- 1School of Health and Rehabilitation Science, The Ohio State University, Columbus, OH, United States
- 2Department of Otolaryngology—Head and Neck Surgery, The Ohio State University, Columbus, OH, United States
- 3Department of Mechanical and Aerospace Engineering, The Ohio State University, Columbus, OH, United States
- 4Department of Mechanical and Aerospace Engineering, University of Dayton, Dayton, OH, United States
- 5Department of Biomedical Engineering, The Ohio State University, Columbus, OH, United States
Aging is associated with progressive declines in both the vestibular and human balance systems. While vestibular lesions certainly contribute to imbalance, the specific contributions of age-related vestibular declines to age-related balance impairment is poorly understood. This gap in knowledge results from the absence of a standardized method for measuring age-related changes to the vestibular balance pathways. The purpose of this manuscript is to provide an overview of the existing body of literature as it pertains to the methods currently used to infer vestibular contributions to age-related imbalance.
Introduction
In the United States, 28–49% of older adults (≥65 years old) fall each year (1, 2). Falls are the most common cause of accidental death in older adults (1), and in non-fatal cases, the costs to manage fall-related sequelae are poised to exceed 55 billion dollars in 2020 (3, 4). Despite a great deal of research in the area of fall prevention (5, 6), falls, and fall-related deaths continue to rise (1) and the growing age of the United States population (4) is likely to perpetuate this trend. Although a fall can result from a variety of environmental or physiological causes (7–9), a principal contributor to falls among older adults is an age-related decline in balance (8–12). In this paper, we define the “human balance system” as the sensorimotor system that permits us to stand upright (and walk/run) even though the passive biomechanics are unstable, being comprised of interconnected inverted pendulums that are each unstable. Balance reflects “…the ability to maintain equilibrium in a gravitational field by keeping or returning the center of body mass over its base of support” (13) and is the critical component for successful (a) quiet stance, (b) compensatory postural reactions, and (c) anticipatory postural responses. It follows, then, that imbalance (i.e., the lack of balance) be defined as the inability to maintain equilibrium during quiet stance and to anticipate or respond appropriately to postural perturbations. Imbalance can be influenced by a myriad of age-associated declines in sensorimotor function, including somatosensation (14–16), vestibular function (17), vision (18), cognition (19), and strength (20, 21). Therefore, balance tests are often used to indirectly quantify these different sensorimotor contributors with different balance tests quantifying different balance elements. To develop targeted, personalized interventions to combat this epidemic of falls, it is crucial that we develop methods that isolate the primary contributors to an older adult's imbalance and fall risk.
Although deficits in any one of the aforementioned physiologic systems could likely contribute to imbalance, this review aims only to summarize studies that attempt to quantify contributions of the vestibular system to age-related balance impairment. The rationale for this decision is as follows: (1) Recent estimates suggest that falls due to vestibular impairment represent between the 3rd and the 10th leading cause of death amongst older adults (22), (2) 35% of adults over the age of 40 show evidence of vestibular dysfunction (23) and amongst adults that report unsteadiness, one third also report symptoms of dizziness (24), (3) increased noise within the vestibular system (assayed by roll tilt perceptual thresholds) has been shown to mediate 46% of age-related balance impairment in asymptomatic adults above age 40 (17), and (4) vestibular function has been shown to be modifiable with rehabilitation (25–32). Thus, we embark on this effort because despite the overabundance of evidence supporting both age-related declines in vestibular function (22, 23, 33–36) and a significant contribution of the vestibular system to postural control (37, 38), an understanding of the specific contributions of the vestibular system to age-related balance declines is lacking; recent proceedings from a National Institutes of Health workshop identified this as a critical gap in our understanding of fall prevention (39).
In an effort to address this critical knowledge gap, this manuscript aims to review the methods currently used to quantify vestibular contributions to age-related balance dysfunction including (I) measures of the peripheral vestibular end organs (i.e., otoliths and semicircular canals) that correlate with balance, (II) external stimulation of the descending vestibular pathways, (III) objective balance assessments, and (IV) measures of perceived vertical. We also highlight a recent line of research relating vestibular perceptual thresholds to age-related balance declines (V). We note that several areas of vestibular function (e.g., spatial cognition, navigation, and autonomic function) likely relevant to falls, but not directly relevant to balance, were intentionally omitted from this review given our focus on vestibular contributions to age-related imbalance. An indirect goal of this review is to motivate researchers and clinicians to develop novel methods for quantifying specific contributions of the aging vestibular system to balance impairment.
Tests of the Peripheral Vestibular Labyrinth
Semicircular Canals
Caloric irrigation is a gold standard for diagnosing a unilateral impairment in the horizontal semicircular canal and superior vestibular nerve (40), yet few studies have investigated its ability to predict balance impairment in healthy (i.e., without vestibular pathology) older adults. Jacobson et al. (41) compared the results of caloric irrigation to performance on a Modified Romberg Balance Test (MRBT) in a group of 45 asymptomatic adults over the age of 40—the age at which balance and vestibular function are thought to begin to decline (22, 23). Of the 20 subjects found to have an impaired caloric response, only 11 showed evidence of imbalance and of the 25 subjects found to have a normal caloric response, nine subjects demonstrated concurrent balance impairment (41). Similarly, in a sample of adults with balance impairment, Whitney et al. (42) identified an abnormal caloric response in only 13% (4/13) of recurrent fallers, while 44% (23/52) of subjects without a positive fall history demonstrated an abnormal caloric response. Collectively these results suggest that impairments in the low frequency vestibulo-ocular reflex (VOR) may not reflect impairment in the descending balance pathways.
Rotational chair testing measures bilateral horizontal canal function by quantifying the phase and gain of the mid to high frequency yaw VOR (43). Peterka and Black (35) found that although balance clearly declined with age, the incidence of abnormal VOR gain (±3 SD from the mean), was similar between adults with and without evidence of balance dysfunction (36). In a sample of healthy older adults, Baloh et al. (34) showed an age dependent decline in the VOR over a 5-year period despite stable balance performance. When these same participants were reassessed after an 8–10-year period, a multivariate regression model showed that although the VOR continued to decline with age, MRI evidence of white matter hyperintensities, visual acuity, and auditory function were the only variables significantly associated with changes on the Tinetti Gait and Balance (TGB) scale (a clinical measure of balance performance) (44). A subsequent study by Kerber et al. (45) did however identify a significant correlation between changes in the gain and phase of the low-frequency (0.05 Hz) VOR and TGB scores after a 9-year period. While this correlation remained after correcting for age (p < 0.001), the authors suggest that this relationship likely represents a common age-related central pathology, potentially white matter hyperintensities, that affect both balance and the VOR, rather than a causal relationship between age-related VOR declines and balance impairment (44–46). The aphysiologic vestibular stimulus (i.e., lacking head motion) during caloric irrigation (41) and the restricted frequency range of the vestibular stimuli during both caloric irrigation (0.006 Hz) and rotational chair testing [0.01–1.0 Hz (43)], relative to typical human motion [~0.8–3.2 Hz (47, 48)], pose possible explanations for the limited association between these standard assessments of canal function and balance impairment among older adults.
The video head impulse test (vHIT) instead measures canal function through the delivery of passive head rotations (i.e., impulses) that reach accelerations [~3,000°/s/s (49)] and frequencies [~6 Hz (50)] consistent with naturalistic human motion (48, 51). Over the past decade vHIT has been used to lateralize peripheral vestibular lesions (52–54) and to differentiate peripheral from central causes of an acute vestibular syndrome (55–57). However, the use of vHIT in healthy (i.e., without occult vestibular pathology) older adults has received little attention by comparison. Anson et al. (15) showed that a yaw vHIT VOR gain of <0.80 bilaterally (eye velocity <80% of head velocity) was associated with greater amounts of postural sway in the eyes closed, compliant standing (i.e., foam) condition of the MRBT. Anson and colleagues subsequently showed that for every 0.1 decrease in VOR gain, the likelihood of failing (i.e., falling prior to 40 s) this same test increased by 8% (58). Compared to the low frequency range of calorics and rotational chair testing, the high frequency content (1–6 Hz) of impulsive testing provides a stimulus to the vestibular system that is more representative of the demands of typical human locomotion [0.8–3.2 Hz (51)], providing a potential explanation for its superior association with balance impairments among older adults (50). Yet, in a group of 183 healthy older adults, Xie et al. (59) showed that yaw vHIT VOR gain was not associated with one's gait speed, narrow base of support balance, or the ability to rise from a chair. Thus, high frequency horizontal canal function, assayed using vHIT, may be most relevant for maintaining quiet stance in situations where visual and proprioceptive feedback are made less reliable (e.g., standing in a hallway at night on a thick rug while feeling for a light switch).
Whereas vHIT provides an objective measure of VOR gain, a bedside version of the HIT (bHIT) provides a less sensitive (53), but still clinically useful indicator of normal vs. abnormal (overt refixation saccade = abnormal function) horizontal canal function (60). Agrawal et al. (61) showed that after controlling for age, older adults with a positive bHIT walked 0.23 m/s slower, had 0.44 additional falls in the past year, and were 5 times more likely to have fallen in the previous 5 years. Davalos-Bichara and Agrawal (62) went on to show that 100% of older adults (n = 15) with an abnormal bilateral bHIT were unable to sustain balance in condition 4 of the MRBT [similar to the results of Anson et al. (15) when using vHIT] (62). Also since bHIT is dependent upon observation of an overt re-fixation saccade, rather than a continuous measure of VOR gain, these correlations may be representative of a more severe loss of horizontal canal function. However, despite the diagnostic utility of VOR assessments, the VOR is rarely used during daily life without an accompanying input from the visual system; thus, measures of dynamic visual acuity have been developed as complementary measures of the “real world” function of the VOR, and by extension, the horizontal canals.
The Dynamic Visual Acuity Test (DVAT) (63) is a common method for measuring the functional (or real world) status of the VOR. The DVAT requires a subject to interpret the orientation of a letter whilst the head is rotated about an earth vertical axis at a constant velocity of 120–180°/s (64). DVAT has been shown to degrade with age (63, 65) and with peripheral vestibular loss (28, 29, 63), yet few studies have investigated the relationship between DVAT and imbalance among older adults without a known vestibular diagnosis. Hall et al. (25) observed that by performing exercises which target improvements in dynamic visual acuity (i.e., gaze stabilization exercises) both fall risk and dynamic balance were improved in a sample of older adults with non-specific, presumably age-related, dizziness. Using a similar test that measured visual acuity while walking on a treadmill, Lord and colleagues however found no relationship between dynamic visual acuity and measures of balance, falls, or gait among older adults (14, 66–69).
The Gaze Stabilization Test (GST) is a methodologically distinct, yet conceptually similar test of dynamic visual acuity that measures the maximum speed at which a subject can rotate their head while retaining the ability to perceive the correct orientation of a stable (i.e., fixed size) visual target (70). In a group of 86 healthy older adults, Ward et al. (71) showed that after correcting for age, deficits on the GST (performed in yaw) were associated with poorer performance on a series of eyes closed balance tasks. Honaker et al. (72) similarly showed that in a sample of older adults the GST was significantly correlated with the Dynamic Gait Index (DGI), a measure of dynamic, ambulatory balance (r = 0.65, p = 0.00009). Honaker et al. (72) also showed that the GST (using a cutoff velocity of 100.5°/s) was able to identify participants with a positive fall history and participants who were deemed to be at a heightened risk for falls based upon their DGI scores (Sensitivity: 0.80 and 0.79, Specificity: 0.87 and 0.81, respectively). Whitney et al. (73) however showed no relationship between the GST and scores on either the DGI or the timed up and go (TUG) in a control group consisting of 20 healthy older adults.
Otolith Organs
Cervical vestibular evoked myogenic potentials (cVEMPs) use an auditory tone burst to probe the portion of the descending vestibulospinal pathway between the saccule and the motor neurons in the cervical spinal cord (74, 75). McCaslin et al. (76) showed that increased cVEMP asymmetry was associated with greater postural sway on the sensory organization test (SOT); however, this finding was made in young adults (<65 years of age) with symptoms suggestive of vestibular pathology, limiting extension to the healthy aging population. In a sample of healthy (i.e., asymptomatic) older adults, Layman et al. (77) showed a significant relationship between gait speed, an indirect measure of postural stability (78), and the latency of cVEMPs. In the only study to our knowledge to compare balance and cVEMPs in healthy older adults, Anson et al. (15) showed that a bilateral absence of cVEMP responses was associated with a mild decrease in total sway area. The interpretation of this finding is unclear since the observed effect was opposite to what would be expected (i.e., increased sway area) if abnormal cVEMPs were indeed representative of the influence of otolith dysfunction on age-related imbalance; such findings may represent a guarded postural control strategy. The effect of cVEMP loss on postural sway was also substantially smaller than that of proprioception and was only significant when sway was measured during quiet stance on a firm surface with the eyes open (15), a condition where vestibular function is least likely to be required.
The proximal location of the measured myogenic response (the sternocleidomastoid) limits the interpretation of cVEMP abnormalities as evidence of age-related decline in the distal vestibulospinal pathways that mediate balance; this provides one potential explanation for the limited correlation observed between balance and cVEMPs. To address this limitation, Rudisill and Hain (79) investigated a novel variant of cVEMPs, the aptly named “leg-VEMP,” to probe the integrity of the more distal vestibulospinal pathways by recording EMG responses from the medial gastrocnemius. The primary limitation to measuring vestibular evoked myogenic potentials distally in a muscle that controls upright stance is the increased distance between the saccule and the targeted motor neurons. The added distance results in a lower amplitude and more variable EMG signal which limits any meaningful interpretation (79). Use of an intermediary muscle group, proximal to the MG but distal to the SCM (i.e., triceps brachii) (80, 81) has been found to produce a more robust response but yields little added benefit compared to traditional cVEMPs when the goal is to investigate the influence of saccular dysfunction on postural control.
Electrical Stimulation of the Descending Vestibular Pathways
One limitation of cVEMPs is that auditory stimuli provide a rather weak stimulus to the vestibular labyrinth, with the intensity being limited by a risk of noise-induced damage to the middle ear. Galvanic vestibular stimulation (GVS) uses a direct electrical current applied to the skin overlying the mastoid processes to stimulate the vestibular afferents [predominantly irregular (82, 83)] that innervate both the otolith organs and semicircular canals (82, 84). In healthy adults, GVS causes a stereotyped response including postural sway toward the anode (i.e., away from the cathode) (85–87), ocular torsion (88–90), head and/or body tilt (91), an illusory sense of rotation (92, 93) or tilt (88), and an increase in EMG activity specifically in the muscles actively engaged in a postural task (e.g., soleus during upright stance) (94–96) [see (97, 98) for comprehensive reviews on GVS]. Studies that use GVS to probe descending vestibular control of balance typically measure either lower extremity EMG responses or the magnitude of GVS-induced postural sway (97). While well-characterized in healthy young adults (94) and subjects with vestibular pathology (85, 86), relatively few studies have investigated the responses to GVS in healthy older adults.
