- 1Centre for Water and Geospatial Studies, Tamil Nadu Agricultural University, Coimbatore, India
- 2ICAR-Indian Institute of Rice Research, Hyderabad, Telangana, India
- 3Department of Soil Science and Agricultural Chemistry, Tamil Nadu Agricultural University, Coimbatore, India
- 4Department of Environmental Science, Mother Terasa College of Agriculture, Pudukkottai, Tamil Nadu, India
Land degradation and climate change, two intricately intertwined phenomena, demand appropriate management solutions to effectively tackle the escalating issues of food and nutritional security. In this context, the realm of agriculture confronts formidable challenges in its pursuit of soil resource reclamation, improving water quality, mitigating climate change, and maintaining soil and natural resources for posterity. Central to these aspirations is the preservation of an optimum organic matter, serving as a linchpin threshold is crucial for protecting the physical, chemical, and biological integrity of the soil, while simultaneously sustaining agricultural productivity. To address these multifaceted challenges, the introduction of diverse organic amendments has emerged as a crucial strategy. Noteworthy among these is the application of biochar, which functions as a soil conditioner capable of bolstering soil health, mitigating the impact of climate change, and securing global food security. Biochar is a carbon-enriched substance produced through pyrolysis of assorted biomass waste. It has a larger surface area, higher cation exchange capacity, and an extended carbon storage capability. The strategic integration of biochar production and subsequent soil application engenders an array of benefits, encompassing the amelioration of soil physical properties, augmented retention and the availability of nutrients, and the enhancement of biological activity, resulting in higher agricultural yields and societal benefits through the curtailment of soil to atmosphere greenhouse gas emissions. Additionally, biochar demonstrates its efficacy in the realm of environmental restoration by serving as a medium for extraction and elimination of heavy metals, which often pervade aquatic ecosystems and soil matrices. This review addressed the need for biochar production, characterization, soil health, the possibility for environmental restoration, and crop yield fluctuations owing to climate change.
Introduction
The primary goal of contemporary and prospective agriculture revolves around the provision of both safe and nutritious food to the expanding global population (1). However, in the context of global climate change, its consequences on soil health and agricultural production have become more significant (2). Adopting suitable crops, fertilization techniques, pest management protocols, and irrigation practices typically leads to high yields, which strengthens food security and ensures nutritional adequacy (3). Despite high production, farmers remain worried about a problem linked to the existing high-intensive agricultural production systems.
Soil health stands as a cornerstone in the realm of resilient and sustainable food production. This paradigm emphasizes the convergence of physical, chemical, and biological measurements of soil quality, all of which intricately affect both farmer profitability and the environment (1). Conversely, the preservation of ample soil organic matter assumes paramount importance in upholding sustainable soil health (4). A substantial volume of carbon dioxide (CO2) finds its release into the environment through the combustion and inherent decomposition of biomass, particularly within the realm of agricultural waste (5). The conversion of agricultural residues and agroforestry byproducts into biochar through a thermo-chemical process, specifically pyrolysis, emerges as an alternative avenue for managing and disposing of surplus agricultural waste with enhanced efficiency (6). Pyrolysis is the chemical breakdown of a substance under oxygen-devoid conditions at extremely high temperatures (3). To make biochar an affordable and cost-effective soil conditioner, a farmer-centric approach which facilitates farmers to produce biochar themselves from agricultural residues by adopting low-cost biochar production techniques (7). A new practical and cost-effective in-situ method called “burn and soil cover” (B-SC), allows farmers to convert crop residues into biochar. It involves burning air-dried crop residue waste in the field, achieving approximately 90% combustion before covering the residue with soil to slow down the process.
Biochar is a carbon-rich substance produced from biomass through the pyrolysis process. Biochar is prepared from various biological waste materials, such as cow dung, poultry manure goat manure, municipal solid waste, and other organic wastes (8). In recent times, the application of biochar has garnered notable attention due to its multifaceted advantages. It not only serves as a carbon sink, contributing to carbon sequestration but also ameliorates soil compaction and enhances the physical condition of the soil. Biochar application is a novel approach for long-term carbon storage in the soil (9). The high carbon content and stability of biochar make it a promising option for carbon sequestration. The aromatic nature of biochar makes it resistant to breakdown by biological and environmental factors, resulting in a higher carbon content compared to the original biomass-retained soil. Ample evidence reported that biochar can remain in the soil for hundreds to thousands of years (10). The labile biochar components typically last around 108 days, while recalcitrant components can persist for about 556 years (11). This variation is mainly due to the chemical recalcitrance of biochar, which arises from the presence of aromatic groups (9). The impact of biochar on different soil carbon fractions is unclear due to varying experimental conditions, biochar types, and soil types. Chagas et al. (12) conducted a meta-analysis and found significant increases in total C (64%), organic C (84%), microbial biomass C (20%), labile C (23%), and fulvic acid (42%). Application of a high rate of biochar in low-carbon fine-textured soil of temperate zones increased total carbon content in short-term trials. Thus, considering experimental settings, biochar types, and soil characteristics is crucial for optimizing biochar application rates to maximize soil carbon sequestration and enhance labile carbon fractions.
Furthermore, biochar application increases nutrient availability in the soil, thus contributing to improved nutrient availability for plants (3). Additionally, biochar carries organic substances and nutrients within its matrix. Upon incorporation, it increases soil pH, electrical conductivity (EC), and organic carbon, thereby exerting a positive influence on soil fertility (13). The utility of biochar extends to its role as a long-lasting mechanism for carbon storage within the soil matrix. This attribute holds the potential to decelerate or even arrest the escalation of greenhouse gas (GHG) emissions into the atmosphere, making a notable contribution to mitigating climate change (14). On an ecological front, biochar improves environmental quality by lowering the leaching loss of soil nutrients, diminishes the bioavailability of environmental pollutants, and sequesters carbon while concurrently lowering GHG emissions. Notably, biochar application improves impaired or degraded soil environments and augments crop yields by creating congenial environments (8).
Biochar emerges as a green material for heavy metal elimination, attributed to its well-designed physical and chemical properties, such as substantial specific surface area and high sorption capacity (15). Its credentials extend to environmental compatibility, economic cost, and significant efficacy in the realm of removal processes (16). These collective features render biochar a suitable material for heavy metal sequestration. Moreover, the biochar surface hosts a repertoire of diverse functional groups, such as carboxyl, alcohol, and hydroxyl groups, which engender the prospect of intricate formations between these groups and heavy metal ions (13). Empirical evidence from contemporary research substantiates the assertions. Interestingly, numerous instances of biochar exhibit equivalent or superior adsorption capabilities for heavy metals in comparison to commercially available activated charcoal (17).
Alterations in the physical characteristics, including surface area, surface charge, pore size, and pore volume, exert a direct influence on the adsorption potential of biochar (15), driving the synthesis of engineered biochar. This synthetic biochar, depending on the method of modification, can manifest large surface areas, an augmented number of surface functional groups, elevated cation exchange capacity (CEC), and alkaline properties (18). The arena of biochar engineering can be tailored to manipulate specific attributes suitable for distinct objectives and contextual scenarios (15). These developments underscore the prowess of engineered biochar as a superior agricultural soil amendment and environmental remediation agent, particularly proficient in absorbing heavy metals for their subsequent transformation in soil (19).
The incorporation of biochar into agricultural soils assumes a pivotal role in improving soil fertility, amplifying crop productivity, and concurrently contributing to the alleviation of global climate change. This is achieved through the dual mechanisms of curtailing GHG emissions and sequestering atmospheric carbon within the soil matrix (20). As a result, it is critical to understand the entire spectrum of biochar related aspects, including production, characterization, and its multifarious applications. The present work summarizes the possible application of biochar as a soil amendment, a soil pollution abatement, and a tool for reducing GHG emissions.
Through an in-depth analysis of the literature surrounding biochar as a sustainable soil conditioner, this review serves the purpose of consolidating existing knowledge and pinpointing gaps in current research, thereby shedding light on areas requiring further investigation. Emphasizing the positive outcomes of biochar, it underscores its role in advancing sustainable agricultural practices. To enhance clarity and streamline presentation, this review organized the impact of biochar into four distinct subheadings: “Impact of biochar on crop yield, Soil properties, Environmental pollution abatement, and Carbon sequestration and climate change”. Furthermore, our exploration extends beyond theoretical realms, delving into the practical applications of biochar and meticulously discussing its economic implications and prospects. Recognizing the diverse audience interested in this critical subject, including policymakers, farmers, and other stakeholders, we acknowledge the importance of providing well-informed, evidence-based information. As such, this review stands poised to serve as a reliable reference for decision-makers seeking to implement sustainable practices in both agriculture and environmental management.
Methodology
For this review, a total of 352 research articles/book chapters on biochar were downloaded, following which a meticulous selection process was undertaken. The keywords used for searching the articles were biochar, climate change resilience, soil attributes and heavy metal remediation. Initially, research articles unrelated to soil health, those lacking a focus on soil carbon, and book chapters, were excluded, resulting in a refined pool of 196 articles. Subsequently, a thorough examination was conducted on 101 articles that specifically delve into the dynamics of soil organic carbon (SOC), GHG emissions (CH4, N2O, CO2), as well as the remediation of heavy metals contamination in soil.
Impact of biochar on soil properties
The incorporation of biochar into the soil exerts a direct impact on various soil attributes governing its behavior, encompassing water retention, aggregation, permeability, as well as swelling-shrinking capacity. Moreover, biochar substantially affects the soil’s capacity for cation storage and its responsiveness to temperature fluctuations (21). Biochar also imparts noteworthy changes in the soil’s physical characteristics, resulting in a net increase of total soil-specific surface area and a reduction in the soil bulk density, consequently eliciting improvements in soil structure and aeration dynamics (22). The use of biochar has a significant impact on the soil’s chemical properties (Table 1), such as pH, EC, CEC, and the amount of soil organic matter (36). However, the extent of these impacts is influenced by the type and inherent attributes of the soil, the rate and frequency of application, the nature of feedstock used, preparatory conditions, and the manufacturing technique employed in biochar production (18). Furthermore, biochar demonstrates a propensity for alleviating the consequences of drought, primarily by increasing soil moisture content. This, in turn, engenders a dual impact of reducing nutrient leaching and mitigating soil erosion (37). Biochar is a revolutionary climate mitigation soil amendment that improves soil carbon storage and crop production with the greatest impact in deteriorated acidic or nutrient-poor soils (22).
