- 1Department of Chemistry, University of Missouri, Columbia, MO, United States
- 2Department of Nutrition and Exercise Physiology, University of Missouri, Columbia, MO, United States
- 3Departments of Radiology, Neurology and Psychological Sciences, and the Thompson Center, Columbia, MO, United States
- 4Department of Pathology and Anatomical Sciences, University of Missouri, Columbia, MO, United States
- 5Department of Bioengineering, University of Illinois at Chicago, Chicago, IL, United States
- 6Biochemistry Department, University of Missouri, Columbia, MO, United States
Phospholipids in the central nervous system (CNS) are rich in polyunsaturated fatty acids (PUFAs), particularly arachidonic acid (ARA) and docosahexaenoic acid (DHA). Besides providing physical properties to cell membranes, these PUFAs are metabolically active and undergo turnover through the “deacylation-reacylation (Land's) cycle”. Recent studies suggest a Yin-Yang mechanism for metabolism of ARA and DHA, largely due to different phospholipases A2 (PLA2s) mediating their release. ARA and DHA are substrates of cyclooxygenases and lipoxygenases resulting in an array of lipid mediators, which are pro-inflammatory and pro-resolving. The PUFAs are susceptible to peroxidation by oxygen free radicals, resulting in the production of 4-hydroxynonenal (4-HNE) from ARA and 4-hydroxyhexenal (4-HHE) from DHA. These alkenal electrophiles are reactive and capable of forming adducts with proteins, phospholipids and nucleic acids. The perceived cytotoxic and hormetic effects of these hydroxyl-alkenals have impacted cell signaling pathways, glucose metabolism and mitochondrial functions in chronic and inflammatory diseases. Due to the high levels of DHA and ARA in brain phospholipids, this review is aimed at providing information on the Yin-Yang mechanisms for regulating these PUFAs and their lipid peroxidation products in the CNS, and implications of their roles in neurological disorders.
Phospholipids in the Central Nervous System Are Enriched in ARA and DHA
The mammalian brain is rich in lipids, with phospholipids playing important roles in biological functions. Changes in composition of membrane phospholipids can lead to alteration of cell functions, including signal transduction, cell-cell recognition, DNA replication, and protein trafficking (1), and with implications in neurological diseases, such as stroke, traumatic brain injury (TBI), depression (2), as well as Alzheimer's and Parkinson's diseases (AD and PD) (3, 4). Major phospholipids in brain include phosphatidylcholine (PC), phosphatidylethanolamine (PE), phosphatidylethanolamine plasmalogen (PEpl), together with smaller amounts of phosphatidylserine (PS), phosphatidylinositol (PI), and cardiolipin (CL) (5) (Figure 1). While these phospholipids are present in different types of cell membranes, CL is specifically present in the inner layer of mitochondrial membrane (6). Phospholipids have fatty acids (FAs) that are largely saturated or monoenes in the sn-1 position of the glycerol moiety, whereas the sn-2 position contains mainly polyunsaturated fatty acids (PUFAs). A characteristic feature for PE in brain is the large proportion of PEpl with alkenyl group in the sn-1 position. These PEpl are abundant in the myelin sheaths (7). The PUFAs in PE are enriched in docosahexaenoic acid (22:6 n-3, DHA), whereas the PUFAs in PC have both DHA and arachidonic acid (20:4 n-3, ARA). PS is an anionic phospholipid with high levels of palmitic acid (16:0) and DHA, and translocation of this phospholipid from the inner to outer membrane surface through the flippases and scramblases can serve as an initiator for apoptotic processes through binding with annexin V (8, 9). PI is comprised of high levels of stearic acid (18:0) and ARA, and the inositol head group can be phosphorylated to form PIP and PIP2. Hydrolysis of PIP2 by phospholipase C results in the production of diacylglycerols and inositol phosphates (5), which are second messengers for activation of protein kinase C (PKC) and for mobilization of calcium from intracellular stores, respectively (10). An obvious difference between the PUFAs in the central nervous system (CNS) and the peripheral system is the low levels of linoleic acid (18:2 n-6) in CNS (11).
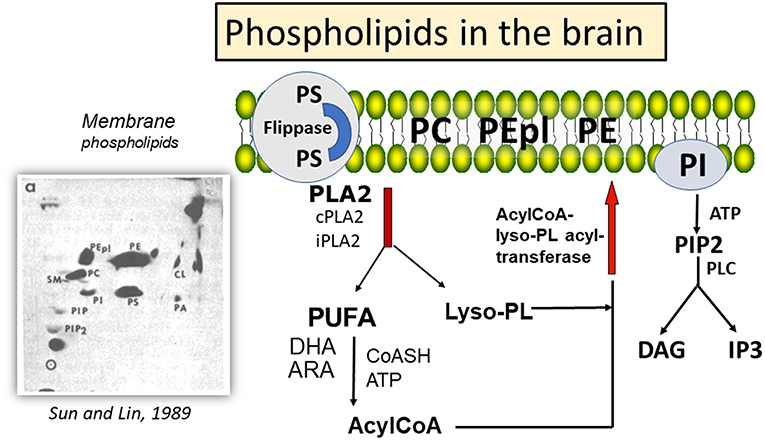
Figure 1. Mechanism for deacylation-reacylation of polyunsaturated fatty acids in phospholipids, and relative amount of phospholipids in the brain. aHigh performance thin layer chromatography (HPTLC) separation of phospholipids in mouse cortex and detection by charring with cupric acetate; PE, phosphatidylethanolamine; PEpl, PE plasmalogen; PC, phosphatidylcholine; PS, phosphatidylserine; PI, phosphatidylinositol; PA, phosphatidic acid; Cl, cardiolipin; PIP, phosphatidylinositol-phosphate; PIP2, phosphatidylinositol 4,5-bisphosphate. HPTLC chromatograph was reprinted from Sun and Lin (5), with permission from Elsevier.
In the mammalian brain membranes, the PUFAs in the phospholipids (mainly PC and PE) are metabolically active and undergo turnover through the “deacylation-reacylation cycle”, also known as the “Land's cycle” (12, 13) (Figure 1). This cycle enables PUFAs to be released from membrane phospholipids through phospholipases A2 (PLA2s) and subsequently return to the membrane phospholipids through the lysophospholipid acyltransferases. In the CNS, different PLA2s are responsible for the release of DHA and ARA from phospholipids, thus suggesting a Yin-Yang mechanism for their metabolic functions (14). Besides production of eicosanoids and docosanoids, which are lipid mediators, these PUFAs are also substrates of oxygen free radicals, resulting in alkenal products that are metabolically active. In this review, attention is focused on factors regulating metabolism of ARA and DHA through different PLA2s, and the role of their peroxidation products in health and disease.
ARA Release by cPLA2
As reviewed by Sun et al., release of ARA from phospholipids is catalyzed mainly by the Group IV calcium-dependent cytosolic PLA2α (cPLA2), a ubiquitous enzyme present in all cells in the CNS (15). Besides the requirement for calcium which binds to the C2 domain, a characteristic property of the cPLA2 is its susceptible to phosphorylation and activation by protein kinases, including the mitogen activated protein kinases (MAPKs) and PKC (16). A study with primary neurons demonstrated ability for NMDA (an excitatory glutamate receptor agonist) to stimulate phosphorylation of cPLA2 through activation of ERK1/2 (17). Studies with microglial cells also indicated the ability of lipopolysaccharides (LPS) to stimulate p-cPLA2 through p-ERK1/2 (18, 19).
Activation of cPLA2 and release of ARA have been implicated in a number of neurologic disorders and brain injury. Subjects with traumatic brain injury (TBI) showed a significant increase in PUFAs, including ARA, in the cerebrospinal fluid as compared with non-TBI controls (20, 21). In a study with acute stroke subjects, the decrease in DHA/ARA ratios in serum was attributed to the increase in cPLA2 activity and release of ARA (22, 23). Indeed, increases in p-cPLA2 were observed in animal models of cerebral ischemia (15). Furthermore, the transient increase in p-cPLA2 suggests tight regulation of this enzyme under pathological conditions (24).
A number of studies with animal models have demonstrated the involvement of cPLA2 in the pathophysiology of AD (25). Evidence included increases in ARA as well as its metabolites in the brain tissue of AD mutant mice (26). Studies with cultured neurons also demonstrated ability for the cytotoxic amyloid beta (Aβ) to stimulate phosphorylation of ERK1/2 and p-cPLA2 (17). Since action of cPLA2 not only releases PUFAs but also lysophospholipids, the increase in lysophosphatidylcholine (LPC) in AD mouse models was marked with a progressive decline in behavior (13). The changes in FAs as well as LPC are in agreement with perturbation of the “Land's cycle.” Using mass spectrometry (MS)-based shotgun lipidomics, analysis indicated increases in MAPK, PLA2, unesterified PUFAs, as well as LPC in the brains of AD transgenic mouse model (14). Using antisense nucleotide against cPLA2, studies by Levy's group demonstrated ability for aggregated Aβ to up-regulate cPLA2 in neurons and microglia (27, 28). Taken together, these studies demonstrate an important role for cPLA2 and the release of ARA in response to brain injury as well as in different neurologic disorders.
The Release of DHA by iPLA2
The group VI calcium-independent PLA2s (iPLA2) are comprised of a number of isoforms and can release PUFAs (including ARA) from phospholipids. However, several studies suggested preference for the release of DHA through iPLA2β (29–32). As reviewed by Farooqui and Horrocks, iPLA2β is abundant in the brain, and is able to influence a number of CNS functions (33, 34). iPLA2-knockout mice showed a decrease in DHA metabolism, further demonstrating the association between DHA and iPLA2 (35). Recently, there is suggestion for a genetic link for this iPLA2 to PD (36). Mutation of iPLA2 was also linked to an infantile autosomal recessive gene disorder with brain iron accumulation, a neurologic disorder showing severe psychomotor and cognitive dysfunction (37, 38). The study by Shinzawa et al. showed neurologic impairments as well as accumulation of spheroids containing tubulovesicular membranes in a mouse model depleting this group of iPLA2 (39).
A study by Gattaz et al. indicated a significant decrease in activity of iPLA2 in platelets from AD patients and subjects with mild cognitive impairment (MCI) compared to normal controls (40). In fact, subjects with MCI also showed alterations of other types of PLA2, including cPLA2 and sPLA2. Chew et al. provided evidence for iPLA2 to play a role on synaptic plasticity and nociception, and these neuronal abnormalities could be reversed by suppressing iPLA2 through antisense oligonucleotide (41). Studies using hippocampal slices indicated involvement of iPLA2 in induction of long-term potentiation (42), and in agreement with the release of DHA through iPLA2, impairments in long-term potentiation could be reversed upon treatment with DHA (43, 44). Taken together, these studies provide support for the role of iPLA2 and DHA in modulating brain function.
Lipid Mediators Derived From ARA and DHA
PUFAs are substrates for a number of oxygen enzymes including cyclooxygenases (COX), lipoxygenases (LOX), cytochrome P450 (CytP450), soluble epoxide hydrolase, and prostaglandin dehydrogenase, resulting in the production of a wild array of lipid mediators (Figure 2). Depending on the cell types and conditions, these lipid mediators have shown protective and detrimental effects. ARA is converted to prostaglandin H2 (PGH2) through COX1/2, and in turn, PGH2 is further converted to prostanoids (prostaglandins and thromboxanes) with both inflammatory and anti-inflammatory properties (18, 45). ARA also serves as substrate for LOX 5/12/15 to produce leukotrienes and lipoxins, and these lipid mediators work through specific receptors to mediate a variety of responses (46, 47). Interestingly, in contrary to the leukotrienes which exert cytotoxic and inflammatory effects in blood cells, lipoxin A2 and lipoxin B4 (synthesized through 5- and 15-lipoxygenases) are mediators with anti-inflammatory and immunomodulatory properties (see overview by Parkinson) (48). In a study by Livne-Bar et al, metabolomics screening for neuroprotective signals in astrocyte conditioned medium indicated the ability for lipoxin A4 and B4 to suppress injury-induced damage in retina (49). In another study on an intracerebral hemorrhage model in rat, lipoxin A4 was shown to offer anti-inflammatory effects and ameliorated the ischemic injury (50).
