- 1School of Food and Science, Zhejiang Pharmaceutical University, Ningbo, China
- 2School of Food and Pharmacy, Zhejiang Ocean University, Zhoushan, China
- 3Zhoushan Yueyang Food Co., Ltd., Zhoushan, Zhejiang, China
- 4Zhejiang Xinjiahe Biotechnology Co., Ltd., Wenzhou, Zhejiang, China
- 5Aquatic Products Processing Factory of China National Zhoushan Marine Fisheries Company, Zhoushan, China
Chitin, the second most abundant biopolymer in nature after cellulose, has a diverse array of applications in the pharmaceutical, medical, agricultural, textile, cosmetic, wastewater treatment, and food industries. This versatility is attributed to its essential functional properties, including biodegradability, biocompatibility, and non-toxicity. The primary source of commercial chitin is the shells of crustaceans. However, the quantity and quality of chitin extracted from various crustacean shells have not yet been systematically reported or compared. In this study, we compared the composition of three types of crustacean shells: Antarctic krill (AK), White shrimp (WS), and Crayfish (CF). We employed a consistent sequence of deproteinization, decalcification, and bleaching to extract chitin from these shells. The extracted chitin was characterized by X-ray diffraction (XRD), Fourier-transform infrared spectroscopy (FT-IR), thermogravimetric analysis, and scanning electron microscopy (SEM). Our findings indicated that the chitin content in Antarctic krill reached 24.06%, significantly exceeding that of the other two crustaceans. Notably, scanning electron microscopy (SEM) characterization confirmed that chitin samples from different biological sources exhibit significant structural heterogeneity. This study, through multidimensional morphological analysis, provides critical experimental evidence for both the targeted synthesis of chitin-based functional materials and the high-value-added conversion of crustacean-derived by-products.
1 Introduction
Chitin, a biopolymer that is widely distributed in nature, is a linear polysaccharide with important biological functions and industrial applications. This unique chemical structure gives chitin good biocompatibility and degradability properties (Marsh et al., 2001; Muzzarelli et al., 2012). The diversity of crystalline forms of chitin is an important structural feature. Currently, chitin has three forms, namely, α, β, and γ structures, and these structural features directly affect its physical and functional properties, among which α-chitin is the most common (Delezuk et al., 2019). Chitin is found everywhere in nature, mainly in the cell walls of fungi, algae, and mosses; in the shells of crustaceans and arthropods; and in the horny layers of soft-bodied invertebrates. It is considered the second-largest biopolymer after cellulose (Hamed et al., 2016; Zahedi et al., 2018; Marzieh et al., 2019; Yu et al., 2020). Furthermore, chitin has many special effects, including biodegradability, biocompatibility and avirulence, and these properties give it the potential for a wide range of applications in different fields such as pharmaceuticals, biomedicine, agriculture, textiles, cosmetics, purification of wastewater and food production (Ghorbel-Bellaaj et al., 2013; Hamed et al., 2016).
The current global seafood market is substantial; however, the processing of seafood, including shrimp, crabs, and other crustaceans, generates a significant amount of by-products with no food value, such as exoskeletons and heads. In fact, the inedible parts of crustaceans account for approximately 50-70% of the total yield, representing a considerable percentage (Gildberg and Stenberg, 2001). In the absence of effective disposal methods, this waste poses a significant environmental hazard. Currently, most of this waste is either discarded into the sea, incinerated, sent to landfills, or left to decompose on land (Xu et al., 2013). Consequrntly, the use of these offcuts for the production of chitin is an economical and environmentally friendly method that reduces seafood waste and produces compounds with important biological properties and high value (Hamed et al., 2016).
Antarctic krill play an important role in the Antarctic ecosystem and are key players in the Antarctic marine food chain (Cruz et al., 2018). As the most phylogenetically basal extant crustacean, Antarctic krill retains the ancestral multilayered exoskeletal architecture characteristic of paleocrustaceans. Its distinctive chitinous lamellar organization establishes it as a pivotal model organism for investigating biological-chitin coevolutionary mechanisms. This keystone species serves not only as a critical bioindicator of abyssal ecosystems, but its exceptional polar adaptability coupled with prodigious annual biomass (estimated at 500 million tonnes globally) has positioned it at the forefront of contemporary research in polar marine ecology and bioprospecting initiatives. The consumption of Antarctic krill accounts for approximately 12 per cent of the total resources, indicating that most krill resources are not yet effectively utilized. Waste from shrimp processing, such as the heads and shells of Antarctic krill, accounts for approximately 75% of the total mass of krill, and these fractions are often regarded as low-value raw materials or even treated as waste. For example, during krill harvesting and processing, large quantities of heads and shells are discarded or used only to produce cheap products, which not only results in a waste of resources, but also pollutes coastal resources. However, these by-products are actually enriched with chitin, proteins and minerals with high value-added potential (Chen et al., 2017). White shrimp, as the backbone of global aquaculture (accounting for more than 80% of total shrimp farming), has significant commercial value in Asia and the Americas (Hongkulsup et al., 2016), which generates a significant amount of by-products during processing. The crayfish demonstrates dual environmental and economic significance. As an invasive species proliferating in European and North American aquatic ecosystems, its ecological impact necessitates mitigation strategies, while in China, 50–70% of its processing by-products remain non-edible, underscoring the imperative for circular economy research (Nguyen et al., 2015).
There are many chitin extraction methods, such as the acid-base method, microbial fermentation, enzyme digestion, EDTA method, ionic liquid leaching method, and the hot glycerol pretreatment method. Enzymatic and microbial fermentation methods are gradually becoming a research hotspot owing to milder process conditions and less pollution, but there are still problems such as insufficient deproteinization, expensive commercial enzymes, high cost, and long time-consuming, which are not suitable for industrial mass production (Qian et al., 2012; Kim and Park, 2015). Although the EDTA method is simple to operate, it requires a large amount of investment in the short term (Dou et al., 2014). The ionic liquid method has the problem of difficult recovery of ionic liquids (Qin et al., 2010), and the thermal glycerol pretreatment method and the enhanced atmospheric pressure plasma method form a small amount of waste; however, there is still some doubt about the yield and quality of the chitin produced (Devi and Dhamodharan, 2017; Borić et al., 2018). The acid-base method is one of the most mature chitin extraction methods, and has the benefit of simple procedure, high efficiency, and good product quality.
The extraction of chitin from crustacean shells is typically achieved through a process of chemical deproteinization and decalcification, which involve the removal of proteins and minerals using alkaline and acidic reagents, respectively. In this study, we investigated exoskeletons of three different crustaceans and prepared chitin following a consistent sequence of deproteinization, decalcification, and bleaching. Three different sources of chitin were found to be α-chitin. This study aimed to elucidate the diversity of chitin derived from these crustaceans, examining both yield and chemical quality, as well as potential material applications. To achieve these objectives, we analyzed the composition of shrimp shells from three crustaceans, and the extracted chitin was systematically characterized using X-ray diffraction (XRD), thermogravimetric analysis (TGA), scanning electron microscopy (SEM), and Fourier-transform infrared spectroscopy (FTIR). To elucidate the differences between different sources of chitin and lay a research foundation for the full utilization of shrimp by-products and marine environmental protection. In this study, we chose some abbreviations to represent our research subjects in order to be more concise in the elaboration. AK stands for Antarctic krill, WS stands for White shrimp, and CF stands for Crayfish.
