- 1Genetic Department, Luiz de Queiroz College of Agriculture, University of São Paulo, São Paulo, Brazil
- 2Soil Science Department, Federal University of Ceará, Fortaleza, Brazil
- 3Soil Science Department, Luiz de Queiroz College of Agriculture, University of São Paulo, São Paulo, Brazil
- 4Agricultural Department, Federal University of Amapá, Macapá, Brazil
- 5Laboratory of Microbial Genetic and Biotechnology, Federal University of Agreste of Pernambuco, Garanhuns, Brazil
Burkholderia sp. is a bacterial genus extremely versatile in the environment and has been reported for a great potential to promote plant growth via different mechanisms. Here we evaluate the plant growth-promoting mechanisms in twenty-six Burkholderia strains. Strains were evaluated for their ability to promote plant growth by means of: indole-3-acetic acid (IAA) production under different conditions of pH, salt stress and the presence or absence of L-tryptophan; exopolysaccharides (EPS) production and quorum sensing (ALH). The strains were also characterized in terms of their genetic variability and species identification through Sanger sequencing. Then, the bacteria most responsive in the greatest number of plant-growth promotion mechanisms were selected for a corn seed germination test. All bacteria synthesized IAA in medium with 0.0 or 5.0 mM of L-tryptophan in combination with either 1 or 5% of NaCl, and pH values of either 4.5 or 7.2. The EPS production was confirmed for 61.54% of the bacterial strains. Quorum sensing also occurred in 92.3% of the selected bacteria. The Jaccard similarity coefficient revealed 16 clusters with high genetic variability between bacterial strains. Bacterial strains were assigned to seven species: B. anthina, B. cepacia, B. gladioli, B. ambifaria, B. graminis, B. heleia, and Burkholderia spp. The corn seed bacterization did not affect the germination velocity index (GSI), as well as the first count of germinated seeds (FC). However, inoculations formulated with B. heleia strain G28, B. gladioli strain UAGC723, and B. graminis strain UAGC348 promoted significant increases in root length, seedling height and fresh and dry seedling phytomass, respectively. These results indicate the high biotechnological potential of several strains in the genus Burkholderia sp. as seed inoculants, favoring germination and seedling initial development.
Introduction
Plants provide a wide variety of niches for growth and proliferation for a great diversity of microorganisms, including bacteria, fungi, protists, nematodes, and viruses, which make up the plant microbiota (1, 2). These microorganisms can form complex interactions with plants and have key roles in promoting plant productivity and health (3, 4). The plant-bacteria interaction has been the basis of bioproducts that promote increased productivity of agricultural crops, especially inoculants with mechanisms that promote plant-growth, nutrient acquisition and increases of plant tolerance and resistance to abiotic stresses and pests attack (5–7). Most of these interactions occur in the soil-plant interface using specific niches such as the rhizosphere, endosphere and rhizoplane (1, 2, 8). The rhizosphere consists of the root zone directly influenced by plant exudates, but the microorganisms inhabiting these regions may also interfere in both plant development and soil fertility (5, 9). Moreover, endophytic bacteria (endosphere) occur in all plant species and contribute to plant development by providing microbiological compounds capable to protect the plant, stimulate plant growth and nutrient absorption (4, 10). The root epiphytic bacteria, which are inhabitants of the root surface (rhizoplane) have also a crucial role in stimulating plant development (3, 9).
In particular, the plant growth promoting bacteria (PGPB) have stood out in the field of biotechnology for their role in disease suppression (biological control), increased nutrient availability (biofertilization), phytohormone production (phytostimulation) and increases in resistance to abiotic stresses (8, 11).
Recently, the genus Burkholderia received special attention due to its metabolic versatility and capacity to occupy different niches (12, 13). This genus comprehends more than 88 cataloged bacterial species divided into clusters (14–16). The first grouping includes human, animal, and plant pathogens such as Burkholderia glumae, Burkholderia pseudomallei and Burkholderia mallei, as well as the 17 defined species of the Burkholderia cepacia complex). While the second cluster comprises more than 30 non-pathogenic species, which are associated with plants and therefore can be considered potentially beneficial. This latter Burkholderia cluster is also known as the plant-associated beneficial and environmental (PBE) group (14). Many studies provided evidence of the great biotechnological potential of the Burkholderia PBE group, which includes degradation of aromatic compounds and toxic molecules; plant colonization via growth promoting mechanisms; and establishment of symbiotic relationship with plants, as well as increasing plant resistance against abiotic disturbances (17, 18). In this context, a major challenge for agriculture is to produce food during the present climate change scenario, which predicts an increase in global temperature in the magnitude of 1.5–2.0°C or more, as well as reduced availability and regularity of rainfall (19, 20). Therefore, the present greatest challenge for biotechnology in agriculture is to prospect and develop bioproducts (microbial inoculants) capable of promoting plant growth in scenarios of lack of rainfall and thermal stress, as well as greater incidence of pests and diseases (6, 11). However, different factors should be considered when developing these inoculants, including selection of efficient PGPB based on the target crop, soil type, indigenous microbial community, and environmental factors (8, 15).
This research had the following objectives: (i) select Burkholderia spp. previously isolated from sugarcane plants, according to their plant growth-promoting mechanisms, as production of indole-3-acetic acid (IAA) and/or exopolysaccharides (EPS), or development of quorum sensing (QS) molecules under stress conditions, (ii) evaluate the genetic diversity of Burkholderia strains through methods of molecular biology, and (iii) verify the effectiveness of inoculants based on bacterial isolates which increase germination and initial development of maize plants (Zea mays).
Materials and Methods
Bacterial Isolates
We evaluated a total number of 25 pure bacterial strains belonging to the microorganism collection from the Microbial Genetics and Biotechnology Laboratory, Academic Unit of Garanhuns, Federal Rural University of Pernambuco, Brazil. Bacteria strains were isolated from sugarcane plants cultivated at the Carpina Experimental Station (7° 51' 3” S, 35° 15' 17” W), located at ~184 m above sea level. This station belongs to the Federal Rural University of Pernambuco, and located in the Carpina municipality, Pernambuco state, Brazil. The local climate is As, according to Köppen's classification system, presenting an annual rainfall of 1,350 mm, concentrated between March and October (21). All bacterial isolates were identified by partial sequencing of the 16S rRNA gene. The bacterial strains were removed from glycerol storage (−20 °C freezer) and re-cultured in TSA 10% (Trypticase Soy Agar, Merck, Darmstadt, Germany) culture medium (4.0 g of TSA L−1) and (15.0 g of agar L−1) (22).
Indole-3-Acetic Acid (IAA) Production
The IAA production was evaluated via a colorimetric method based on absorbance in UV-Vis spectrophotometer, as described by Crozier et al. (23). The 25 bacterial strains were tested for their IAA production potential in the presence and absence of L-tryptophan, under different conditions of salt stress (1.0 and 5.0% NaCl) and pH (4.5 and 7.2) in isolation. All analyses were performed in triplicates. Therefore, the bacterial strains were transferred to Petri dishes containing the culture medium Tryptone Soya Agar (TSA) 10%. After 24 h, isolated colonies were transferred again to 10% TSA liquid medium in tubes. Then, 10 μL of inoculum were transferred to tubes containing 10,0 mL of 10% TSA liquid medium containing either zero or 5.0 mM of L-tryptophan (absence or presence of the IAA precursor); sodium chloride (NaCl) in the concentrations of 1 and 5% in both, pH of 4.5 and 7.2. All the tubes were kept under constant shaking (125 rpm) for 24 h, at 28°C and darkroom. We applied two extreme NaCl concentrations and pH values to increase our capacity to find efficient strains with the ability to respond to stressful environmental conditions. The experimental design was a factorial design (2 × 2 × 2), with three replicates, totaling 24 experimental units per bacterium.