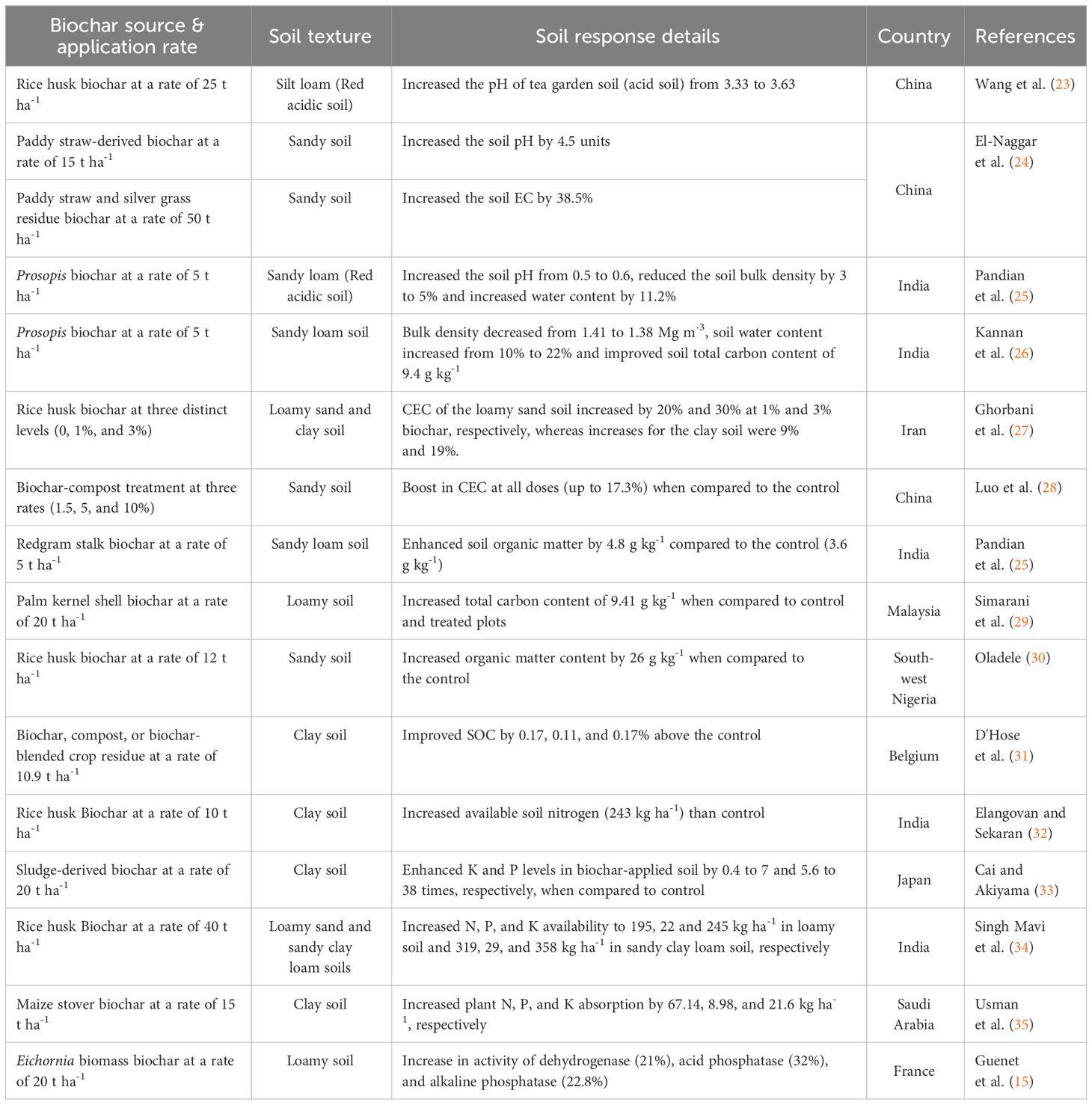
Table 1 The effect of varied rates and sources of biochar application on soil properties across various soil texture.
Biochar produced at lower temperatures (300 or 400 °C) results in an acidic biochar composition, whereas higher temperature (700 °C) processing yields biochar with an alkaline nature. This observation holds remarkable significance, given that the agricultural use of biochar serves a dual purpose (38). When contemplating the incorporation of biochar into acidic soil, those generated at higher temperatures offer a valuable amendment for soil neutralization, concurrently contributing to enhanced soil fertility (39). The application of alkaline biochar elevates the pH of an acidic and neutral soils. Conversely, biochars produced at lower temperatures holds practical utility in addressing issues of alkalinity in alkaline soils (40). Beyond its ameliorative effects on soil pH, biochar functions as an exceptional soil amendment, simultaneously imparting plant nutrients while acting as a carbon sequestration agent (41). The application of biochar also holds the potential to improve the physical health of the soil, fostering robust root proliferation while concurrently augmenting the facilitation of air, water and gas exchanges within the soil matrix (18).
pH
A specially designed biochar composite application effectively reduced the soil pH and increased micronutrient bioavailability (40). Interestingly, research conducted by Yang et al. (18) showcased that the degree of enhancement in soil pH was more pronounced with increasing rates of finely textured rice straw biochar compared to coarser textured biochar. The use of rice husk derived biochar increased the pH of tea garden soil (acid soil) from an acidic pH of 3.33 to 3.63. Changing soil pH concurrently boosting ion exchange capacity, reduces leaching loss of nutrients and curbs losses due to volatilization mechanisms (42). Utilizing biochar as a nutrient carrier holds promise in offering a sustainable long-term solution to issues associated with alkaline soils. Soil applied with 10 t ha-1 of biochar exhibited the greatest average pH compared to control soil registering lowest value (18). Incubation experiment conducted by Zhao et al. (43), employing various crop straw derived biochar having varied pH from 7.69 to 10.26. The results revealed that the application of multiple biochars at 1% and 2% w/w significantly lowered the soil pH in Ultisol (pH 4.31). The application of paddy straw-derived biochar (pH of 10.50) into sandy soil (initial pH 5.24) led to an impressive rise of 4.5 pH units in comparison to the control (24). Similarly, cotton stalk biochar at 5 t ha-1 elevated acidic red soil pH from 5.7 to 6.0 (25). However, in contrast, Li et al. (44) observed that adding biochar (10, 20, 40, and 60 t ha-1) had no impact on soil pH in a semi-arid area.
Electrical conductivity (EC)
The incorporation of biochar into soil enhances its EC. It has been demonstrated that biochars generated at higher pyrolysis temperatures, especially those derived from wood and paper waste, showcase higher EC (43). Paradoxically, this rise in alkalinity proved counterproductive, impeding the growth and development of halophytic plants. Prapagdee and Tawinteung (45) observed that the application of cassava stalk biochar at 10% (w/w), increased soil EC. Similarly, the addition of rice straw (30 t ha-1) and silver grass residue-derived biochar (50 t ha-1) into sandy soil (EC of 0.07 dS m-1) enhanced the EC by 38.5% (24). In a semi-arid tropical Alfisol, the application of Prosopis biochar at 5 t ha-1 raised soil pH to 6.33 and EC to 0.42 dS m-1 (25). On the contrary, the application of a huge amount of biochar mixed with chemical fertilizer enhanced the EC, which had no negative impacts on plant development (17). The salinity fluctuations associated with biochar applications are mainly due to crop growth conditions, water availability, and the amount of biochar supply. Hence, understanding the soluble salt content in biochar solutions assumes paramount importance, as excessive amounts of biochar addition to the soil may potentially damage salt-sensitive plant species (46).
Bulk density and water retention
Soil amending with biochar positively influences the soil bulk density and the magnitude of change varies with dose of biochar and soil type. Pandian et al. (25) studied the effect of various levels of Prosopis biochar (0, 2.5, and 5 t ha-1) in red soil and found that the application of 5 t ha-1 reduced soil bulk density by 3 to 5% while increasing water content by 11.2%. In a 3-year investigation, Kannan et al. (26) found that the application of Prosopis biochar (5 t ha-1) on sandy soil decreased bulk density from 1.41 to 1.38 Mg m-3 and increased soil water content from 10 to 22%. Similarly, Oladele et al. (47) discovered that the application of rice husk biochar at 12 t ha-1 at a soil depth of 0 to 10 cm enhanced the soil moisture content (12%). Higher biochar levels were associated with increased soil moisture content at the measured soil depths. Lateef et al. (48) observed that water retention capacity in soil with biochar was 67.17%, while soil without biochar was 55.5%, after 20 days of research. Similarly, Liu et al. (13), also observed that the water retention capacity of biochar in soil was greater than that of untreated soil. This might be owing to the increase of soil organic matter and total porosity.
Cation exchange capacity (CEC)
The high CEC of biochar significantly contributes to improving soils with low fertility. Ghorbani et al. (27) assessed the impact of rice husk biochar at three different concentrations (0, 1%, and 3% by w/w) in two distinct soil types (loamy sand and clay). They found that the application of biochar at 1% and 3% increased the CEC of the loamy sandy soil by 20% and 30%, while the comparable increases for the clay soil were 9% and 19%, respectively. Similarly, Luo et al. (28) used three rates (1.5, 5, and 10% by w/w) of biochar to improve the properties and productivity of degraded soils. They found an increase in CEC at all doses (up to 17.3%) compared to control (2.72 cmol kg-1) with CEC values of 3.03, 3.19, and 3.15 cmol kg-1 for 1.5, 5 and 10% of biochar application, respectively.
Soil organic carbon (SOC)
Since biochar is a carbon-rich material, its application naturally increases the organic carbon content of soils. The carbon content in biochar varies greatly depending on the feedstock and pyrolysis temperature (24). The carbon stability of biochar is affected by the feedstock; for example, biochar derived from wood has a greater stability in soil than biochar derived from rice wastes due to the higher lignin content in the woody biomass (29). Furthermore, the ratio of aliphatic and aromatic carbon fractions, condensation of aromatic carbon, and pyrolysis temperature are other critical factors that decide biochar stability. For instance, biochar produced at higher temperature contains more aromatic carbon, resulting more stability and slowly degrade in the soil. (49).
Biochar emerged as a valuable supplement for low-fertile soils to enhance SOC content, and to mitigate climate change (50). Pandian et al. (25) noticed that the application of red gram stalk biochar at 5 t ha-1 enhanced SOC by 4.8 g kg-1 compared to the control (3.6 g kg-1). Similarly, Oladele (30) also noticed application of rice husk biochar at 12 t ha-1 resulted significant increase in organic matter content (26 g kg-1). Applying biochar, compost and biochar-blended crop residue at 10.9 t ha-1 significantly increased SOC by 0.17, 0.11 and 0.17% compared to control (31). The co application of palm kernel shell biochar at 20 t ha-1 and chemical fertilizers augmented total C content (9.41 g kg-1) compared to control (29). Ajayi and Horn (51) studied the impact of wood chips biochar on the properties of two distinct soil types, namely fine-sand and sandy loamy silt. They found that the addition of biochar at 5 t ha-1 increased the total carbon content of both soils (6.78 g kg-1 and 5.02 g kg-1, respectively). Similarly, Kannan et al. (26) also observed an enhancement in soil total carbon content (9.4 g kg-1) by the application of redgram stalk biochar at 5 t ha-1 in low pH red soil.
Biochar application improves soil health by augmenting organic and total carbon, resulting in better soil physical health, nutrient availability and microbiome (50). Additionally, biochar application improves organo-mineral interactions via adsorption and ligand exchange processes. This transformative process emphasizes its role in boosting soil stability and contributing to the SOC built-up (52). Further, Fe and Al oxyhydroxides and clay minerals, intricate interaction with different organic fractions stabilize the organic matter in subtropical soils. (53).
N, P and K availability and loss
Fertilizers intercalated with biochar are an effective way to raise the availability and concentrations of essential nutrients in soil. During the critical reproductive growth phase (flowering and grain filling), soil treated with biochar has the highest concentrations of N, P, and K compared to conventional sources. A number of approaches have been put forth to reduce the loss of nutrients and improve their retention in soil treated with biochar. The co-application of biochar (12 t ha-1) and nitrogen fertilizer (90 kg ha-1) significantly improved soil N, P, and K status (47). Elangovan and Sekaran (32) utilized different quantities of biochar (0, 2.5, 5, 7.5, and 10 t ha-1) in conjunction with synthetic fertilizers (75% and 100% NPK). The results showed that the combined application of 100% NPK and 10 t ha-1 of biochar increased soil available nitrogen (243 kg ha-1). The research conducted by Liao et al. (21) also indicated that nitrogen fertilizer (15N labeled fertilizer) infused with biochar caused a significant delay in the release of nitrogen from the treated soils.
Nitrogen supply and plant N requirement are better synchronized as nitrogen is gradually released from the urea-biochar composite, resulting in higher N uptake and minimal losses (37). Zheng et al. (54) observed that urea along with biochar treatment boosted rice N absorption by 73% compared to urea treatment alone. The increased nitrogen content in urea biochar composite, along with its steady and gradual nutrient release profile, are responsible for the increased residual N availability. This phenomenon reduces losses resulting from denitrification, leaching, or volatilization, and in the end, it creates an environment that is favorable for increased biomass production and elevated soil organic matter (55). Biochar-based fertilizer and acidified biochar-coated urea showed lower ammonia volatilization than pristine urea and unacidified biochar-coated urea (36). Furthermore, Krause et al. (56) discovered that adding biochar results in lower N leaching through nitrate storage, which raises crop productivity.