DHA is metabolized by a specific LOX (e.g., 15-lipoxygenase, Alox15) to form oxylipin intermediates leading to synthesis of resolvin D1 (RvD1), maresins, and neuroprotectin D1 (NPD1) (Figure 2). These structurally distinct molecules possess pro-resolving and pro-homeostatic properties (51–56). RT-PCR and Western blot analysis indicated high Alox 15 expression in the prefrontal cortex, olfactory bulb, and the hippocampal brain area (51). A study with human neuroblastoma cells demonstrated upregulation of Alox15 in association with histone deacetylases (HDAC), thus suggesting an epigenetic link (57). Aspirin-triggered RvD1 was shown to mitigate inflammation by enhancing the pro-resolution status as well as protecting the brain from synaptic dysfunction (58). Systemic prophylaxis treated with aspirin-triggered RvD1 was shown to improve surgery-induced cognitive decline and abolish synaptic dysfunction (59). Studies by Bazan's group indicated ability for NPD1to rescue inflammatory responses by inhibiting production of pro-inflammatory cytokines such as interleukin-1β (60). In a laser-induced mouse model, intraperitoneal injection of NPD1 could inhibit choroidal neovascularization, further confirming the mechanism for NPD1 to upregulate the resolution phase of the inflammatory response (61). Taken together, despite a Yin-Yang mechanism for metabolism of ARA and DHA, considerable cross-talks may occur among the lipid mediators in conferring detrimental and pro-resolving actions in different cell types and systems.
Lipid Peroxidation of ARA and DHA
4-Hydroxy-alkenals
PUFAs, including DHA, ARA, linoleic acid, and linolenic acid, are susceptible to lipid peroxidation mediated by oxygen free radicals generated from a number of factors, including radiation, cellular response to xenobiotics, cytokines, bacterial and viral invasions, and mitochondrial oxidative stress (62, 63). A growing body of research has demonstrated the important role of lipid peroxidation in disease pathology, including diabetes, atherosclerosis, cardiovascular diseases, as well as neuroinflammation and neurodegenerative diseases (64–68). Oxygen free radicals can target the esterified PUFAs in membrane phospholipids as well as the free PUFAs in the cell cytoplasm. Peroxidation of these PUFAs is generally comprised of three main steps: initiation, propagation, and termination (69). These lipid peroxides are highly reactive, and upon interaction with iron, they can induce ferroptosis, which is a form of cell death different from that mediated by caspases (70, 71).
Depending on the position of the double bond, lipid peroxidation of n-3 and n-6 PUFAs can undergo fragmentation at different sites to give rise to a large number of carbonyl-containing lipid oxidation compounds including α, β-hydroxy aldehydes, acrolein, glyoxal, and malondialdehyde (72–74). The most prominent products are 4-hydroxyhexenal (4-HHE) from DHA and 4-hydroxynonenal (4-HNE) from ARA (75). In addition, ARA can react with 12-lipoxygenase to produce 12-hydroperoxy-eicosatetraenoate (12-HpETE), and in turn, undergoes peroxidation to form reactive 4-hydroxydodecadienal (4-HDDE) (69, 76, 77). These reactive aldehydes can readily undergo oxidation and reduction, and converted to acids or alcohols, which are more stable metabolites (78). Due to the high reactivity, these alkenal compounds are regarded as “second messengers of free radical reactions” (79), and biomarker of oxidative stress in inflammatory diseases [see review by Ito et al. (68)].
An important property of the 4-hydox-alkenal products is their ability to form adducts with proteins, phospholipids, and nucleic acids through Michael addition and Schiff base reactions (73, 74, 77, 78, 80–83). The phospholipid adducts are formed largely with phosphatidylethanolamine (PE), especially the alkenylacyl PE subtype (77). These alkenal compounds are present widely in the body organs, in different cell types (84), and in body fluids including plasma and urine (76). Studies by Sasson's group provided evidence for binding of 4-HNE and 4-HDDE to peroxisome proliferator-activated receptors (PPARs). The activation of PPARs leads to a mechanism for their hormetic and regulatory effects against the pathology of metabolic and vascular diseases such as diabetes and atherosclerosis (69, 85–88) (Figure 3). Other studies demonstrated formation of protein adducts with 4-HNE in other metabolic and inflammatory diseases (78), such as rheumatoid arthritis (89), neuroborrelious (90), and in age-related disorders and low back pain (91, 92).
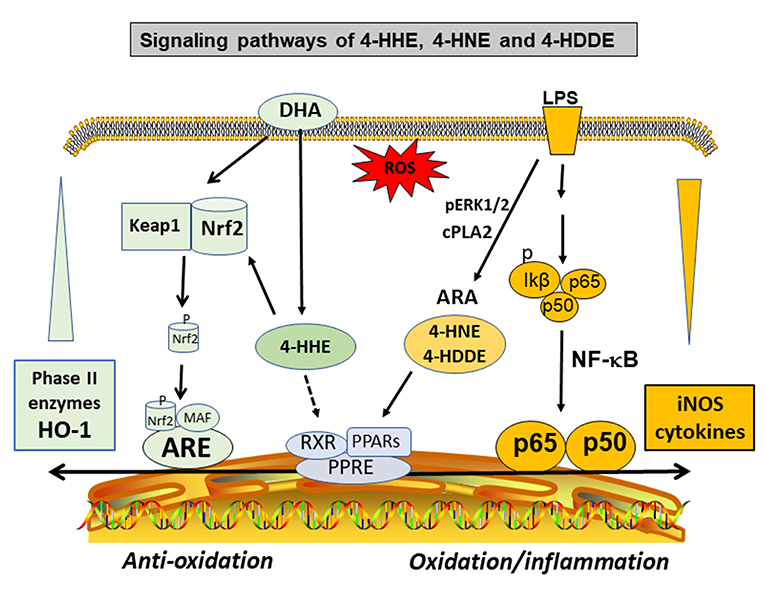
Figure 3. Hormetic and signaling effects of 4-hydoxy alkenals involving inhibition of NF-κB and cPLA2/ARA pathways, and activation of PPARs and Nrf2/HO-1 pathways.
The involvement of 4-HNE and 4-HDDE in inflammatory diseases has led to studies searching for mechanisms regulating the release of ARA. Studies with microglial cells demonstrated the ability for lipopolysaccharides (LPS) to stimulate ARA release through phosphorylation of ERK1/2 and cPLA2 (15, 18), and in turn, stimulation of this pathway leads to an increased production of 4-HNE (84). Activation of Toll-like receptors by LPS also induces COX-2, which acts on ARA to produce prostanoids. A study by Uchida demonstrated the involvement of 4-HNE in the induction of COX-2 expression (through the p38MAPK pathway), implicating the role for 4-HNE in a feed-forward mechanism to enhance inflammation (93). The acyl groups in cardiolipin (CL) are comprised with high levels of PUFAs (especially linoleic acid), and is thus a good source for production of 4-HNE. Therefore, peroxidation of CL and production of 4-HNE could account for oxidative disturbances of mitochondrial function (6, 94).
The high levels of DHA in the brain and retina have alerted studies on the pathophysiological role of its peroxidation product, mainly 4-HHE [see review by Long et al. (95)]. However, despite similar properties for both 4-HNE and 4-HHE, subtle differences were observed. For example, in a study with primary neurons in culture, application in vitro of both 4-HHE and 4-HNE showed differences in toxicity with LD50's of 23 and 18 μmol/L, respectively (96). In this study, cytotoxicity mediated by both alkenals could be suppressed by adding thiol scavengers such as glutathione (GSH) and N-acetyl cysteine, suggesting their ability to form adducts with these compounds. The study by Soulage et al. observed increase in circulating 4-HHE levels in type 2 diabetic human and in the diabetic Zucker rats (97). In this same study, exogenous 4-HHE was shown to reduce the glutathione concentration in L6 muscle cells (97). In a review by Long and Picklo, 4-HHE was 5- to 20-fold more potent in forming adducts with glutathione as compared with 4-HNE (95). Besides interaction with the GSH pathways, recent studies provided evidence for 4-HHE to exert hormetic effects by stimulating the stress response pathway involving nuclear factor-like 2 (Nrf2) and synthesis of anti-oxidant enzymes such as heme oxygenase-1 (HO-1) (95, 98–100) (Figure 3). In fact, our recent study with microglial cells also indicated ability for 4-HHE to induce higher levels of HO-1 as compared to 4-HNE (84).
Despite of perceived hormetic effects, the alkenal compounds may have deleterious and toxic effects, especially when tested at high concentrations and at in vitro settings. In a study by Awada et al. increase in 4-HHE in plasma was observed in mice consuming a diet with oxidized lipids, and the increase in 4-HHE was linked to oxidation and inflammation in the small intestine (101). In this study, the damaging effect on intestinal absorption was attributed to ability for 4-HHE to form protein adducts and alter glutathione metabolism (101). However, in a review by Mauerhofer et al., oxidized phospholipids could offer protective effects, including activation of PPARs, suppression of the toll-like receptors, and up-regulation of the Nrf2 anti-oxidant pathway (102). These diverse results clearly suggest the importance of future studies to further examine oxidized phospholipids and specific protein adducts formed by the reactive hydroxyl-alkenal compounds under different pathological conditions (103).
Methods for Analysis of 4-HHE and 4-HNE
Immunohistochemistry Assay of Protein Adducts
The reactivity of 4-HHE and 4-HNE to form adducts with biomolecules suggests the possibility to detect the adducts by antibody-based methods (73). An immunoblot assay was used to detect 4-HNE in human blood (104). In a study in which plasma proteins were separated by two dimension electrophoresis, increases in HNE-protein adducts were observed in autism subjects as compared to controls (105). Using a mouse monoclonal antibody that specifically recognizes the 4-HNE-histidine epitope, an ELISA method was developed for assaying 4-HNE in cell lysates (106), and increased levels of the 4-HNE-histidine adduct were observed in the middle frontal gyrus of AD patients as compared with controls (107). Using an anti-4-HNE adducted protein, increases in 4-HNE were observed in the hippocampus and entorhinal cortex in rat brain after binge ethanol administration (108). Due to the increasing interest to study the role of 4-HDDE, an oxidative by-product of ARA, a monoclonal antibody was recently developed against the 4-HDDE adduct (109). However, many of these antibody assays lack sensitivity and specificity, and commercial availability is limited (110). Therefore, future studies using advance techniques to detect specific protein adducts will enhance understanding the role of these peroxidation products in different disease conditions.
Efforts to develop immunohistochemical techniques to detect localization of 4-HNE in tissues and cells have also become important tools to understand the effects of oxidative damage in tissues and cells (111). In the study by Mamalis et al., increases in immunohistochemistry staining of 4-HNE together with other oxidant markers such as 8-hydroxydeoxyguanosine and advanced glycation end products, were detected in damaged human epidermis due to excessive exposure to UV light (112). In another study, age differences in 4-HNE immunoreactivity were observed in the mouse hippocampal CA1 area comparing groups at 40–42 to 50–59 weeks (113). In addition with an increase in oxidative stress with age, the increase in 4-HNE was marked by a decrease in mitochondrial dysfunction. Interestingly, administration of epigallocatechin-gallate, a polyphenol abundant in green tea extract, could attenuate 4-HNE immunoreactivity in the aged brain (114).
