- Departamento de Bioquímica y Biología Molecular, Campus de Excelencia Internacional Agroalimentario CEIA3, Universidad de Córdoba, Córdoba, Spain
The marine picocyanobacterium Synechococcus sp. WH7803 possesses two glutamine synthetases (GSs; EC 6.3.1.2), GSI encoded by glnA and GSIII encoded by glnN. This is the first work addressing the physiological regulation of both enzymes in a marine cyanobacterial strain. The increase of GS activity upon nitrogen starvation was similar to that found in other model cyanobacteria. However, an unusual response was found when cells were grown under darkness: the GS activity was unaffected, reflecting adaptation to the environment where they thrive. On the other hand, we found that GSIII did not respond to nitrogen availability, in sharp contrast with the results observed for this enzyme in other cyanobacteria thus far studied. These features suggest that GS activities in Synechococcus sp. WH7803 represent an intermediate step in the evolution of cyanobacteria, in a process of regulatory streamlining where GSI lost the regulation by light, while GSIII lost its responsiveness to nitrogen. This is in good agreement with the phylogeny of Synechococcus sp. WH7803 in the context of the marine cyanobacterial radiation.
Introduction
A significant proportion of phytoplankton biomass production in oligotrophic seas is contributed by unicellular cyanobacteria of the genera Synechococcus and Prochlorococcus (Partensky et al., 1999; Flombaum et al., 2013; Biller et al., 2015). The majority of Synechococcus strains are capable of utilizing oxidized forms of nitrogen, nitrate, and nitrite (Scanlan et al., 2009), whereas the majority of Prochlorococcus are not (López-Lozano et al., 2002; Moore et al., 2002; Berube et al., 2015). The use of different nitrogen (N) sources by these marine picocyanobacteria is of particular interest because there is ecophysiological and genomic evidence for the critical role that nutrition plays in the ecology and evolution of these organisms (Scanlan, 2003; Flombaum et al., 2013; Biller et al., 2015; Kent et al., 2016). The spatial distribution of these two groups of marine picocyanobacteria depends on different factors such as nutrients or temperature (Partensky et al., 1999; Johnson et al., 2006; Zinser et al., 2006; Zwirglmaier et al., 2007; Pittera et al., 2014; Kent et al., 2016). Synechococcus spp. thrives in mesotrophic and moderately oligotrophic waters where they are able to exploit both oxidized and reduced forms of nitrogen. On the other hand, Prochlorococcus spp. are much more abundant in the most highly stratified, nutrient-poor waters, where they displace Synechococcus spp. as the dominant picocyanobacterial group.
Glutamine synthetase (GS; EC 6.3.1.2) catalyzes glutamine synthesis from glutamate and ammonia at the expenditure of ATP. Glutamine is then used by glutamate synthase (GOGAT) to synthesize glutamate by transferring an amide group to 2-oxoglutarate. The net glutamate molecule produced in each GS/GOGAT cycle is the way to incorporate nitrogen into various organic compounds such as other amino acids, purines, pyrimidines, and amino sugars. Two different types of GS have been found in cyanobacteria. Most strains have only one GS (known as GS type I, GSI), encoded by glnA. Prokaryotic GSI is composed of 12 identical subunits of about 50 kDa each (Valentine et al., 1968; Yamashita et al., 1989). Some cyanobacterial strains have, in addition to GSI, an hexameric GS (known as GS type III, GSIII), encoded by glnN (Reyes and Florencio, 1994; García-Domínguez et al., 1997; Reyes et al., 1997; Crespo et al., 1998; Sauer et al., 2000; Florencio and Reyes, 2002). Although these two types of GS are quite different in amino acid sequence, they share five domains that can be found in all known GSs (Reyes and Florencio, 1994; Crespo et al., 1998). GSIII, initially identified in an anaerobic bacterium that grows in mammalian intestines Bacteroides fragilis (Hill et al., 1989), is composed of six identical subunits, each of 75 kDa with little homology to GSI. This GS was the most recently discovered family among GSs and thus has been described in a limited number of bacteria including a few cyanobacterial strains, but not in eukaryotes.
The role of GSIII in cyanobacterial nitrogen metabolism is a subject that requires further investigation. Induction of GSIII under conditions of nitrogen deficiency is found in several cyanobacterial strains where GSIII coexists with GSI (Reyes and Florencio, 1994; García-Domínguez et al., 1997; Sauer et al., 2000), suggesting that the presence of GSIII may be required under this condition. The recovery rate after prolonged nitrogen starvation was negatively affected in a glnN mutant in Synechococcus PCC 7942 (Sauer et al., 2000), but the role of GSIII in that recovery remains unclear.
While nitrogen metabolism in freshwater cyanobacteria has been studied for several decades and is well known (Herrero et al., 2001; Muro-Pastor and Florencio, 2003; Flores and Herrero, 2005; Muro-Pastor et al., 2005; Luque and Forchhammer, 2008), there are very scarce studies on physiological nitrogen assimilation in marine picocyanobacteria due to their comparatively recent isolation, and their difficult culture in the laboratory. Several physiological studies in marine Synechococcus strains (Lindell et al., 1998, 2002; Collier et al., 1999; Lindell and Post, 2001; Bird and Wyman, 2003; Wyman and Bird, 2007; Collier and Lovindeer, 2012) and in Prochlorococcus (El Alaoui et al., 2001; Gómez-Baena et al., 2001, 2006; Lindell et al., 2002; López-Lozano et al., 2002, 2009; Moore et al., 2002; Kamennaya et al., 2008; Kamennaya and Post, 2011; McDonagh et al., 2012; Berube et al., 2015) have been published, but little information about the physiological behavior of GSI and GSIII from Synechococcus sp. WH7803 is available.
In the present work, we have studied the physiological regulation of GSI and GSIII in cultures of Synechococcus sp. WH7803 subjected to different ecological conditions such as nutrient limitation, different nitrogen sources or darkness. We also assessed the effect of L-methionine sulfoximine (MSX), an inhibitor of GS. We measured GS transferase activity, the concentration of both enzymes by Western blotting, and gene expression. We found that GSI from Synechococcus sp. WH7803 responds to nitrogen deficiency although an unusual response was found when cells were grown in darkness. Under the conditions tested in this study GSIII did not show any specific role in the assimilation of ammonium.
Materials and Methods
Culture Conditions
Synechococcus sp. WH7803 was grown in a chemically defined artificial seawater (ASW) medium (Moore et al., 2007). Cells were grown in polycarbonate Nalgene flasks (10 L), in a culture room under continuous blue light at 40 μE m-2 s-1 and 24°C. Growth was determined by measuring the absorbance of cultures at 550 nm.
Cell Collection
Cells were collected when cultures reached A550 of 0.1–0.12 (exponential phase of growth), by centrifugation at 26,000 g for 8 min at 4°C using an Avanti J-25 Beckman centrifuge equipped with a JA-14 rotor. To analyze the effect of different sources of nitrogen, the pellet was washed with ASW medium without nitrogen and then resuspended in the same medium and supplemented with different nitrogen sources. For the experiments requiring darkness, culture bottles were completely covered with two layers of aluminum foil. A total of 100 μM MSX (Sigma) was dissolved in culture media. After pouring most of the supernatant and carefully pipetting out the remaining medium, the pellet for protein assays was resuspended in Tris–HCl 50 mM pH 7.5 and for RNA assays in 10 mM sodium acetate (pH 4.5), 200 mM sucrose and 5 mM EDTA. The pellet obtained was frozen at -80°C.
Preparation of Cell Extracts
Cell extracts were broken with glass beads. After thawing, the samples were centrifuged at 16,900 g for 10 min and 4°C. The supernatants were poured and the pellets were resuspended in 250 μL 50 mM Tris–HCl pH 7.5. These suspensions were mixed with 140.6 mg glass beads B. Braun Melsungen AG (0.10–0.11 mm diameter). After that, five cycles of 3 min vortex–3 min ice were done. The mixtures were centrifuged at 16,900 g for 5 min at 4°C. Finally the supernatants were used for enzymatic activities. For western blotting some changes were introduced in the protocol in order to increase the concentration of the sample: the pellet was resuspended in 50 μL 50 mM Tris–HCl pH 7.5 and mixed with 28 mg glass beads B. Braun Melsungen AG (0.10–0.11 mm of diameter).
GS Enzymatic Assays
Glutamine synthetase transferase activity was determined as previously described (El Alaoui et al., 2001). The composition of the reaction mixture was: 100 mM glutamine, 10 mM sodium hydroxylamine, 50 μM manganese chloride, 10 μM ADP, and 50 mM sodium arsenate in 0.2 M 3-(N-morpholino)propanesulfonic acid (MOPS) pH 7. One unit of enzymatic activity is the amount of enzyme that transforms 1 μmol of substrate per minute.
Protein concentration was determined using the Bio-Rad Protein Assay kit, based on the method described by Bradford (1976).
Detection of GSI and GSIII by Western Blotting
Sodium dodecyl sulphate (SDS) electrophoresis was performed on a Mini-Protean III system (Bio-Rad) using 12% polyacrylamide resolving gels and 4% polyacrylamide stacking gels, loading 30 μg protein per lane. Proteins were transferred to a nitrocellulose membrane (Sigma) utilizing a semidry Trans-Blot SD System (Bio-Rad), for 1 h at 100 mA. After transfer the membrane was treated as follows: staining with 0.2% (w/v) Ponceau S in 5 % (v/v) acetic acid to check for equivalent protein loading and transfer efficiency; washing for 15 min with Tris-Buffered Saline and Tween 20 (TBS-T) (20 mM Tris–HCl pH 7.4, 150 mM NaCl, and 0.1% Tween 20). Blocking with TBS-T containing 1% (w/v) bovine serum albumin for 2 h and washing threefold for 15 min with TBS-T; incubation with primary antibody (anti-GSI from Synechocystis PCC 6803 produced in rabbit) diluted 1:5000 (v/v) in TBS-T 1% (w/v) bovine serum albumin overnight, with shaking at 4°C. Washing threefold for 15 min with TBS-T Buffer; incubation with secondary antibody (anti-immunoglobulin from rabbit produced in goat, linked with peroxidase, Sigma) diluted 1:4000 (v/v) in TBS-T for 20 min with moderate shaking at room temperature. Washing threefold for 15 min with TBS-T Buffer. Then, the immunoreacting material was detected with ECL Plus Western Blotting Detection System (Amersham), adding 1 mL solution A supplemented with 25 μL solution B. Chemiluminescent signal was detected using a LAS-3000 camera (Fujifilm) and images analyzed using Multi-Gauge V3.0 (Fujifilm).
Western blotting of GSIII was carried out following the protocol described above for GSI with these modifications: incubation with primary antibody (anti-GSIII from Synechocystis PCC 6803) diluted 1/4000 (v/v) in TBS-T 1% bovine serum albumin overnight, with shaking at 4°C. The incubation with secondary antibody (anti-immunoglobulin from rabbit, linked with peroxidase, Sigma) diluted 1:2000 (v/v) in TBS-T for 30 min with moderate shaking at room temperature.