Welgampola and Colebatch (99) found a selective age-related change in the short latency GVS induced EMG responses in the soleus, such that the short latency responses in older adults were more delayed, more asymmetric, and lower in amplitude when compared to young adults. Dalton et al. (100) similarly found evidence of a selective decrease in the amplitude of short latency EMG signals in the soleus and medial gastrocnemius of older adults. Dalton et al. (100) also found a compensatory increase in tibialis anterior EMG responses to GVS, leading the authors to suggest a potential vestibular initiated compensation for age-related balance declines. Deshpande et al. (101) studied the influence of a combined stimulus including both GVS and a vibratory stimulus applied unilaterally to the dorsal aspect of the neck. They found that when walking with vision occluded, the young adult and older adult groups showed a similar increase in trunk velocity in the frontal plane. However, compared to young adults, the amount of lateral path deviation caused by the stimulus was attenuated in the older adult group, but this was only in regard to the response to the vibratory stimulus, not GVS (101). Tax et al. (86) however found that older adults showed a decrease in GVS induced postural sway during quiet stance. Yet, when compared to patients with bilateral vestibular hypofunction (86, 100), the reduction in GVS induced sway was minimal, potentially reflecting a more subtle decline in the descending vestibular pathways in older adults relative to patients with bilateral vestibular loss. Additional studies are needed determine if postural and EMG responses to GVS are predictive of balance impairment and fall risk in older adults.
Balance Assessments
Measuring Sensory Contributions to Balance
The Romberg (RBT) and Sharpened Romberg (SRBT) balance tests determine an individual's ability to stand with a narrow base of support (RBT, feet together; SRBT, heel to toe) when vision is removed. Performance is determined by the subject's ability to maintain balance for a predetermined time interval (typically between 15 and 60 s) and is graded as success (able to remain upright) or failure (unable to remain upright). The insensitivity of the RBT to vestibular dysfunction prompted Fregly and Graybiel (102) to investigate the SRBT (and heel to toe walking) as a method to screen for vestibular disorders. Fregly and Graybiel (102) showed that individuals with the lowest caloric responses showed the greatest deficits in both the SRBT and heel to toe walking when the eyes were closed. However, more recently, in an older adult population, Longridge et al. (103) showed that the SRBT was unable to separate healthy older adults from those with known vestibulopathy, with most subjects falling regardless of the presence of vestibular pathology; such differences from the original findings of Fregly and Graybiel (102) are likely explained by an age-associated floor effect of the SRBT due to the high degree of challenge. Such limitations provide support for the use of more comprehensive tests when attempting to detect vestibular contributions to imbalance.
One of the most frequently used methods is computerized dynamic posturography (CDP), and more specifically the sensory organization test (SOT) (36, 104–106). The SOT uses a sophisticated motion platform to quantify changes in a patient's postural sway (i.e., movement of the center of pressure, CoP) when the fidelity of visual and/or somatosensory cues is altered (105, 106). Pairing movement of either the visual surround and/or the surface with the participant's postural sway (i.e., sway referencing) diminishes one's ability to use proprioceptive and/or visual feedback for postural control. Therefore, the magnitude of postural sway is dependent upon the individual's ability to suppress any conflicting visual and/or proprioceptive cues and weight the remaining veridical cues (107). The vestibular system is the only unperturbed sensory modality controlling upright stance in conditions five and six of the SOT (SOT-5, SOT-6; Table 1), where proprioception is unreliable, and vision is either unreliable (SOT-6) or removed (SOT-5). As a result, patients with an acute vestibular lesion demonstrate marked instability in these conditions (111–115) and performance on SOT-5 and SOT-6 (relative unperturbed quiet stance, SOT-1) has been adopted as a surrogate measure of vestibulospinal function.
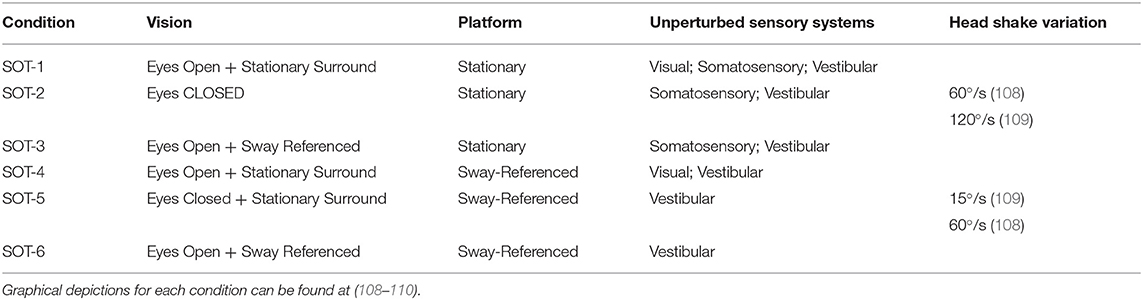
Table 1. Table consists of conditions for the SOT, including parameters for head-shake variations established by both Mishra et al. (108) and Honaker et al. (109), respectively.
Evidence supporting use of SOT to detect age-related vestibular declines is not as straight forward. The output of the SOT, postural sway, is a complex sensorimotor response that requires the integration and weighting of multiple sensory cues and a sensorimotor transformation that ultimately produces a response using the distal musculature (116). Older adults experience an increase in reaction times (117), a decline in lower extremity strength (20), and reduced distal somatosensation (14, 16, 118); each of which can potentially influence balance (118). As a result, postural sway in SOT-5 and SOT-6 cannot with certainty be attributed solely to an age-related decline in vestibular function. This is highlighted by impaired performance in SOT-5 and SOT-6 (i.e., the “vestibular conditions”), in both healthy adults (119, 120) and in patients with various central nervous system pathologies (121), two populations without vestibular impairment.
Consistent with this notion, studies using SOT-5 and SOT-6 to probe vestibular contributions to age-related imbalance have produced conflicting results over the past 30 years (122). Woollacott et al. (123) showed that older adults were more likely to fall during SOT-5 rather than SOT-6, prompting the authors to propose a pattern of imbalance consistent with dysfunction in the vestibular periphery. Similarly, Ledin et al. (124) showed that sway was significantly increased in SOT 5, but not SOT-6, when comparing participants 70–75 years of age to participants 60–69 years of age. However, several authors have since identified a different pattern of imbalance among older adults, consistent with an age-related deficiency in the ability to resolve sensory conflict (46, 119, 122, 125–128), rather than a loss of peripheral vestibular inputs. Whipple et al. (122) and Wolfson et al. (126) each showed that when compared to young adults, older adults showed a larger increase in sway and a greater prevalence of falls, in conditions with inaccurate (SOT-6), rather than absent (SOT-5), visual feedback. Peterka and Black (36), observed that older adults fell most often when experiencing conflicting visual and proprioceptive cues (SOT-6), and when a fall did not occur, sway was increased in SOT-6 relative to SOT-5. Interestingly, the older adults in Peterka and Black's study that were able to remain upright for SOT-5 showed similar amounts of sway to the young adult control group (125). More recently, Pedalini et al. (127) showed that while older adults with symptoms of vestibular impairment performed worse on SOT-4–6 than asymptomatic older adults, asymptomatic adults also performed significantly worse than young adults in each of these same conditions. Thus, instability in SOT conditions 4–6 may be sensitive to aging, independent of vestibular decline. These findings lend support to the general conclusion that the instability observed in the vestibular conditions of the SOT may be indicative of an age-related inability to suppress conflicting sensory cues (107), suggesting a breakdown in the central integration (36, 122, 126) or reweighting of multiple sensory cues, both vestibular and extra-vestibular, rather than direct evidence of impaired descending vestibular function.
Furthermore, even in subjects with a known vestibular lesion, the sensitivity of the SOT to vestibular loss has been shown to be only around 50% (109, 121, 129). In an effort to improve sensitivity, Mishra et al. (108) studied the effect of adding active yaw head rotations to SOT-2 and SOT-5 (Table 1). Yet, the aptly named head shake SOT (HS-SOT) was found to offer little benefit over the traditional SOT secondary to a ceiling effect in HS-SOT-2 and a floor effect in HS-SOT-5 (108). Park et al. (130) tested the HS-SOT in a sample of 102 healthy older adults. The ratio between SOT-5 and HS-SOT-5 was found to be 54% higher in older adults compared to young adults; the authors concluded that the increase in sway with the addition of the head shake stimulus was indicative of impaired vestibular function in the older adult group (130). However, as stated in the original study by Mishra et al. (108), the HS-SOT-5 is exceptionally challenging, such that subjects with a known history of vestibular hypofunction could not be differentiated from healthy adults due to universally poor performance. Thus, the increased instability in HS-SOT-5 compared to SOT-5 seen by Park et al. (130) may simply reflect the added challenge, rather than the presence of vestibular impairment. Honaker et al. (131) has since addressed this floor effect by decreasing the rate of head rotation in SOT-5 (Table 1), improving upon the sensitivity (70%) of HS-SOT for unilateral vestibular loss (109); future studies should consider investigating this paradigm in an older adult population.
The clinical test of sensory interaction and balance (CTSIB) is a simplified version of the SOT, developed specifically for use in a clinical setting (13, 132). Subjects perform tasks analogous to the six conditions of the SOT, with the exception that sway referenced supports are replaced by foam and sway referenced visual surrounds are replaced by an opaque globe, meant to distort but not remove visual inputs (132). CTSIB conditions are scored based on the amount of time one can maintain upright stance, up to a maximum of 30 s, in each condition (132). Cohen et al. (133) investigated the use of the CTSIB in patients with vestibular disorders compared to older adults. Cohen et al. (133) showed that conditions 4 and 6, but not condition 5, of the CTSIB were more affected by vestibular dysfunction than by healthy aging, suggesting a potential role for conditions 4 (globe plus firm surface) and 6 (globe plus foam surface) as a screening tool for vestibular dysfunction in older adults. Many clinicians and researchers have however opted for the use of a further simplified test, the modified CTSIB (mCTSIB), which consists only of static stance on both firm and foam surfaces, with the eyes open or closed (134, 135).
Condition 4 of the mCTSIB (i.e., condition 5 of the CTSIB) requires the participant to stand with the eyes closed, atop a foam pad meant to degrade somatosensory feedback from the distal lower extremity, similar to the sway referenced conditions of the SOT. Hong et al. (136) showed that although the full mCTSIB was a poor predictor of performance on the SOT, condition 4 of the mCTSIB correlated with SOT-5, demonstrating validity of this relatively simple test as an appropriate method for degrading somatosensory feedback. Since closing the eyes also removes visual input, leaving the vestibular system as the only undisturbed contributor to upright stance, an inability to maintain balance in condition 4 of the mCTSIB has been proposed as an indicator of impaired vestibulospinal function. An analogous test [a modified Romberg Balance Test (MRBT)], was recently used in a nationally representative sample of older adults, the National Health and Nutrition Evaluation Survey (NHANES), as a screening tool to estimate vestibular impairment (23). Agrawal et al. (23) reported that 34.5% of adults over the age of 40 and more than half of adults over age 60 failed condition 4 of the MRBT (i.e., could not stand 20 s on foam with eyes closed) with failure being associated with over a 6-fold increase in the odds of having experienced a fall in the previous year (23). The authors suggested that such findings represent the burden of vestibular dysfunction among older Americans (23). This suggestion has however been subsequently challenged. As described above, Jacobson et al. (41) found weak correlations between vestibular function tests and failure on the MRBT in a sample of adults over the age of 40, drawing the conclusion that the MRBT may be inappropriate as a screening tool for vestibular impairment in older adults. However, poor agreement between posturography and vestibular function tests need not discredit the applicability of such assessments, as one should expect dissimilar findings when using tests which probe different vestibular function contributions.
Static Posturography and Computational Approaches
As an alternative to the SOT assessment and the simpler RBT, sRBT, and mCTSIB assessments, a potential middle ground exists using digitized posturography tools, such as force plates, pressure sensors, and inertial measurement units, to track movement of the CoP during quiet stance. Such an approach improves upon the sensitivity of the time to failure scoring of traditional clinical balance tests (137) and takes less time to administer than the complete set of conditions performed in the SOT. Baloh et al. (138) used force plates to measure the velocity of postural sway in conditions analogous to the mCTSIB. Baloh found that older adults with a history of imbalance demonstrated an increase in sway velocity, regardless of the presence of vestibular hypofunction, when standing on foam with the eyes closed (138). Therefore, a critical consideration to making posturography during quiet stance valuable as a vestibular assessment is whether computational approaches can extract characteristics of the CoP motion that discriminate between those with vestibular hypofunction and those with normal vestibular function.
Recently, Gilfriche et al. (139) analyzed CoP data using a modified version of detrended fluctuation analysis (140), Frequency Specific Fractal Analysis (FsFA), which converts time scales (N) to frequency spectra (Fc) (Fc = Sample Frequency/N) (141). Gilfriche found that performing a fatiguing lower body exercise influenced the scaling exponent that described CoP fluctuations at higher frequencies (2–20 Hz), a frequency range thought to be dominated by somatosensory control, with no change in the lower frequency scaling exponent (<0.5 Hz), a frequency range suggested by Gilfriche to reflect visuo-vestibular control (139). Yet, such assumptions are in opposition to the preferential sensitivity of the vestibular afferents to high, rather than low, frequency stimuli (141). Consistent with this opposing view, using empirical mode decomposition, Yeh et al. (142) showed that young adults with vestibular disorders could be differentiated from healthy older adults by a characteristic increase in power within the high frequency range of the CoP time series. Thus, while these computationally driven analyses of quiet stance appear to be sensitive to both physiologic changes (i.e., somatosensory loss) and the effects of aging, the capacity to use these methods to detect alterations in postural sway that result specifically from age-related vestibular decline is still largely unknown. Future analysis of CoP complexity may benefit from 1) analyzing the dynamics of postural sway using SOT-5, condition 4 of the mCTSIB/MRBT (see above), or pseudorandom perturbations (see below), rather than unperturbed quiet stance and 2) by determining the association between the CoP frequency spectra and objective measures of sensory precision (e.g., perceptual thresholds).
