- 1Department of Neurology, The First People’s Hospital of Xiaoshan District, Xiaoshan Affiliated Hospital of Wenzhou Medical University, Hangzhou, Zhejiang, China
- 2Department of Biomedical Engineering, Wenzhou Medical University, Wenzhou, China
Cancer arises from the uncontrolled proliferation of tumor cells within the body. As the incidence of cancer continues to rise, its treatment has become a critical focus for clinicians. The body possesses a tumor immune surveillance mechanism designed to inhibit the proliferation of tumor cells. When tumor-associated mutant antigens appear on the cell surface, antigen-presenting cells, as dendritic cells, present these specific antigens to immune cells, such as T lymphocytes, thereby promoting an immune response and enhancing the cytotoxic effect on tumor cells. Concurrently, the immune system develops immune memory, akin to the response elicited by vaccination. While traditional diseases like tuberculosis and tetanus can be prevented and treated through vaccination, the development of cancer vaccines remains in its nascent stages. Tumor-specific antigens for cancer vaccines can originate from the patient’s own tumor cells or be generated through mutation. Thus, enhancing the presentation of tumor-specific antigens to immune cells is pivotal in anti-tumor immunotherapy. Advances in nanoscience offer novel approaches to tumor immunotherapy. Nanoparticles (NPs) engineered through nanotechnology have garnered significant attention due to their diverse, favorable, and stable properties. These NPs can effectively encapsulate chemotherapy drugs and proteins, facilitating targeted delivery in vivo. Common NPs carriers include liposomes, polymeric nanoparticles, and metal nanoparticles, among others. The development of intelligent NPs delivery systems can enhance efficient antigen presentation, thereby augmenting tumor immune responses. Tumor-specific antigens can be sourced from tumor cells or generated through mutation. In this review, we summarize current methodologies for obtaining various tumor-specific antigens and discuss how these antigens can be delivered to immune cells via different intelligent NPs to bolster anti-tumor immunity. Additionally, from a clinical translation perspective, we explore the challenges and opportunities associated with enhancing tumor immune responses through the smart NP delivery of cancer vaccines. We aim for this review to inspire new strategies in cancer treatment through the use of intelligent NPs and to advance research in cancer vaccines.
1 Introduction
The human body is composed of cells, and during normal metabolic processes, new healthy cells replace old ones that have aged or become damaged, thereby maintaining the body’s normal functions (Tian et al., 2023; Yi et al., 2023). When certain bodily functions become weakened or impaired, overall health declines, potentially leading to various diseases. The body continuously generates new cells, including cancerous ones (Yi et al., 2023; Lahiri et al., 2023; Duong et al., 2023). During cell proliferation, exposure to carcinogens can induce genetic mutations, resulting in abnormal cell morphology (Lahiri et al., 2023; Bo and Wang, 2023). If the immune system’s functionality is compromised, its ability to recognize and eliminate these mutant cells diminishes, allowing uncontrolled proliferation and eventual tumor formation (Duong et al., 2023; Bo and Wang, 2023; Lim et al., 2023; Meng et al., 2023). Tumors are generally classified as malignant or benign, while benign tumors grow slowly and do not invade surrounding tissues (Lim et al., 2023; Meng et al., 2023; Zhang et al., 2022; Wang, 1999). Clinically, they are often treated surgically, and once completely excised, they typically do not recur, causing minimal harm to the body (Meng et al., 2023). In contrast, malignant tumors proliferate rapidly, evade immune regulation, invade adjacent organs, and are prone to metastasis (Zhang et al., 2022; Wang, 1999; Jiang et al., 2000). Due to their high mutation rates and aggressive growth, traditional treatments such as chemotherapy, radiotherapy, and surgical resection often fail to completely eradicate malignant tumor cells, leading to frequent relapse after a single treatment (Lim et al., 2023; Nomura et al., 2017). Cancer, synonymous with malignant tumors, is characterized by uncontrolled cellular proliferation (Jiang et al., 2020; Lu et al., 2022). In competition with normal cells, cancer cells deplete the body’s nutrients and energy, releasing harmful substances like proteases that damage surrounding tissues, expand their invasive reach, and impair organ function (Trabbic et al., 2021; Molino et al., 2016; Almeida et al., 2015).
The incidence of cancer is rising annually within the population. In 2019, it was estimated that there were 23.6 million new cancer cases globally, with a 95% uncertainty interval ranging from 22.2 to 24.9 million. Excluding nonmelanoma skin cancer, the number of new cases was 17.2 million. Additionally, there were about 10.0 million cancer-related deaths worldwide, with a 95% uncertainty interval of 9.36–10.6 million. The global impact of cancer was further highlighted by an estimated 250 million disability-adjusted life years (DALYs), with a range of 235–264 million (Kocarnik et al., 2022).
Various factors influence cancer development, with gender being a significant biological factor affecting many cancer types. There are notable differences in cancer incidence, prognosis, and mortality between male and female subpopulations. Although lung cancer incidence is consistently higher among males than females in the general population, with a male-to-female ratio ranging from 1.5 to 20, the incidence in females is increasing while it is declining in males in many developed countries (Zhu et al., 2019).
Carcinomas, which are the most common type of cancer, along with most glioblastomas, leukemias, lymphomas, melanomas, and sarcomas, typically appear after the age of 50 in over 90% of cases. Their incidence steadily increases until around 85 years of age. Cancer frequency continues to rise in older individuals until it reaches a plateau between 85 and 90 years, as aging and cancer development share common mechanisms, and other aging-related processes fail to prevent carcinogenesis (Montégut et al., 2024).
Cancer cells typically express immunogenic proteins or glycoproteins, known as antigens, on their surfaces (Montero et al., 2024). The expression of tumor-associated antigens (TAA) increases during oncogenic transformation, whereas it is minimal in normal tissue cells (Buonaguro et al., 2011; M Candeias and S Gaipl, 2016; Liu et al., 2022; Srivatsan et al., 2014). Clinically, common TAA used for detection include carcinoembryonic antigen (CEA) and alpha-fetoprotein (AFP) (Barrett, 2024). Tumor-specific antigens are abnormal proteins resulting from genetic mutations in tumor cells, unique to these cells and absent in normal tissues. These proteins are highly immunogenic, making them more readily recognized by immune cells (Barrett, 2024; Riccardo et al., 2017; Fan et al., 2023; Syn et al., 2016). Consequently, research on tumor-specific antigens offers novel approaches and insights for tumor immunotherapy. By designing and presenting tumor-specific antigens to immune cells, the body’s immune function can be enhanced (Schlom and Gulley, 2018).
NPs are ultrafine particles with diameters on the nanometer scale that can be synthesized artificially (Knutson and Disis, 2005; Diao and Liu, 2023; Ou et al., 2023). Due to their advantageous physical properties, such as size, shape, potential, and structure, they have garnered significant interest from researchers (Wu M. et al., 2023). Currently, NPs are primarily categorized into three types: lipid-based nanoparticles, inorganic nanoparticles, and polymer nanoparticles (Table 1). These NPs serve as carriers to modulate the release rate of chemical drugs during in vivo delivery (Igarashi and Sasada, 2020; Bräunlein and Krackhardt, 2017; Duan, 2018). Additionally, they can modify drugs to enhance protection, improve delivery efficiency, and minimize drug loss in the physiological environment during the delivery process (Türeci et al., 2018; Mai et al., 2020). Furthermore, NPs can interact with proteins, influencing their function; for instance, the binding of certain metal NPs to proteins can disrupt protein structure (Jiang et al., 2015). NPs can also facilitate cell communication by delivering therapeutic proteins to cells (Chen et al., 2024; Cao et al., 2018). Moreover, they can modify antigen molecules on their surface to enhance the immunogenicity of the overall structure (Ahn et al., 2014). Consequently, NPs have gained considerable attention in the field of tumor immunotherapy. They deliver tumor-specific antigens to enhance the immune system’s ability to target tumor cells, offering a novel approach to tumor immunotherapy (Nandi et al., 2024; Guasp et al., 2024).
The interaction between NPs and tumor-specific antigens is analogous to vaccine construction (Kranz et al., 2016; Forchhammer et al., 2024; Sahin et al., 2020). Initially, tumor-specific antigens are screened, relevant protein information is analyzed, pathogenic mechanisms are studied, and suitable antigens are selected as components of cancer vaccines (Grego et al., 2020). Subsequently, the vaccine structure is designed to modify the cancer vaccine by binding it to NPs and processing it in various ways to assess antigen protein activity and function (Malyala and Singh, 2010; Zhao et al., 2018; Yang et al., 2020). Finally, the safety and reliability of the cancer vaccine are verified in vivo, its immunogenicity is tested, and its ability to induce an immune response is evaluated (Cyganowski et al., 2018; Brinas et al., 2012). Once the tumor-specific antigens in cancer vaccines are recognized by the immune system in vivo, tumor immune memory is generated, akin to the mechanism of traditional commercial vaccines (Kamei et al., 1999; Jalali et al., 2012; Gong et al., 2024). Due to the clinical translation requirements of cancer vaccines, clinical trials of varying scales are conducted. The clinical outcomes are primarily assessed in terms of safety and efficacy and are reported to regulatory authorities (Luo et al., 2024; Wu C. et al., 2023; Ma et al., 2023). Upon passing the qualification review, commercial production and promotion can proceed.