RNA Isolation
RNA was isolated from 500 mL culture samples. Cells were harvested as described above. Total RNA was extracted using TRIsure RNA Isolation Reagent (Bioline) following the manufacturer’s recommendations, with the addition of 133 μL 8 M LiCl, an additional precipitation step included at the end of the procedure to improve the RNA quality. RNA was treated with RNase-free DNaseI (Ambion) following the manufacturer’s instructions, and the absence of contaminating genomic DNA was assessed using a PCR control test.
qRT-PCR Determination of Gene Expression
The synthesis of cDNA by reverse transcriptase (RT) from the RNA samples was carried out using the iScriptTM cDNA Synthesis kit from Quanta as recommended by the manufacturer. One microgram RNA was reverse transcribed in a 20 mL total reaction volume. Specific primers to amplify fragments of the genes from Synechococcus sp. WH7803 were designed using the Oligo 4.05 software (Molecular Biology Insights, Inc.), on the basis of the corresponding Synechococcus sp. WH7803 genome sequence (Dufresne et al., 2008). During the optimization of quantitative real-time PCR (qRT-PCR) reactions, products were checked for single amplification of DNA fragments of the expected size by agarose gel electrophoresis. The sequences of the primers used for glnAI were:
RT-FGS1SY: ATTTATCTGGCAGCGGTTTG
RT-RGS1SY: TTCAATGGTGTCAACGCTGT.
and for glnN were:
RT-FGNSY: CTCCGGAAAGCATGTGAACT
RT-RGNSY: GCAGCACAGAACAACAGGAA
Real-time quantitative PCR reactions were performed in triplicate. The reaction mixtures contained 1× concentration of SsoFast EvaGreen Supermix from Bio-Rad, 0.128–0.384 μM forward and reverse primers (depending on the efficiency calculations) and the corresponding cDNA. The efficiency of the reactions was calculated and optimized following a method described previously (Pfaffl, 2001).
An iCycler IQ multicolor real-time PCR detection system from Bio-Rad was used for quantitative detection of amplified PCR products using the following thermal cycling conditions: 95°C for 2 min, and 50 cycles of 95°C for 15 s, followed by 58°C for 30 s and 72°C for 30 s. At the end, reactions were checked to discard false amplifications by verifying the melting point of PCR products, determining the fluorescence between 65 and 100°C, with increases of 0.5°C, measured each 10 s. DNA contamination in the qRT-PCR experiments was discarded by using a no RT control sample.
The relative change in gene expression was endogenously normalized to that of the rnpB gene (RT-FRNSY: TGAGGAGAGTGCACAGAAA and RT-RRNSY: GTTTACCGAGCCAGCACCT), encoding RNase P, calculated using the 2-ΔΔCt method (Pfaffl, 2001). No change in rnpB gene expression was observed under our experimental conditions.
Genomic Sequences and Phylogenetic Analysis
All available cyanobacterial glnA and glnN sequences were retrieved from CYORF1, Cyanobase2, Integrated Microbial Genomes3, and NCBI4 databases. Molecular weight and pI values of the corresponding GS subunits were calculated using the tool compute pI/MW from ExPASy5. Alignment of deduced protein sequences and phylogenetic tree were obtained using JalView 2.9 0b2 (Waterhouse et al., 2009) and MEGA 7 (Kumar et al., 2016). Protein BLAST analysis were performed using NCBI BLASTp tool6.
Statistical Analysis
Experiments were carried out with at least three independent biological samples. Results are shown with error bars corresponding to the standard deviation. Significance of data was assessed by using the Student’s t-test, and indicated in figures with asterisks: ∗p ≤ 0.05; ∗∗p ≤ 0.01.
Results
Phylogeny of GSIII from Synechococcus
Information about GSIII from marine and freshwater cyanobacteria is summarized in Table 1. All analyzed picocyanobacteria harbor the glnA gene (encoding type I GS). Surprisingly, searches showed that Synechococcus sp. RS9917 had two GSIII enzymes while no GSI was found. This is a rare case amongst cyanobacteria, but not the only one. It has been described that the cyanobacterium Pseudanabaena sp. PCC 6903 possesses only GSIII and is devoid of GSI (Crespo et al., 1998). As can be seen in Table 1, the length of the nucleotide and amino acid sequences are highly similar among the different strains, the molecular weights are also close (from 76.8 to 79.4 kDa). The estimated size of GSIII from Synechococcus sp. WH7803 was confirmed by Western blotting (Figures 4B, 7C and 9C). The main difference found among the various GSIII enzymes was primary amino acid sequence (Table 1). The highest similarity was between the GSIII from Synechococcus sp. WH7805 and Synechococcus sp. WH7803 (94% identity). The lowest similarities were obtained between sequences from the freshwater cyanobacteria with respect to Synechococcus sp. WH7803, around 63 and 65% identity. The size of the GSIII enzymes sequenced to date is approximately 250 amino acids longer than GSI.
An alignment of the deduced amino acid sequences of the glnN gene of several marine and freshwater cyanobacteria is shown in Figure 1, and Supplementary Figure S1 provides an alignment of the available glnN sequences in cyanobacteria. The five typical regions conserved in the GS enzyme (Reyes and Florencio, 1994) are highlighted with a square. These regions correspond to five β-sheets involved in the GS active site and conserved regions (Yamashita et al., 1989; Reyes and Florencio, 1994). Region I is characterized by the sequence DGSS. Glutamic acid residues present in regions II and V are ligands to the Mn2+ metal associated with the active site. Region III is defined as the putative ATP binding site, seven residues are identical between the GSI, GSII, and GSIII (marked with asterisks). Finally, region IV is the glutamate-binding site and its sequence, NR-XXX-P-X-P, is conserved among the GSIII shown in Figure 1. The highlighted boxes represent typical conserved regions of GSIII enzymes sequenced to date (Crespo et al., 1998).
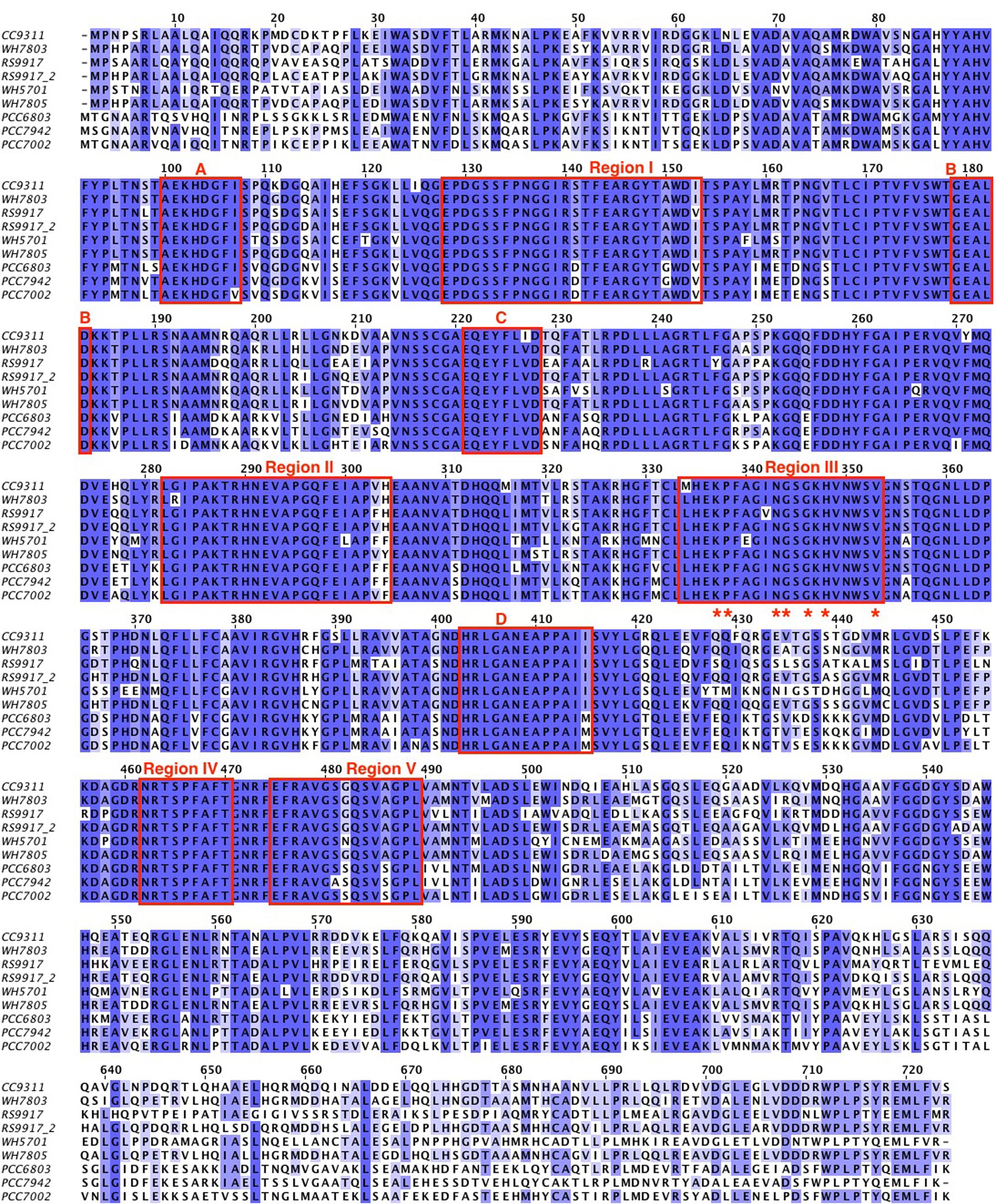
FIGURE 1. Alignment of different GSIII from cyanobacteria. The amino acid sequences were retrieved from different databases as explained in the Section “MATERIALS AND METHODS.” The cyanobacteria used were: WH7803 (Synechococcus sp. WH7803), CC9311 (Synechococcus sp. CC9311), PCC7002 (Synechococcus sp. PCC 7002), RS9917 (Synechococcus sp. RS9917), RS9917_2 (the second GSIII annotated from Synechococcus sp. RS9917), WH5701 (Synechococcus sp. WH5701), WH7805 (Synechococcus sp. WH7805), PCC6803 (Synechocystis sp. PCC 6803), and PCC7942 (Synechococcus sp. PCC 7942). The alignment was performed using the software JalView 2.9 0b2 and refined manually. The color is based on the % identity to a consensus sequence. The boxes marked with I, II, III, IV, and V are the regions typical from GSs and the boxes A, B, C, and D are the regions that defined GSIII.
The phylogenetic tree shown in Figure 2 was deduced from the full alignment from Supplementary Figure S1. It grouped the sequences from marine Synechococcus glnN genes in a cluster which includes two sequences from Cyanobium. The Microcystis sequences are clearly separated from the rest.