Pseudorandom Perturbations
Peterka (37) developed a closed loop model of postural control to describe sagittal plane control of the center of mass when presented with ambiguous or unreliable sensory cues. In the model, postural sway results from an active corrective torque at the ankle proportional to the relative weighting of visual, proprioceptive, and vestibular cues (37). To empirically test the validity of this closed loop model, subjects were exposed to continuous rotations of the support platform or visual surround using a pseudorandom sequence of summed sinusoids while in upright stance. The perturbations were delivered under various sensory conditions (Table 2), including a sway-referenced platform or visual surround (as is done in the SOT). The use of external perturbations allows for the calculation of transfer functions that quantify the response of the postural control system as a function of the frequency spectra and amplitude of the imposed platform perturbation (37, 143). Peterka compared the gain and phase (i.e., the magnitude and timing of sway relative to the platform, respectively) of postural sway derived from these transfer functions to compare the behavior of healthy young adults to those with compensated bilateral vestibular loss (37). Peterka showed that when only vestibular and proprioceptive cues were available (i.e., with eyes closed) patients with compensated unilateral (UVL) or bilateral vestibular loss (BVL) adopted a distinct pattern of sway in response to anteroposterior tilts of the platform (37, 144). At large amplitudes of tilt, it becomes advantageous to realign the body with gravitational vertical, rather than the platform; these participants however showed a monotonic increase in postural sway at increasing angles of platform tilt, reflecting a tendency to continue to align the center of mass with the support surface (37, 144). In the context of the closed loop model of postural control, this pattern can be interpreted as a persistent reliance upon proprioceptive feedback or a deficiency in the reweighting of the absent (BVL) or impaired (UVL) vestibular cues; this is highlighted by the comparison to healthy adults who instead readily reweight the intact vestibular cue, aligning the body with gravitational vertical rather than the platform during larger amplitude perturbations (37, 144).
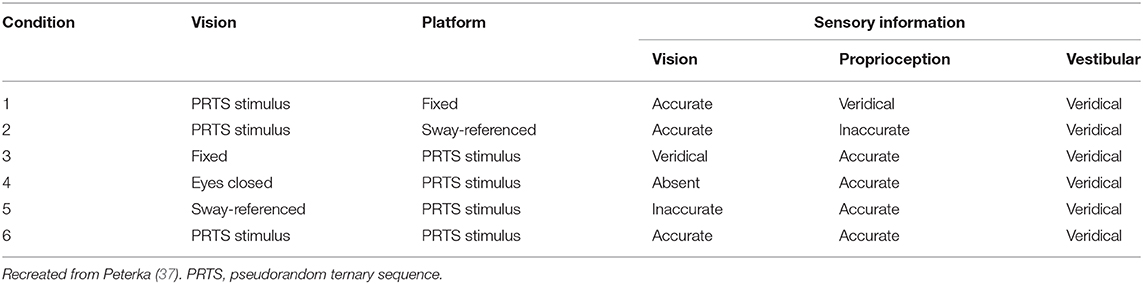
Table 2. Conditions of the protocol developd by Peterka (37) using pseudorandom perturbations to model postural sway.
The aforementioned studies (37, 144) included only patients who were more than a year removed from their injury and therefore were believed to be well-compensated (145); this highlights the sensitivity of this method to subtle vestibular declines, such as occur with aging (34). Cenciarini et al. (146) used pseudorandom platform tilts in a sample of healthy older adult subjects and showed that, relative to young adults, older adults showed a compensatory increase in stiffness and damping parameters, interpreted by the authors as evidence that older adults adopt a unique compensatory strategy to maintain balance (146). Unfortunately investigating the weighting of vestibular and proprioceptive cues was not a goal of their experiment. Wiesmeir et al. (147) showed that unlike patients with vestibular loss, healthy older adults showed a retained capacity to increase the use of vestibular cues at higher amplitudes of platform tilt. Yet, they also found that relative to young adults, older adults displayed a consistently increased weighting of proprioceptive cues. Since the group of older adults were asymptomatic and had normal VOR function on rotational testing, the observed age-related changes in sensory reweighting mechanisms may be related to subclinical declines in vestibular pathways distinct from those mediating the VOR. Yet, the relevant vestibular structure (i.e., otolith, canal, or central) underlying the ability to orient one's self with gravitational vertical during dynamic pseudorandom perturbations remains unknown.
Standardized Measures of Functional Mobility
The Fukuda (i.e., Unterburger) Stepping Test is a classic bedside test designed to assess descending vestibulospinal control by measuring the degree of rotation that occurs while stepping in place with the eyes closed (148). Studies showing poor sensitivity and specificity of the Fukuda Stepping Test for vestibular dysfunction (131, 149) have led many to adopt use of more comprehensive functional assessments. Several standardized tests incorporate elements meant explicitly to perturb the vestibular system (e.g., rotating the head and/or body) to determine a vestibular cause to postural instability. The timed up and go (TUG), DGI, and functional gait assessment (FGA) measure dynamic ambulatory balance and are capable of predicting fall risk among older adults (150–152) and patients with vestibular loss (153, 154). The DGI and FGA require subjects to ambulate while rotating their head in either pitch or yaw (151, 155), whereas the TUG requires subjects to walk a distance and then turn the body 180 degrees while walking (154). Although individuals with vestibular impairment struggle with the aforementioned tasks (154, 156, 157), it is worth emphasizing that the vestibular system is not the sole respondent to the imposed stimuli. Chang and Schubert (158) showed that DGI scores improved independent of VOR gain improvement in a sample of patients recovering from unilateral vestibular deafferentation surgery. Actively rotating the head involves vestibular, cervical proprioceptive, motor efferent, and visual cues; a compensatory change to any of these extra-vestibular systems could therefore improve balance performance independent of vestibular loss or recovery. Thus, imbalance provoked by movement of the head, while certainly a symptom of suboptimal vestibular function, cannot definitively indicate vestibular loss. Cohen et al. (134) did however find that the addition of yaw and pitch head movements improved the sensitivity of eyes closed heel to toe walking and the mCTSIB to vestibular pathology (e.g., Meniere's disease, BPPV) in older adults aged 40–79; whether this finding is generalizable specifically to sub-clinical vestibular declines, rather than the identification of vestibular pathology, remains to be seen.
Overall, functional outcome measures allow clinicians to efficiently predict fall risk and are often used to guide rehabilitative efforts (152, 155, 159). The capacity to mimic real world balance perturbations (e.g., stepping over an object) likely explains their predictive abilities. However, this functional emphasis conversely prevents such tests from isolating the culpable system causing the balance disturbance and this is particularly true in the evaluation of the older adult patient, as imbalance on clinical balance tests can be caused by a number of extra-vestibular age-related changes in sensorimotor function (e.g., kinesthesia, strength) (160).
Perception of Subjective Vertical
Subjective Visual Vertical
Tests of subjective visual vertical (SVV) quantify the relative contributions of otolith (predominantly utricle), visual, and somatosensory cues by estimating an individual's perception of gravitational vertical [See (161) for a review]. While methods vary, SVV generally requires an individual to orient an illuminated bar with what they perceive to be true vertical; testing can take place either in the dark (i.e., no veridical visual cues) or in the presence of a moving (dynamic SVV) or inaccurate (rod-and-frame SVV) visual cue. Tobis et al. (162) found that older adult fallers showed a significant bias in SVV in the direction of a tilted frame that surrounded the vertical bar (i.e., rod-and-frame SVV), suggesting an increased weighting of visual, relative to otolith cues, presumably as a compensation for vestibular declines (161). Lord and Webster (163) showed that when compared to healthy older adults, older adults with a positive fall history displayed greater errors (deviation from true vertical) in both static (fixed background) and dynamic (rotating background) SVV tasks. Barr et al. (164) showed that when in the presence of a rotating visual surround, a deviation in SVV of at least 6.5° was associated with greater fall risk and a significant decrease in postural sway when standing with the eyes open or closed, and when standing in the presence of a moving visual field; such patterns suggest the adoption of a maladaptive stiffening of one's postural control strategy. Together, these results suggest that changes in the weighting of otolith relative to visual cues may predict altered postural control strategies and fall risk in older adults.
The presence of a stereotyped bias in SVV toward the side of an acute unilateral vestibular lesion suggests that the otolith organs are at least one of the principle contributors to one's estimate of subjective vertical (165). Yet, SVV is restored within weeks to months after a vestibular lesion (166, 167). This suggests that an individual with persistent vestibular loss can restore SVV by appropriately weighting residual vestibuar cues with intact visual, somatosensory (168) and/or somatic graviceptive (e.g., vascular receptors signaling a shift in blood volume within the trunk) cues (169). Also, it appears that a maladaptive reweighting of verticality cues, rather than isolated vestibular loss, may explain the correlations observed between SVV, fall risk, and postural sway in older adults, as such relationships are observed when SVV is measured in the presence of a spurious visual reference (i.e., tilted frame or rotating background) (162–164).
Subjective Postural Vertical
SPV is typically measured by having an individual report when they perceive their body to be aligned with gravitational vertical. SPV can be quantified both by the accuracy (deviation relative to upright) and precision (variability around the perception of upright) of their estimate of vertical (161). Barbierri et al. (170) showed that SPV gradually tilts backward with age, with nearly a doubling of backward inclination in healthy adults over 50 (−1.15° ± 1.40) when compared to adults 18–49 years of age (−0.45° ± 0.97, p < 0.01). Manckoundia et al. (171) found that this age-associated posterior displacement of the SPV was highly correlated (r = 0.95, p < 0.000001) with the extent of backward instability during a series of balance and mobility tasks (e.g., sitting, standing with and without vision and transitioning between sitting and standing). This deviation of SPV among older adults has been posited as a potential explanation for an asymmetrical pattern of postural instability experienced by older adults referred to as “backward disequilibrium” (170–172). Menant et al. (173) showed that both the precision and accuracy of SPV in the mediolateral direction was also significantly correlated with lateral sway (r = 0.20 and 0.16, respectively) measured during stance in a foam, eyes closed condition. This relationship persisted after correcting for age, gender, and other general indicators of mobility (i.e., the physiological profile assessment); considering the large sample size (N = 195), this finding provides convincing evidence that perceived vertical may be a potentially useful indicator of age-related balance dysfunction.
Yet, Bisdorf et al. (165) showed that neither chronic nor acute vestibular lesions were sufficient to induce a bias in SPV. Anastopolous similarly showed that SPV remained veridical following an acute vestibular lesion, despite a consistent tilt of SVV toward the side of the vestibular lesion (174). Ito and Gresty (175) showed that even in individuals with a total surgical loss of vestibular function (due to surgery to correct Neurofibromatosis Type II), the accuracy of SPV remained similar to healthy adults. These studies suggest that unlike SVV, otolith dysfunction has minimal impact on the accuracy (i.e., bias) of SPV. Instead, the increased variability of SPV in patients with bilateral vestibular loss and acute unilateral lesions suggests that graviceptive otolith cues may instead be more relevant for reducing the variability in one's estimate of postural vertical (161, 165). A clear bias in SPV has however been observed in individuals with somatosensory loss affecting the trunk (176, 177). Thus, the association between imbalance and the observed age-related shift in SPV, may be indicative of the impact of age-related somatosensory declines or alterations in the central integration of somatic graviceptive, somatosensory, and vestibular cues.
Vestibular Noise
Vestibular Perceptual Thresholds
Neural noise (referred to herein as “noise”) is the random fluctuation in neural activity that occurs within each sensory system (178, 179). While low levels of external noise can enhance sensory signals [i.e., stochastic resonance (180)], excess internal noise impairs the communication between neurons, and has been posited as a potential cause of age-related cognitive decline (178, 181, 182). Neurophysiologic studies have provided evidence for age-related increases in neural noise, showing a relationship between electroencephalogram (EEG) 1/f power spectral density and both language and visual working memory in older adults (182). Behavioral studies have similarly shown that background sensory noise impairs the perception of visual images in older adults (178). Psychophysical assessments of self-motion perception (“vestibular perceptual thresholds”) provide evidence that neural noise may also affect the aging vestibular system (22, 183, 184).
Consistent with classic signal detection theory (185), a vestibular threshold represents the minimum stimulus value at which a subject can accurately (at a pre-defined accuracy level) perceive the direction of passive self-motion while using predominantly vestibular cues (186). In other words, a perceptual threshold is the magnitude of a vestibular stimulus required to overcome the level of internal noise within an individual's vestibular system, thereby allowing the self-motion signal to be reliably perceived by the subject (e.g., “I moved right”); thus, vestibular thresholds are an assay of vestibular noise (186). Although methods may vary, one-interval direction recognition tasks (e.g., “did I move right or left?”) are the most common psychophysical technique for quantifying vestibular perceptual thresholds (186). By quantifying vestibular noise during self-motion, vestibular thresholds can independently assess each vestibular modality (tilt, rotation, and translation). While a comprehensive review of vestibular perceptual testing is beyond the scope of this review, we refer the interested reader to several reviews on the topics of signal detection theory and vestibular perceptual thresholds (185–189).
Vestibular Thresholds and Age-Related Imbalance
Vestibular thresholds have been shown to increase after age 40 in all planes of motion including supero-inferior translations, interaural translations, roll tilt, and yaw rotation (22). Despite thresholds being moderately correlated with one another, only low frequency (0.2 Hz or 5 s per motion) roll tilt perceptual thresholds show a significant association with age-related imbalance (190). Elevated roll tilt thresholds were found to be associated with an increase in the likelihood of failing (i.e., falling prior to the end of the 30 s trial) the “eyes closed, standing on a compliant surface” test condition of the MRBT in a sample of healthy adults over the age of 40 (190); this statistically significant relationship remained even after accounting for age. In a subsequent mediation analysis of these data, Beylergil et al. (17) showed that roll-tilt thresholds significantly mediated the relationship between age and balance impairment. Despite the multitude of factors that contribute to age-related balance impairment (e.g., vision, cognition, kinesthesia), this analysis showed that age-related increases in roll tilt thresholds accounted for nearly half (46%) of the relationship between age and balance impairment (17). We posit three possible explanations for this admittedly surprising finding, (1) roll tilt perceptual thresholds may represent a physiologically relevant vestibular cue (190) due to the inherent need for human beings to respond to changes in head position relative to gravity (i.e., tilt) in order to remain upright, (2) roll tilt perceptual thresholds may reflect a more global measure of central vestibular function, as the precision of roll tilt perception is dependent upon the ability of the aging brain to perform (191–197) complex neural computations to resolve ambiguous gravitoinertial cues from the otolith organs, and (3) canal and otolith cues are integrated during most, if not all, naturalistic human motions (198), and thus impaired roll tilt perception may specifically reflect imperfections associated with canal-otolith integration. Yet, similar to vHIT, such comparisons have been restricted to balance performance in quiet stance on a foam pad with the eyes closed, and thus one must be cautious with the extrapolation of these findings to alternative aspects of human balance.