In order to quantify the IAA production, 2 mL of the bacterial cultures were centrifuged at 12,000 g for 5 min. The supernatant was treated with Salkowski reagent (2% of FeCl3 0.5 M in 35% perchloric acid), in the proportion of 3:1, and this mixture was incubated in the darkroom to react for 30 min. The samples were evaluated in a spectrophotometer, with the absorbance at 530 nm, and a positive result was confirmed via formation of a pink color. Absorbance readings were converted to IAA concentration using a standard curve.
Exopolysaccharide (EPS) Production
In order to detect EPS production, the bacterial culture (10 μL) was transferred to sterile disks on the surface of agar medium containing 10% sucrose, at pH 7.5, and at a temperature of 28°C for 48 h. At the end, the production of EPS was detected visually via the production of a halo, whose presence characterized the result as positive, and quantified according to the halo's size. To confirm EPS production, the bacteria positive for EPS production were transferred to tubes containing 2 mL of ethanol with a platinum loop. The test was considered positive when the precipitation of EPS could be detected and negative when the tube remained cloudy (22, 24). The experiment was performed in triplicates in a completely randomized design.
Quorum Sensing Molecule Production
The identification of bacterial strains producers of a quorum sensing (QS) molecule (N-Acyl lactone homoserine - ALH) was performed using a biosensing bacterium, also called ALH, i.e., Agrobacterium tumefaciens NTL4 (25). The tests were prepared in bioassays where A. tumefaciens NTL4 was inoculated in a line at the border of a Petri dish containing LB (Lúria Bertani) medium with 10 μg mL−1 of X-gal (5-Bromo-4-Chloro-3-Indolyl-Beta-D-Galacto-Pyranoside) added. The bacterial strains were inoculated perpendicularly to the line of A. tumefaciens NTL4. The discoloration to a blue color of the A. tumefaciens colonies after 48 h of inoculation indicates the ALH production by the selected bacterial strain. This experiment was performed in a completely randomized design in duplicate.
Genetic Diversity of Burkholderia ssp.
The genetic diversity of Burkholderia spp. isolates was verified by the REP-PCR (Repetitive sequence-based PCR) technique. For this, the REP-PCR was prepared in independent reactions containing the primers BOX-1AR, ERIC 1 and ERIC 2 separately for each bacterial strain (26). The reactions containing the primer BOX−1AR (5'-CTACGGCAAGGCGACGCTGACG-3') were prepared for a final volume of 25 μL. They contained 0.5-10 ng of DNA template, 10 μM of primer, 25 mM of each dNTP, 10 mg mL−1 of BSA, 100% of DMSO (dimethyl sulfoxide), 5X Gitschier buffer (1M (NH4)2SO4, 1M Tris-HCL pH 8,8, 1M MgCl2, 0,5M EDTA pH 8,8, 14,4M β-mercaptoethanol), 5U μL−1 of Taq DNA Polymerase (Invitrogen, Carlsbad, CA). The amplification reactions were done in a thermocycler (Applied Biosystems), with thermal conditions of 95°C for 2 min; 35 x 92°C for 30s, 50°C for 1 min, 65°C for 8 min; 68°C for 10 min.
The second PCR reaction used the primer ERIC 1 (5'-ATGTAAGCTCCTGGGGATTC-3') and ERIC 2 (5'–AAGTAAGTGACTGGGGTGAG-3') was performed to a final volume of 25 μL, containing 0,5–10 ng of DNA template, 100 μM of each primer, 2,5 mM of each dNTP, 10X PCR buffer, 50 mM of MgCl2, 5U μL−1 of Taq DNA Polymerase HOT FIREPol (Solis BioDyne). The amplification reactions were performed in a thermocycler (Applied Biosystem) programed with thermal conditions of 94°C for 5 min; 30 × 94°C for 1 min, 52°C for 1 min, 65°C for 8 min; 65°C for 16 min. The PCR products were checked via gel electrophoresis (1.5% agarose gel p/v) in a TAE buffer 1 × (40 mM of Tris-acetate; 1 mM of EDTA) dyed with Gel Red (Biotium, Hayward, CA, USA) and 10X Loading Buffer (Invitrogen). The DNA segments were visualized under ultraviolet light and photo-documented. The bands observed were used to build a similarity dendrogram via Jaccard coefficient using Past software (version 4.07b) (27).
Maize Seeds Inoculation Tests
Maize seeds were used to evaluate the effect of eleven bacterial strains in improving germination attributes. To perform the seed inoculation, the pure cultures of bacterial strains were diluted in PBS buffer to an optical density of 1.0 at 630 nm (~ 108 mL−1 cells) (1, 28). The maize seeds were immersed in this suspension for 30 min under constant shaking (125 rpm). Inoculated seeds were placed in Germitest paper, were watered, rolled up, and stored, at a temperature of 28°C, with a photoperiod of 12 h, for seven days (29). The experiment consisted of a completely randomized design with a control (non-inoculated seeds) and 11 treatments, with three replicates. We sampled 25 seeds per replicate (900 experimental units) (Table 1).
To evaluate the growth promotion for seedling growth, we followed the rules for seed analysis (30) and evaluated the following variables: first counting test (FC) (corresponding to the first counting of germinated seeds), germination velocity index (GVI), epicotyl and radicle length, and both, seedling fresh and dry biomass (g) as proposed by Maguire (29).
Statistical Analyses
The normality of IAA, EPS and corn seed growth promotion data was checked by the Shapiro–Wilk test (P > 0.05). Then, the data were submitted to ANOVA and the significant interactions were tested by the Scott-Knott test at 5% probability level using the PAST software version 4.0 (27). All data combined were evaluated in a biplot of Principal Components Analysis (PCA) based on the correlation matrix between the observations and the variables under study (31). The multivariate analysis was performed using the CANOCO Version 4.5 (32). The Pearson correlation matrices were plotted in the R program Version 4.0 (33).
Results and Discussion
The PCA biplot comprised 75.41% of total data variability in bacterial strains IAA production with or without L-tryptophan addition (0.0 or 5.0 mM), the presence of NaCl (1.0 or 5.0%), and under different pH values (pH 4.5 and pH 7.2). The first principal component (PCO1) explained 57.88% and the second (PCO2) explained 17.53% of the total data variance (Figure 1).
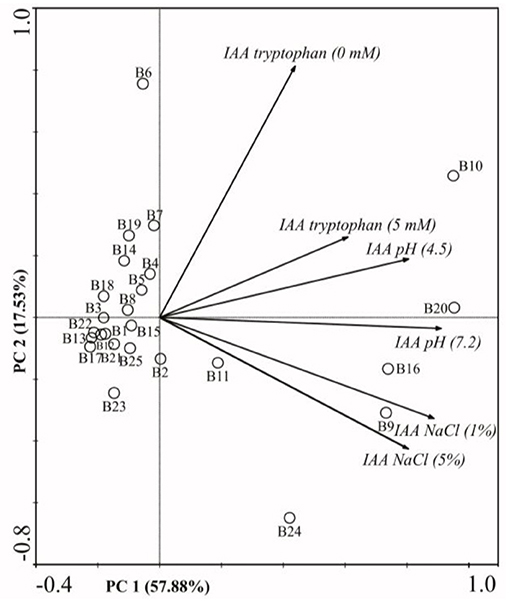
Figure 1. Biplot for two principal components based on the correlation matrix between bacteria from the genus Burkholderia sp. (B1 to B25) and the production of IAA in different L-tryptophan (0 and 5mM) and NaCl (1 and 5%) concentrations, and pH ranges (4.5 and 7.2). Burkholderia anthina (B1: UAGC76, B5: UAGC127, B8: UAGC105, B14: UAGC745), Burkholderia cepacia (B2: UAGC78, B3: UAGC114, B4: UAGC125, B6: UAGC130, B7: UAGC131), Burkholderia graminis (B9: UAGC348), Burkholderia gladioli (B10: UAGC723, B12: UAGC740, B21: G2, B22: G1, B23: G10, B25:G29), Burkholderia sp. (B11: UAGC739, B15: UAGC857, B16: UAGC871, B17: UAGC904, B18: UAGC913, B19: UAGC942, B20: UAGC867), Burkholderia heleia (B24: G28) and Burkholderia ambifaria (B13: UAGC741).