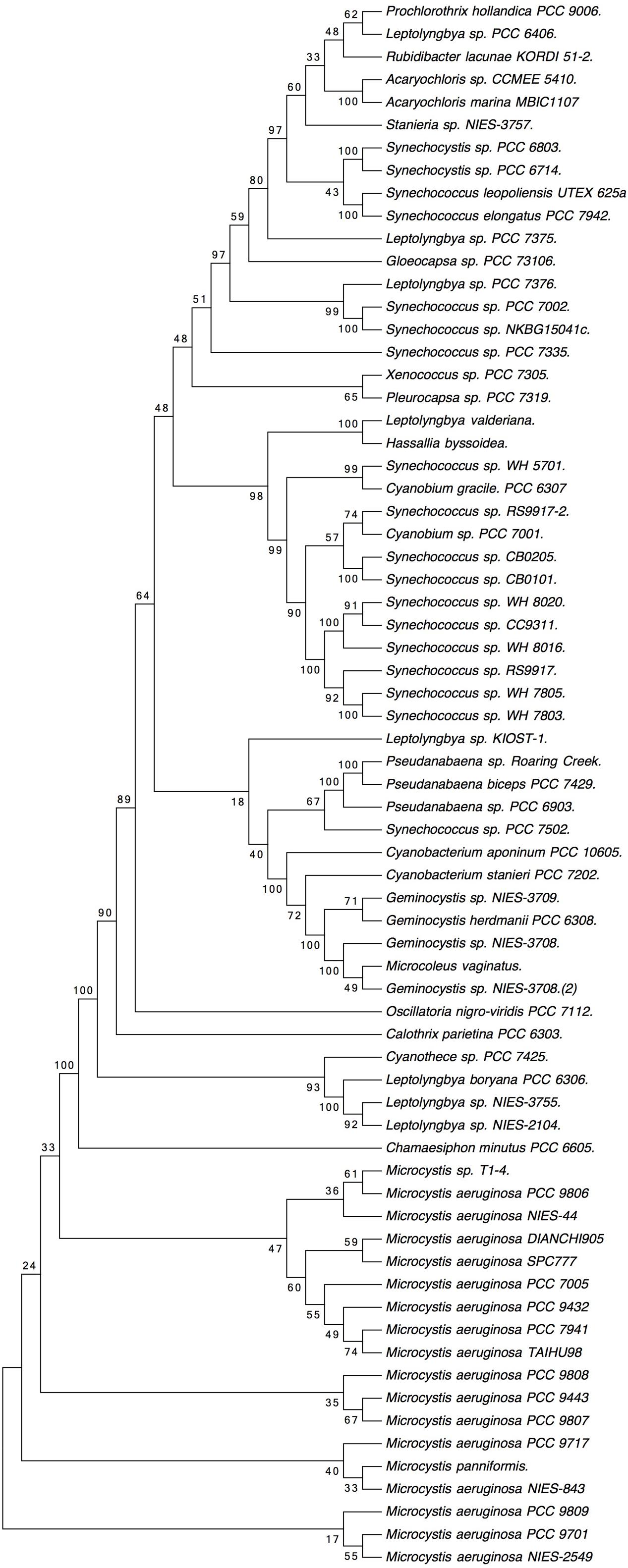
FIGURE 2. Phylogenetic tree based on cyanobacterial GSIII sequences, obtained from the alignment shown in Supplementary Figure S1. Molecular phylogenetic analysis by maximum likelihood method. The evolutionary history was inferred by using the maximum likelihood method based on the Whelan and Goldman model (Whelan and Goldman, 2001). The bootstrap consensus tree inferred from 100 replicates is taken to represent the evolutionary history of the taxa analyzed (Felsenstein, 1985). Branches corresponding to partitions reproduced in less than 50% bootstrap replicates are collapsed. The percentage of replicate trees in which the associated taxa clustered together in the bootstrap test (100 replicates) are shown next to the branches. Initial tree(s) for the heuristic search were obtained automatically by applying neighbor-join and BioNJ algorithms to a matrix of pairwise distances estimated using a Jones, Taylor and Thornton (JTT) model, and then selecting the topology with superior log likelihood value. A discrete Gamma distribution was used to model evolutionary rate differences among sites (five categories; +G, parameter = 0.7824). The rate variation model allowed for some sites to be evolutionarily invariable ([+I], 16.3612% sites). The analysis involved 69 amino acid sequences. All positions with less than 95% site coverage were eliminated. That is, fewer than 5% alignment gaps, missing data, and ambiguous bases were allowed at any position. There were a total of 722 positions in the final dataset. Evolutionary analyses were conducted in MEGA7 (Kumar et al., 2016).
Synechococcus sp. WH7803 possesses GSI encoded by glnA and GSIII encoded by the glnN. There are scarce physiological studies about GSI and none about the role of GSIII in marine cyanobacteria. Therefore, we set out to clarify their physiological functions.
Nitrogen Source-Mediated Regulation
Previous studies have shown that several conditions, such as darkness and nitrogen starvation, cause strong effects on GS regulation in most freshwater cyanobacteria but have little effect on the Prochlorococcus enzyme (El Alaoui et al., 2001, 2003). Therefore, we set out to study the effect of these parameters on the activity and concentration of GS from Synechococcus sp. WH7803 in order to compare the results obtained with those described for Prochlorococcus. It is important to note that the medium used for Synechococcus growth was artificial, so there was no trace of nitrogen from using natural seawater. GS activity showed a significant increase (p = 0.0166) after 24 h under nitrogen starvation (Figure 3) in agreement with an increase in its concentration (Figure 4A). Similar results were described in Synechococcus sp. WH7805, where GS activity was higher after 25 h of nitrogen starvation than in the presence of ammonium (Collier and Lovindeer, 2012). Similarly, glnA gene expression reached a maximum peak at 3 h of nitrogen starvation (Figure 5A; p = 0.0001). These results suggest that GS from Synechococcus sp. WH7803 is up-regulated by nitrogen starvation, this being the standard response in most photosynthetic organisms including cyanobacteria (Mérida et al., 1991). Moreover, a potential NtcA binding site was found upstream of glnA in the genome of this strain. These results suggest NtcA-dependent regulation of this gene. ntcA expression has been shown to be negatively regulated by ammonium in Synechococcus sp. WH7803 (Lindell et al., 1998). Furthermore, the expression of ntcA in this strain was induced when cells were exposed to nitrogen stress (Lindell and Post, 2001).
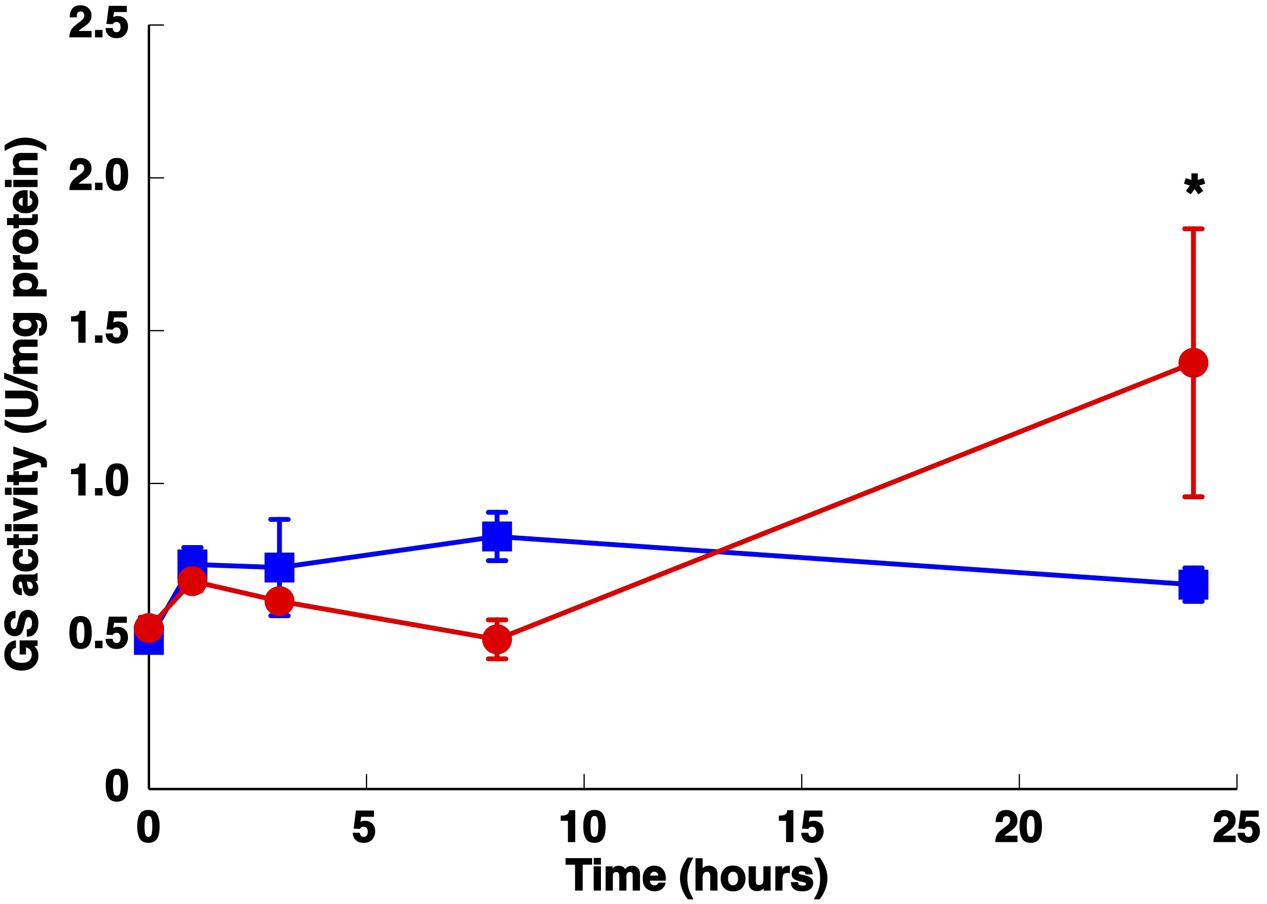
FIGURE 3. Effect of nitrogen starvation on GS activity. Cultures were subjected to nitrogen starvation and aliquots were taken at the indicated times. The graph represents four independent biological replicates. Error bars correspond to standard deviation. Squares, control (800 μM of ammonium); circles, nitrogen starvation. ∗p ≤ 0.05.
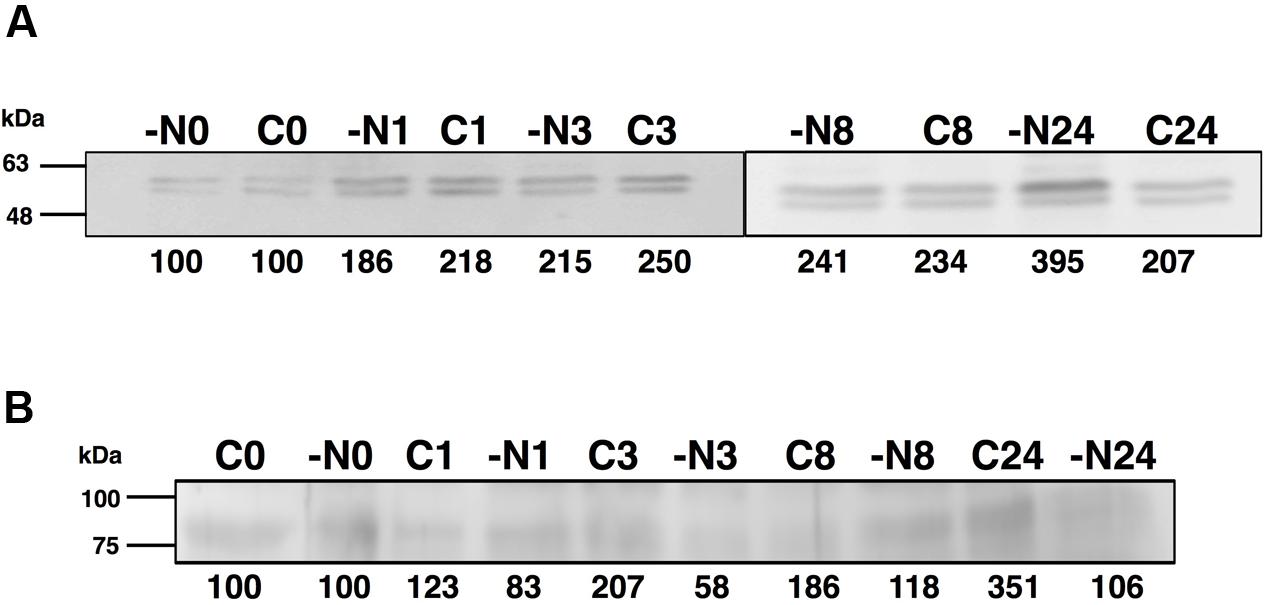
FIGURE 4. Effect of nitrogen starvation on GSI (A) and GSIII (B) concentration. Cultures were subjected to nitrogen starvation and aliquots were taken at the indicated times. Western blotting was performed as described in the Section “Materials and Methods.” Lanes are marked with C (control) and -N (nitrogen starvation), followed by sampling time (in hours). Quantification of bands below the picture, 100% corresponds to the intensity of the control sample at time 0.