To better comprehend the impact of cancer vaccines on enhancing tumor immune responses in vivo, we delivered tumor cell-derived specific antigens using advanced NPs. In this review, we first examine the current status and challenges of traditional cancer vaccine research, then introduce the types of cancer vaccines designed based on tumor-specific antigens and finally discuss and summarize the methods and strategies of different NPs for delivering cancer vaccines. We hope this review contributes to ongoing cancer vaccine research. Additionally, we introduce existing research on nanotechnology in tumor immunotherapy, analyze the challenges faced by this immunotherapy, and address the difficulties in clinical translation, aiming to provide new insights for developing the next-generation of more efficient cancer vaccines based on tumor-specific antigens.
2 Cancer vaccines development and research
2.1 Traditional cancer vaccines challenges and limitations
Vaccines have historically been a highly effective strategy for preventing infectious diseases (Montero et al., 2024). Traditional cancer vaccines incorporate TAA, which facilitate the immune system’s ability to recognize and target tumor cells (Buonaguro et al., 2011). This mechanism enables the immune system to continuously monitor and eliminate mutated cells, thereby preventing tumor development (Montégut et al., 2024; M Candeias and S Gaipl, 2016). However, over the past few decades, research on traditional cancer vaccines has yielded limited success. Several key challenges have been identified. To begin with, traditional cancer vaccines typically deliver antigens that are common to the majority of patients with the same cancer type. While this approach simplifies development and production thereby making it more cost-effective, it fails to account for individual variations in immune responses (Liu et al., 2022). Tumor cells are heterogeneous, and patients’ immune systems respond differently to the same antigen, limiting the vaccines’ therapeutic efficacy across a diverse population (Srivatsan et al., 2014). In addition, cancer cells possess mechanisms of mutation and immune evasion. When cancer cells mutate, they can alter or suppress their antigen expression, rendering traditional cancer vaccines ineffective, as these vaccines rely on fixed, unmodifiable antigens (Barrett, 2024) (Figure 1). The development of new blood vessels is crucial because the growth and metastatic spread of cancer cells rely on a sufficient supply of oxygen and nutrients, as well as the efficient removal of waste products (Nishida et al., 2006). Tumor angiogenesis is a hallmark of cancer progression, and increasing evidence suggests that it also induces immunosuppressive microenvironment and anti-tumor immune evasion (Majidpoor and Mortezaee, 2021).
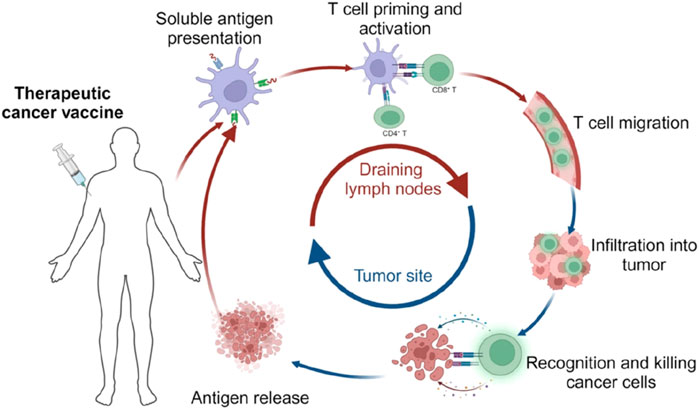
Figure 1. The cancer vaccine mechanism in vivo. [copyright acquired from Fan et al. (2023)].
Additionally, traditional cancer vaccines face the issue of immune tolerance (Fan et al., 2023). Due to prolonged exposure to a single tumor-associated antigen, patients may develop immune tolerance, wherein the immune system becomes desensitized to the antigen. This further diminishes the effectiveness of traditional cancer vaccines over time.
As a result, it is more effective to focus on autologous tumor cell-derived cancer vaccines, which can harness the individual heterogeneity and complexity of tumors to develop more personalized and effective treatment strategies (Fan et al., 2023; Syn et al., 2016). This approach leverages the unique antigenic profile of a patient’s tumor cells, allowing for a more tailored immune response that is better suited to overcoming the specific challenges of each tumor.
2.2 Advantages and involved technologies of tumor cell-derived antigens
Cancer vaccines are a critical component of cancer immunotherapy (Schlom and Gulley, 2018). Compared to traditional cancer vaccines, those based on tumor cells have garnered significantly more attention (Igarashi and Sasada, 2020). Unlike other immunotherapies that target specific antigens, tumor cell-derived vaccines offer the advantage of carrying a comprehensive array of both known and unknown tumor antigens and circumventing major histocompatibility complex (MHC) restrictions (Srivatsan et al., 2014). This characteristic helps prevent the immune escape of tumor cells, which occurs when certain antigens are lost during tumor progression (Knutson and Disis, 2005). Additionally, tumor cell-derived cancer vaccines encompass tumor neoantigens—antigenic foreign bodies generated by mutations in tumor cells (Bräunlein and Krackhardt, 2017). The emergence of neoantigens enables the body’s immune system, including CD4+ and CD8+ T cells, to mount anti-tumor responses through immune surveillance (Bräunlein and Krackhardt, 2017). The incorporation of tumor neoantigens in cancer vaccines provides a novel strategy for harnessing the body’s immune system to combat tumors (Diao and Liu, 2023). By recognizing and eliminating tumor-associated specific antigens, the immune system effectively targets and destroy tumor cells, inhibit their growth, and ultimately control malignant tumors (Igarashi and Sasada, 2020). This approach has the potential to extend the survival of patients with malignant tumors and may even lead to curative outcomes.
The primary factor influencing cancer vaccine efficacy is the presence of tumor cell-derived antigens (Schlom and Gulley, 2018). When these antigens successfully trigger a specific immune response, the use of immune adjuvants can amplify the immune response cascade, facilitating the efficient uptake of the cancer vaccine by antigen-presenting cells (APCs) and the robust activation of specific T cells (Knutson and Disis, 2005). Consequently, optimizing and designing antigens through advanced technological approaches is a crucial step in the development of effective cancer vaccines, as it ensures a potent and targeted immune response (Zanetti, 2017).
Therefore, we can enhance the therapeutic effectiveness of cancer vaccines derived from tumor cells through various engineering strategies (Sobhani et al., 2022). The current approaches include genetically modifying cell surface protein expression by introducing genes to boost the immunogenicity of tumor vaccines; modifying the cell surface by attaching functionalized groups to increase the targeting specificity and immunogenicity of the vaccines; and loading internal cargo, such as antigens and adjuvants, into tumor cell-derived vaccines (Sun et al., 2023). These engineering strategies significantly improve the immunogenicity of tumor vaccines (Figure 2).