2 Materials and methods
2.1 Raw material and reagents
Three kinds of crustaceans (Antarctic krill, White shrimp and crayfish, labeled as WS, AK and CF, respectively) were used as raw materials, and WS, AK and CF were bought from farmers’ markets. All the chemical reagents were of analytically pure grade.
2.2 Chitin preparation process
The shrimp shells were deproteinized with sodium hydroxide. Firstly, the dried shrimp shells were properly crushed, and the crushed shrimp shells were mixed with 25% NaOH solution in a glass container with a solid-liquid ratio of 1:10, and heated with stirring at 95°C for 2 h. The resulting solid fraction was centrifuged to neutrality using ultrapure water and dried at 60°C. The concentrations of NaOH were 25% and 10% for the second and third deproteinizations, respectively, and the solid-liquid ratio was kept constant.
This study employed a staged demineralization protocol: In the initial phase, shrimp shell specimens were immersed in 10% (w/v) hydrochloric acid solution under constant 60°C with 1:10 (m/v) solid-liquid ratio, subjected to continuous mechanical agitation for 120 min. The subsequent phase maintained identical thermal conditions while optimizing the reaction system to reduce processing duration to 60 min, achieving gradient decalcification.
After deproteinization and decalcification, a bleaching step was carried out on the remaining material with an aqueous solution of 10% H2O2 with stirring at 80°C for 2 h. Repeat the bleaching operation 2–3 times until the chitin became white and free of impurities visible to the naked eye, and centrifuged to neutrality with ultrapure water. The purified chitin samples were dried in an incubator at 65°C (Sagheer et al., 2009).
The extraction process of chitin is described in Figure 1. Chitin content was determined by the difference between the mass of raw shrimp shells and the mass of chitin after acid and alkaline treatment (Abdou et al., 2008).
2.3 Determination of protein content
Determination of protein content of WS, AK and CF by Kjeldahl method, Protein content is Nitrogen content×6.25 (Mildenberger et al., 2023).
2.4 Determination of moisture content
Moisture was determined by drying shrimp shells at 105°C until a constant weight was obtained (Kolar, 1992).
2.5 Determination of fat content
Shrimp shells were ground into a particle size of approximately 40 mesh powder, accurately weighed 3g shrimp shell powder, loaded with 2 chronic filter paper made of filter paper tube, and then put the filter paper tube into the Soxhlet extractor extraction tube, connected to the fat flask, was dried to a constant weight, from the condenser tube on the end of the addition of petroleum ether to the flask volume of 2/3, was placed at the bottom of the heated heater flask, so that the extracted liquid was refluxed once every 7 min. The extraction was completed when the extract was picked up with a frosted glass rod and no oil spot remained. After the oil extraction was completed, all extracts were concentrated on a rotameter until no liquid was left in the bottle (Bontzolis et al., 2024). Finally, the spin vials were placed in a desiccator for 30 min to cool to room temperature, and then weighed repeatedly until a constant weight was achieved.
2.6 Determination of ash content
Ash was determined by high temperature scorching method, weighing a certain amount of sample, put in the muffle furnace, the first furnace temperature slowly rise to (250 ± 10) °C, and at this temperature to maintain 60 min, continue to slowly rise to (550 ± 10) °C, and at this temperature burning 2 h. The ash dish was removed from the furnace, placed on an asbestos plate, cooled in air for approximately 5 min, moved to the dryer in the cold to room temperature, and then weighed. Finally, check cauterization was carried out for 30 min each time at a temperature of (550 ± 10) °C until the change in mass was less than 0.0002 g.
The ash content is calculated by the following equation, where mash means the sum of the mass of the ash and the container, mcont is the mass of the container, and mar is the original mass of the shrimp shell powder and the container (Campalani et al., 2024).
2.7 Free amino acid analysis
The shrimp shells of WS, AK, and CF were each 2 g, ground into three centrifugal tubes, added 15% trichloroacetic acid solution 15ml, shaken well, and allowed to stand at room temperature for 30 min. Put all three centrifuge tubes into a centrifuge and centrifuge at 10,000 r/min at 4°C for 10 min, suck up 5 ml of supernatant accurately by pipette, and adjust the pH of the supernatant to about 2.0; then, the three kinds of supernatant were sucked up with a syringe and then packed into sample bottles by 0.22 μm microporous filtration for on-line testing (Zhao et al., 2010).
Analytical conditions: LA8080 Amino Acid Analyzer, column (4.8 mm×160 mm, 7 μm); holding temperature 50°C; flow rate 1: 0.45 mL/min, flow rate 2: 0.30 mL/min, mobile phases citric acid and sodium citrate and ninhydrin buffer.
2.8 Fourier transform infrared spectroscopy analysis
The products obtained at each step of the experiment were characterized using an infrared spectrometer (Ulusal et al., 2024). The samples were analyzed from 500 to 4000 cm-1 Spectra were obtained from 32 scans of dried samples at room temperature with a resolution of 4 cm-1 at intervals of 0.482 cm-1 and background scans were performed for background correction prior to data acquisition.
2.9 X-ray diffraction analysis
The crystalline properties of the three finished chitin products were measured on a Bruker XRD analyzer with the following parameters: λ = 1.5406 Å, voltage 40 kV, current 40 mA, and scanning range 5° to 90°. The crystallinity index was calculated as follows (Hongkulsup et al., 2016).
where I110 is the maximum intensity at 2θ≈20°, and Iam is the intensity of amorphous diffraction peaks at 2θ≈16° (Hongkulsup et al., 2016).
2.10 Thermogravimetric analysis
Thermogravimetric analysis (TGA) was carried out on a STA2500 (Germany - NETZSCH) thermogravimetric analyzer by placing 5 mg of the finished chitin in a crucible and increasing the temperature from room temperature to 800°C using nitrogen as a medium (Campalani et al., 2024).
2.11 Scanning electron microscope
The dried samples were ground, a toothpick was dipped into a small amount of sample on the sampler, fixed with double-sided tape, and sent into a coating machine to spray gold to improve the electrical conductivity of the sample surface. The surface morphology of the three shrimp shell raw materials, the three finished chitin products, and 15 processed samples were observed using a scanning electron microscope (Sigma 500) (Wang et al., 2013).
2.12 Data analysis
The experiments were conducted in three parallel and data were analyzed using SPSS software.
3 Result and discussion
3.1 Composition of shrimp shells
It can be seen that the three types of crustacean shells show different morphologies and structures: Antarctic krill exhibits a characteristic multilamellar helical architecture with significantly reduced mean thickness (9.3 ± 1.8μm) compared to the other taxa. This ultralight biomineralization strategy likely represents an evolutionary optimization for pelagic survival in polar ecosystems. The white shrimp demonstrates intermediate exoskeletal thickness (21.6 ± 3.2μm), correlating with its nearshore habitat’s calcium availability. Notably, the Crayfish possesses the thickest cuticle (27.9 ± 2.7μm) featuring corrugated surface morphology.
