- 1Department of Neurosurgery, Johns Hopkins University School of Medicine, Baltimore, MD, United States
- 2Department of Neurosurgery, Johns Hopkins Medicine, Institute for Brain Protection Sciences, Johns Hopkins All Children’s Hospital, St. Petersburg, FL, United States
- 3Department of Surgery, St. Jude Children’s Research Hospital, Memphis, TN, United States
- 4Le Bonheur Neuroscience Institute, Le Bonheur Children’s Hospital, Memphis, TN, United States
- 5Department of Neurosurgery, University of Tennessee Health Science Center, Memphis, TN, United States
Intramedullary astrocytomas represent approximately 30%–40% of all intramedullary tumors and are the most common intramedullary tumor in children. Surgical resection is considered the mainstay of treatment in symptomatic patients with neurological deficits. Gross total resection (GTR) can be difficult to achieve as astrocytomas frequently present as diffuse lesions that infiltrate the cord. Therefore, GTR carries a substantial risk of new post-operative deficits. Consequently, subtotal resection and biopsy are often the only surgical options attempted. A midline or paramedian sulcal myelotomy is frequently used for surgical resection, although a dorsal root entry zone myelotomy can be used for lateral tumors. Intra-operative neuromonitoring using D-wave integrity, somatosensory, and motor evoked potentials is critical to facilitating a safe resection. Adjuvant radiation and chemotherapy, such as temozolomide, are often administered for high-grade recurrent or progressive lesions; however, consensus is lacking on their efficacy. Biopsied tumors can be analyzed for molecular markers that inform clinicians about the tumor’s prognosis and response to conventional as well as targeted therapeutic treatments. Stratification of intramedullary tumors is increasingly based on molecular features and mutational status. The landscape of genetic and epigenetic mutations in intramedullary astrocytomas is not equivalent to their intracranial counterparts, with important difference in frequency and type of mutations. Therefore, dedicated attention is needed to cohorts of patients with intramedullary tumors. Targeted therapeutic agents can be designed and administered to patients based on their mutational status, which may be used in coordination with traditional surgical resection to improve overall survival and functional status.
Introduction
Astrocytomas of the spinal cord are rare tumors accounting for nearly 30%–40% of all intramedullary spinal cord tumors (1, 2). They constitute the most common type of intramedullary tumor in children, representing 40%–60% of intramedullary spinal cord neoplasms (1, 3, 4). Intramedullary astrocytomas are frequently classified according to the World Health Organization (WHO) categorization system using a 1 through 4 grading system. Traditionally, these grades corresponded to their histological features, and included pilocytic astrocytomas as Grade 1, diffuse or fibrillary tumors as Grade 2, anaplastic astrocytomas as Grade 3, and glioblastoma (GBM) as Grade 4 (5, 6). More recently, updated guidelines incorporate genetic markers into the grading system, in addition to traditional histological features (7). Low-grade tumors (Grades 1 and 2) account for most intramedullary astrocytomas, whereas high-grade lesions (Grades 3 and 4) represent about 25% of cases and confer a poor prognosis (5). Most pediatric tumors are low-grade pilocytic astrocytomas, whereas high-grade lesions occur more frequently in adults (1, 8–10).
Astrocytomas of the spinal cord tend to present eccentric to the central canal and frequently present with poor margins (5, 11). Infiltrative tumors tend to be higher grade, whereas low-grade tumors tend to be more circumscribed, particularly pilocytic astrocytomas (12). Novel techniques in image analysis and radiomics are increasingly being used to classify the pathological diagnosis prior to resection. As one example, a lateralization index has been developed to consider the distance between the center of the cord and the lateral edge of the tumor, with astrocytomas displaying greater dispersion than ependymomas (13). Machine learning techniques can be applied to classify the tumor’s malignant status or automatically segment the spinal cord to quantify the tumor morphology (14, 15). Indeed, the tumor molecular landscape can affect its appearance on imaging in ways that are perceptible only by machine learning and high-throughput radiomic algorithms. This new field of radiogenomics uses quantitative imaging techniques to relate imaging features with the genotype of the tumor, allowing prediction of prognosis, stratification of patients, and determination of therapy (16).
Intramedullary tumors can present with nonspecific symptoms, such as axial back pain and radiculopathy, complicating diagnosis (10). Growth of the neoplasm results in progressive deformation of the spinal cord, contributing to numbness and sensory deficits, weakness and motor deficits, and bowel and bladder dysfunction (17, 18). Astrocytomas typically present hypo-or isointense on T1-weighted and hyperintense on T2-weighted magnetic resonance imaging (MRI) and can include syrinx formation (18) (Figure 1).
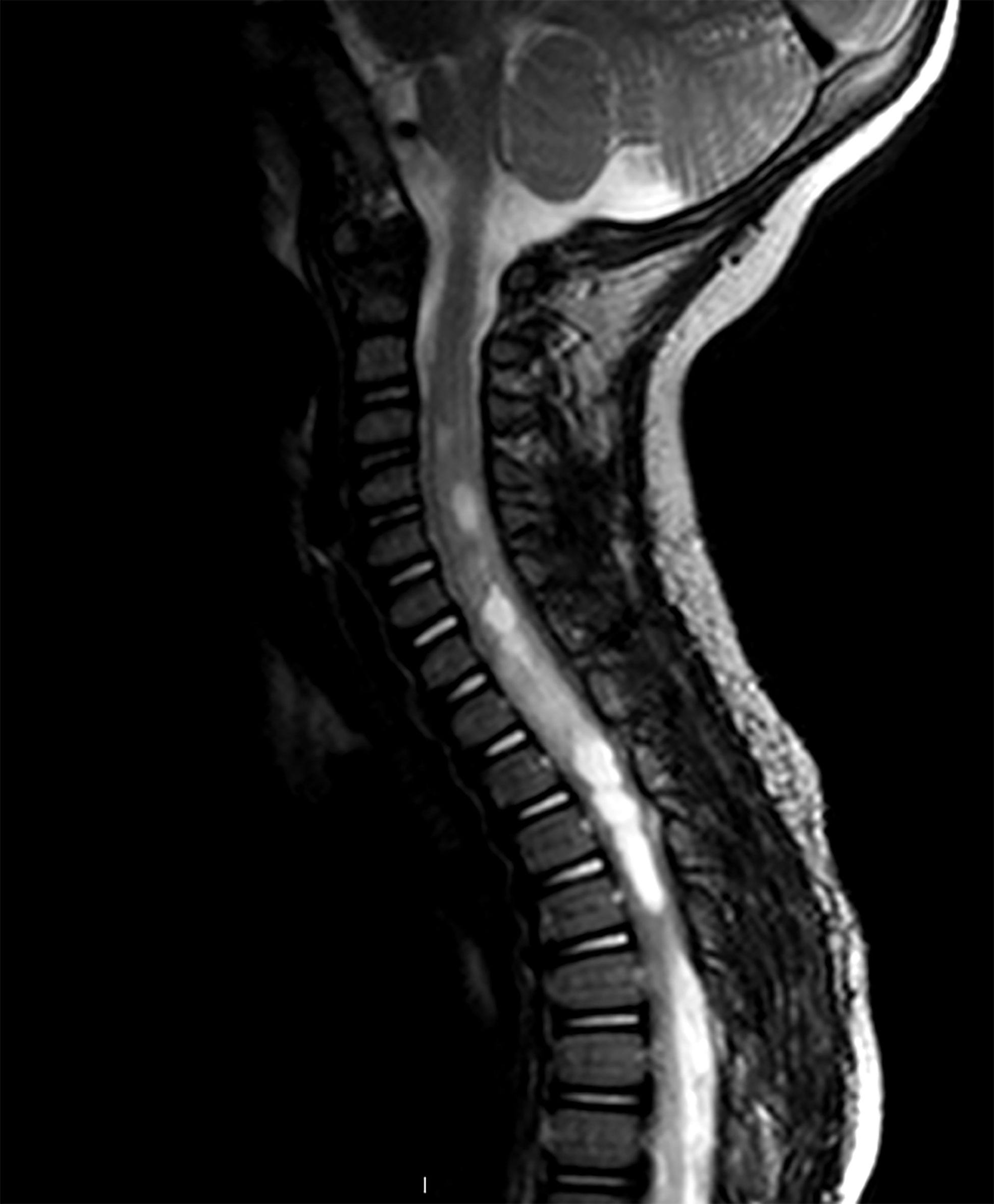
Figure 1 Sagittal T2-weighted MRI of pediatric patient with an intramedullary mass extending from C4-T3. Increased T2 signal is seen both cranially and caudally to the tumor. The lesion appears enhancing, although several areas of central non-enhancement consistent with necrosis are visible. Pathology was consistent with a Grade 2 astrocytoma.
Survival for patients with intramedullary astrocytomas is poorer compared to ependymomas and hemangioblastomas, with reported overall rates ranging from 40%–65% over 15 years (17). These poorer outcomes reflect the infiltrative nature of astrocytomas, resulting in low rates of gross total resection (GTR) or near total resection. In addition, their poorly delineated margins can result in increased post-operative morbidity that contributes to reduced survival (18). The WHO grade is an important prognostic factor, as high-grade astrocytomas can progress aggressively in short timespans, often conferring mortality within a few months (3, 19–21). Older age has also been identified as a risk factor of increased mortality (3). Higher-grade astrocytomas are also less likely to respond to adjuvant therapy. Nonetheless, surgical treatment with adjuvant therapy is often advocated for intramedullary astrocytomas. However, this view does not account for genetic phenotypes that influence survival or response to therapy, and new insights into the molecular markers of astrocytomas may challenge traditional approaches (22). Although there is an overlap in the genetic profile of intracranial and intramedullary astrocytomas, distinct differences have been noted that these can influence prognosis and treatment, and intramedullary astrocytomas should therefore be analyzed separately from their intracranial counterpart (23).
Management
Surgery
Surgical intervention is considered the mainstay of treatment in patients with intramedullary astrocytomas experiencing neurological deficits or myelopathy, with a less clear role for patients with pain or incidental findings. Although GTR is believed to improve local tumor control, it often cannot be achieved without risking significant post-operative morbidity to patients, given the infiltrative nature of astrocytomas and lack of clear margins (24–26). Consequently, surgeons must balance the potential improvement in survival from more extensive resections with the increased risk of worsening and/or new neurological deficits (27). Reported rates of GTR are lower compared to intramedullary ependymomas, which often present with distinct cleavage planes (17, 28, 29). Patients with low-grade astrocytomas, particularly pilocytic astrocytomas, may present with dissection planes that facilitate gross total resection; however, GTR can be difficult to achieve in high-grade astrocytomas (17, 28, 30). Using intra-operative findings and neuromonitoring, and considering the patient’s pre-operative baseline deficits, surgeons must determine the maximal safe resection feasible. Tumors lacking clear planes or presenting with imaging findings suggestive of a high-grade lesion should be considered for near total or subtotal resection (STR) with biopsy (Figure 2) (31). Patients with significant comorbidities, advanced age, or paraplegia should also be considered for STR when the benefits of GTR are expected to be minimal.
