Any product that may be evaluated in this article, or claim that may be made by its manufacturer, is not guaranteed or endorsed by the publisher.
References
Becerra J., Junqueira L. C., Bechara I. J., Montes G. S. (1996). Regeneration of fin rays in teleosts: a histochemical, radioautographic, and ultrastructural study. Arch. Histol. Cytol. 59, 15–35. doi: 10.1679/aohc.59.15
Becerra J., Montes G. S., Bexiga S. R., Junqueira L. C. (1983). Structure of the tail fin in teleosts. Cell Tissue Res. 230, 127–137. doi: 10.1007/BF00216033
Böckelmann P. K., Ochandio B. S., Bechara I. J. (2010). Histological study of the dynamics in epidermis regeneration of the carp tail fin (Cyprinus carpio, Linnaeus 1758). Braz. J. Biol. 70, 217–223. doi: 10.1590/s1519–69842010000100030
Castro S. R., Obregón E. B., Rauco M. R. (2011). Hypoxia is like an ethiological factor in vertebral column deformity of salmon (Salmo salar). Aquaculture 316, 13–19. doi: 10.1016/j.aquaculture.2011.03.012
Christou M., Iliopoulou M., Witten P. E., Koumoundouros G. (2018). Segmentation pattern of zebrafish caudal fin is affected by developmental temperature and defined by multiple fusions between segments. J. Exp. Zool. 330, 330–340. doi: 10.1002/jez.b.22825
Distler J. H., Hirth A., Kurowska-Stolarska M., Gay R. E., Gay S., Distler O. (2003). Angiogenic and angiostatic factors in the molecular control of angiogenesis. Q. J. Nucl. Med. Mol. Imaging 47, 149–161.
Dunel-Erb S., Bailly Y., Laurent P. (1982). Neuroepithelial cells in fish gill primary lamellae. J. Appl. Physiol. 53, 1342–1353. doi: 10.1152/jappl.1982.53.6.1342
Goldsmith M. I., Iovine M. K., O’Reilly-Pol T., Johnson S. L. (2006). A developmental transition in growth control during zebrafish caudal fin development. Dev. Biol. 296, 450–457. doi: 10.1016/j.ydbio.2006.06.01
Griffioen A. W., Molema G. (2000). Angiogenesis: potentials for pharmacologic intervention in the treatment of cancer, cardiovascular diseases, and chronic inflammation. Pharmacol. Rev. 52, 237–268.
Haas H. J. (1962). Studies on mechanisms of joint and bone formation in skeleton rays of fish fins. Dev. Biol. 5, 1–34. doi: 10.1016/0012–1606(62)90002–7
Hanken J., Wassersug R. (1981). The visible skeleton. A new double-stain technique reveals the native of the ‘hard’ tissues. Funct. Photography. 16, 22–26.
Hochachka P. W., Lutz P. L. (2001). Mechanism, origin, and evolution of anoxia tolerance in animals. Comp. Biochem. Physiol. Part B. Comp. Biochem. 130, 435–459. doi: 10.1016/S1096–4959(01)00408–0
Iovine M. K., Johnson S. L. (2000). Genetic analysis of isometric growth control mechanisms in the zebrafish caudal fin. Genetics 155, 1321–1329. doi: 10.1093/genetics/155.3.1321
Jain I., Stroka C., Yan J. Y., Huang W. M., Iovine M. K. (2007). Bone growth in zebrafish fins occurs via multiple pulses of cell proliferation. Dev. Dynamics. 236, 2668–2674. doi: 10.1002/dvdy.21270
Jonz M. G., Nurse C. A. (2003). Neuroepithelial cells and associated innervation of the zebrafish gill: A confocal immunofluorescence study. J. Compar. Neurol. 461, 1-17. doi: 10.1002/cne.10680
Jonz M. G., Nurse C. A. (2006). Epithelial mitochondria-rich cells and associated innervation in adult and developing zebrafish. J. Comp. Neurol. 497, 817–832. doi: 10.1002/cne.21020
Lauriano E. R., Capillo G., Icardo J. M., Fernandes J. M. O., Kiron V., Kuciel M., et al. (2021). Neuroepithelial cells (NECs) and mucous cells express a variety of neurotransmitters and neurotransmitter receptors in the gill and respiratory air-sac of the catfish Heteropneustes fossilis (Siluriformes, Heteropneustidae): A possible role in local immune defence. Zoology 148, 125958. doi: 10.1016/j.zool.2021.125958