The separation of chitin from three sources—Antarctic krill, White shrimp, and crayfish—revealed notable differences in the chemical composition of the raw materials, as shown in Table 1. The highest moisture content was observed in Antarctic white shrimp at 11.31%, while the lowest was recorded in Antarctic krill at 9.16%. In terms of ash content, Antarctic krill had the highest value at 21.03%, whereas White shrimp exhibited the lowest at 14.96%. It has been reported that White shrimp has a measured mineral content of 42.7% (dry basis), with a predominant composition of the Ca, C, O ternary system, which is consistent with the carbonate depositional characteristics of the carapace of this species (Choorit et al., 2008). In contrast, Peng et al. found that the apatite phase was the dominant mineral phase in the ash of Antarctic krill crusts by XRD characterization, and its characteristic elemental composition was the Ca-P-O-F ternary system (Peng et al., 2019). In the study of freshwater crustaceans, Dorsa confirmed that calcium carbonate crystals dominate in Crayfish shells, and their three-dimensional structural network provides mechanical support for the shells. It is worth noting that although trace elements such as P, Mg, Fe, and Zn exist only in trace form in the crustacean shell, these metallic elements have important regulatory roles in the physiological and metabolic activities of crustaceans through their participation in enzymatic reactions and ion channel regulation (Dorsa, 1994). Oil content was highest in crayfish at 11.03% and lowest in White shrimp at 2.23%. Additionally, the protein content was the highest in crayfish (46.04%) and lowest in White shrimp (18.85%). Although the chitin content in crayfish was low, its protein content (46.04%) was significantly higher than the average protein content of shrimp shells, which ranges from 29.2% to 34.0% (El Knidri et al., 2018).
The chitin content of raw crustaceans is generally 15-26%, and in the present study, Antarctic krill contained 24.06% chitin, which was much higher than that of the other two shrimp species and also higher than the 5.19% reported by Yuan et al (Yu et al., 2020). From a commercialization perspective, Antarctic krill has a large biomass. Current estimates suggest that its standing biomass is approximately 342–536 million tons (tkinson et al., 2009) and has a high chitin yield, which makes it more suitable for large-scale mass production of chitin than the other two types of shrimp.
3.2 Analysis of free amino acids
Table 2, lists the amino acid composition of shrimp shell materials from three crustaceans, including essential amino acids (EAA, such as His, Thr, Val, Met, Phe, Ile, Leu, Lys) and non-essential amino acids (NEAA, such as Asp, Glu, Ser, Gly, Arg, Ala, Tyr, Cys) (Awasthi et al., 2024). Sixteen amino acids were measured in this study, including eight essential and eight inessential amino acids. The total EAA levels recorded in this study varied from 2.5 μg/g to 0.52 μg/g, while the total NEAA levels ranged from 2.9 μg/g to 0.46 μg/g. Notably, the shrimp shell meal derived from White shrimp exhibited the highest total EAA and NEAA contents among the three types of shrimp shell meal analyze.
FAA (Functional Amino Acids) are a class of biologically active compounds found in fruits and vegetables that play a vital role in human daily life (Nagao and Yamakado, 2016). These amino acids are not only involved in protein synthesis but also play significant roles in metabolic regulation, immune function, and antioxidants. However, the human body is unable to produce essential amino acids (EAA) on its own, and these amino acids must be consumed throughout the diet (Sá et al., 2021). Shrimp shell meal is a natural food source rich in EAA, especially lysine. Shrimp shell meal not only provides high-quality protein but is also rich in minerals and trace elements such as calcium, phosphorus, and zinc, which have positive effects on bone health, immune function, and metabolic regulation. Lysine deficiency can lead to immunodeficiency (Chen et al., 2003), it can be used for the prevention and treatment of herpes labialis, and it can be used orally or applied directly to the skin (Zuraini et al., 2006).
From the amino acid analysis of the shrimp shell powder of the three crustaceans listed in the table, it can be seen that the shrimp shell powder of White shrimp contains 7 essential amino acids, and 6 non-essential amino acids; crayfish shrimp shell powder contains 8 essential amino acids, 6 non-essential amino acids; Antarctic krill shrimp shell powder includes 7 essential amino acids, 8 non-essential amino acids. From the comprehensive degree of amino acids, Antarctic krill shrimp shells contain amino acid species that are the most abundant in the shells of the three crustaceans.
3.3 Deproteination
As shown in Table 3, after three strong alkali treatments, for WS, the recovery of solids was only 35.47%; for AK and Cf, the recovery of solids was 42.25% and 55.02%, and in summary, it can be concluded that CF had the highest recovery of solids after three deproteinization treatments, which may be due to the fact that the shrimp shells of crayfish are thicker and harder than those of the other two crustaceans, resulting in the accessibility of the protein to the NaOH solution. Accessibility is not high and calcium in shrimp shells affects the efficiency of deproteinization as mentioned in Theruvathil K. Sini et al (Sini et al., 2007). Even after three deproteinizations, some of the proteins are still resistant to hydrolysis under alkaline treatment. According to Figure 2, it can be seen that the peaks are still present at 2926 cm-1 and according to Marwa Hamdi et al., the peaks at 2926 cm-1 and 2854 cm-1 are characteristic of protein occurrence (Tolaimate et al., 2003).
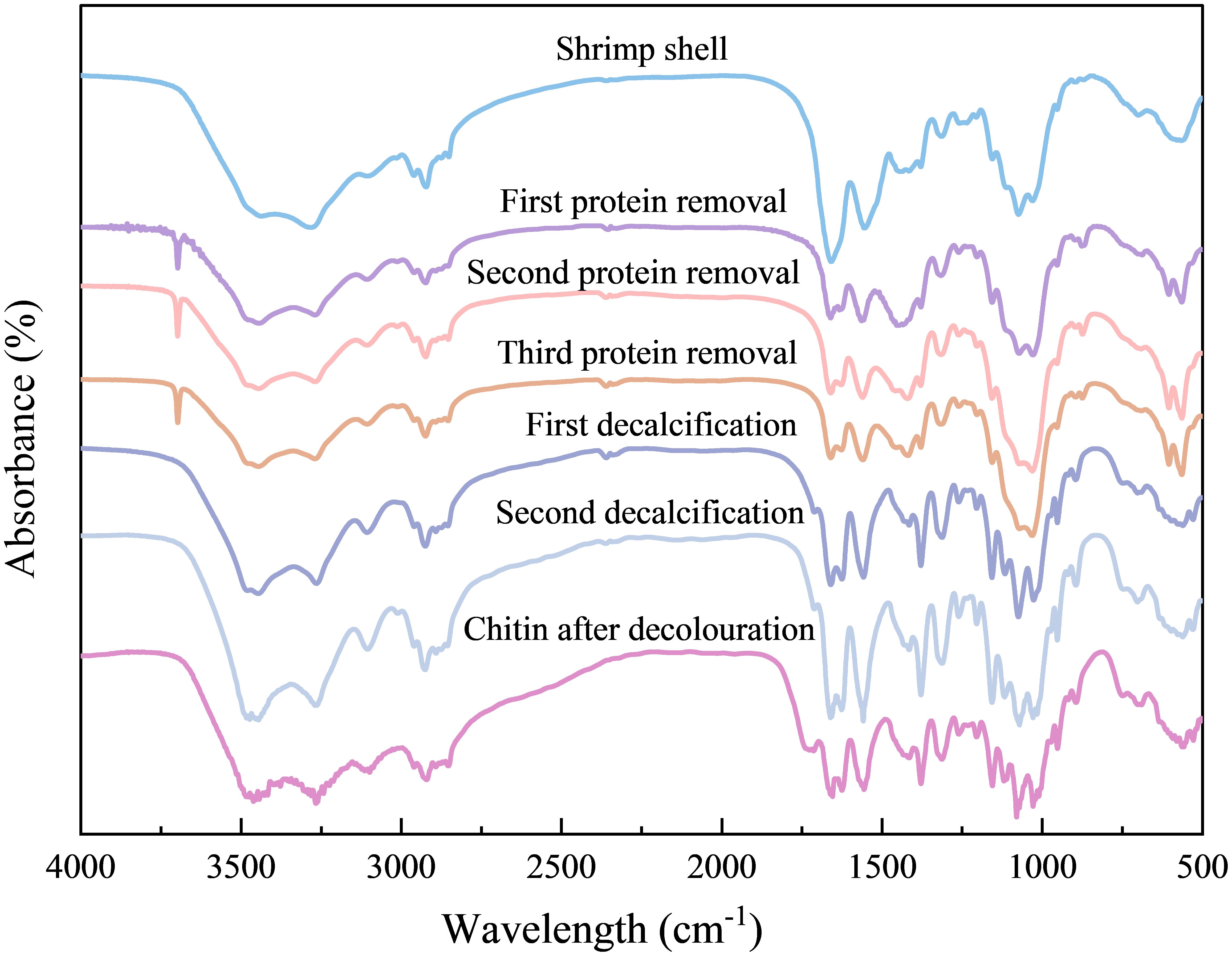
Figure 2. FT-IR spectral changes during chemical processing of Antarctic krill shells. Similar FT-IR spectral trends were observed in all other samples (data not shown).