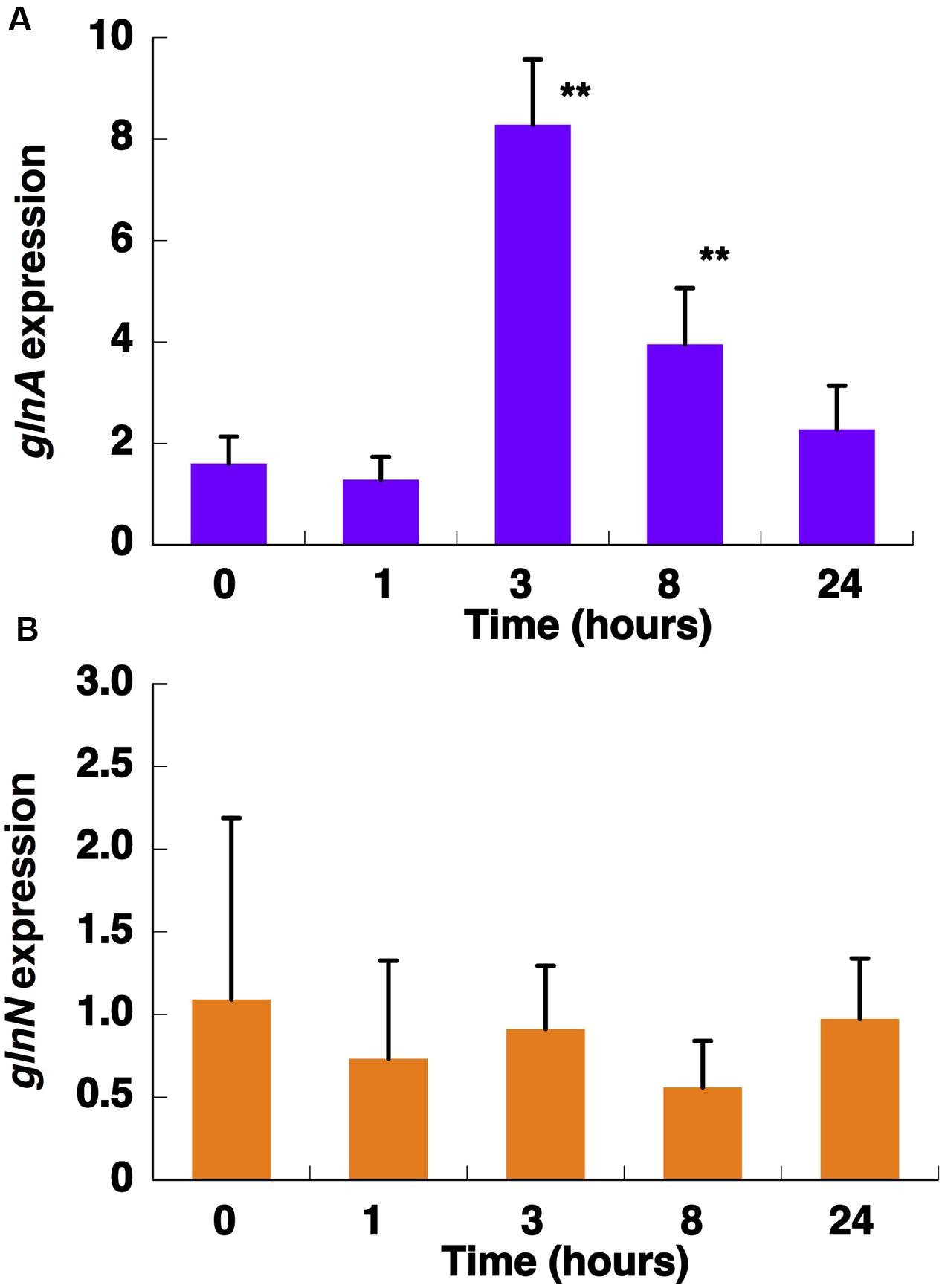
FIGURE 5. Effect of nitrogen starvation on glnA (A) and glnN (B) expression. Cultures were subjected to nitrogen starvation and aliquots were taken at the indicated times. Gene expression was measured by quantitative real-time PCR (qRT-PCR). Data are the average of four independent biological replicates. Error bars correspond to standard deviation. ∗∗p ≤ 0.01.
The concentration of GSIII did not significantly change along the nitrogen deprivation time course, in good agreement with glnN expression (Figures 4B and 5B, respectively). This fact differs from what is described to date in freshwater cyanobacteria such as Synechocystis PCC 6803 (Reyes et al., 1997) or Synechococcus PCC 7942 (Sauer et al., 2000): in both organisms, glnN was highly up-regulated after nitrogen depletion. Besides, in the upstream region of glnN we detected two non-canonical sites for NtcA without a corresponding TATA box (Supplementary Figure S2). A closer canonical NtcA binding site including a TATA box was found 500 nucleotides upstream of the putative glnN transcription start site. These characteristics suggest that this gene might not be regulated by this transcriptional factor in Synechococcus sp. WH7803. However, previous studies have shown that an imperfect NtcA binding motif upstream of glnN allowed up-regulation of its expression under nitrogen starvation in Synechococcus sp. PCC 7942 (Sauer et al., 2000).
We studied GS activity in Synechococcus sp. WH7803 cultures at different times after transfer to medium containing different nitrogen sources or without nitrogen; urea was not tested, as Synechococcus sp. WH7803 lacks the genes required for its assimilation (Collier et al., 1999). The highest GS activity was found after 120 h growth with nitrate as nitrogen source (Figure 6A). Longer-term experiments were performed in order to further analyze the response of GS to those nitrogen sources. After 384 h growth on the different nitrogen sources, GS activity was significantly higher under nitrogen starvation (p = 0.0363) and with nitrate (p = 0.0007) compared to the ammonium grown control. GS activity significantly decreased (p = 0.0179) when the cultures were grown with nitrite as nitrogen source.
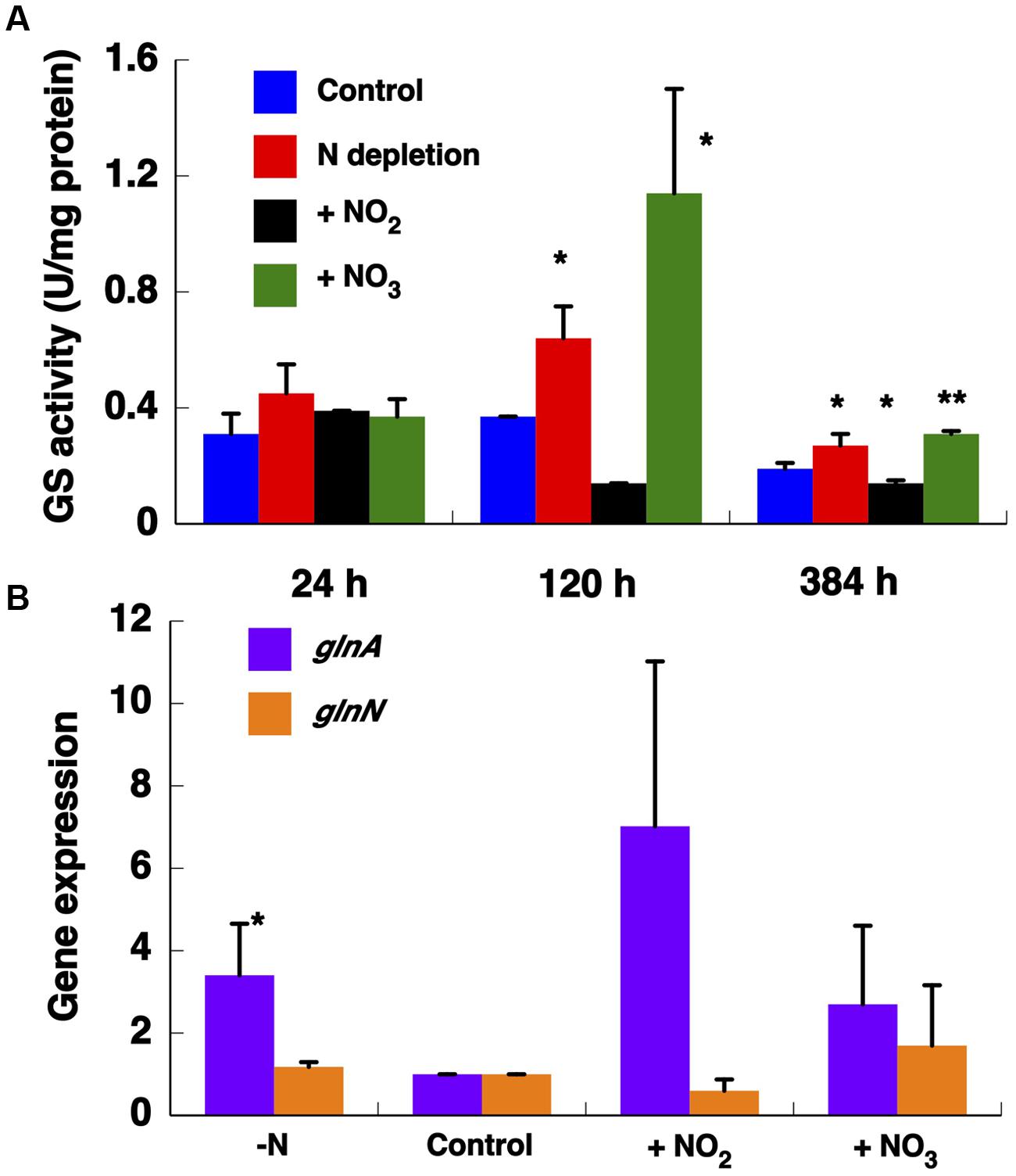
FIGURE 6. Effect of different nitrogen sources on GS activity (A) and on the expression of GSs (B). The cultures were subjected to nitrogen starvation, 800 μM ammonium (control), 800 μM nitrate, and 800 μM nitrite. The cells were collected after 24, 120, and 384 h of treatment. The chart represents the average of three independent biological replicates. Error bars correspond to standard deviation. Gene expression was measured by qRT-PCR with samples after 384 h growing with different nitrogen sources. ∗p ≤ 0.05; ∗∗p ≤ 0.01.
The expression of both genes encoding GS was determined after 384 h (Figure 6B). We observed that glnN expression was not affected after transfer to different nitrogen sources, in contrast to the pattern found for glnA whose expression was significantly up-regulated under nitrogen depletion (p = 0.0247). These results are consistent with the observed variation in GS activity. However, the highest glnA expression was detected after transfer to nitrite medium (p = 0.0596) in contrast to the activity shown in Figure 6A.
Physiological Response to Darkness
The GSs of most photosynthetic organisms, including cyanobacteria, are regulated by light (Rowell et al., 1979; Marqués et al., 1992; Flores and Herrero, 1994). Hence, the effect of darkness was investigated. The growth of Synechococcus sp. WH7803 on ammonium-containing medium (800 μM ammonium) under darkness decreased slightly (not shown) and the GS activity remained almost unchanged even after 24 h of darkness (Figure 7A). This is an unusual response, as darkness promotes the down regulation of GS in most studied cyanobacteria (Rowell et al., 1979; Marqués et al., 1992; Flores and Herrero, 1994). This uncommon response was also described for Prochlorococcus PCC 9511 (El Alaoui et al., 2001). The concentration of the enzyme in Synechococcus sp. WH7803 showed a decrease after darkness, in contrast to the lack of effect of darkness on GS activity (Figure 7B). The same fact was described in Prochlorococcus SS120, a decrease of 20% of the GS concentration in crude extracts was found after 24 h darkness (El Alaoui et al., 2001).