Relatively few studies have developed effective immune assay for 4-HHE. Using a mouse monoclonal IgG1 antibody, mAbHHE53, which is specific for protein-histidine bound HHE, increases in 4-HHE in autopsy samples of spinal cords were observed in amyotrophic lateral sclerosis patients (115). However, despite that the mouse monoclonal IgG1 antibody was developed to target 4-HHE, there is evidence that both 4-HNE and 4-HHE were detected (116). Considering that different PLA2s are responsible for the release of ARA and DHA and subsequently their peroxidation products, future studies for production of more specific immune products for the alkenals will help to advance understanding of their metabolism.
LC-MS/MS Analysis for Simultaneous Measurement of 4-HHE and 4-HNE
Methods for accurate and precise analysis of free 4-HHE and 4-HNE present in biological samples include gas or liquid chromatography (GC or LC) and sometimes coupled with MS (73, 95). Depending on the chromophore, these peroxidation products could be quantified through LC with UV detection at 220 nm (117). However, due to lack of sensitivity and precision, GC-MS and LC-MS methods were developed as an alternative to the spectrophotometric detection. The classic multiple reaction monitoring assay performed on a triple quadrupole mass spectrometer has long been used for simultaneous quantification of 4-hydroxyalkenals, and this technique is capable of monitoring characteristic transitions for each targeted compound. Without derivatization, 4-hydroxyalkenals in human T cell leukemia extracts or mouse liver samples can be detected by selected reaction monitoring as [M+H] + (118, 119), but the sensitivity of this analysis is not sufficient to detect the levels of 4-hydroxyalkenals in small biological samples. Subsequently, different approaches were used to derivatize 4-hydroxyalkenals in order to increase detection sensitivity (120–124). Wang et al. enhanced sensitivity with a shotgun lipidomics-base method using the Michael adduct of 4-HNE with carnosine (122). Another agent is 1,3-cyclohexanedione (CHD), which takes advantage of the intrinsic reactivity of aldehydes, allowing for more specific and sensitive detection in different biological matrices (84, 100, 125–127). Because the alkenyl aldehydes are rather unstable, derivatization can help to stabilize these molecules. Recently, we applied the CHD-derivatization strategy to quantify the levels of 4-hydroxyalkenal species in different biological matrices over a wide range of concentrations (84, 128). Since phospholipids are common interfering species in reverse-phase LC, and can cause significant ionization suppression in the mass spectrometer, we employed a solid phase extraction (SPE) strategy to remove phospholipids while preserving the concentrations of the analytes (129). Our results for analysis of 4-HNE in biological samples, including plasma and brain tissue, fall into the range from 0.1 to 1.5 nmol/mg protein, depending on the samples and conditions (63, 120, 130, 131).
Yin-Yang Mechanisms Regulating the Production of 4-HHE and 4-HNE
With an established LC-MS/MS protocol to simultaneously measure 4-HNE and 4-HHE, an experiment was carried out to examine factors regulating levels of the hydroxyl-alkenals in BV-2 microglial cells stimulated with LPS and/or supplemented with DHA (84). Results showed that treating cells with exogenous DHA led to a dose-dependent increase in 4-HHE but not 4-HNE, whereas stimulation of cells with LPS resulted in an increase in 4-HNE but not 4-HHE (84). Since LPS is known to stimulate cPLA2 and release of ARA in microglial cells (18), an association between 4-HNE and the cPLA2/ARA pathway was confirmed using inhibitors for cPLA2, which readily suppressed the LPS-induced increase in 4-HNE in these cells (84). Quercetin is a botanical polyphenol known to suppress LPS-induced cPLA2 and NF-κB related pro-inflammatory responses in microglial cells (132). Along this line, treatment of cells with quercetin also suppressed LPS-induced 4-HNE (133). Taken together, these studies demonstrated a link between stimulation of the cPLA2/ARA pathway and production of 4-HNE, and thus assay of 4-HNE can serve as a marker for the inflammatory pathway.
Our study with microglial cells indicated an increase in levels of 4-HHE upon treating microglial cells with DHA within a relatively short period of time of 6 hr (84). These results suggest that despite of uptake of DHA and incorporation into membrane phospholipids, some DHA may directly enter the cells and make available for lipid peroxidation to form 4-HHE. Studies by Ishikado and Nagayama with endothelial cells also attributed ability for DHA to exert anti-inflammatory effects through increased production of 4-HHE, and in turn, ability for 4-HHE to upregulate the antioxidant stress pathway with increased synthesis of HO-1 (125, 126). Consequently, the ability for DHA to suppress inflammatory responses through its oxidative product is a novel phenomenon worthy of future studies with animal models (Figure 3).
n-3 PUFAs Supplementation and Lipid Peroxidation Products: Implications on Neurological Disorders
To-date, n-3 PUFAs in the form of fish oil are one of the most highly consumed dietary supplements by humans (134). Although fish oil contains substantial amounts of eicosapentaenoic acid (EPA) together with DHA, EPA is an intermediate for biosynthesis of DHA, and is present in relatively low levels in brain. In recent years, there is enormous interest to examine effects of dietary fish oil and DHA supplement on health and diseases [see review by Sun et al. (135)]. However, few studies have focused on the hormetic and deleterious effects of the peroxidative products. In a recent study, human subjects consuming Atlantic salmon showed increases in n-3 PUFAs in the phospholipids (e.g. PC) and triacylglycerols in plasma (136). In another study, healthy male subjects given different levels of DHA supplement (up to 1600 mg of DHA per day) showed a dose-dependent increase in levels of 4-HHE in the plasma (137). In agreement with the Yin-Yang mechanism for production of 4-HHE and 4-HNE, results of Calzada's study showed that DHA supplementation did not alter levels of 4-HNE. In this study, DHA supplementation not only did not alter redox homeostasis, but also prevented low-density lipoproteins from oxidation (137).
In a review by Trepanier et al. there is evidence for dietary n-3 PUFA to exert anti-inflammatory effects and neuroinflammatory outcomes in a number of animal models with neurological disorders (138). Studies with animal and cell models also pointed to the role of microglial cells in mediating the neuroinflammatory responses (139). Treating DHA to cells in vitro could mitigate LPS-induced pro-inflammatory responses through interaction with the toll-like receptor 4 (84, 140). Nevertheless, these effects in vitro are dependent on the treatment conditions, as high levels of DHA was shown to cause profound cell swelling and induce pyroptotic cell death (141). In a study with human microglial cells, both EPA and DHA were effective in decreasing the pro-inflammatory M1 markers and stimulating the anti-inflammatory M2 markers (142).
Lipid Peroxidation in Cerebral Ischemia and Brain Injury
Although increase in oxidative stress has been implicated in many forms of brain injuries and ischemic stroke, relatively few studies have successfully assessed the peroxidation products under these conditions (143–145). In a study by Zhang et al., mice fed a fish oil diet showed resistance to insult due to focal cerebral ischemia. The protective effects of n-3 PUFA supplements were attributed to ability of 4-HHE to upregulate the antioxidant pathway involving Nrf2 and synthesis of HO-1 (146). Considering the important role for the Nrf2 pathway to promote transcription of a number of antioxidant genes for regulating the body defense systems, recognition of a metabolic link between DHA and/or 4-HHE with the Nrf2 pathway may provide therapeutic strategy against oxidative damage due to cerebral ischemia and other brain injuries (147, 148).
Lipid Peroxidation in Neurological and Inflammatory Pain
There is emerging evidence for a connection between activated immune cells with oxidative stress, inflammation, and nociceptive responses (149–151). Low back pain is among the highest prevalence neurologic disorders in humans (152–154). Acrolein, a reactive aldehyde derived from a number of environmental factors, has been regarded as an important factor contributing to the hyperalgesia following traumatic injury. Besides acrolein, there is indication that other oxidant stressors such as 4-HNE and H2O2, may also augment the transient receptor potential channels (TRPA1 and TRPV1) in mediation of inflammatory responses and pain (155–157). More importantly, 4-HNE was shown to activate these channels through binding specific sites on the channel protein (158). In agreement with this phenomenon, inhibitors to acrolein and antibodies to oxidized phospholipids were able to mitigate the channel activity as well as diminish pain sensation (159). Future studies to further understand how the channel proteins are activated by these oxidant stressors will undoubted be an important path to advance therapy to mitigate neuropathic and inflammatory pain.
Lipid Peroxidation Associated With Binge Alcohol Consumption
Indications that chronic alcohol consumption is linked to increases in oxidative stress in the brain have led to studies searching for the mechanism whereby ethanol may induce lipid peroxidation products (160). Studies by Collins' group used an animal model as well as an organotypic slice culture model to demonstrated a link between binge alcohol administration with increases in PLA2s together with PARP-1 and aquaporin-4 (AQP4) (108, 161, 162). Using a commercial antibody against 4-HNE protein adduct, binge ethanol administration was shown to induce increases in 4-HNE in specific brain regions, including the hippocampus and entorhinal cortex (108). In agreement with the increase in PLA2 activity, a time-dependent increase in levels of 4-HNE adduct was also observed in the brain slice culture experiment exposed to ethanol (161). This experiment further showed that supplementation of DHA (25 μM) to the slice culture could readily abrogate the oxidative markers due to the binge ethanol treatment (108). Consequently, future studies are needed to elucidate the mechanism for DHA to inhibit oxidative stress due to binge alcohol administration.
Lipid Peroxidation Associated With Alzheimer's Disease (AD)
Oxidative stress is known to play an important role in AD pathogenesis; lipid peroxidation together with the increases in production of 4-HNE as well as 4-HHE have been reported in the progression of this disease (163–166). Oligomeric Aβ could target lipid peroxidation resulting in increase in 4-HNE which in turn, form adducts with cysteine, histidine, and lysine residues (167, 168). Accumulation of HNE-adducts may lead to irreversible changes and thus impairing metabolic functions in the development of AD pathology (169). In an AD transgenic mouse model, administration of oligomeric Aβ resulted in an increase of free PUFAs together with levels of 4-HNE in the brain, suggesting increase in lipid peroxidation activity (14). Since lipid peroxidation is a relatively early event of oxidative stress, increases in alkenal compounds were detected in the hippocampal gyrus in individuals in the preclinical phase (165, 170). In addition, increased levels of 4-HNE and acrolein were observed in the brains of mild cognitive impairment and in early AD patients (131). In the late AD stage, propagation and amplification of oxidative stress were marked by increases in malondialdehyde (MDA), acrolein, and 4-HNE (165).
In a group of AD patients showing age-related decline, DHA supplements appeared to show improvement in learning and memory in this group of patients (171). Dietary supplement of DHA also protected AD-model mice from amyloid and dendritic pathology (172–174). In a study to examine effects of DHA supplementation on different phospholipids in brain regions of AD mouse model, results indicated significant increases in PC and PE levels in cortex, hippocampus and cerebellum (175). However, further studies are needed to examine whether the changes in brain lipids due to dietary DHA supplementation are associated with altered lipid peroxidation activity in the brain.
DHA and Lipid Peroxidation Associated With Autism Spectrum Disorder (ASD)
As reviewed by Romano et al. lipid peroxidation appears to play a role in several neuropsychiatric disorders, including bipolar diorder, depression, schizophrenia and Huntington's disease (176). Autism spectrum disorder (ASD) is a neurodevelopmental disorder that occurs in the early stage of life. The pathogenesis of ASD remains controversial; nevertheless, interactions between multiple genes and environmental risk factors are strong candidates (177). Early studies showed alterations in FA profiling in plasma of ASD patients with specific reduction of DHA (178). A study on proteomes in plasma of ASD and control subjects indicated significant increase in proteins involved in acute inflammatory response together with protein adducts to 4-HNE, thus suggesting a link between oxidative stress and lipid peroxidation in the pathophysiology of ASD (105). In particular, younger children with autism seem to be more vulnerable to oxidative stress and thus showing a greater increase in lipid peroxidation products. In a study on Egyptian autistic children, levels of malondialdehyde (MDA) were significantly higher in children with autism <6 years of age, whereas this difference diminished with increasing age of the children (179). In another study with older patients, significantly higher levels of 4-HNE protein adducts were found in erythrocyte membranes and plasma from autistic patients as compared with controls (180).