Several strategies aimed at minimizing N leaching and enhancing its retention in biochar-amended soil (57). Adsorption of NO3 and NH4+, immobilization, and ionic exchange with biochar are notable examples (13). This is ascribed to the mechanical disturbance in soil caused by the biochar application, which increased soil aeration and nitrification led to significant transformation of NH4+ into NO3 (58). The effect of biochar on NO3-N adsorption capacity is not only due to CEC but also other features such as pore size and distribution, surface area, and functional groups (18), which are known to differ among biochar forms. The anionic adsorption potential and high surface area of biochar are two probable reasons for nitrate ion adsorption and preservation in soil (44).
As regards P, Liao et al. (21) observed higher levels of Olsen-P in soils after the application of biochar-based fertilizer while, Cai and Akiyama (33) observed an enhancement in K and P levels in sludge-derived biochar-treated soils by 0.4 to 7 and 5.6 to 38 times, respectively, compared to control. Maikol et al. (59) studied the effects of different quantities of biochar and nitrogen fertilizer on loamy sandy and sandy clay loam soils. They found that the application of biochar at 40 t ha-1 with 150 kg ha-1 of nitrogen fertilizer increased N, P, and K availability by 195, 22, and 245 kg ha-1 in loamy soil and 319, 29, and 358 kg ha-1 in sandy clay loam soil, respectively.
Biochar-based fertilizers boosted the plant N, P, and K absorption by 40, 46, and 26%, respectively (60). Application of rice husk biochar with NPK boosted N absorption by 20.7% compared to NPK treatment alone (36). Similarly, Liu et al. (13) observed an enhanced CEC, increased water retention capacity, and the promotion of microbial nitrogen immobilization following the introduction of biochar into the soil. Usman et al. (35), also discovered that application of biochar at 15 t ha-1 increased the plant N, P, and K absorption by 67.14, 8.98, and 21.6 kg ha-1, respectively. Thus, combining inorganic N fertilizers with biochar increased soil fertility and supported soil ecology through nutrient retention and carbon build-up.
Nutrient use efficiency (NUE)
By acting as a soil modifier, biochar helps in improving crop yield and nutrient utilization efficiency (NUE), which reduces soil acidification and leads to an overall increase in agricultural productivity, improved soil quality, enhanced physico-chemical and biological properties of soil (61). Biochar can improve plant growth and production by increasing NUE and contributing to an enhancement in soil water holding capacity and mycorrhizal competence (62). The effects of biochar on soil change entirely depend on the biochar application rate and technique used (63).
Amin and Eissa (64) used Zucchini plants with varying quantities of biochar (0, 6.3, 12.6, and 25.5 g pot-1) and revealed that the application of biochar at 25.5 g recorded the greatest N agronomic efficiency (86.9%) and partial factor production (131.4) in a pot study. Zhang et al. (65) also found that application of biochar at 20 t ha-1 raised wheat NUE from 5.2 to 38%, while greater biochar dose decreased NUE. Zhang et al. (66) discovered that co-application of varied levels of biochar (0, 10, and 40 t ha-1) with N fertilizer increased agronomic use efficiency (6 kg of additional grain kg-1 of N fertilized) compared to a control. Similarly, Liao et al. (21) observed that application of biochar-based fertilizer significantly increased NUE (58.8%) than control.
Soil microorganisms
Biochar is an effective means of stimulating living organisms and improving the natural environment, besides providing a conducive habitat for diverse soil microorganisms (58). It enhances microbial activity and biomass while changing the composition and abundance of microbial communities due to the high surface area and increased surface hydrophobicity of biochar (44). Apart from microorganism diversity, shifts in microbial community composition is also plausible due to changed soil conditions as well as resource availability (e.g., labile C, nutrients, and water), and alterations in abiotic factors (e.g., toxic substances), or variance in natural habitat (67). Such transformations in soil characteristics and microbial population would affect the degradation of biochar (49). The bacterial population in cotton soils subjected to continuous cultivation for different durations (two, six, eleven, and fourteen years) and subsequently treated with varying levels of biochar (0, 12.5 and 20 t ha-1) was studied by Han et al. (68). They found that, there was a relative abundance of Sphingomonas and Pseudomonas in biochar-treated soils compared to untreated soil. The findings indicated that biochar application has a considerable influence on the soil bacterial community, which may promote microbial diversity of cotton soils in continuous cropping systems.
The application of biochar serves as a shelter for soil-dwelling microorganisms and protect them from natural predators (18). Guenet et al. (15) unveiled that the application of Eichornia biomass derived biochar at 20 kg ha-1 resulted in substantial enhancements in enzyme activity; specifically, dehydrogenase activity increased by 21%, while acid phosphatase and alkaline phosphatase activity increased by 32% and 22.8% respectively. Biochar application extends mycorrhizal fungi function through multiple mechanisms: (i) altering soil physico-chemical properties, (ii) indirect influence on mycorrhizae by modifying the surrounding microbial environment, (iii) interfering with plant-fungus signaling and allelochemical detoxification on biochar, and (iv) providing shield against fungal grazers (13). Biochar’s porous structure enhances the habitat for mycorrhizal fungi and other soil microorganisms, thereby improving soil quality (14). The effect of biochar on different types of soil’s characteristics was depicted in Figure 1.
Impact of biochar on crop yield
Crop yield under biochar applied field showed different responses in various soil types and climatic conditions. A meta-analysis results revealed that the application of biochar increases in net primary crop productivity, grain yield, and dry matter (46). The yield-influencing potential of biochar on agricultural and horticultural crops is depicted in Figures 2, 3. The micro-to-macro plot level effect of biochar on crop yield is discussed in detail to understand pros and cons of biochar. The application of Eucalyptus citriodora Hook biochar at 2.5 g kg-1 to slightly alkaline soil enhanced basil productivity by 10% under pot experiment (69). Rice straw biochar at 10 t ha-1 increased carrot and bean yields compared to control in low pH (5.2) soil (70).
Direct applications of manure-derived biochar supply significant quantities of nutrients to the soils resulting higher crop yield. Applying poultry manure biochar at 10, 25 and 50 t ha-1 augmented radish yield by 12% compared to control (28). In another study, Pandian et al. (25) found that the application of redgram stalks biochar at 5 t ha-1 boosted groundnut dry matter production and pod yield by 24 and 29% in acidic red soil under rainfed conditions. The co-application biochar (25 t ha-1), FYM (10 t ha-1) and nitrogen (30 kg ha-1) enhanced mung bean growth and biological yield (4330 kg ha-1) compared to control (3189 kg ha-1) (9). Even in soils with salinity, the application of maize stalk biochar increased soybean yield by 11% and wheat by 28% (21). In grape cultivation, the yield increase was even more substantial, reaching 66% (13). The application of biochar at 50 t ha-1 resulted in a yield increase of 1.17 t ha-1 for maize and 0.43 t ha-1 for soybeans than the control (55). In a separate study, Berihun et al. (71) found that applying Lantana biochar at a rate of 18 t ha-1 significantly improved maize grain yield (528 kg ha-1) compared to control (134 kg ha-1). Investigating the impact of biochar on upland rice grain yield in northern Laos, Maghsoodi et al. (72) found that, applying biochar at a rate of 4 t ha-1 increased the rice yield by 4.7 t ha-1 compared to the control yield of 2.8 t ha-1. Biochar has the tendency to increase nutrient availability in soils by increasing ion retention, potentially resulting in higher plant yields (15). However, the favorable effect of biochar is strongly dependent on soil properties and additional research is essential to explore its effect on various crops. Table 2 illustrates the influence of different rates and sources of biochar application on crop response across various soil types.
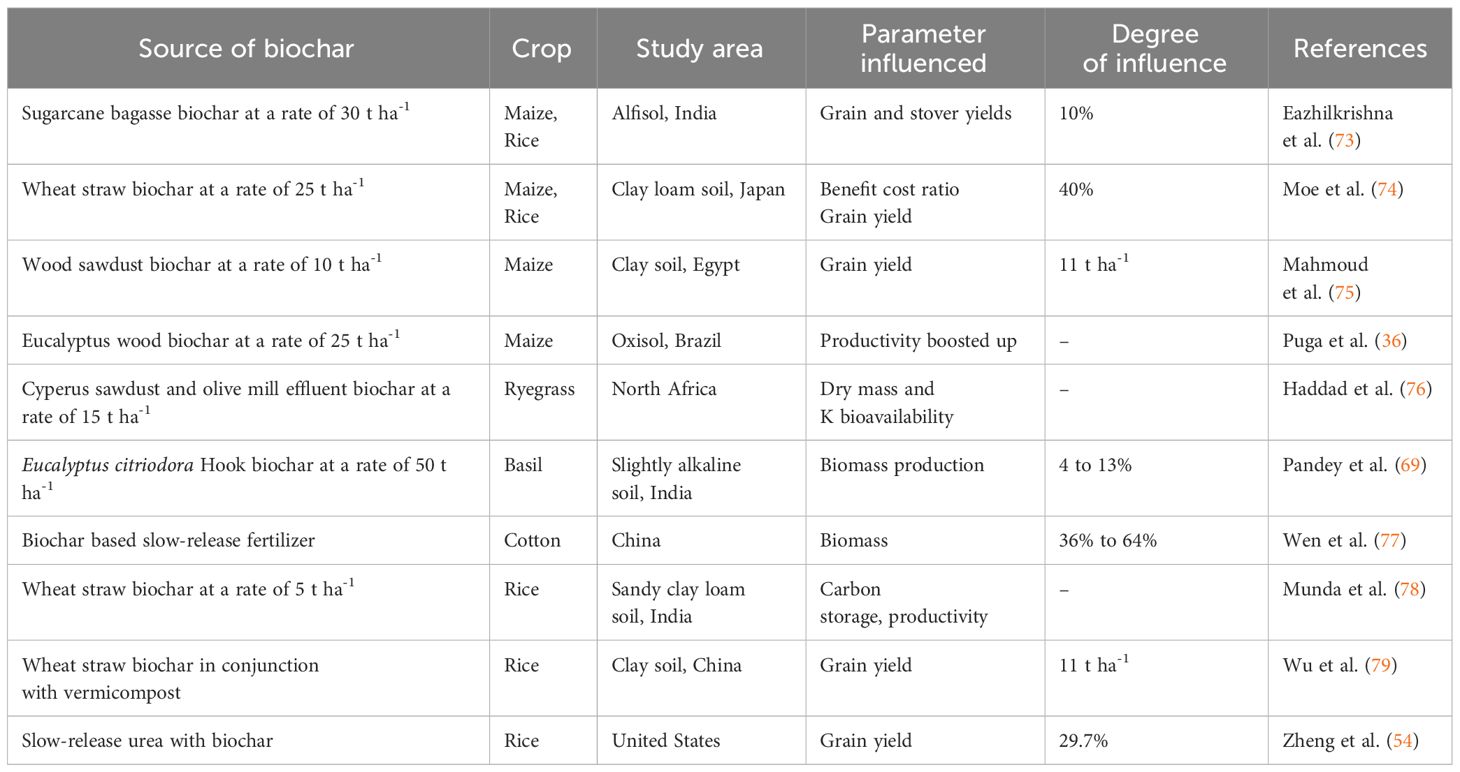
Table 2 Influence of different sources of biochar application on crop response across various soil texture.
Impact of biochar on environment pollution abatement
A relatively new and promising technique for treating wastewater is the use of biochar to extract organic and heavy metal pollutants from aqueous media (13). Biochar appears to be a workable way to deal with these environmental problems brought on by climate vulnerability (80). Although biochar’s effectiveness in immobilizing heavy metals varies based on its own chemical and physical properties as well as those of the treated soil (2). The promising mechanisms of biochar on heavy metal abatement was shown in Figure 4. Moreover, the effect of biochar on heavy metal concentrations in plants varies with soil type, biochar type, plant species, and metal pollutants (13). It was reported that the application of biochar reduced the accumulation of Cd, Pb, Cu, and Zn in plant tissues by 38, 39, 25, and 17%, respectively (81).