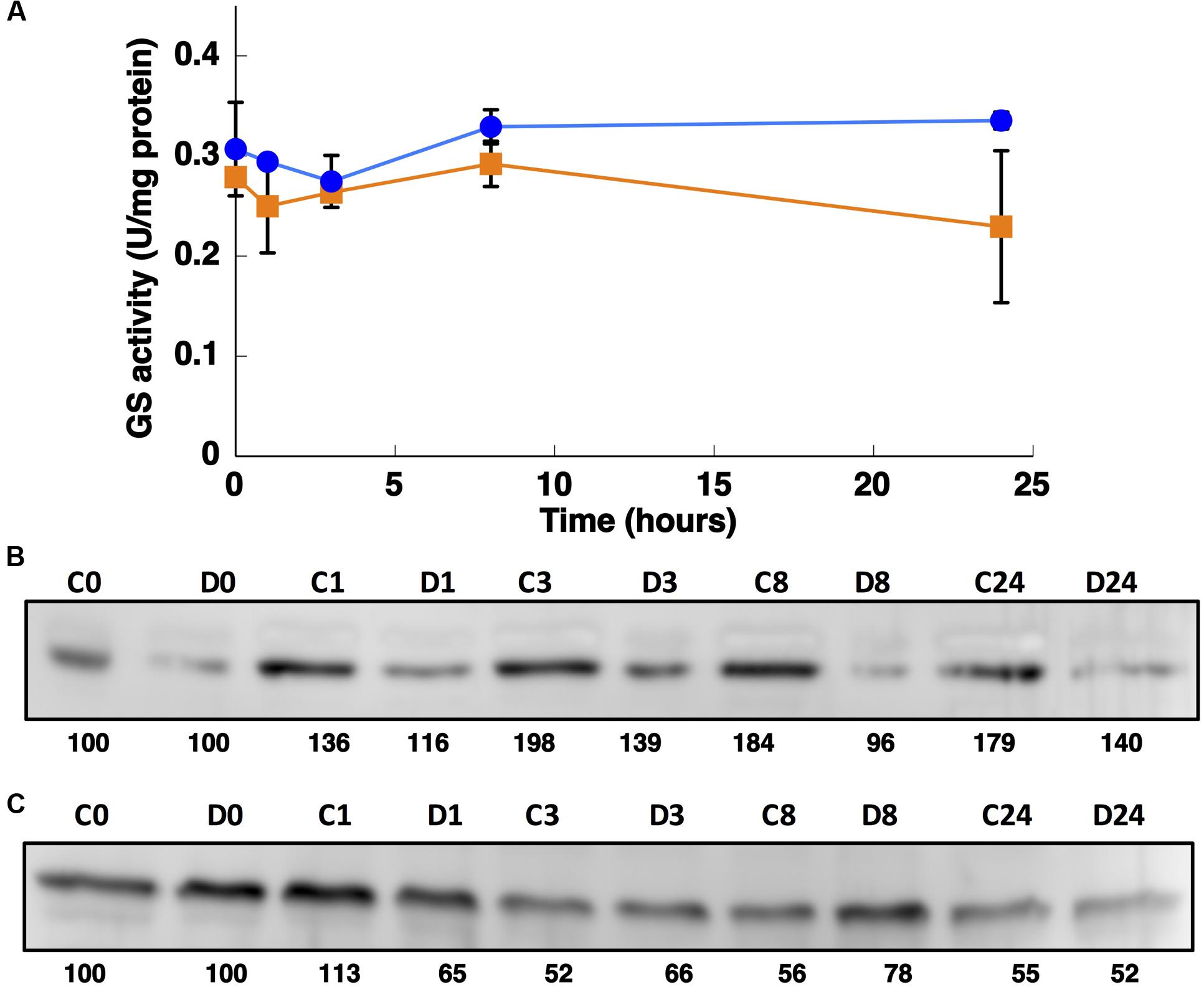
FIGURE 7. Effect of darkness on GS activity (A) and the concentration of GSI (B) and GSIII (C). Synechococcus sp. WH7803 was subjected to darkness (squares) and 40 μE m2 s-1 blue light (control, circles) and aliquots were taken at the indicated times. The graph represents four independent biological replicates. Errors bars correspond to standard deviation. (B,C) Lanes are marked with C (control) and D (darkness), followed by sampling time (in hours). Quantification of bands below the picture, 100% corresponds to the intensity of the control sample at time 0.
Interestingly, the expression of glnA and glnN showed an identical pattern under darkness (Figure 8). Both were, however, up-regulated significantly after 8 h darkness (p = 0.0419 for glnA and p = 0.0242 for glnN). glnA gene expression did not match the level of the GSI protein, in contrast to glnN, which was is in good agreement with the level of the GSIII protein found (Figure 7C), with a peak of expression and accumulation of the protein occurring after 8 h darkness (Figure 8). However, a clear effect on the expression of glnA in Prochlorococcus SS120, which decreased ca. seven times, has been observed after 24 h under darkness (López-Lozano et al., 2009). Besides, it has been established in other cyanobacteria that the regulation of nitrogen metabolism is affected by darkness, since the expression of the global regulator, ntcA, decreases under this condition in Synechocystis sp. PCC 6803 (Alfonso et al., 2001). Our results on GS protein concentration were consistent with this model, there was a decrease in the GSI concentration under darkness (Figure 7B). Nevertheless, GSIII concentration did not show a clear pattern in the absence of light.
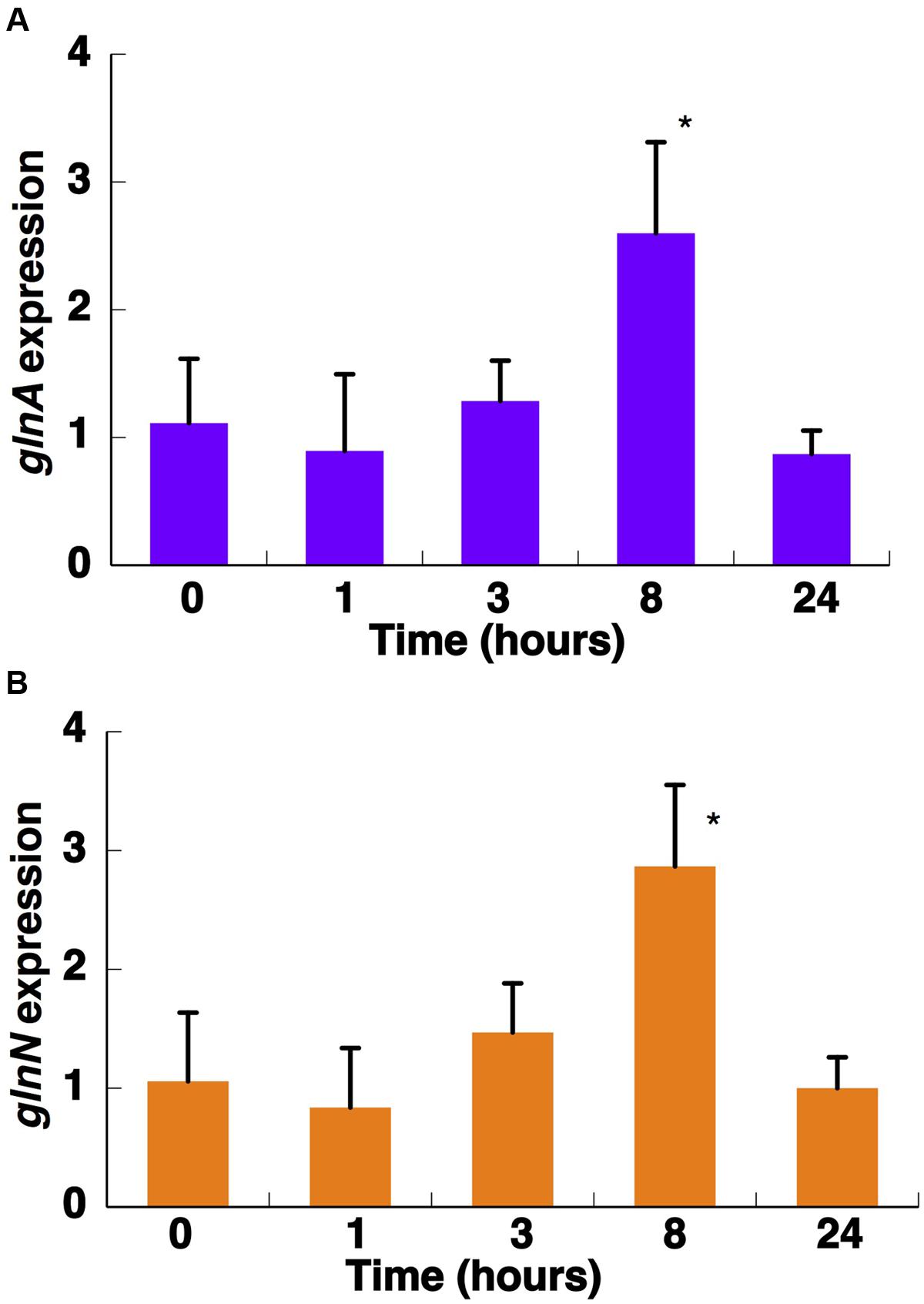
FIGURE 8. Effect of darkness on the expression of glnA (A) and glnN (B). Cultures were subjected to darkness and 40 μE m2 s-1 blue light (control) and aliquots were taken at the indicated times. Gene expression was measured by qRT-PCR. The chart represents the average from two independent biological replicates. Error bars correspond to standard deviation. ∗p ≤ 0.05.
Effect of the GS Inhibitor MSX
The effect of 100 μM MSX, blocking specifically the GS enzyme (Pace and McDermott, 1952), was also studied in cultures of Synechococcus sp. WH7803. As shown in Figure 9A, GS activity was not affected by the addition of 100 μM MSX. It is possible that the existence of two GSs in Synechococcus sp. WH7803 could compensate for the inhibition provoked by this treatment. If this hypothesis is true, it might mean that GSIII is not inactivated by MSX in Synechococcus sp. WH7803.
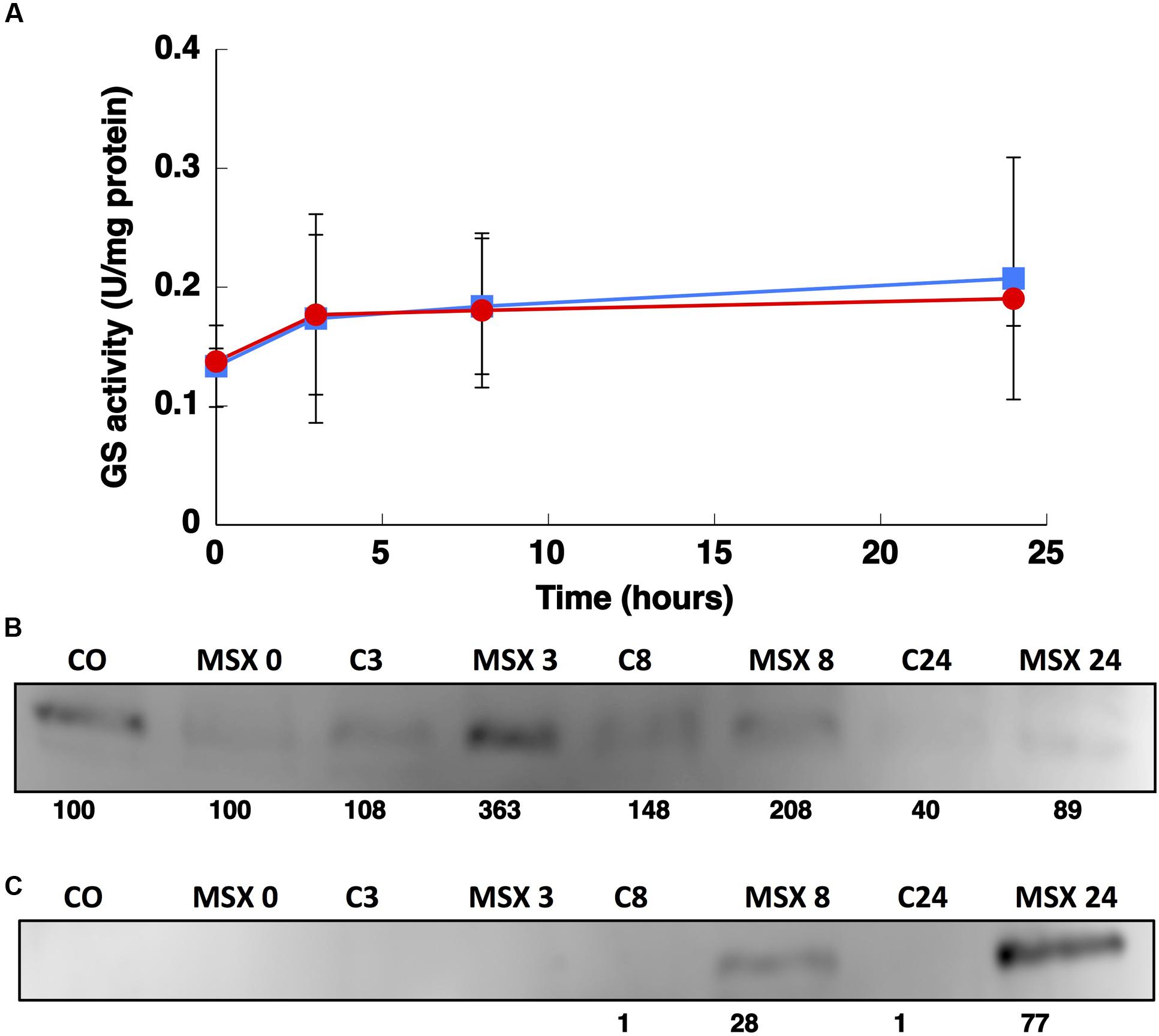
FIGURE 9. Effect of MSX on GS activity (A) and the concentration of GSI (B) and GSIII (C). A total of 100 μM MSX was added to the culture and aliquots were taken at the indicated times. The graph represents four independent biological replicates. Error bars correspond to standard deviation. Control samples are shown with squares and MSX-treated samples with circles.