In a study with a gene/stress mouse model, maternal DHA supplementation was shown to mitigate autistic behaviors in offspring pups (181). In order to investigate mechanism(s) whereby DHA supplementation could suppress autistic behavior, Yang et al. examined FAs and peroxidation products in mouse pups from mothers given a control diet or a diet containing 1% DHA (128). Results showed increases in DHA and decreases in ARA levels in all regions of the pup brain. However, DHA-supplemented diet increased 4-HHE levels mainly in the cerebral cortex and hippocampal regions, whereas levels of 4-HNE were not changed (128). Considering the important role of neurons in mediating memory and cognitive functions in the cerebral cortex and hippocampus, the specific increase in 4-HHE in these brain regions upon supplementation with DHA suggests greater peroxidation activity associated with these brain regions. Further investigations are needed to examine the physiologic role of 4-HHE in brain development. This dietary regimen also altered DHA, ARA, and 4-HHE in mouse heart and plasma, and interestingly, analysis of these alkenals in plasma indicated not only an increase in 4-HHE but also a decrease in 4-HNE. A study by Nakagawa et al. showed a similar increases in DHA and decreases in ARA as well as elevated levels of 4-HHE but not 4-HNE in adult mice fed dietary fish oil in multiple peripheral organs of adult mice (100). Consequently, more studies are needed to examine whether increase in 4-HHE due to maternal DHA supplement can enhance the hormetic effects during brain development (88).
Concluding Remarks and Future Perspective
The abundance of DHA and ARA in phospholipids in the CNS remains a subject of interest for understanding the physiologic role of their metabolites. The current review demonstrated a Yin-Yang mechanism for metabolism of DHA and ARA due to their release by different PLA2s, and these mechanisms led to diverse down-stream pathways for production of lipid mediators that are pro-inflammatory and pro-resolving.
Peroxidation of DHA and ARA by oxygen free radicals results in production of 4-HHE, 4-HNE, 4-HDDE, and other carbonyl products, which can be detected in body organs and body fluids. These reactive aldehydes are electrophiles, and can confer cytotoxic and protective effects depending on the conditions for production. There is increasing evidence for the hormetic and anti-inflammatory effects of these alkenals through interaction with PPARs, suppression of NF-κB inflammation, and upregulation of the stress pathway involving Nrf2 and synthesis of HO-1. However, more efforts are needed to identify binding of these alkenals to specific proteins in pathological conditions. Many neurological disorders are accompanied by oxidative stress, neuroinflammation and apoptotic cell death. Although it is not possible to elucidate individual mechanism(s) for the pathogenesis of these disorders, this review provided an emphasis on an increase in 4-HHE due to supplementation of DHA, and the increase in 4-HNE associated with the inflammatory pathway involving activation of cPLA2 and release of ARA. Due to the complexity of membrane lipids, advance techniques for lipidomics are needed to better understand mechanisms of oxidative stress and source of lipid peroxidation products in disease processes.
Author Contributions
BY and GS initiated writing this review. KF, DB, ZG, JL, WF, and CG contributed substantially to the concept and editing of the review.
Funding
This review was supported by the MU Research Council grant (17-009) from the University of Missouri to DB, the National Institutes of Health grant R01-AG044404 (to JL), and the Charles W. Gehrke Proteomics Center FY18 mini grant (to BY and GS).
Conflict of Interest Statement
The authors declare that the research was conducted in the absence of any commercial or financial relationships that could be construed as a potential conflict of interest.
References
1 Dowhan W. Molecular basis for membrane phospholipid diversity: why are there so many lipids? Annu Rev Biochem. (1997) 66:199–232. doi: 10.1146/annurev.biochem.66.1.199
2 Tiemeier H, van Tuijl HR, Hofman A, Kiliaan AJ, Breteler MM. Plasma fatty acid composition and depression are associated in the elderly: the Rotterdam Study. Am J Clin Nutr. (2003) 78:40–6. doi: 10.1093/ajcn/78.1.40
3 Soderberg M, Edlund C, Kristensson K, Dallner G. Fatty acid composition of brain phospholipids in aging and in Alzheimer's disease. Lipids. (1991) 26:421–5. doi: 10.1007/BF02536067
4 Wang S, Zhang S, Liou LC, Ren Q, Zhang Z, Caldwell GA, et al. Phosphatidylethanolamine deficiency disrupts alpha-synuclein homeostasis in yeast and worm models of Parkinson disease. Proc Natl Acad Sci USA. (2014) 111:E3976–85. doi: 10.1073/pnas.1411694111
5 Sun GY, Lin TN. Time course for labeling of brain membrane phosphoinositides and other phospholipids after intracerebral injection of [32P]-ATP. Evaluation by an improved HPTLC procedure. Life Sci. (1989) 44:689–96. doi: 10.1016/0024-3205(89)90475-X
6 Liu W, Porter NA, Schneider C, Brash AR, Yin H. Formation of 4-hydroxynonenal from cardiolipin oxidation: Intramolecular peroxyl radical addition and decomposition. Free Radic Biol Med. (2011) 50:166–78. doi: 10.1016/j.freeradbiomed.2010.10.709
7 Sun GY, Horrocks LA. Acyl and alk-1-enyl group compositions of the alk-1'-enyl acyl and the diacyl glycerophosphoryl ethanolamines of mouse and ox brain. J Lipid Res. (1969) 10:153–7.
8 Hankins HM, Baldridge RD, Xu P, Graham TR. Role of flippases, scramblases and transfer proteins in phosphatidylserine subcellular distribution. Traffic. (2015) 16:35–47. doi: 10.1111/tra.12233
9 Kim HY, Huang BX, Spector AA. Phosphatidylserine in the brain: metabolism and function. Prog Lipid Res. (2014) 56:1–18. doi: 10.1016/j.plipres.2014.06.002
10 Huang HM, Sun GY. Effects of ATP on phosphatidylinositol-phospholipase C and inositol 1-phosphate accumulation in rat brain synaptosomes. J Neurochem. (1988) 50:366–74. doi: 10.1111/j.1471-4159.1988.tb02921.x
11 Sun GY, Horrocks LA. The fatty acid and aldehyde composition of the major phospholipids of mouse brain. Lipids. (1968) 3:79–83. doi: 10.1007/BF02530973
12 Sun GY, MacQuarrie RA. Deacylation-reacylation of arachidonoyl groups in cerebral phospholipids. Ann N Y Acad Sci. (1989) 559:37–55. doi: 10.1111/j.1749-6632.1989.tb22597.x
13 Granger MW, Liu H, Fowler CF, Blanchard AP, Taylor M, Sherman SPM, et al. Distinct disruptions in Land's cycle remodeling of glycerophosphocholines in murine cortex mark symptomatic onset and progression in two Alzheimer's Disease mouse models. J Neurochem. (2018) 149:499–517. doi: 10.1111/jnc.14560
14 Palavicini JP, Wang C, Chen L, Hosang K, Wang J, Tomiyama T, et al. Oligomeric amyloid-beta induces MAPK-mediated activation of brain cytosolic and calcium-independent phospholipase A2 in a spatial-specific manner. Acta Neuropathol Commun. (2017) 5:56. doi: 10.1186/s40478-017-0460-6
15 Sun GY, Chuang DY, Zong Y, Jiang J, Lee JC, Gu Z, et al. Role of cytosolic phospholipase A2 in oxidative and inflammatory signaling pathways in different cell types in the central nervous system. Mol Neurobiol. (2014) 50:6–14. doi: 10.1007/s12035-014-8662-4
16 Burke JE, Dennis EA. Phospholipase A2 structure/function, mechanism, and signaling. J Lipid Res. (2009) 50 (Suppl):S237–42. doi: 10.1194/jlr.R800033-JLR200
17 Shelat PB, Chalimoniuk M, Wang JH, Strosznajder JB, Lee JC, Sun AY, et al. Amyloid beta peptide and NMDA induce ROS from NADPH oxidase and AA release from cytosolic phospholipase A2 in cortical neurons. J Neurochem. (2008) 106:45–55. doi: 10.1111/j.1471-4159.2008.05347.x
18 Chuang DY, Simonyi A, Kotzbauer PT, Gu Z, Sun GY. Cytosolic phospholipase A2 plays a crucial role in ROS/NO signaling during microglial activation through the lipoxygenase pathway. J Neuroinflammation. (2015) 12:199. doi: 10.1186/s12974-015-0419-0
19 Malada-Edelstein YF, Hadad N, Levy R. Regulatory role of cytosolic phospholipase A2 alpha in the induction of CD40 in microglia. J Neuroinflammation. (2017) 14:33. doi: 10.1186/s12974-017-0811-z
20 Hogan SR, Phan JH, Alvarado-Velez M, Wang MD, Bellamkonda RV, Fernandez FM, et al. Discovery of lipidome alterations following traumatic brain injury via high-resolution metabolomics. J Proteome Res. (2018) 17:2131–43. doi: 10.1021/acs.jproteome.8b00068
21 Farias SE, Heidenreich KA, Wohlauer MV, Murphy RC, Moore EE. Lipid mediators in cerebral spinal fluid of traumatic brain injured patients. J Trauma. (2011) 71:1211–8. doi: 10.1097/TA.0b013e3182092c62
22 Ueno Y, Tanaka R, Yamashiro K, Miyamoto N, Hira K, Kurita N, et al. Age stratification and impact of eicosapentaenoic acid and docosahexaenoic acid to arachidonic acid ratios in ischemic stroke patients. J Atheroscler Thromb. (2017) 25:593–605. doi: 10.5551/jat.40691
23 Suda S, Katsumata T, Okubo S, Kanamaru T, Suzuki K, Watanabe Y, et al. Low serum n-3 polyunsaturated fatty acid/n-6 polyunsaturated fatty acid ratio predicts neurological deterioration in Japanese patients with acute ischemic stroke. Cerebrovasc Dis. (2013) 36:388–93. doi: 10.1159/000355683
24 Kishimoto K, Li RC, Zhang J, Klaus JA, Kibler KK, Dore S, et al. Cytosolic phospholipase A2 alpha amplifies early cyclooxygenase-2 expression, oxidative stress and MAP kinase phosphorylation after cerebral ischemia in mice. J Neuroinflammation. (2010) 7:42. doi: 10.1186/1742-2094-7-42