Qin et al. (82) reported that the application of biochar derived from manure exhibited greater efficacy in reducing Cd and Pb concentrations in plants than biochar produced from other feedstocks. The impact of biochar on Pb concentrations within plant tissues was found to be minimal, yet its influence on Cu concentrations was markedly significant in alkaline soils (5). On contrary, biochar with a high pH and application rate was shown to yield a greater reduction in heavy metal absorption by plants (83).
Heavy metal absorption potential biochar was due to the presence of functional groups such as cellulose, hemicelluloses, lipids, carbohydrates, and proteins in agricultural residual feedstocks (84) may be physically activated during pyrolysis (85). Further, the surface of biochar contains chemically reactive groups such as hydroxyl, carboxyl and ketones further endow biochar with an enormous ability for absorption of heavy metals and harmful elements, such as Al and Mn in acid soils, and As, Cd, Cu, Ni, and Pb in heavy metal contaminated soils (17). Because of its carbon-structured matrix, high degree of porosity, surface area, and strong attraction for non-polar substances such as PAHs, dioxins, furans, and other compounds, biochar play an important surface sorbent with the potential to effectively modulate contaminants within the environment (85).
Apart from functional and chemically reactive groups, the remarkable super-sorbent property of biochar enables efficient removal of both organic and inorganic pollutants from soil and water environments (86). Given the vast diversity and low cost of biochar feedstocks, it emerge as a more-effective remediation technology for heavy metal adsorption remediation compared to activated charcoal (47). Considering the biochar feedstocks, biochar generated from rice husk (87), maize straw (5), peanut straw (67), olive pomace (88), and oak wood and bark (89) are effective for heavy metal removal. Similarly, iron-impregnated magnetic biochars have also shown promise in the removal of heavy metals like Cr (VI) using zinc and chitosan-modified biochars (85), Pb (II), Cu (II), and Cd (II) using KMnO4-treated wood biochar (90).
Phosphorus removal has been demonstrated in various investigations utilizing Ca, Mg, and Al-modified biochars (16). Several organic compounds have been reported to be removed using catalytic and degradative nanoparticles such as nanoscale zerovalent ions and graphitic C3N4 (91). The use of nanocomposites holds promised product for water treatment, it remains a relatively nascent area of research and requires further investigation, particularly on the reuse, desorption, and removal of these metal-attached nanocomposites (90).
Hence, a variety of raw materials and specific pyrolysis temperatures as well as possible adaptations of the biochar preparation are used to produce and determine its physical and chemical properties, such as pH, EC, CEC, or organic carbon content, and to a large extent of biochar-pollutant interaction potential (59). For cultivated soils, biochar is a conditioner that improves soil fertility, thereby ensuring food security, preserves farmland diversity, and reduces the use of chemical fertilizers (92). Furthermore, biochar enhances water quality by assisting in the retention of nutrients and agrochemicals in soils for use by plants and crops, resulting in less pollution (93). A biochar-based method can help with waste management, bioenergy generation, and long-term soil health management (90). Table 3 summarizes the impact of biochar application on heavy metal removal in soils.
Impact of biochar on carbon sequestration and climate change
One crucial strategy for slowing down the effects of human-caused climate change is soil carbon sequestration, a method of capturing and storing carbon to stop it from entering the atmosphere (81). Transporting carbon to a stable or inert passive carbon reservoir is a feasible option. Oo et al. (99) also highlighted that biochar may be used to combat climate change by storing carbon in soil and replacing the usage of fossil fuels. Biochar could reduce CO2 and methane (CH4) emissions that can be made from agricultural and forestry residues and when it is added into the soil they remain for long periods of time (82). Switching even a smaller amount of the carbon circulating between the atmosphere and plants to a much slower biochar cycle would have a significant impact on atmospheric CO2 concentrations (43). Since annual CO2 uptake by plants from the atmosphere via photosynthesis is eight times greater than anthropogenic GHG emissions. Because of its molecular structure and origins, biochar is more physically and chemically stable than the original carbon form (93). Besides, the trapped carbon is difficult to release as CO2, making this a promising strategy for carbon sequestration (43). As supported by Zheng et al. (54), who claimed that biochar can store 2.2 gigatonnes of CO2 per year by 2050.
During the pyrolysis process, the resultant products such as bio-oil and syngas are utilized as fuels, thereby releasing CO2 into the atmosphere. This CO2 is subsequently absorbed by plants and transformed back into biomass, completing a cyclical process (100). Biochar has the potential to reduce net yearly CO2 emissions by a cumulative CO2eC equivalent of 0.21 Pg, which is approximately 12% of current anthropogenic CO2e emissions (43). Still there is not enough evidence to support conclusions on how the production and use of biochar impact greenhouse gas budgets of the entire system from a systematic review work (101). However, it is reasonable to infer that biochar’s role in climate change mitigation cannot be described as a cause-effect relationship, and that there may be advantages in the long run. As a result, biochar serves as both a source and sink of carbon in the soil (40).
In addition to CO2, the release of CH4 and nitrous oxide (N2O) from the soil has the capacity to influence the climate (99). Biochar exhibits the potential to mitigate GHG emissions through a variety of mechanisms (Figure 5; Table 4). Soil microorganisms produce CH4 in anaerobic conditions via methanogenesis (109). CH4 has a significantly greater capacity to trap thermal energy in the Earth’s troposphere compared to CO2, contributing to the phenomenon of global warming (40). Incorporating biochar into the soil at a rate of 2% resulted in a negligible CH4 emissions (52). The reduction of CH4 emissions necessitates enhanced soil aeration, which can curtail the occurrence of anaerobic conditions conducive to methanogenesis (100). The prolonged presence of biochar in the soil, due to its inherent persistence, leads to diminished emissions of GHG (110).
Nitrification and denitrification are the two processes by which soil microorganisms produce N2O. When biochar is added to soil, it imparts low-density and porous qualities relative to the soil, which ultimately enhances soil aeration and reduces N2O emissions (13). Although, enhanced soil aeration usually reduces N2O emissions, it can also have the opposite effect by raising N2O emissions due to its beneficial influence on soil moisture levels (66). Cai and Akiyama (33) reported that application of biochar into soil immobilizes bioavailable nitrogen (inorganic or organic form) resulting in lowered N2O emissions. The biological immobilization of inorganic nitrogen is aided by biochar’s low nitrogen concentrations and high carbon-to-nitrogen ratios. This process helps to decrease ammonia volatilization by assisting in the retention of nitrogen in the soil system (109).
Numerous mechanisms contribute to the reduction of N2O emissions through biochar application, encompassing shifts in soil pH, enhancement of soil aeration, immobilization of nitrogen, interaction with available organic carbon and nitrogen within the soil matrix, modulation of enzymatic activities, and potential alterations in microbial communities engaged in N2O production (13). Feng and Zhu (110) also emphasized that the biochar is capable of mitigating gaseous nitrogen emissions within agricultural landscapes. Empirical field observations have underscored the substantial reduction in N2O emissions facilitated by biochar application (111). Although the extent of this impact has been shown to be contingent upon various factors encompassing crop type, soil characteristics, and specific attributes of the utilized biochar (99). The influence of soil moisture content is also of paramount significance in N2O production dynamics. Higher moisture levels (>70%) promote anaerobic conditions conducive to denitrification, whereas lower moisture levels (50%) are linked with nitrification processes. Notably, a higher moisture content (80%) results in an N2O production rate approximately 8 to 23 times greater than that observed under lower moisture conditions (40%) (111). It is important to recognize that the impact of biochar on soil N2O flux is inherently influenced by a constellation of factors including soil type, soil moisture levels, supplemental fertilizer application, specific biochar feedstock, and the pyrolysis temperature employed (33). These multifaceted interactions underscore the complexity of biochar’s influence on N2O emissions and necessitate a comprehensive understanding of the context-specific factors at play.
It is currently well established that biochar regulates the final stage of denitrification and promotes the conversion of N2O to N2, possibly due to the liming action, which allows improved expression of the nosZ gene (109). The meta-analysis performed by Cayuela et al. (112) revealed that the H/C molar ratio of biochar is the main feature that leads to lower N2O emissions in soil, indicating C polymerization in the biochar. Previous studies have also found that biochar has an aging impact on N2O emissions (99). Combined application of four forms of biochar (chicken manure, filter cake, sewage sludge, and eucalyptus sawdust) decreased N2O emissions 96% to 69% (113). The amount of biochar used influences the amount of N2O reduced; greater application rates (20- 60%) reduced N2O by up to 74%, whereas lower application rates (2-10%) had no effect (114). Microcosm research discovered that introducing biochar (produced at 550°C from green waste) to sandy loam Haplic Calcisol soil, slowly enhanced soil N2O emissions by 54%. It enhanced nitrification, which is a necessary step for increased N2O emissions (114).
The abundance of genes for the ammonia oxidation process in amoA bacteria resulted in enhanced N2O generation (115). Huppi et al. (116), observed an improved electron accepting capacity, which reduces electron transfer for nitrification and N2O adsorption on biochar, may have resulted in lower N2O emissions. Biochar effectively adsorbs NH3 from soil and acts as a buffer, potentially reducing ammonia volatilization from agricultural areas (117). They discovered a lower NO3-N pool in biochar-amended soil plots and hypothesized that biochar particles increase NH3 adsorption and absorption (56). Furthermore, biochar is an effective adsorber of dissolved ammonium, nitrate, phosphate, and other ionic solutes in soil and water, as well as hydrophobic organic contaminants (39). Since conflicting results have been reported indicating reduced and increased N-losses from biochar-modified soils, an intermediate solution should be considered when applying biochar to agricultural land.
The carbon cycle associated with biochar formation encompasses various indirect sources of GHG. A comprehensive life cycle assessment of pyrolysis operations revealed that the predominant share of GHG, amounting to 89%, arises from the operation and maintenance of the processes. In contrast, construction, equipment, and transportation contribute 7.2%, 3.33%, and 0.23%, respectively (18). Comparisons of the global warming potential among diverse sources from crops, poultry litter, sewage sludge, cattle manure, and food waste underscored consistently negative values across most cycles. On average, the calculated value was -0.9 kg CO2eq kg-1, implying that the consumption of GHG outweighs their emission (109). In an alternative investigation, the introduction of biochar into paddy soil displayed the capacity to decrease overall indirect CO2 emissions, although concurrently leading to heightened CH4 emissions (118). Notably, the intricate interplay of factors such as biochar’s physical and chemical attributes, soil composition, microbial community, water and fertilizer management substantially influence the dynamics of CH4 emissions (39).
Researchers were interested in the notion of sequestering carbon by adding biochar into the soil to ameliorate global warming through soil carbon sequestration (85). The application of biochar in crop fields as a nutrient source has been viewed as a promising soil-based greenhouse mitigation strategy for environmental management (38). The investigation on the properties of 76 biochars through 40 types of research had found that low N content in biochars (C/N ratio > 30) was the possible reason for soil emission mitigation and has an important prospect for carbon sequestration (95). Apart from low N content, another important quality of biochar is its resistance to decomposition. Biochar retain carbon in soils over millions of years, as evident from the Terra Preta soils of northern Brazil in the Amazon basin region (91). Similarly, Wang et al. (40) also reported that even with a low fraction (3%) of biochar carbon, the remainder remains in the soil and contributing to long-term soil stability.
Over 10% of the present anthropogenic carbon emissions could be avoided by even rerouting 1% of the plants’ annual net carbon uptake into biochar (43). According to Sarfaraz et al. (50), 20% of the total carbon biomass is converted into biochar i.e. estimated to be 3 billion tons. If 100% biomass (60.6 billion tons) is converted back into biochar by pyrolysis, the atmospheric carbon emissions will be lowered by about 3 billion tons. A constant carbon content in the soil is achieved by adding biochar with the same carbon content because of their stability in the soil (119). As a carbon sink, biochar increases terrestrial carbon stocks by staying in the soil for long stretches of time and showing strong resistance to both chemical and biological degradation. The usage of biochar presents a chance to lower carbon emissions while sequestering carbon for soil remediation, as most people agree that soil is only a limited carbon sink (16).