The inhibitor induced a quick rise in the expression of glnA (3 h; Figure 10A; p = 0.0116) in good agreement with the accumulation of the protein observed using Western blotting (Figure 9B). A similar response was described for Prochlorococcus SS120 after 1 h of the treatment, then the expression recovered to levels similar to those found at the beginning of the experiment (López-Lozano et al., 2009).
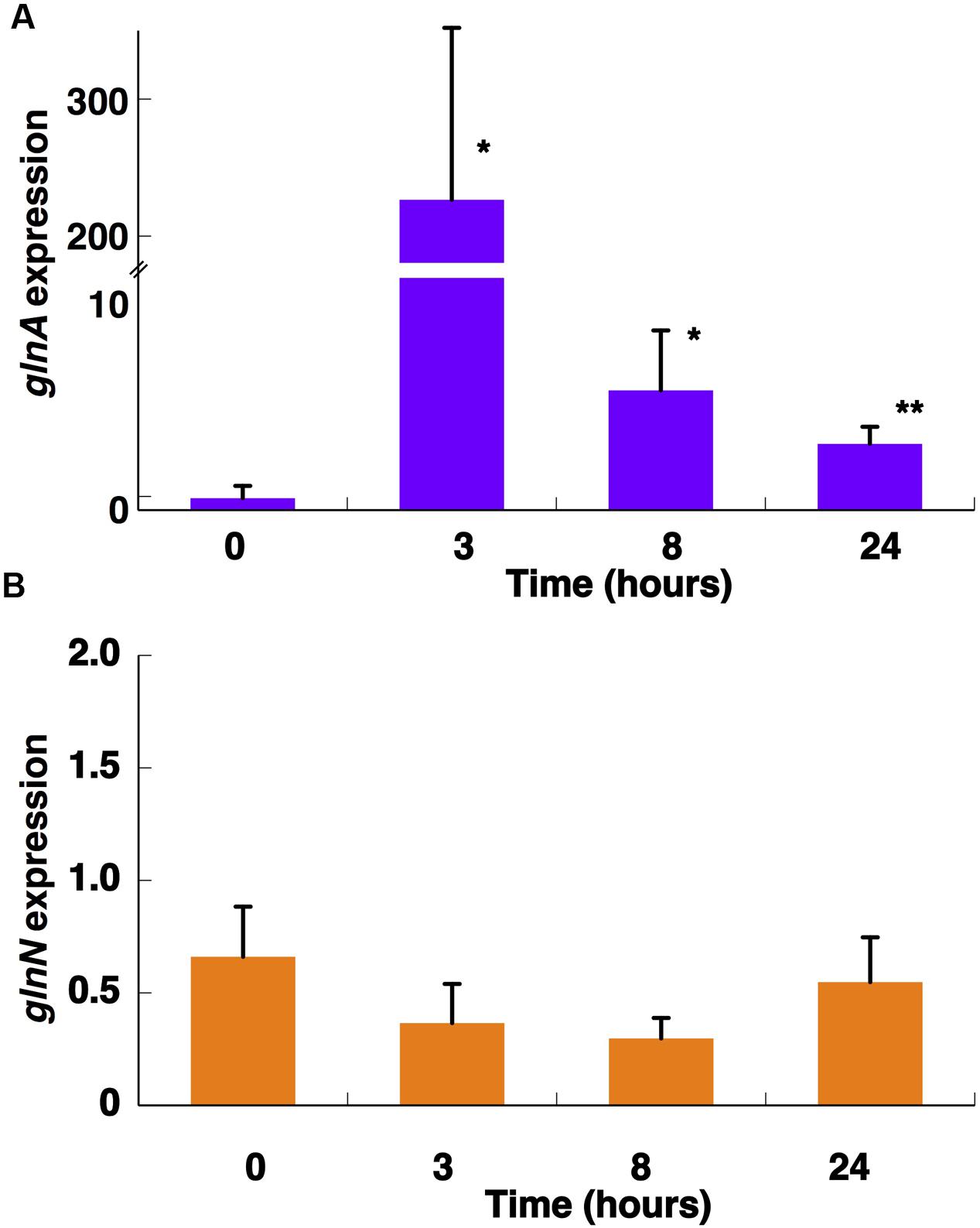
FIGURE 10. Effect of MSX on the expression of glnA (A) and glnN (B). A total of 100 μM MSX was added to the cultures and aliquots were taken at the indicated times. Gene expression was measured by qRT-PCR. The chart represents the average from four independent biological replicates. Error bars correspond to standard deviation. ∗p ≤ 0.05; ∗∗p ≤ 0.01.
Interestingly, the expression of glnN was not affected by MSX treatment but the concentration of the protein increased after 8 and 24 h (Figure 9C). There are no other studies addressing the effect of MSX on glnN, so it is not possible to make direct comparisons; however, these results, together with those obtained with different nitrogen sources, suggest that glnN in Synechococcus sp. WH7803 does not respond to changes in nitrogen availability.
NtcA is a transcription factor which stimulates the expression of different nitrogen-metabolism related genes upon nitrogen stress in cyanobacteria (Luque et al., 1994). As mentioned before, glnA has a promoter controlled by NtcA in Synechococcus sp. WH7803, but glnN expression does not seem to be regulated by this transcription factor. Our results on gene expression (i.e., increase in glnA but not in glnN expression in nitrogen-starved cells; Figure 5) fit nicely with the presence of the putative NtcA-binding sites described above in Synechococcus sp. WH7803 (Supplementary Figure S2). Furthermore, our gene expression results with MSX-treated cultures (Figure 10) were in good agreement with previous reports addressing ntcA regulation in the same Synechococcus sp. WH7803 strain studied in this paper: ntcA transcript levels in ammonium-grown cells were significantly increased after MSX addition (Lindell and Post, 2001).
Discussion
The present work is the first study analyzing in detail the changes observed in the two GSs (GSI and GSIII) from Synechococcus sp. WH7803 under conditions representative of the actual challenges faced by natural Synechococcus populations (i.e., different nitrogen sources—such as nitrate, nitrite, or ammonium—very low nitrogen concentrations in the ocean, or fluctuations in the received irradiance). Furthermore, there are no studies that show the role of GSIII in marine cyanobacteria and there are no reports on physiological regulation of GSI in WH7803. This strain is a model cyanobacterium, representative of the Synechococcus clades inhabiting mesotrophic areas of the ocean. Like the closely related Prochlorococcus it possesses a relatively streamlined genome with a small number of genes (Dufresne et al., 2008).
The glnN gene encodes a new type of GS that differs widely in size, structure, and amino acid sequence from previously known GSI of prokaryotes. The molecular mass of this GSIII, as deduced from the predicted amino acid sequence, is about 79.4 kDa and it was verified through Western blotting (Figures 4B, 7C and 9C). The native GSIII may be composed of six identical subunits arranged in a hexameric structure. All possible combinations of prokaryotic GSs have been shown to occur in cyanobacteria: only GSI (i.e., all Prochlorococcus strains thus far sequenced), only GSIII (i.e., Synechococcus sp. RS9917) and both GSI and GSIII (i.e., Synechococcus sp. WH7803; Crespo et al., 1998; Scanlan et al., 2009).
In relation to nitrogen deprivation, the results showed that GS activity from Synechococcus sp. WH7803 was up-regulated by nitrogen starvation (Figure 3), and GSI protein (Figure 4A) and glnA expression (Figure 5A) were higher under nitrogen deficiency. This fact was the standard response found in most photosynthetic organisms including cyanobacteria (Mérida et al., 1991; Marqués et al., 1992). However, it differs from what was found in its main competitor Prochlorococcus (El Alaoui et al., 2001). There is an enormous diversity in the Prochlorococcus radiation (Urbach and Chisholm, 1998; Rocap et al., 1999, 2002; Garczarek et al., 2007; Partensky and Garczarek, 2010), therefore the comparison between one strain of Synechococcus, WH7803, and the whole group of its main competitor Prochlorococcus is complicated. This complexity is even higher considering that Prochlorococcus strains only have GSI. While Synechococcus sp. WH7803 showed an increment in GS activity under nitrogen deprivation (Figure 3), it slightly decreased in Prochlorococcus PCC 9511 (El Alaoui et al., 2001). On the other hand, the concentration of GSI enzyme from Synechococcus (Figure 4A) increased ca. twofold compared to control samples after 24 h of nitrogen starvation, but there were only minor changes in Prochlorococcus (El Alaoui et al., 2001). Regarding gene expression, the increase in glnA expression in Synechococcus sp. WH7803 (Figure 5A) was a similar response to that found in Synechococcus sp. WH8103 after 8 h nitrogen deprivation (Bird and Wyman, 2003). Interestingly, a similar pattern for Prochlorococcus sp. SS120 (López-Lozano et al., 2009) and for MED4 and MIT9313 (Tolonen et al., 2006) was described.
The first striking result of our studies was that GSIII protein levels and glnN expression were not altered when the cells were transferred to medium without any nitrogen source (Figures 4B and 5B). This result is in sharp contrast to those found in Synechocystis sp. PCC 6803 (Reyes and Florencio, 1994; García-Domínguez et al., 1997). In that case the induction of GSIII occurred only under conditions of nitrogen deficiency. Another important feature of GSIII in Synechococcus sp. WH7803 is that it contains a non-canonical binding site for NtcA, suggesting that it might not be regulated in a NtcA-dependent manner. This is in good agreement with the lack of response under nitrogen starvation, leading us to think that GSIII is not essential for nitrogen stress response in this strain.
Synechococcus usually coexists with Prochlorococcus in very oligotrophic regions (Partensky et al., 1999). Their competition for scarce nutrients has led to different survival strategies, including streamlining of regulatory mechanisms and differential utilization of available nitrogen sources (López-Lozano et al., 2002; Moore et al., 2002; Rocap et al., 2003; García-Fernández et al., 2004; Tolonen et al., 2006; Dufresne et al., 2008; Scanlan et al., 2009; Berube et al., 2015). This is an important factor, given nitrogen is a key element limiting growth in these regions of the ocean (Graziano et al., 1996). Most known marine Synechococcus strains are able to utilize oxidized forms of nitrogen. This fact represents a key difference with most Prochlorococcus strains isolated thus far (López-Lozano et al., 2002; Moore et al., 2002; Berube et al., 2015), although there are several strains that have been recently shown to grow on nitrate (Berube et al., 2015). With this in mind, we wanted to know how the GSs from Synechococcus sp. WH7803 physiologically respond to the addition of different nitrogen sources. GS activity was determined following growth with different nitrogen sources for various times. Activity was ca. threefold higher with nitrate than in control samples growing on ammonium after 120 h. Collier and Lovindeer (2012) studied GS activity in several marine Synechococcus strains. In all cases, the highest GS activity was observed in cultures using nitrate as nitrogen source after 25 h. These results need to be put in the context of this strain of Synechococcus being representative of a clade (clade V) widespread but in low abundance in various oceanic waters (Scanlan et al., 2009). Therefore, the ecological distribution of this clade extends where nitrate is available (Scanlan et al., 2009), consistent with the high GS activity in this condition.