25 Gentile MT, Reccia MG, Sorrentino PP, Vitale E, Sorrentino G, Puca AA, et al. Role of cytosolic calcium-dependent phospholipase A2 in Alzheimer's disease pathogenesis. Mol Neurobiol. (2012) 45:596–604. doi: 10.1007/s12035-012-8279-4
26 Sanchez-Mejia RO, Newman JW, Toh S, Yu GQ, Zhou Y, Halabisky B, et al. Phospholipase A2 reduction ameliorates cognitive deficits in a mouse model of Alzheimer's disease. Nat Neurosci. (2008) 11:1311–8. doi: 10.1038/nn.2213
27 Sagy-Bross C, Hadad N, Levy R. Cytosolic phospholipase A2alpha upregulation mediates apoptotic neuronal death induced by aggregated amyloid-beta peptide1–42. Neurochem Int. (2013) 63:541–50. doi: 10.1016/j.neuint.2013.09.007
28 Szaingurten-Solodkin I, Hadad N, Levy R. Regulatory role of cytosolic phospholipase A2alpha in NADPH oxidase activity and in inducible nitric oxide synthase induction by aggregated Abeta1–42 in microglia. Glia. (2009) 57:1727–40. doi: 10.1002/glia.20886
29 Green JT, Orr SK, Bazinet RP. The emerging role of group VI calcium-independent phospholipase A2 in releasing docosahexaenoic acid from brain phospholipids. J Lipid Res. (2008) 49:939–44. doi: 10.1194/jlr.R700017-JLR200
30 Strokin M, Sergeeva M, Reiser G. Prostaglandin synthesis in rat brain astrocytes is under the control of the n-3 docosahexaenoic acid, released by group VIB calcium-independent phospholipase A2. J Neurochem. (2007) 102:1771–82. doi: 10.1111/j.1471-4159.2007.04663.x
31 Strokin M, Sergeeva M, Reiser G. Role of Ca2+-independent phospholipase A2 and n-3 polyunsaturated fatty acid docosahexaenoic acid in prostanoid production in brain: perspectives for protection in neuroinflammation. Int J Dev Neurosci. (2004) 22:551–7. doi: 10.1016/j.ijdevneu.2004.07.002
32 Rapoport SI. Arachidonic acid and the brain. J Nutr. (2008) 138:2515–20. doi: 10.1093/jn/138.12.2515
33 Ramanadham S, Ali T, Ashley JW, Bone RN, Hancock WD, Lei X. Calcium-independent phospholipases A2 and their roles in biological processes and diseases. J Lipid Res. (2015) 56:1643–68. doi: 10.1194/jlr.R058701
34 Farooqui AA, Horrocks LA. Brain phospholipases A2: a perspective on the history. Prostaglandins Leukot Essent Fatty Acids. (2004) 71:161–9. doi: 10.1016/j.plefa.2004.03.004
35 Cheon Y, Kim HW, Igarashi M, Modi HR, Chang L, Ma K, et al. Disturbed brain phospholipid and docosahexaenoic acid metabolism in calcium-independent phospholipase A(2)-VIA (iPLA(2)beta)-knockout mice. Biochim Biophys Acta. (2012) 1821:1278–86. doi: 10.1016/j.bbalip.2012.02.003
36 Malley KR, Koroleva O, Miller I, Sanishvili R, Jenkins CM, Gross RW, et al. The structure of iPLA2beta reveals dimeric active sites and suggests mechanisms of regulation and localization. Nat Commun. (2018) 9:765. doi: 10.1038/s41467-018-03193-0
37 Gregory A, Westaway SK, Holm IE, Kotzbauer PT, Hogarth P, Sonek S, et al. Neurodegeneration associated with genetic defects in phospholipase A(2). Neurology. (2008) 71:1402–9. doi: 10.1212/01.wnl.0000327094.67726.28
38 Morgan NV, Westaway SK, Morton JE, Gregory A, Gissen P, Sonek S, et al. PLA2G6, encoding a phospholipase A2, is mutated in neurodegenerative disorders with high brain iron. Nat Genet. (2006) 38:752–4. doi: 10.1038/ng1826
39 Shinzawa K, Sumi H, Ikawa M, Matsuoka Y, Okabe M, Sakoda S, et al. Neuroaxonal dystrophy caused by group VIA phospholipase A2 deficiency in mice: a model of human neurodegenerative disease. J Neurosci. (2008) 28:2212–20. doi: 10.1523/JNEUROSCI.4354-07.2008
40 Gattaz WF, Talib LL, Schaeffer EL, Diniz BS, Forlenza OV. Low platelet iPLA(2) activity predicts conversion from mild cognitive impairment to Alzheimer's disease: a 4-year follow-up study. J Neural Transm. (2014) 121:193–200. doi: 10.1007/s00702-013-1088-8
41 Chew WS, Shalini SM, Torta F, Wenk MR, Stohler C, Yeo JF, et al. Role of prefrontal cortical calcium-independent phospholipase A2 in antinociceptive effect of the norepinephrine reuptake inhibitor antidepresssant maprotiline. Neuroscience. (2017) 340:91–100. doi: 10.1016/j.neuroscience.2016.10.037
42 Wolf MJ, Izumi Y, Zorumski CF, Gross RW. Long-term potentiation requires activation of calcium-independent phospholipase A2. FEBS Lett. (1995) 377:358–62. doi: 10.1016/0014-5793(95)01371-7
43 Fujita S, Ikegaya Y, Nishikawa M, Nishiyama N, Matsuki N. Docosahexaenoic acid improves long-term potentiation attenuated by phospholipase A(2) inhibitor in rat hippocampal slices. Br J Pharmacol. (2001) 132:1417–22. doi: 10.1038/sj.bjp.0703970
44 Mazzocchi-Jones D. Impaired corticostriatal LTP and depotentiation following iPLA2 inhibition is restored following acute application of DHA. Brain Res Bull. (2015) 111:69–75. doi: 10.1016/j.brainresbull.2014.12.010
45 Farooqui AA, Horrocks LA. Phospholipase A2-generated lipid mediators in the brain: the good, the bad, and the ugly. Neuroscientist. (2006) 12:245–60. doi: 10.1177/1073858405285923
46 Phillis JW, Horrocks LA, Farooqui AA. Cyclooxygenases, lipoxygenases, and epoxygenases in CNS: their role and involvement in neurological disorders. Brain Res Rev. (2006) 52:201–43. doi: 10.1016/j.brainresrev.2006.02.002
47 Innes JK, Calder PC. Omega-6 fatty acids and inflammation. Prostaglandins Leukot Essent Fatty Acids. (2018). doi: 10.1016/j.plefa.2018.03.004
48 Parkinson JF. Lipoxin and synthetic lipoxin analogs: an overview of anti-inflammatory functions and new concepts in immunomodulation. Inflamm Allergy Drug Targets. (2006) 5:91–106. doi: 10.2174/187152806776383125
49 Livne-Bar I, Wei J, Liu HH, Alqawlaq S, Won GJ, Tuccitto A, et al. Astrocyte-derived lipoxins A4 and B4 promote neuroprotection from acute and chronic injury. J Clin Invest. (2017) 127:4403–14. doi: 10.1172/JCI77398
50 Song Y, Yang Y, Cui Y, Gao J, Wang K, Cui J. Lipoxin A4 Methyl Ester Reduces Early Brain Injury By Inhibition Of The Nuclear Factor Kappa B (NF-kappaB)-Dependent Matrix Metallopeptidase 9 (MMP-9) pathway in a rat model of intracerebral hemorrhage. Med Sci Monit. (2019) 25:1838–47. doi: 10.12659/MSM.915119
51 Shalini SM, Ho CF, Ng YK, Tong JX, Ong ES, Herr DR, et al. Distribution of Alox15 in the rat brain and its role in prefrontal cortical resolvin D1 formation and spatial working memory. Mol Neurobiol. (2018) 55:1537–50. doi: 10.1007/s12035-017-0413-x
52 Marcheselli VL, Hong S, Lukiw WJ, Tian XH, Gronert K, Musto A, et al. Novel docosanoids inhibit brain ischemia-reperfusion-mediated leukocyte infiltration and pro-inflammatory gene expression. J Biol Chem. (2003) 278:43807–17. doi: 10.1074/jbc.M305841200
53 Mukherjee PK, Marcheselli VL, Serhan CN, Bazan NG. Neuroprotectin D1: a docosahexaenoic acid-derived docosatriene protects human retinal pigment epithelial cells from oxidative stress. Proc Natl Acad Sci USA. (2004) 101:8491–6. doi: 10.1073/pnas.0402531101
54 Serhan CN. Pro-resolving lipid mediators are leads for resolution physiology. Nature. (2014) 510:92–101. doi: 10.1038/nature13479
55 Belayev L, Hong SH, Menghani H, Marcell SJ, Obenaus A, Freitas RS, et al. Docosanoids promote neurogenesis and angiogenesis, blood-brain barrier integrity, penumbra protection, and neurobehavioral recovery after experimental ischemic stroke. Mol Neurobiol. (2018) 55:7090–106. doi: 10.1007/s12035-018-1136-3
56 Bazan NG. Docosanoids and elovanoids from omega-3 fatty acids are pro-homeostatic modulators of inflammatory responses, cell damage and neuroprotection. Mol Aspects Med. (2018) 64:18–33. doi: 10.1016/j.mam.2018.09.003
57 Ho CF, Bon CP, Ng YK, Herr DR, Wu JS, Lin TN, et al. Expression of DHA-metabolizing enzyme Alox15 is regulated by selective histone acetylation in neuroblastoma cells. Neurochem Res. (2017) 43:540–55. doi: 10.1007/s11064-017-2448-9
58 Serhan CN, Hong S, Gronert K, Colgan SP, Devchand PR, Mirick G, et al. Resolvins: a family of bioactive products of omega-3 fatty acid transformation circuits initiated by aspirin treatment that counter proinflammation signals. J Exp Med. (2002) 196:1025–37. doi: 10.1084/jem.20020760
59 Terrando N, Gomez-Galan M, Yang T, Carlstrom M, Gustavsson D, Harding RE, et al. Aspirin-triggered resolvin D1 prevents surgery-induced cognitive decline. FASEB J. (2013) 27:3564–71. doi: 10.1096/fj.13-230276
60 Bazan NG. Neuroprotectin D1 (NPD1): a DHA-derived mediator that protects brain and retina against cell injury-induced oxidative stress. Brain Pathol. (2005) 15:159–66. doi: 10.1111/j.1750-3639.2005.tb00513.x
61 Sheets KG, Zhou Y, Ertel MK, Knott EJ, Regan CE Jr., Elison JR Neuroprotectin D1 attenuates laser-induced choroidal neovascularization in mouse. Mol Vis. (2010) 16:320–9. Available online at: http://www.molvis.org/molvis/v16/a38/
62 Ray PD, Huang BW, Tsuji Y. Reactive oxygen species (ROS) homeostasis and redox regulation in cellular signaling. Cell Signal. (2012) 24:981–90. doi: 10.1016/j.cellsig.2012.01.008
63 Schopfer FJ, Cipollina C, Freeman BA. Formation and signaling actions of electrophilic lipids. Chem Rev. (2011) 111:5997–6021. doi: 10.1021/cr200131e
64 Zhong H, Yin H. Role of lipid peroxidation derived 4-hydroxynonenal (4-HNE) in cancer: focusing on mitochondria. Redox Biol. (2015) 4:193–9. doi: 10.1016/j.redox.2014.12.011
65 Kumagai T, Matsukawa N, Kaneko Y, Kusumi Y, Mitsumata M, Uchida K. A lipid peroxidation-derived inflammatory mediator: identification of 4-hydroxy-2-nonenal as a potential inducer of cyclooxygenase-2 in macrophages. J Biol Chem. (2004) 279:48389–96. doi: 10.1074/jbc.M409935200
66 Pratico D, V MYL, Trojanowski JQ, Rokach J, Fitzgerald GA. Increased F2-isoprostanes in Alzheimer's disease: evidence for enhanced lipid peroxidation in vivo. FASEB J. (1998) 12:1777–83. doi: 10.1096/fasebj.12.15.1777