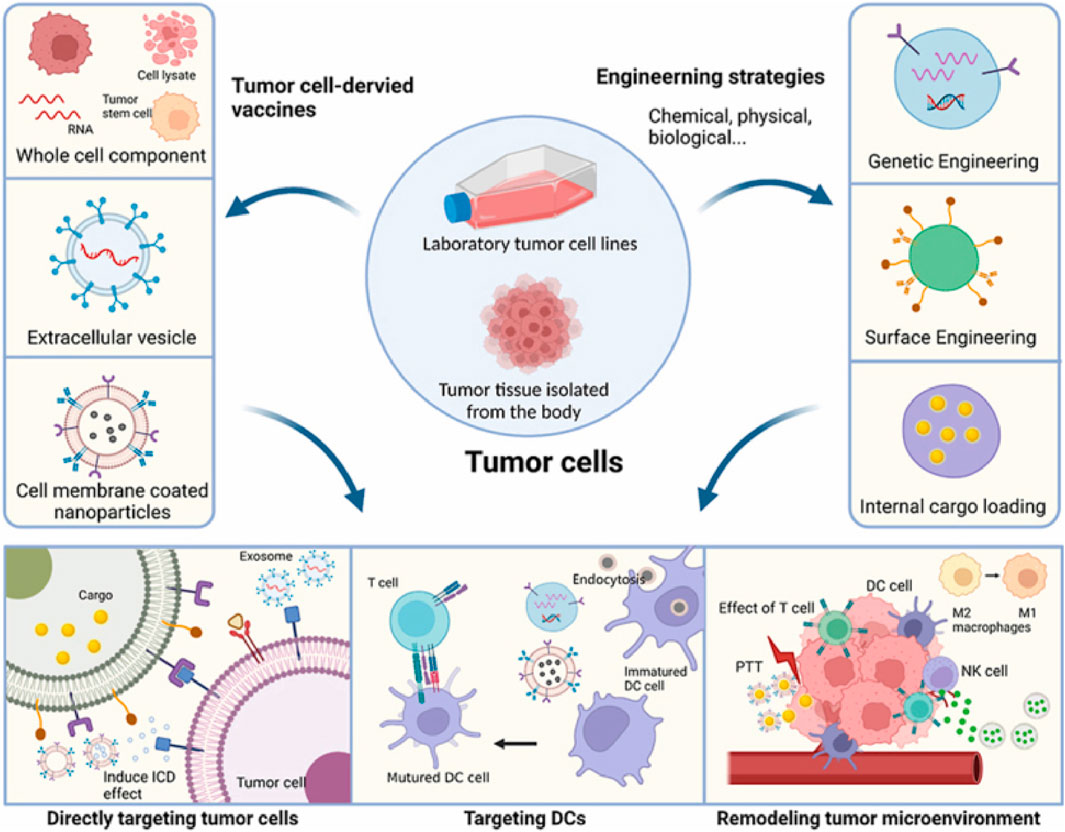
Figure 2. Illustration of novel strategies for the development of engineered tumor cell-derived antigens vaccines. [copyright acquired from Zhang et al. (2023)].
Given the heterogeneity of tumors, cryogenic freezing technology is widely accepted as an effective method to preserve TAA (Schlom and Gulley, 2018). Specifically, tumor cells are harvested from individual tumor tissues, and inactivated cancer cells can be utilized as cancer vaccines while maintaining the intact structure of the tumor cells through processes like cryopreservation and liquid nitrogen freezing (Knutson and Disis, 2005). This approach enables the preservation of all cancer cell antigens, thereby facilitating the activation of a broad spectrum of cancer cell-specific immune responses.
Nanotechnology provides a comprehensive platform to address these challenges by enhancing antigen uptake, facilitating antigens presentation, and augmenting immune system activation (Figure 3). NPs have become pivotal in the intersection of nanotechnology and cancer therapy. These nanoscale carriers, capable of encapsulating therapeutic agents such as drugs or genes, offer several advantages over conventional treatments (Lahiri et al., 2023). Notably, these advantages include precise targeting of cancer cells, minimized damage to healthy cells, and increased efficacy of therapeutic payloads (Duong et al., 2023). The advancement of NPs-based targeting of cancer cells is realized through two primary strategies: passive targeting and active targeting. Passive targeting leverages the unique properties of tumor cells, such as their permeable vasculature, to facilitate the accumulation of nanocarriers in the tumor microenvironment (Yi et al., 2023; Duong et al., 2023). In contrast, active targeting involves the modification of nanocarrier surfaces with specific targeting ligands that bind to receptors on cancer cell surfaces, enabling precise and enhanced cellular interaction (Bo and Wang, 2023).
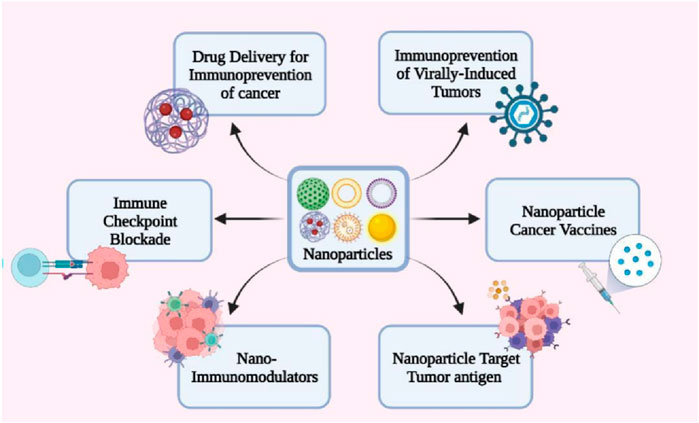
Figure 3. Nanotechnology applications in cancer immunoprevention. [copyright acquired from Koyande et al. (2022)].
Furthermore, when specific genes in cells undergo mutations that lead to cancer, Clustered Regularly Interspaced Short Palindromic Repeats (CRISPR), and CRISPR-associated protein 9 (CRISPR-Cas9), as an innovative scientific technology, can be employed to directly correct these mutations within the patient’s cells (Allemailem et al., 2022). CRISPR-Cas9 represents a groundbreaking advancement in gene editing technology. It operates by binding to and locating specific DNA regions through a CRISPR array that identifies target sites, guided by CRISPR RNA (crRNA) to facilitate DNA double-strand cleavage (Selvakumar et al., 2022). This technology has the potential to correct gene mutations in tumor cells at their source, offering a novel approach to cancer treatment (Wang et al., 2022). However, this method of gene therapy remains in the experimental phase, and significant challenges persist in achieving the commercialization necessary for personalized cancer treatment (Rasul et al., 2022).
3 Smart NPs for cancer vaccines delivery
NPs and exosomes are emerging as powerful tools in various fields, particularly in medicine and biotechnology. NPs, due to their small size and customizable properties, are widely used for targeted drug delivery, enhancing the solubility and stability of therapeutic agents, and improving imaging techniques for diagnostics (Singh and Lillard, 2009). They can be engineered to deliver drugs directly to specific cells or tissues, minimizing side effects and improving treatment efficacy (Hsu et al., 2023). Exosomes, which are naturally occurring extracellular vesicles, play a crucial role in cell-to-cell communication and have potential applications in cancer diagnostics and therapeutics (Li et al., 2025). They can be harnessed to deliver biomolecules such as proteins, RNA, and DNA, offering a natural and biocompatible delivery system, as cancer vaccines (Liu et al., 2019). Additionally, exosomes are being explored as biomarkers for tumor disease diagnosis and prognosis, given their ability to reflect the physiological state of their cells of origin (Kalluri and LeBleu, 2020). Together, NPs and exosomes hold promise for advancing personalized medicine, improving drug delivery systems, and enhancing diagnostic capabilities (Figure 4).
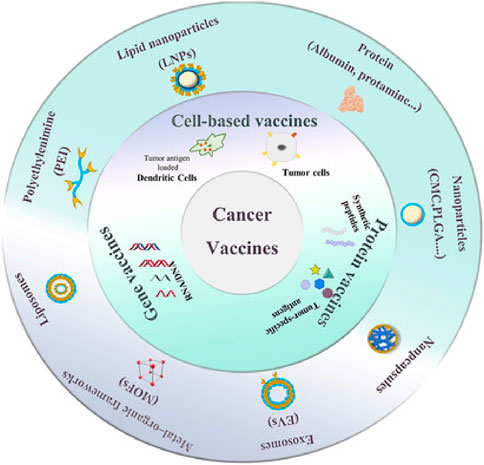
Figure 4. Application of smart nanoparticle delivery in therapeutic cancer vaccines [Adapted with permission from Guo et al. (2023)].
3.1 Liposome nanoparticles
Liposome nanoparticles are based on liposomes, are frequently employed as effective carriers in cancer immunotherapy (Trabbic et al., 2021; Molino et al., 2016). Liposomes are characterized by an amphiphilic phospholipid bilayer and an internal aqueous core structure, making them suitable for carrying nucleic acid drugs such as deoxyribonucleic acid (DNA) or ribonucleic acid (RNA). The delivery of tumor-specific antigens via LNPs can enhance the activation efficiency of the body’s innate tumor immune system, primarily by improving antigen immunogenicity (Mai et al., 2020; Jiang et al., 2015). Recent studies have demonstrated that cationic liposome-polymer hybrid nanosystems (DOTAP-hNP) can induce immunogenic tumor cell death by inhibiting tumor cell-related enzyme activities. This process generates a substantial amount of tumor antigen at the tumor site, facilitating the recognition by antigen-presenting cells and the subsequent tumor eradication by effector cells. Furthermore, LNPs can be used in conjunction with immune checkpoints to synergistically enhance anti-tumor immunotherapy (Chen et al., 2024).
In recent years, Liposome nanoparticles have played a significant role in messenger ribonucleic acid (mRNA) vaccine research. These NPs encapsulate specific mRNA sequences within an inner hydrophilic core, allowing for safe delivery to the target site in vivo without leakage (Mai et al., 2020; Cao et al., 2018). These advantages support the design of various personalized tumor antigens, enabling encoding, packaging, transport, and elicitation of immune responses, thereby advancing the commercialization of cancer vaccines (Figure 5).