L-tryptophan plays an important role in the IAA biosynthesis, and its presence in the culture medium increases the phytohormone production by the majority of PGPB (34, 35). In the first quadrant, the bacteria strains B10 (B. gladioli) and B20 (Burkholderia sp.) were positively correlated with the presence of L-tryptophan in the medium (Figure 1), producing 226.71 and 205.54 μg of AIA mL−1, respectively, and significantly differing from the other bacterial strains (Table 2).
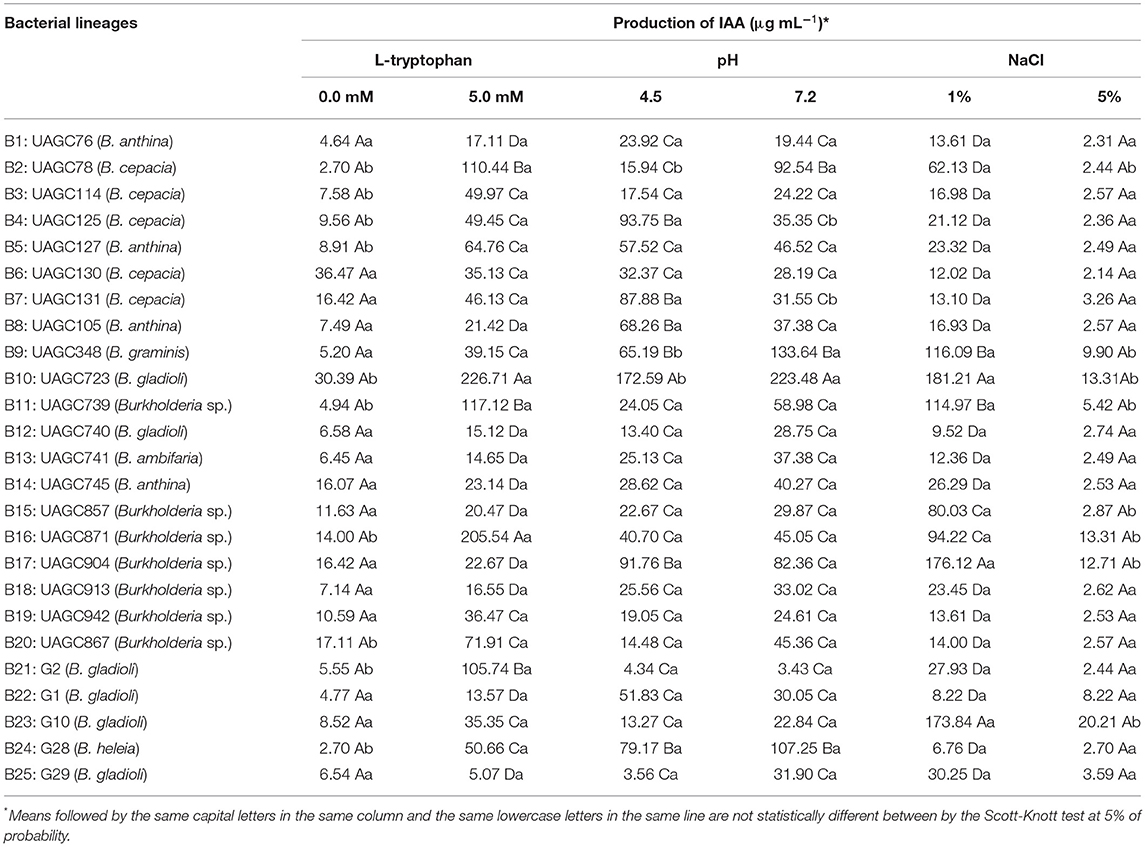
Table 2. Production of indole acetic acid (IAA) in vitro in the absence (0.0 mM) or presence (5.0 mM) of L-tryptophan, pH values 4.5 (acidic) and 7.2 (basic), and NaCl concentration (1.0 and 5.0%) by bacterial lineages belonging to the genus Burkholderia sp.
However, the L-tryptophan eigenvector also positively correlated with the bacteria B. cepacia (B2, B3, and B4), B. gladioli (B10 and B22), B. anthina (B5), B. heleia (B24) and Burkholderia spp. (B19 e B20), suggesting their dependency on the same biological pathway to synthesize this phytohormone in vitro (Figure 1). Several studies showed the microbial potential in synthesizing plant hormones. Liu et al. (34) reported a higher production of IAA in Burkholderia pyrrocinia JK-SH007, which also showed its dependence on tryptophan in the IAA synthesis pathway. These authors also suggest IAA production as one of the main growth mechanisms of the plant for the genus Burkholderia sp.
Very similar results were also reported by Jiang et al. (36), which tested Burkholderia sp. for different PGP mechanisms, with IAA synthesis reaching only 3.8 μg mL−1 in the L-tryptophan dependent pathway. In fact, most members of the genus Burkholderia sp. are within the PBE group (plant-associated beneficial and environmental group) (37). The Burkholderia PBE group have a wide host range and are considered one of the most potent plant growth-promoting rhizobacteria, which are present in both bulk soil and rhizosphere and have been reported to colonize more than 30 plant species (38, 39). The Burkholderia spp. can be found in the rhizosphere of the main agricultural crops, such as corn, rice, wheat, sugarcane, tomatoes, cassava and potatoes (37, 38). This group of plant growth-promoting bacteria acts mainly through IAA production, biological nitrogen fixation (BNF), siderophore production, inorganic phosphate solubilization, and enhances tolerance to abiotic stress, and inhibition of plant pathogens (20, 34).
Related to IAA production, the pH also presented a correlation with the eigenvectors in both acid (pH 4.5) and basic (pH 7.2) medium (Figure 1). The isolate B10 (B. gladioli) showed the highest values of IAA production of all bacteria (172.59 and 223.48 μg mL−1) at pH 4.5 or 7.2, respectively. Although this bacterium produced a little less IAA under acid conditions, this still is a quite high value and this result shows its potential in synthesizing this phytohormone in acid soils, becoming an important PGP agent in environments with adverse conditions a final period. This is an extremely desirable biotechnological feature in bio-inoculants that will be applied in the soil of tropical climate regions (20, 40). The expression of the PGPB biotechnological potential under adverse pH conditions, such as those frequently reported in Brazilian agricultural soils (pH 4.5–6.5) becomes a determining factor in the establishment of the biological agent, that makes up the bio-inoculant (19, 40). When translating into the bio-product effectiveness in field conditions, those attributes are also fundamental (8, 40).