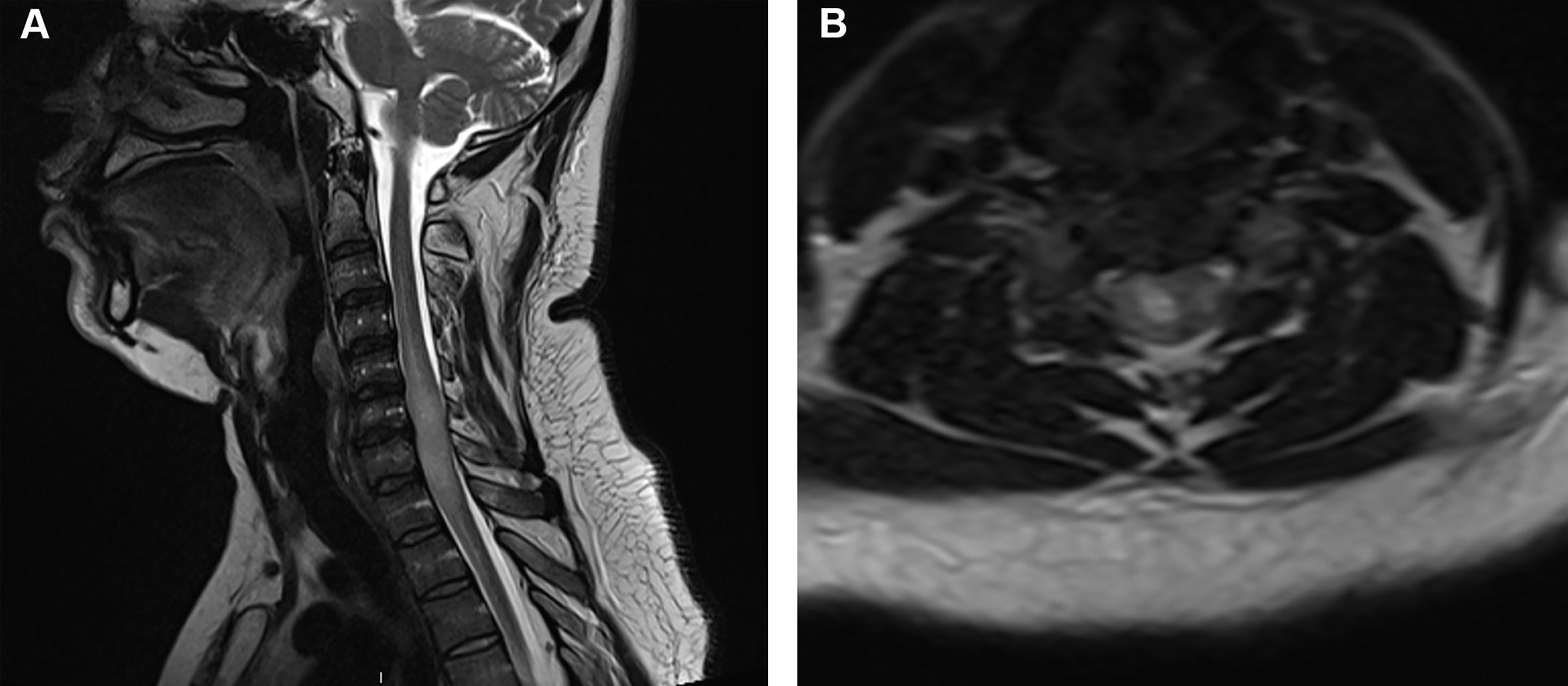
Figure 2 Adult patient with an intramedullary astrocytoma seen on (A) sagittal T2-weighted MRI and (B) axial T2-weighed MRI extending from the C4 to T3 vertebral levels with effacement of the subarachnoid cerebrospinal fluid. The patient presented with lower limb paralysis and loss of bladder and bowel function. Intra-operatively, a subtotal resection was performed with removal of visible tumor; however, the tumor was noted to be infiltrative. Pathology revealed an H3K27M-altered diffuse midline glioma resulting in a WHO Grade 4 diagnosis, although the histological appearance resembled anaplastic astrocytoma.
Patients are usually positioned in the prone position and a posterior midline approach used for subperiosteal dissection and removal of the bony elements relevant for the dural exposure. Mean arterial pressure should be preserved above 80–90 mmHg to maintain spinal cord perfusion (for pediatric patients, we advocate maintaining the pre-operative MAP values to slightly above it). The dura is opened in the midline and can be tacked to surrounding muscle tissue using dural tacking sutures (32, 33). Intra-operative ultrasound can be used to visualize the lesion and confirm location prior to myelotomy, minimizing cord manipulation. Intramedullary tumors generally appear hyperechoic on ultrasound compared to healthy spinal cord, and infiltrative astrocytomas may have blurry margins (34).
Thereafter, an operating microscope should be introduced to the field to minimize damage to cord tissue and identify dissection planes. A midline myelotomy between the dorsal columns is usually performed. The dorsal medial vein can be identified to help identify the midline. Other relative safe entry zones include the dorsal intermediate or dorsolateral sulci, also known as the dorsal root safe entry zone. For lesions positioned laterally, the midline myelotomy can be used, but alternative routes include the dorsal root entry zone or an anterior to dorsal root entry zone myelotomy can be performed (32, 35). Some advocate that the pia mater be tacked to the dura to maintain the operative field, although we prefer using dynamic retraction to minimize stress and tension on the spinal cord (36, 37).
Once the tumor is reached, fine dissection is made along the borders and disconnection of the tumoral blood supply is performed when feasible. In many cases, the main technique will include debulking of the tumor inner core followed by disconnecting and dissecting the tumor from the healthy spinal cord. This is carried out interchangeably, which again allows for dynamic retraction and tension on the spinal cord. An ultrasonic aspirator can be used to remove the tumor (38). Highly infiltrative astrocytomas should be considered for biopsy only, while tumors appearing low grade or those with a relatively clear plane can be attempted for complete removal (39). After hemostasis is achieved, a watertight dural closure can be performed, followed by closure of the superficial layers (39). Again, intra-operative ultrasound can be used to confirm the resection extent. An expansile duraplasty is sometimes performed after biopsy or debulking to provide increased space to reduce the risk of cerebrospinal fluid leak and alleviate clinical deficits resulting from tumor growth (36, 40, 41).
Intra-operative neuromonitoring should be performed to facilitate maximal safe resection. Studies have suggested improved functional outcomes for patients with intra-operative monitoring compared to those without monitoring (37, 42). Institutional studies have reported using somatosensory evoked potentials, motor evoked potentials, and transcranial motor evoked potential monitoring (17, 39). Loss of evoked potentials without recovery is often considered an endpoint for resection due to its ability to predict new post-operative deficits, although protocols differ (17, 32, 43). A systematic review suggested that motor evoked potentials have a sensitivity of 84% and sensitivity of 83% for detecting injury (44). Neuromonitoring with somatosensory and motor evoked potentials can involve a slight delay of several seconds from the time of insult to recognition. D-wave epidural or intradural monitoring is used by some surgeons due to its faster, real-time feedback, with a decrease in amplitude ≥50% often used as an endpoint (39, 43). D-waves are not influenced by blood pressure, heart rate, and anesthetic drugs, unlike motor and somatosensory evoked potentials. A review of 28 patients with intramedullary tumors illustrated that D-wave specificity, compared to motor and somatosensory evoked potentials, was most consistent over time, with a specificity nearly twice as large as motor evoked potentials (45). However, D-waves cannot always be obtained, particularly in patients with pre-existing deficits and patients with tumors located near the conus medullaris due to the limited tracts available (17, 43).
Both laminectomy and laminoplasty have been described for intramedullary surgery. Some studies have suggested that laminoplasty can reduce the likelihood of post-operative spinal deformity, particularly in children at risk of kyphotic deformity (46). Ahmed et al. noted that laminoplasty can help promote wound healing, reduce the risk of a CSF leak, and facilitate a safer extradural dissection plane in patients undergoing repeat surgery (47). However, a separate study of 66 pediatric patients undergoing resection of intramedullary tumors did not observe laminoplasty to result in a significantly different rate in the incidence of instrumented fusion for spinal deformity in pediatric patients undergoing resection of intramedullary tumors compared to those receiving laminectomy (48). Some surgeons prefer laminoplasty in pediatric cohorts; however, additional studies are needed (17). A spinous process-splitting hemi-laminoplasty could also be considered, although reports are lacking in the literature for intramedullary tumors (49).
Consensus is lacking concerning the optimal surgical treatment strategy and the effect of resection extent on post-operative outcomes (41). Several studies have failed to observe a significant effect of extent of resection in patients with intramedullary astrocytomas, and some have shown surgery to worsen functional outcomes (6, 21, 50). In contrast, others have identified a role for extent of resection independent of underlying histology for improving post-operative survival (24, 51). A national database study of 348 patients by Luksik et al. determined that GTR or partial resection significantly improve survival in children with intramedullary astrocytomas compared to those receiving biopsy only or no surgery (3). A separate national database study by Adams et al. of 135 patients with primary malignant intramedullary astrocytomas also established extent of resection as a significant predictor of survival (51). Resection can improve local control, delaying tumor progression and improving survival (52). A single-institutional study of 94 patients found that GTR was achieved in 13% of patients and a near-total resection achieved in another 34%. Their 10-year survival rate was 55%, although patients who survived 10 years largely had WHO Grades 1 and 2 tumors, as higher-grade tumor patients had shorter overall survival (17). Higher tumor grades were also significantly associated with shorter time to tumor progression after surgery, and chemotherapy did not improve survival (24). Separately, a French multi-center study of 95 patients achieved a GTR rate of 38% with 10-year survival of 77% (53). A systematic review by Hamilton et al. suggested that GTR improves overall survival in patients with intramedullary astrocytomas irrespective of underlying grade (54). However, this may come at the expense of worsening neurological deficits (41). Similarly, Golpayegani et al. conducted a meta-analysis of 1,079 patients and found that GTR significantly improved survival compared to STR on Kaplan–Meier survival analysis (31). In contrast, some authors advocate a more cautious approach, noting that GTR should be attempted where feasible, particularly in low-grade lesions, but cautioning against its use in high-grade lesions (41, 55).
New advances in intra-operative neuronavigation and imaging have been described for intramedullary tumors that may help facilitate a maximal safe resection. In addition to intra-operative B-mode ultrasound for tumor visualization, contrast-enhanced ultrasound has been reported to improve lesion localization and myelotomy planning with good correlation to pre-operative MRI. The contrast consists of microbubbles that can illustrate the tumor vascular network, and outline the tumor to a greater extent than B-mode imaging. In addition, contrast administration after resection can help confirm the resection extent (56). Augmented reality operating microscopes have been reported to help visualize tumor outline and assist with resection using principles similar to intracranial augmented reality-based applications (57). Similarly, multimodal neuronavigation techniques are limited but have been described, including one that incorporates diffusion tensor imaging, three-dimensional virtual reality imaging, and the operating microscope to visualize tractography in real time (58). Although these technologies are associated with learning curves, we anticipate that continued refinement of these technologies will lead to increased uptake by surgeons and help improve intramedullary lesion resection. In addition, we expect that improvements in tractography and diffusion tensor imaging will allow for improved pre-operative surgical planning to minimize the risks of neurological deficits (59, 60).
Adjuvant therapy
Radiation therapy (RT) and chemotherapy are sometimes used in patients with high-grade lesions or patients with progressive disease following resection, with a less clear role following STR of low-grade lesions (61). Potential benefits in survival from RT must be balanced against the cord’s limited capacity to tolerate radiation and potential for injury (21). Additionally, RT in children can affect growth and result in radionecrosis and vasculopathy (62). The literature is conflicting on the therapeutic benefits of RT, and the optimal timing and dose of radiation are unclear (54). Some studies have not found any significant effect of RT on overall survival (6, 63). Other studies have suggested a deleterious effect on overall survival, although this may reflect selection bias as high-grade lesions with poor prognosis are more likely to receive RT (3, 24). A meta-analysis suggested that RT worsened outcomes for low-grade lesions but improved overall survival in high-grade astrocytomas (54). Similarly, MInehan et al. identified a survival benefit for infiltrative tumors but not pilocytic astrocytomas (64). RT is, therefore, often administered in patients with high-grade infiltrative lesions with a poor prognosis. Proton therapy has a sharp dose fall-off and can also be administered for spinal cord tumors, which may reduce damage to surrounding tissues and improve safety. However, like conventional RT, no clear benefit has been identified in the literature. Indelicato et al. found that intramedullary tumors treated with proton beam therapy had inferior local control compared to cerebellar and cerebral tumors, whereas Kahn et al. found on multivariable analysis that 10 patients with intramedullary tumors treated with proton beam therapy fared worse than those treated with photon beam RT (65, 66). The authors suggest that the sharp dose fall-off may limit effective treatment for highly infiltrative tumors (66).
Similarly, the efficacy of chemotherapy is unclear, with limited data supporting its use (67). It is usually reserved for high-grade lesions that recur or progress despite resection or RT, or for pediatric patients less than 3 years old in whom RT is contraindicated (17, 21, 24, 68). Some case series have reported promising results using chemotherapy for intramedullary astrocytomas, favoring its use for recurrent or unresectable lesions (21, 62, 69). However, a systematic review of nine studies of patients with spinal cord glioblastoma treated with temozolomide did not confer a significant survival benefit, although owing to the rarity of the disease, the sample size only included 19 patients (70). Chemotherapeutic regimens are heterogenous, although temozolomide is often administered based on the Stupp protocol employed for intracranial GBM (24, 71). Other agents include carboplatin, vincristine, vinblastine, and irinotecan (24).
Novel treatment strategies are necessary to improve outcomes in patients with spinal cord astrocytomas, particularly those with high-grade aggressive lesions not amenable to GTR. Outcomes remain poor in these patients, with average overall survival of only a few months (3, 17, 21). Furthermore, although the WHO grade is an important prognosticator, not all patients with the same histological grade will experience the same outcomes. Lesions of the same grade can differ in their degree of infiltration and prognosis, suggesting that important genetic and molecular differences exist that can inform the optimal treatment paradigm. Moreover, identification of genetic mutations and molecular biomarkers may represent an opportunity for personalized, targeted therapy, offering patients a new option for treatment beyond surgical resection.