3.4 Demineralisation
During chitin recovery from shrimp waste, effective demineralization is essential to enhance product quality, as residual minerals not only compromise chitin purity but also impair its functional performance. Mineral content serves as a critical quality parameter, given that high-purity chitin exhibits superior solubility, chemical reactivity, and application-specific functionality (Tolaimate et al., 2003). As shown in Table 3, the acid-treated shrimp shells exhibited progressive dry weight reduction, with removal rates reaching 12.62% (AK), 19.26% (WS), and 39.28% (CF), indicating differential demineralization efficiency among the treatment groups. Bleaching step.
In the shrimp shells after strong acid and alkali treatment, the color has obviously lightened, as shown in Figure 3, the bleaching effect of hydrogen peroxide can make the resulting chitin products whiter, with better sensory effects.
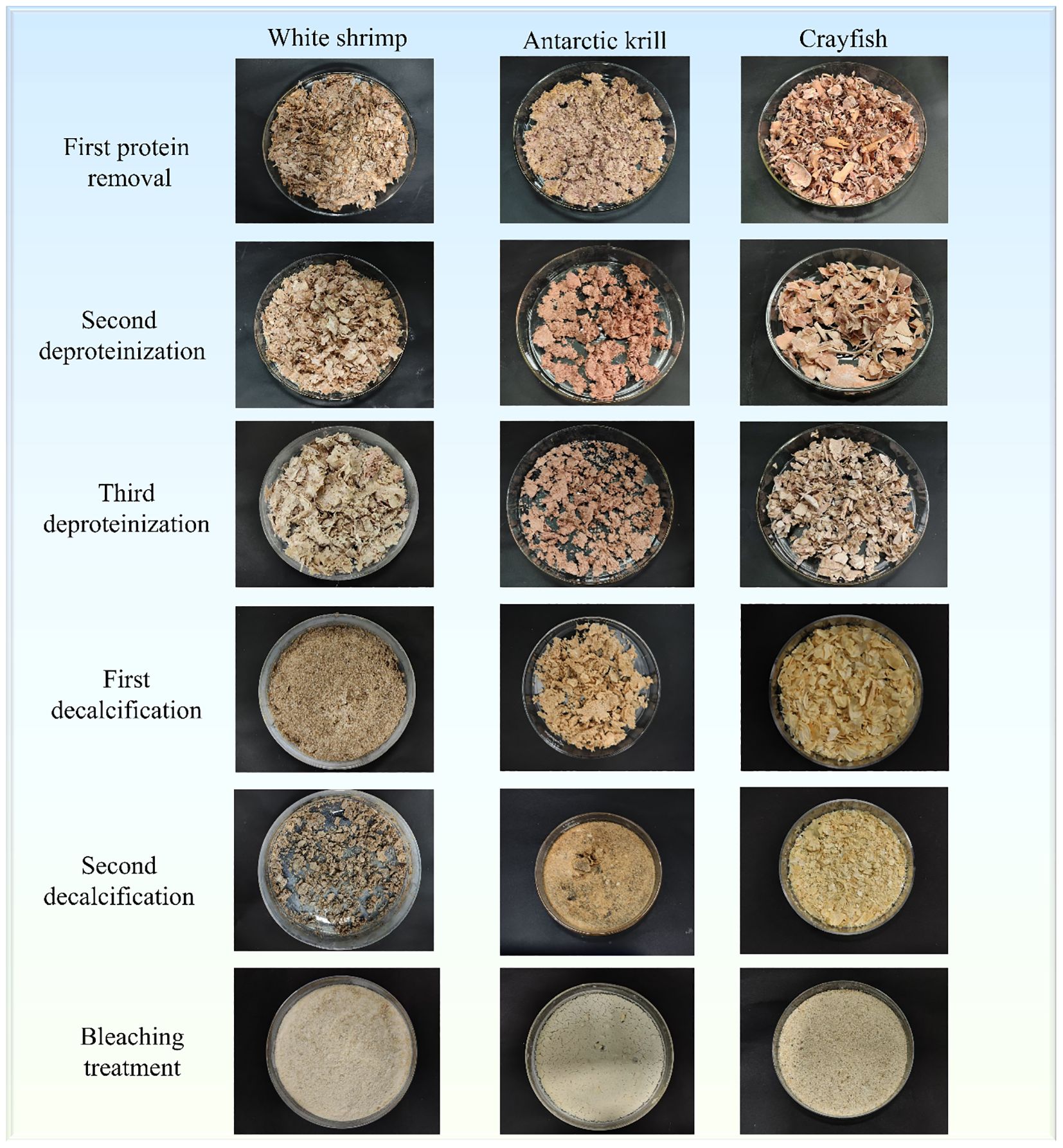
Figure 3. Morphological and color changes in shrimp shells of three crustaceans during chitin extraction process.
3.5 SEM analyses
According to the study, the reticulum is the main structure of chitin, with internally pliable proteins ruleless attached to the chitin skeleton and inorganic compounds firmly anchored in the chitinous interstices (Lavall et al., 2007). At the nanoscale, proteins are usually covalently bound to chitin molecules to form nanofibers. Chitin is a loose and porous structure. In this thesis, SEM scanning electron microscopy was used to observe the structural morphology of shrimp shells after different treatment stages and to speculate on the protein and calcium residues in the shells, and the results were as follows Figure 4.