The PCA biplot also revealed a positive correlation between the bacterial strains B4 and B7 (both Burkholderia sp.) with the acid medium (pH 4.5), which confirmed their ability for IAA production under these conditions, quantified as 93.75 and 87.88 μg of IAA mL−1, respectively. In contrast, the bacteria B2 (B. cepacia), B9 (B. graminis) and B10 (B. gladioli) (also known as root endophytes) showed a positive correlation with pH 7.2 (Figure 1). These bacteria synthesized the highest amount of IAA in this pH condition (92.54, 133.64 and 223.48 μg mL−1), which evidenced the influence of the medium pH on the IAA synthesis in vitro (Table 2). This demonstrates a great variability of Burkholderia species in their affinity with either high or low pH values, besides species with abilities for both. In this context, Jasim et al. (41) tested the capacity Burkholderia sp. to synthesize IAA under different adverse conditions and observed achieved the highest production (71.66 μg mL−1) in a medium with low pH (4.0) instead of high pH (7.0). Pereira and Castro (42) suggested that, given the proximity of endophytic bacteria with their plant host, they have the advantage of more plant protection and face less environmental restrictions. Therefore, when compared to other bacteria that occupy different habitats, like the rhizoplane and rhizosphere, the endophytic bacteria may be more sensitive to changes in environmental conditions and abiotic stress (13, 34).
The eigenvectors referring to the NaCl addition (1 and 5%) related with each other in the PCA biplot, as revealed by their proximity and small angle between them (Figure 1). The bacteria positively related with these two variables were influenced strongly by the salt concentration in the medium. We observed a strong correlation between the eigenvector NaCl 1.0% and the bacterial strains B10 (B. gladioli), B16 (Burkholderia sp), and B24 (B. heleia). They also presented statistically similar IAA production under this condition. However, when the salt concentration increased, we observed an exponential reduction of IAA biosynthesis.
Pereira et al. (43) reported highly decreased IAA production under saline conditions (2.5 to 10% NaCl), not even reaching 30 μg mL−1. However, Rojas-Tapías et al. (44) found one bacterium (strain C5) capable to synthesize IAA only under salinity conditions. It is already known that IAA production by PGPB contributes to plant development, but as shown in our experiment, adverse environmental conditions may interfere in this production.
The rainfall scarcity will intensify due to the global climate changes, which can promote salt accumulation in the soil (40), especially in semi-arid regions of Northeastern Brazil (45). This will become a huge challenge to be overcome (20, 46, 47). In this context, the bacterial isolates with high potential to express biosynthesis pathways of phytohormones and other plant-stimulating compounds under salt stress conditions can become important allies of agriculture (8, 20), enabling the production of corn in these areas. Our research highlights potential bacterial candidates for the formulation of a bioproduct based on their capacity to produce IAA (B10 >> B17 >> B9 >> B11), when considering a 1% salt stress condition. For higher salt concentrations a new selection would have to be made.
EPS production was characterized as positive in 62% of Burkholderia spp. strains, with 38.46% of the strains showing high EPS production capacity (halo > 14 mm). B. graminis (B9) and B. gladioli (B25 and B22) produced significantly more EPS than all other bacteria with a measured halo of 45.85, 52.77, and 54.07 mm, respectively (Table 3 and Supplementary Figure S1). The high capacity of bacteria to synthesize EPS is related to stressful environmental conditions (47, 48). Under unfavorable environmental conditions, EPS production acts as a protective mechanism for bacteria, helping them to maintain the microbial community (49). In addition, EPS favors the plant colonization by bacteria which increases their tolerance to salt stress and drought (50). da Silva et al. (51) isolated and tested bacteria tolerant to salinity stress and observed a high production of EPS, reporting halos varying from 7.46 to 60.33 mm. The EPS production is an important mechanism that helps in plant colonization by PGPB and increases the tolerance of plants to environmental stresses (48). Therefore, EPS production is a desired biotechnological characteristic in microbial strains that make up the bio-products used in sustainable agriculture (47). The bacteria that presented a high EPS production capacity have an advantage over the soil indigenous bacterial community (52). Bacterial strains form dense biofilms that protect them against stressful environmental conditions such as salt stress, acidification, or drought (46, 53). Furthermore, biofilms enhance the ability of bacterial colonization (47, 48), which in the case of a plant growth-promoting bacterium is something very desirable (22, 52).
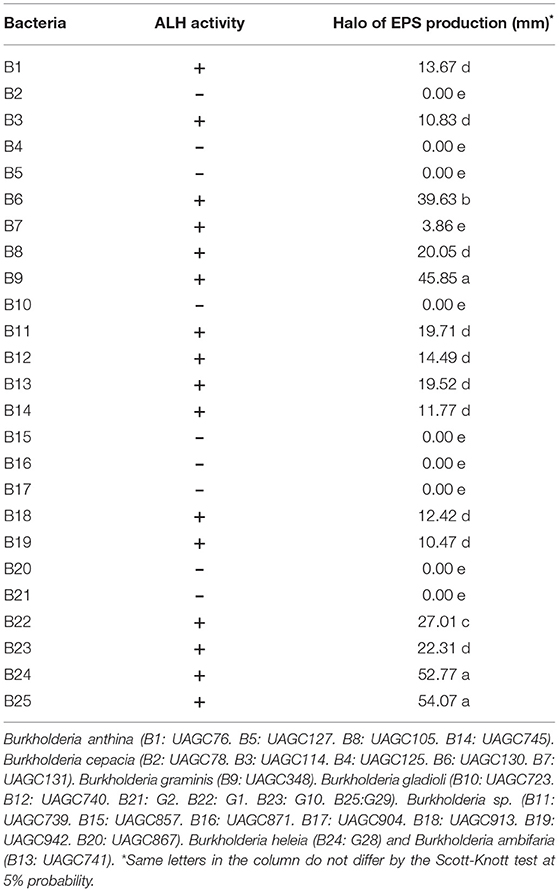
Table 3. ALH activity and Halo of production EPS (mm) at 48 hours after inoculation by bacteria from the genus Burkholderia sp.
The production of ALH (N-acyl lactone homoserine) was detected in 92.3% of our selected bacteria from the genus Burkholderia sp. (Table 3 and Supplementary Figure S2). Evaluating the QS production in halotolerant bacteria associated with sugarcane, Leite et al. (54) observed a smaller proportion of bacteria producing QS. From a total of 102 bacteria tested, only 49% presented positive production. Poonguzhali et al. (55) highlight the importance of understanding the QS production in bacteria from the genus Burkholderia sp., given their versatility in occupying diverse niches, their relevance in controlling plant pathogens and their capability of colonizing plants.
The QS biosynthesis detected by ALH activity is an important mechanism present in bacteria (56), acting in chemical signaling between bacteria-bacteria and bacteria-plants (18, 57). The QS molecules regulate several processes, including symbioses, virulence, competence, conjugation, antibiotic production, motility, sporulation, and biofilm formation. In general, Gram-negative bacteria use ALH as autoinducers, and Gram-positive bacteria use processed oligopeptides to communicate (18). For Burkholderia spp. ALH activity is an essential mechanism that regulates their capacity and versatility to colonize several niches, such as the endosphere, phyllosphere and rhizosphere (12, 58, 59) through chemical signaling between bacteria-plant (Quorum sensing mechanism). For plants, the ALH activity can promote the increase of primary root elongation and growth rate. These effects were mainly attributed to changes in auxin levels (56, 60).
The Jaccard similarity coefficient revealed the presence of 16 clusters with high genetic variability at 30% similarity. The bacteria grouped in clusters 1 and 2 suggested that those bacteria occupy different niches and originated from distinct sugarcane varieties, they share similarities. However, the clusters 3 and 12 grouped together bacteria isolated from the same niche and sugarcane variety (Figure 2).