67 Song H, Konan LM, Cui J, Johnson CE, Langenderfer M, Grant D, et al. Ultrastructural brain abnormalities and associated behavioral changes in mice after low-intensity blast exposure. Behav Brain Res. (2018) 347:148–57. doi: 10.1016/j.bbr.2018.03.007
68 Ito F, Sono Y, Ito T. Measurement and clinical significance of lipid peroxidation as a biomarker of oxidative stress: oxidative stress in diabetes, atherosclerosis, and chronic inflammation. Antioxidants. (2019) 8:E72. doi: 10.3390/antiox8030072
69 Cohen G, Riahi Y, Sunda V, Deplano S, Chatgilialoglu C, Ferreri C, et al. Signaling properties of 4-hydroxyalkenals formed by lipid peroxidation in diabetes. Free Radic Biol Med. (2013) 65:978–87. doi: 10.1016/j.freeradbiomed.2013.08.163
70 Stockwell BR, Friedmann Angeli JP, Bayir H, Bush AI, Conrad M, Dixon SJ, et al. Ferroptosis: a regulated cell death nexus linking metabolism, redox biology, and disease. Cell. (2017) 171:273–85. doi: 10.1016/j.cell.2017.09.021
71 Yang WS, Stockwell BR. Ferroptosis: death by lipid peroxidation. Trends Cell Biol. (2016) 26:165–76. doi: 10.1016/j.tcb.2015.10.014
72 Li PL, Gulbins E. Bioactive lipids and redox signaling: molecular mechanism and disease pathogenesis. Antioxid Redox Signal. (2018) 28:911–15. doi: 10.1089/ars.2017.7467
73 Sousa BC, Pitt AR, Spickett CM. Chemistry and analysis of HNE and other prominent carbonyl-containing lipid oxidation compounds. Free Radic Biol Med. (2017) 111:294–308. doi: 10.1016/j.freeradbiomed.2017.02.003
74 Ayala A, Munoz MF, Arguelles S. Lipid peroxidation: production, metabolism, and signaling mechanisms of malondialdehyde and 4-hydroxy-2-nonenal. Oxid Med Cell Longev. (2014) 2014:360438. doi: 10.1155/2014/360438
75 Van Kuijk FJ, Holte LL, Dratz EA. 4-Hydroxyhexenal: a lipid peroxidation product derived from oxidized docosahexaenoic acid. Biochim Biophys Acta. (1990) 1043:116–8. doi: 10.1016/0005-2760(90)90118-H
76 Guichardant M, Bacot S, Moliere P, Lagarde M. Hydroxy-alkenals from the peroxidation of n-3 and n-6 fatty acids and urinary metabolites. Prostaglandins Leukot Essent Fatty Acids. (2006) 75:179–82. doi: 10.1016/j.plefa.2006.05.006
77 Bacot S, Bernoud-Hubac N, Baddas N, Chantegrel B, Deshayes C, Doutheau A, et al. Covalent binding of hydroxy-alkenals 4-HDDE, 4-HHE, and 4-HNE to ethanolamine phospholipid subclasses. J Lipid Res. (2003) 44:917–26. doi: 10.1194/jlr.M200450-JLR200
78 Castro JP, Jung T, Grune T, Siems W. 4-Hydroxynonenal (HNE) modified proteins in metabolic diseases. Free Radic Biol Med. (2017) 111:309–15. doi: 10.1016/j.freeradbiomed.2016.10.497
79 Jaganjac M, Tirosh O, Cohen G, Sasson S, Zarkovic N. Reactive aldehydes–second messengers of free radicals in diabetes mellitus. Free Radic Res. (2013) 47 (Suppl.1):39–48. doi: 10.3109/10715762.2013.789136
80 Stone MP, Cho YJ, Huang H, Kim HY, Kozekov ID, Kozekova A, et al. Interstrand DNA cross-links induced by alpha,beta-unsaturated aldehydes derived from lipid peroxidation and environmental sources. Acc Chem Res. (2008) 41:793–804. doi: 10.1021/ar700246x
81 Grimsrud PA, Xie H, Griffin TJ, Bernlohr DA. Oxidative stress and covalent modification of protein with bioactive aldehydes. J Biol Chem. (2008) 283:21837–41. doi: 10.1074/jbc.R700019200
82 Zarkovic N. Antioxidants and second messengers of free radicals. Antioxidants (Basel). (2018) 7:158. doi: 10.3390/antiox7110158
83 Luczaj W, Gegotek A, Skrzydlewska E. Antioxidants and HNE in redox homeostasis. Free Radic Biol Med. (2017) 111:87–101. doi: 10.1016/j.freeradbiomed.2016.11.033
84 Yang B, Li R, Greenlief CM, Fritsche KL, Gu Z, Cui J, et al. Unveiling anti-oxidative and anti-inflammatory effects of docosahexaenoic acid and its lipid peroxidation product on lipopolysaccharide-stimulated BV-2 microglial cells. J Neuroinflammation. (2018) 15:202. doi: 10.1186/s12974-018-1232-3
85 Sasson S. 4-Hydroxyalkenal-activated PPARdelta mediates hormetic interactions in diabetes. Biochimie. (2017) 136:85–9. doi: 10.1016/j.biochi.2016.10.007
86 Riahi Y, Sin-Malia Y, Cohen G, Alpert E, Gruzman A, Eckel J, et al. The natural protective mechanism against hyperglycemia in vascular endothelial cells: roles of the lipid peroxidation product 4-hydroxydodecadienal and peroxisome proliferator-activated receptor delta. Diabetes. (2010) 59:808–18. doi: 10.2337/db09-1207
87 Manea A, Manea SA, Todirita A, Albulescu IC, Raicu M, Sasson S, et al. High-glucose-increased expression and activation of NADPH oxidase in human vascular smooth muscle cells is mediated by 4-hydroxynonenal-activated PPARalpha and PPARbeta/delta. Cell Tissue Res. (2015) 361:593–604. doi: 10.1007/s00441-015-2120-0
88 Maulucci G, Daniel B, Cohen O, Avrahami Y, Sasson S. Hormetic and regulatory effects of lipid peroxidation mediators in pancreatic beta cells. Mol Aspects Med. (2016) 49:49–77. doi: 10.1016/j.mam.2016.03.001
89 Luczaj W, Gindzienska-Sieskiewicz E, Jarocka-Karpowicz I, Andrisic L, Sierakowski S, Zarkovic N, et al. The onset of lipid peroxidation in rheumatoid arthritis: consequences and monitoring. Free Radic Res. (2016) 50:304–13. doi: 10.3109/10715762.2015.1112901
90 Moniuszko-Malinowska A, Luczaj W, Jarocka-Karpowicz I, Pancewicz S, Zajkowska J, Andrisic L, et al. Lipid peroxidation in the pathogenesis of neuroborreliosis. Free Radic Biol Med. (2016) 96:255–63. doi: 10.1016/j.freeradbiomed.2016.04.032
91 Barrera G, Pizzimenti S, Daga M, Dianzani C, Arcaro A, Cetrangolo GP, et al. Lipid peroxidation-derived aldehydes, 4-hydroxynonenal and malondialdehyde in aging-related disorders. Antioxidants. (2018) 7:102. doi: 10.3390/antiox7080102
92 Zhang H, Forman HJ. 4-hydroxynonenal-mediated signaling and aging. Free Radic Biol Med. (2017) 111:219–25. doi: 10.1016/j.freeradbiomed.2016.11.032
93 Uchida K. HNE as an inducer of COX-2. Free Radic Biol Med. (2017) 111:169–72. doi: 10.1016/j.freeradbiomed.2017.02.004
94 Xiao M, Zhong H, Xia L, Tao Y, Yin H. Pathophysiology of mitochondrial lipid oxidation: role of 4-hydroxynonenal (4-HNE) and other bioactive lipids in mitochondria. Free Radic Biol Med. (2017) 111:316–27. doi: 10.1016/j.freeradbiomed.2017.04.363
95 Long EK, Picklo MJ Sr. Trans-4-hydroxy-2-hexenal, a product of n-3 fatty acid peroxidation: make some room HNE. Free Radic Biol Med. (2010) 49:1–8. doi: 10.1016/j.freeradbiomed.2010.03.015
96 Long EK, Murphy TC, Leiphon LJ, Watt J, Morrow JD, Milne GL, et al. Trans-4-hydroxy-2-hexenal is a neurotoxic product of docosahexaenoic (22:6; n-3) acid oxidation. J Neurochem. (2008) 105:714–24. doi: 10.1111/j.1471-4159.2007.05175.x
97 Soulage CO, Sardon Puig L, Soulere L, Zarrouki B, Guichardant M, Lagarde M, et al. Skeletal muscle insulin resistance is induced by 4-hydroxy-2-hexenal, a by-product of n-3 fatty acid peroxidation. Diabetologia. (2018) 61:688–99. doi: 10.1007/s00125-017-4528-4
98 Chen ZH, Saito Y, Yoshida Y, Sekine A, Noguchi N, Niki E. 4-Hydroxynonenal induces adaptive response and enhances PC12 cell tolerance primarily through induction of thioredoxin reductase 1 via activation of Nrf2. J Biol Chem. (2005) 280:41921–7. doi: 10.1074/jbc.M508556200
99 Huang Y, Li W, Kong AN. Anti-oxidative stress regulator NF-E2-related factor 2 mediates the adaptive induction of antioxidant and detoxifying enzymes by lipid peroxidation metabolite 4-hydroxynonenal. Cell Biosci. (2012) 2:40. doi: 10.1186/2045-3701-2-40
100 Nakagawa F, Morino K, Ugi S, Ishikado A, Kondo K, Sato D, et al. 4-Hydroxy hexenal derived from dietary n-3 polyunsaturated fatty acids induces anti-oxidative enzyme heme oxygenase-1 in multiple organs. Biochem Biophys Res Commun. (2014) 443:991–6. doi: 10.1016/j.bbrc.2013.12.085
101 Awada M, Soulage CO, Meynier A, Debard C, Plaisancie P, Benoit B, et al. Dietary oxidized n-3 PUFA induce oxidative stress and inflammation: role of intestinal absorption of 4-HHE and reactivity in intestinal cells. J Lipid Res. (2012) 53:2069–80. doi: 10.1194/jlr.M026179
102 Mauerhofer C, Philippova M, Oskolkova OV, Bochkov VN. Hormetic and anti-inflammatory properties of oxidized phospholipids. Mol Aspects Med. (2016) 49:78–90. doi: 10.1016/j.mam.2016.02.003
103 Catala A. Lipid peroxidation of membrane phospholipids generates hydroxy-alkenals and oxidized phospholipids active in physiological and/or pathological conditions. Chem Phys Lipids. (2009) 157:1–11. doi: 10.1016/j.chemphyslip.2008.09.004
104 Basu A, Kurien BT, Tran H, Byrd B, Maher J, Schell J, et al. Strawberries decrease circulating levels of tumor necrosis factor and lipid peroxides in obese adults with knee osteoarthritis. Food Funct. (2018) 9:6218–26. doi: 10.1039/C8FO01194J
105 Cortelazzo A, De Felice C, Guerranti R, Signorini C, Leoncini S, Zollo G, et al. Expression and oxidative modifications of plasma proteins in autism spectrum disorders: Interplay between inflammatory response and lipid peroxidation. Proteomics Clin Appl. (2016) 10:1103–12. doi: 10.1002/prca.201500076
106 Borovic S, Rabuzin F, Waeg G, Zarkovic N. Enzyme-linked immunosorbent assay for 4-hydroxynonenal-histidine conjugates. Free Radic Res. (2006) 40:809–20. doi: 10.1080/10715760600693422
107 Cutler RG, Kelly J, Storie K, Pedersen WA, Tammara A, Hatanpaa K, et al. Involvement of oxidative stress-induced abnormalities in ceramide and cholesterol metabolism in brain aging and Alzheimer's disease. Proc Natl Acad Sci USA. (2004) 101:2070–5. doi: 10.1073/pnas.0305799101