The GSs of most photosynthetic organisms, including cyanobacteria, are regulated by light (Rowell et al., 1979; Marqués et al., 1992; Flores and Herrero, 1994). Our study found an unusual response in Synechococcus sp. WH7803, also described for Prochlorococcus PCC 9511 (El Alaoui et al., 2001), that GS activity was not affected by darkness. This is unusual since darkness promotes the down-regulation of GS activity in most studied cyanobacteria (Rowell et al., 1979; Marqués et al., 1992; Flores and Herrero, 1994). The situation found was not completely shared with Prochlorococcus. GS activity (Figure 7A) did not change under darkness, although degradation of the GSI enzyme occurred. glnA and glnN showed a peak of gene expression after 8 h darkness, the contrary pattern for glnA expression being found in Prochlorococcus sp. SS120 (López-Lozano et al., 2009). Moreover, this is the only condition in which we could observe a clear response in glnN expression.
The effect of MSX on GS regulation has been studied in many unicellular organisms such as cyanobacteria (Orr and Haselkorn, 1982; El Alaoui et al., 2001, 2003; López-Lozano et al., 2009; Rangel et al., 2009) and green algae (García-Fernández et al., 1995). This inhibitor provokes a rapid GS inactivation in Anabaena PCC 7120 (Orr and Haselkorn, 1982) and in Prochlorococcus sp. PCC 9511 (El Alaoui et al., 2001). However, we observed clearly different results in Synechococcus sp. WH7803: GS activity did not change with the addition of 100 μM MSX, although glnA expression was considerably higher 3 h after addition, whilst glnN expression did not change. Regarding the concentration of GSI in Prochlorococcus following MSX treatment, we found different responses depending on the strain: for SS120 the concentration of GS decreased 32%, although in the case of PCC 9511 increased 17% (El Alaoui et al., 2001).
The phylogenetic analysis of glnA from different marine and freshwater cyanobacteria showed that marine Synechococcus and Prochlorococcus sequences are grouped together, separated from freshwater model strains, in agreement with previous phylogenetic studies on glnA in marine cyanobacteria (El Alaoui et al., 2003). Combining these data with the results discussed above it can be suggested that the structure of GS has not been significantly modified in the marine picocyanobacterial clade during evolution, in contrast with the possible modulation of its regulatory properties, as previously proposed (El Alaoui et al., 2003).
This study shows that GSIII in Synechococcus sp. WH7803 does not have the same physiological role described for other cyanobacteria, since it is not responsive to nitrogen deficiency, unlike GSIII from Synechocystis sp. PCC 6803 (Reyes et al., 1997; Sauer et al., 2000), Pseudanabaena sp. PCC 6903 (Crespo et al., 1998) and Synechococcus sp. PCC 7942 (Sauer et al., 2000). The lack of response under different key conditions suggests that in this strain GSIII coexists with GSI but has no clear, specific functionality. Our results indicate its expression is constitutive but without any important role in the conditions tested in this study. The proposed role for GSIII in the recovery after prolonged nitrogen starvation (Sauer et al., 2000) seems rather unlikely in Synechococcus sp. WH7803, given its lack of responsiveness to nitrogen starvation.
Therefore, this could represent an intermediate situation in the evolution of the marine cyanobacterial radiation, since there are other marine Synechococcus that have lost this gene (i.e., Synechococcus sp. WH8102, WH8103, BL107) and all the sequenced Prochlorococcus lack GSIII. glnN from Synechococcus sp. WH7803 might be in the process of being lost, and the removal of its regulation by nitrogen availability could be the first sign of this process. The loss of glnN is understandable in a group of organisms characterized by a trend to compact their genomes (Strehl et al., 1999), and it might be similar to the process leading to the disappearance of other important genes (Kettler et al., 2007), e.g., narB, in recently evolved clades of marine Synechococcus and Prochlorococcus (Scanlan et al., 2009; Berube et al., 2015). Hence, this enzyme is probably not necessary in a relatively stable environment (García-Fernández et al., 2004), where the presence of a single gene encoding GS (glnA) might be enough for ammonium assimilation.
In this context, the glnA/GSI response in Synechococcus sp. WH7803 also supports an intermediate position in the progressive modification of its regulation during the evolution of cyanobacteria. In this way, GS regulation observed in freshwater strains such as Synechocystis sp. PCC 6803 (responding to both nitrogen availability and darkness; Marqués et al., 1992) would lose first the responsiveness to light, leading to the appearance of strains like Synechococcus sp. WH7803; the capability to respond to nitrogen starvation would be reduced in further evolved cyanobacteria, such as Prochlorococcus (El Alaoui et al., 2001, 2003; Lindell et al., 2002; Tolonen et al., 2006). This hypothesis is in good agreement with the intermediate phylogenetic position of Synechococcus sp. WH7803 in the cyanobacterial radiation (Scanlan et al., 2009).
Conclusion
We suggest that Synechococcus sp. WH7803 shows regulatory features which fit in the context of a progressive streamlining, from early branching members of the cyanobacterial tree (enabled to react to changing environments) to late branching marine strains which are progressively adapted to more stable ocean niches, therefore losing the capability to perform fine regulation with respect to light and nutrient availability.
Author Contributions
MD-M and JD performed research; MD-M, JD, and JG-F designed research, analyzed data, wrote and approved the final version of the manuscript.
Funding
This work was supported by grants BFU-2009-08008/BMC and BFU-20103-44767 (Spanish Ministerio de Economía y Competitividad, co-funded by the European Social Fund from the European Union), P12-BIO-2141 (Proyectos de Excelencia, Junta de Andalucía, Spain), and Universidad de Córdoba (Programa Propio de Investigación). MD-M received a grant from projects BFU-2009-08008/BMC and P07-CVI-3055 (Proyectos de Excelencia, Junta de Andalucía, Spain).
Conflict of Interest Statement
The authors declare that the research was conducted in the absence of any commercial or financial relationships that could be construed as a potential conflict of interest.
Acknowledgments
We thank Prof. Wolfgang R. Hess and Dr. Claudia Steglich (University of Freiburg, Germany) for providing Synechococcus sp. WH7803. We are indebted to Prof. Javier Florencio and Dr. Maribel Muro-Pastor (Instituto de Bioquímica Vegetal y Fotosíntesis, Seville, Spain) for providing the anti-GSI and anti-GSIII antibodies from Synechocystis sp. PCC 6803. We thank the Servicio Centralizado de Apoyo a la Investigación (SCAI, Universidad de Córdoba) for DNA sequencing.
Supplementary Material
The Supplementary Material for this article can be found online at: http://journal.frontiersin.org/article/10.3389/fmicb.2016.00969
FIGURE S1 | Alignment of available cyanobacterial GSIII sequences. The amino acid sequences were retrieved from different databases as explained in the “MATERIALS AND METHODS”. Sequences were aligned with the MEGA7 software (Kumar et al., 2016), by using the MUSCLE method with default settings.
FIGURE S2 | Non-canonical NtcA-binding sites and putative TATA box in the region upstream glnN in the genome of Synechococcus sp. strain WH7803.
Footnotes
- ^http://cyano.genome.jp/
- ^http://genome.microbedb.jp/cyanobase/
- ^https://img.jgi.doe.gov
- ^http://www.ncbi.nlm.nih.gov/
- ^http://web.expasy.org/compute_pi/
- ^http://blast.ncbi.nlm.nih.gov/Blast.cgi?PAGE=Proteins
References
Alfonso, M., Perewoska, I., and Kirilovsky, D. (2001). Redox control of ntcA gene expression in Synechocystis sp. PCC 6803. Nitrogen availability and electron transport regulate the levels of the NtcA protein. Plant Physiol. 125, 969–981. doi: 10.1104/pp.125.2.969
Berube, P., Biller, S., Kent, A., Berta-Thompson, J., Roggensack, S., Roache-Johnson, K., et al. (2015). Physiology and evolution of nitrate acquistion in Prochlorococcus. ISME J. 9, 1195–1207. doi: 10.1038/ismej.2014.211
Biller, S., Berube, P., Lindell, D., and Chisholm, S. (2015). Prochlorococcus: the structure and function of collective diversity. Nat. Rev. Microbiol. 13, 13–27. doi: 10.1038/nrmicro3378
Bird, C., and Wyman, M. (2003). Nitrate/nitrite assimilation system of the marine picoplanktonic cyanobacterium Synechococcus sp. strain WH 8103: efect of nitrogen source and availability on gene sxpression. Appl. Environ. Microbiol. 69, 7009–7018. doi: 10.1128/AEM.69.12.7009-7018.2003
Bradford, M. (1976). A rapid and sensitive method for the quantitation of microgram quantities of protein utilizing the principle of protein-dye binding. Anal. Biochem. 72, 248–254. doi: 10.1016/0003-2697(76)90527-3
Collier, J., Brahamsha, B., and Palenik, B. (1999). The marine cyanobacterium Synechococcus sp. WH7805 requires urease (urea amidohydrolase, EC-3.5.1.5) to utilize urea as a nitrogen source - Molecular genetic and biochemical analysis of the enzyme. Microbiology 145, 447–459. doi: 10.1099/13500872-145-2-447
Collier, J., and Lovindeer, R. (2012). Differences in growth and physiology of marine Synechococcus (cyanobacteria) on nitrate versus ammonium are not determined solely by nitrogen source redox state. J. Phycol. 48, 106–116. doi: 10.1111/j.1529-8817.2011.01100.x
Crespo, J., Garcia-Dominguez, M., and Florencio, F. (1998). Nitrogen control of the glnN gene that codes for GS Type-III, the only glutamine synthetase in the cyanobacterium Pseudanabaena sp. PCC 6903. Mol. Microbiol. 30, 1101–1112. doi: 10.1046/j.1365-2958.1998.01143.x
Dufresne, A., Ostrowski, M., Scanlan, D., Garczarek, L., Mazard, S., Palenik, B., et al. (2008). Unraveling the genomic mosaic of a ubiquitous genus of marine cyanobacteria. Genome Biol. 9:R90. doi: 10.1186/gb-2008-9-5-r90
El Alaoui, S., Diez, J., Humanes, L., Toribio, F., Partensky, F., and García-Fernández, J. (2001). In vivo regulation of glutamine synthetase activity in the marine chlorophyll b-containing cyanobacterium Prochlorococcus sp. strain PCC 9511 (Oxyphotobacteria). Appl. Environ. Microbiol. 67, 2202–2207. doi: 10.1128/AEM.67.5.2202-2207.2001
El Alaoui, S., Diez, J., Toribio, F., Gómez-Baena, G., Dufresne, A., and García-Fernández, J. (2003). Glutamine synthetase from the marine cyanobacteria Prochlorococcus spp.: characterization, phylogeny and response to nutrient limitation. Environ. Microbiol. 5, 412–423. doi: 10.1046/j.1462-2920.2003.00433.x
Felsenstein, J. (1985). Confidence limits on phylogenies: an approach using the bootstrap. Evolution 39, 783–791. doi: 10.2307/2408678
Flombaum, P., Gallegos, J. L., Gordillo, R. A., Rincón, J., Zabala, L. L., Jiao, N., et al. (2013). Present and future global distributions of the marine cyanobacteria Prochlorococcus and Synechococcus. Proc. Natl. Acad. Sci. U.S.A. 110, 9824–9829. doi: 10.1073/pnas.1307701110
Florencio, F., and Reyes, J. (2002). “Regulation of ammonium assimilation in cyanobacteria,” in Photosynthetic Nitrogen Assimilation and Associated Carbon Metabolism, eds C. Foyer and G. Noctor (Dordretch: Kluwer Academic Publishers), 93–113.