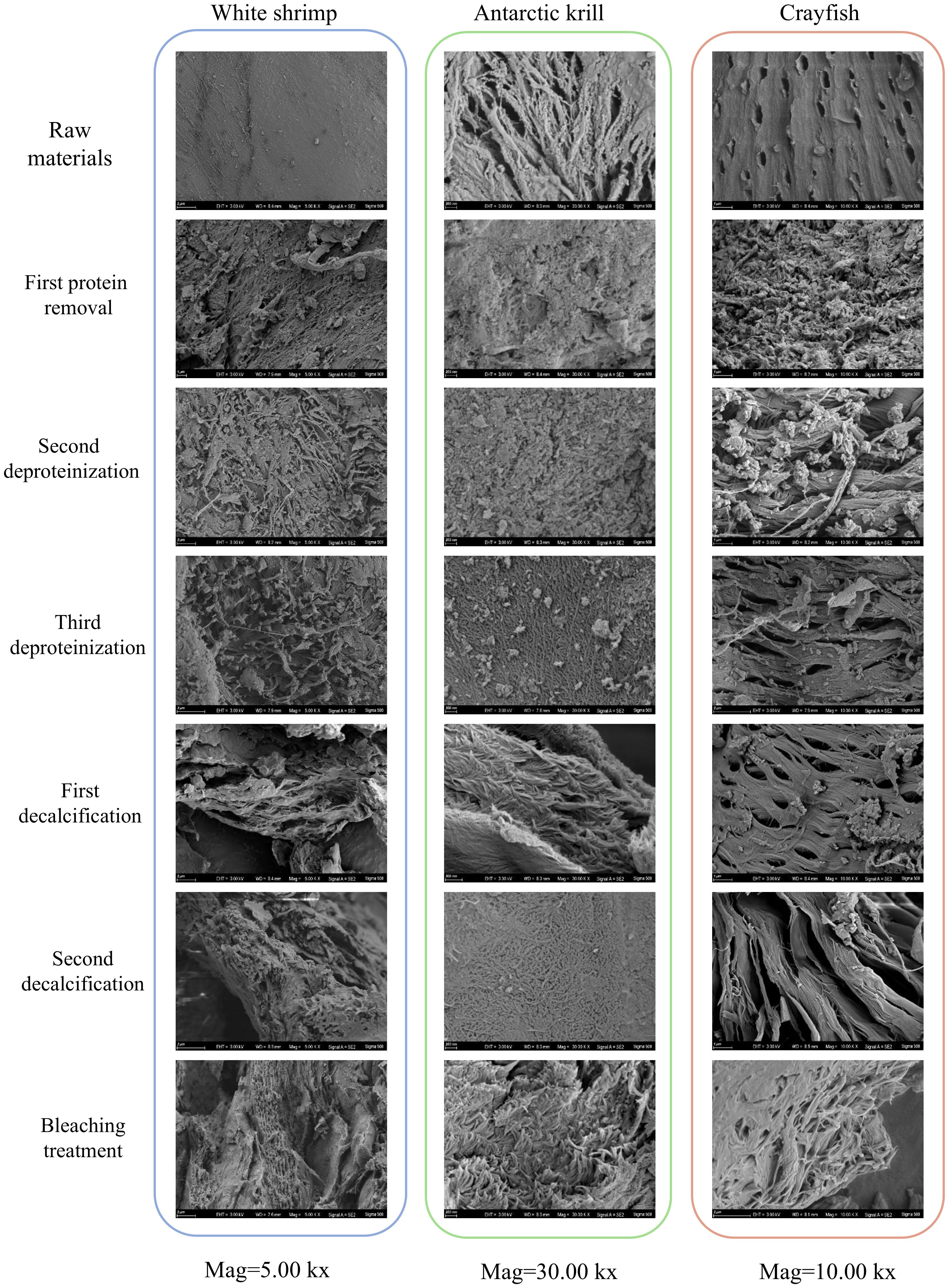
Figure 4. Morphological changes in three shrimp shells in their original form and during treatment by scanning electron microscopy.
From the SEM scanning electron micrographs of shrimp shells of three crustaceans, it can be seen that the surface of the shrimp shell material of White shrimp is dense, the surface of the shrimp shell material of Antarctic krill shows obvious fibrous, and the surface of the shrimp shell material of crayfish has obvious small holes arranged in an orderly manner. After three times of deproteinizations of the shrimp shell material of the three crustaceans, the surface of the shrimp shell appeared to have an obvious porous structure, this is the same as the results of Nidal Daraghmeh et al (Daraghmeh et al., 2015).
Four surface morphology structures of chitin have been identified: lamellar porous structure, microfibrous structure, porous fibrous structure and fractured spongy structure (Yu et al., 2020). In contrast to after two decalcifications, chitin prepared from White shrimp and Antarctic krill showed a lamellar structure, which is similar to the results of Sagheer et al (Xin et al., 2020). Chitin prepared using crayfish was porous and fibrous, suggesting that the surface morphology of chitin may be related to differences in source, several researchers have emphasized the important role of surface morphology in determining the field of application of chitin (Kumar, 2000; Aranaz et al., 2009), the pores on the surface of chitin can effectively enhance its ability to absorb metal ions, and the surface structure of broken fiber-like chitin is often applied to the textile industry, in this paper, three kinds of chitin from different crustaceans have porous fiber-like structure, suitable for application in the textile industry (Yuan et al., 2024).
In general, chitin prepared from crustaceans consists of long, tightly packed fibers (Sagheer et al., 2009; Wang et al., 2013), while the morphological structure of chitin extracted from arthropods consists of nanofibers adhering to each other (Erdogan and Kaya, 2016).
3.6 FT-IR analysis
Structural characterization of chitin extracted from Antarctic krill, White shrimp, and crayfish using infrared spectroscopy, The FTIR absorption spectra are consistent with those reported in the literature, indicating that better quality chitin polymer was obtained. From Figure 5, it can be seen that there is a characteristic band of chitin at 3450 cm-1 indicating the stretching and vibration of O-H, and the stretching vibration peak of N-H at 3267 cm-1 (El Knidri et al., 2016), The peak at 315 cm-1 is a feature peak of the amide III bond, and the characteristic peak of amide II (N-H) is located at 1560 cm-1 (Hu et al., 2007; Greven et al., 2019), the absorption peak at 1028 cm-¹ is caused by the stretching vibration of the -C-O-C- bond in the glucosamine ring (Kumari et al., 2015). The amide I bonds of α-chitin and β-chitin in the infrared spectra show different characteristic absorption peaks. α-chitin has two distinct absorption peaks at 1660 cm-1 and 1625 cm-1, while β-chitin has only one characteristic absorption peak at 1656 cm-1. This difference reflects the difference in the molecular structure of the two chitin species, which may be due to differences in their crystal structures or hydrogen bonding networks. These characteristic absorption peaks can be used as important spectral markers to distinguish α-chitin from β-chitin (Erdogan and Kaya, 2016). It can be seen that the chitin extracted from three different crustaceans in this study were all α-chitin.
3.7 XRD analysis
Crystallinity, as a key indicator of the intrinsic quality of polymers, occupies a pivotal position in the field of materials science. In order to explore the similarities and differences in the crystal structure characteristics of chitin from different sources and to accurately assess the degree of its crystalline perfection, the XRD technique is able to non-destructively reveal the information on the crystal structure of the material, which can provide a key structural basis for the optimization of the design of the material properties (Rinaudo, 2006). XRD an analytical tool, was used in this study to systematically characterize chitin samples extracted from shrimp shells of three different crustaceans. Figure 6 Shows that three finished chitin obtained in this study had two major diffraction peaks at 9.36° (020) and 19.34° (110) (Kadokawa et al., 2011), and two weak diffraction peaks at 12.73° (021), 23.37° (101),26.58° (130) (Zhu et al., 2017), it is these diffraction peaks that typify the crystal structure of α-chitin.
Table 4 shows the crystallinity indices of chitin extracted from three shrimp species - white shrimp (WS), Antarctic krill (AK), and crayfish (CF) were determined as 87, 78, and 82 respectively. Notably, WS and CF exhibited higher crystallinity values compared to AK, indicating interspecies variation in chitin structural organization. This study’s WS crystallinity measurement (87%) aligns closely with the 89.12% reported by Choosak Hongkulsup et al. using chemical extraction methods (Hongkulsup et al., 2016). However, discrepancies emerge in AK comparisons: while Yuan et al. achieved 80.6% crystallinity through lactic acid/enzymatic treatment (Yu et al., 2020), our AK samples showed lower values (78%). Similarly, crystallinity for CF (82%) in this work exceeded the 67.2-80.6% range reported by Ping Zhu et al. using alternative extraction protocols (Zhu et al., 2017). Comparative analysis reveals the WS and CF chitin demonstrates superior mechanical performance potential compared to crustacean counterparts, as evidenced by their higher crystallinity relative to crab chitin (67.8%) and fungal sources (47.6-80.0%) (Ifuku et al., 2011; Hajji et al., 2014). These interspecies differences in crystalline organization may significantly influence material properties for industrial applications.