108 Tajuddin N, Moon KH, Marshall SA, Nixon K, Neafsey EJ, Kim HY, et al. Neuroinflammation and neurodegeneration in adult rat brain from binge ethanol exposure: abrogation by docosahexaenoic acid. PLoS ONE. (2014) 9:e101223. doi: 10.1371/journal.pone.0101223
109 Uchida K, Shibata T, Toyokuni S, Daniel B, Zarkovic K, Zarkovic N, et al. Development of a novel monoclonal antibody against 4-hydroxy-2E,6Z-dodecadienal (4-HDDE)-protein adducts: Immunochemical application in quantitative and qualitative analyses of lipid peroxidation in vitro and ex vivo. Free Radic Biol Med. (2018) 124:12–20. doi: 10.1016/j.freeradbiomed.2018.05.079
110 Weber D, Milkovic L, Bennett SJ, Griffiths HR, Zarkovic N, Grune T. Measurement of HNE-protein adducts in human plasma and serum by ELISA-Comparison of two primary antibodies. Redox Biol. (2013) 1:226–33. doi: 10.1016/j.redox.2013.01.012
111 Zarkovic K, Jakovcevic A, Zarkovic N. Contribution of the HNE-immunohistochemistry to modern pathological concepts of major human diseases. Free Radic Biol Med. (2017) 111:110–26. doi: 10.1016/j.freeradbiomed.2016.12.009
112 Mamalis A, Fiadorchanka N, Adams L, Serravallo M, Heilman E, Siegel D, et al. An immunohistochemical panel to assess ultraviolet radiation-associated oxidative skin injury. J Drugs Dermatol. (2014) 13:574–8. Available online at: https://jddonline.com/articles/dermatology/S1545961614P0574X/1/
113 Hayakawa N, Yokoyama H, Kato H, Araki T. Age-related alterations of oxidative stress markers in the mouse hippocampal CA1 sector. Exp Mol Pathol. (2008) 85:135–40. doi: 10.1016/j.yexmp.2008.05.001
114 Srividhya R, Zarkovic K, Stroser M, Waeg G, Zarkovic N, Kalaiselvi P. Mitochondrial alterations in aging rat brain: effective role of (-)-epigallo catechin gallate. Int J Dev Neurosci. (2009) 27:223–31. doi: 10.1016/j.ijdevneu.2009.01.003
115 Shibata N, Yamada S, Uchida K, Hirano A, Sakoda S, Fujimura H, et al. Accumulation of protein-bound 4-hydroxy-2-hexenal in spinal cords from patients with sporadic amyotrophic lateral sclerosis. Brain Res. (2004) 1019:170–7. doi: 10.1016/j.brainres.2004.05.110
116 Shibata N, Nagai R, Uchida K, Horiuchi S, Yamada S, Hirano A, et al. Morphological evidence for lipid peroxidation and protein glycoxidation in spinal cords from sporadic amyotrophic lateral sclerosis patients. Brain Res. (2001) 917:97–104. doi: 10.1016/S0006-8993(01)02926-2
117 Lang J, Celotto C, Esterbauer H. Quantitative determination of the lipid peroxidation product 4-hydroxynonenal by high-performance liquid chromatography. Anal Biochem. (1985) 150:369–78. doi: 10.1016/0003-2697(85)90525-1
118 Gioacchini AM, Calonghi N, Boga C, Cappadone C, Masotti L, Roda A, et al. Determination of 4-hydroxy-2-nonenal at cellular levels by means of electrospray mass spectrometry. Rapid Commun Mass Spectrom. (1999) 13:1573–9
119 Warnke MM, Wanigasekara E, Singhal SS, Singhal J, Awasthi S, Armstrong DW. The determination of glutathione-4-hydroxynonenal (GSHNE), E-4-hydroxynonenal (HNE), and E-1-hydroxynon-2-en-4-one (HNO) in mouse liver tissue by LC-ESI-MS. Anal Bioanal Chem. (2008) 392:1325–33. doi: 10.1007/s00216-008-2383-3
120 Williams TI, Lovell MA, Lynn BC. Analysis of derivatized biogenic aldehydes by LC tandem mass spectrometry. Anal Chem. (2005) 77:3383–9. doi: 10.1021/ac048265+
121 Andreoli R, Manini P, Corradi M, Mutti A, Niessen WM. Determination of patterns of biologically relevant aldehydes in exhaled breath condensate of healthy subjects by liquid chromatography/atmospheric chemical ionization tandem mass spectrometry. Rapid Commun Mass Spectrom. (2003) 17:637–45. doi: 10.1002/rcm.960
122 Wang M, Fang H, Han X. Shotgun lipidomics analysis of 4-hydroxyalkenal species directly from lipid extracts after one-step in situ derivatization. Anal Chem. (2012) 84:4580–6. doi: 10.1021/ac300695p
123 Tie C, Hu T, Jia ZX, Zhang JL. Derivatization strategy for the comprehensive characterization of endogenous fatty aldehydes using HPLC-multiple reaction monitoring. Anal Chem. (2016) 88:7762–8. doi: 10.1021/acs.analchem.6b01756
124 O'Brien-Coker IC, Perkins G, Mallet AI. Aldehyde analysis by high performance liquid chromatography/tandem mass spectrometry. Rapid Commun Mass Spectrom. (2001) 15:920–8. doi: 10.1002/rcm.324
125 Ishikado A, Morino K, Nishio Y, Nakagawa F, Mukose A, Sono Y, et al. 4-Hydroxy hexenal derived from docosahexaenoic acid protects endothelial cells via Nrf2 activation. PLoS ONE. (2013) 8:e69415. doi: 10.1371/journal.pone.0069415
126 Nagayama K, Morino K, Sekine O, Nakagawa F, Ishikado A, Iwasaki H, et al. Duality of n-3 polyunsaturated fatty acids on Mcp-1 expression in vascular smooth muscle: a potential role of 4-Hydroxy hexenal. Nutrients. (2015) 7:8112–26. doi: 10.3390/nu7095381
127 Okada T, Morino K, Nakagawa F, Tawa M, Kondo K, Sekine O, et al. N-3 polyunsaturated fatty acids decrease the protein expression of soluble epoxide hydrolase via oxidative stress-induced P38 kinase in rat endothelial cells. Nutrients. (2017) 9:E654. doi: 10.3390/nu9070654
128 Yang B, Li R, Woo T, Browning JD Jr., Song H, Gu Z, et al. Maternal dietary docosahexaenoic acid alters lipid peroxidation products and (n-3)/(n-6) fatty acid balance in offspring mice. Metabolites. (2019) 9:40. doi: 10.3390/metabo9030040
129 Carmical J, Brown S. The impact of phospholipids and phospholipid removal on bioanalytical method performance. Biomed Chromatogr. (2016) 30:710–20. doi: 10.1002/bmc.3686
130 Markesbery WR, Lovell MA. Four-hydroxynonenal, a product of lipid peroxidation, is increased in the brain in Alzheimer's disease. Neurobiol Aging. (1998) 19:33–6. doi: 10.1016/S0197-4580(98)00009-8
131 Williams TI, Lynn BC, Markesbery WR, Lovell MA. Increased levels of 4-hydroxynonenal and acrolein, neurotoxic markers of lipid peroxidation, in the brain in mild cognitive impairment and early Alzheimer's disease. Neurobiol Aging. (2006) 27:1094–9. doi: 10.1016/j.neurobiolaging.2005.06.004
132 Sun GY, Chen Z, Jasmer KJ, Chuang DY, Gu Z, Hannink M, et al. Quercetin attenuates inflammatory responses in BV-2 Microglial Cells: Role of MAPKs on the Nrf2 pathway and induction of heme oxygenase-1. PLoS ONE. (2015) 10:e0141509. doi: 10.1371/journal.pone.0141509
133 Sun GY, Li R, Yang B, Fritsche KL, Beversdorf DQ, Lubahn DB, et al. Quercetin potentiates docosahexaenoic acid to suppress lipopolysaccharide-induced oxidative/inflammatory responses, alter lipid peroxidation products, and enhance the adaptive stress pathways in BV-2 microglial cells. Int J Mol Sci. (2019) 20:E932. doi: 10.3390/ijms20040932
134 Sun GY, Simonyi A, Fritsche KL, Chuang DY, Hannink M, Gu Z, et al. Docosahexaenoic acid (DHA): an essential nutrient and a nutraceutical for brain health and diseases. Prostaglandins Leukot Essent Fatty Acids. (2017) 124:1–10. doi: 10.1016/j.plefa.2017.08.001
135 Sun GY, Simonyi A, Fritsche KL, Chuang DY, Hannink M, Gu Z, et al. Docosahexaenoic acid (DHA): an essential nutrient and a nutraceutical for brain health and diseases. Prostaglandins Leukot Essent Fatty Acids. (2018) 136:3–13. doi: 10.1016/j.plefa.2017.03.006
136 Zacek P, Bukowski M, Johnson L, Raatz SK, Picklo M. Selective enrichment of n-3 fatty acids in human plasma lipid motifs following intake of marine fish. J Nutr Biochem. (2018) 54:57–65. doi: 10.1016/j.jnutbio.2017.11.002
137 Calzada C, Colas R, Guillot N, Guichardant M, Laville M, Vericel E, et al. Subgram daily supplementation with docosahexaenoic acid protects low-density lipoproteins from oxidation in healthy men. Atherosclerosis. (2010) 208:467–72. doi: 10.1016/j.atherosclerosis.2009.07.049
138 Trepanier MO, Hopperton KE, Orr SK, Bazinet RP. N-3 polyunsaturated fatty acids in animal models with neuroinflammation: an update. Eur J Pharmacol. (2016) 785:187–206. doi: 10.1016/j.ejphar.2015.05.045
139 Fourrier C, Remus-Borel J, Greenhalgh AD, Guichardant M, Bernoud-Hubac N, Lagarde M, et al. Docosahexaenoic acid-containing choline phospholipid modulates LPS-induced neuroinflammation in vivo and in microglia in vitro. J Neuroinflammation. (2017) 14:170. doi: 10.1186/s12974-017-0939-x
140 De Smedt-Peyrusse V, Sargueil F, Moranis A, Harizi H, Mongrand S, Laye S. Docosahexaenoic acid prevents lipopolysaccharide-induced cytokine production in microglial cells by inhibiting lipopolysaccharide receptor presentation but not its membrane subdomain localization. J Neurochem. (2008) 105:296–307. doi: 10.1111/j.1471-4159.2007.05129.x
141 Srikanth M, Chandrasaharan K, Zhao X, Chayaburakul K, Ong WY, Herr DR. Metabolism of Docosahexaenoic Acid (DHA) induces pyroptosis in BV-2 microglial cells. Neuromolecular Med. (2018) 20:504–14. doi: 10.1007/s12017-018-8511-0
142 Hjorth E, Zhu M, Toro VC, Vedin I, Palmblad J, Cederholm T, et al. Omega-3 fatty acids enhance phagocytosis of Alzheimer's disease-related amyloid-beta42 by human microglia and decrease inflammatory markers. J Alzheimers Dis. (2013) 35:697–713. doi: 10.3233/JAD-130131
143 Lee WC, Wong HY, Chai YY, Shi CW, Amino N, Kikuchi S, et al. Lipid peroxidation dysregulation in ischemic stroke: plasma 4-HNE as a potential biomarker? Biochem Biophys Res Commun. (2012) 425:842–7. doi: 10.1016/j.bbrc.2012.08.002
144 Tang SC, Arumugam TV, Cutler RG, Jo DG, Magnus T, Chan SL, et al. Neuroprotective actions of a histidine analogue in models of ischemic stroke. J Neurochem. (2007) 101:729–36. doi: 10.1111/j.1471-4159.2006.04412.x