Noise, Aging, and Velocity Storage
Recent empirical and modeling efforts have further implicated noise as an explanation for age-related changes in the temporal characteristics of the VOR (199) and in age-related changes in postural control (200). During a sustained, constant velocity yaw rotation about an earth vertical axis, activity in the peripheral vestibular afferents degrades quickly, with a time constant of around 4–6 s (141, 201, 202). However, compensatory nystagmus and the subjective perception of rotation persist up to 30 s (34, 43, 203); this propagation of behavioral vestibular activity is accomplished through a mechanism called velocity storage [see (204) for a review on this topic but also see (205, 206) for differing perspectives]. A reduced time constant (i.e., faster decay of nystagmus) has been identified in subjects with unilateral and bilateral vestibular loss (207), in those with central vestibular dysfunction (208), and in asymptomatic older adults (34, 35, 203, 209).
Karmali proposed an explanation for the observed effect of aging on the vestibular time constant (Tc) using a Bayesian framework (199, 210–212). Extending the Tc beyond the time constant of peripheral vestibular afferents requires the repeated integration of canal signals (213) which amplifies the accompanying noise (199). Thus, while prolonging the Tc results in a more accurate estimate of rotation, it comes at the cost of an accumulation of noise (i.e., reducing precision) (212). Using a Bayesian optimal Kalman filter model, Karmali et al. (199) showed that the age-related shortening of the Tc may be proportional to published data describing the rate of age-related decline in the vestibular hair cells (214). Karmali suggested that in order to compensate for this decline in the vestibular signal to noise ratio, the CNS may dynamically shorten the Tc to achieve an optimal balance between accuracy and precision (199, 212).
While vestibular noise, assayed by roll tilt perceptual thresholds, has been found to be associated with age-related balance impairment, similar work comparing the Tc to measures of balance is sparse. In the only study to our knowledge to empirically compare the Tc and balance, Jacobson et al. (215) showed that a lower Tc, even in the presence of normal VOR gain, was associated with greater postural instability among otherwise healthy older adults. It is worth noting that concomitant ischemic pontine lesions were also identified in ~80% of the older adults with a balance impairment and shortened Tc, suggesting that velocity storage may be reflective of central vestibular dysfunction in older adults (215). Additional work is needed to determine if the Tc represents the contribution of central vestibular pathways to age-related balance declines.
Discussion
Measures of low frequency horizontal canal function (i.e., caloric irrigation and rotational chair testing) appear to provide minimal benefit when attempting to identify a vestibular cause to age-related imbalance. Alternative measures of canal function that include the integration of visual cues (i.e., GST) or a high frequency stimulus (i.e., vHIT), have instead demonstrated a capacity to predict postural instability and fall risk in older adults (71, 216). Given that correlations remained even after correcting for age, these findings indicate that vHIT and GST may be suitable measures to quantify specific contributions of the horizontal canal to age-related imbalance. However, one must consider several limitations when relying on assessments of the VOR to quantify the influence of impaired canal function on age-related imbalance.
Measures of the VOR are not solely measures of canal function, but instead reflect the integrity of the motor limb of the reflex (e.g., oculomotor plant) and can be influenced by extra-vestibular oculomotor strategies (e.g., compensatory saccades) (64, 217, 218). This point is highlighted by the typical course of recovery following unilateral vestibular loss (UVD). Dynamic visual acuity improves independent of any restoration in peripheral vestibular function (26, 27, 145, 219) secondary to the recruitment of compensatory saccades (220), the unmasking of cervical proprioceptive inputs (221–224) and the integration of motor efferent signals (64, 217, 218). Also, while the horizontal canals feed into both the ascending and descending vestibular pathways, VOR pathways are anatomically distinct from the vestibulospinal pathways (222, 223), diverging at the level of second order neurons in the vestibular nuclei [VOR via position vestibular pause (PVP) and vestibulospinal via vestibular only (VO) neurons, respectively; see (225) for a review on this topic]. Thus, the association between VOR measures and imbalance may be representative of imbalance that results from unstable gaze (the function of the VOR), rather than as an indication that such measures directly assay the pathway connecting the horizontal canals to the descending vestibular pathways. Nevertheless, VOR assessments irrefutably remain vital to lateralize vestibular loss (226, 227).
Since balance assessments, namely the SOT, directly measure balance performance, in principle, they are likely considered better suited to identify imbalance that results from vestibular impairment. The vestibular conditions of the SOT (i.e., SOT-5 and 6) have however shown limited sensitivity (109, 121, 129) and specificity (119–121) when used as a diagnostic site of lesion test. This is likely a result of the inability to differentiate a patient with restored vestibular function from a patient who has successfully re-weighted extra-vestibular cues and the analytical consideration of the “vestibular sense” as a single sense, when, in fact, it includes at least 3 sensory modalities (rotation, translation, tilt) (228, 229). These issues are accentuated when examining older adults due to (1) the inability to separate dysfunction of the descending vestibular pathways from alternative age-associated sensorimotor declines, (2) the relative insensitivity to subtle changes in vestibular function, and (3) the fact that all vestibular modalities, that may decline at different rates with aging, are lumped into a single entity. This is not meant to dissuade clinicians and researchers from using the SOT when vestibular decline is suspected, but rather to suggest that it be used as a vestibular screening tool and/or be paired with more direct assays of vestibular function. Asai et al. (230) showed that sensitivity of the SOT was improved when considered in conjunction with positive findings on a standard vestibular test battery. Cohen et al., recently showed that combining SOT-5 with a clinical balance test [e.g., the functional mobility test (FMT), Timed Up and Go (TUG), or Dynamic Gait Index (DGI)] improved sensitivity to vestibular impairment in a group of young adults (231).
Some of these limitations of the SOT may be addressable by treating postural sway as the output of a closed loop system, rather than directly as evidence of instability. In the SOT, as is true in nearly all balance tests, increased movement of the CoP is interpreted as evidence of instability. However, considering the magnitude of postural sway in isolation does not in of itself indicate instability, and may instead represent exploratory behavior (232) or could be the result of a compensatory re-weighting of sensory cues (37). By measuring sway evoked by continuous pseudorandom perturbations, one can compute transfer functions which determine if the center of mass orients relative to the stimulus (i.e., tilting platform), suggesting persistent weighting of proprioceptive cues, or with gravitational vertical, suggesting an amplitude dependent reweighting of vestibular cues (37, 233); this approach provides a quantitative explanation for the observed postural sway (37). Thus, in experimental conditions that alter the reliability of sensory cues (i.e., eyes closed or sway referencing) such an approach may offer improvements on the SOT by determining the postural control strategy being used to respond to the externally imposed perturbations and the relative weights assigned to each sensory channel. Pseudorandom stimuli also have the advantage of providing a less predictable perturbation to the vestibular system, an approach previously shown to be more discriminatory for identifying vestibular pathology (217).
Yet, an inherent limitation to the use of any complex sensorimotor task, including the SOT or pseudorandom perturbations, is that the output (i.e., sway as measured using CoP or CoM) is the only quantifiable indicator of performance. As a result, if one is attempting to determine if vestibular sensory function is the cause of a patient's imbalance or falls, this can only be indirectly inferred from the pattern of motor outputs. Similar to compensatory adjustments of the VOR by the oculomotor limb of the VOR, the results of posturographic assessments must be considered in light of potential downstream alterations in the motor limb of the vestibulospinal reflexes. In an oversimplified example, consider for a moment the optimal output of the vestibulospinal reflex in response to a perturbation, such as a sudden translation of the head. If the vestibular afferents, due to an age-related loss of hair cells (214), trigger a motor response that compensates for only 80% of the perturbation, the descending motor system could (in theory) simply boost its gain to 120% and the net output of postural sway will mirror a system with normal vestibular function. Redundancies and compensatory mechanisms, while beneficial to maintain mobility and prevent falls, can make isolating the cause of balance disturbance a daunting task; such limitations motivate attempts to directly stimulate the vestibular labyrinth.
Beyond head motion, GVS may provide the most direct method for measuring the descending vestibular reflexes. The interpretation of GVS is however bounded by the dependence of the motor outputs on the context of the experimental task (234). Width of the base of support (91, 235), upper extremity support (96), availability of vision (96, 235, 236), reliability of proprioceptive feedback (234, 235), and testing posture (sitting vs. standing) (91, 96) have each been shown to modulate the magnitude of GVS induced EMG and/or postural responses, and thus can result in attenuated or enhanced responses to GVS, independent of the integrity of the descending vestibular pathways. The use of GVS paradigms in older adults can therefore only be interpreted relative to the reliability of extra-vestibular sensory cues [somatosensory (236, 237) and visual (234)] which are known to also decline with age (14, 118, 238, 239). Comparing responses to GVS in healthy older adults to older adults with vestibular decline, using tightly controlled experimental conditions that control for age-related visual and somatosensory loss, could however yield an interesting avenue for future research.
The limitations of existing assessments have motivated our recent study of vestibular perceptual thresholds as an assay of vestibular noise (17, 22, 190). As mentioned above, research out of our lab identified a significant association between 0.2 Hz roll tilt perceptual thresholds and age-related imbalance, yet, we emphasize that we are not suggesting that an inability to perceive roll-tilt is the cause of age-related balance impairment. We instead put forward the hypothesis that age-related changes in roll tilt vestibular thresholds reflect central vestibular noise that affects both perception and balance and thus may provide a method to quantify contributions of the central vestibular system to age-related balance impairment. The significant mediating effect of roll tilt thresholds and balance may therefore implicate central vestibular noise as a principle contributor to age-related imbalance. Considering that the participants studied were without symptoms of vestibular impairment, the observed age-related increase in vestibular thresholds also highlights the potential impact of sub-threshold (i.e., below the threshold for triggering symptoms) vestibular noise on age-related imbalance.
Although speculative, one potential explanation for this association is an age-related increase in noise within a specialized class of neurons within the vestibular nuclei [vestibular-only (VO) neurons] that act as relays for both vestibulospinal control and self-motion perception [see (240) for a review]. As justification for this hypothesis, the VOR and self-motion perceptual pathways relay through different neurons in the VN, and as such show qualitatively dissimilar behavior (193, 194). As an alternative explanation, since the perception of tilt is dependent upon one's ability to use vertical canal cues to disambiguate changes in net gravitoinertial force sensed by the otoliths (195), roll tilt thresholds may instead more generally represent a measure of age-related changes in central canal-otolith integration. Wiesmeir showed that postural responses to pseudorandom tilts of the support surface differed significantly between young and older adults within the frequency range (0.15–0.40 Hz) (147) where canal and otolith cues have been shown to be optimally integrated when rotating about an earth horizontal axis (241). Similar performance between age groups at 0.05 Hz suggests that at frequencies below the threshold for vertical SCC activation, where otolith cues predominate, the gain of postural sway is instead unaffected by age (241). Such findings lend support to our hypothesis that an age-associated increase in central vestibular noise, manifesting as a breakdown in central canal-otolith integration, may serve as a principle contributor to age-related balance declines.
While such explanations are plausible, as of now empirical proof is lacking, thus it does remain possible that roll tilt perceptual thresholds and balance are simply parallel yet independent consequences of aging. However as previously stated by Karmali et al. (190), this alternative is unlikely given that one would expect that if a common central nervous system pathology caused the correlation between roll tilt thresholds and balance, that most, if not all, vestibular thresholds, not just 0.2 Hz roll tilt, would display this same predictive relationship. Nevertheless, correlation does not equal causation, therefore additional studies are needed to (1) determine if the relationship between vestibular noise and age-related balance impairment is indeed causal, (2) to confirm or refute these findings in an independent sample of older adults, and (3) determine the mechanism explaining the association. Determining if alternative measures of vestibular noise, such as the vestibular time constant (199, 212) or measures of VOR variability (242), display similar associations with age-related balance impairment may provide further insights.
Summary
A standardized method for determining the specific contributions of the vestibular system to age-related balance impairment has yet to be developed. Although traditional measures of vestibular function allow one to infer the integrity of the peripheral or central vestibular structures, the extent by which they capture the specific subclinical age-related changes that affect the vestibular balance pathways appears limited (Table 3). Standardized balance assessments are sensitive to acute vestibular lesions and predict fall risk, yet, fail to account for the multi-modal nature of the vestibular system. One hypothesis arising from recent basic research (17, 22, 23, 190) is that roll tilt vestibular thresholds reflect central vestibular noise that affects both perception and balance and thus may provide a method to quantify contributions of the central vestibular system to age-related balance impairment. Identifying a method to detect vestibular contributions to age-related postural instability would support efforts to screen for vestibular mediated fall risk and could potentially permit earlier implementation of targeted rehabilitative interventions.
Author Contributions
AW and DM conceptualized the manuscript. AW created the initial draft of the manuscript. OA, AC, KB, and DM contributed to the writing and editing of the manuscript. All authors contributed to the article and approved the submitted version.
Funding
DM was funded by NIH/NIDCD R01-DC014924. DM, AC, and KB were funded by DoD Congressionally Directed Medical Research Program (CDMRP) grant W81XWH1920003. AC was funded by NIH/NIA R42-AG062065. AW was funded in part by a Promotion of Doctoral Studies (PODS) I Scholarship from the Foundation for Physical Therapy Research.
Conflict of Interest
The authors declare that the research was conducted in the absence of any commercial or financial relationships that could be construed as a potential conflict of interest.