Figure 5. Charged protamine-concentrated mRNA forms a stable polycation-mRNA complex, which is subsequently encapsulated within cationic liposomes composed of DOTAP, cholesterol, and DSPE-PEG. [copyright acquired from Mai et al. (2020)].
3.2 Polymeric nanoparticles
Polymeric nanoparticles frequently serve as vehicles for drug delivery and targeting in anti-tumor immunotherapy. These NPs are typically derived from natural or synthetic polymers (Türeci et al., 2018). Their multifunctional nature allows for precise control over drug release, enabling targeted delivery to specific organs or tissue sites. Currently, polymeric nanoparticles commonly utilized in cancer therapy are primarily based on poly-ε-caprolactone (PCL), polylactic acid (PLA), and polylactic-co-glycolic acid (PLGA) (Mai et al., 2020). These materials offer a broad range of raw material sources, a straightforward preparation process, and excellent biocompatibility and stability. The surfaces or interiors of polymeric nanoparticles can be modified and functionalized to meet the design requirements of orthognathic vaccines that deliver tumor-specific antigens (Jiang et al., 2015) (Figure 6).
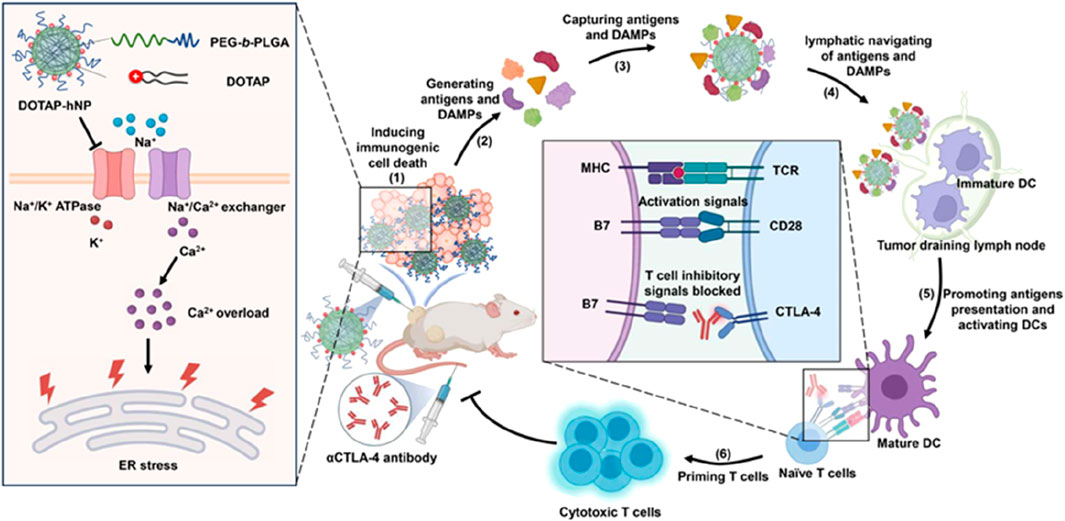
Figure 6. A schematic diagram depicts the cationic lipid-polymer composite NPs driving the in-situ production of tumor antigens and the lymphatic pathway transport to the primary system for anti-tumor immunity. [copyright acquired from Chen et al. (2024)].
Moreover, polymeric nanoparticles can enhance the production of specific antigens at tumor sites by inhibiting the ion exchange channels of tumor cells, thereby capturing existing antigens, activating the body’s tumor immune system, and facilitating the destruction of tumor cells (Mai et al., 2020; Chen et al., 2024). Additionally, polymeric nanoparticles can work synergistically with checkpoint antibodies to enhance the host immune system’s anti-tumor response. Some studies have also demonstrated that polymeric nanoparticles can mimic the physiological behavior of certain pathogens in vivo, amplifying the body’s immune signals and promoting the development of effective protective immune responses during vaccination (Chen et al., 2024). These advantages suggest that polymeric nanoparticles hold significant potential in the design of cancer vaccines incorporating tumor-specific antigens.
3.3 Metallic nanoparticles
Metal nanoparticles can be composed of atomic aggregates from various metals, such as silver and copper (Jiang et al., 2015). The preparation of metal nanoparticles is relatively straightforward, as they can be fabricated with different shapes, diameters, and surface charges using electrochemical methods (Cao et al., 2018) (Figure 7).
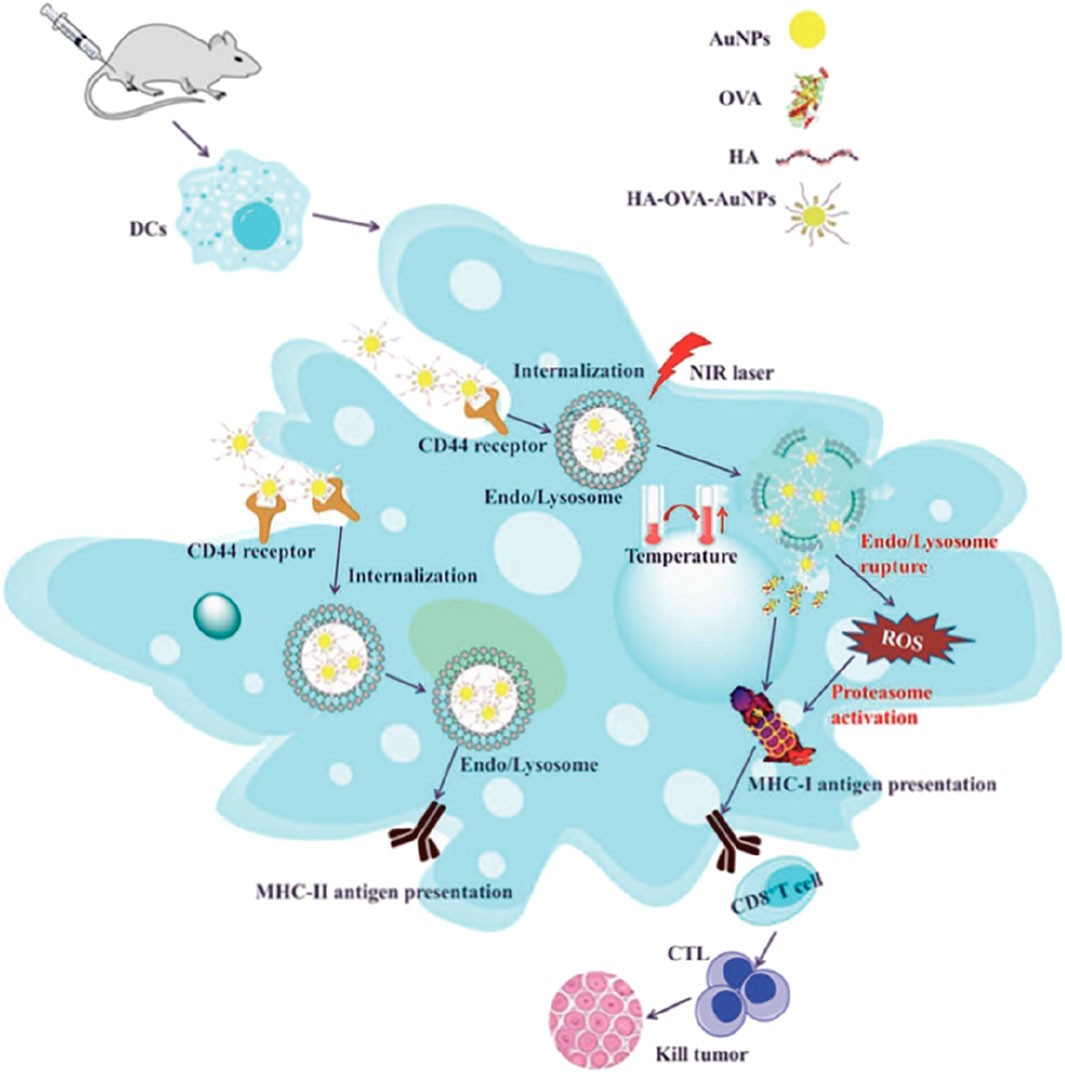
Figure 7. A schematic illustration depicting the enhanced anticancer immune responses elicited by HA-OVA-AuNPs when subjected to laser irradiation. [copyright acquired from Cao et al. (2018)].
Magnetic nanoparticles can be sourced not only from metal elements but also from biological resources such as bacteria, fungi, and plants. These bioresources naturally produce magnetic substances like magnetite (Fe3O4) through biomineralization processes (Winkler et al., 2023). These green, material-derived magnetic nanoparticles are environmentally friendly and hold promise for applications in cancer therapy, particularly hyperthermia. Magnetic hyperthermia (MHT) is an innovative cancer treatment method where magnetic nanoparticles generate localized heat when exposed to an alternating magnetic field (Pérez-Domínguez et al., 2022). This heat can selectively destroy tumor cells while sparing surrounding healthy tissue. Bioderived magnetic nanoparticles are especially effective due to their biocompatibility and their ability to be functionalized for targeted delivery.