145 Guo JM, Liu AJ, Zang P, Dong WZ, Ying L, Wang W, et al. ALDH2 protects against stroke by clearing 4-HNE. Cell Res. (2013) 23:915–30. doi: 10.1038/cr.2013.69
146 Zhang M, Wang S, Mao L, Leak RK, Shi Y, Zhang W, et al. Omega-3 fatty acids protect the brain against ischemic injury by activating Nrf2 and upregulating heme oxygenase 1. J Neurosci. (2014) 34:1903–15. doi: 10.1523/JNEUROSCI.4043-13.2014
147 Liu L, Locascio LM, Dore S. Critical role of Nrf2 in experimental ischemic stroke. Front Pharmacol. (2019) 10:153. doi: 10.3389/fphar.2019.00153
148 Zhang R, Xu M, Wang Y, Xie F, Zhang G, Qin X. Nrf2-a promising therapeutic target for defensing against oxidative stress in stroke. Mol Neurobiol. (2017) 54:6006–17. doi: 10.1007/s12035-016-0111-0
149 Raoof R, Willemen H, Eijkelkamp N. Divergent roles of immune cells and their mediators in pain. Rheumatology. (2018) 57:429–40. doi: 10.1093/rheumatology/kex308
150 Sawicki CM, Kim JK, Weber MD, Faw TD, McKim DB, Madalena KM, et al. Microglia promote increased pain behavior through enhanced inflammation in the spinal cord during repeated social defeat stress. J Neurosci. (2019) 39:1139–49. doi: 10.1523/JNEUROSCI.2785-18.2018
151 Hackel D, Pflucke D, Neumann A, Viebahn J, Mousa S, Wischmeyer E, et al. The connection of monocytes and reactive oxygen species in pain. PLoS ONE. (2013) 8:e63564. doi: 10.1371/journal.pone.0063564
152 Khan AN, Jacobsen HE, Khan J, Filippi CG, Levine M, Lehman RA Jr., et al. Inflammatory biomarkers of low back pain and disc degeneration: a review. Ann N Y Acad Sci. (2017) 1410:68–84. doi: 10.1111/nyas.13551
153 Klyne DM, Barbe MF, Hodges PW. Systemic inflammatory profiles and their relationships with demographic, behavioural and clinical features in acute low back pain. Brain Behav Immun. (2017) 60:84–92. doi: 10.1016/j.bbi.2016.10.003
154 van den Berg R, Jongbloed EM, de Schepper EIT, Bierma-Zeinstra SMA, Koes BW, Luijsterburg PAJ. The association between pro-inflammatory biomarkers and nonspecific low back pain: a systematic review. Spine J. (2018) 18:2140–51. doi: 10.1016/j.spinee.2018.06.349
155 Lin Y, Chen Z, Tang J, Cao P, Shi R. Acrolein contributes to the neuropathic pain and neuron damage after ischemic-reperfusion spinal cord injury. Neuroscience. (2018) 384:120–30. doi: 10.1016/j.neuroscience.2018.05.029
156 Pereira I, Mendes SJ, Pereira DM, Muniz TF, Colares VL, Monteiro CR, et al. Transient receptor potential ankyrin 1 channel expression on peripheral blood leukocytes from rheumatoid arthritic patients and correlation with pain and disability. Front Pharmacol. (2017) 8:53. doi: 10.3389/fphar.2017.00053
157 De Logu F, Nassini R, Materazzi S, Carvalho Goncalves M, Nosi D, Rossi Degl'Innocenti D, et al. Schwann cell TRPA1 mediates neuroinflammation that sustains macrophage-dependent neuropathic pain in mice. Nat Commun. (2017) 8:1887. doi: 10.1038/s41467-017-01739-2
158 Trevisani M, Siemens J, Materazzi S, Bautista DM, Nassini R, Campi B, et al. 4-Hydroxynonenal, an endogenous aldehyde, causes pain and neurogenic inflammation through activation of the irritant receptor TRPA1. Proc Natl Acad Sci USA. (2007) 104:13519–24. doi: 10.1073/pnas.0705923104
159 Oehler B, Kistner K, Martin C, Schiller J, Mayer R, Mohammadi M, et al. Inflammatory pain control by blocking oxidized phospholipid-mediated TRP channel activation. Sci Rep. (2017) 7:5447. doi: 10.1038/s41598-017-05348-3
160 Sun AY, Ingelman-Sundberg M, Neve E, Matsumoto H, Nishitani Y, Minowa Y, et al. Ethanol and oxidative stress. Alcohol Clin Exp Res. (2001) 25(5 Suppl ISBRA):237S−43. doi: 10.1111/j.1530-0277.2001.tb02402.x
161 Moon KH, Tajuddin N, Brown J III, Neafsey EJ, Kim HY, Collins MA. Phospholipase A2, oxidative stress, and neurodegeneration in binge ethanol-treated organotypic slice cultures of developing rat brain. Alcohol Clin Exp Res. (2014) 38:161–9. doi: 10.1111/acer.12221
162 Collins MA, Tajuddin N, Moon KH, Kim HY, Nixon K, Neafsey EJ. Alcohol, phospholipase A2-associated neuroinflammation, and omega3 docosahexaenoic acid protection. Mol Neurobiol. (2014) 50:239–45. doi: 10.1007/s12035-014-8690-0
163 Butterfield DA, Bader Lange ML, Sultana R. Involvements of the lipid peroxidation product, HNE, in the pathogenesis and progression of Alzheimer's disease. Biochim Biophys Acta. (2010) 1801:924–9. doi: 10.1016/j.bbalip.2010.02.005
164 Selley ML, Close DR, Stern SE. The effect of increased concentrations of homocysteine on the concentration of (E)-4-hydroxy-2-nonenal in the plasma and cerebrospinal fluid of patients with Alzheimer's disease. Neurobiol Aging. (2002) 23:383–8. doi: 10.1016/S0197-4580(01)00327-X
165 Bradley MA, Xiong-Fister S, Markesbery WR, Lovell MA. Elevated 4-hydroxyhexenal in Alzheimer's disease (AD) progression. Neurobiol Aging. (2012) 33:1034–44. doi: 10.1016/j.neurobiolaging.2010.08.016
166 Di Domenico F, Tramutola A, Butterfield DA. Role of 4-hydroxy-2-nonenal (HNE) in the pathogenesis of alzheimer disease and other selected age-related neurodegenerative disorders. Free Radic Biol Med. (2017) 111:253–61. doi: 10.1016/j.freeradbiomed.2016.10.490
167 Butterfield DA, Boyd-Kimball D. Oxidative Stress, Amyloid-beta Peptide, and Altered key molecular pathways in the pathogenesis and progression of Alzheimer's Disease. J Alzheimers Dis. (2018) 62:1345–67. doi: 10.3233/JAD-170543
168 Butterfield DA, Boyd-Kimball D. Redox proteomics and amyloid beta-peptide: insights into Alzheimer disease. J Neurochem. (2018). doi: 10.1111/jnc.14589. [Epub ahead of print].
169 Barone E, Head E, Butterfield DA, Perluigi M. HNE-modified proteins in Down syndrome: involvement in development of Alzheimer disease neuropathology. Free Radic Biol Med. (2017) 111:262–9. doi: 10.1016/j.freeradbiomed.2016.10.508
170 Bradley MA, Markesbery WR, Lovell MA. Increased levels of 4-hydroxynonenal and acrolein in the brain in preclinical Alzheimer disease. Free Radic Biol Med. (2010) 48:1570–6. doi: 10.1016/j.freeradbiomed.2010.02.016
171 Yurko-Mauro K, McCarthy D, Rom D, Nelson EB, Ryan AS, Blackwell A, et al. Beneficial effects of docosahexaenoic acid on cognition in age-related cognitive decline. Alzheimers Dement. (2010) 6:456–64. doi: 10.1016/j.jalz.2010.01.013
172 Calon F, Lim GP, Yang F, Morihara T, Teter B, Ubeda O, et al. Docosahexaenoic acid protects from dendritic pathology in an Alzheimer's disease mouse model. Neuron. (2004) 43:633–45. doi: 10.1016/j.neuron.2004.08.013
173 Cole GM, Frautschy SA. Docosahexaenoic acid protects from amyloid and dendritic pathology in an Alzheimer's disease mouse model. Nutr Health. (2006) 18:249–59. doi: 10.1177/026010600601800307
174 Lim GP, Calon F, Morihara T, Yang F, Teter B, Ubeda O, et al. A diet enriched with the omega-3 fatty acid docosahexaenoic acid reduces amyloid burden in an aged Alzheimer mouse model. J Neurosci. (2005) 25:3032–40. doi: 10.1523/JNEUROSCI.4225-04.2005
175 Bascoul-Colombo C, Guschina IA, Maskrey BH, Good M, O'Donnell VB, Harwood JL. Dietary DHA supplementation causes selective changes in phospholipids from different brain regions in both wild type mice and the Tg2576 mouse model of Alzheimer's disease. Biochim Biophys Acta. (2016) 1861:524–37. doi: 10.1016/j.bbalip.2016.03.005
176 Romano A, Serviddio G, Calcagnini S, Villani R, Giudetti AM, Cassano T, et al. Linking lipid peroxidation and neuropsychiatric disorders: focus on 4-hydroxy-2-nonenal. Free Radic Biol Med. (2017) 111:281–93. doi: 10.1016/j.freeradbiomed.2016.12.046
177 Schaaf CP, Zoghbi HY. Solving the autism puzzle a few pieces at a time. Neuron. (2011) 70:806–8. doi: 10.1016/j.neuron.2011.05.025
178 Brown CM, Austin DW. Autistic disorder and phospholipids: a review. Prostaglandins Leukot Essent Fatty Acids. (2011) 84:25–30. doi: 10.1016/j.plefa.2010.09.007
179 Meguid NA, Dardir AA, Abdel-Raouf ER, Hashish A. Evaluation of oxidative stress in autism: defective antioxidant enzymes and increased lipid peroxidation. Biol Trace Elem Res. (2011) 143:58–65. doi: 10.1007/s12011-010-8840-9
180 Pecorelli A, Leoncini S, De Felice C, Signorini C, Cerrone C, Valacchi G, et al. Non-protein-bound iron and 4-hydroxynonenal protein adducts in classic autism. Brain Dev. (2013) 35:146–54. doi: 10.1016/j.braindev.2012.03.011
Keywords: arachidonic acid, docosahexaenoic acid, cPLA2, iPLA2, lipid peroxidation, 4-hydroxyhexenal, 4-hydroxynonenal, neurodegeneration
Citation: Yang B, Fritsche KL, Beversdorf DQ, Gu Z, Lee JC, Folk WR, Greenlief CM and Sun GY (2019) Yin-Yang Mechanisms Regulating Lipid Peroxidation of Docosahexaenoic Acid and Arachidonic Acid in the Central Nervous System. Front. Neurol. 10:642. doi: 10.3389/fneur.2019.00642
Received: 27 March 2019; Accepted: 31 May 2019;
Published: 18 June 2019.
Edited by:
Elisabetta Albi, Università Degli Studi Di Perugia, ItalyReviewed by:
Shlomo Sasson, Hebrew University of Jerusalem, IsraelAmeer Taha, University of California, Davis, United States
Akhlaq A. Farooqui, The Ohio State University, United States
Copyright © 2019 Yang, Fritsche, Beversdorf, Gu, Lee, Folk, Greenlief and Sun. This is an open-access article distributed under the terms of the Creative Commons Attribution License (CC BY). The use, distribution or reproduction in other forums is permitted, provided the original author(s) and the copyright owner(s) are credited and that the original publication in this journal is cited, in accordance with accepted academic practice. No use, distribution or reproduction is permitted which does not comply with these terms.
*Correspondence: Grace Y. Sun, c3VuZ0BtaXNzb3VyaS5lZHU=