References
1. Burns E, Kakara R. Deaths from falls among persons aged ≥65 years - United States, 2007-2016. MMWR Morb Mortal Wkly Rep. (2018) 67:509–14. doi: 10.15585/mmwr.mm6718a1
2. Bergen G, Stevens MR, Burns ER. Falls and fall injuries among adults aged ≥65 years - United States, 2014. MMWR Morb Mortal Wkly Rep. (2016) 65:993–8. doi: 10.15585/mmwr.mm6537a2
3. Florence CS, Bergen G, Atherly A, Burns E, Stevens J, Drake C. Medical costs of fatal and nonfatal falls in older adults: medical costs of falls. J Am Geriatr Soc. (2018) 66:693–8. doi: 10.1111/jgs.15304
4. Houry D, Florence C, Baldwin G, Stevens J, McClure R. The CDC injury center's response to the growing public health problem of falls among older adults. Am J Lifestyle Med. (2016) 10:74–7. doi: 10.1177/1559827615600137
5. Guirguis-Blake JM, Michael YL, Perdue LA, Coppola EL, Beil TL. Interventions to prevent falls in older adults: updated evidence report and systematic review for the US Preventive Services Task Force. JAMA. (2018) 319:1705. doi: 10.1001/jama.2017.21962
6. Gillespie LD, Robertson MC, Gillespie WJ, Sherrington C, Gates S, Clemson LM, et al. Interventions for preventing falls in older people living in the community. Cochrane Database Syst Rev. (2012) 9:CD007146. doi: 10.1002/14651858.CD007146.pub3
7. Blake AJ, Morgan K, Bendall MJ, Dallosso H, Ebrahim SBJ, Arie THD, et al. FALLS BY ELDERLY PEOPLE AT HOME: PREVALENCE AND ASSOCIATED FACTORS. Age Ageing. (1988) 17:365–72. doi: 10.1093/ageing/17.6.365
8. Tinetti ME, Speechley M, Ginter SF. Risk factors for falls among elderly persons living in the community. N Engl J Med. (1988) 319:1701–7. doi: 10.1056/NEJM198812293192604
9. Nevitt MC. Risk factors for recurrent nonsyncopal falls: a prospective study. JAMA. (1989) 261:2663. doi: 10.1001/jama.1989.03420180087036
10. Bergland A. Risk factors for serious fall related injury in elderly women living at home. Inj Prev. (2004) 10:308–13. doi: 10.1136/ip.2003.004721
11. Bergland A, Jarnlo G-B, Laake K. Predictors of falls in the elderly by location. Aging Clin Exp Res. (2003) 15:43–50. doi: 10.1007/BF03324479
12. Muir SW, Berg K, Chesworth B, Klar N, Speechley M. Quantifying the magnitude of risk for balance impairment on falls in community-dwelling older adults: a systematic review and meta-analysis. J Clin Epidemiol. (2010) 63:389–406. doi: 10.1016/j.jclinepi.2009.06.010
13. Horak FB. Clinical measurement of postural control in adults. Phys Ther. (1987) 67:1881–5. doi: 10.1093/ptj/67.12.1881
14. Lord SR, Clark RD, Webster IW. Postural stability and associated physiological factors in a population of aged persons. J Gerontol. (1991) 46:M69–M76. doi: 10.1093/geronj/46.3.M69
15. Anson E, Bigelow RT, Swenor B, Deshpande N, Studenski S, Jeka JJ, et al. Loss of peripheral sensory function explains much of the increase in postural sway in healthy older adults. Front Aging Neurosci. (2017) 9:202. doi: 10.3389/fnagi.2017.00202
16. Deshpande N, Simonsick E, Metter EJ, Ko S, Ferrucci L, Studenski S. Ankle proprioceptive acuity is associated with objective as well as self-report measures of balance, mobility, and physical function. AGE. (2016) 38:53. doi: 10.1007/s11357-016-9918-x
17. Beylergil SB, Karmali F, Wang W, Bermúdez Rey MC, Merfeld DM. Vestibular roll tilt thresholds partially mediate age-related effects on balance. In: John Leigh R, Ramat S, Shaikh A, editors. Progress in Brain Research (Amsterdam: Elsevier). p. 249-67.
18. Hong T, Mitchell P, Burlutsky G, Samarawickrama C, Wang JJ. Visual impairment and the incidence of falls and fractures among older people: longitudinal findings from the blue mountains eye study. Investig Opthalmol Vis Sci. (2014) 55:7589. doi: 10.1167/iovs.14-14262
19. Semenov YR, Bigelow RT, Xue Q-L, Lac S du, Agrawal Y. Association between vestibular and cognitive function in U.S. adults: data from the national health and nutrition examination survey. J Gerontol A Biol Sci Med Sci. (2016) 71:243–50. doi: 10.1093/gerona/glv069
20. Carter ND, Khan KM, Mallinson A, Janssen PA, Heinonen A, Petit MA, McKay HA, Fall-Free BC Research Group. Knee extension strength is a significant determinant of static and dynamic balance as well as quality of life in older community-dwelling women with osteoporosis. Gerontology. (2002) 48:360–8. doi: 10.1159/000065504
21. Muehlbauer T, Gollhofer A, Granacher U. Associations between measures of balance and lower-extremity muscle strength/power in healthy individuals across the lifespan: a systematic review and meta-analysis. Sports Med Auckl NZ. (2015) 45:1671–92. doi: 10.1007/s40279-015-0390-z
22. Bermúdez Rey MC, Clark TK, Wang W, Leeder T, Bian Y, Merfeld DM. Vestibular perceptual thresholds increase above the age of 40. Front Neurol. (2016) 7:162. doi: 10.3389/fneur.2016.00162
23. Agrawal Y, Carey JP, Della Santina CC, Schubert MC, Minor LB. Disorders of balance and vestibular function in US adults: data from the National Health and Nutrition Examination Survey, 2001-2004. Arch Intern Med. (2009) 169:938. doi: 10.1001/archinternmed.2009.66
24. Lin HW, Bhattacharyya N. Balance disorders in the elderly: epidemiology and functional impact. Laryngoscope. (2012) 122:1858–61. doi: 10.1002/lary.23376
25. Hall CD, Heusel-Gillig L, Tusa RJ, Herdman SJ. Efficacy of gaze stability exercises in older adults with dizziness. J Neurol Phys Ther. (2010) 34:64–9. doi: 10.1097/NPT.0b013e3181dde6d8
26. Scherer M, Migliaccio AA, Schubert MC. Effect of vestibular rehabilitation on passive dynamic visual acuity. J Vestib Res. (2008) 18:147–57.
27. Schubert MC, Della Santina CC, Shelhamer M. Incremental angular vestibulo-ocular reflex adaptation to active head rotation. Exp Brain Res. (2008) 191:435–46. doi: 10.1007/s00221-008-1537-z
28. Herdman SJ, Schubert MC, Das VE, Tusa RJ. Recovery of dynamic visual acuity in unilateral vestibular hypofunction. Arch Otolaryngol Neck Surg. (2003) 129:819. doi: 10.1001/archotol.129.8.819
29. Herdman SJ, Hall CD, Schubert MC, Das VE, Tusa RJ. Recovery of dynamic visual acuity in bilateral vestibular hypofunction. Arch Otolaryngol Head Neck Surg. (2007) 133:383–9. doi: 10.1001/archotol.133.4.383
30. Hillier SL, Hollohan V. Vestibular rehabilitation for unilateral peripheral vestibular dysfunction. Cochrane Database Syst Rev. (2007) 4:CD005397. doi: 10.1002/14651858.CD005397.pub2
31. McDonnell MN, Hillier SL. Vestibular rehabilitation for unilateral peripheral vestibular dysfunction. Cochrane Database Syst Rev. (2015) 1:CD005397. doi: 10.1002/14651858.CD005397.pub4
32. Hillier SL, McDonnell M. Vestibular rehabilitation for unilateral peripheral vestibular dysfunction. Cochrane Database Syst Rev. (2011) 2:CD005397. doi: 10.1002/14651858.CD005397.pub3
33. Pothula VB, Chew F, Lesser THJ, Sharma AK. Falls and vestibular impairment. Clin Otolaryngol Allied Sci. (2004) 29:179–82. doi: 10.1111/j.0307-7772.2004.00785.x
34. Baloh RW, Enrietto J, Jacobson KM, Lin A. Age-related changes in vestibular function: a longitudinal study. Ann N Y Acad Sci. (2001) 942:210–9. doi: 10.1111/j.1749-6632.2001.tb03747.x
35. Peterka R, Black FO. Age-related changes in human vestibulo-ocular reflexes: sinusoidal rotation and caloric tests. J Vestib Res. (1990) 1:49–59.
36. Peterka R, Black FO. Age-related changes in human posture control: sensory organization tests. J Vestib Res. (1990) 1:73–85.
37. Peterka RJ. Sensorimotor integration in human postural control. J Neurophysiol. (2002) 88:1097–118. doi: 10.1152/jn.2002.88.3.1097
38. Horak FB. Postural compensation for vestibular loss. Ann N Y Acad Sci. (2009) 1164:76–81. doi: 10.1111/j.1749-6632.2008.03708.x
39. Agrawal Y, Merfeld DM, Horak FB, Redfern MS, Manor B, Westlake KP, et al. Aging, vestibular function, and balance: proceedings of a National Institute on Aging/National Institute on Deafness and Other Communication Disorders Workshop. J Gerontol Ser A. (2020) 75:2471–80. doi: 10.1093/gerona/glaa097
40. Shepard NT, Jacobson GP. The caloric irrigation test. In: Furman J, Lempert T, editors. Handbook of Clinical Neurology (Amsterdam: Elsevier). p. 119–31.
41. Jacobson GP, McCaslin DL, Piker EG, Gruenwald J, Grantham S, Tegel L. Insensitivity of the “Romberg test of standing balance on firm and compliant support surfaces” to the results of caloric and VEMP tests. Ear Hear. (2011) 32:e1–5. doi: 10.1097/AUD.0b013e31822802bb
42. Whitney SL, Marchetti GF, Schade AI. The relationship between falls history and computerized dynamic posturography in persons with balance and vestibular disorders. Arch Phys Med Rehabil. (2006) 87:402–7. doi: 10.1016/j.apmr.2005.11.002
43. Furman JM. Rotational testing. In: Furman J, Lempert T, editors. Handbook of Clinical Neurology (Amsterdam: Elsevier). p. 177–86.
44. Baloh RW, Ying SH, Jacobson KM. A longitudinal study of gait and balance dysfunction in normal older people. Arch Neurol. (2003) 60:835–9. doi: 10.1001/archneur.60.6.835
45. Kerber KA, Ishiyama GP, Baloh RW. A longitudinal study of oculomotor function in normal older people. Neurobiol Aging. (2006) 27:1346–53. doi: 10.1016/j.neurobiolaging.2005.07.009
46. Kerber KA, Enrietto JA, Jacobson KM, Baloh RW. Disequilibrium in older people: a prospective study. Neurology. (1998) 51:574–80. doi: 10.1212/WNL.51.2.574
47. Grossman GE, Leigh RJ, Bruce EN, Huebner WP, Lanska DJ. Performance of the human vestibuloocular reflex during locomotion. J Neurophysiol. (1989) 62:264–72. doi: 10.1152/jn.1989.62.1.264
48. Carriot J, Jamali M, Chacron MJ, Cullen KE. Statistics of the vestibular input experienced during natural self-motion: implications for neural processing. J Neurosci. (2014) 34:8347–57. doi: 10.1523/JNEUROSCI.0692-14.2014
49. Halmagyi GM, Curthoys IS. A clinical sign of canal paresis. Arch Neurol. (1988) 45:737–9. doi: 10.1001/archneur.1988.00520310043015
50. McCaslin DL, Jacobson GP, Bennett ML, Gruenwald JM, Green AP. Predictive properties of the video head impulse test: measures of caloric symmetry and self-report dizziness handicap. Ear Hear. (2014) 35:e185–e91. doi: 10.1097/AUD.0000000000000047
51. Grossman GE, Leigh RJ, Abel LA, Lanska DJ, Thurston SE. Frequency and velocity of rotational head perturbations during locomotion. Exp Brain Res. (1988) 70:470–6. doi: 10.1007/BF00247595
52. MacDougall HG, Weber KP, McGarvie LA, Halmagyi GM, Curthoys IS. The video head impulse test: diagnostic accuracy in peripheral vestibulopathy. Neurology. (2009) 73:1134–41. doi: 10.1212/WNL.0b013e3181bacf85
53. Yip CW, Glaser M, Frenzel C, Bayer O, Strupp M. Comparison of the bedside head-impulse test with the video head-impulse test in a clinical practice setting: a prospective study of 500 outpatients. Front Neurol. (2016) 7:58. doi: 10.3389/fneur.2016.00058
54. Lee J-Y, Kwon E, Kim H-J, Choi J-Y, Oh HJ, Koo J-W, et al. Dissociated results between caloric and video head impulse tests in dizziness: prevalence, pattern, lesion location, and etiology. J Clin Neurol. (2020) 16:277. doi: 10.3988/jcn.2020.16.2.277
55. Newman-Toker DE, Curthoys IS, Halmagyi GM. Diagnosing stroke in acute vertigo: the HINTS family of eye movement tests and the future of the “Eye ECG.” Semin Neurol. (2015) 35:506–21. doi: 10.1055/s-0035-1564298
56. Mantokoudis G, Saber Tehrani AS, Wozniak A, Eibenberger K, Kattah JC, Guede CI, et al. VOR gain by head impulse video-oculography differentiates acute vestibular neuritis from stroke. Otol Neurotol. (2015) 36:457–65. doi: 10.1097/MAO.0000000000000638
57. Newman-Toker DE, Saber Tehrani AS, Mantokoudis G, Pula JH, Guede CI, Kerber KA, et al. Quantitative video-oculography to help diagnose stroke in acute vertigo and dizziness: toward an ECG for the eyes. Stroke. (2013) 44:1158–61. doi: 10.1161/STROKEAHA.111.000033
58. Anson E, Pineault K, Bair W, Studenski S, Agrawal Y. Reduced vestibular function is associated with longer, slower steps in healthy adults during normal speed walking. Gait Posture. (2019) 68:340–5. doi: 10.1016/j.gaitpost.2018.12.016
59. Xie Y, Anson ER, Simonsick EM, Studenski SA, Agrawal Y. Compensatory saccades are associated with physical performance in older adults: data from the baltimore longitudinal study of aging. Otol Neurotol. (2017) 38:373–8. doi: 10.1097/MAO.0000000000001301
61. Agrawal Y, Davalos-Bichara M, Zuniga MG, Carey JP. Head impulse test abnormalities and influence on gait speed and falls in older individuals. Otol Neurotol. (2013) 34:1729–35. doi: 10.1097/MAO.0b013e318295313c
62. Davalos-Bichara M, Agrawal Y. Normative results of healthy older adults on standard clinical vestibular tests. Otol Neurotol. (2014) 35:297–300. doi: 10.1097/MAO.0b013e3182a09ca8
63. Herdman SJ, Tusa RJ, Blatt P, Suzuki A, Venuto PJ, Roberts D. Computerized dynamic visual acuity test in the assessment of vestibular deficits. Am J Otol. (1998) 19:790–6.