Lee Y., Grill S., Sanchez A., Murphy-Ryan M., Poss K. D. (2005). Fgf signaling instructs position-dependent growth rate during zebrafish fin regeneration. Development 132, 5173–5183. doi: 10.1242/dev.02101
Nanka O., Valásek P., Dvoráková M., Grim M. (2006). Experimental hypoxia and embryonic angiogenesis. Dev. Dynamics. 235, 723–733. doi: 10.1002/dvdy.20689
Nikinmaa M., Rees B. B. (2005). Oxygen-dependent gene expression in fishes. Am. J. Physiol. Reguatory. Integr. Comp. Physiol. 288, R1079–R1090. doi: 10.1152/ajpregu.00626.2004
Pellón M., Rojas M., Yaikin P., del Sol M. (2015). Estudio morfológico de la retina de salmones (Salmo salar). Int. J. Morphol. 33, 788–793. doi: 10.4067/S0717–95022015000200058
Pellón M., Smok C., Vásquez B., del Sol M., Rojas M. (2022). Bioestructura de la Piel de la Aleta Caudal del Salmón del Atlántico, en Estado Smolt y sus Interacciones con el Estado Chalimus del Caligus Rogercresseyi. Int. J. Morphol. 40, 489–494. doi: 10.4067/S0717–95022022000200489
Peñailillo P. (2011). Estudio morfológico de branquias de alevines Salmo salar sometidos a hipoxia [Thesis]. Available online at: https://repositorio.uChile.cl/bitstream/handle/2250/183536/Pe%c3%b1ailillo%20Gonz%c3%a1lez%2c%20Paola%20%28Tesis%29.pdf?sequence=1&isAllowed=y (Accessed 13 december 2023).
Podrabsky J. E., Lopez J. P., Fan T. W., Higashi R., Somero G. N. (2007). Extreme anoxia tolerance in embryos of the annual killifish Austrofundulus limnaeus: insights from a metabolomics analysis. J. Exp. Biol. 210, 2253–2266. doi: 10.1242/jeb.005116
Quesada C. G., Herdman J., Berasain G. E., Miranda L. A. (2023). Influence of sewage discharge on dissolved oxygen concentration and fish diversity in the Girado stream and Chascomús lake. Environ. Monit. Assess. 195, 1433. doi: 10.1007/s10661–023-12070–3
Quint E., Smith A., Avaron F., Laforest L., Miles J., Gaffield W., et al. (2002). Bone patterning is altered in the regenerating zebrafish caudal fin after ectopic expression of sonic hedgehog and bmp2b or exposure to cyclopamine. Proc. Natl. Acad. Sci. United. States America 99, 8713–8718. doi: 10.1073/pnas.122571799
Reed M., Jonz M. G. (2016). Neurochemical signalling associated with gill oxygen sensing and ventilation: A receptor focused mini-review. Front. Physiol. 13. doi: 10.3389/fphys.2022.9400201
Rojas M., Pellón M., Cerda C., Ulloa R., Suarez R., Godoy M., et al. (2020). Deformación de la Aleta Caudal en Alevín de Salmón del Atlántico (Salmo salar), en Etapa de Saco Vitelino. Int. J. Morphol. 38, 1631–1638. doi: 10.4067/S0717–95022020000601631
Rojas M., Pellón M., del Sol M. (2015). Desarrollo de la Aleta Caudal del Salmón (Salmo salar). Int. J. Morphol. 33, 514–521. doi: 10.4067/S0717–95022015000200017
Rojas D. A., Perez-Munizaga D. A., Centanin L., Antonelli M., Wappner P., Allende M. L., et al. (2007). Cloning of hif-1alpha and hif-2alpha and mRNA expression pattern during development in zebrafish. Gene Expression Patterns. 7, 339–345. doi: 10.1016/j.modgep.2006.08.002
Rolland-Lagan A. G., Paquette M., Tweedle V., Akimenko M. A. (2012). Morphogen-based simulation model of ray growth and joint patterning during fin development and regeneration. Development 139, 1188–1197. doi: 10.1242/dev.073452
Rytkönen K., Vuori K. A., Primmer C. R., Nikinmaa M. (2007). Comparison of hypoxia-inducible factor-1 alpha in hypoxia-sensitive and hypoxia-tolerant fish species. Comp. Biochem. Physiol. Part D. Genomics Proteomics 2, 177–186. doi: 10.1016/j.cbd.2007.03.001