Moreover, in targeted drug delivery, imaging, and diagnostics, magnetic nanoparticles can be used to deliver therapy and imaging agents simultaneously, allowing for real-time monitoring of treatment effects (Andreozzi et al., 2025). This provides immediate feedback on treatment outcomes. Magnetic heating techniques offer significant potential for treating deep-seated or inoperable tumors, where precise and localized treatment is crucial (Zhang et al., 2024).
These NPs possess unique physical and chemical properties, including the ability to convert light energy into kinetic energy and kinetic energy into thermal energy (Guasp et al., 2024). Additionally, due to quantum effects, metal nanoparticles exhibit higher surface activity compared to other materials, allowing for functional modification of their surfaces to attach specific functional groups, thereby facilitating drug loading and protein conjugation (Ahn et al., 2014).
Consequently, metal nanoparticles can be combined with tumor-specific antigens and transported through the bloodstream in vivo, enhancing the efficiency of antigen presentation and serving as immune adjuvants (Nandi et al., 2024). Furthermore, due to the distinctive pH, glutathione (GSH), reactive oxygen species (ROS), and physiological conditions present in the tumor microenvironment, metal nanoparticles can respond to physiological and chemical signals within this environment (Cao et al., 2018). As a result, intelligent responsive metal nanoparticle-based cancer vaccines are currently receiving significant attention. These vaccines aim to achieve tumor immunotherapy by responding to multiple complex signals within the tumor microenvironment.
3.4 Exosome
Exosome-derived cancer vaccines represent a promising Frontier in cancer immunotherapy, leveraging the natural properties of exosomes to stimulate an immune response against tumors (Li et al., 2025) (Figures 8A, B). Exosomes are small extracellular vesicles secreted by cells, including cancer cells, and they carry a variety of biomolecules such as proteins, lipids, and nucleic acids. In the context of cancer, exosomes can be engineered or isolated to contain tumor antigens, which are molecules that can trigger an immune response (Liu et al., 2019). These exosome-derived vaccines aim to present these antigens to the immune system in a way that enhances the body’s ability to recognize and attack cancer cells. One of the key advantages of using exosomes is their inherent ability to facilitate communication between cells, which can be harnessed to effectively deliver antigens to immune cells, such as dendritic cells, thereby activating T cells to target the tumor (Kalluri and LeBleu, 2020). Additionally, exosomes have a natural biocompatibility and stability in circulation, which can improve the delivery and efficacy of the vaccine (Zhao et al., 2024). However, challenges remain in standardizing the production and purification of exosomes, ensuring the consistency and potency of the vaccine, and overcoming the immunosuppressive environment often created by tumors (Liang et al., 2024). Despite these hurdles, ongoing research and clinical trials are exploring the potential of exosome-derived vaccines to provide a personalized and effective approach to cancer treatment, offering hope for improved outcomes in patients with various types of cancer (Orooji et al., 2024).
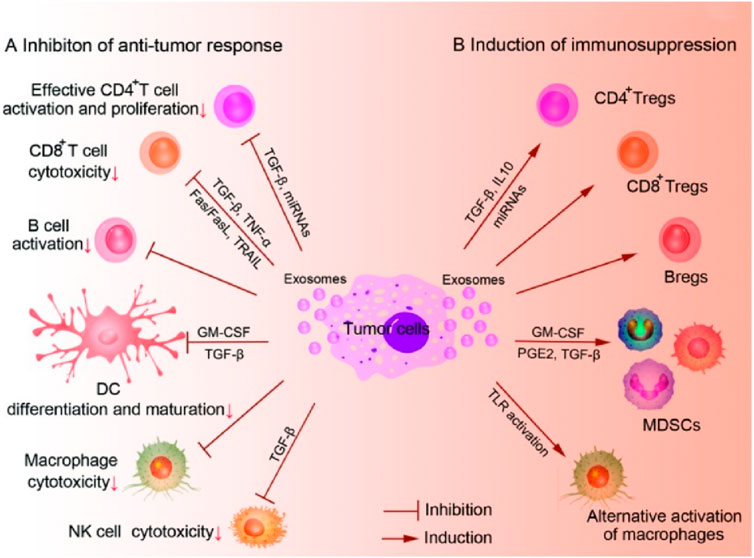
Figure 8. A potential application of exosomes in immunotherapy and cancer vaccines. (A): The role of inhibiting the anti-tumor response. (B): The role of inducing immunosuppression. [copyright acquired from Liu et al. (2015)].
4 Clinical trials
Currently, over 100 biomedical companies are actively engaged in tumor vaccine research and development, with CureVac, BioNTech, and Moderna showing significant progress and holding prominent positions in the industry (Nandi et al., 2024) (Figures 9a–d). BioNTech has a diverse range of research projects, including tumor-associated antigen vaccines (FixVAC®), semi-personalized vaccines (RNA-Warehouse), and personalized vaccines (IVAC®-Mutanome) (Guasp et al., 2024). Moderna primarily focuses on personalized vaccines and tumor neoantigen vaccines that target KRAS mutations.
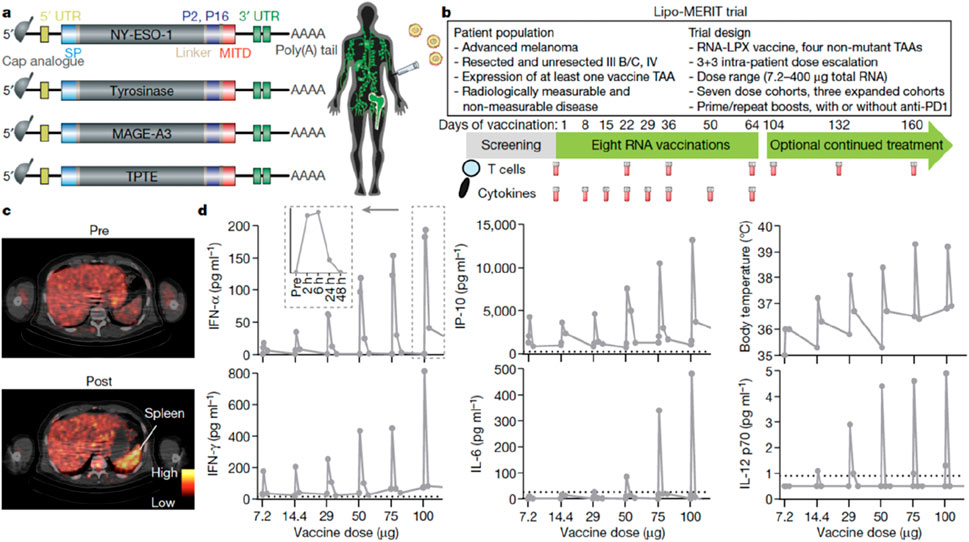
Figure 9. Design of the Clinical Trial and Immune Activation Induced by the Vaccine. (a): the structure of TAA RNA; (b): the design of the clinical trial; (c): the measurement results of the metabolic activity of the spleen; and (d): the plasma cytokine levels and body temperature of the patients. [copyright acquired from Sahin et al. (2020)].
Notably, FixVac is designed to target dendritic cells and utilizes a NP liposomal RNA (RNA-LPX) formulation, which has been shown to promote long-lasting and effective anti-tumor responses in mice following intravenous injection (Kranz et al., 2016). FixVac encodes four TAA: MAGE-A3, tyrosinase, NY-ESO-1, and TPTE. While the expression of these TAAs is limited in normal tissues, they are highly expressed in melanoma, making them capable of triggering strong immune responses (Forchhammer et al., 2024).
According to research findings, FixVac induces a short-term cytokine response by recognizing specific melanoma epitopes and exhibiting a strong cytotoxic effect on tumor cells. Additionally, FixVac may synergize with PD-1 checkpoint inhibitors, potentially enhancing sensitivity to treatment even in cases where there is preexisting resistance to the immune checkpoint inhibitor (Guasp et al., 2024).
5 Conclusion
In conclusion, in this review, we begin by analyzing the current status and challenges associated with traditional cancer vaccine research. We then introduce various types of cancer vaccines that are designed based on tumor-specific antigens. Finally, we discuss and summarize the methods and strategies employed by different NPs for the delivery of cancer vaccines. We aim for this review to contribute to ongoing cancer vaccine research. Additionally, we examine existing research on the application of nanotechnology in cancer immunotherapy, analyze the challenges this form of immunotherapy faces, and explore the difficulties encountered in clinical translation. Our goal is to provide new insights for the development of a new generation of more efficient cancer vaccines based on tumor-specific antigens.