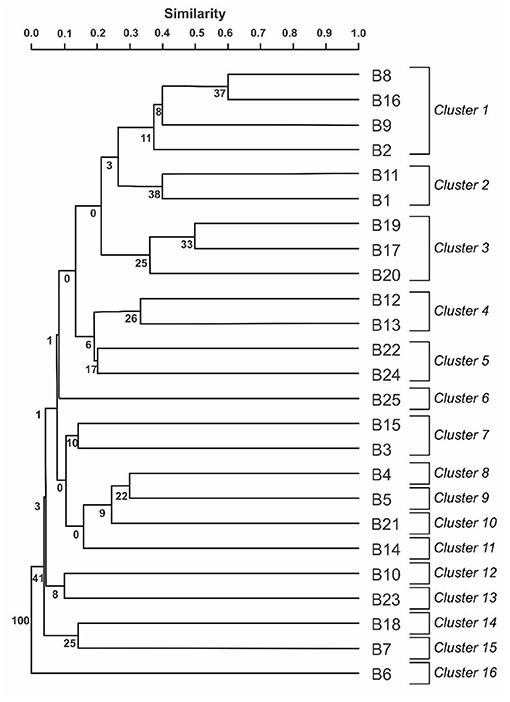
Figure 2. Dendrogram obtained by groupings performed by UPGMA algorithm (unweighted Pair-Group Method with arithmetical Average) from the genetic similarity matrix of bacteria from the genus Burkholderia. The numbers of nodes in the dendrogram indicate the value of the percentage of times that the group occurred on the same node during the 10,000 repetitions Bootstrap. Burkholderia anthina (B1: UAGC76, B5: UAGC127, B8: UAGC105, B14: UAGC745), Burkholderia cepacia (B2: UAGC78, B3: UAGC114, B4: UAGC125, B6: UAGC130, B7: UAGC131), Burkholderia graminis (B9: UAGC348), Burkholderia gladioli (B10: UAGC723, B12: UAGC740, B21: G2, B22: G1, B23: G10, B25:G29), Burkholderia sp. (B11: UAGC739, B15: UAGC857, B16: UAGC871, B17: UAGC904, B18: UAGC913, B19: UAGC942, B20: UAGC867), Burkholderia heleia (B24: G28) and Burkholderia ambifaria (B13: UAGC741).
Our results differed from the findings of Cordero et al. (61), which found only four clusters at 30% similarity when used the REP-PCR to study the genetic diversity of their microorganisms, suggesting a low genetic diversity in maize rhizosphere, endosphere and bulk soil in Argentina. Munday et al. (62) highlight the efficiency of REP-PCR in detecting small changes in bacterial genomes, being capable to differentiate between different strains within the same species via amplification of specific DNA sizes and regions of each bacterial strain.
Bacterial inoculation did not increase the germination velocity index (GVI) in the first count of germinated seeds (FC). However, we report a higher effect of bacteria inoculation in the other investigated variables (radicle length, epicotyl length, total biomass, fresh mass, and total dry biomass) in relation to the control treatment (Table 4). This result is contrary to that reported by Kang et al. (63), who reported significant increases in seed germination and plant growth of lettuce and Chinese cabbage inoculated with bacteria of the genus Burkholderia sp. strain KCTC 11096.
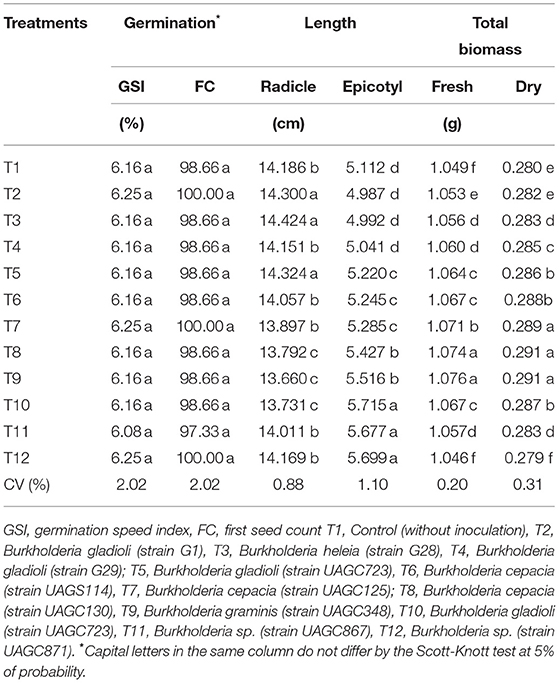
Table 4. Early germination and seedling development characteristics after crossed inoculation of bacteria promoting plant growth. the genus Burkholderia sp. in maize seeds (Zea mays).
The PCA biplot comprised 81.48% of total data variability in the bacterial strains, data according to the FC, IVG, epicotyl and radicle length, seedling fresh and dry biomass. The first principal component (PCA1) explained 49.73% and the second principal component (PCA2) explained 31.75% of the total data variability (Figure 3).
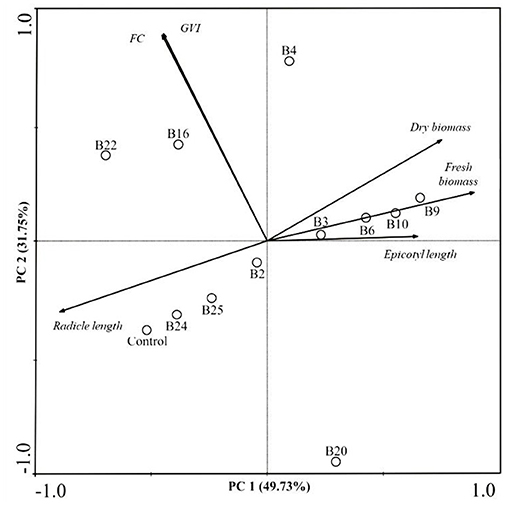
Figure 3. Biplot for two principal components based on the correlation matrix between bacteria from the genus Burkholderia (B2, B3, B4, B6, B9, B10, B16, B20, B22, B24, and B25) and germination variables evaluated in maize seeds. FC, First count test; GVI, germination velocity index; Control (without bacterial inoculation); Burkholderia cepacia (B2: UAGC78, B3: UAGC114, B4: UAGC125 and B6: UAGC130); Burkholderia graminis (B9: UAGC348); Burkholderia gladioli (B10: UAGC723, B22: G1 and B25:G29); Burkholderia sp. (B16: UAGC871 and B20: UAGC867) and Burkholderia heleia (B24: G28).
We report a positive correlation between the bacteria B2 (B. cepacia), B24 (B. heleia), and B25 (B. gladioli) with the radicle length (Figure 3). These treatments corresponded to the higher values in the radicle length 14.3, 14.42, and 14.32 cm, respectively. Also, we observed an increase in radicle length (+1.67%), shoot length (+11.79%), and total growth (+1.38%) when compared with the control treatment (no bacterial inoculation) (Table 4).
The first PCA quadrant revealed a positive correlation between the bacteria B. cepacia (B3, B4 and B6), B. graminis (B9), and B. gladioli (B10) with the variables corresponding to epicotyl length, and seedling fresh and dry biomass, which suggests an active contribution of these bacteria in the plant growth promotion (Figure 3). A similar outcome occurred in other analyses where the treatments B6 (B. cepacia) and B9 (B. graminis) resulted in the highest values of the total seedling fresh biomass (1.074 and 1.076 g, respectively). Therefore, the treatments with B. cepacia (B4 and B6), and B. graminis (B9) resulted in the highest values of total plant dry biomass (0.289–0.291 g) (Table 4).
The bacteria capable to promote increases in plant variables also presented high production of IAA, even under different abiotic conditions, reaching values of 226.71 μg mL−1. Santos et al. (64) also reported promising results when he tested bacteria from different genera in promoting plant growth and detected that all bacteria were capable to produce IAA, but the highest production reached 25.0 μg mL−1. When those microbes were inoculated in cucumber seeds, the bacteria of the genus Burkholderia sp. promoted an increase of 62.4% in root growth (64).