Saltys H. A., Jonz M. G., Nurse C. A. (2006). Comparative study of gill neuroepithelial cells and their innervation in teleosts and Xenopus tadpoles. Cell Tissue Res. 323, 1–10. doi: 10.1007/s00441–005-0048–5
Semenza G. L. (2000). HIF-1: mediator of physiological and pathophysiological responses to hypoxia. J. Appl. Physiol. 88, 1474–1480. doi: 10.1152/jappl.2000.88.4.1474
Sfakianakis D. G., Renieri E., Kentouri M., Tsatsakis A. M. (2015). Effect of heavy metals on fish larvae deformities: A review. Environ. Res. 137, 246–255. doi: 10.1016/j.envres.2014.12.014
Shang E. H., Wu R. S. (2004). Aquatic hypoxia is a teratogen and affects fish embryonic development. Environ. Sci. Technol. 38, 4763–4767. doi: 10.1021/es0496423
Sims K. D. Jr., Eble M., Iovine M. K. (2009). Connexin43 regulates joint location in zebrafish fins. Dev. Biol. 327, 410–418. doi: 10.1016/j.ydbio.2008.12.027
Venegas F., Montiel E., Forno P., Rojas M. (2003). Histology of the Jaw deformation in salmon of Southern Chile (Salmo salar). Int. J. Morphol. 21, 211–219. doi: 10.4067/S0717–95022003000300005
Vuori K. A., Soitamo A., Vuorinen P., Nikinmaa M. (2004). Baltic salmon (Salmo salar) yolk-sac fry mortality is associated with disturbances in the function of hypoxia-inducible transcription factor (HIF-1 α) and consecutive gene expression. Aquat. Toxicol. 68, 301–313. doi: 10.1016/j.aquatox.2004.03.019
Wills A. A., Kidd A. R., Lepilina A., Poss K. D. (2008). Fgfs control homeostatic regeneration in adult zebrafish fins. Development 135, 3063–3070. doi: 10.1242/dev.024588
Zaccone G., Lauriano E. R., Kuciel M., Capillo G., Pergolizzi S., Alesci A., et al. (2017). Identification and distribution of neuronal nitric oxide synthase and neurochemical markers in the neuroepithelial cells of the gill and the skin in the giant mudskipper, Periophthalmodon schlosseri. Zoology 125, 41–52. doi: 10.1016/j.zool.2017.08.002
Zaccone G., Mauceri A., Fasulo S. (2006). Neuropeptides and nitric oxide synthase in the gill and the air-breathing organs of fishes. J. Exp. Zool. Part A.: Comp. Exp. Biol. 305, 428–439. doi: 10.1002/jez.a.267
Zarember K. A., Malech H. L. (2005). HIF-1alpha: a master regulator of innate host defenses? J. Clin. Invest. 115, 1702–1704. doi: 10.1172/JCI25740
Keywords: deformation, hypoxia, hypoxic environment, Atlantic salmon, Salmo salar
Citation: Rojas M, Salvatierra R, Smok C, Sandoval C, Souza-Mello V and del Sol M (2024) Effect of hypoxia on the post-hatching growth of the body of the fry and the caudal fin of the Atlantic Salmon (Salmo salar). Front. Mar. Sci. 11:1425671. doi: 10.3389/fmars.2024.1425671
Received: 30 April 2024; Accepted: 11 June 2024;
Published: 25 June 2024.
Edited by:
Seyyed Morteza Hoseini, Iranian Fisheries Science Research Institute (IFSRI), IranReviewed by:
Giacomo Zaccone, University of Messina, ItalyElisabeth Ytteborg, Fisheries and Aquaculture Research (Nofima), Norway
Copyright © 2024 Rojas, Salvatierra, Smok, Sandoval, Souza-Mello and del Sol. This is an open-access article distributed under the terms of the Creative Commons Attribution License (CC BY). The use, distribution or reproduction in other forums is permitted, provided the original author(s) and the copyright owner(s) are credited and that the original publication in this journal is cited, in accordance with accepted academic practice. No use, distribution or reproduction is permitted which does not comply with these terms.
*Correspondence: Cristian Sandoval, Y3Jpc3RpYW4uc2FuZG92YWxAdWZyb250ZXJhLmNs