Biochar production techniques
Farmers play a critical role in the adoption of biochar for soil applications, which is essential for carbon sequestration. While scientific literature and field studies show positive results on the application of biochar on commercial farms, they lack wider acceptance (120). High pyrolysis and transportation costs hinder biochar widespread field use (121). Low-cost manufacturing technologies are urgently needed to utilize its benefits. Farmer-scale biochar production is more economically viable than industrial methods (122).
To enhance biochar accessibility and cost-effectiveness for soil improvement, a farmer-centric approach involves farmers producing biochar from agricultural residues themselves, directly addressing the cost issue (7). A new practical and cost-effective in-situ method called “burn and soil cover” (B-SC), allows farmers to convert crop residues into biochar. It involves burning air-dried elephant grass and maize waste in the field, achieving approximately 90% combustion before covering the residue with soil to slow down the process. This process yielded 18.0 ± 1.3 kg/100 kg of elephant grass and 13.7 ± 1.3 kg/100 kg of maize residue biochar, suitable for soil improvement (122).
The B-SC biochar production method is a cost-effective solution, requiring minimal inputs like labor, open fields, agricultural residue and inexpensive equipment. Each farmer processed 10 kg of maize residue in an average of 24.4 ± 4.1 minutes (n=10), significantly reducing biomass combustion time and emissions compared to standard field burning (122). Compared to standard field burning, B-SC significantly reduced biomass combustion time and emitted lower levels of smoke and thermal energy, making it a cost-effective and environmentally friendly approach for biochar production and utilization by farmers (122).
Method of field application
The application of biochar to soil is adaptable, catering to both small-scale and large-scale farming operations (123). While manual application suits smallholders, concerns about airborne particles limit its scalability. Broadcasting biochar over large areas requires significant volumes, whereas furrow application, requiring minimal quantities, shows promise in maize fields, targeting the root rhizosphere (113). In Western Australia, deep biochar banding has succeeded with wheat (124), showcasing its potential for customized use. These findings underscore the need for flexible biochar application methods that can adapt to diverse agricultural landscapes. Blending biochar with decomposed manures, composts, and organic inputs not only diminishes odours and colors but also enhances long-term nutrient efficiency (52). Studies using biochar at 5-50 t ha-1 concentrations have shown promise, but cost considerations may hinder widespread adoption (21). Optimal rates of 5-10 t ha-1 (0.5-1 kg m-2) soil basal application have improved soil quality and crop yields across various crops like maize, rice, pea, mustard, and soybeans (72). The co-application of biochar and organics can reduce application rates of biochar with enrichment of essential nutrients offering a sustainable soil health management to enhanced agricultural outcomes.
Use of biochar and its economic implication
Economic viability is vital for farmers seeking to enhance productivity and revenue. Challenges like mineral loss, excessive energy, and labor consumption in agrochemical production can be addressed through biochar-based and encapsulated biochar-based fertilizers (123). The economic feasibility of integrating biochar into agriculture has become a subject of growing interest due to its potential to enhance soil fertility and crop productivity. While the upfront costs of biochar production and application pose initial financial challenges, numerous studies suggest that the long-term benefits may outweigh the expenses. Biochar costs vary widely, from 325-550 USD t-1 in higher-income nations to as high as 5000 USD t-1 in certain regions like the UK and USA (125). In Australia, it can cost up to 800 USD t-1, making it a significant investment for users, especially considering that 20-100 t ha-1 are needed over multiple seasons (124). In the Philippines and India, biochar is priced below 100 USD t-1 due to low labor costs (117).
The combination of organic fertilizer (110 t ha-1) with biochar (8.5 t ha-1) resulted in the highest net revenue (Rs. 82,692 ha-1) (18). Similarly, Elangovan and Sekaran (32) found that co application of biochar at 10 t ha-1, 100% RDF, and FYM at 12.5 t ha-1 resulted in the highest net return of Rs. 67,928 ha-1. Co-application of 100% NPK, biochar at 2 t ha-1 with Azospirillium at 10 kg ha-1 boosted the benefit-cost ratio of sugarcane to 1:1.96 (126). Employing 100% STCR-based (Soil Test Crop Response) NPK in conjunction with biochar composite at 5 t ha-1 produced the highest net income of Rs. 1,33,989 ha-1 for okra, with benefit-cost ratio of 1:2.3 (127). The development of scalable, cost-effective production methods will be pivotal in determining its widespread adoption in agricultural systems.
Combined application of biochar (3 t ha-1) with inorganic fertilizer (70% of recommended dose) in pepper significantly boosted the economic returns (9,597 USD ha-1) compared to conventional fertilization (economic returns: 6,493 USD ha-1) (128). Cotton straw/bentonite biochar-based fertilizer costs 206 USD t-1, much lower than commercial chemical P and K fertilizer at 830 USD t-1 (129). While adding encapsulating/coating materials may raise costs, encapsulated biochar-based fertilizers could enhance sustained release mechanisms, potentially reducing fertilization frequency, time, and manpower needs (18).
Biochar can improve nutrient retention, water holding capacity, and soil structure can lead to increased yields and reduced dependency on external inputs, thereby contributing to cost savings for farmers. Additionally, biochar’s carbon sequestration properties align with environmental sustainability goals, potentially opening avenues for carbon credit programs that could further enhance the economic viability of biochar utilization in agriculture. The economic feasibility is mainly driven by various factors such as feedstock availability, production methods and local agricultural practices. However, affordability doesn’t guarantee acceptance, as seen in western Kenya, where despite biochar increasing maize and sugarcane yields by 32%, farmers were hesitant to adopt it, even with subsidies (123). Many developing countries also face energy challenges, making bioenergy from biomass an appealing alternative (21). There is a pressing need for low-cost pyrolysis methods that yield high-quality biochar with minimal emissions (56). To boost profitability, carbon trading schemes have been proposed, offering compensation for public benefits like reduced emissions and increased carbon sequestration. Nonetheless, the impact of such interventions on technology competitiveness should be carefully assessed to ensure long-term viability (109).
Despite its numerous benefits, biochar is underutilized. Research has primarily focused on its role as a soil amendment, where it competes with fertilizers on cost (56). Farmers’ limited understanding of biochar could hinder its adoption. To promote its use, understanding the economic conditions for production and application is crucial. The economic viability of biochar and biochar-based fertilizers hinges on factors such as production costs, market demand, and their impact on farmers and the environment (37). A comprehensive evaluation, including market research and techno-economic analysis, can provide valuable insights into biochar’s economic feasibility and its role in sustainable agriculture.
Conclusions
Agricultural and municipal waste can harm the environment but can also be turned into valuable resources like biochar. Biochar made from organic waste by pyrolysis offers various benefits: it removes pollutants, absorbs CO2, and boosts soil health. Global trial data show it improves soil and can reduce, increase, or have a neutral effect on greenhouse gases. It’s especially beneficial in low pH and coarse-textured soils for increasing crop productivity. However, its impact on heavy metal reduction varies based on biochar types and metals. A farmer-centric approach involves farmers producing biochar from agricultural residues, addressing cost issues. Compared to standard burning, burn and soil cover significantly reduces combustion time and emits lower levels of smoke and thermal energy, making it cost-effective and eco-friendly. Biochar application is adaptable for small and large-scale farming, and co-application with organics enriches nutrients, offering sustainable soil health management for better agricultural outcomes. Understanding economic conditions for production and application is crucial to promote biochar use. Viability depends on production costs, market demand and their impact on farmers and the environment. Standardizing production techniques and use across soils and crops are vital for climate resilience and soil health. Biochar stores carbon, cuts greenhouse gases and enhances soil and plant growth, offering a potential “multi-win” situation for humanity.
Prospects
When considering the production and application of biochar, it is important to consider both the financial and environmental benefits. The development of tailored biochar technologies incorporating various organic and inorganic components holds the potential to improve soil quality, agricultural production and environmental remediation. In addition, the use of chemicals adsorbed on biochar and regeneration of biochar are promising avenues that require further investigation. Although the potential for carbon sequestration and greenhouse gas emissions reduction is recognized in many ecosystems, there is a significant gap in field-based assessments that should be addressed, particularly in the context of a changing climate. Additionally, current studies are aimed at proving that biochar is a green solution that has advantages for the environment and human health.
Although there isn’t much-published data on how biochar actually affects trace gas emissions in the real world, this information could have a big influence on the applications of biochar overall. Creating precise prediction models is essential to include this component in upcoming biochar projects. Although the production of biochar offers a promising solution for managing municipal solid waste, more research is needed to determine efficient production techniques, as well as to characterize and standardize the process across a range of crops. Conducting research on climate change over an extended period is imperative in evaluating the long-term impacts of applying biochar on soil health and crop productivity. These initiatives will help advance the comprehensive and wise application of biochar technology to promote a circular economy of agroecosystems.
Author contributions
PK: Conceptualization, Supervision, Writing – original draft, Writing – review & editing. SV: Writing – original draft, Writing – review & editing. MA: Writing – original draft, Writing – review & editing. SP: Writing – original draft, Writing – review & editing. CS: Writing – original draft, Writing – review & editing.
Funding not received
The author(s) declare that no financial support was received for the research, authorship, and/or publication of this article.
Acknowledgments
The authors acknowledge the Tamil Nadu Agricultural University, Coimbatore, Tamil Nadu, India, and Western Sydney University, Australia for providing library and web source facilities for the review paper. The authors also thank the Institutional Development Plan (IDP) – Indian Council of Agricultural Research (ICAR) – National Agricultural Higher Education Project (NAHEP) for funding to visit the Western Sydney University.
Conflict of interest
The authors declare that the research was conducted in the absence of any commercial or financial relationships that could be construed as a potential conflict of interest.
Publisher’s note
All claims expressed in this article are solely those of the authors and do not necessarily represent those of their affiliated organizations, or those of the publisher, the editors and the reviewers. Any product that may be evaluated in this article, or claim that may be made by its manufacturer, is not guaranteed or endorsed by the publisher.