Molecular landscape
The past decade has seen increasing interest in identifying the genomic landscape of intramedullary astrocytomas. Detection of target genes or biomarkers can improve prognostication and may represent novel targets for personalized or genetic therapy. Molecular markers were included in the WHO classification of central nervous system tumors for the first time in 2016, which defined new lesions and subcategorized tumors based on genetics. For example, the guidelines defined the entity known as diffuse midline glioma H3 K27M-mutant to refer to midline tumors primarily found in children with K27M mutations in the histone H3 gene H3F3A (72). Glioblastomas were also categorized based on IDH mutation status.
Most recently, the 2021 WHO classification substantially updated the classification of tumors with a greater emphasis on genetic, epigenetic, and molecular markers in addition to traditional histological grading. IDH-mutant astrocytomas are considered a distinct entity and categorized as Grade 2, 3, or 4, while CDKN2A/B deletions provide further subclassification to IDH-mutant astrocytomas (7). In some cases, the presence of a particular genetic mutation is sufficient to determine the tumor grade irrespective of the underlying histological appearance. Glioblastoma can be defined as an IDH wild-type, H3 wild-type tumor with TERT promoter mutation, EGFR amplification, +7/-10 chromosome copy-number alterations, microvascular proliferation, or necrosis, illustrating the aggressive nature of IDH wild-type tumors that were previously categorized only as Grade 2 or 3 based on histological appearance (73). In addition to recognition of genetic mutations, alterations of molecular pathways, such as the MAPK pathway, are also considered for tumor categorization (7).
Importantly, the increase in knowledge of astrocytoma genetics has mainly been focused on intracranial astrocytomas and may not necessarily translate to spinal cord astrocytomas. Like we see with the appearing anatomical distinction in ependymomas (stating supratentorial, infratentorial, and spinal cord ependymomas are different tumors), there is high likelihood that in the future, we will learn distinctive features between spinal cord astrocytomas and the intracranial ones.
Although the genetics of intracranial astrocytomas have been extensively studied, intramedullary astrocytomas have been shown to harbor a unique set of genetic mutations and molecular biomarkers (Table 1) (82–84). Therefore, chemotherapeutic and targeted therapies designed for intracranial astrocytomas may not translate successfully to spinal cord neoplasms, whose underlying genetic mutations inform their natural history and response to therapy (83). As an example, Bettegowda et al. have noted that the frequency of KIAA1549-BRAF fusions in spinal pilocytic astrocytomas is lower than the reported frequency in their intracranial counterpart (85). Moreover, Lebrun et al. illustrated that the breakpoints involved in the KIAA1549-BRAF fusion differed in their frequency between intracranial and intramedullary pilocytic astrocytomas (86). Consequently, a thorough understanding of the molecular landscape of intramedullary tumors is critical for improving treatment strategies and aiding surgical management.
H3K27M
Somatic missense mutations at amino acid position 27 of the H3F3A or HIST1H3B genes are commonly seen in astrocytomas of midline structures, including the pons, thalamus, and spinal cord, resulting in the discrete entity designated diffuse midline glioma, H3 K27M-altered (87). They are estimated to account approximately between 18% and 55% of intramedullary astrocytomas (86, 88, 89). Originally designed in the 2016 WHO guidelines as H3 K27M-mutant lesions, the updated nomenclature reflects other mechanisms can alter the pathway beyond genetic mutations (7). Most commonly, a missense mutation change a lysine to methionine residue (K27M) in the histone H3 variants H3.3 or H3.1, resulting in a change in genetic expression that is believed to drive tumor formation and growth (87, 90). These gliomas are also associated with a loss of trimethylation in residual H3K27 and an increase in H3K27 acetylation, thereby upregulating expression of proto-oncogenes and suppressing cellular differentiation (90, 91).
H3 K27M-altered diffuse midline gliomas are aggressive lesions most commonly appearing in children and young adults (Figure 2) (87, 92). Although they can appear in several midline structures, the spinal cord is the most common site of H3 K27M-altered gliomas in adults (93). They are designated WHO Grade 4, although their underlying microscopic appearance may appear Grade 2, 3, or 4 (88, 94). Indeed, the majority of H3 K27M-altered gliomas appear as high-grade lesions, but approximately 40% can appear as Grade 2 in histology (88, 95). Cheng et al. studied 59 patients with intramedullary astrocytomas, of which 28 were designated H3K27M-altered, and found that shorter symptom duration was significantly associated with the H3K27M mutation, but did not identify significant differences on MRI (88). Gu et al. reported five cases of intramedullary diffuse midline gliomas with the H3K27M-alteration and noted that all were initially mistaken as benign based on their radiographic appearance (93). Interestingly, the H3K27M-alteration has not been shown to present alongside IDH mutations, suggesting the two are mutually exclusive (96, 97).
H3 K27M-altered tumors portend a poor prognosis and significantly worsen overall survival for histological Grade 2 lesions, although histological Grade 2 lesions bearing the H3 K27M-alteration have improved survival compared to histological Grade 4 H3 K27M-altered gliomas (95). Several studies have shown that they do not significantly worsen survival in histologic Grade 3 or 4 lesions compared to high-grade wild-type astrocytomas, illustrating that histological grade is still an important consideration in evaluating these lesions, although statistical significance in those studies may have been limited by small sample sizes (88, 95). Adults with the H3K27M-altered gliomas may have improved prognosis compared to pediatric patients (93). Inhibition of the histone demethylase JMJD3 can increase H3K27 methylation and has shown encouraging results in in vitro and in vivo mice models of brainstem glioma, illustrating the importance of genetic analysis of astrocytomas to identify potential targeted therapeutics and improve patient outcomes (98). The investigational compounds imipridone ONC201 and IDO1 inhibitor indoximod are also being investigated for treatment of H3K27M-altered gliomas both intracranially and in the spinal cord (74, 99).
MGMT
The O6-methylguanine-DNA methyltransferase (MGMT) protein is a DNA repair enzyme that repairs damaged guanine nucleotides by transferring methyl groups from the guanine O6 site to cysteine residues, thereby providing resistance to alkylating agents such as temozolomide (TMZ) that methylate purine bases (100, 101). Epigenetic methylation of CpG islands of the MGMT promoter results in heterochromatinization and downregulation of the gene, increasing susceptibility of tumors to alkylating agents (75). Consequently, several clinical trials have illustrated that MGMT promoter methylation is associated with improved survival compared to unmethylated tumors and is an independent prognostic factor in high-grade astrocytoma (102, 103). Indeed, a meta-analysis of 34 studies by Binabaj et al. illustrated that MGMT methylation improves overall survival in GBM patients compared to unmethylated patients (104). Interestingly, MGMT promoter methylation is rarely observed in H3K27M-altered gliomas. Consequently, the increased preponderance of the H3K27M-alteration in intramedullary astrocytomas compared to intracranial astrocytomas may explain the former’s decreased responsiveness to temozolomide (105).
Stratification of intramedullary astrocytomas by MGMT methylation status can help assess prognosis and determine the value of adjuvant chemotherapy compared to resection and radiotherapy. Rovin and Winn described a 28-year-old woman with a high-grade intramedullary astrocytoma lacking expression of MGMT whose residual tumor regressed favorably after surgery, radiation, and temozolomide, illustrating the significance of MGMT methylation status (106). Sun et al. classified 47 spinal cord gliomas based on MGMT expression, administering TMZ alone to patients with negative expression but providing both TMZ and the anti-neoplastic agent cisplatin to patients with positive expression (100). Other targeted therapies are being studied, including MGMT inhibitors such as O6-benzylguanine and O6-(4-bromothenyl) guanine (75).
BRAF
Genetic changes in the BRAF gene are frequently found in pilocytic astrocytomas, a low-grade glioma common in the pediatric population (107, 108). BRAF encodes the B-raf protein, an intracellular serine/threonine kinase involved in the mitogen-activated protein kinase pathway (MAPK) regulating cellular growth, proliferation, differentiation, and tumorigenesis (107). Fusion between BRAF and KIAA1549 results in loss of the BRAF N-terminal auto-inhibitory domain, producing a fusion oncogene and constitutive activation of the MAPK pathway (109, 110). The BRAFV600E point mutation has also been described in pilocytic astrocytomas, as it disrupts the auto-inhibitory mechanism of B-raf, thereby promoting B-raf activation (109). BRAF fusions are associated with a favorable prognosis, in part related to B-raf’s role in promoting oncogene-induced senescence in tumors, as well as its frequent occurrence in pilocytic astrocytomas, which inherently have favorable outcomes (109, 111–113).
Although BRAF changes can occasionally be found in higher grade gliomas, they mostly occur in pilocytic astrocytomas. The KIAA1549-BRAF fusion is estimated in nearly 80% of cerebellar pilocytic astrocytomas, but only around 50% of non-cerebellar pilocytic astrocytomas, including those of the spinal cord (86). Chai et al. observed the BRAF V600E mutation in 12% of intramedullary astrocytomas, noting its occurrence only in H3K27M–wild-type neoplasms (95). They also noted significantly improved survival for histological Grade 2 or 3 astrocytomas with a BRAF V600E mutation compared to Grade 2 or 3 wild-type astrocytomas (95). Of note, the frequency of fusion breakpoint location differ between intracranial and intramedullary astrocytomas harboring a KIAA1549-BRAF fusion. Lebrun et al. noted that the KIAA1549 (15)-BRAF(9) fusion was most common in intramedullary pilocytic astrocytomas, identified in 80% of tumors with the fusion oncogene, while the KIAA1549(16)-BRAF(9) fusion was most common in intracranial pilocytic astrocytomas (86). The implications are not entirely elucidated, but nonetheless illustrate the distinct molecular landscape of intramedullary astrocytomas compared to their intracranial counterparts. BRAF-MEK inhibitors can potentially be used to improve outcomes in patients harboring BRAF fusions or mutations (84, 114). Balasubramanian et al. treated an adult patient with a BRAF V600E pilocytic astrocytoma with vemurafenib, a B-raf inhibitor, and cobimetinib, a MEK inhibitor, with 1-year follow-up showing tumor stability and reduction of T2 cord signal cranial and caudal to the lesion (76).
IDH
Mutations in the isocitrate dehydrogenase (IDH) gene are common in intracranial Grades 2 and 3 astrocytomas; however, their prevalence is rarer in intramedullary astrocytomas (115). IDH1 and IDH2 are enzymes responsible for conversion of isocitrate in the citric acid cycle, and mutations result in accumulation of R-2-hydroxyglutarate (116). This metabolite competitively inhibits histone demethylases, resulting in broad epigenetic changes to DNA methylation and gene expression (83). IDH mutations can be used intracranially as both a diagnostic marker to distinguish diffuse from pilocytic astrocytomas, given its rare incidence in pilocytic astrocytomas, and as a prognostic marker, with IDH-mutant tumors having a more favorable prognosis compared to wild-type tumors (115, 117).
The prognosis of IDH mutations is less clear for intramedullary astrocytomas, given their low frequency (84, 85). Indeed, a review of 58 patients with spinal cord astrocytomas by Chai et al. did not identify any IDH mutations (95). Five cases of IDH1 mutations in intramedullary astrocytomas were noted by Konovalov et al. using next generation sequencing, noting that rare and unique variants were identified that are not conventionally tested using immunohistochemistry. Therefore, the true prevalence of IDH1 mutations in spinal cord astrocytomas may be greater than reported, as only the dominant intracranial R132H variant is generally tested using immunohistochemistry (115). Similarly, Takai et al. reported an IDH1 mutation in an adult female with a histological Grade 2 intramedullary diffuse astrocytoma. A R132S mutation was noted using Sanger sequencing, again differing from the dominant R132H traditionally examined (118). Although IDH mutations are generally a favorable prognostic factor, the patient only survived 11 months, below the average expected for astrocytomas of similar histological grade. In contrast, Nagashima et al. reported R132C and R132H mutations in two patients with Grade 2 intramedullary astrocytomas, noting a favorable prognosis with both patients alive with 22–37 months of follow-up (119). Next generation sequencing and identification of larger cohorts of IDH-mutant intramedullary astrocytomas is needed to determine its prognostic value.
TP53
The tumor suppressor protein p53 is encoded by the TP53 gene and is involved in regulation of the cell cycle, including cell cycle arrest and apoptosis upon detection of damage (120). TP53 is altered in over 20% of GBMs, but is also commonly mutated in lower grade adult diffuse astrocytomas (86, 121, 122). Mutation promotes initiation, maintenance, and progression of tumor growth and has been linked to adverse outcomes (123). Lebrun et al. found that TP53 mutations were present in 21% of examined intramedullary astrocytomas, including 53% of high-grade lesions. In particular, H3K27M-mutant astrocytomas had the highest rate of TP53 mutations, accounting for 64% of cases (86). The median survival of five patients with intramedullary TP53-mutated astrocytomas was reported by Alvi et al. as 11.5 months (124). Noor et al. suggested that outcomes after chemotherapy may be improved in low-grade gliomas with a TP53 mutation; however, this study was not specific to intramedullary astrocytomas (120).