64. Herdman SJ, Schubert MC, Tusa RJ. Role of central preprogramming in dynamic visual acuity with vestibular loss. Arch Otolaryngol Neck Surg. (2001) 127:1205. doi: 10.1001/archotol.127.10.1205
65. Verbecque E, Van Criekinge T, Vanloot D, Coeckelbergh T, Van de Heyning P, Hallemans A, et al. Dynamic Visual Acuity test while walking or running on treadmill: reliability and normative data. Gait Posture. (2018) 65:137–42. doi: 10.1016/j.gaitpost.2018.07.166
66. Lord SR, Lloyd DG, Li SK. Sensori-motor function, gait patterns and falls in community-dwelling women. Age Ageing. (1996) 25:292–9. doi: 10.1093/ageing/25.4.292
67. Lord S, Castell S. Effect of exercise on balance, strength and reaction time in older people. Aust J Physiother. (1994) 40:83–8. doi: 10.1016/S0004-9514(14)60454-2
68. Lord SR, Ward JA. Age-associated differences in sensori-motor function and balance in community dwelling women. Age Ageing. (1994) 23:452–60. doi: 10.1093/ageing/23.6.452
69. Lord S, Sturnieks D. The physiology of falling: assessment and prevention strategies for older people. J Sci Med Sport. (2005) 8:35–42. doi: 10.1016/S1440-2440(05)80022-2
70. Goebel JA, Tungsiripat N, Sinks B, Carmody J. Gaze stabilization test: a new clinical test of unilateral vestibular dysfunction. Otol Neurotol Off Publ Am Otol Soc Am Neurotol Soc Eur Acad Otol Neurotol. (2007) 28:68–73. doi: 10.1097/01.mao.0000244351.42201.a7
71. Ward BK, Mohammed MT, Brach JS, Studenski SA, Whitney SL, Furman JM. Physical performance and a test of gaze stabilization in older adults. Otol Neurotol. (2010) 31:168–72. doi: 10.1097/MAO.0b013e3181c4c3e2
72. Honaker JA, Lee C, Shepard NT. Clinical use of the gaze stabilization test for screening falling risk in community-dwelling older adults. Otol Neurotol Off Publ Am Otol Soc Am Neurotol Soc Eur Acad Otol Neurotol. (2013) 34:729–35. doi: 10.1097/MAO.0b013e31827d8a5f
73. Whitney SL, Marchetti GF, Pritcher M, Furman JM. Gaze stabilization and gait performance in vestibular dysfunction. Gait Posture. (2009) 29:194–8. doi: 10.1016/j.gaitpost.2008.08.002
74. Hunter JB, Patel NS, O'Connell BP, Carlson ML, Shepard NT, McCaslin DL, et al. Cervical and ocular VEMP testing in diagnosing superior semicircular canal dehiscence. Otolaryngol Neck Surg. (2017) 156:917–23. doi: 10.1177/0194599817690720
75. Colebatch JG, Rosengren SM, Welgampola MS. Vestibular-evoked myogenic potentials. In: Furman J, Lempert T, editors. Handbook of Clinical Neurology (Amsterdam: Elsevier). p. 133–55.
76. McCaslin DL, Jacobson GP, Grantham SL, Piker EG, Verghese S. The influence of unilateral saccular impairment on functional balance performance and self-report dizziness. J Am Acad Audiol. (2011) 22:542–9; quiz 560–1. doi: 10.3766/jaaa.22.8.6
77. Layman AJ, Li C, Simonsick E, Ferrucci L, Carey JP, Agrawal Y. Association between saccular function and gait speed: data from the baltimore longitudinal study of aging. Otol Neurotol. (2015) 36:260–6. doi: 10.1097/MAO.0000000000000544
78. Xie YJ, Liu EY, Anson ER, Agrawal Y. Age-Related imbalance is associated with slower walking speed: an analysis from the National Health and Nutrition Examination Survey. J Geriatr Phys Ther. (2017) 40:183–9. doi: 10.1519/JPT.0000000000000093
79. Rudisill HE, Hain TC. Lower extremity myogenic potentials evoked by acoustic stimuli in healthy adults. Otol Neurotol Off Publ Am Otol Soc Am Neurotol Soc Eur Acad Otol Neurotol. (2008) 29:688–92. doi: 10.1097/MAO.0b013e3181730377
80. Cherchi M, Bellinaso NP, Card K, Covington A, Krumpe A, Pfeifer MS, et al. Sound evoked triceps myogenic potentials. Otol Neurotol. (2009) 30:545–50. doi: 10.1097/MAO.0b013e31819d89eb
81. Brooke RE, Herbert NC, Thyer NJ. Repeatability of sound-evoked triceps myogenic potentials. Int J Audiol. (2014) 53:880–6. doi: 10.3109/14992027.2014.938780
82. Kim J, Curthoys IS. Responses of primary vestibular neurons to galvanic vestibular stimulation (GVS) in the anaesthetised guinea pig. Brain Res Bull. (2004) 64:265–71. doi: 10.1016/j.brainresbull.2004.07.008
83. Goldberg JM, Smith CE, Fernandez C. Relation between discharge regularity and responses to externally applied galvanic currents in vestibular nerve afferents of the squirrel monkey. J Neurophysiol. (1984) 51:1236–56. doi: 10.1152/jn.1984.51.6.1236
84. Curthoys IS, MacDougall HG. What galvanic vestibular stimulation actually activates. Front Neurol. (2012) 3:117. doi: 10.3389/fneur.2012.00117
85. Welgampola MS, Ramsay E, Gleeson MJ, Day BL. Asymmetry of balance responses to monaural galvanic vestibular stimulation in subjects with vestibular schwannoma. Clin Neurophysiol. (2013) 124:1835–9. doi: 10.1016/j.clinph.2013.03.015
86. Tax CMW, Bom AP, Taylor RL, Todd N, Cho K-KJ, Fitzpatrick RC, et al. The galvanic whole-body sway response in health and disease. Clin Neurophysiol. (2013) 124:2036–45. doi: 10.1016/j.clinph.2012.12.041
87. Cauquil AS, Bousquet P, Salon M-CC, Dupui P, Bessou P. Monaural and binaural galvanic vestibular stimulation in human dynamic balance function. Gait Posture. (1997) 6:210–7. doi: 10.1016/S0966-6362(97)00011-8
88. Watson SR, Brizuela AE, Curthoys IS, Colebatch JG, MacDougall HG, Halmagyi GM. Maintained ocular torsion produced by bilateral and unilateral galvanic (DC) vestibular stimulation in humans. Exp Brain Res. (1998) 122:453–8. doi: 10.1007/s002210050533
89. MacDougall HG, Brizuela AE, Burgess AM, Curthoys IS, Halmagyi GM. Patient and normal three-dimensional eye-movement responses to maintained (DC) surface galvanic vestibular stimulation. Otol Neurotol. (2005) 26:500–11. doi: 10.1097/01.mao.0000169766.08421.ef
90. MacDougall HG, Brizuela AE, Burgess AM, Curthoys IS. Between-subject variability and within-subject reliability of the human eye-movement response to bilateral galvanic (DC) vestibular stimulation. Exp Brain Res. (2002) 144:69–78. doi: 10.1007/s00221-002-1038-4
91. Day BL, Séverac Cauquil A, Bartolomei L, Pastor MA, Lyon IN. Human body-segment tilts induced by galvanic stimulation: a vestibularly driven balance protection mechanism. J Physiol. (1997) 500:661–72. doi: 10.1113/jphysiol.1997.sp022051
92. Fitzpatrick RC, Marsden J, Lord SR, Day BL. Galvanic vestibular stimulation evokes sensations of body rotation. NeuroReport. (2002) 13:2379–83. doi: 10.1097/00001756-200212200-00001
93. Peters RM, Blouin J-S, Dalton BH, Inglis JT. Older adults demonstrate superior vestibular perception for virtual rotations. Exp Gerontol. (2016) 82:50–7. doi: 10.1016/j.exger.2016.05.014
94. Fitzpatrick RC, Day BL. Probing the human vestibular system with galvanic stimulation. J Appl Physiol. (2004) 96:2301–16. doi: 10.1152/japplphysiol.00008.2004
95. Watson SRD, Colebatch JG. Vestibular-evoked electromyographic responses in soleus: a comparison between click and galvanic stimulation. Exp Brain Res. (1998) 119:504–10. doi: 10.1007/s002210050366
96. Britton TC, Day BL, Brown P, Rothwell JC, Thompson PD, Marsden CD. Postural electromyographic responses in the arm and leg following galvanic vestibular stimulation in man. Exp Brain Res. (1993) 94:143–51. doi: 10.1007/BF00230477
97. Dlugaiczyk J, Gensberger KD, Straka H. Galvanic vestibular stimulation: from basic concepts to clinical applications. J Neurophysiol. (2019) 121:2237–55. doi: 10.1152/jn.00035.2019
98. Curthoys IS. A critical review of the neurophysiological evidence underlying clinical vestibular testing using sound, vibration and galvanic stimuli. Clin Neurophysiol. (2010) 121:132–44. doi: 10.1016/j.clinph.2009.09.027
99. Welgampola M, Colebatch J. Selective effects of ageing on vestibular-dependent lower limb responses following galvanic stimulation. Clin Neurophysiol. (2002) 113:528–34. doi: 10.1016/S1388-2457(02)00020-2
100. Dalton BH, Blouin J-S, Allen MD, Rice CL, Inglis JT. The altered vestibular-evoked myogenic and whole-body postural responses in old men during standing. Exp Gerontol. (2014) 60:120–8. doi: 10.1016/j.exger.2014.09.020
101. Deshpande N, Patla AE. Postural responses and spatial orientation to neck proprioceptive and vestibular inputs during locomotion in young and older adults. Exp Brain Res. (2005) 167:468–74. doi: 10.1007/s00221-005-0182-z
102. Fregly AR, Graybiel A. Labyrinthine defects as shown by ataxia and caloric tests. Acta Otolaryngol (Stockh). (1970) 69:216–22. doi: 10.3109/00016487009123356
103. Longridge NS, Mallinson AI. Clinical romberg testing does not detect vestibular disease. Otol Neurotol. (2010) 31:803–6. doi: 10.1097/MAO.0b013e3181e3deb2
104. Nashner LM. A model describing vestibular detection of body sway motion. Acta Otolaryngol (Stockh). (1971) 72:429–36. doi: 10.3109/00016487109122504
105. Nashner LM, Black FO, Wall C. Adaptation to altered support and visual conditions during stance: patients with vestibular deficits. J Neurosci Off J Soc Neurosci. (1982) 2:536–44. doi: 10.1523/JNEUROSCI.02-05-00536.1982
106. Nashner LM, Peters JF. Dynamic posturography in the diagnosis and management of dizziness and balance disorders. Neurol Clin. (1990) 8:331–49. doi: 10.1016/S0733-8619(18)30359-1
107. Horak FB, Nashner LM, Diener HC. Postural strategies associated with somatosensory and vestibular loss. Exp Brain Res. (1990) 82:167–77. doi: 10.1007/BF00230848
108. Mishra A, Davis S, Speers R, Shepard NT. Head shake computerized dynamic posturography in peripheral vestibular lesions. Am J Audiol. (2009) 18:53–9. doi: 10.1044/1059-0889(2009/06-0024)
109. Honaker JA, Janky KL, Patterson JN, Shepard NT. Modified head shake sensory organization test: Sensitivity and specificity. Gait Posture. (2016) 49:67–72. doi: 10.1016/j.gaitpost.2016.06.024
110. Broglio SP, Sosnoff JJ, Rosengren KS, McShane K. A comparison of balance performance: computerized dynamic posturography and a random motion platform. Arch Phys Med Rehabil. (2009) 90:145–50. doi: 10.1016/j.apmr.2008.06.025
111. Cass SP, Kartush JM, Graham MD. Clinical assessment of postural stability following vestibular nerve section. Laryngoscope. (1991) 101:1056–9. doi: 10.1288/00005537-199110000-00005
112. Cass SP, Kartush JM, Graham MD. Patterns of vestibular function following vestibular nerve section. Laryngoscope. (1992) 102:388–94. doi: 10.1288/00005537-199204000-00004
113. Black FO, Shupert CL, Peterka RJ, Nashner LM. Effects of unilateral loss of vestibular function on the vestibulo-ocular reflex and postural control. Ann Otol Rhinol Laryngol. (1989) 98:884–9. doi: 10.1177/000348948909801109
114. Fetter M, Diener HC, Dichgans J. Recovery of postural control after an acute unilateral vestibular lesion in humans. J Vestib Res Equilib Orientat. (1990) 1:373–83.
115. Barin K, Seitz CM, Welling DB. Effect of head orientation on the diagnostic sensitivity of posturography in patients with compensated unilateral lesions. Otolaryngol Head Neck Surg Off J Am Acad Otolaryngol-Head Neck Surg. (1992) 106:355–62. doi: 10.1177/019459989210600407
116. Forbes PA, Chen A, Blouin J-S. Sensorimotor control of standing balance. Handb Clin Neurol. (2018) 159:61–83. doi: 10.1016/B978-0-444-63916-5.00004-5
117. Johari K, den Ouden D-B, Behroozmand R. Effects of aging on temporal predictive mechanisms of speech and hand motor reaction time. Aging Clin Exp Res. (2018) 30:1195–202. doi: 10.1007/s40520-018-0902-4
118. Ko S-U, Simonsick E, Deshpande N, Ferrucci L. Sex-specific age associations of ankle proprioception test performance in older adults: results from the Baltimore Longitudinal Study of Aging. Age Ageing. (2015) 44:485–90. doi: 10.1093/ageing/afv005
119. Hamid MA, Hughes GB, Kinney SE. Specificity and sensitivity of dynamic posturography. A retrospective analysis. Acta Oto-Laryngol Suppl. (1991) 481:596–600. doi: 10.3109/00016489109131480
120. Ford-Smith CD, Wyman JF, Elswick RK, Fernandez T, Newton RA. Test-retest reliability of the sensory organization test in noninstitutionalized older adults. Arch Phys Med Rehabil. (1995) 76:77–81. doi: 10.1016/S0003-9993(95)80047-6
121. Voorhees RL. Dynamic posturography findings in central nervous system disorders. Otolaryngol Neck Surg. (1990) 103:96–101. doi: 10.1177/019459989010300114
122. Whipple R, Wolfson L, Derby C, Singh D, Tobin J. Altered sensory function and balance in older persons. J Gerontol. (1993) 48:71–6. doi: 10.1093/geronj/48.Special_Issue.71
123. Woollacott MH, Shumway-Cook A, Nashner LM. Aging and posture control: changes in sensory organization and muscular coordination. Int J Aging Hum Dev. (1986) 23:97–114. doi: 10.2190/VXN3-N3RT-54JB-X16X
124. Ledin T, Kronhed AC, Möller C, Möller M, Odkvist LM, Olsson B. Effects of balance training in elderly evaluated by clinical tests and dynamic posturography. J Vestib Res Equilib Orientat. (1990) 1:129–38.