Likewise, Cappellari et al. (65), studied two plant growth promoting bacteria in the seeds of Tagetes minuta and reported an increase of 70% in plant size, seedling fresh and dry biomass. On the other hand, Zafar et al. (66), when inoculating PGPB induced an increase of 65% in shoot growth and 74% in root length, when compared to the control (seedlings with no inoculation). Naveed et al. (59) also found promising results when he inoculated bacteria of the genera Burkholderia and Enterobacter in maize seeds, detecting an increase of shoot biomass, leaf area, and chlorophyll content.
Conclusions
Our results showed that the genus Burkholderia is able to synthesize IAA under different abiotic conditions. Specifically, the B10 bacterium (Burkholderia ambifaria strain UGAC723) stood out because it produced a higher amount of IAA, regardless of the absence or presence of L-tryptophan, pH or NaCl concentration in the culture medium. Most of the bacteria studied showed positive response for EPS and quorum sensing molecule production. Strains of Burkholderia sp. also showed a high potential for colonization/bacterization during the germination of corn seeds promoting increases in root length, shoot length, total fresh and dry biomass of the corn seedling. The bacteria B6 (Burkholderia cepacia strain UAGC130) and B9 (Burkholderia graminis strain UAGC348) are potential candidates for the formulation of a bio-inoculant. Furthermore, the versatility of the genus Burkholderia sp. and its ability to colonize plants and express plant growth promotion mechanisms under extreme environmental conditions of salinity make this bacterium an important biotechnological tool for agricultural use in semiarid regions, such as the northeast of Brazil.
Data Availability Statement
The original contributions presented in the study are included in the article/Supplementary Material, further inquiries can be directed to the corresponding author.
Author Contributions
IS contributed to the selection, characterization of Burkholderia ssp. strains, and setup of the trials. AP and AS contributed to data analysis, writing, and review of the manuscript. FS and JO contributed with the germination tests and genetic characterization of the bacteria. EC, MV, and JS contributed to the research conception, writing, and review of the manuscript. All authors contributed to the article and approved the submitted version.
Conflict of Interest
The authors declare that the research was conducted in the absence of any commercial or financial relationships that could be construed as a potential conflict of interest.
Publisher's Note
All claims expressed in this article are solely those of the authors and do not necessarily represent those of their affiliated organizations, or those of the publisher, the editors and the reviewers. Any product that may be evaluated in this article, or claim that may be made by its manufacturer, is not guaranteed or endorsed by the publisher.
Acknowledgments
We would like to thank to the Coordination for the Improvement of Higher Education Personnel (CAPES, Brazil, Finance Code 001) for granting scholarship to the first and third authors.
Supplementary Material
The Supplementary Material for this article can be found online at: https://www.frontiersin.org/articles/10.3389/fsoil.2021.805094/full#supplementary-material
References
1. Trivedi P, Leach JE, Tringe SG, Sa T, Singh BK. Plant–microbiome interactions: from community assembly to plant health. Nat Rev Microbiol. (2020) 18:607–21. doi: 10.1038/s41579-020-0412-1
2. de Andrade PA, de Souza AJ, Lira SP, Assis MA, Berlinck RG, Andreote FD. The bacterial and fungal communities associated with Anthurium ssp. leaves: insights into plant endemism and microbe association. Microbiol. Res. (2021) 244:126667. doi: 10.1016/j.micres.2020.126667
3. Andreote FD, Gumiere T, Durrer A. Exploring interactions of plant microbiomes. Sci Agric. (2014) 71:528–39. doi: 10.1590/0103-9016-2014-0195
4. Taulé C, Vaz-Jauri P, Battistoni F. Insights into the early stages of plant–endophytic bacteria interaction. World J Microbiol Biotechnol. (2021) 37:1–9. doi: 10.1007/s11274-020-02966-4
5. Vishwakarma K, Kumar N, Shandilya C, Mohapatra S, Bhayana S, Varma A. Revisiting plant–microbe interactions and microbial consortia application for enhancing sustainable agriculture: a review. Front Microbiol. (2020) 0:3195. doi: 10.3389/fmicb.2020.560406
6. Sabkia MH, Ongb PY, Ibrahimc N, Leea CT, Klemešd JJ, Lie C, et al. A review on abiotic stress tolerance and plant growth metabolite framework by plant growth-promoting bacteria for sustainable agriculture. Chem. Eng. Trans. (2021) 83:367–72. doi: 10.3303/CET2183062
7. Xiong C, Singh BK, He J-Z, Han Y-L, Li P-P, Wan L-H, et al. Plant developmental stage drives the differentiation in ecological role of the maize microbiome. Microbiome. (2021) 9:1–15. doi: 10.1186/s40168-021-01118-6
8. Pereira APA, de Souza AJ, de Chaves MG, Fracetto GGM, Garcia KGV, Filho PFM, et al. Mechanisms of the phytomicrobiome for enhancing soil fertility and health new and future developments. In: Microbial Biotechnology and Bioengineering. (2021). p. 1–14. doi: 10.1016/B978-0-444-64325-4.00001-8
9. Li E, de Jonge R, Liu C, Jiang H, Friman V-P, Pieterse CMJ, et al. Rapid evolution of bacterial mutualism in the plant rhizosphere. Nat Commun. (2021) 12:1–13. doi: 10.1038/s41467-021-24005-y
10. Chandra P, Wunnava A, Verma P, Chandra A, Sharma RK. Strategies to mitigate the adverse effect of drought stress on crop plants—influences of soil bacteria: a review. Pedosphere. (2021) 31:496–509. doi: 10.1016/S1002-0160(20)60092-3
11. Abdelaal K, Alkahtani M, Attia K, Hafez Y, Király L, Künstler A. The role of plant growth-promoting bacteria in alleviating the adverse effects of drought on plants. Biology. (2021) 10:590. doi: 10.3390/biology10060520
12. Kong P, Hong C. Endophytic Burkholderia sp. SSG as a potential biofertilizer promoting boxwood growth. PeerJ. (2020) 8:e9547. doi: 10.7717/peerj.9547
13. Hwang H-H, Chien P-R, Huang F-C, Hung S-H, Kuo C-H, Deng W-L, et al. (2021). A Plant Endophytic Bacterium, Burkholderia seminalis Strain 869T2, Promotes Plant Growth in Arabidopsis, Pak Choi, Chinese Amaranth, Lettuces, and Other Vegetables. Microorganisms. (2021) 9:1703–1703. doi: 10.3390/microorganisms9081703
14. Suárez-Moreno ZR, Caballero-Mellado J, Coutinho BG, Mendonça-Previato L, James EK, Venturi V. Common Features of Environmental and Potentially Beneficial Plant-Associated Burkholderia. Microb Ecol. (2012) 63:249–66. doi: 10.1007/s00248-011-9929-1