Deregulation of TP53 can promote overexpression of mutant-p53, which accumulates in the nucleus and drives tumorigenesis through its interaction with oncoproteins (120, 121). Under physiological conditions, p53 expression levels are kept low unless activated by cellular stress or DNA damage (125). Mutant-p53 has a longer half-life compared to wild-type–p53 and can, thus, be more readily detected by immunohistochemistry (125). Both TP53 mutations and overexpression of p53 correlate with a poor prognosis (122). Similar to TP53 mutations, p53 overexpression is particularly found in high-grade astrocytomas. Govindan et al. detected immunoreactivity for p53 in 5/6 intramedullary GBMs, while Santi et al. reported p53 in 7/10 intramedullary high-grade astrocytomas (126, 127). High levels have been reported especially in secondary GBM, which are tumors that developed from lower grade lesions (127). Of note, Sarkar et al. reported high p53 expression in astrocytomas that progressed to GBM compared with recurrent tumors that remained at the same histological grade, suggesting inactivation of the p53 tumor suppressor pathway may play a role in tumor progression to GBM (127, 128).
Cyclin-dependent kinases
Cyclin-dependent kinases play a critical role in cell cycle regulation. Homozygous deletion of the CDKN2A locus is associated with a poor prognosis and is associated with progression to high-grade gliomas (129). The locus encodes the p14 and p16 tumor suppressor proteins, which induce cell cycle arrest, such that deletion of the gene promotes cellular proliferation (130). Although frequently encountered in astrocytomas, few studies have examined CDKN2A deletion specifically in intramedullary tumors, although Horbinski et al. noted that homozygous deletion was seen more frequently in tumors of the spinal cord, midbrain, and brainstem than those of the cerebellum or cerebrum (131). These deletions have particularly been found in IDH-mutant astrocytomas, leading some to argue that the IDH-mutant category should be stratified further using genetic markers such as CKDN2A (132, 133). Lu et al. conducted a systematic review of nine studies analyzing a total of 2,193 IDH-mutant gliomas, concluding that homozygous deletion of CDKN2A was a significant predictor of both shorter progression-free and overall survival in astrocytomas. However, the study was not specific to spinal cord astrocytomas, and further research is needed in this population.
Relatedly, CDK4 amplification has been associated with poor outcomes in astrocytomas (133). Unlike p14 and p16, CDK4 drives cell cycle progression by forming a complex, which releases the transcription factor E2F from the Rb protein (134). Therefore, both CDK4 amplification and deletion of CDKN2A function to promote cellular proliferation. Lin et al. described a refractory intramedullary astrocytoma with CDK4 amplification detected on next generation sequencing. The patient underwent two surgeries and received radiation therapy, TMZ, and bevacizumab, but nonetheless progressed. Treatment with Palbociclib, a selective CKD4 inhibitor, was effective at promoting tumor regression, and the patient was alive at the 2-year follow-up visit, illustrating the potential of personalized therapy for patients with intramedullary tumors (80).
ATRX
The ATRX gene regulates chromatin remodeling and genetic stability by depositing the histone variant H3.3 at heterochromatin and telomeres (83, 135). Inactivation can arise from mutations, deletions, and fusions, and is strongly associated with IDH1 mutations in diffuse and anaplastic astrocytomas (83, 135). Although most studies have investigated their frequency and prognosis in intracranial lesions, Lebrun et al. confirmed an increased prevalence of ATRX mutations among high-grade intramedullary astrocytomas compared to low-grade astrocytomas, detecting mutations in 33% of histological Grade 3 and 4 tumors, but only 13% of Grade 1 and 2 tumors (86). Overall, 10/61 cases were reported with ATRX mutations. Additionally, they were noted to occur more frequently in H3K27M–wild-type intramedullary astrocytomas compared to H3K27M-mutant astrocytomas. Yuzawa et al. reported a case of a 27-year-old man with neurofibromatosis Type I with a histological Grade 2 tumor. ATRX immunoreactivity was retained; however, residual tumor progressed in size 7 years later and appeared under histology as GBM with loss of ATRX expression. The case suggests that loss or inactivation of ATRX can be associated with malignant transformation of astrocytoma (136). Tumors lacking ATRX expression can potentially be targeted with epigenetic strategies aimed at restoring proper chromatin configuration. In addition, these tumors may be sensitive to pharmaceutical agents that damage DNA, given the genomic instability that arises in ATRX-mutant cells (81).
Discussion
Treatment strategies for primary intramedullary astrocytomas are centered around surgical resection of symptomatic lesions causing neurological deficits. The fact that treatment still relies mainly on the results of surgical resection reflects the lack of meaningful results from adjuvant therapy, as well as the lack of knowledge about the genetic features of intramedullary astrocytomas that would allow for targeted therapy. Although GTR or near-total resection can be achieved for well-circumscribed tumors presenting with a distinct cleavage plane, most astrocytomas infiltrate the cord and lack clear margins, precluding GTR without worsening the patient’s functional status and neurological deficits (137). Consequently, rates of GTR are low for intramedullary astrocytomas, while STR and biopsy are often the only feasible choices (24). For example, one retrospective study of 94 patients with intramedullary astrocytomas established a GTR rate of 13%, near-total resection rate of 34%, STR rate of 44%, and biopsy rate of 10% (24). Similarly, another institutional study of 12 patients with astrocytomas found that a dissection plane could be developed in only 29% of cases, compared to nearly all ependymomas (138). Nonetheless, the maximal safe resection can often be a near-total resection, and extent of resection has been associated with overall survival (24). Adjuvant radiation and chemotherapy can be provided, but their efficacy is not well-established, with conflicting reports in the literature on their impact on survival (24). Overall survival remains particularly poor in high-grade intramedullary astrocytomas, with a 5-year overall survival rate of approximately 14% for histological Grade 4 astrocytomas (17, 139). However, most studies investigating survival outcomes and the efficacy of resection have focused on histological grading systems from prior WHO categorization systems, rather than the updated 2021 guidelines incorporating molecular features.
Identification of molecular markers in tumor samples may help improve detection, assessing prognosis, and treatment of intramedullary tumors. Tumors with a clear plane can still undergo GTR during the index surgery, as the molecular features will not be known until the tumor specimen is analyzed post-operatively. However, STR and biopsy may suffice for most astrocytomas when the goal of surgery is obtaining sufficient tissue to identify genetic alterations. The tumor can be examined for its responsiveness to RT, TMZ chemotherapy, and targeted therapy, thus reducing the occurrence of post-operative neurological deficits associated with maximal resection of infiltrative lesions. In cases with a large tumor burden where the adjuvant treatment is not known to be beneficial, increasing the resection extent may be advocated before initiating adjuvant treatment.
Advances in liquid biopsy technology may replace the need for an invasive surgical biopsy. Short fragments of circulating tumor DNA can be detected in cerebrospinal fluid and peripheral blood, offering a noninvasive method of assessing tumor mutational burden (140). Several studies have illustrated the feasibility of detecting DNA from H3K27M-mutated diffuse midline gliomas in cerebrospinal fluid (140). Methodologies to rapidly sequence IDH, TP53, and ATRX mutations in cerebrospinal fluid have also been described (141). However, this work has mainly been performed for intracranial tumors, and clinical validation is needed in intramedullary cohorts, where anatomic sequestration may limit its role (142). Translation of the technology to intramedullary tumors may allow for sampling of tumor DNA prior to any surgical intervention, providing the surgeon, oncology team, and patients with a set of defined mutations informing them of prognosis and treatment options. The benefit would be greatest in high-grade tumors with infiltrative margins where GTR is not feasible and surgical intervention carries substantial risks. Patients with high-grade lesions deemed resistant to radiation, chemotherapy, and targeted therapy can decide on the benefits of surgical debulking. Liquid biopsies can also allow for monitoring of tumors throughout treatment, offering an enhanced view of tumor evolution and regression and the effectiveness of targeted therapy (143).
The effectiveness of conventional radiation and chemotherapy for intramedullary astrocytomas is limited (24). However, by defining the key mutations in a tumor, either through surgical or liquid biopsy, an opportunity exists for personalized and targeted therapy. Although much of the research on targeted therapies is focused on intracranial tumors, treatment may be extended to astrocytomas of the spinal cord harboring the same genetic mutations. Further work is needed to confirm findings restricted to intracranial cohorts, given that subtle genetic differences may account for discrepant responses. Dabrafenib, a B-raf inhibitor, and trametinib, a MEK inhibitor, can be administered to patients with overactivation of the MAPK pathway stemming from a BRAF V600E mutation. These drugs are administered for melanomas and lung cancers harboring BRAF V600E mutations, and the combination showed meaningful responses in 33% of high-grade and 69% of low-grade gliomas in a Phase II clinical trial (144). Another trial of dabrafenib in pediatric patients with refractory or progressive BRAF V600E tumors illustrated an objective response rate in 44% of patients, larger than the response traditionally observed with chemotherapy, although the results may be limited to intracranial tumors (145). Additionally, several IDH1 inhibitors are being tested for treatment of IDH-mutant astrocytomas, including ivosidenib and enasidenib. Identification of an IDH mutation also suggests that the tumor may have increased susceptibility to agents that damage DNA, along with conventional TMZ therapy, given the association between IDH mutations and impaired DNA repair (78). MGMT analogs, such as O6-benzylguanine and O6-(4-bromothenyl) guanine, can inactivate MGMT and sensitize gliomas to TMZ (75). A Phase II trial combining O6-benzylguanine with Gliadel wafers, or carmustine implants that deliver local chemotherapy, showed efficacy in patients with recurrent GBM, although patients were not stratified on MGMT status (146). The immune system can also be directed against molecular markers on astrocytomas by genetically modifying cytotoxic T lymphocytes to express a chimeric antigen receptor that recognizes a specific tumor antigen (147, 148). A Phase I clinical trial was reported showing that chimeric antigen receptor T cells can target the disialoganglioside GD2 expressed on H3K27M-mutant diffuse midline gliomas with clinical and radiographic improvement noted in three of four patients (149).
Challenges persist in the successful integration of targeted therapy with surgical treatment, RT, and chemotherapy for intramedullary astrocytomas. Despite an increasing number of reports in the literature concerning the mutational landscape of intramedullary astrocytomas, most studies have been limited to intracranial lesions. Important differences in the frequency and types of mutations have been noted across the two regions—for example, although KIAA1549-BRAF fusions are detected in astrocytomas in both regions, their frequency and breakpoint locations have been noted to differ (85, 86). The rarity of intramedullary astrocytomas has posed a challenge to accumulating large cohorts for genetic and epigenetic analysis. Consequently, research into targeted therapies is often limited to intracranial, rather than intramedullary, gliomas, although some trials, such as the imipridone ONC201 drug for H3 K27-M altered gliomas, include patients with spinal cord tumors (99). Additionally, biopsy specimens of spinal cord tumors are often small, given the tumors’ size and infiltrative nature, which can render genetic analysis difficult (83). Moreover, chemotherapy and targeted treatments can be limited by the blood-spinal cord barrier, which functions similarly to the blood-brain barrier to form a specialized micro-environment and restrict diffusion of large molecules into the central nervous system (150). Novel treatment strategies are being designed to improve permeability of the blood-spinal cord barrier, including the use of focused ultrasound to cause microbubble-induced transient opening of the barrier and improve drug delivery (151, 152).
Furthermore, although genetic differences exist between intracranial and intramedullary tumors, considerably less research has investigated differences across tumors by location in the spinal cord. It is reasonable to suspect that further sub-classification of tumors is possible within the spinal cord, just as genetic differences exist for intracranial astrocytomas according to region of the brain. Intramedullary tumors are usually reported with greater frequency in the cervical cord compared to the thoracic cord, which some authors’ suggestion reflects the higher density of gray matter in the cervical location (17, 153). Future investigations should examine whether cervical and thoracic astrocytomas differ in their genetic landscape.
The dismal prognosis of intramedullary astrocytomas, particularly high-grade lesions, may eventually be improved by combining traditional surgical management with molecular analysis and targeted therapy. An algorithm that may guide future surgical management is presented in Figure 3. Symptomatic patients with well-circumscribed tumors and distinct cleavage planes, often seen in pilocytic astrocytomas, may undergo GTR with careful attention to neuromonitoring. In contrast, patients with diffuse, infiltrative astrocytomas may warrant only an STR with biopsy to identify the genomic features of the astrocytoma. Subsequent treatment can be tailored to the tumor using radiation, TMZ, and targeted agents, and liquid biopsies and serial MRIs can be used to monitor treatment response. A combination of agents may be needed, given the diverse range of mutations and heterogeneity seen across tumor cells within a patient. Recurrent or progressive tumors can be analyzed for new genetic markers that may represent opportunities for additional therapy. Tumors broadly resistant to targeted agents may be candidates for repeat resection after a discussion with the patient about their prognosis and surgical risks.