References
1. Azad N, Behmanesh J, Rezaverdinejad V, Abbasi F, Navabian M. An analysis of optimal fertigation implications in different soils on reducing environmental impacts of agricultural nitrate leaching. Sci Rep. (2020) 10:1–15. doi: 10.1038/s41598-020-64856-x
2. Bai J, Song J, Chen D, Zhang Z, Yu Q, Ren G, et al. Biochar combined with N fertilization and straw return in wheat-maize agroecosystem: Key practices to enhance crop yields and minimize carbon and nitrogen footprints. Agricult Ecosyst Environ. (2023) 347:108366. doi: 10.1016/j.scitotenv.2021.152073
3. Banik C, Bakshi S, Laird DA, Smith RG, Brown RC. Impact of biochar-based slow-release N-fertilizers on maize growth and nitrogen recovery efficiency. J Environ Qual. (2023) 52(3):630–40. doi: 10.1002/jeq2.20468
4. Borchard N, Schirrmann M, Cayuela ML, Kammann C, Wrage-Mönnig N, Estavillo JM, et al. Biochar, soil and land-use interactions that reduce nitrate leaching and N2O emissions: a meta-analysis. Sci Total Environ. (2019) 651:2354–64. doi: 10.1016/j.scitotenv.2018.10.060
5. Chen ZS, Liu T, Dong JF, Chen G, Li Z, Zhou JL, et al. Sustainable application for agriculture using biochar-based slow-release fertilizers: A review. ACS Sustain Chem Eng. (2022) 11(1):1–12. doi: 10.1021/acssuschemeng.2c05691
6. Castejón-del Pino R, Cayuela ML, Sánchez-García M, Sánchez-Monedero MA. Nitrogen availability in biochar-based fertilizers depending on activation treatment and nitrogen source. Waste Manage. (2023) 158:76–83. doi: 10.1016/j.wasman.2023.01.007
7. Nsamba HK, Hale SE, Cornelissen G, Bachmann RT. Sustainable technologies for small-scale biochar production—a review. J Sustain Bioenergy Syst. (2015) 5(1):10–31. doi: 10.4236/jsbs.2015.51002
8. Abdul G, Zhu X, Chen B. Structural characteristics of biochar-graphene nanosheet composites and their adsorption performance for phthalic acid esters. Chem Eng J. (2017) 319:9–20. doi: 10.1016/j.cej.2017.02.074
9. Hussain Z, Khan N, Ullah S, Liaqat A, Nawaz F, Khalil AUR, et al. Response of mung bean to various levels of biochar, farmyard manure and nitrogen. World J Agric Sci. (2017) 13:pp.26–33. doi: 10.5829/idosi.wjas.2017.26.33
10. Lee JM, Park DG, Kang SS, Choi EJ, Gwon HS, Lee HS, et al. Short-term effect of biochar on soil organic carbon improvement and nitrous oxide emission reduction according to different soil characteristics in agricultural land: A laboratory experiment. Agronomy. (2022) 12:1879. doi: 10.3390/agronomy12081879
11. Wang J, Xiong Z, Kuzyakov Y. Biochar stability in soil: meta-analysis of decomposition and priming effects. GCB-Bioenergy. (2016) 8:512–23. doi: 10.1111/gcbb.12266
12. Chagas JKM, de Figueiredo CC, Ramos MLG. Biochar increases soil carbon pools: Evidence from a global meta-analysis. J Environ Manage. (2022) 305:114403. doi: 10.1016/j.jenvman.2021.114403
13. Liu Q, Zhang Y, Liu B, Amonette JE, Lin Z, Liu G, et al. How does biochar influence soil N cycle? A meta-analysis. Plant Soil. (2018) 426:211–25. doi: 10.1007/s11104-018-3619-4
14. Lyu H, Gao B, He F, Zimmerman AR, Ding C, Huang H, et al. Effects of ball milling on the physicochemical and sorptive properties of biochar: Experimental observations and governing mechanisms. Environ pollut. (2018) 233:54–63. doi: 10.1016/j.envpol.2017.10.037
15. Guenet B, Gabrielle B, Chenu C, Arrouays D, Balesdent J, Bernoux M, et al. Can N2O emissions offset the benefits from soil organic carbon storage? Global Change Biol. (2021) 27:237–56. doi: 10.1111/gcb.15342
16. Shaheen SM, El-Naggar A, Wang J, Hassan NE, Niazi NK, Wang H, et al. Biochar as an (Im) mobilizing agent for the potentially toxic elements in contaminated soils. Biochar Biomass waste. (2019) 14:255–74. doi: 10.1016/B978-0-12-811729-3.00014-5
17. He Q, Li X, Ren Y. Analysis of the simultaneous adsorption mechanism of ammonium and phosphate on magnesium-modified biochar and the slow release effect of fertiliser. Biochar. (2022) 4:25. doi: 10.1007/s42773-022-00150-5
18. Yang F, Zhang S, Sun Y, Du Q, Song J, Tsang DC. A novel electrochemical modification combined with one-step pyrolysis for preparation of sustainable thorn-like iron-based biochar composites. Bioresour Technol. (2019) 274:379–85. doi: 10.1016/j.biortech.2018.10.042
19. Jayarathna MKNW, Dharmakeerthi RS, Rathnayaka WMUK. Biochar based slow-release urea fertilizer: Production and assessing the effects on growth of lowland rice and nitrogen dynamics in an Alfisol. Trop Agric Res. (2021) 32:168–78. doi: 10.4038/tar.v32i2.8464
20. Jellali S, Khiari B, Al-Harrasi M, Charabi Y, Al-Sabahi J, Al-Abri M, et al. Industrial sludge conversion into biochar and reuse in the context of circular economy: Impact of pre-modification processes on pharmaceuticals removal from aqueous solutions. Sustain Chem Pharm. (2023) 33:101114. doi: 10.1016/j.jenvman.2021.114368
21. Liao J, Liu X, Hu A, Song H, Chen X, Zhang Z. Effects of biochar-based controlled release nitrogen fertilizer on nitrogen-use efficiency of oilseed rape (Brassica napus L.). Sci Rep. (2020) 10:1–14. doi: 10.1038/s41598-020-67528-y
22. Piveta LB, Noldin JA, Roma-Burgos N, Viana VE, Benedetti L, Pinto JJO, et al. Weedy rice (Oryza spp.) diversity in southern Brazil. Weed Sci. (2021) 69:547–57. doi: 10.1017/wsc.2021.23
23. Wang Y, Yin R, Liu R. Characterization of biochar from fast pyrolysis and its effect on chemical properties of the tea garden soil. J Anal Appl Pyrol. (2014) 110:375–81. doi: 10.1016/j.jaap.2014.10.006
24. El-Naggar A, Awad YM, Tang XY, Liu C, Niazi NK, Jien SH, et al. Biochar influences soil carbon pools and facilitates interactions with soil: A field investigation. Land degrad Dev. (2018) 29:2162–71. doi: 10.1002/ldr.2896
25. Pandian K, Subramaniayan P, Gnasekaran P, Chitraputhirapillai S. Effect of biochar amendment on soil physical, chemical and biological properties and groundnut yield in rainfed Alfisol of semi-arid tropics. Arch Agron Soil Sci. (2016) 62:1293–310. doi: 10.1080/03650340.2016.1139086
26. Kannan P, Paramasivan M, Marimuthu S, Swaminathan C, Bose J. Applying both biochar and phosphobacteria enhances Vigna mungo L. growth and yield in acid soils by increasing soil pH, moisture content, microbial growth and P availability. Agricult Ecosyst Environ. (2021) 308:107258. doi: 10.1016/j.agee.2020.107258
27. Ghorbani M, Asadi H, Abrishamkesh S. Effects of rice husk biochar on selected soil properties and nitrate leaching in loamy sand and clay soil. Int Soil Water Conserv Res. (2019) 7:258–65. doi: 10.1016/j.iswcr.2019.05.005
28. Luo X, Liu G, Xia Y, Chen L, Jiang Z, Zheng H, et al. Use of biochar-compost to improve properties and productivity of the degraded coastal soil in the Yellow River Delta, China. J Soils Sediments. (2017) 17:780–9. doi: 10.1007/s11368-016-1361-1
29. Simarani K, Azlan Halmi MF, Abdullah R. Short-term effects of biochar amendment on soil microbial community in humid tropics. Arch Agron Soil Sci. (2018) 64:1847–60. doi: 10.1080/03650340.2018.1464149
30. Oladele SO. Changes in physicochemical properties and quality index of an Alfisol after three years of rice husk biochar amendment in rainfed rice–Maize cropping sequence. Geoderma. (2019) 353:359–71. doi: 10.1016/j.geoderma.2019.06.038
31. D'Hose T, Debode J, De Tender C, Ruysschaert G, Vandecasteele B. Has compost with biochar applied during the process added value over biochar or compost for increasing soil quality in an arable cropping system? Appl Soil Ecol. (2020) 156:103706. doi: 10.1016/j.apsoil.2020.103706
32. Elangovan R, Sekaran NC. Biochar for soil health enhancement. Madras Agric J. (2017) 104(march:1. doi: 10.29321/MAJ2019.000213
33. Cai Y, Akiyama H. Effects of inhibitors and biochar on nitrous oxide emissions, nitrate leaching, and plant nitrogen uptake from urine patches of grazing animals on grasslands: a meta-analysis. Soil Sci Plant Nutr. (2017) 63:405–14. doi: 10.1080/00380768.2017.1367627
34. Singh Mavi M, Singh G, Singh BP, Singh Sekhon B, Choudhary OP, Sagi S, et al. Interactive effects of rice-residue biochar and N-fertilizer on soil functions and crop biomass in contrasting soils. J Soil Sci Plant Nutr. (2018) 18:41–59. doi: 10.4067/S0718-95162018005000201
35. Usman AR, Abduljabbar A, Vithanage M, Ok YS, Ahmad M, Ahmad M, et al. Biochar production from date palm waste: Charring temperature induced changes in composition and surface chemistry. J Anal Appl Pyrol. (2015) 115:392–400. doi: 10.1016/j.jaap.2015.08.016
36. Puga AP, Grutzmacher P, Cerri CEP, Ribeirinho VS, de Andrade CA. Biochar-based nitrogen fertilizers: Greenhouse gas emissions, use efficiency, and maize yield in tropical soils. Sci Total Environ. (2020) 704:135375. doi: 10.1016/j.scitotenv.2019.135375
37. Mustaffa MRAF, Pandian K, Chitraputhirapillai S, Kuppusamy S, Dhanushkodi K. Synthesis of biochar-embedded slow-release nitrogen fertilizers: Mesocosm and field scale evaluation for nitrogen use efficiency, growth and rice yield. Soil Use Manage. (2023) 40(1):1–17. doi: 10.1111/sum.12959
38. Shi W, Bian R, Li L, Lian W, Liu X, Zheng J, et al. Assessing the impacts of biochar-blended urea on nitrogen use efficiency and soil retention in wheat production. GCB Bioenergy. (2022) 14:65–83. doi: 10.1111/gcbb.12904
39. Xiao Y, Peng F, Zhang Y, Wang J, Zhuge Y, Zhang S, et al. Effect of bag-controlled release fertilizer on nitrogen loss, greenhouse gas emissions, and nitrogen applied amount in peach production. J Clean Product. (2019) 234:258–74. doi: 10.1016/j.jclepro.2019.06.219
40. Wang K, Hou J, Zhang S, Hu W, Yi G, Chen W, et al. Preparation of a new biochar-based microbial fertilizer: Nutrient release patterns and synergistic mechanisms to improve soil fertility. Sci Total Environ. (2023) 860:160478. doi: 10.1016/j.scitotenv.2022.160478
41. Stanley N, Mahanty B. Preparation and characterization of biogenic CaCO3-reinforced polyvinyl alcohol–alginate hydrogel as controlled-release urea formulation. Polym Bull. (2020) 77:529–40. doi: 10.1007/s00289-019-02763-6
42. Rafiq MK, Bachmann RT, Rafiq MT, Shang Z, Joseph S, Long R. Influence of pyrolysis temperature on physico-chemical properties of corn stover (Zea mays L.) biochar and feasibility for carbon capture and energy balance. PloS One. (2016) 11:e0156894. doi: 10.1371/journal.pone.0156894
43. Zhao H, Xie T, Xiao H, Gao M. Biochar-based fertilizer improved crop yields and N utilization efficiency in a maize–Chinese cabbage rotation system. Agriculture. (2022) 12:1030. doi: 10.3390/agriculture12071030
44. Li C, Zhao C, Zhao X, Wang Y, Lv X, Zhu X, et al. Beneficial effects of biochar application with nitrogen fertilizer on soil nitrogen retention, absorption and utilization in maize production. Agronomy. (2023) 13:113. doi: 10.3390/agronomy13010113