15. Afzal I, Shinwari ZK, Sikandar S, Shahzad S. Plant beneficial endophytic bacteria: Mechanisms, diversity, host range and genetic determinants. Microbiol Res. (2019) 221:36–49. doi: 10.1016/j.micres.2019.02.001
16. Lobo CB, Juárez Tomás MS, Viruel E, Ferrero MA, Lucca ME. Development of low-cost formulations of plant growth-promoting bacteria to be used as inoculants in beneficial agricultural technologies. Microbiol Res. (2019) 219:12–25. doi: 10.1016/j.micres.2018.10.012
17. Talbi C, Delgado MJ, Girard L, Ramírez-Trujillo A, Caballero-Mellado J, Bedmar EJ. Burkholderia phymatum Strains Capable of Nodulating Phaseolus vulgaris Are Present in Moroccan Soils. Appl Environ Microbiol. (2010) 76:4587. doi: 10.1128/AEM.02886-09
18. Hartmann A, Klink S, Rothballer M. Plant Growth Promotion and Induction of Systemic Tolerance to Drought and Salt Stress of Plants by Quorum Sensing Auto-Inducers of the N-acyl-homoserine Lactone Type: Recent Developments. Front Plant Sci. (2021) 0:1026. doi: 10.3389/fpls.2021.683546
19. Jansson JK, Hofmockel KS. Soil microbiomes and climate change. Nat Rev Microbiol. (2019) 18:35–46. doi: 10.1038/s41579-019-0265-7
20. Sangiorgio D, Cellini A, Donati I, Pastore C, Onofrietti C, Spinelli F. Facing climate change: application of microbial biostimulants to mitigate stress in horticultural Crops Agronomy. (2020) 10:794. doi: 10.3390/agronomy10060794
21. Alvares CA, Stape JL, Sentelhas PC, De Moraes Gonçalves JL, Sparovek G. Köppen's climate classification map for Brazil. Meteorol Zeitschrift. (2013) 22:711–28. doi: 10.1127/0941-2948/2013/0507
22. Leite MC, Pereira AP, Souza AJ, Andreote FD, Freire FJ, Sobral JK. Bioprospection and genetic diversity of endophytic bacteria associated with cassava plant. Revista Caatinga. (2018) 31:315–325. doi: 10.1590/1983-21252018v31n207rc
23. Crozier A, Arruda P, Jasmim JM, Monteiro AM, Sandberg G. Analysis of indole-3-acetic acid and related indoles in culture medium from Azospirillum lipoferum and Azospirillum brasilense. Appl Environ Microbiol. (1988) 54:2833–7. doi: 10.1128/aem.54.11.2833-2837.1988
24. Kavamura VN, Santos SN, da Silva JL, Parma MM, Ávila LA, Visconti A, et al. Screening of Brazilian cacti rhizobacteria for plant growth promotion under drought. Microbiol Res. (2013) 168:183–91. doi: 10.1016/j.micres.2012.12.002
25. Quecine MC, Araújo WL, Rossetto PB, Ferreira A, Tsui S, Lacava PT, et al. Sugarcane Growth Promotion by the Endophytic Bacterium Pantoea agglomerans 3.31. Appl Environ Microbiol. (2012) 78:7511–8. doi: 10.1128/AEM.00836-12
26. Pasanen T, Koskela S, Mero S, Tarkka E, Tissari P, Vaara M, et al. Rapid molecular characterization of Acinetobacter baumannii clones with rep-PCR and evaluation of carbapenemase genes by new multiplex PCR in Hospital District of Helsinki and Uusimaa. PloS one. (2014) 9:e85854. doi: 10.1371/JOURNAL.PONE.0085854
27. Hammer DAT, Ryan PD, Hammer Ø, Harper DAT, Ryan PD. Past: paleontological statistics software package for education and data analysis. Palaeontol Electr. (2001) 4:9.
28. Pankievicz VCS, Amaral FP, Santos KFDN, Agtuca B, Xu Y, Schueller MJ, et al. Robust biological nitrogen fixation in a model grass–bacterial association. Plant J. (2015) 81:907–19. doi: 10.1111/tpj.12777
29. Maguire JD. Speed of germination—aid in selection and evaluation for seedling emergence and vigor1. Crop Sci. (1962) 2:176–7. doi: 10.2135/cropsci1962.0011183X000200020033x
30. BRASIL (2021). Regras para análise de sementes. Brasília: MAPA/ACS- Ministério da Agricultura, Pecuária e Abastecimento / Secretaria de Defesa Agropecuária. Available online at: https://www.gov.br/agricultura/pt-br/assuntos/laboratorios/arquivos-publicacoes-laboratorio/regras-para-analise-de-sementes.pdf/view. (accessed September 11, 2021).
31. Buttigieg PL, Ramette A. A guide to statistical analysis in microbial ecology: a community-focused, living review of multivariate data analyses. FEMS Microbiol Ecol. (2014) 90:543–50. doi: 10.1111/1574-6941.12437
32. Lepš J, Šmilauer P. Multivariate analysis of ecological data using CANOCO. In: Multivariate Analysis of Ecological Data using CANOCO. (2003). doi: 10.1017/CBO9780511615146
33. R Core Team (2021). R: A language and environment for statistical computing. Available online at: https://www.R-project.org/. (accessed September 12, 2021).
34. Liu W-H, Chen F-F, Wang C-E, Fu H-H, Fang X-Q, Ye J-R, et al. Indole-3-acetic acid in burkholderia pyrrocinia JK-SH007: enzymatic identification of the indole-3-acetamide synthesis pathway. Front Microbiol. (2019) 10:2559. doi: 10.3389/fmicb.2019.02559
35. Hassen AI, Khambani LS, Swanevelder ZH, Mtsweni NP, Bopape FL, van Vuuren A, et al. Elucidating key plant growth promoting (PGPR) traits in Burkholderia sp. Nafp2/4-1b (=SARCC-3049) using gnotobiotic assays and whole genome sequence analysis. Lett Appl Microbiol. (2021) 73:658–71. doi: 10.1111/lam.13556
36. Jiang C, Sheng X, Qian M, Wang Q. Isolation and characterization of a heavy metal-resistant Burkholderia sp. from heavy metal-contaminated paddy field soil and its potential in promoting plant growth and heavy metal accumulation in metal-polluted soil. Chemosphere. (2008) 72:157–64. doi: 10.1016/j.chemosphere.2008.02.006
37. Ghosh R, Mandal NC. Use of plant growth–promoting burkholderia species with rock phosphate–solubilizing potential toward crop improvement. In: Microbial Services in Restoration Ecology. (2020). p. 139–156. doi: 10.1016/B978-0-12-819978-7.00010-5
38. Mannaa M, Park I, Seo Y-S. Genomic features and insights into the taxonomy, virulence, and benevolence of plant-associated burkholderia species. Int J Molec Sci. (2019) 20:121. doi: 10.3390/ijms20010121
39. Elshafie HS, Camele I. An overview of metabolic activity, beneficial and pathogenic aspects of burkholderia spp. Metabolites. (2021) 11:321. doi: 10.3390/metabo11050321
40. del Buono D. Can biostimulants be used to mitigate the effect of anthropogenic climate change on agriculture? It is time to respond. Sci Total Environ. (2021) 751:141763. doi: 10.1016/j.scitotenv.2020.141763
41. Jasim B, Jimtha John C, Shimil V, Jyothis M, Radhakrishnan EK. Studies on the factors modulating indole-3-acetic acid production in endophytic bacterial isolates from Piper nigrum and molecular analysis of ipdc gene. J Appl Microbiol. (2014) 117:786–99. doi: 10.1111/jam.12569
42. Pereira SIA and Castro PML. Phosphate-solubilizing rhizobacteria enhance Zea mays growth in agricultural P-deficient soils. Ecol Eng. (2014) 73:526–35. doi: 10.1016/J.ECOLENG.2014.09.060
43. Pereira AD, Silva MD, Oliveira JD, Ramos AD, Freire MB, Freire FJ, et al. Salinity influence on the growth and production of indole acetic acid by endophytic Burkholderia spp. from sugarcane. Biosci J. (2012) 28:112–21.