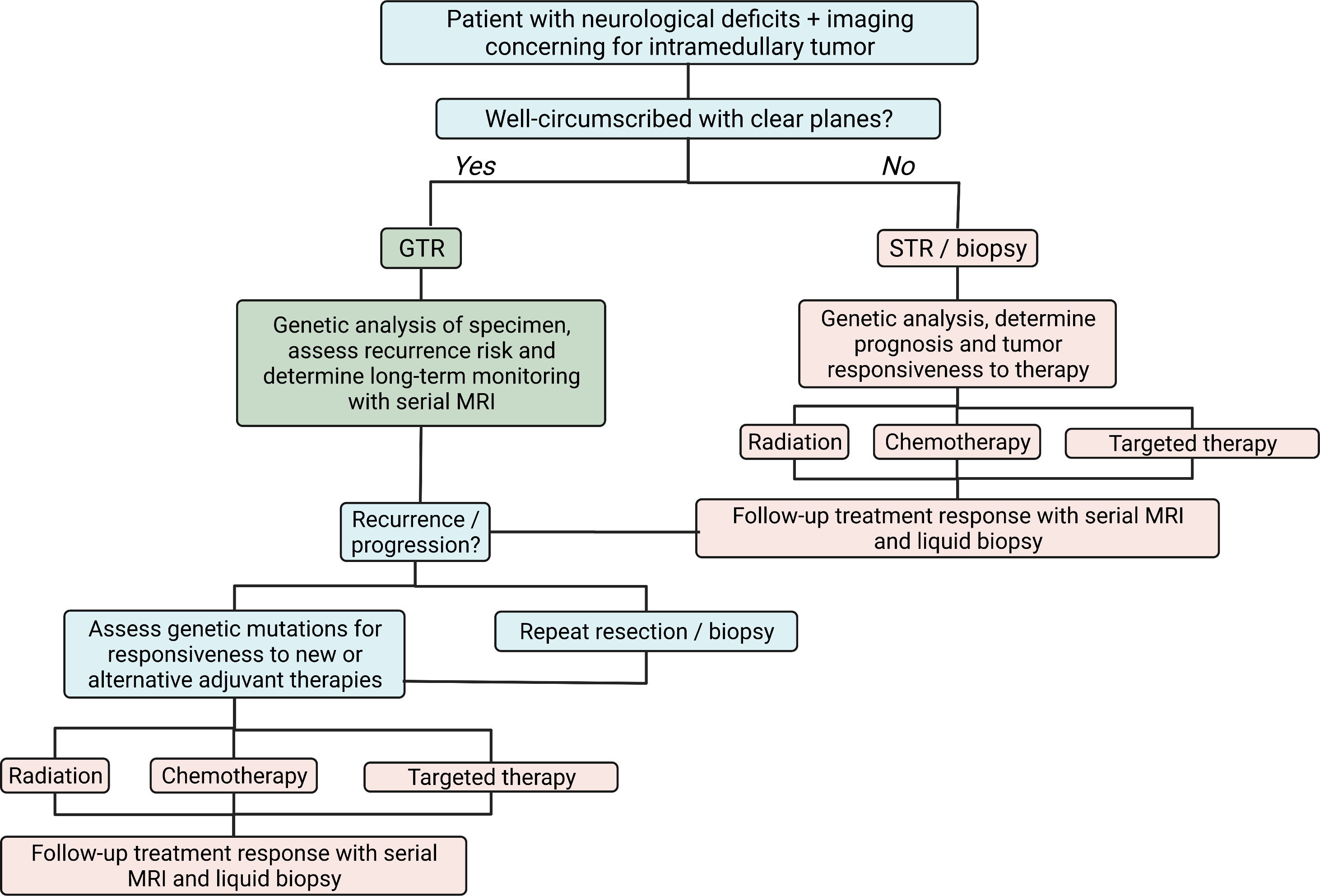
Figure 3 Algorithm to guide surgical decision-making and adjuvant treatment in patients with intramedullary astrocytomas using genetic analysis of tumors. Created with BioRender.com.
Conclusion
Intramedullary astrocytomas are rare tumors that frequently present with infiltrative margins. Surgical resection using a midline myelotomy or dorsal root entry zone myelotomy is considered the mainstay of treatment; however, GTR is often not feasible in diffuse lesions without substantial risk of new post-operative deficits. Overall survival in high-grade astrocytomas is poor with few available treatment options. Molecular analysis of spinal cord tumors can help determine prognosis and therapeutic opportunities. Mutations in H3K27M, MGMT, BRAF, IDH, TP53, CDKN2A, CDK4, and ATRX have been described in these lesions and influence the aggressiveness and behavior of the tumor. Biopsy specimens from surgical resection can be analyzed to determine the unique genomic landscape of an intramedullary astrocytoma, and assess its responsiveness to radiation, chemotherapy, and targeted therapy, allowing surgical and oncological teams to select an optimal treatment regimen. Multi-institutional large cohorts are needed to validate the frequency and implications of genetic mutations in intramedullary astrocytomas, and clinical trials are of critical need to determine therapeutic agents for personalized therapy.
Author contributions
Conceptualization – NS and GJ. Investigation - AH. Writing: Original Draft - AH. Writing: Review and Editing – NS and GJ. Visualization – AH and GJ. Supervision – NS and GJ. All authors contributed to the article and approved the submitted version.
Conflict of interest
The authors declare that the research was conducted in the absence of any commercial or financial relationships that could be construed as a potential conflict of interest.
Publisher’s note
All claims expressed in this article are solely those of the authors and do not necessarily represent those of their affiliated organizations, or those of the publisher, the editors and the reviewers. Any product that may be evaluated in this article, or claim that may be made by its manufacturer, is not guaranteed or endorsed by the publisher.
Abbreviations
GBM, Glioblastoma; GTR, Gross total resection; IDH, Isocitrate dehydrogenase; MGMT, O6-methylguanine-DNA methyltransferase; MRI, Magnetic resonance imaging; RT, Radiation therapy; STR, Subtotal resection; TMZ, Temozolomide; WHO, World Health Organization.
References
1. Samartzis D, Gillis CC, Shih P, O’Toole JE, Fessler RG. Intramedullary spinal cord tumors: Part I–epidemiology, pathophysiology, and diagnosis. Glob Spine J (2015) 5:425. doi: 10.1055/S-0035-1549029
2. Yang S, Yang X, Wang H, Gu Y, Feng J, Qin X, et al. Development and validation of a personalized prognostic prediction model for patients with spinal cord astrocytoma. Front Med (2022) 8:802471/BIBTEX. doi: 10.3389/FMED.2021.802471/BIBTEX
3. Luksik AS, Garzon-Muvdi T, Yang W, Huang J, Jallo GI. Pediatric spinal cord astrocytomas: a retrospective study of 348 patients from the SEER database. J Neurosurg Pediatr (2017) 19:711–9. doi: 10.3171/2017.1.PEDS16528
4. Jallo GI, Freed D, Epstein F. Intramedullary spinal cord tumors in children. Child’s Nerv Syst (2003) 19:641–9. doi: 10.1007/S00381-003-0820-3
5. Kandemirli SG, Reddy A, Hitchon P, Saini J, Bathla G. Intramedullary tumours and tumour mimics. Clin Radiol (2020) 75:876.e17–876.e32. doi: 10.1016/J.CRAD.2020.05.010
6. Khalid S, Kelly R, Carlton A, Wu R, Peta A, Melville P, et al. Adult intradural intramedullary astrocytomas: A multicenter analysis. J Spine Surg (2019) 5:19. doi: 10.21037/JSS.2018.12.06
7. Louis DN, Perry A, Wesseling P, Brat DJ, Cree IA, Figarella-Branger D, et al. The 2021 WHO classification of tumors of the central nervous system: a summary. Neuro Oncol (2021) 23:1231–51. doi: 10.1093/NEUONC/NOAB106
8. Guss ZD, Moningi S, Jallo GI, Cohen KJ, Wharam MD, Terezakis SA. Management of pediatric spinal cord astrocytomas: Outcomes with adjuvant radiation. Int J Radiat Oncol Biol Phys (2013) 85:1307. doi: 10.1016/J.IJROBP.2012.11.022
9. Shao J, Jones J, Ellsworth P, Habboub G, Cioffi G, Patil N, et al. A comprehensive epidemiological review of spinal astrocytomas in the united states. J Neurosurg Spine (2020) 34:303–9. doi: 10.3171/2020.6.SPINE191532
10. Iyer RR, Jallo GI. Intramedullary spinal cord tumors. Brain and Spine Surgery in the Elderly. Eds. Berhouma M., Krolak-Salmon P. Springer, Cham (2017). doi: 10.1007/978-3-319-40232-1_16
11. Seaman SC, Bathla G, Park BJ, Woodroffe RW, Smith M, Menezes AH, et al. MRI Characteristics and resectability in spinal cord glioma. Clin Neurol Neurosurg (2021) 200. doi: 10.1016/J.CLINEURO.2020.106321
12. Shih RY, Koeller KK. Intramedullary masses of the spinal cord: Radiologic-pathologic correlation. Radiographics (2020) 40:1125–45. doi: 10.1148/RG.2020190196/ASSET/IMAGES/LARGE/RG.2020190196.FIG11A.JPEG
13. Herbrecht A, Messerer M, Parker F. Development of a lateralization index for intramedullary astrocytomas and ependymomas. Neurochirurgie (2017) 63:410–2. doi: 10.1016/J.NEUCHI.2016.04.006
14. Lemay A, Gros C, Zhuo Z, Zhang J, Duan Y, Cohen-Adad J, et al. Automatic multiclass intramedullary spinal cord tumor segmentation on MRI with deep learning. NeuroImage Clin (2021) 31. doi: 10.1016/J.NICL.2021.102766
15. Liu H, Jiao M, Yuan Y, Ouyang H, Liu J, Li Y, et al. Benign and malignant diagnosis of spinal tumors based on deep learning and weighted fusion framework on MRI. Insights Imaging (2022) 13:1–11. doi: 10.1186/S13244-022-01227-2/FIGURES/7
16. Liu D, Chen J, Hu X, Yang K, Liu Y, Hu G, et al. Imaging-genomics in glioblastoma: Combining molecular and imaging signatures. Front Oncol (2021) 11:699265/BIBTEX. doi: 10.3389/FONC.2021.699265/BIBTEX
17. Hersh AM, Patel J, Pennington Z, Porras JL, Goldsborough E, Antar A, et al. Perioperative outcomes and survival after surgery for intramedullary spinal cord tumors: a single-institutional series of 302 patients. J Neurosurg Spine (2022) 37(2):1–11. doi: 10.3171/2022.1.SPINE211235
18. Koeller KK, Rosenblum RS, Morrison AL. Neoplasms of the spinal cord and filum terminale: radiologic-pathologic correlation. Radiographics (2000) 20:1721–49. doi: 10.1148/RADIOGRAPHICS.20.6.G00NV151721
19. Cohen AR, Wisoff JH, Allen JC, Epstein F. Malignant astrocytomas of the spinal cord. J Neurosurg (1989) 70:50–4. doi: 10.3171/JNS.1989.70.1.0050
20. Houten JK, Cooper PR. Spinal cord astrocytomas: Presentation, management and outcome. J Neurooncol. (2000) 47:219–24. doi: 10.1023/A:1006466422143
21. Beneš V, Barsa P, Beneš V, Suchomel P. Prognostic factors in intramedullary astrocytomas: a literature review. Eur Spine J (2009) 18:1397–422. doi: 10.1007/S00586-009-1076-8
22. Campello C, Parker F, Slimani S, Le Floch A, Herbrecht A, Aghakhani N, et al. Adult intramedullary gliomas. Neurochirurgie (2017) 63:381–90. doi: 10.1016/J.NEUCHI.2016.10.003
23. Cossu G, Lacroix C, Adams C, Daniel RT, Parker F, Messerer M. Neuroglial intramedullary tumors: The collaboration between neurosurgeons and neuropathologists. Neurochirurgie (2017) 63:413–8. doi: 10.1016/J.NEUCHI.2016.06.006
24. Hersh AM, Antar A, Pennington Z, Aygun N, Patel J, Goldsborough 3E, et al. Predictors of survival and time to progression following operative management of intramedullary spinal cord astrocytomas. J Neuro-Oncol (2022) 1:1–11. doi: 10.1007/S11060-022-04017-4
25. McGirt MJ, Goldstein IM, Chaichana KL, Tobias ME, Kothbauer KF, Jallo GI. Extent of surgical resection of malignant astrocytomas of the spinal cord: outcome analysis of 35 patients. Neurosurgery (2008) 63:55–60. doi: 10.1227/01.NEU.0000335070.37943.09
26. Wong AP, Dahdaleh NS, Fessler RG, Melkonian SC, Lin Y, Smith ZA, et al. Risk factors and long-term survival in adult patients with primary malignant spinal cord astrocytomas. J Neurooncol. (2013) 115:493–503. doi: 10.1007/S11060-013-1251-Y
27. Hersh AM, Patel J, Pennington Z, Antar A, Goldsborough E, Porras JL, et al. A novel online calculator to predict nonroutine discharge, length of stay, readmission, and reoperation in patients undergoing surgery for intramedullary spinal cord tumors. Spine J (2022) 22(8):1345–55. doi: 10.1016/J.SPINEE.2022.03.005
28. Alizada O, Kemerdere R, Ulu MO, Akgun MY, Isler C, Kizilkilic O, et al. Surgical management of spinal intramedullary tumors: Ten-year experience in a single institution. J Clin Neurosci (2020) 73:201–8. doi: 10.1016/J.JOCN.2019.12.054
29. Karikari IO, Nimjee SM, Hodges TR, Cutrell E, Hughes BD, Powers CJ, et al. Impact of tumor histology on resectability and neurological outcome in primary intramedullary spinal cord tumors: A single-center experience with 102 patients. Neurosurgery (2015) 76:S4–S13. doi: 10.1227/01.neu.0000462073.71915.12
30. Butenschoen VM, Hubertus V, Janssen IK, Onken J, Wipplinger C, Mende KC, et al. Surgical treatment and neurological outcome of infiltrating intramedullary astrocytoma WHO II–IV: a multicenter retrospective case series. J Neurooncol. (2021) 151:181. doi: 10.1007/S11060-020-03647-W
31. Golpayegani M, Edalatfar M, Ahmadi A, Sadeghi-Naini M, Salari F, Hanaei S, et al. Complete versus incomplete surgical resection in intramedullary astrocytoma: Systematic review with individual patient data meta-analysis. Global Spine J (2022) 2022. doi: 10.1177/21925682221094766