45. Prapagdee S, Tawinteung N. Effects of biochar on enhanced nutrient use efficiency of green bean, Vigna radiata L. Environ Sci pollut Res. (2017) 24:9460–7. doi: 10.1007/s11356-017-8633-1
46. Zhou S, Jiang Z, Shen J, Yao Q, Yang X, Li X, et al. Biochar-amended compost as a promising soil amendment for enhancing plant productivity: A meta-analysis study. Sci Total Environ. (2023) 879:163067. doi: 10.1016/j.scitotenv.2023.163067
47. Oladele S, Adeyemo A, Adegaiye A, Awodun M. Effects of biochar amendment and nitrogen fertilization on soil microbial biomass pools in an Alfisol under rain-fed rice cultivation. Biochar. (2019) 1:163–76. doi: 10.1007/s42773-019-00017-2
48. Lateef A, Nazir R, Jamil N, Alam S, Shah R, Khan MN, et al. Synthesis and characterization of environmental friendly corncob biochar based nano-composite–A potential slow release nano-fertilizer for sustainable agriculture. Environ Nanotechnol Monit Manage. (2019) 11:100212. doi: 10.1016/j.enmm.2019.100212
49. Karimi A, Moezzi A, Chorom M, Enayatizamir N. Application of biochar changed the status of nutrients and biological activity in a calcareous soil. J Soil Sci Plant Nutr. (2020) 20:450–9. doi: 10.1007/s42729-019-00129-5
50. Sarfaraz Q, Silva LSD, Drescher GL, Zafar M, Severo FF, Kokkonen A, et al. Characterization and carbon mineralization of biochars produced from different animal manures and plant residues. Sci Rep. (2020) 10:p.955. doi: 10.1038/s41598-020-57987-8
51. Ajayi AE, Horn R. Modification of chemical and hydrophysical properties of two texturally differentiated soils due to varying magnitudes of added biochar. Soil Tillage Res. (2016) 164:34–44. doi: 10.1016/j.still.2016.01.011
52. Weng Z, Van Zwieten L, Singh BP, Tavakkoli E, Joseph S, Macdonald LM, et al. Biochar built soil carbon over a decade by stabilizing rhizodeposits. Nat Climate Change. (2017) 7:371–6. doi: 10.1038/nclimate3276
53. Sánchez-García M, Sánchez-Monedero MA, Roig A, López-Cano I, Moreno B, Benitez E, et al. Compost vs biochar amendment: a two-year field study evaluating soil C build-up and N dynamics in an organically managed olive crop. Plant Soil. (2016) 408:1–14. doi: 10.1007/s11104-016-2794-4
54. Zheng Y, Zimmerman AR, Gao B. Comparative investigation of characteristics and phosphate removal by engineered biochars with different loadings of magnesium, aluminum, or iron. Sci Total Environ. (2020) 747:141277. doi: 10.1016/j.scitotenv.2020.141277
55. Kätterer T, Roobroeck D, Andrén O, Kimutai G, Karltun E, Kirchmann H, et al. Biochar addition persistently increased soil fertility and yields in maize-soybean rotations over 10 years in sub-humid regions of Kenya. Field Crops Res. (2019) 235:18–26. doi: 10.1016/j.fcr.2019.02.015
56. Krause HM, Hüppi R, Leifeld J, El-Hadidi M, Harter J, Kappler A, et al. Biochar affects community composition of nitrous oxide reducers in a field experiment. Soil Biol Biochem. (2018) 119:143–51. doi: 10.1016/j.soilbio.2018.01.018
57. Jin Z, Chen X, Chen C, Tao P, Han Z, Zhang X. Biochar impact on nitrate leaching in upland red soil, China. Environ Earth Sci. (2016) 75:1–10. doi: 10.1007/s12665-016-5906-9
58. Abujabhah IS, Doyle R, Bound SA, Bowman JP. The effect of biochar loading rates on soil fertility, soil biomass, potential nitrification, and soil community metabolic profiles in three different soils. J soils sediments. (2016) 16:2211–22. doi: 10.1007/s11368-016-1411-8
59. Maikol N, Haruna AO, Maru A, Asap A, Medin S. Utilization of urea and chicken litter biochar to improve rice production. Sci Rep. (2021) 11:9955. doi: 10.1038/s41598-021-89332-y
60. Chew J, Zhu L, Nielsen S, Graber E, Mitchell DR, Horvat J, et al. Biochar-based fertilizer: supercharging root membrane potential and biomass yield of rice. Sci Total Environ. (2020) 713:136431. doi: 10.1016/j.scitotenv.2019.136431
61. Hagner M, Kemppainen R, Jauhiainen L, Tiilikkala K, Setälä H. The effects of birch (Betula spp.) biochar and pyrolysis temperature on soil properties and plant growth. Soil tillage Res. (2016) 163:224–34. doi: 10.1016/j.still.2016.06.006
62. Haider G, Steffens D, Müller C, Kammann CI. Standard extraction methods may underestimate nitrate stocks captured by field-aged biochar. J Environ Qual. (2016) 45:1196–204. doi: 10.2134/jeq2015.10.0529
63. Edenborn SL, Edenborn HM, Krynock RM, Haug KZ. Influence of biochar application methods on the phytostabilization of a hydrophobic soil contaminated with lead and acid tar. J Environ Manage. (2015) 150:226–34. doi: 10.1016/j.jenvman.2014.11.023
64. Amin AEEAZ, Eissa MA. Biochar effects on nitrogen and phosphorus use efficiencies of zucchini plants grown in a calcareous sandy soil. J Soil Sci Plant Nutr. (2017) 17:912–21. doi: 10.4067/S0718-95162017000400006
65. Zhang H, Marchant-Forde JN, Zhang X, Wang Y. Effect of cornstalk biochar immobilized bacteria on ammonia reduction in laying hen manure composting. Molecules. (2020) 25:1560. doi: 10.3390/molecules25071560
66. Zhang S, Gao N, Shen T, Yang Y, Gao B, Li YC, et al. One-step synthesis of superhydrophobic and multifunctional nano copper-modified bio-polyurethane for controlled-release fertilizers with “multilayer air shields”: new insight of improvement mechanism. J Mater Chem A. (2019) 7:9503–9. doi: 10.1039/C9TA00632J
67. Jiang X, Denef K, Stewart CE, Cotrufo MF. Controls and dynamics of biochar decomposition and soil microbial abundance, composition, and carbon use efficiency during long-term biochar-amended soil incubations. Biol Fertil Soils. (2016) 52:1–14. doi: 10.1007/s00374-015-1047-7
68. Han G, Lan J, Chen Q, Yu C, Bie S. Response of soil microbial community to application of biochar in cotton soils with different continuous cropping years. Sci Rep. (2017) 7:1–11. doi: 10.1038/s41598-017-10427-6
69. Pandey V, Patel A, Patra DD. Biochar ameliorates crop productivity, soil fertility, essential oil yield and aroma profiling in basil (Ocimum basilicum L.). Ecol Eng. (2016) 90:361–6. doi: 10.1016/j.ecoleng.2016.01.020
70. Agegnehu G, Nelson PN, Bird MI. Crop yield, plant nutrient uptake and soil physicochemical properties under organic soil amendments and nitrogen fertilization on Nitisols. Soil Tillage Res. (2016) 160:1–13. doi: 10.1016/j.still.2016.02.003
71. Berihun T, Tolosa S, Tadele M, Kebede F. Effect of biochar application on growth of garden pea (Pisum sativum L.) in acidic soils of Bule Woreda Gedeo Zone Southern Ethiopia. Int J Agron. (2017) 2017:1–9. doi: 10.1155/2017/6827323
72. Maghsoodi MR, Najafi N, Reyhanitabar A, Oustan S. Hydroxyapatite nanorods, hydrochar, biochar, and zeolite for controlled-release urea fertilizers. Geoderma. (2020) 379:114644. doi: 10.1016/j.geoderma.2020.114644
73. Eazhilkrishna N, Thilagavathi T, Baskar M. Effect of nutrient enriched biochar on yield and NPK uptake of maize grown in alfisol. Int J Curr Microbiol Appl Sci. (2017) 6:1326–34. doi: 10.20546/ijcmas.2017.607.159
74. Moe K, Htwe AZ, Dien DC, Kajihara Y, Yamakawa T. Effects of organic fertilizer applied using the estimated mineralizable nitrogen method on nitrogen uptake, growth characteristics, yield, and yield components of Genkitsukushi rice (Oryza sativa). J Plant Nutr. (2020) 43:1400–17. doi: 10.1080/01904167.2020.1730893
75. Mahmoud E, El-Beshbeshy T, El-Kader NA, El Shal R, Khalafallah N. Impacts of biochar application on soil fertility, plant nutrients uptake and maize (Zea mays L.) yield in saline sodic soil. Arab J Geosci. (2019) 12:1–9. doi: 10.1007/s12517-019-4937-4
76. Haddad K, Jeguirim M, Jellali S, Thevenin N, Ruidavets L, Limousy L. Biochar production from Cypress sawdust and olive mill wastewater: Agronomic approach. Sci Total Environ. (2021) 752:141713. doi: 10.1016/j.scitotenv.2020.141713
77. Wen P, Wu Z, Han Y, Cravotto G, Wang J, Ye BC. Microwave-assisted synthesis of a novel biochar-based slow-release nitrogen fertilizer with enhanced water-retention capacity. ACS Sustain Chem Eng. (2017) 5:7374–82. doi: 10.1021/acssuschemeng.7b01721
78. Munda S, Bhaduri D, Mohanty S, Chatterjee D, Tripathi R, Shahid M, et al. Dynamics of soil organic carbon mineralization and C fractions in paddy soil on application of rice husk biochar. Biomass Bioenergy. (2018) 115:1–9. doi: 10.1016/j.biombioe.2018.04.002
79. Wu D, Feng Y, Xue L, Liu M, Yang B, Hu F, et al. Biochar combined with vermicompost increases crop production while reducing ammonia and nitrous oxide emissions from a paddy soil. Pedosphere. (2019) 29:82–94. doi: 10.1016/S1002-0160(18)60050-5
80. Ahmad Z, Gao B, Mosa A, Yu H, Yin X, Bashir A, et al. Removal of Cu (II), Cd (II) and Pb (II) ions from aqueous solutions by biochars derived from potassium-rich biomass. J clean product. (2018) 180:437–49. doi: 10.1016/j.jclepro.2018.01.133
81. Oliveira GA, Gevaerd A, Mangrich AS, Marcolino-Junior LH, Bergamini MF. Biochar obtained from spent coffee grounds: Evaluation of adsorption properties and its application in a voltammetric sensor for lead (II) ions. Microchem J. (2021) 165:106114. doi: 10.1016/j.microc.2021.106114
82. Qin P, Wang H, Yang X, He L, Müller K, Shaheen SM, et al. Bamboo-and pig-derived biochars reduce leaching losses of dibutyl phthalate, cadmium, and lead from co-contaminated soils. Chemosphere. (2018) 198:450–9. doi: 10.1016/j.chemosphere.2018.01.162
83. Duan R, Hu HQ, Fu QL, Kou CL. Remediation of Cd/Ni contaminated soil by biochar and oxalic acid activated phosphate rock. Huan jing ke xue= Huanjing kexue. (2017) 38:4836–43. doi: 10.13227/j.hjkx.201704028
84. Tan Z, Wang Y, Zhang L, Huang Q. Study of the mechanism of remediation of Cd-contaminated soil by novel biochars. Environ Sci pollut Res. (2017) 24:24844–55. doi: 10.1007/s11356-017-0109-9
85. Shen Z, Zhang J, Hou D, Tsang DC, Ok YS, Alessi DS. Synthesis of MgO-coated corncob biochar and its application in lead stabilization in a soil washing residue. Environ Int. (2019) 122:357–62. doi: 10.1016/j.envint.2018.11.045
86. Son EB, Poo KM, Chang JS, Chae KJ. Heavy metal removal from aqueous solutions using engineered magnetic biochars derived from waste marine macro-algal biomass. Sci Total Environ. (2018) 615:161–8. doi: 10.1016/j.scitotenv.2017.09.171
87. Meng J, Tao M, Wang L, Liu X, Xu J. Changes in heavy metal bioavailability and speciation from a Pb-Zn mining soil amended with biochars from co-pyrolysis of rice straw and swine manure. Sci Total Environ. (2018) 633:300–7. doi: 10.1016/j.scitotenv.2018.03.199