125. Peterka RJ. Postural control model interpretation of stabilogram diffusion analysis. Biol Cybern. (2000) 82:335–43. doi: 10.1007/s004220050587
126. Wolfson L, Whipple R, Derby CA, Amerman P, Murphy T, Tobin JN, et al. A dynamic posturography study of balance in healthy elderly. Neurology. (1992) 42:2069–75. doi: 10.1212/WNL.42.11.2069
127. Pedalini MEB, Cruz OLM, Bittar RSM, Lorenzi MC, Grasel SS. Sensory organization test in elderly patients with and without vestibular dysfunction. Acta Otolaryngol (Stockh). (2009) 129:962–5. doi: 10.1080/00016480802468930
128. Horak FB, Shupert CL, Mirka A. Components of postural dyscontrol in the elderly: a review. Neurobiol Aging. (1989) 10:727–38. doi: 10.1016/0197-4580(89)90010-9
129. Di Fabio RP. Sensitivity and specificity of platform posturography for identifying patients with vestibular dysfunction. Phys Ther. (1995) 75:290–305. doi: 10.1093/ptj/75.4.290
130. Park MK, Lim H-W, Cho JG, Choi C-J, Hwang SJ, Chae SW. A head shake sensory organization test to improve the sensitivity of the sensory organization test in the elderly. Otol Neurotol Off Publ Am Otol Soc Am Neurotol Soc Eur Acad Otol Neurotol. (2012) 33:67–71. doi: 10.1097/MAO.0b013e318238f75f
131. Honaker JA, Boismier TE, Shepard NP, Shepard NT. Fukuda stepping test: sensitivity and specificity. J Am Acad Audiol. (2009) 20:311–4; quiz 335. doi: 10.3766/jaaa.20.5.4
132. Shumway-Cook A, Horak FB. Assessing the influence of sensory interaction of balance. Suggestion from the field. Phys Ther. (1986) 66:1548–50. doi: 10.1093/ptj/66.10.1548
133. Cohen H, Blatchly CA, Gombash LL. A study of the clinical test of sensory interaction and balance. Phys Ther. (1993) 73:346–51. doi: 10.1093/ptj/73.6.346
134. Cohen HS, Mulavara AP, Stitz J, Sangi-Haghpeykar H, Williams SP, Peters BT, et al. Screening for vestibular disorders using the modified clinical test of sensory interaction and balance and tandem walking with eyes closed. Otol Neurotol Off Publ Am Otol Soc Am Neurotol Soc Eur Acad Otol Neurotol. (2019) 40:658–65. doi: 10.1097/MAO.0000000000002173
135. Park MK, Kim K-M, Jung J, Lee N, Hwang SJ, Chae SW. Evaluation of uncompensated unilateral vestibulopathy using the modified clinical test for sensory interaction and balance. Otol Neurotol Off Publ Am Otol Soc Am Neurotol Soc Eur Acad Otol Neurotol. (2013) 34:292–6. doi: 10.1097/MAO.0b013e31827c9dae
136. Hong SK, Park JH, Kwon SY, Kim J-S, Koo J-W. Clinical efficacy of the Romberg test using a foam pad to identify balance problems: a comparative study with the sensory organization test. Eur Arch Otorhinolaryngol. (2015) 272:2741–7. doi: 10.1007/s00405-014-3273-2
137. Edginton Bigelow K, Berme N. Development of a protocol for improving the clinical utility of posturography as a fall-risk screening tool. J Gerontol A Biol Sci Med Sci. (2011) 66A:228–33. doi: 10.1093/gerona/glq202
138. Baloh RW, Jacobson KM, Enrietto JA, Corona S, Honrubia V. Balance disorders in older persons: quantification with posturography. Otolaryngol Neck Surg. (1998) 119:89–92. doi: 10.1016/S0194-5998(98)70177-9
139. Gilfriche P, Deschodt-Arsac V, Blons E, Arsac LM. Frequency-specific fractal analysis of postural control accounts for control strategies. Front Physiol. (2018) 9:293. doi: 10.3389/fphys.2018.00293
140. Hausdorff JM, Mitchell SL, Firtion R, Peng CK, Cudkowicz ME, Wei JY, et al. Altered fractal dynamics of gait: reduced stride-interval correlations with aging and Huntington's disease. J Appl Physiol Bethesda Md (1985). (1997) 82:262–9. doi: 10.1152/jappl.1997.82.1.262
141. Fernandez C, Goldberg JM. Physiology of peripheral neurons innervating semicircular canals of the squirrel monkey. II. Response to sinusoidal stimulation and dynamics of peripheral vestibular system. J Neurophysiol. (1971) 34:661–75. doi: 10.1152/jn.1971.34.4.661
142. Yeh J-R, Hsu L-C, Lin C, Chang F-L, Lo M-T. Nonlinear analysis of sensory organization test for subjects with unilateral vestibular dysfunction. PLoS One. (2014) 9:e91230. doi: 10.1371/journal.pone.0091230
143. Cenciarini M, Peterka RJ. Stimulus-dependent changes in the vestibular contribution to human postural control. J Neurophysiol. (2006) 95:2733–50. doi: 10.1152/jn.00856.2004
144. Peterka RJ, Statler KD, Wrisley DM, Horak FB. Postural compensation for unilateral vestibular loss. Front Neurol. (2011) 2:57. doi: 10.3389/fneur.2011.00057
145. Mantokoudis G, Schubert MC, Saber Tehrani AS, Wong AL, Agrawal Y. Early adaptation and compensation of clinical vestibular responses after unilateral vestibular deafferentation surgery. Otol Neurotol. (2014) 35:148–54. doi: 10.1097/MAO.0b013e3182956196
146. Cenciarini M, Loughlin PJ, Sparto PJ, Redfern MS. Medial-lateral postural control in older adults exhibits increased stiffness and damping. In: 2009 Annual International Conference of the IEEE Engineering in Medicine and Biology Society (Minneapolis, MN: IEEE). p. 7006–7009.
147. Wiesmeier IK, Dalin D, Maurer C. Elderly use proprioception rather than visual and vestibular cues for postural motor control. Front Aging Neurosci. (2015) 7:97. doi: 10.3389/fnagi.2015.00097
148. Fukuda T. The stepping test: two phases of the labyrinthine reflex. Acta Otolaryngol (Stockh). (1959) 50:95–108. doi: 10.3109/00016485909129172
149. Honaker JA, Shepard NT. Performance of fukuda stepping test as a function of the severity of caloric weakness in chronic dizzy patients. J Am Acad Audiol. (2012) 23:616–22. doi: 10.3766/jaaa.23.8.6
150. Shumway-Cook A, Gruber W, Baldwin M, Liao S. The effect of multidimensional exercises on balance, mobility, and fall risk in community-dwelling older adults. Phys Ther. (1997) 77:46–57. doi: 10.1093/ptj/77.1.46
151. Wrisley DM, Kumar NA. Functional gait assessment: concurrent, discriminative, and predictive validity in community-dwelling older adults. Phys Ther. (2010) 90:761–73. doi: 10.2522/ptj.20090069
152. Lusardi MM, Fritz S, Middleton A, Allison L, Wingood M, Phillips E, et al. Determining risk of falls in community dwelling older adults: a systematic review and meta-analysis using posttest probability. J Geriatr Phys Ther. (2017) 40:1–36. doi: 10.1519/JPT.0000000000000099
153. Whitney SL, Hudak MT, Marchetti GF. The dynamic gait index relates to self-reported fall history in individuals with vestibular dysfunction. J Vestib Res Equilib Orientat. (2000) 10:99–105.
154. Whitney SL, Marchetti GF, Schade A, Wrisley DM. The sensitivity and specificity of the Timed “Up & Go” and the Dynamic Gait Index for self-reported falls in persons with vestibular disorders. J Vestib Res Equilib Orientat. (2004) 14:397–409.
155. Beninato M, Fernandes A, Plummer LS. Minimal clinically important difference of the functional gait assessment in older adults. Phys Ther. (2014) 94:1594–603. doi: 10.2522/ptj.20130596
156. Gill-Body KM, Beninato M, Krebs DE. Relationship among balance impairments, functional performance, and disability in people with peripheral vestibular hypofunction. Phys Ther. (2000) 80:748–58. doi: 10.1093/ptj/80.8.748
157. Wrisley DM, Walker ML, Echternach JL, Strasnick B. Reliability of the dynamic gait index in people with vestibular disorders. Arch Phys Med Rehabil. (2003) 84:1528–33. doi: 10.1016/S0003-9993(03)00274-0
158. Chang T-P, Schubert MC. Association of the Video head impulse test with improvement of dynamic balance and fall risk in patients with dizziness. JAMA Otolaryngol Neck Surg. (2018) 144:696. doi: 10.1001/jamaoto.2018.0650
159. Panzer VP, Wakefield DB, Hall CB, Wolfson LI. Mobility assessment: sensitivity and specificity of measurement sets in older adults. Arch Phys Med Rehabil. (2011) 92:905–12. doi: 10.1016/j.apmr.2011.01.004
160. Pisciottano MVC, Pinto SS, Szejnfeld VL, Castro CHM. The relationship between lean mass, muscle strength and physical ability in independent healthy elderly women from the community. J Nutr Health Aging. (2014) 18:554–8. doi: 10.1007/s12603-013-0414-z
161. Dakin CJ, Rosenberg A. Gravity estimation and verticality perception. In: Day BL, Lord SR, editors. Handbook of Clinical Neurology (Amsterdam: Elsevier). p. 43–59.
162. Tobis JS, Reinsch S, Swanson JM, Byrd M, Scharf T. Visual perception dominance of fallers among community-dwelling older adults. J Am Geriatr Soc. (1985) 33:330–3. doi: 10.1111/j.1532-5415.1985.tb07132.x
163. Lord SR, Webster IW. Visual field dependence in elderly fallers and non-fallers. Int J Aging Hum Dev. (1990) 31:267–77. doi: 10.2190/38MH-2EF1-E36Q-75T2
164. Barr C, McLoughlin JV, van den Berg MEL, Sturnieks DL, Crotty M, Lord SR. Visual field dependence is associated with reduced postural sway, dizziness and falls in older people attending a falls clinic. J Nutr Health Aging. (2016) 20:671–5. doi: 10.1007/s12603-015-0681-y
165. Bisdorff AR, Wolsley CJ, Anastasopoulos D, Bronstein AM, Gresty MA. The perception of body verticality (subjective postural vertical) in peripheral and central vestibular disorders. Brain J Neurol. (1996) 119 (Pt 5):1523–34. doi: 10.1093/brain/119.5.1523
166. Böhmer A, Mast F. Assessing otolith function by the subjective visual vertical. Ann N Y Acad Sci. (1999) 871:221–31. doi: 10.1111/j.1749-6632.1999.tb09187.x
167. Böhmer A. The subjective visual vertical as a clinical parameter for acute and chronic vestibular (otolith) disorders. Acta Otolaryngol (Stockh). (1999) 119:126–7. doi: 10.1080/00016489950181495
168. Yardley L. Contribution of somatosensory information to perception of the visual vertical with body tilt and rotating visual field. Percept Psychophys. (1990) 48:131–4. doi: 10.3758/BF03207079
169. Mittelstaedt H. Somatic graviception. Biol Psychol. (1996) 42:53–74. doi: 10.1016/0301-0511(95)05146-5
170. Barbieri G, Gissot A-S, Pérennou D. Ageing of the postural vertical. AGE. (2010) 32:51–60. doi: 10.1007/s11357-009-9112-5
171. Manckoundia P, Mourey F, Pfitzenmeyer P, Hoecke JV, Pérennou D. Is backward disequilibrium in the elderly caused by an abnormal perception of verticality? A pilot study. Clin Neurophysiol. (2007) 118:786–93. doi: 10.1016/j.clinph.2006.11.274
172. Scheets PL, Sahrmann SA, Norton BJ, Stith JS, Crowner BE. What is backward disequilibrium and how do i treat it? A complex patient case study. J Neurol Phys Ther JNPT. (2015) 39:119–26. doi: 10.1097/NPT.0000000000000084
173. Menant JC, St George RJ, Fitzpatrick RC, Lord SR. Perception of the postural vertical and falls in older people. Gerontology. (2012) 58:497–503. doi: 10.1159/000339295
174. Anastasopoulos D, Haslwanter T, Bronstein A, Fetter M, Dichgans J. Dissociation between the perception of body verticality and the visual vertical in acute peripheral vestibular disorder in humans. Neurosci Lett. (1997) 233:151–3. doi: 10.1016/S0304-3940(97)00639-3
175. Ito Y, Gresty MA. Shift of subjective reference and visual orientation during slow pitch tilt for the seated human subject. Brain Res Bull. (1996) 40:417–21. doi: 10.1016/0361-9230(96)00136-0
176. Anastasopoulos D, Bronstein A, Haslwanter T, Fetter M, Dichgans J. The role of somatosensory input for the perception of verticality. Ann N Y Acad Sci. (1999) 871:379–83. doi: 10.1111/j.1749-6632.1999.tb09199.x
177. Mazibrada G, Tariq S, Pérennou D, Gresty M, Greenwood R, Bronstein AM. The peripheral nervous system and the perception of verticality. Gait Posture. (2008) 27:202–8. doi: 10.1016/j.gaitpost.2007.03.006
178. Cremer R, Zeef EJ. What kind of noise increases with age? J Gerontol. (1987) 42:515–8. doi: 10.1093/geronj/42.5.515
179. Faisal AA, Selen LPJ, Wolpert DM. Noise in the nervous system. Nat Rev Neurosci. (2008) 9:292–303. doi: 10.1038/nrn2258
180. White O, Babič J, Trenado C, Johannsen L, Goswami N. The promise of stochastic resonance in falls prevention. Front Physiol. (2019) 9:1865. doi: 10.3389/fphys.2018.01865
181. Welford AT. THE MEASUREMENT OF SENSORY-MOTOR PERFORMANCE : SURVEY AND REAPPRAISAL OF TWELVE YEARS' PROGRESS. Ergonomics. (1960) 3:189–230. doi: 10.1080/00140136008930484
182. Voytek B, Kramer MA, Case J, Lepage KQ, Tempesta ZR, Knight RT, et al. Age-related changes in 1/f neural electrophysiological noise. J Neurosci. (2015) 35:13257–65. doi: 10.1523/JNEUROSCI.2332-14.2015
183. Roditi RE, Crane BT. Directional asymmetries and age effects in human self-motion perception. J Assoc Res Otolaryngol JARO. (2012) 13:381–401. doi: 10.1007/s10162-012-0318-3
184. Crane BT. Perception of combined translation and rotation in the horizontal plane in humans. J Neurophysiol. (2016) 116:1275–85. doi: 10.1152/jn.00322.2016
185. Green DM, Swets JA. Signal Detection Theory and Psychophysics. Oxford, England: John Wiley (1966).
186. Merfeld DM. Signal detection theory and vestibular thresholds: I. Basic theory and practical considerations. Exp Brain Res. (2011) 210:389–405. doi: 10.1007/s00221-011-2557-7
187. Lim K, Merfeld DM. Signal detection theory and vestibular perception: II. Fitting perceptual thresholds as a function of frequency. Exp Brain Res. (2012) 222:303–20. doi: 10.1007/s00221-012-3217-2
188. Chaudhuri SE, Merfeld DM. Signal detection theory and vestibular perception: III. Estimating unbiased fit parameters for psychometric functions. Exp Brain Res. (2013) 225:133–46. doi: 10.1007/s00221-012-3354-7
189. Macmillan NA, Creelman CD. Detection Theory: A User's Guide, 2nd edn. Mahwah, NJ: Lawrence Erlbaum Associates (2005).