3.8 Thermogravimetric analysis
Thermal stability can be analyzed by techniques such as TGA, which is a key analytical point for exploring the range of applications of chitin and its secondary products (Villetti et al., 2002). Chitin is a biopolymer that requires high thermal energy to dissociate its crystal structure (Wang et al., 2013), which was analyzed thermogravimetrically in a dynamic nitrogen environment from 20°C to 800°C at a ramp rate of 10°C min-1. The thermogravimetric analysis curves of chitin are shown in Figure 7. The DTG curves for each of the three sources of chitin showed different weight loss processes, with two main peaks for WS and three main peaks for both AK and CF. The mass loss of CF and AK in the 40-140°C interval is mainly due to the removal of adsorbed water, while the weight loss in the 260-400°C interval is attributed to the thermal depolymerization reaction of the polysaccharide main chain Crystalline structure and thermal property characterization of chitin from Antarctic krill (Wang et al., 2013). The mass loss corresponding to the two characteristic peaks in the DTG curve of WS is mainly attributed to the removal of adsorbed water and the thermal degradation process of the polymer backbone (Charii et al., 2024).
The maximum degradation temperature (DTGmax) was 380.7°C for WS, 377.8°C for AK, and 371.5°C for CF. The TGA analytical technique has been reported to determine the conformation of chitin based on the DTGmax value, which is generally higher than 350°C for α-chitin (Greven et al., 2019). Combined FT-IR and XRD results both show that chitin extracted from three crustaceans possesses the structural features of α-chitin. Total mass loss was 79% for AK, and 78.22% and 77.49% for WS and CF, respectively. These results indicate that all three sources of chitin exhibit good thermal stability and can be used as an alternative for the production of thermally stable materials.
4 Conclusion
In this study, shrimp shells were deproteinized and decalcified by alternating acid and alkali treatments, and subsequently extracted to obtain high-purity chitin by oxidative bleaching process. Chitin is commonly found in the exoskeletons of various crustaceans. This study examines chitin extracted from three different crustaceans, revealing that distinct sources of chitin exhibit varying structures. Chitin derived from White shrimp and Antarctic krill presents a fractured sponge-like structure, while chitin from crayfish shows a porous fibrous form. These differences may be attributed to the morphological characteristics of the shrimp shell materials. Although the chitin extracted from White shrimp and Antarctic krill displays similar structural features, the chitin from White shrimp demonstrates superior thermal stability. The study yielded chitin extraction rates of 15.57% from White shrimp, 24.06% from Antarctic krill, and 12.04% from crayfish, with all three chitins classified as α-chitin. The results indicate that the three-step deproteinization, two-step decalcification, and decolorization methods employed in this study effectively removed calcium and protein from shrimp shells. Chitin is a structural polysaccharide, a natural non-toxic carbohydrate known for its biocompatibility, degradability, and high mechanical strength. The increasing global demand for processed shrimp products has led to a rapid accumulation of shrimp by-products. Extracting chitin from these by-products can effectively broaden the application scope of crustacean resources and mitigate marine pollution.
Data availability statement
The original contributions presented in the study are included in the article/supplementary material. Further inquiries can be directed to the corresponding author.
Author contributions
CY: Conceptualization, Writing – original draft. XC: Conceptualization, Writing – review & editing. ZC: Methodology, Writing – review & editing. YZ: Methodology, Writing – review & editing. RY: Data curation, Writing – original draft, Writing – review & editing. YX: Software, Writing – original draft. QZ: Investigation, Writing – review & editing. YH: Project administration, Writing – review & editing. HL: Resources, Writing – review & editing.
Funding
The author(s) declare that financial support was received for the research and/or publication of this article. This study is financially supported by National Key R&D Program of China (No. 2023YFD2401501), Science and Technology Project of Zhoushan (No.2024C03004) and the project of “Research on the extraction of chitin from different shrimp shells and its structural diversity formation mechanism based on different shrimp shells” (NO.2025010).
Acknowledgments
Thanks to National Key R&D Program of China (No. 2023YFD2401501), Science and Technology Project of Zhoushan (No.2024C03004) and the project of “Research on the extraction of chitin from different shrimp shells and its structural diversity formation mechanism based on different shrimp shells”. The authors would like to thank Dean Zuman Chen, Mr. Hao Lan, and Mr. Yadong Zhao for their support and guidance.
Conflict of interest
Author YX was employed by the company Zhoushan Yueyang Food Co., Ltd. Author QZ was employed by the company Zhejiang Xinjiahe Biotechnology Co., Ltd. Author YH was employed by the company Aquatic Products Processing Factory of China National Zhoushan Marine Fisheries Company.
The remaining authors declare that the research was conducted in the absence of any commercial or financial relationships that could be constructed as a potential conflict of interest.
Generative AI statement
The author(s) declare that no Generative AI was used in the creation of this manuscript.
Publisher’s note
All claims expressed in this article are solely those of the authors and do not necessarily represent those of their affiliated organizations, or those of the publisher, the editors and the reviewers. Any product that may be evaluated in this article, or claim that may be made by its manufacturer, is not guaranteed or endorsed by the publisher.