88. Marcińczyk M, Krasucka P, Duan W, Pan B, Siatecka A, Oleszczuk P. Ecotoxicological characterization of engineered biochars produced from different feedstock and temperatures. Sci Total Environ. (2023) 861:160640. doi: 10.1016/j.jclepro.2022.130685
89. Lei S, Shi Y, Qiu Y, Che L, Xue C. Performance and mechanisms of emerging animal-derived biochars for immobilization of heavy metals. Sci Total Environ. (2019) 646:1281–9. doi: 10.1016/j.scitotenv.2018.07.374
90. Ni BJ, Huang QS, Wang C, Ni TY, Sun J, Wei W. Competitive adsorption of heavy metals in aqueous solution onto biochar derived from anaerobically digested sludge. Chemosphere. (2019) 219:351–7. doi: 10.1016/j.chemosphere.2018.12.053
91. Song J, Zhang S, Li G, Du Q, Yang F. Preparation of montmorillonite modified biochar with various temperatures and their mechanism for Zn ion removal. J hazard mater. (2020) 391:121692. doi: 10.1016/j.jhazmat.2019.121692
92. Bayu D, Dejene A, Alemayehu R, Gezahegn B. Improving available phosphorus in acidic soil using biochar. J Soil Sci Environ Manage. (2017) 8:pp.87–94. doi: 10.5897/JSSEM2015.0540
93. Wang J, Wang S. Preparation, modification and environmental application of biochar: A review. J Clean Product. (2019) 227:1002–22. doi: 10.1016/j.jclepro.2019.04.282
94. Alam MS, Gorman-Lewis D, Chen N, Flynn SL, Ok YS, Konhauser KO, et al. Thermodynamic analysis of nickel (II) and zinc (II) adsorption to biochar. Environ Sci Technol. (2018) 52:6246–55. doi: 10.1021/acs.est.7b06261
95. Bashir S, Hussain Q, Shaaban M, Hu H. Efficiency and surface characterization of different plant derived biochar for cadmium (Cd) mobility, bioaccessibility and bioavailability to Chinese cabbage in highly contaminated soil. Chemosphere. (2018) 211:632–9. doi: 10.1016/j.chemosphere.2018.07.168
96. Ho SH, Zhu S, Chang JS. Recent advances in nanoscale-metal assisted biochar derived from waste biomass used for heavy metals removal. Bioresour Technol. (2017) 246:123–34. doi: 10.1016/j.biortech.2017.08.061
97. Huang X, Liu Y, Liu S, Tan X, Ding Y, Zeng G, et al. Effective removal of Cr (VI) using β-cyclodextrin–chitosan modified biochars with adsorption/reduction bifuctional roles. RSC Adv. (2016) 6:94–104. doi: 10.1039/C5RA22886G
98. Zama EF, Zhu YG, Reid BJ, Sun GX. The role of biochar properties in influencing the sorption and desorption of Pb (II), Cd (II) and As (III) in aqueous solution. J clean product. (2017) 148:127–36. doi: 10.1016/j.jclepro.2017.01.125
99. Oo AZ, Sudo S, Akiyama H, Win KT, Shibata A, Yamamoto A, et al. Effect of dolomite and biochar addition on N2O and CO2 emissions from acidic tea field soil. PloS One. (2018) 13:e0192235. doi: 10.1371/journal.pone.0192235
100. Saarnio S. Impacts of biochar amendment on greenhouse gas emissions from agricultural soils. Agric Environ Appl biochar: Adv barriers. (2016) 63:259–93. doi: 10.2136/sssaspecpub63.2014.0045
101. Tu C, Wei J, Guan F, Liu Y, Sun Y, Luo Y. Biochar and bacteria inoculated biochar enhanced Cd and Cu immobilization and enzymatic activity in a polluted soil. Environ Int. (2020) 137:105576. doi: 10.1016/j.envint.2020.105576
102. Awasthi MK, Duan Y, Awasthi SK, Liu T, Zhang Z, Kim SH, et al. Effect of biochar on emission, maturity and bacterial dynamics during sheep manure compositing. Renewable Energy. (2020) 152:421–9. doi: 10.1016/j.renene.2020.01.065
103. Mao H, Lv Z, Sun H, Li R, Zhai B, Wang Z, et al. Improvement of biochar and bacterial powder addition on gaseous emission and bacterial community in pig manure compost. Bioresour Technol. (2018) 258:195–202. doi: 10.1016/j.biortech.2018.02.082
104. Chen Y, Zhang X, Chen W, Yang H, Chen H. The structure evolution of biochar from biomass pyrolysis and its correlation with gas pollutant adsorption performance. Bioresour Technol. (2017) 246:101–9. doi: 10.1016/j.biortech.2017.08.138
105. Awasthi MK, Wang Q, Chen H, Wang M, Ren X, Zhao J, et al. Evaluation of biochar amended biosolids co-composting to improve the nutrient transformation and its correlation as a function for the production of nutrient-rich compost. Bioresour Technol. (2017) 237:156–66. doi: 10.1016/j.biortech.2017.01.044
106. Agyarko-Mintah E, Cowie A, Van Zwieten L, Singh BP, Smillie R, Harden S, et al. Biochar lowers ammonia emission and improves nitrogen retention in poultry litter composting. Waste Manage. (2017) 61:129–37. doi: 10.1016/j.wasman.2016.12.009
107. Vandecasteele B, Sinicco T, D'Hose T, Nest TV, Mondini C. Biochar amendment before or after composting affects compost quality and N losses, but not P plant uptake. J Environ Manage. (2016) 168:200–9. doi: 10.1016/j.jenvman.2015.11.045
108. Chowdhury MA, de Neergaard A, Jensen LS. Potential of aeration flow rate and bio-char addition to reduce greenhouse gas and ammonia emissions during manure composting. Chemosphere. (2014) 97:16–25. doi: 10.1016/j.chemosphere.2013.10.030
109. Shaaban M, Wu Y, Khalid MS, Peng QA, Xu X, Wu L, et al. Reduction in soil N2O emissions by pH manipulation and enhanced nosZ gene transcription under different water regimes. Environ pollut. (2018) 235:625–31. doi: 10.1016/j.envpol.2017.12.066
110. Feng Z, Zhu L. Impact of biochar on soil N2O emissions under different biochar-carbon/fertilizer-nitrogen ratios at a constant moisture condition on a silt loam soil. Sci Total Environ. (2017) 584:776–82. doi: 10.1016/j.scitotenv.2017.01.115
111. Ameloot N, Maenhout P, De Neve S, Sleutel S. Biochar-induced N2O emission reductions after field incorporation in a loam soil. Geoderma. (2016) 267:10–6. doi: 10.1016/j.geoderma.2015.12.016
112. Cayuela ML, Jeffery S, van Zwieten L. The molar H: Corg ratio of biochar is a key factor in mitigating N2O emissions from soil. Agricult Ecosyst Environ. (2015) 202:135–8. doi: 10.1016/j.agee.2014.12.015
113. Xu X, Zhao Y, Sima J, Zhao L, Mašek O, Cao X. Indispensable role of biochar-inherent mineral constituents in its environmental applications: A review. Bioresour Technol. (2017) 241:887–99. doi: 10.1016/j.biortech.2017.06.023
114. Harter J, Weigold P, El-Hadidi M, Huson DH, Kappler A, Behrens S. Soil biochar amendment shapes the composition of N2O-reducing microbial communities. Sci Total Environ. (2016) 562:379–90. doi: 10.1016/j.scitotenv.2016.03.220
115. Lin Y, Ding W, Liu D, He T, Yoo G, Yuan J, et al. Wheat straw-derived biochar amendment stimulated N2O emissions from rice paddy soils by regulating the amoA genes of ammonia-oxidizing bacteria. Soil Biol Biochem. (2017) 113:89–98. doi: 10.1016/j.soilbio.2017.06.001
116. Huppi R, Felber R, Neftel A, Six J, Leifeld J. Effect of biochar and liming on soil nitrous oxide emissions from a temperate maize cropping system. SOIL. (2015) 1:707–17. doi: 10.5194/soil-1-707-2015
117. Yan M, Tian H, Song S, Tan HT, Lee JT, Zhang J, et al. Effects of digestate-encapsulated biochar on plant growth, soil microbiome and nitrogen leaching. J Environ Manage. (2023) 334:117481. doi: 10.1016/j.jenvman.2023.117481
118. Sun X, Han X, Ping F, Zhang L, Zhang K, Chen M, et al. Effect of rice-straw biochar on nitrous oxide emissions from paddy soils under elevated CO2 and temperature. Sci total Environ. (2018) 628:1009–16. doi: 10.1016/j.scitotenv.2018.02.046
119. Rombel A, Krasucka P, Oleszczuk P. Sustainable biochar-based soil fertilizers and amendments as a new trend in biochar research. Sci total Environ. (2022) 816:151588. doi: 10.1016/j.scitotenv.2021.151588
120. Rathnayake D, Schmidt HP, Leifeld J, Mayer J, Epper CA, Bucheli TD, et al. Biochar from animal manure: A critical assessment on technical feasibility, economic viability, and ecological impact. GCB Bioenergy. (2023) 15:1078–104. doi: 10.1111/gcbb.13082
121. Panwar NL, Pawar A, Salvi BL. Comprehensive review on production and utilization of biochar. SN Appl Sci. (2019) 1:1–19. doi: 10.1007/s42452-019-0172-6
122. Zhou Q, BA H, Tong Z, Gao B, Liu G. An in-situ technique for producing low-cost agricultural biochar. Pedosphere. (2018) 28:690–5. doi: 10.1016/S1002-0160(17)60482-X
123. Bhatt N, Buddhi D, Suthar S. Synthesizing biochar-based slow-releasing fertilizers using vermicompost leachate, cow dung, and plant weed biomass. J Environ Manage. (2023) 326:116782. doi: 10.1016/j.jenvman.2022.116782
124. Heinrich T, Park H, Orozco R, Ding Z, Álvarez-López V, Mosquera-Losada MR, et al. Biochar production from late-harvest grass–Challenges and potential for farm-scale implementation. Sustain Product Consumpt. (2023) 37:256–67. doi: 10.1016/j.spc.2023.02.019
125. Kochanek J, Soo RM, Martinez C, Dakuidreketi A, Mudge AM. Biochar for intensification of plant-related industries to meet productivity, sustainability and economic goals: A review. Resour Conserv Recycling. (2022) 179:106109. doi: 10.1016/j.resconrec.2021.106109
126. Snekapriya VK, Jayachandran M. Effect of sugarcane trash biochar on growth and yield of sugarcane seed crop. Madras Agric J. (2018) 105(march:1. doi: 10.29321/MAJ.2018.000119
127. Karthikeyan B, Saliha BB, Kannan P, Vellaikumar S. Effect of biochar composite and organic sources on soil properties and yield of bhendi (Abelmoschus esculentus L.). J Appl Nat Sci. (2021) 13:1198–205. doi: 10.31018/jans.v13i4.2972
128. Zhang M, Liu Y, Wei Q, Liu L, Gu X, Gou J, et al. Chemical fertilizer reduction combined with biochar application ameliorates the biological property and fertilizer utilization of pod pepper. Agronomy. (2023) 13:1616. doi: 10.3390/agronomy13061616
Keywords: biochar, soil conditioner, soil health, heavy metal pollution, climate change, GHGs, crop yield
Citation: Pandian K, Vijayakumar S, Mustaffa MRAF, Subramanian P and Chitraputhirapillai S (2024) Biochar – a sustainable soil conditioner for improving soil health, crop production and environment under changing climate: a review. Front. Soil Sci. 4:1376159. doi: 10.3389/fsoil.2024.1376159
Received: 25 January 2024; Accepted: 02 April 2024;
Published: 07 May 2024.
Edited by:
Jay Prakash Verma, Banaras Hindu University, IndiaReviewed by:
Adnane Beniaich, Mohammed VI Polytechnic University, MoroccoAvanthi Deshani Igalavithana, University of South Australia, Australia
Copyright © 2024 Pandian, Vijayakumar, Mustaffa, Subramanian and Chitraputhirapillai. This is an open-access article distributed under the terms of the Creative Commons Attribution License (CC BY). The use, distribution or reproduction in other forums is permitted, provided the original author(s) and the copyright owner(s) are credited and that the original publication in this journal is cited, in accordance with accepted academic practice. No use, distribution or reproduction is permitted which does not comply with these terms.
*Correspondence: Shanmugam Vijayakumar, dmlqaXRuYXVAZ21haWwuY29t; Kannan Pandian, a2FubmFuLnBAdG5hdS5hYy5pbg==