32. Persson O, Fletcher-Sandersjöö A, Burström G, Edström E, Elmi-Terander A. Surgical treatment of intra- and juxtamedullary spinal cord tumors: A population based observational cohort study. Front Neurol (2019) 10:814. doi: 10.3389/fneur.2019.00814
33. Pojskić M, Arnautović KI. Microsurgical resection of low-grade spinal cord astrocytoma: 2-dimensional operative video. Oper Neurosurg (2019) 17:E107. doi: 10.1093/ONS/OPY386
34. Prada F, Vetrano IG, Filippini A, Del Bene M, Perin A, Casali C, et al. Intraoperative ultrasound in spinal tumor surgery. J Ultrasound (2014) 17:195. doi: 10.1007/S40477-014-0102-9
35. Ren J, He C, Hong T, Li X, Ma Y, Yu J, et al. Anterior to dorsal root entry zone myelotomy (ADREZotomy): A new surgical approach for the treatment of ventrolateral deep intramedullary spinal cord cavernous malformations. Spine (Phila Pa 1976) (2018) 43:E1024–32. doi: 10.1097/BRS.0000000000002607
36. Manzano G, Green BA, Vanni S, Levi AD. Contemporary management of adult intramedullary spinal tumors–pathology and neurological outcomes related to surgical resection. Spinal Cord (2008) 46:540–6. doi: 10.1038/sc.2008.51
37. Takami T, Naito K, Yamagata T, Ohata K. Surgical management of spinal intramedullary tumors: Radical and safe strategy for benign tumors. Neurol Med Chir (Tokyo) (2015) 55:317. doi: 10.2176/NMC.RA.2014-0344
38. Woodroffe RW, Zanaty M, Kirby P, Dlouhy BJ, Menezes AH. Resection of a pediatric intramedullary spinal cord tumor: 2-dimensional operative video. Oper Neurosurg (Hagerstown Md) (2019) 16:518. doi: 10.1093/ONS/OPY185
39. Hussain I, Parker WE, Barzilai O, Bilsky MH. Surgical management of intramedullary spinal cord tumors. Neurosurg Clin N Am (2020) 31:237–49. doi: 10.1016/J.NEC.2019.12.004
40. Tabibkhooei A, Sadeghipour A, Fattahi A. Thoracolumbar pilomyxoid astrocytoma concomitant with spinal scoliosis: A case report and literature review. Surg Neurol Int (2019) 10. doi: 10.25259/SNI_548_2019
41. Teng YD, Abd-El-Barr M, Wang L, Hajiali H, Wu L, Zafonte RD. Spinal cord astrocytomas: progresses in experimental and clinical investigations for developing recovery neurobiology-based novel therapies. Exp Neurol (2019) 311:135–47. doi: 10.1016/J.EXPNEUROL.2018.09.010
42. Sala F, Palandri G, Basso E, Lanteri P, Deletis V, Faccioli F, et al. Motor evoked potential monitoring improves outcome after surgery for intramedullary spinal cord tumors: A historical control study. Neurosurgery (2006) 58:1129–43. doi: 10.1227/01.NEU.0000215948.97195.58
43. Cheng JS, Ivan ME, Stapleton CJ, Quinones-Hinojosa A, Gupta N, Auguste KI. Intraoperative changes in transcranial motor evoked potentials and somatosensory evoked potentials predicting outcome in children with intramedullary spinal cord tumors. J Neurosurg Pediatr (2014) 13:591. doi: 10.3171/2014.2.PEDS1392
44. Rijs K, Klimek M, Scheltens-de Boer M, Biesheuvel K, Harhangi BS. Intraoperative neuromonitoring in patients with intramedullary spinal cord tumor: A systematic review, meta-analysis, and case series. World Neurosurg (2019) 125:498–510.e2. doi: 10.1016/J.WNEU.2019.01.007
45. Kimchi G, Knoller N, Korn A, Eyal-Mazuz Y, Sapir Y, Peled A, et al. Delayed variations in the diagnostic accuracy of intraoperative neuromonitoring in the resection of intramedullary spinal cord tumors. Neurosurg Focus (2021) 50:1–7. doi: 10.3171/2021.2.FOCUS201084
46. McGirt MJ, Chaichana KL, Atiba A, Bydon A, Witham TF, Yao KC, et al. Incidence of spinal deformity after resection of intramedullary spinal cord tumors in children who underwent laminectomy compared with laminoplasty. J Neurosurg Pediatr (2008) 1:57–62. doi: 10.3171/PED-08/01/057
47. Ahmed R, Menezes AH, Awe OO, Mahaney KB, Torner JC, Weinstein SL. Long-term incidence and risk factors for development of spinal deformity following resection of pediatric intramedullary spinal cord tumors: Clinical article. J Neurosurg Pediatr (2014) 13:613–21. doi: 10.3171/2014.1.PEDS13317
48. Hersh DS, Iyer RR, Garzon-Muvdi T, Liu A, Jallo GI, Groves ML. Instrumented fusion for spinal deformity after laminectomy or laminoplasty for resection of intramedullary spinal cord tumors in pediatric patients. Neurosurg Focus (2017) 43:E12. doi: 10.3171/2017.7.FOCUS17329
49. Lee YS, Kim YB, Park SW. Spinous process-splitting hemilaminoplasty for intradural and extradural lesions. J Korean Neurosurg Soc (2015) 58:494. doi: 10.3340/JKNS.2015.58.5.494
50. Raco A, Esposito VV, Lenzi J, Piccirilli M, Delfini R, Cantore G. Long-term follow-up of intramedullary spinal cord tumors: A series of 202 cases. Neurosurgery (2005) 56:972–81. doi: 10.1227/01.NEU.0000158318.66568.CC
51. Adams H, Avendaño J, Raza SM, Gokaslan ZL, Jallo GI, Quiñones-Hinojosa A. Prognostic factors and survival in primary malignant astrocytomas of the spinal cord: a population-based analysis from 1973 to 2007. Spine (Phila Pa 1976) (2012) 37:E727–E735. doi: 10.1097/BRS.0B013E31824584C0
52. Haque W, Verma V, Barber S, Tremont IW, Brian Butler E, Teh BS. Management, outcomes, and prognostic factors of adult primary spinal cord gliomas. J Clin Neurosci (2021) 84:8–14. doi: 10.1016/J.JOCN.2020.12.015
53. Parker F, Campello C, Lejeune JP, David P, Herbrecht A, Aghakhani N, et al. Intramedullary astrocytomas: A French retrospective multicenter study. Neurochirurgie (2017) 63:402–9. doi: 10.1016/J.NEUCHI.2016.09.007
54. Hamilton KR, Lee SS, Urquhart JC, Jonker BP. A systematic review of outcome in intramedullary ependymoma and astrocytoma. J Clin Neurosci (2019) 63:168–75. doi: 10.1016/J.JOCN.2019.02.001
55. Nagoshi N, Tsuji O, Suzuki S, Nori S, Yagi M, Okada E, et al. Clinical outcomes and a therapeutic indication of intramedullary spinal cord astrocytoma. Spinal Cord (2021) 60:216–22. doi: 10.1038/S41393-021-00676-8
56. Barkley A, McGrath LB, Hofstetter CP. Intraoperative contrast-enhanced ultrasound for intramedullary spinal neoplasms: patient series. J Neurosurg Case Lessons (2021) 1:2021. doi: 10.3171/CASE2083
57. Carl B, Bopp M, Saß B, Pojskic M, Nimsky C. Augmented reality in intradural spinal tumor surgery. Acta Neurochir. (Wien) (2019) 161:2181–93. doi: 10.1007/S00701-019-04005-0
58. Benjamin CG, Frempong-Boadu A, Hoch M, Bruno M, Shepherd T, Pacione D. Combined use of diffusion tractography and advanced intraoperative imaging for resection of cervical intramedullary spinal cord neoplasms: A case series and technical note. Oper Neurosurg (Hagerstown Md) (2019) 17:525–30. doi: 10.1093/ONS/OPZ039
59. Choudhri AF, Whitehead MT, Klimo P, Montgomery BK, Boop FA. Diffusion tensor imaging to guide surgical planning in intramedullary spinal cord tumors in children. Neuroradiology (2014) 56:169. doi: 10.1007/S00234-013-1316-9
60. Granata F, Racchiusa S, Mormina E, Barres V, Garufi G, Grasso G, et al. Presurgical role of MRI tractography in a case of extensive cervicothoracic spinal ependymoma. Surg Neurol Int (2017) 8:56–6. doi: 10.4103/SNI.SNI_33_17
61. Ahmed R, Menezes AH, Torner JC. Role of resection and adjuvant therapy in long-term disease outcomes for low-grade pediatric intramedullary spinal cord tumors. J Neurosurg Pediatr (2016) 18:594–601. doi: 10.3171/2016.5.PEDS15356
62. Mora J, Cruz O, Gala S, Navarro R. Successful treatment of childhood intramedullary spinal cord astrocytomas with irinotecan and cisplatin. Neuro Oncol (2007) 9:39. doi: 10.1215/15228517-2006-026
63. Jallo GI, Danish S, Velasquez L, Epstein F. Intramedullary low-grade astrocytomas: long-term outcome following radical surgery. J Neurooncol. (2001) 53:61–6. doi: 10.1023/A:1011886516506
64. Minehan KJ, Brown PD, Scheithauer BW, Krauss WE, Wright MP. Prognosis and treatment of spinal cord astrocytoma. Int J Radiat Oncol (2009) 73:727–33. doi: 10.1016/J.IJROBP.2008.04.060
65. Indelicato DJ, Rotondo RL, Uezono H, Sandler ES, Aldana PR, Ranalli NJ, et al. Outcomes following proton therapy for pediatric low-grade glioma. Int J Radiat Oncol (2019) 104:149–56. doi: 10.1016/J.IJROBP.2019.01.078
66. Kahn J, Loeffler JS, Niemierko A, Chiocca EA, Batchelor T, Chakravarti A. Long-term outcomes of patients with spinal cord gliomas treated by modern conformal radiation techniques. Int J Radiat Oncol Biol Phys (2011) 81:232–8. doi: 10.1016/J.IJROBP.2010.05.009
67. Hamilton KR, Lee SS, Urquhart JC, Jonker BP. A systematic review of outcome in intramedullary ependymoma and astrocytoma. J Clin Neurosci (2019) 63:168–75. doi: 10.1016/J.JOCN.2019.02.001
68. Harrop JS, Ganju A, Groff M, Bilsky M. Primary intramedullary tumors of the spinal cord. Spine (Phila Pa 1976) (2009) 34:S69–77. doi: 10.1097/BRS.0B013E3181B95C6F
69. Lowis SP, Pizer BL, Coakham H, Nelson RJ, Bouffet E. Chemotherapy for spinal cord astrocytoma: can natural history be modified? Childs Nerv Syst (1998) 14:317–21. doi: 10.1007/S003810050233
70. Hernández-Durán S, Bregy A, Shah AH, Hanft S, Komotar RJ, Manzano GR. Primary spinal cord glioblastoma multiforme treated with temozolomide. J Clin Neurosci (2015) 22:1877–82. doi: 10.1016/J.JOCN.2015.04.017
71. Akinduro OO, Garcia DP, Higgins DMO, Vivas-Buitrago T, Jentoft M, Solomon DA, et al. A multicenter analysis of the prognostic value of histone H3 K27M mutation in adult high-grade spinal glioma. J Neurosurg Spine (2021) 35(6):1–10. doi: 10.3171/2021.2.SPINE201675
72. Louis DN, Perry A, Reifenberger G, von Deimling A, Figarella-Branger D, Cavenee WK, et al. The 2016 world health organization classification of tumors of the central nervous system: a summary. Acta Neuropathol. (2016) 131:803–20. doi: 10.1007/S00401-016-1545-1/TABLES/5
73. Komori T. Grading of adult diffuse gliomas according to the 2021 WHO classification of tumors of the central nervous system. Lab Investig (2021) 102:126–33. doi: 10.1038/s41374-021-00667-6