Furthermore, it is essential to conduct further investigations into the immune response mechanisms and potential side effects associated with these vaccines, necessitating comprehensive preclinical and clinical studies. Such efforts are vital to ensuring that these innovative therapies can be safely and effectively applied in clinical settings, ultimately enhancing outcomes for patients receiving cancer treatment.
Author contributions
JQ: Conceptualization, Data curation, Formal Analysis, Investigation, Methodology, Project administration, Software, Supervision, Validation, Writing – original draft, Writing – review and editing. CW: Conceptualization, Data curation, Formal Analysis, Investigation, Methodology, Project administration, Software, Supervision, Validation, Writing – original draft, Writing – review and editing, Resources, Visualization.
Funding
The author(s) declare that no financial support was received for the research and/or publication of this article.
Conflict of interest
The authors declare that the research was conducted in the absence of any commercial or financial relationships that could be construed as a potential conflict of interest.
Generative AI statement
The author(s) declare that no Generative AI was used in the creation of this manuscript.
Publisher’s note
All claims expressed in this article are solely those of the authors and do not necessarily represent those of their affiliated organizations, or those of the publisher, the editors and the reviewers. Any product that may be evaluated in this article, or claim that may be made by its manufacturer, is not guaranteed or endorsed by the publisher.
References
Ahn, S., Lee, I. H., Kang, S., Kim, D., Choi, M., Saw, P. E., et al. (2014). Gold nanoparticles displaying tumor-associated self-antigens as a potential vaccine for cancer immunotherapy. Adv. Healthc. Mater. 3 (8), 1194–1199. doi:10.1002/adhm.201300597
Allemailem, K. S., Alsahli, M. A., Almatroudi, A., Alrumaihi, F., Alkhaleefah, F. K., Rahmani, A. H., et al. (2022). Current updates of CRISPR/Cas9-mediated genome editing and targeting within tumor cells: an innovative strategy of cancer management. Cancer Commun. 42 (12), 1257–1287. doi:10.1002/cac2.12366
Almeida, J. P. M., Lin, A. Y., Figueroa, E. R., Foster, A. E., and Drezek, R. A. (2015). In vivo gold nanoparticle delivery of peptide vaccine induces anti-tumor immune response in prophylactic and therapeutic tumor models. Small 11 (12), 1453–1459. doi:10.1002/smll.201402179
Andreozzi, A., Brunese, L., Cafarchio, A., Netti, P., and Vanoli, G. (2025). Effects of magnetic nanoparticle distribution in cancer therapy through hyperthermia. Int. J. Therm. Sci. 208, 109428. doi:10.1016/j.ijthermalsci.2024.109428
Barrett, B. C. (2024). Developing mutant KRAS targeted vaccines for pancreatic cancer interception. Philadelphia, PA: Drexel University.
Bo, Y., and Wang, H. (2023). Biomaterialbased in situ cancer vaccines. Adv. Mater. 36, 2210452. doi:10.1002/adma.202210452
Bräunlein, E., and Krackhardt, A. M. (2017). Identification and characterization of neoantigens as well as respective immune responses in cancer patients. Front. Immunol. 8, 1702. doi:10.3389/fimmu.2017.01702
Brinas, R. P., Sundgren, A., Sahoo, P., Morey, S., Rittenhouse-Olson, K., Wilding, G. E., et al. (2012). Design and synthesis of multifunctional gold nanoparticles bearing tumor-associated glycopeptide antigens as potential cancer vaccines. Bioconjugate Chem. 23 (8), 1513–1523. doi:10.1021/bc200606s
Buonaguro, L., Petrizzo, A., Tornesello, M. L., and Buonaguro, F. M. (2011). Translating tumor antigens into cancer vaccines. Clin. Vaccine Immunol. 18 (1), 23–34. doi:10.1128/cvi.00286-10
Cao, F., Yan, M., Liu, Y., Liu, L., and Ma, G. (2018). Photothermally controlled MHC class I restricted CD8+ T-cell responses elicited by hyaluronic acid decorated gold nanoparticles as a vaccine for cancer immunotherapy. Adv. Healthc. Mater. 7 (10), 1701439. doi:10.1002/adhm.201701439
Chen, J., Su, M., Xu, C., Cao, Z., Yang, X., and Wang, J. (2024). Cationic lipid-polymer hybrid nanoparticle drives in situ generation and lymphatic navigation of tumor antigens to prime systemic antitumor immunity. Nano Today 57, 102335. doi:10.1016/j.nantod.2024.102335
Cyganowski, P., Dzimitrowicz, A., Jamroz, P., Jermakowicz-Bartkowiak, D., and Pohl, P. (2018). Polymerization-driven immobilization of dc-apgd synthesized gold nanoparticles into a quaternary ammonium-based hydrogel resulting in a polymeric nanocomposite with heat-transfer applications. Polymers 10 (4), 377. doi:10.3390/polym10040377
Diao, L., and Liu, M. (2023). Rethinking antigen source: cancer vaccines based on whole tumor cell/tissue lysate or whole tumor cell. Adv. Sci. 10 (22), 2300121. doi:10.1002/advs.202300121
Duan, H. (2018). Novel therapeutic strategies for solid tumor based on body’s intrinsic antitumor immune system. Cell. Physiology Biochem. 47 (2), 441–457. doi:10.1159/000489979
Duong, H. T. T., Yin, Y., Le, T. M. D., Jeong, J. H., and Lee, D. S. (2023). Highly prolonged release of the cancer vaccine and immunomodulator via a two-layer biodegradable microneedle for prophylactic treatment of metastatic cancer. Biomacromolecules 24 (3), 1209–1219. doi:10.1021/acs.biomac.2c01270
Fan, T., Zhang, M., Yang, J., Zhu, Z., Cao, W., and Dong, C. (2023). Therapeutic cancer vaccines: advancements, challenges, and prospects. Signal Transduct. Target. Ther. 8 (1), 450. doi:10.1038/s41392-023-01674-3
Forchhammer, S., Pop, O. T., Hahn, M., Aebischer, V., Seitz, C. M., Schroeder, C., et al. (2024). Expression of the tumor antigens NY-ESO-1, tyrosinase, MAGE-A3, and TPTE in pediatric and adult melanoma: a retrospective case control study. Virchows Arch. 485, 335–346. doi:10.1007/s00428-024-03846-0
Gong, N., Alameh, M. G., El-Mayta, R., Xue, L., Weissman, D., and Mitchell, M. J. (2024). Enhancing in situ cancer vaccines using delivery technologies. Nat. Rev. Drug Discov. 23 (8), 607–625. doi:10.1038/s41573-024-00974-9
Grego, E. A., Siddoway, A. C., Uz, M., Liu, L., Christiansen, J. C., Ross, K. A., et al. (2020). Polymeric nanoparticle-based vaccine adjuvants and delivery vehicles. Nanoparticles Ration. Vaccine Des. 433, 29–76. doi:10.1007/82_2020_226
Guasp, P., Reiche, C., Sethna, Z., and Balachandran, V. P. (2024). RNA vaccines for cancer: principles to practice. Cancer Cell 42, 1163–1184. doi:10.1016/j.ccell.2024.05.005
Guo, J., Tang, L., Li, K., Ma, Q., Luo, S., Cheng, R., et al. (2023). Application of nanotechnology in therapeutic cancer vaccines. Adv. NanoBiomed Res. 3 (7), 2200122. doi:10.1002/anbr.202200122
Hsu, C. Y., Rheima, A. M., Kadhim, M. M., Ahmed, N. N., Mohammed, S. H., Abbas, F. H., et al. (2023). An overview of nanoparticles in drug delivery: properties and applications. South Afr. J. Chem. Eng. 46, 233–270. doi:10.1016/j.sajce.2023.08.009