44. Rojas-Tapias D, Moreno-Galván A, Pardo-Díaz S, Obando M, Rivera D, Bonilla R. Effect of inoculation with plant growth-promoting bacteria (PGPB) on amelioration of saline stress in maize (Zea mays). Appl Soil Ecol. (2012) 61:264–72. doi: 10.1016/j.apsoil.2012.01.006
45. de Lima DRM, dos Santos IB, Oliveira JTC, da Costa DP, de Queiroz JVJ, Romagnoli EM, et al. Genetic diversity of N-fixing and plant growth-promoting bacterial community in different sugarcane genotypes, association habitat and phenological phase of the crop. Arch Microbiol. (2020) 203:1089–105. doi: 10.1007/s00203-020-02103-7
46. Kumar A, Singh S, Gaurav AK, Srivastava S, Verma JP. Plant growth-promoting bacteria: biological tools for the mitigation of salinity stress in plants. Front Microbiol. (2020) 11:1216. doi: 10.3389/fmicb.2020.01216
47. Morcillo RJL, Manzanera M. The effects of plant-associated bacterial exopolysaccharides on plant abiotic stress tolerance. Metabolites. (2021) 11:337. doi: 10.3390/metabo11060337
48. Naseem H, Ahsan M, Shahid MA, Khan N. Exopolysaccharides producing rhizobacteria and their role in plant growth and drought tolerance. J Basic Microbiol. (2018) 58:1009–22. doi: 10.1002/jobm.201800309
49. Chouhan GK, Verma JP, Jaiswal DK, Mukherjee A, Singh S, de Araujo Pereira AP, et al. Phytomicrobiome for promoting sustainable agriculture and food security: opportunities, challenges, and solutions. Microbiol Res. (2021) 248:126763. doi: 10.1016/j.micres.2021.126763
50. Qurashi AW, Sabri AN. Bacterial exopolysaccharide and biofilm formation stimulate chickpea growth and soil aggregation under salt stress. Braz J Microbiol. (2012) 43:1183–91. doi: 10.1590/S1517-83822012000300046
51. da Silva FG, dos Santos IB, dos Souza AJ, Farias ARB, da Silva Diniz MBG., Kuklinsky Sobral J, et al. Bioprospecting and plant growth-promoting bacteria tolerant to salinity associated with Atriplex nummularia L. in saline soils. Afric J Microbiol Res. (2016) 10:1203–14. doi: 10.5897/AJMR2016.8202
52. Ghosh D, Gupta A, Mohapatra S. A comparative analysis of exopolysaccharide and phytohormone secretions by four drought-tolerant rhizobacterial strains and their impact on osmotic-stress mitigation in Arabidopsis thaliana. World J Microbiol Biotechnol. (2019) 35:1–15. doi: 10.1007/s11274-019-2659-0
53. Shultana R, Zuan ATK, Yusop MR, Saud HM. Characterization of salt-tolerant plant growth-promoting rhizobacteria and the effect on growth and yield of saline-affected rice. PLoS ONE. (2020) 15:e0238537. doi: 10.1371/journal.pone.0238537
54. Leite MCBS, Farias ARB, Freire FJ, Andreote FD, Kuklinsky-Sobral J, Freire MBGS. Isolation, bioprospecting and diversity of salt-tolerant bacteria associated with sugarcane in soils of Pernambuco, Brazil. Rev Bras de Eng Agricola e Ambient. (2014) 18:73–9. doi: 10.1590/18071929/AGRIAMBI.V18NSUPPS73-S79
55. Poonguzhali S, Madhaiyan M, Sa T. Quorum-sensing signals produced by plant-growth promoting Burkholderia strains under in vitro and in planta conditions. Res Microbiol. (2007) 158:287–94. doi: 10.1016/j.resmic.2006.11.013
56. Shrestha A, Grimm M, Ojiro I, Krumwiede J, Schikora A. Impact of quorum sensing molecules on plant growth and immune system. Front Microbiol. (2020) 0:1545. doi: 10.3389/fmicb.2020.01545
57. Miller MB, Bassler BL. Quorum sensing in bacteria. Annu Rev Microbiol. (2001) 55:165–99. doi: 10.1146/annurev.micro.55.1.165
58. Coutinho BG, Mitter B, Talbi C, Sessitsch A, Bedmar EJ, Halliday N, et al. Regulon studies and in planta role of the braI/R quorum-sensing system in the plant-beneficial burkholderia cluster. Appl Environ Microbiol. (2013) 79:4421–32. doi: 10.1128/AEM.00635-13
59. Naveed M, Mitter B, Reichenauer TG, Wieczorek K, Sessitsch A. Increased drought stress resilience of maize through endophytic colonization by Burkholderia phytofirmans PsJN and Enterobacter sp. FD17. Environ Exper Botany. (2014) 97:30–9. doi: 10.1016/j.envexpbot.2013.09.014
60. Oliveira JTC, Pereira APA, Souza AJ, Silva GT, Diniz WPS, Figueredo EF, et al. Plant growth-promoting mechanisms and genetic diversity of bacteria strains isolated from brachiaria humidicola and brachiaria decumbens. An Acad Bras Cienc. (2021) 93:e20191123. doi: 10.1590/0001-3765202120191123
61. Cordero P, Cavigliasso A, Príncipe A, Godino A, Jofré E, Mori G, et al. Genetic diversity and antifungal activity of native Pseudomonas isolated from maize plants grown in a central region of Argentina. Syst Appl Microbiol. (2012) 35:342–51. doi: 10.1016/J.SYAPM.2012.04.005
62. Munday CI, O'Loingsigh T, Tapper NJ, de Deckker P, Allison GE. Utilisation of Rep-PCR to track microbes in aerosols collected adjacent to their source, a saline lake in Victoria, Australia. Sci Total Environ. (2013) 450:317–25. doi: 10.1016/J.SCITOTENV.2013.02.028
63. Kang S-M, Khan AL, Hussain J, Ali L, Kamran M, Waqas M, et al. Rhizonin A from Burkholderia sp. KCTC11096 and its growth promoting role in lettuce seed. Germin Molec. (2012) 17:7980–8. doi: 10.3390/molecules17077980
64. Santos AF, Martins CYS, Santos PO, Corrêa ÉB, Barbosa HR, Sandoval APS, et al. Diazotrophic bacteria associated with sisal (Agave sisalana Perrine ex Engelm): potential for plant growth promotion. Plant and Soil. (2014) 385:37–48. doi: 10.1007/s11104-014-2202-x
65. Cappellari LR, Santoro MV, Nievas F, Giordano W, Banchio E. Increase of secondary metabolite content in marigold by inoculation with plant growth-promoting rhizobacteria. Appl Soil Ecol. (2013) 70:16–22. doi: 10.1016/J.APSOIL.2013.04.001
Keywords: indole acetic acid, exopolysaccharide, quorum sensing, initial development, promotion mechanisms, Zea mays
Citation: dos Santos IB, Pereira APdA, de Souza AJ, Cardoso EJBN, da Silva FG, Oliveira JTC, Verdi MCQ and Sobral JK (2022) Selection and Characterization of Burkholderia spp. for Their Plant-Growth Promoting Effects and Influence on Maize Seed Germination. Front. Soil Sci. 1:805094. doi: 10.3389/fsoil.2021.805094
Received: 29 October 2021; Accepted: 17 December 2021;
Published: 12 January 2022.
Edited by:
Jacobo Arango, International Center for Tropical Agriculture (CIAT), ColombiaReviewed by:
Dan Naylor, University of California, Berkeley, United StatesSilvia Maribel Contreras Ramos, CONACYT Centro de Investigación y Asistencia en Tecnología y Diseño del Estado de Jalisco (CIATEJ), Mexico
Copyright © 2022 dos Santos, Pereira, de Souza, Cardoso, da Silva, Oliveira, Verdi and Sobral. This is an open-access article distributed under the terms of the Creative Commons Attribution License (CC BY). The use, distribution or reproduction in other forums is permitted, provided the original author(s) and the copyright owner(s) are credited and that the original publication in this journal is cited, in accordance with accepted academic practice. No use, distribution or reproduction is permitted which does not comply with these terms.
*Correspondence: Adijailton José de Souza, YWRpamFpbHRvbmpzb3V6YUBhbHVtbmkudXNwLmJy; Elke Jurandy Bran Nogueira Cardoso, ZWpibmNhcmRAdXNwLmJy
†In Memoriam