190. Karmali F, Bermúdez Rey MC, Clark TK, Wang W, Merfeld DM. Multivariate analyses of balance test performance, vestibular thresholds, and age. Front Neurol. (2017) 8:578. doi: 10.3389/fneur.2017.00578
191. Angelaki DE, McHenry MQ, Dickman JD, Newlands SD, Hess BJ. Computation of inertial motion: neural strategies to resolve ambiguous otolith information. J Neurosci Off J Soc Neurosci. (1999) 19:316–327. doi: 10.1523/JNEUROSCI.19-01-00316.1999
192. Laurens J, Meng H, Angelaki DE. Neural representation of orientation relative to gravity in the Macaque Cerebellum. Neuron. (2013) 80:1508–18. doi: 10.1016/j.neuron.2013.09.029
193. Merfeld DM, Park S, Gianna-Poulin C, Black FO, Wood S. Vestibular perception and action employ qualitatively different mechanisms. I. Frequency Response of VOR and Perceptual Responses During Translation and Tilt. J Neurophysiol. (2005) 94:186–98. doi: 10.1152/jn.00904.2004
194. Merfeld DM, Park S, Gianna-Poulin C, Black FO, Wood S. Vestibular perception and action employ qualitatively different mechanisms. II. VOR and Perceptual responses during combined Tilt&Translation. J Neurophysiol. (2005) 94:199–205. doi: 10.1152/jn.00905.2004
195. Angelaki DE, Yakusheva TA. How vestibular neurons solve the tilt/translation ambiguity: comparison of brainstem, cerebellum, and thalamus. Ann N Y Acad Sci. (2009) 1164:19–28. doi: 10.1111/j.1749-6632.2009.03939.x
196. Angelaki DE, Shaikh AG, Green AM, Dickman JD. Neurons compute internal models of the physical laws of motion. Nature. (2004) 430:560–4. doi: 10.1038/nature02754
197. Green AM, Angelaki DE. An integrative neural network for detecting inertial motion and head orientation. J Neurophysiol. (2004) 92:905–25. doi: 10.1152/jn.01234.2003
198. Carriot J, Jamali M, Brooks JX, Cullen KE. Integration of canal and otolith inputs by central vestibular neurons is subadditive for both active and passive self-motion: implication for perception. J Neurosci. (2015) 35:3555–65. doi: 10.1523/JNEUROSCI.3540-14.2015
199. Karmali F, Whitman GT, Lewis RF. Bayesian optimal adaptation explains age-related human sensorimotor changes. J Neurophysiol. (2018) 119:509–20. doi: 10.1152/jn.00710.2017
200. Maurer C, Peterka RJ. A new interpretation of spontaneous sway measures based on a simple model of human postural control. J Neurophysiol. (2005) 93:189–200. doi: 10.1152/jn.00221.2004
201. Dai M, Klein A, Cohen B, Raphan T. Model-based study of the human cupular time constant. J Vestib Res Equilib Orientat. (1999) 9:293–301.
202. Goldberg JM, Fernandez C. Physiology of peripheral neurons innervating semicircular canals of the squirrel monkey. I. Resting discharge and response to constant angular accelerations. J Neurophysiol. (1971) 34:635–60. doi: 10.1152/jn.1971.34.4.635
203. Baloh RobertW, Demer JosephL. Optokinetic-vestibular interaction in patients with increased gain of the vestibulo-ocular reflex. Exp Brain Res. (1993) 97: doi: 10.1007/BF00228703
204. Raphan T. Vestibular, locomotor, and vestibulo-autonomic research: 50 years of collaboration with Bernard Cohen. J Neurophysiol. (2020) 123:329–45. doi: 10.1152/jn.00485.2019
205. Merfeld DM, Young LR, Oman CM, Shelhamer MJ. A multidimensional model of the effect of gravity on the spatial orientation of the monkey. J Vestib Res Equilib Orientat. (1993) 3:141–61.
206. Robinson DA. Vestibular and optokinetic symbiosis: an example of explaining by modelling. In: Control Gaze Brainstem Interneurons. (1977). Available online at: https://ci.nii.ac.jp/naid/10008955613/en/
207. Jenkins HA, Honrubia V, Baloh RH. Evaluation of multiple-frequency rotatory testing in patients with peripheral labyrinthine weakness. Am J Otolaryngol. (1982) 3:182–8. doi: 10.1016/S0196-0709(82)80052-5
208. King S, Priesol AJ, Davidi SE, Merfeld DM, Ehtemam F, Lewis RF. Self-motion perception is sensitized in vestibular migraine: pathophysiologic and clinical implications. Sci Rep. (2019) 9:14323. doi: 10.1038/s41598-019-50803-y
209. Dimitri PS, Wall C, Oas JG, Rauch SD. Application of multivariate statistics to vestibular testing: discriminating between Menière's disease and migraine associated dizziness. J Vestib Res Equilib Orientat. (2001) 11:53–65.
210. Berniker M, Kording K. Bayesian approaches to sensory integration for motor control: Bayesian approaches to sensory integration for motor control. Wiley Interdiscip Rev Cogn Sci. (2011) 2:419–28. doi: 10.1002/wcs.125
211. Laurens J, Droulez J. Bayesian processing of vestibular information. Biol Cybern. (2007) 96:389–404. doi: 10.1007/s00422-006-0133-1
212. Karmali F. The velocity storage time constant: balancing between accuracy and precision. In: John Leigh R, Ramat S, Shaikh A, editors. Progress in Brain Research (Amsterdam: Elsevier). p. 269–76.
213. Raphan T, Matsuo V, Cohen B. Velocity storage in the vestibulo-ocular reflex arc (VOR). Exp Brain Res. (1979) 35:229–48. doi: 10.1007/BF00236613
214. Rauch SD, Velazquez-Villaseñor L, Dimitri PS, Merchant SN. Decreasing hair cell counts in aging humans. Ann N Y Acad Sci. (2006) 942:220–7. doi: 10.1111/j.1749-6632.2001.tb03748.x
215. Jacobson GP, McCaslin DL, Patel S, Barin K, Ramadan NM. Functional and anatomical correlates of impaired velocity storage. J Am Acad Audiol. (2004) 15:324–33. doi: 10.3766/jaaa.15.4.6
216. Anson E, Bigelow RT, Studenski S, Deshpande N, Agrawal Y. Failure on the foam eyes closed test of standing balance associated with reduced semicircular canal function in healthy older adults. Ear Hear. (2019) 40:340–4. doi: 10.1097/AUD.0000000000000619
217. Della Santina CC, Cremer PD, Carey JP, Minor LB. Comparison of head thrust test with head autorotation test reveals that the vestibulo-ocular reflex is enhanced during voluntary head movements. Arch Otolaryngol Neck Surg. (2002) 128:1044. doi: 10.1001/archotol.128.9.1044
218. Dichgans J, Bizzi E, Morasso P, Tagliasco V. Mechanisms underlying recovery of eye-head coordination following bilateral labyrinthectomy in monkeys. Exp Brain Res. (1973) 18:548–62. doi: 10.1007/BF00234137
219. Schubert MC, Hall CD, Das V, Tusa RJ, Herdman SJ. Oculomotor strategies and their effect on reducing gaze position error. Otol Neurotol. (2010) 31:228–31. doi: 10.1097/MAO.0b013e3181c2dbae
220. Schubert MC, Migliaccio AA, Clendaniel RA, Allak A, Carey JP. Mechanism of dynamic visual acuity recovery with vestibular rehabilitation. Arch Phys Med Rehabil. (2008) 89:500–7. doi: 10.1016/j.apmr.2007.11.010
221. Sadeghi SG, Minor LB, Cullen KE. Neural correlates of motor learning in the vestibulo-ocular reflex: dynamic regulation of multimodal integration in the macaque vestibular system. J Neurosci Off J Soc Neurosci. (2010) 30:10158–68. doi: 10.1523/JNEUROSCI.1368-10.2010
222. Sadeghi SG, Minor LB, Cullen KE. Multimodal integration after unilateral labyrinthine lesion: single vestibular nuclei neuron responses and implications for postural compensation. J Neurophysiol. (2011) 105:661–73. doi: 10.1152/jn.00788.2010
223. Sadeghi SG, Minor LB, Cullen KE. Neural correlates of sensory substitution in vestibular pathways following complete vestibular loss. J Neurosci Off J Soc Neurosci. (2012) 32:14685–95. doi: 10.1523/JNEUROSCI.2493-12.2012
224. Schubert MC, Das V, Tusa RJ, Herdman SJ. Cervico-ocular reflex in normal subjects and patients with unilateral vestibular hypofunction. Otol Neurotol Off Publ Am Otol Soc Am Neurotol Soc Eur Acad Otol Neurotol. (2004) 25:65–71. doi: 10.1097/00129492-200401000-00013
225. Cullen KE. “Physiology of central pathways,” In: Furman J, Lempert T, editors. Handbook of Clinical Neurology (Amsterdam: Elsevier). p. 17–40. doi: 10.1016/B978-0-444-63437-5.00002-9
226. Halmagyi GM, Curthoys IS, Cremer PD, Henderson CJ, Todd MJ, Staples MJ, et al. The human horizontal vestibulo-ocular reflex in response to high-acceleration stimulation before and after unilateral vestibular neurectomy. Exp Brain Res. (1990) 81:479–90. doi: 10.1007/BF02423496
227. Halmagyi GM, Chen L, MacDougall HG, Weber KP, McGarvie LA, Curthoys IS. The video head impulse test. Front Neurol. (2017) 8:258. doi: 10.3389/fneur.2017.00258
228. Wolfe JM, Kluender KR, Dennis LM, Bartoshuk LM, Herz RS, Lederman SJ, et al. Sensation & Perception, 4th edn. Sunderland, MA: Sinauer Associates, Inc., Publishers (2015).
229. Angelaki DE, Cullen KE. Vestibular System: The Many Facets of a Multimodal Sense. Annu Rev Neurosci. (2008) 31:125–50. doi: 10.1146/annurev.neuro.31.060407.125555
230. Asai M, Watanabe Y, Ohashi N, Mizukoshi K. Evaluation of vestibular function by dynamic posturography and other equilibrium examinations. Acta Oto-Laryngol Suppl. (1993) 504:120–4. doi: 10.3109/00016489309128136
231. Cohen HS, Kimball KT. Usefulness of some current balance tests for identifying individuals with disequilibrium due to vestibular impairments. J Vestib Res Equilib Orientat. (2008) 18:295–303.
232. Zhou J, Habtemariam D, Iloputaife I, Lipsitz LA, Manor B. The complexity of standing postural sway associates with future falls in community-dwelling older adults: the MOBILIZE Boston Study. Sci Rep. (2017) 7:2924. doi: 10.1038/s41598-017-03422-4
233. Peterka RJ. Sensory integration for human balance control. In: Day BL, Lord SR, editors. Handbook of Clinical Neurology (Amsterdam: Elsevier). p. 27–42.
234. Fitzpatrick R, Burke D, Gandevia SC. Task-dependent reflex responses and movement illusions evoked by galvanic vestibular stimulation in standing humans. J Physiol. (1994) 478:363–72. doi: 10.1113/jphysiol.1994.sp020257
235. Welgampola M, Colebatch J. Vestibulospinal reflexes: quantitative effects of sensory feedback and postural task. Exp Brain Res. (2001) 139:345–53. doi: 10.1007/s002210100754
236. Day BL. Vestibular-evoked postural responses in the absence of somatosensory information. Brain. (2002) 125:2081–8. doi: 10.1093/brain/awf212
237. Horak FB, Hlavacka F. Somatosensory loss increases vestibulospinal sensitivity. J Neurophysiol. (2001) 86:575–85. doi: 10.1152/jn.2001.86.2.575
238. Klein R, Klein BEK, Lee KE, Cruickshanks KJ, Gangnon RE. Changes in visual acuity in a population over a 15-year period: the Beaver Dam Eye Study. Am J Ophthalmol. (2006) 142:539–49.e2. doi: 10.1016/j.ajo.2006.06.015
239. Hong T, Mitchell P, Rochtchina E, Fong CS, Chia E-M, Wang JJ. Long-term changes in visual acuity in an older population over a 15-year period. Ophthalmology. (2013) 120:2091–9. doi: 10.1016/j.ophtha.2013.03.032
240. Cullen KE. Vestibular processing during natural self-motion: implications for perception and action. Nat Rev Neurosci. (2019) 20:346–63. doi: 10.1038/s41583-019-0153-1
241. Lim K, Karmali F, Nicoucar K, Merfeld DM. Perceptual precision of passive body tilt is consistent with statistically optimal cue integration. J Neurophysiol. (2017) 117:2037–52. doi: 10.1152/jn.00073.2016
Keywords: vestibular, balance, aging, perceptual threshold, falls
Citation: Wagner AR, Akinsola O, Chaudhari AMW, Bigelow KE and Merfeld DM (2021) Measuring Vestibular Contributions to Age-Related Balance Impairment: A Review. Front. Neurol. 12:635305. doi: 10.3389/fneur.2021.635305
Received: 30 November 2020; Accepted: 18 January 2021;
Published: 09 February 2021.
Edited by:
Michael Strupp, Ludwig Maximilian University of Munich, GermanyReviewed by:
Klaus Jahn, Schoen Clinic Bad Aibling—Department of Neurology, GermanyChristoph Helmchen, Luebeck University of Applied Sciences, Germany
Alexander A. Tarnutzer, University of Zurich, Switzerland
Copyright © 2021 Wagner, Akinsola, Chaudhari, Bigelow and Merfeld. This is an open-access article distributed under the terms of the Creative Commons Attribution License (CC BY). The use, distribution or reproduction in other forums is permitted, provided the original author(s) and the copyright owner(s) are credited and that the original publication in this journal is cited, in accordance with accepted academic practice. No use, distribution or reproduction is permitted which does not comply with these terms.
*Correspondence: Andrew R. Wagner, d2FnbmVyLjEzODZAb3N1LmVkdQ==; Daniel M. Merfeld, bWVyZmVsZC42QG9zdS5lZHU=