Igarashi, Y., and Sasada, T. (2020). Cancer vaccines: toward the next breakthrough in cancer immunotherapy. J. Immunol. Res. 2020 (1), 5825401–5825413. doi:10.1155/2020/5825401
Jalali, S. A., Sankian, M., Tavakkol-Afshari, J., and Jaafari, M. R. (2012). Induction of tumor-specific immunity by multi-epitope rat HER2/neu-derived peptides encapsulated in LPD Nanoparticles. Nanomedicine Nanotechnol. Biol. Med. 8 (5), 692–701. doi:10.1016/j.nano.2011.09.010
Jiang, P. L., Lin, H. J., Wang, H. W., Tsai, W. Y., Lin, S. F., Chien, M. Y., et al. (2015). Galactosylated liposome as a dendritic cell-targeted mucosal vaccine for inducing protective anti-tumor immunity. Acta biomater. 11, 356–367. doi:10.1016/j.actbio.2014.09.019
Jiang, X. P., Yang, D. C., Elliott, R. L., and Head, J. F. (2000). Vaccination with a mixed vaccine of autogenous and allogeneic breast cancer cells and tumor associated antigens CA15-3, CEA and CA125-results in immune and clinical responses in breast cancer patients. Cancer biotherapy & Radiopharm. 15 (5), 495–505. doi:10.1089/cbr.2000.15.495
Jiang, Y., Krishnan, N., Zhou, J., Chekuri, S., Wei, X., Kroll, A. V., et al. (2020). Engineered cell-membrane-coated nanoparticles directly present tumor antigens to promote anticancer immunity. Adv. Mater. 32 (30), 2001808. doi:10.1002/adma.202001808
Kalluri, R., and LeBleu, V. S. (2020). The biology, function, and biomedical applications of exosomes. science 367 (6478), eaau6977. doi:10.1126/science.aau6977
Kamei, H., Koide, T., Koijima, T., Hashimoto, Y., and Hasegawa, M. (1999). Effect of gold on tumor-associated antigens. Cancer biotherapy & Radiopharm. 14 (5), 403–406. doi:10.1089/cbr.1999.14.403
Knutson, K. L., and Disis, M. L. (2005). Tumor antigen-specific T helper cells in cancer immunity and immunotherapy. Cancer Immunol. Immunother. 54, 721–728. doi:10.1007/s00262-004-0653-2
Kocarnik, J. M., Compton, K., Dean, F. E., Fu, W., Gaw, B. L., Harvey, J. D., et al. (2022). Cancer incidence, mortality, years of life lost, years lived with disability, and disability-adjusted life years for 29 cancer groups from 2010 to 2019: a systematic analysis for the global burden of disease study 2019. JAMA Oncol. 8 (3), 420–444. doi:10.1001/jamaoncol.2021.6987
Koyande, N. P., Srivastava, R., Padmakumar, A., and Rengan, A. K. (2022). Advances in nanotechnology for cancer immunoprevention and immunotherapy: a review. Vaccines 10 (10), 1727. doi:10.3390/vaccines10101727
Kranz, L. M., Diken, M., Haas, H., Kreiter, S., Loquai, C., Reuter, K. C., et al. (2016). Systemic RNA delivery to dendritic cells exploits antiviral defence for cancer immunotherapy. Nature 534 (7607), 396–401. doi:10.1038/nature18300
Lahiri, A., Maji, A., Potdar, P. D., Singh, N., Parikh, P., Bisht, B., et al. (2023). Lung cancer immunotherapy: progress, pitfalls, and promises. Mol. cancer 22 (1), 40. doi:10.1186/s12943-023-01740-y
Li, J., Wang, J., and Chen, Z. (2025). Emerging role of exosomes in cancer therapy: progress and challenges. Mol. Cancer 24 (1), 13. doi:10.1186/s12943-024-02215-4
Liang, K., Sun, Y., Xie, L., Liu, Y., You, Y., Xu, J., et al. (2024). Biologically self-assembled tumor cell-derived cancer nanovaccines as an all-in-one platform for cancer immunotherapy. ACS nano 18 (8), 6702–6717. doi:10.1021/acsnano.4c01050
Lim, S., Park, J. H., and Chang, H. (2023). Enhanced anti-tumor immunity of vaccine combined with anti-PD-1 antibody in a murine bladder cancer model. Investigative Clin. Urology 64 (1), 74. doi:10.4111/icu.20220031
Liu, J., Fu, M., Wang, M., Wan, D., Wei, Y., and Wei, X. (2022). Cancer vaccines as promising immuno-therapeutics: platforms and current progress. J. Hematol. Oncol., 15(1): 28, doi:10.1186/s13045-022-01247-x
Liu, S. L., Sun, P., Li, Y., and Lu, Y. (2019). Exosomes as critical mediators of cell-to-cell communication in cancer pathogenesis and their potential clinical application. Transl. Cancer Res. 8 (1), 298–311. doi:10.21037/tcr.2019.01.03
Liu, Y., Gu, Y., and Cao, X. (2015). The exosomes in tumor immunity. Oncoimmunology 4 (9), e1027472. doi:10.1080/2162402x.2015.1027472
Lu, Y., Wu, C., Yang, Y., Chen, X., Ge, F., Wang, J., et al. (2022). Inhibition of tumor recurrence and metastasis via a surgical tumor-derived personalized hydrogel vaccine. Biomaterials Sci. 10 (5), 1352–1363. doi:10.1039/d1bm01596f
Luo, Y., Luo, X., Ru, Y., Zhou, X., Liu, D., Huang, Q., et al. (2024). Copper (II)-based nano-regulator correlates cuproptosis burst and sequential immunogenic cell death for synergistic cancer immunotherapy. Biomaterials Res. 28, 0039. doi:10.34133/bmr.0039
Ma, Y., Luo, Y., Tang, X., Jiang, W., Li, H., and Wang, J. (2023). Ferric iron coordinated cisplatin prodrug reprograms the immune-cold tumor microenvironment through tumor hypoxia relief for enhanced cancer photodynamic-immunotherapy. Nano Res. 16 (7), 9930–9939. doi:10.1007/s12274-023-5919-0
Mai, Y., Guo, J., Zhao, Y., Ma, S., Hou, Y., and Yang, J. (2020). Intranasal delivery of cationic liposome-protamine complex mRNA vaccine elicits effective anti-tumor immunity. Cell. Immunol. 354, 104143. doi:10.1016/j.cellimm.2020.104143
Majidpoor, J., and Mortezaee, K. (2021). Angiogenesis as a hallmark of solid tumors-clinical perspectives. Cell. Oncol. 44, 715–737. doi:10.1007/s13402-021-00602-3
Malyala, P., and Singh, M. (2010). Micro/nanoparticle adjuvants: preparation and formulation with antigens. Methods Protoc. 626, 91–101. doi:10.1007/978-1-60761-585-9_7
M Candeias, S., and S Gaipl, U. (2016). The immune system in cancer prevention, development and therapy. Anti-cancer agents Med. Chem. Former. Curr. Med. Chemistry-Anti-cancer agents 16 (1), 101–107. doi:10.2174/1871520615666150824153523
Meng, S., Whitt, A. G., Stamp, B. F., Eaton, J. W., and Yaddanapudi, K. (2023). Exosome-based cancer vaccine for prevention of lung cancer. Stem Cell Investig. 10, 2. doi:10.21037/sci-2022-030
Molino, N. M., Neek, M., Tucker, J. A., Nelson, E. L., and Wang, S. W. (2016). Viral-mimicking protein nanoparticle vaccine for eliciting anti-tumor responses. Biomaterials 86, 83–91. doi:10.1016/j.biomaterials.2016.01.056
Montégut, L., López-Otín, C., and Kroemer, G. (2024). Aging and cancer. Mol. Cancer 23 (1), 106. doi:10.1186/s12943-024-02020-z
Montero, D. A., Vidal, R. M., Velasco, J., Carreño, L. J., Torres, J. P., Benachi O., M. A., et al. (2024). Two centuries of vaccination: historical and conceptual approach and future perspectives. Front. public health 11, 1326154. doi:10.3389/fpubh.2023.1326154
Nandi, A., Dey, Y. N., Maity, D., and Das, A. (2024). “Vaccine development strategies and impact,” in Diagnosis and analysis of COVID-19 using artificial intelligence and machine learning-based techniques. Academic Press, 149–180.