74. Wierzbicki K, Ravi K, Franson A, Bruzek A, Cantor E, Harris M, et al. Targeting and therapeutic monitoring of H3K27M-mutant glioma. Curr Oncol Rep (2020) 22:19. doi: 10.1007/S11912-020-0877-0
75. Yu W, Zhang L, Wei Q, Shao A. O 6-Methylguanine-DNA methyltransferase (MGMT): Challenges and new opportunities in glioma chemotherapy. Front Oncol (2020) 9:1547. doi: 10.3389/FONC.2019.01547
76. Balasubramanian A, Gunjur A, Gan HK, Perchyonok Y, Cher LM. Response to combined BRAF/MEK inhibition in adult BRAF V600E mutant spinal pilocytic astrocytoma. J Clin Neurosci (2020) 79:269–71. doi: 10.1016/J.JOCN.2020.07.028
77. Schreck KC, Grossman SA, Pratilas CA. BRAF mutations and the utility of RAF and MEK inhibitors in primary brain tumors. Cancers (Basel) (2019) 11:1–18. doi: 10.3390/CANCERS11091262
78. Karpel-Massler G, Nguyen TTT, Shang E, Siegelin MD. Novel IDH1 targeted glioma therapies. CNS Drugs (2019) 33:1155. doi: 10.1007/S40263-019-00684-6
79. Hu J, Cao J, Topatana W, Juengpanich S, Li S, Zhang B, et al. Targeting mutant p53 for cancer therapy: direct and indirect strategies. J Hematol Oncol (2021) 14:157. doi: 10.1186/S13045-021-01169-0
80. Lin J, Yu L, Fu Y, Chen H, Zheng X, Wang S, et al. A refractory case of CDK4-amplified spinal astrocytoma achieving complete response upon treatment with a palbociclib-based regimen:a case report. BMC Cancer (2020) 20:1–6. doi: 10.1186/S12885-020-07061-3/TABLES/1
81. Haase S, Garcia-Fabiani MB, Carney S, Altshuler D, Núñez FJ, Méndez FM, et al. Mutant ATRX: uncovering a new therapeutic target for glioma. Expert Opin Ther Targets (2018) 22:599. doi: 10.1080/14728222.2018.1487953
82. Chi JH, Cachola K, Parsa AT. Genetics and molecular biology of intramedullary spinal cord tumors. Neurosurg Clin N Am (2006) 17:1–5. doi: 10.1016/J.NEC.2005.10.002
83. Zadnik PL, Gokaslan ZL, Burger PC, Bettegowda C. Spinal cord tumours: advances in genetics and their implications for treatment. Nat Rev Neurol (2013) 9:257. doi: 10.1038/NRNEUROL.2013.48
84. Azad TD, Jiang B, Bettegowda C. Molecular foundations of primary spinal tumors-implications for surgical management. Ann Transl Med (2019) 7:222–2. doi: 10.21037/ATM.2019.04.46
85. Zhang M, Iyer RR, Azad TD, Wang Q, Garzon-Muvdi T, Wang J, et al. Genomic landscape of intramedullary spinal cord gliomas. Sci Rep (2019) 9. doi: 10.1038/S41598-019-54286-9
86. Lebrun L, Meléndez B, Blanchard O, De Nève N, Van Campenhout C, Lelotte J, et al. Clinical, radiological and molecular characterization of intramedullary astrocytomas. Acta Neuropathol Commun (2020) 8:1–14. doi: 10.1186/S40478-020-00962-1/TABLES/3
87. Solomon DA, Wood MD, Tihan T, Bollen AW, Gupta N, Phillips JJJ, et al. Diffuse midline gliomas with histone H3-K27M mutation: A series of 47 cases assessing the spectrum of morphologic variation and associated genetic alterations. Brain Pathol (2016) 26:569–80. doi: 10.1111/BPA.12336
88. Cheng L, Wang L, Yao Q, Ma L, Duan W, Guan J, et al. Clinicoradiological characteristics of primary spinal cord H3 K27M-mutant diffuse midline glioma. J Neurosurg Spine (2021) 36(2):1–12. doi: 10.3171/2021.4.SPINE2140
89. Zhang YW, Chai RC, Cao R, Jiang WJ, Liu WH, Xu YL, et al. Clinicopathological characteristics and survival of spinal cord astrocytomas. Cancer Med (2020) 9:6996. doi: 10.1002/CAM4.3364
90. Ishi Y, Takamiya S, Seki T, Yamazaki K, Hida K, Hatanaka KC, et al. Prognostic role of H3K27M mutation, histone H3K27 methylation status, and EZH2 expression in diffuse spinal cord gliomas. Brain Tumor Pathol (2020) 37:81–8. doi: 10.1007/S10014-020-00369-9
91. Argersinger DP, Rivas SR, Shah AH, Jackson S, Heiss JD. New developments in the pathogenesis, therapeutic targeting, and treatment of H3K27M-mutant diffuse midline glioma. Cancers (Basel) (2021) 13. doi: 10.3390/CANCERS13215280
92. Lu VM, Alvi MA, McDonald KL, Daniels DJ. Impact of the H3K27M mutation on survival in pediatric high-grade glioma: a systematic review and meta-analysis. J Neurosurg Pediatr (2018) 23:308–16. doi: 10.3171/2018.9.PEDS18419
93. Gu Q, Huang Y, Zhang H, Jiang B. Case report: Five adult cases of H3K27-altered diffuse midline glioma in the spinal cord. Front Oncol (2021) 11:701113/BIBTEX. doi: 10.3389/FONC.2021.701113/BIBTEX
94. Low JT, Wang SH, Peters KB. Diffuse midline glioma with H3 K27M-mutation in an 83-year-old woman. CNS Oncol (2021) 10. doi: 10.2217/CNS-2020-0030
95. Chai RC, Zhang YW, Liu YQ, Chang YZ, Pang B, Jiang T, et al. The molecular characteristics of spinal cord gliomas with or without H3 K27M mutation. Acta Neuropathol Commun (2020) 8. doi: 10.1186/S40478-020-00913-W
96. Wang YZ, Zhang YW, Liu WH, Chai RC, Cao R, Wang B, et al. Spinal cord diffuse midline gliomas with H3 K27m-mutant: Clinicopathological features and prognosis. Neurosurgery (2021) 89:300–6. doi: 10.1093/NEUROS/NYAB174
97. Sturm D, Witt H, Hovestadt V, Khuong-Quang DA, Jones DTW, Konermann C, et al. Hotspot mutations in H3F3A and IDH1 define distinct epigenetic and biological subgroups of glioblastoma. Cancer Cell (2012) 22:425–37. doi: 10.1016/J.CCR.2012.08.024
98. Hashizume R, Andor N, Ihara Y, Lerner R, Gan H, Chen X, et al. Pharmacologic inhibition of histone demethylation as a therapy for pediatric brainstem glioma. Nat Med (2014) 20:1394–6. doi: 10.1038/NM.3716
99. Kurz SC, Tarapore R, Odia Y, Butowski NA, Koschmann CJ, Aguilera D, et al. Clinical experience of ONC201 in patients with recurrent H3 K27M-mutant spinal cord glioma. Journal of Clinical Oncology (2020) 38:2563. doi: 10.1200/JCO.2020.38.15_SUPPL.2563
100. Sun P, Fan DJ, Fan T, Li X, Qi XL, Zhao XG, et al. A prospective clinical study on MGMT protein expression and the effect of gene promoter methylation on sensitivity to chemotherapeutics in spinal glioma. J Inflammation Res (2021) 14:4777–84. doi: 10.2147/JIR.S321790
101. Zhang J FG, Stevens M, D. Bradshaw T. Temozolomide: mechanisms of action, repair and resistance. Curr Mol Pharmacol (2012) 5:102–14. doi: 10.2174/1874467211205010102
102. Hegi ME, Diserens A-C, Gorlia T, Hamou M-F, de Tribolet N, Weller M, et al. MGMT gene silencing and benefit from temozolomide in glioblastoma. N Engl J Med (2005) 352:997–1003. doi: 10.1056/NEJMOA043331
103. Mansouri A, Hachem LD, Mansouri S, Nassiri F, Laperriere NJ, Xia D, et al. MGMT promoter methylation status testing to guide therapy for glioblastoma: Refining the approach based on emerging evidence and current challenges. Neuro Oncol (2019) 21:167. doi: 10.1093/NEUONC/NOY132
104. Binabaj MM, Bahrami A, ShahidSales S, Joodi M, Joudi Mashhad M, Hassanian SM, et al. The prognostic value of MGMT promoter methylation in glioblastoma: A meta-analysis of clinical trials. J Cell Physiol (2018) 233:378–86. doi: 10.1002/JCP.25896
105. Banan R, Christians A, Bartels S, Lehmann U, Hartmann C. Absence of MGMT promoter methylation in diffuse midline glioma, H3 K27M-mutant. Acta Neuropathol Commun (2017) 5:98. doi: 10.1186/S40478-017-0500-2/FIGURES/1
106. Rovin RA, Winn R. Expression of O6-methylguanine–deoxyribose nucleic acid methyltransferase and temozolomide response in a patient with a malignant spinal cord astrocytoma: Case report. J Neurosurg Spine (2007) 6:447–50. doi: 10.3171/SPI.2007.6.5.447
107. Horbinski C. To BRAF or not to BRAF: Is that even a question anymore? J Neuropathol Exp Neurol (2013) 72:2. doi: 10.1097/NEN.0B013E318279F3DB
108. Kurani H, Gurav M, Shetty O, Chinnaswamy G, Moiyadi A, Gupta T, et al. Pilocytic astrocytomas: BRAFV600E and BRAF fusion expression patterns in pediatric and adult age groups. Child’s Nerv Syst (2019) 35:1525–36. doi: 10.1007/S00381-019-04282-1/TABLES/3
109. Penman CL, Faulkner C, Lowis SP, Kurian KM. Current understanding of BRAF alterations in diagnosis, prognosis, and therapeutic targeting in pediatric low-grade gliomas. Front Oncol (2015) 5:54. doi: 10.3389/FONC.2015.00054
110. Collins VP, Jones DTW, Giannini C. Pilocytic astrocytoma: pathology, molecular mechanisms and markers. Acta Neuropathol. (2015) 129:775. doi: 10.1007/S00401-015-1410-7
111. Sun M, Wang L, Lu D, Zhao Z, Teng L, Wang W, et al. Concomitant KIAA1549-BRAF fusion and IDH mutation in pediatric spinal cord astrocytoma: A case report and literature review. Brain Tumor Pathol (2021) 38:132–7. doi: 10.1007/S10014-021-00394-2/TABLES/1
112. Behling F, Schittenhelm J. Oncogenic BRAF alterations and their role in brain tumors. Cancers (Basel) (2019) 11. doi: 10.3390/CANCERS11060794
113. Marrazzo A, Cacchione A, Rossi S, Carboni A, Gandolfo C, Carai A, et al. Intradural pediatric spinal tumors: An overview from imaging to novel molecular findings. Diagn. (Basel Switzerland) (2021) 11. doi: 10.3390/DIAGNOSTICS11091710
114. Shankar GM, Lelic N, Gill CM, Thorner AR, Van Hummelen P, Wisoff JH, et al. BRAF alteration status and the histone H3F3A gene K27M mutation segregate spinal cord astrocytoma histology. Acta Neuropathol. (2016) 131:147. doi: 10.1007/S00401-015-1492-2
115. Konovalov NA, Asyutin DS, Shayhaev EG, Kaprovoy SV, Timonin SY. Rare cases of IDH1 mutations in spinal cord astrocytomas. Acta Naturae (2020) 12:70. doi: 10.32607/ACTANATURAE.10915
116. Losman JA, Kaelin WG. What a difference a hydroxyl makes: mutant IDH, (R)-2-hydroxyglutarate, and cancer. Genes Dev (2013) 27:836. doi: 10.1101/GAD.217406.113
117. Ellezam B, Theeler BJ, Walbert T, Mammoser AG, Horbinski C, Kleinschmidt-DeMasters BK, et al. Low rate of R132H IDH1 mutation in infratentorial and spinal cord grade II and III diffuse gliomas. Acta Neuropathol. (2012) 124:449–51. doi: 10.1007/S00401-012-1011-7/TABLES/1