References
Abdou E. S., Nagy K. S. A., Elsabee M. Z. (2008). Extraction and characterization of chitin and chitosan from local sources. Bioresource Technol. 99, 1359–1367. doi: 10.1016/j.biortech.2007.01.051
Aranaz I., Marian M., Harris R., Panos I., Miralles B., Acosta N., et al. (2009). Functional characterization of chitin and chitosan. Curr. Opin. Chem. Biol. 3, 3203–3230. doi: 10.2174/187231309788166415
Awasthi S., Kaushik N., Plaha N. S., Kaur V., Kumar A. (2024). Exploring lipid health indices and protein quality in ninety Indian linseed varieties by comprehensive analysis of fatty acid composition, lignan content, and amino acid composition. Ind Crops Prod. 212, 118366. doi: 10.1016/j.indcrop
Bontzolis C. D., Dimitrellou D., Plioni I., Kandylis P., Soupioni M., Koutinas A. A., et al. (2024). Effect of solvents on aniseed aerial plant extraction using soxhlet and ultrasound methods, regarding antimicrobial activity and total phenolic content. Food Chem. 4, 100609. doi: 10.1016/j.focha.2024.100609
Borić M., Puliyalil H., Novak U., Likozar B. (2018). An intensified atmospheric plasma-based process for the isolation of the chitin biopolymer from waste crustacean biomass. Green Chem. 20, 1199–1204. doi: 10.1039/C7GC03735J
Campalani C., Bertuol I., Bersani C., Calmanti R., Filonenko S., Rodriguez-Padron D., et al. (2024). Green extraction of chitin from hard spider crab shells. Carbohydr Polym. 345, 122565. doi: 10.1016/j.carbpol.2024.122565
Charii H., Boussetta A., Benhamou A. A., Mennani M., Essifi K., Ablouh E. L. H. (2024). Exploring the potential of chitin and chitosan extracted from shrimp shell waste in enhancing urea-formaldehyde wood adhesives. Int. J. Adhes Adhes. 129, 103599. doi: 10.1016/j.ijadhadh.2023.103599
Chen X., Jiang Q., Xu Y., Xia W. (2017). Recovery of Chitin from Antarctic krill (Euphausia superba) Shell Waste by Microbial Deproteinization and Demineralization. J. Aquat. Food Prod. 26, 1210–1220. doi: 10.1080/10498850.2015.1094686
Chen C., Sander J. E., Dale N. M. (2003). The effect of dietary lysine deficiency on the immune response to Newcastle disease vaccination in chickens. Avian Dis. 47, 1346–1351. doi: 10.1637/7008
Choorit W., Patthanamanee W., ManurakChinakorn S. (2008). Use of response surface method for the determination of demineralization efficiency in fermented shrimp shells. Bioresour Technol. 99, 6168–6173. doi: 10.1016/j.biortech.2007.12.032
Cruz F. S., Ernst B., Arata J. A., Parada C. (2018). Spatial and temporal dynamics of the Antarctic krill fishery in fishing hotspots in the Bransfield Strait and South Shetland Islands. Fish Res. 208, 157–166. doi: 10.1016/j.fishres.2018.07.020
Daraghmeh N., Chowdhry B. Z., Leharne S. A., Al Omari M. M., Badwan A. A. (2015). Co-processed chitin-mannitol as a new excipient for Oro-dispersible tablets. MarDrugs. 13, 1739–1764. doi: 10.3390/md13041739
Delezuk J. A. M., Pavinatto A., Campana-Filho S. P. (2019). Influence of the process parameters on β-chitin and α-chitin extraction: probing about the grinding and particles size. Mater Today Proc. 14, 722–732. doi: 10.1016/j.matpr.2019.02.012
Devi R., Dhamodharan R. (2017). Pretreatment in hot glycerol for facile and green separation of chitin from prawn shell waste. ACS Sustain Chem. Eng. 6, 846–853. doi: 10.1021/acssuschemeng.7b03195
Dorsa W. J. (1994). Effects of heat, lactic acid, and modified atmosphere packaging on listeria monocytogenes on cooked crawfish tail meat. doi: 10.31390/gradschool_disstheses.5692
Dou Y., Hu P., Gu P. (2014). Extracting chitin in freshwater crayfish shell with ultrasonic assisted EDTA method. GDAAS. 22, 9. doi: 10.16768/j.issn.1004-874x.2014.22.009
El Knidri H., Belaabed R., Addaou A., Laajeb A., Lahsini A. (2018). Extraction, chemical modification and characterization of chitin and chitosan. Int. J. Biol. Macromol. 120, 1181–1189. doi: 10.1016/j.ijbiomac.2018.08.139
El Knidri H., El Khalfaouy R., Laajeb A., Addaou A., Lahsini A. (2016). Eco-friendly extraction and characterization of chitin and chitosan from the shrimp shell waste via microwave irradiation. Process Saf. Environ. 104, 395–405. doi: 10.1016/j.psep.2016.09.020
Erdogan S., Kaya M. (2016). High similarity in physicochemical properties of chitin and chitosan from nymphs and adults of a grasshopper. Int. J. Biol. Macromol. 89, 118–126. doi: 10.1016/j.ijbiomac.2016.04.059
Ghorbel-Bellaaj O., Hajji S., Younes I., Chaabouni M., Nasri M., Jellouli K. (2013). Optimization of chitin extraction from shrimp waste with Bacillus pumilus A1 using response surface methodology. Int. J. Biol. Macromol. 61, 243–250. doi: 10.1016/j.ijbiomac.2013.07.001
Gildberg A., Stenberg E. (2001). A new process for advanced utilisation of shrimp waste. Process Biochem. 36, 809–812. doi: 10.1016/S0032-9592(00)00278-8
Greven H., Kaya M., Junker K., Akyuz L., Amemiya C. T. (2019). Characterization of tongue worm (Pentastomida) chitin supports α- rather than β-chitin. Zool Anz. 279, 111–115. doi: 10.1016/j.jcz.2019.01.009
Hajji S., Younes I., Ghorbel-Bellaaj O., Hajji R., Rinaudo M., Nasri M., et al. (2014). Structural differences between chitin and chitosan extracted from three different marine sources. Int. J. Bio Macromol. 65, 298–306. doi: 10.1016/j.ijbiomac.2014.01.045
Hamed I., Özogul F., Regenstein J. M. (2016). Industrial applications of crustacean by-products (chitin, chitosan, and chitooligosaccharides): A review. Trends Food Sci. Technol. 48, 40–50. doi: 10.1016/j.tifs.2015.11.007
Hongkulsup C., Khutoryanskiy V. V., Niranjan K. (2016). Enzyme assisted extraction of chitin from shrimp shells (Litopenaeus vannamei). J. Chem. Technol. Biotechnol. 91, 1250–1256. doi: 10.1002/jctb.4714
Hu X., Du Y., Tang Y., Wang Q., Feng T., Yang J., et al. (2007). Solubility and property of chitin in NaOH/urea aqueous solution. Carbohydr Polym. 70, 451–458. doi: 10.1016/j.carbpol.2007.05.002
Ifuku S., Nomura R., Morimoto M., Saimoto H. (2011). Preparation of chitin nanofibers from mushrooms. Materials 4, 1417–1425. doi: 10.3390/ma4081417
Kadokawa J. I., Hirohama K., Mine S., Kato T., Yamamoto K. (2011). Facile preparation of chitin/cellulose composite films using ionic liquids. J. Polym Environ. 20, 37–42. doi: 10.1007/s10924-011-0331-3
Kim Y. J., Park R. D. (2015). Progress in bioextraction processes of chitin from crustacean biowastes. J. Korean Soc. Appl. Biol. Chem. 58, 545–554. doi: 10.1007/s13765-015-0080-4
Kolar K. (1992). Gravimetric determination of moisture and ash in meat and meat products: NMKL interlaboratory study. J. AOAC Int. 75, 1016–1022. doi: 10.1093/jaoac/75.6.1016
Kumar M. N. V. R. (2000). A review of chitin and chitosan applications. React Funct. Polym. 46, 1–27. doi: 10.1016/S1381-5148(00)00038-9
Kumari S., Rath P., Sri Hari Kumar A., Tiwari T. N. (2015). Extraction and characterization of chitin and chitosan from fishery waste by chemical method. Environ. Technol. 3, 77–85. doi: 10.1016/j.eti.2015.01.002
Lavall R. L., Assis O. B. G., Campana-Filho S. P. S. (2007). β-Chitin from the pens of Loligo sp.: Extraction and characterization. Bioresource Technol. 98, 2465–2472. doi: 10.1016/j.biortech.2006.09.002