Nishida, N., Yano, H., Nishida, T., Kamura, T., and Kojiro, M. (2006). Angiogenesis in cancer. Vasc. health risk Manag. 2 (3), 213–219. doi:10.2147/vhrm.2006.2.3.213
Nomura, T., Hirata, K., Shimaoka, T., Yamakawa, M., Koizumi, N., Suzuki, R., et al. (2017). Cancer vaccine therapy using tumor endothelial cells as antigens suppresses solid tumor growth and metastasis. Biol. Pharm. Bull. 40 (10), 1661–1668. doi:10.1248/bpb.b17-00145
Orooji, N., Fadaee, M., Kazemi, T., and Yousefi, B. (2024). Exosome therapeutics for non-small cell lung cancer tumorigenesis. Cancer Cell Int. 24 (1), 360. doi:10.1186/s12935-024-03544-6
Ou, W., Stewart, S., White, A., Kwizera, E. A., Xu, J., Fang, Y., et al. (2023). In-situ cryo-immune engineering of tumor microenvironment with cold-responsive nanotechnology for cancer immunotherapy. Nat. Commun. 14 (1), 392. doi:10.1038/s41467-023-36045-7
Pérez-Domínguez, S., Caballero-Mancebo, S., Marcuello, C., Martínez-Júlvez, M., Medina, M., and Lostao, A. (2022). Nanomechanical study of enzyme: coenzyme complexes: bipartite sites in plastidic ferredoxin-NADP+ reductase for the interaction with NADP+. Antioxidants 11 (3), 537. doi:10.3390/antiox11030537
Rasul, M. F., Hussen, B. M., Salihi, A., Ismael, B. S., Jalal, P. J., Zanichelli, A., et al. (2022). Strategies to overcome the main challenges of the use of CRISPR/Cas9 as a replacement for cancer therapy. Mol. Cancer 21 (1), 64–30. doi:10.1186/s12943-021-01487-4
Riccardo, F., Bolli, E., Macagno, M., Arigoni, M., Cavallo, F., and Quaglino, E. (2017). Chimeric DNA vaccines: an effective way to overcome immune tolerance. Cancer vaccines. 405, 99–122. doi:10.1007/82_2014_426
Sahin, U., Oehm, P., Derhovanessian, E., Jabulowsky, R. A., Vormehr, M., Gold, M., et al. (2020). An RNA vaccine drives immunity in checkpoint-inhibitor-treated melanoma. Nature 585, 107–112. doi:10.1038/s41586-020-2537-9
Schlom, J., and Gulley, J. L. (2018). Vaccines as an integral component of cancer immunotherapy. Jama 320 (21), 2195–2196. doi:10.1001/jama.2018.9511
Selvakumar, S. C., Preethi, K. A., Ross, K., Tusubira, D., Khan, M. W. A., Mani, P., et al. (2022). CRISPR/Cas9 and next generation sequencing in the personalized treatment of Cancer. Mol. Cancer 21 (1), 83. doi:10.1186/s12943-022-01565-1
Singh, R., and Lillard, Jr J. W. (2009). Nanoparticle-based targeted drug delivery. Exp. Mol. pathology 86 (3), 215–223. doi:10.1016/j.yexmp.2008.12.004
Sobhani, N., Scaggiante, B., Morris, R., Chai, D., Catalano, M., Tardiel-Cyril, D. R., et al. (2022). Therapeutic cancer vaccines: from biological mechanisms and engineering to ongoing clinical trials. Cancer Treat. Rev. 109, 102429. doi:10.1016/j.ctrv.2022.102429
Srivatsan, S., Patel, J. M., Bozeman, E. N., Imasuen, I. E., He, S., Daniels, D., et al. (2014). Allogeneic tumor cell vaccines: the promise and limitations in clinical trials. Hum. vaccines & Immunother. 10 (1), 52–63. doi:10.4161/hv.26568
Sun, Y., Tian, Y., Wu, S., Huang, A., Hu, Y., Liao, Z., et al. (2023). Engineering irradiated tumor-derived microparticles as personalized vaccines to enhance anti-tumor immunity. Cell Rep. Med. 4 (12), 101303. doi:10.1016/j.xcrm.2023.101303
Syn, N. L. X., Yong, W. P., Goh, B. C., and Lee, S. C. (2016). Evolving landscape of tumor molecular profiling for personalized cancer therapy: a comprehensive review. Expert Opin. drug metabolism & Toxicol. 12 (8), 911–922. doi:10.1080/17425255.2016.1196187
Tian, Y., Liu, Z., Wang, J., Li, L., Wang, F., Zhu, Z., et al. (2023). Nanomedicine for combination urologic cancer immunotherapy. Pharmaceutics 15 (2), 546. doi:10.3390/pharmaceutics15020546
Trabbic, K. R., Kleski, K. A., and Barchi, Jr J. J. (2021). Stable gold-nanoparticle-based vaccine for the targeted delivery of tumor-associated glycopeptide antigens. ACS bio & med chem Au 1 (1), 31–43. doi:10.1021/acsbiomedchemau.1c00021
Türeci, Ö., Löwer, M., Schrörs, B., Lang, M., Tadmor, A., and Sahin, U. (2018). Challenges towards the realization of individualized cancer vaccines. Nat. Biomed. Eng. 2 (8), 566–569. doi:10.1038/s41551-018-0266-2
Wang, R. F. (1999). Human tumor antigens: implications for cancer vaccine development. J. Mol. Med. 77, 640–655. doi:10.1007/s001099900042
Wang, S. W., Gao, C., Zheng, Y. M., Yi, L., Lu, J. C., Huang, X. Y., et al. (2022). Current applications and future perspective of CRISPR/Cas9 gene editing in cancer. Mol. cancer 21 (1), 57. doi:10.1186/s12943-022-01518-8
Winkler, R., Ciria, M., Ahmad, M., Plank, H., and Marcuello, C. (2023). A review of the current state of magnetic force microscopy to unravel the magnetic properties of nanomaterials applied in biological systems and future directions for quantum technologies. Nanomaterials 13 (18), 2585. doi:10.3390/nano13182585
Wu, C., He, W., Chen, Y., Cai, J., Zeng, F., Lu, Z., et al. (2023b). Personalized bacteria loaded with autoantigens for the enhancement of tumor immunotherapy. Adv. Healthc. Mater. 12 (11), 2203026. doi:10.1002/adhm.202203026
Wu, M., Xiao, Y., Huang, J., Wang, Y., Zhang, Y., Xu, J., et al. (2023a). Liquid nitrogen frozen cells for chemotherapy drug delivery and vaccination of melanoma. J. Cancer Res. Clin. Oncol. 149 (15), 13705–13716. doi:10.1007/s00432-023-05117-y
Yang, Y., Tang, J., Song, H., Yang, Y., Gu, Z., Fu, J., et al. (2020). Dendritic mesoporous silica nanoparticle adjuvants modified with binuclear aluminum complex: coordination chemistry dictates adjuvanticity. Angew. Chem. Int. Ed. 59 (44), 19610–19617. doi:10.1002/anie.202006861
Yi, Y., Yu, M., Li, W., Zhu, D., Mei, L., and Ou, M. (2023). Vaccine-like nanomedicine for cancer immunotherapy. J. Control. Release 355, 760–778. doi:10.1016/j.jconrel.2023.02.015
Zanetti, M. (2017). A second chance for telomerase reverse transcriptase in anticancer immunotherapy. Nat. Rev. Clin. Oncol. 14 (2), 115–128. doi:10.1038/nrclinonc.2016.67
Zhang, X., Cui, H., Zhang, W., Li, Z., and Gao, J. (2023). Engineered tumor cell-derived vaccines against cancer: the art of combating poison with poison. Bioact. Mater., 22: 491–517. doi:10.1016/j.bioactmat.2022.10.016
Zhang, X., Zhang, Y., Zhao, L., Wang, J., Li, J., Wang, X., et al. (2022). Exploitation of tumor antigens and construction of immune subtype classifier for mRNA vaccine development in bladder cancer. Front. Immunol. 13, 1014638. doi:10.3389/fimmu.2022.1014638
Zhang, Z., Du, Y., Shi, X., Wang, K., Qu, Q., Liang, Q., et al. (2024). NIR-II light in clinical oncology: opportunities and challenges. Nat. Rev. Clin. Oncol. 21 (6), 449–467. doi:10.1038/s41571-024-00892-0
Zhao, G., Wang, Y., Xing, S., Jiang, Y., Ding, J., Cai, Y., et al. (2024). Exosome-based anticancer vaccines: from bench to bedside. Cancer Lett. 595, 216989. doi:10.1016/j.canlet.2024.216989
Zhao, Z., Harris, B., Hu, Y., Harmon, T., Pentel, P. R., Ehrich, M., et al. (2018). Rational incorporation of molecular adjuvants into a hybrid nanoparticle-based nicotine vaccine for immunotherapy against nicotine addiction. Biomaterials 155, 165–175. doi:10.1016/j.biomaterials.2017.11.021
Keywords: liposome nanoparticles, polymeric nanoparticles, metallic nanoparticles, smart delivery, cancer vaccines, tumor immunity, clinical translation
Citation: Qiu J and Wu C (2025) Smart nanoparticle delivery of cancer vaccines enhances tumor immune responses: a review. Front. Nanotechnol. 7:1564267. doi: 10.3389/fnano.2025.1564267
Received: 21 January 2025; Accepted: 31 March 2025;
Published: 14 April 2025.
Edited by:
S. Manjura Hoque, Atomic Energy Centre Dhaka, BangladeshReviewed by:
Carlos Marcuello, Instituto de Nanociencia y Materiales de Aragón (INMA), SpainWenshen Wang, Johns Hopkins University, United States
Copyright © 2025 Qiu and Wu. This is an open-access article distributed under the terms of the Creative Commons Attribution License (CC BY). The use, distribution or reproduction in other forums is permitted, provided the original author(s) and the copyright owner(s) are credited and that the original publication in this journal is cited, in accordance with accepted academic practice. No use, distribution or reproduction is permitted which does not comply with these terms.
*Correspondence: Chenghu Wu, d3VjaGVuZ2h1MDEwQDE2My5jb20=