118. Takai K, Tanaka S, Sota T, Mukasa A, Komori T, Taniguchi M. Spinal cord astrocytoma with isocitrate dehydrogenase 1 gene mutation. World Neurosurg (2017) 108:991.e13–991.e16. doi: 10.1016/J.WNEU.2017.08.142
119. Nagashima Y, Nishimura Y, Ohka F, Eguchi K, Aoki K, Ito H, et al. Driver genetic mutations in spinal cord gliomas direct the degree of functional impairment in tumor-associated spinal cord injury. Cells (2021) 10. doi: 10.3390/CELLS10102525
120. Noor H, Briggs NE, McDonald KL, Holst J, Vittorio O. Tp53 mutation is a prognostic factor in lower grade glioma and may influence chemotherapy efficacy. Cancers (Basel) (2021) 13:5362. doi: 10.3390/CANCERS13215362/S1
121. Zhang Y, Dube C, Gibert M, Cruickshanks N, Wang B, Coughlan M, et al. The p53 pathway in glioblastoma. Cancers (Basel) (2018) 10. doi: 10.3390/CANCERS10090297
122. Walker C, Baborie A, Crooks D, Wilkins S, Jenkinson MD. Biology, genetics and imaging of glial cell tumours. Br J Radiol (2011) 84:S090. doi: 10.1259/BJR/23430927
123. Marker DF, Agnihotri S, Amankulor N, Murdoch GH, Pearce TM. The dominant TP53 hotspot mutation in IDH -mutant astrocytoma, R273C, has distinctive pathologic features and sex-specific prognostic implications. Neuro-oncol. Adv (2021) 4:1–13. doi: 10.1093/NOAJNL/VDAB182
124. Alvi MA, Ida CM, Paolini MA, Kerezoudis P, Meyer J, Barr Fritcher EG, et al. Spinal cord high-grade infiltrating gliomas in adults: Clinico-pathological and molecular evaluation. Mod. Pathol (2019) 32:1236–43. doi: 10.1038/s41379-019-0271-3
125. Huang K, Chen L, Zhang J, Wu Z, Lan L, Wang L, et al. Elevated p53 expression levels correlate with tumor progression and poor prognosis in patients exhibiting esophageal squamous cell carcinoma. Oncol Lett (2014) 8:1441. doi: 10.3892/OL.2014.2343
126. Santi M, Mena H, Wong K, Koeller K, Olsen C, Rushing EJ. Spinal cord malignant astrocytomas. Cancer (2003) 98:554–61. doi: 10.1002/CNCR.11514
127. Govindan A, Chakraborti S, Mahadevan A, Chickabasavaiah YT, Santosh V, Shankar SK. Histopathologic and immunohistochemical profile of spinal glioblastoma: a study of six cases. Brain Tumor Pathol (2011) 28:297–303. doi: 10.1007/S10014-011-0041-5
128. Sarkar C, Ralte AM, Sharma MC, Mehta VS. Recurrent astrocytic tumours–a study of p53 immunoreactivity and malignant progression. Br J Neurosurg (2002) 16:335–42. doi: 10.1080/02688697021000007588
129. Cagney DN, Miller MB, Dubuc A, Delalle I, Ligon AH, Chukwueke U, et al. Clinical importance of CDKN2A loss and monosomy 10 in pilocytic astrocytoma. Cureus (2019) 11. doi: 10.7759/CUREUS.4726
130. Lu VM, O’Connor KP, Shah AH, Eichberg DG, Luther EM, Komotar RJ, et al. The prognostic significance of CDKN2A homozygous deletion in IDH-mutant lower-grade glioma and glioblastoma: A systematic review of the contemporary literature. J Neurooncol (2020) 148:221–9. doi: 10.1007/S11060-020-03528-2
131. Horbinski C, Hamilton RL, Nikiforov Y, Pollack IF. Association of molecular alterations, including BRAF, with biology and outcome in pilocytic astrocytomas. Acta Neuropathol. (2010) 119:641. doi: 10.1007/S00401-009-0634-9
132. Appay R, Dehais C, Maurage CA, Alentorn A, Carpentier C, Colin C, et al. CDKN2A homozygous deletion is a strong adverse prognosis factor in diffuse malignant IDH-mutant gliomas. Neuro Oncol (2019) 21:1519–28. doi: 10.1093/NEUONC/NOZ124
133. Yang RR, Shi Z-f, Zhang Zy, Chan AKY, Aibaidula A, Wang WW, et al. IDH mutant lower grade (WHO grades II/III) astrocytomas can be stratified for risk by CDKN2A, CDK4 and PDGFRA copy number alterations. Brain Pathol (2020) 30:541–53. doi: 10.1111/BPA.12801
134. Topacio BR, Zatulovskiy E, Cristea S, Xie S, Tambo CS, Rubin SM, et al. Cyclin d-Cdk4,6 drives cell-cycle progression via the retinoblastoma protein’s c-terminal helix. Mol Cell (2019) 74:758. doi: 10.1016/J.MOLCEL.2019.03.020
135. Nandakumar P, Mansouri A, Das S. The role of ATRX in glioma biology. Front Oncol (2017) 7:236. doi: 10.3389/FONC.2017.00236
136. Yuzawa S, Kamikokura Y, Tanino M, Takei H. Malignant transformation of NF1-associated spinal astrocytoma with loss of ATRX expression during the course: A case report. Clin Neuropathol. (2021) 40:201–8. doi: 10.5414/NP301314
137. Abd-El-Barr MM, Huang KT, Chi JH. Infiltrating spinal cord astrocytomas: Epidemiology, diagnosis, treatments and future directions. J Clin Neurosci (2016) 29:15–20. doi: 10.1016/J.JOCN.2015.10.048
138. Baig Mirza A, Gebreyohanes A, Knight J, Bartram J, Vastani A, Kalaitzoglou D, et al. Prognostic factors for surgically managed intramedullary spinal cord tumours: A single-centre case series. Acta Neurochir. (Wien) (2022). doi: 10.1007/S00701-022-05304-9
139. Milano MT, Johnson MD, Sul J, Mohile NA, Korones DN, Okunieff P, et al. Primary spinal cord glioma: a surveillance, epidemiology, and end results database study. J Neuro-Oncol. 2009 981 (2009) 98:83–92. doi: 10.1007/S11060-009-0054-7
140. Azad TD, Jin MC, Bernhardt LJ, Bettegowda C. Liquid biopsy for pediatric diffuse midline glioma: A review of circulating tumor DNA and cerebrospinal fluid tumor DNA. Neurosurg Focus (2020) 48:E9. doi: 10.3171/2019.9.FOCUS19699
141. Martínez-Ricarte F, Mayor R, Martínez-Sáez E, Rubio-Pérez C, Pineda E, Cordero E, et al. Molecular diagnosis of diffuse gliomas through sequencing of cell-free circulating tumor DNA from cerebrospinal fluid. Clin Cancer Res (2018) 24:2812–9. doi: 10.1158/1078-0432.CCR-17-3800
142. Connolly ID, Li Y, Pan W, Johnson E, You L, Vogel H, et al. A pilot study on the use of cerebrospinal fluid cell-free DNA in intramedullary spinal ependymoma. J Neurooncol. (2017) 135:29–36. doi: 10.1007/S11060-017-2557-Y
143. Mattox AK, Yan H, Bettegowda C. The potential of cerebrospinal fluid–based liquid biopsy approaches in CNS tumors. Neuro Oncol (2019) 21:1509. doi: 10.1093/NEUONC/NOZ156
144. Wen PY, Stein A, van den Bent M, De Greve J, Wick A, de Vos FYFL, et al. Dabrafenib plus trametinib in patients with BRAFV600E-mutant low-grade and high-grade glioma (ROAR): a multicentre, open-label, single-arm, phase 2, basket trial. Lancet Oncol (2022) 23:53–64. doi: 10.1016/S1470-2045(21)00578-7/ATTACHMENT/736BD4EB-2418-4DF5-99CC-C280526B6165/MMC1.PDF
145. Hargrave DR, Bouffet E, Tabori U, Broniscer A, Cohen KJ, Hansford JR, et al. Efficacy and safety of dabrafenib in pediatric patients with BRAF V600 mutation–positive relapsed or refractory low-grade glioma: Results from a phase I/IIa study. Clin Cancer Res (2019) 25:7303–11. doi: 10.1158/1078-0432.CCR-19-2177/56400/P/EFFICACY-AND-SAFETY-OF-DABRAFENIB-IN-PEDIATRIC
146. Quinn JA, Jiang SX, Carter J, Reardon DA, Desjardins A, Vredenburgh JJ, et al. Phase II trial of gliadel plus O6-benzylguanine in adults with recurrent glioblastoma multiforme. Clin Cancer Res (2009) 15:1064–8. doi: 10.1158/1078-0432.CCR-08-2130
147. Maggs L, Cattaneo G, Dal AE, Moghaddam AS, Ferrone S. CAR T cell-based immunotherapy for the treatment of glioblastoma. Front Neurosci (2021) 15:662064/BIBTEX. doi: 10.3389/FNINS.2021.662064/BIBTEX
148. Hu J, Liu T, Han B, Tan S, Guo H, Xin Y. Immunotherapy: A potential approach for high-grade spinal cord astrocytomas. Front Immunol (2021) 11:582828/BIBTEX. doi: 10.3389/FIMMU.2020.582828/BIBTEX
149. Majzner RG, Ramakrishna S, Yeom KW, Patel S, Chinnasamy H, Schultz LM, et al. GD2-CAR T cell therapy for H3K27M-mutated diffuse midline gliomas. Nat. (2022) 603:934–41. doi: 10.1038/s41586-022-04489-4
150. Bartanusz V, Jezova D, Alajajian B, Digicaylioglu M. The blood–spinal cord barrier: Morphology and clinical implications. Ann Neurol (2011) 70:194–206. doi: 10.1002/ANA.22421
151. Fletcher SMP, Choi M, Ramesh R, O’Reilly MA. Focused ultrasound-induced blood-spinal cord barrier opening using short-burst phase-keying exposures in rats: A parameter study. Ultrasound Med Biol (2021) 47:1747–60. doi: 10.1016/J.ULTRASMEDBIO.2021.03.007
152. Fletcher SMP, Choi M, Ogrodnik N, O’Reilly MA. A porcine model of transvertebral ultrasound and microbubble-mediated blood-spinal cord barrier opening. Theranostics (2020) 10:7758. doi: 10.7150/THNO.46821
Keywords: intramedullary, astrocytoma, tumor, spinal cord, resection, biomarkers, targeted therapy, genetic
Citation: Hersh AM, Jallo GI and Shimony N (2022) Surgical approaches to intramedullary spinal cord astrocytomas in the age of genomics. Front. Oncol. 12:982089. doi: 10.3389/fonc.2022.982089
Received: 30 June 2022; Accepted: 11 August 2022;
Published: 06 September 2022.
Edited by:
Giuseppe La Rocca, Agostino Gemelli University Polyclinic (IRCCS), ItalyReviewed by:
Kristin Huntoon, University of Texas MD Anderson Cancer Center, United StatesMahmoud Messerer, Centre Hospitalier Universitaire Vaudois (CHUV), Switzerland
Mirza Pojskic, University Hospital of Giessen and Marburg, Germany
Copyright © 2022 Hersh, Jallo and Shimony. This is an open-access article distributed under the terms of the Creative Commons Attribution License (CC BY). The use, distribution or reproduction in other forums is permitted, provided the original author(s) and the copyright owner(s) are credited and that the original publication in this journal is cited, in accordance with accepted academic practice. No use, distribution or reproduction is permitted which does not comply with these terms.
*Correspondence: George I. Jallo, gjallo1@jhu.edu
†ORCID IDs: Andrew M. Hersh, orcid.org/0000-0003-1755-3974
George I. Jallo, orcid.org/0000-0001-9607-6867
Nir Shimony, orcid.org/0000-0002-7930-8782