Marsh R. S., Moe C., Lomneth R. B., Fawcett J. D., Place A. (2001). Characterization of gastrointestinal chitinase in the lizard Sceloporus undulatus garmani(Reptilia: Phrynosomatidae). C B P. 128, 675–682. doi: 10.1016/S1096-4959(00)00364-X
Marzieh M. N., Zahra F., Tahereh E., Sara K. N. (2019). Comparison of the physicochemical and structural characteristics of enzymatic produced chitin and commercial chitin, Int J Biol. Macromol. 139, 270–276. doi: 10.1016/j.ijbiomac.2019.07.217
Mildenberger J., Bruheim I., Solibakke P., Atanassova M. (2023). Development of a protein concentrate for human consumption by direct enzymatic hydrolysis of antarctic krill (Euphausia superba). Lwt. 173, 114254. doi: 10.1016/j.lwt.2022.114254
Muzzarelli R. A. A., Boudrant J., Meyer D., Manno N., DeMarchis M., Paoletti M. G. (2012). Current views on fungal chitin/chitosan, human chitinases, food preservation, glucans, pectins and inulin: A tribute to Henri Braconnot, precursor of the carbohydrate polymers science, on the chitin bicentennial. Carbohydr Polym. 87, 995–1012. doi: 10.1016/j.carbpol.2011.09.063
Nagao K., Yamakado M. (2016). The role of amino acid profiles in diabetes risk assessment. Curr. Opin. Clin. Nutr. Metab. Care 19, 328–335. doi: 10.1097/MCO.0000000000000305
Nguyen T. T., Zhang W., Barber A. R., Su P., He S. (2015). Microwave-intensified enzymatic deproteinization of Australian rock lobster shells (Jasus edwardsii) for the efficient recovery of protein hydrolysate as food functional nutrients. Food Bioproces tech 9, 628–636. doi: 10.1007/s11947-015-1657-y
Peng Y., Ji W., Zhang D., Ji H., Liu S. (2019). Composition and content analysis of fluoride in inorganic salts of the integument of Antarctic krill (Euphausia superba). Sci. Rep. 9, 7853. doi: 10.1038/s41598-019-44337-6
Qian C., Wu W., Ji B. (2012). Progress on the extraction of chitin by microbial fermentation. Food Sci. Technol. 37, 40–44. doi: 10.13684/j.cnki.spkj.2012.03.024
Qin Y., Lu X., Sun N., Rogers R. D. (2010). Dissolution or extraction of crustacean shells using ionic liquids to obtain high molecular weight purified chitin and direct production of chitin films and fibers. Green Chem. 12, 968–971. doi: 10.1039/C003583A
Rinaudo M. (2006). Chitin and chitosan: Properties and applications. Prog. Polym Sci. 31, 603–632. doi: 10.1016/j.progpolymsci.2006.06.001
Sá A. G. A., da Silva D. C., Pacheco M. T. B., Moreno Y. M. F., Carciofi B. A. M. (2021). Oilseed by-products as plant-based protein sources: Amino acid profile and digestibility. Future Foods. 3, 100023. doi: 10.1016/j.fufo.2021.100023
Sagheer F. A. A., Al-Sughayer M. A., Muslim S., Elsabee M. Z. (2009). Extraction and characterization of chitin and chitosan from marine sources in Arabian Gulf. Carbohy Polym. 77, 410–419. doi: 10.1016/j.carbpol.2009.01.032
Sini T. K., Santhosh S., Mathew P. T. (2007). Study on the production of chitin and chitosan from shrimp shell by using Bacillus subtilis fermentation. Carbohyd Res. 342, 2423–2429. doi: 10.1016/j.carres.2007.06.028
tkinson A., Siegel V., Pakhomov E. A., Jessopp M. J., Loeb V. (2009). A re-appraisal of the total biomass and annual production of Antarctic krill. Deep Sea Res. PT I 56, 727–740. doi: 10.1016/j.dsr.2008.12.007
Tolaimate A., Desbrieres J., Rhazi M., Alagui A. (2003). Contribution to the preparation of chitins and chitosans with controlled physico-chemical properties. Polym. 44, 7939–7952. doi: 10.1016/j.polymer.2003.10.025
Ulusal H., Ulusal F., Dagli S., Toprak C. (2024). Extraction of malva sylvestris seed oil by soxhlet method and alginate/chitosan microencapsulation with a new method: Investigation of its cytotoxic effects on human neuroblastoma cell line (SH-SY5Y). J. Radiat. Res. Appl. SC. 17, 100875. doi: 10.1016/j.jrras.2024.100875
Villetti M. A., Crespo J. S., Soldi M. S., Pires A. T. N., Borsali R., Soldi V. (2002). Thermal degradation of natural polymers. J. Therm Anal. Calorim. 67, 295–303. doi: 10.1023/A:1013902510952
Wang Y., Chang Y., Yu L., Zhang C., Xu X., Xue Y., et al. (2013). Crystalline structure and thermal property characterization of chitin from Antarctic krill (Euphausia superba). Carbohydr Polym. 92, 90–97. doi: 10.1016/j.carbpol.2012.09.084
Xin R., Xie W., Xu Z., Che H., Zheng Z., Yang X. (2020). Efficient extraction of chitin from shrimp waste by mutagenized strain fermentation using atmospheric and room-temperature plasma. Int. J. Biol. Macromol. 155, 1561–1568. doi: 10.1016/j.ijbiomac.2019.11.133
Xu Y., Bajaj M., Schneider R., Grage S. I., Ulrich A. S., Winter J., et al. (2013). Transformation of the matrix structure of shrimp shells during bacterial deproteination and demineralization. Microb Cell FACT. 12, 90. doi: 10.1186/1475-2859-12-90
Yu Y., Liu X., Miao J., Leng K. (2020). Chitin from Antarctic krill shell: Eco-preparation, detection, and characterization. Int. J. Biol. Macromol. 164, 4125–4137. doi: 10.1016/j.ijbiomac.2020.08.244
Yuan B., Yu T., Chen S., Zhang Z., Guo Z., Huang G. X., et al. (2024). Physical and chemical characterization of chitin and chitosan extracted under different treatments from black soldier fly. Int. J. Biol. Macromol. 279, 135228. doi: 10.1016/j.ijbiomac.2024.135228
Zahedi S., Safaei Ghomi J., Shahbazi-Alavi H. (2018). Preparation of chitosan nanoparticles from shrimp shells and investigation of its catalytic effect in diastereoselective synthesis of dihydropyrroles. Ultrason Sonochem. 40, 260–264. doi: 10.1016/j.ultsonch.2017.07.023
Zhao F., Zhuang P., Song C., Shi Z., Zhang L. (2010). Amino acid and fatty acid compositions and nutritional quality of muscle in the pomfret, Pampus punctatissimus. Food Chem. 118, 224–227. doi: 10.1016/j.foodchem.2009.04.110
Zhu P., Gu Z., Hong S., Lian H. (2017). One-pot production of chitin with high purity from South American shells using choline chloride-malonic acid deep eutectic solvent’. Carbohydr Polym. 177, 217–223. doi: 10.1016/j.carbpol.2017.09.001
Keywords: chitin, Antarctic krill, white shrimp, crayfish, structure
Citation: Yang C, Chen X, Chen Z, Zhao Y, Yang R, Xia Y, Zeng Q, He Y and Lan H (2025) Source-dependent variations in chitin: a comparative study on Antarctic krill, white shrimp and crayfish. Front. Mar. Sci. 12:1592331. doi: 10.3389/fmars.2025.1592331
Received: 12 March 2025; Accepted: 11 April 2025;
Published: 06 May 2025.
Edited by:
Haisheng Lin, Guangdong Ocean University, ChinaReviewed by:
Lei Du, East China University of Science and Technology, ChinaRu Jia, Ningbo University, China
Copyright © 2025 Yang, Chen, Chen, Zhao, Yang, Xia, Zeng, He and Lan. This is an open-access article distributed under the terms of the Creative Commons Attribution License (CC BY). The use, distribution or reproduction in other forums is permitted, provided the original author(s) and the copyright owner(s) are credited and that the original publication in this journal is cited, in accordance with accepted academic practice. No use, distribution or reproduction is permitted which does not comply with these terms.
*Correspondence: Hao Lan, bGFuaGFvLjg4OEAxNjMuY29t
†These authors have contributed equally to this work