Flores, E., and Herrero, A. (1994). “Assimilatory nitrogen metabolism and its regulation,” in The Molecular Biology of Cyanobacteria, ed. D. Bryant (Dordrecht: Kluwer Academic Publishers), 487–517.
Flores, E., and Herrero, A. (2005). Nitrogen assimilation and nitrogen control in cyanobacteria. Biochem. Soc. Trans. 33, 164–167. doi: 10.1042/BST0330164
García-Domínguez, M., Reyes, J., and Florencio, F. (1997). Purification and characterization of a new type of glutamine synthetase from cyanobacteria. Eur. J. Biochem. 244, 258–264. doi: 10.1111/j.1432-1033.1997.00258.x
García-Fernández, J., López-Ruíz, A., Alhama, J., Roldán, J., and Díez, J. (1995). Effect of glutamine on glutamine-synthetase regulation in the green alga Monoraphidium braunii. Planta 195, 434–439.
García-Fernández, J., Tandeau De Marsac, N., and Diez, J. (2004). Streamlined regulation and gene loss as adaptive mechanisms in Prochlorococcus for optimized nitrogen utilization in oligotrophic environments. Microbiol. Mol. Biol. Rev. 68, 630–638. doi: 10.1128/MMBR.68.4.630-638.2004
Garczarek, L., Dufresne, A., Rousvoal, S., West, N., Mazard, S., Marie, D., et al. (2007). High vertical and low horizontal diversity of Prochlorococcus ecotypes in the Mediterranean Sea in summer. FEMS Microbiol. Ecol. 60, 189–206. doi: 10.1111/j.1574-6941.2007.00297.x
Gómez-Baena, G., Diez, J., García-Fernández, J., El Alaoui, S., and Humanes, L. (2001). Regulation of glutamine synthetase by metal-catalyzed oxidative modification in the marine oxyphotobacterium Prochlorococcus. Biochim. Biophys. Acta (BBA) 1568, 237–244. doi: 10.1016/S0304-4165(01)00226-4
Gómez-Baena, G., García-Fernández, J., Lopez-Lozano, A., Toribio, F., and Diez, J. (2006). Glutamine synthetase degradation is controlled by oxidative proteolysis in the marine cyanobacterium Prochlorococcus marinus strain PCC 9511. Biochim. Biophys. Acta (BBA) 1760, 930–940. doi: 10.1016/j.bbagen.2006.01.016
Graziano, L., Geider, R., Li, W., and Olaizola, M. (1996). Nitrogen limitation of North Atlantic phytoplankton - analysis of physiological condition in nutrient enrichment experiments. Aquat. Microb. Ecol. 11, 53–64. doi: 10.3354/ame011053
Herrero, A., Muro-Pastor, A., and Flores, E. (2001). Nitrogen control in cyanobacteria. J. Bacteriol. 183, 411–425. doi: 10.1128/JB.183.2.411-425.2001
Hill, R. T., Parker, J. R., Goodman, H. J., Jones, D. T., and Woods, D. R. (1989). Molecular analysis of a novel glutamine synthetase of the anaerobe Bacteroides fragilis. J. Gen. Microbiol. 135, 3271–3279.
Johnson, Z., Zinser, E., Coe, A., Mcnulty, N., Woodward, E., and Chisholm, S. (2006). Niche partitioning among Prochlorococcus ecotypes along ocean-scale environmental gradients. Science 311, 1737–1740. doi: 10.1126/science.1118052
Kamennaya, N. A., Chernihovsky, M., and Post, A. F. (2008). The cyanate utilization capacity of marine unicellular Cyanobacteria. Limnol. Oceanogr. 53, 2485–2494. doi: 10.4319/lo.2008.53.6.2485
Kamennaya, N. A., and Post, A. F. (2011). Characterization of cyanate metabolism in marine Synechococcus and Prochlorococcus spp. Appl. Environ. Microbiol. 77, 291–301. doi: 10.1128/AEM.01272-10
Kent, A. G., Dupont, C. L., Yooseph, S., and Martiny, A. C. (2016). Global biogeography of Prochlorococcus genome diversity in the surface ocean. ISME J. doi: 10.1038/ismej.2015.265 [Epub ahead of print].
Kettler, G., Martiny, A., Huang, K., Zucker, J., Coleman, M., Rodrigue, S., et al. (2007). Patterns and implications of gene gain and loss in the evolution of Prochlorococcus. PLoS Genet. 3:e231. doi: 10.1371/journal.pgen.0030231
Kumar, S., Stecher, G., and Tamura, K. (2016). MEGA7: molecular evolutionary genetics analysis version 7.0 for bigger datasets. Mol. Biol. Evol. doi: 10.1093/molbev/msw054
Lindell, D., Erdner, D., Marie, D., Prasil, O., Koblizek, M., Le Gall, F., et al. (2002). Nitrogen stress response of Prochlorococcus strain PCC 9511 (Oxyphotobacteria) involves contrasting regulation of ntcA and amt1. J. Phycol. 38, 1113–1124. doi: 10.1046/j.1529-8817.2002.01205.x
Lindell, D., Padan, E., and Post, A. (1998). Regulation of ntcA expression and nitrite uptake in the marine Synechococcus sp. strain WH 7803. J. Bacteriol. 180, 1878–1886.
Lindell, D., and Post, A. (2001). Ecological aspects of ntcA gene expression and its use as an indicator of the nitrogen status of marine Synechococcus spp. Appl. Environ. Microbiol. 67, 3340–3349. doi: 10.1128/AEM.67.8.3340-3349.2001
López-Lozano, A., Diez, J., El Alaoui, S., Moreno-Vivián, C., and García-Fernández, J. (2002). Nitrate is reduced by heterotrophic bacteria but not transferred to Prochlorococcus in non axenic cultures. FEMS Microbiol. Ecol. 41, 151–160. doi: 10.1111/j.1574-6941.2002.tb00976.x
López-Lozano, A., Gómez-Baena, G., Muñoz-Marín, M., Rangel, O., Diez, J., and García-Fernández, J. (2009). Expression of genes involved in nitrogen assimilation and the C/N balance sensing in Prochlorococcus sp. strain SS120. Gene Exp. 14, 279–289. doi: 10.3727/105221609788681204
Luque, I., Flores, E., and Herrero, A. (1994). Molecular mechanism for the operation of nitrogen control in cyanobacteria. EMBO J. 13, 2862–2869.
Luque, I., and Forchhammer, K. (2008). “Nitrogen assimilation and C/N balance sensing,” in The Cyanobacteria. Molecular Biology, Genomics and Evolution, eds A. Herrero and E. Flores (Norfolk: Caister Academic Press), 335–385.
Marqués, S., Mérida, A., Candau, P., and Florencio, F. (1992). Light-mediated regulation of glutamine synthetase activity in the unicellular cyanobacterium Synechococcus sp. PCC 6301. Planta 187, 247–253. doi: 10.1007/BF00201947
McDonagh, B., Domínguez-Martín, M. A., Gómez-Baena, G., López-Lozano, A., Diez, J., Bárcena, J. A., et al. (2012). Nitrogen starvation induces extensive changes in the redox proteome of Prochlorococcus sp. SS120. Environ. Microbiol. Rep. 4, 257–267. doi: 10.1111/j.1758-2229.2012.00329.x
Mérida, A., Candau, P., and Florencio, F. (1991). Regulation of glutamine synthetase activity in the unicellular cyanobacterium Synechocystis sp. strain PCC 6803 by the nitrogen source: effect of ammonium. J. Bacteriol. 173, 4095–4100.
Moore, L., Coe, A., Zinser, E., Saito, M., Sullivan, M., Lindell, D., et al. (2007). Culturing the marine cyanobacterium Prochlorococcus. Limnol. Oceanogr. 5, 353–362. doi: 10.4319/lom.2007.5.353
Moore, L., Post, A., Rocap, G., and Chisholm, S. (2002). Utilization of different nitrogen sources by the marine cyanobacteria Prochlorococcus and Synechococcus. Limnol. Oceanogr. 47, 989–996. doi: 10.4319/lo.2002.47.4.0989
Muro-Pastor, M., and Florencio, F. (2003). Regulation of ammonium assimilation in cyanobacteria. Plant Physiol. Biochem. 41, 595–603. doi: 10.1016/S0981-9428(03)00066-4
Muro-Pastor, M., Reyes, J., and Florencio, F. (2005). Ammonium assimilation in cyanobacteria. Photosynth. Res. 83, 135–150. doi: 10.1007/s11120-004-2082-7
Orr, J., and Haselkorn, R. (1982). Regulation of glutamine synthetase activity and synthesis in free-living and symbiotic Anabaena spp. J. Bacteriol. 152, 626–635.
Pace, J., and McDermott, E. (1952). Methionine sulphoximine and some enzyme systems involving glutamine. Nature 169, 415–416. doi: 10.1038/169415a0
Partensky, F., Blanchot, J., and Vaulot, D. (1999). “Differential distribution and ecology of Prochlorococcus and Synechococcus in oceanic waters: a review,” in Marine Cyanobacteria, eds L. Charpy and A. Larkum (Monaco: Bulletin de l’Institut Océanographique, Numéro spécial), 457–476.
Partensky, F., and Garczarek, L. (2010). Prochlorococcus: advantages and limits of minimalism. Annu. Rev. Mar. Sci. 2, 305–331. doi: 10.1146/annurev-marine-120308-081034
Pfaffl, M. (2001). A new mathematical model for relative quantification in real-time RT-PCR. Nucleic Acids Res. 29:e45. doi: 10.1093/nar/29.9.e45
Pittera, J., Humily, F., Thorel, M., Grulois, D., Garczarek, L., and Six, C. (2014). Connecting thermal physiology and latitudinal niche partitioning in marine Synechococcus. ISME J. 8, 1221–1236. doi: 10.1038/ismej.2013.228
Rangel, O., Gómez-Baena, G., López-Lozano, A., Diez, J., and García-Fernández, J. (2009). Physiological role and regulation of glutamate dehydrogenase in Prochlorococcus MIT9313. Environ. Microbiol. Rep. 1, 56–64. doi: 10.1111/j.1758-2229.2008.00005.x
Reyes, J., and Florencio, F. (1994). A new type of glutamine synthetase in cyanobacteria: the protein encoded by the glnN gene support nitrogen assimilation in Synechocystis sp. strain PCC 6803. J. Bacteriol. 176, 1260–1267.
Reyes, J., Muro-Pastor, M., and Florencio, F. (1997). Transcription of glutamine synthetase genes (glnA and glnN) from the cyanobacterium Synechocystis sp. strain PCC 6803 is differently regulated in response to nitrogen availability. J. Bacteriol. 179, 2678–2689.
Rocap, G., Distel, D., Waterbury, J., and Chisholm, S. (2002). Resolution of Prochlorococcus and Synechococcus ecotypes by using 16S-23S ribosomal DNA internal transcribed spacer sequences. Appl. Environ. Microbiol. 68, 1180–1191. doi: 10.1128/AEM.68.3.1180-1191.2002
Rocap, G., Larimer, F., Lamerdin, J., Malfatti, S., Chain, P., Ahlgren, N., et al. (2003). Genome divergence in two Prochlorococcus ecotypes reflects oceanic niche differentiation. Nature 424, 1042–1047. doi: 10.1038/nature01947
Rocap, G., Moore, L., and Chisholm, S. (1999). Molecular phylogeny of Prochlorococcus ecotypes. Bull. lInst. Oceanogr. 19, 107–115.
Rowell, P., Sampaio, M., Ladha, J., and Stewart, W. (1979). Alteration of cyanobacterial glutamine synthetase activity in vivo in response to light and NH4+. Arch. Microbiol. 120, 195–200. doi: 10.1007/BF00423065
Sauer, J., Dirmeier, U., and Forchhammer, K. (2000). The Synechococcus strain PCC 7942 glnN product (glutamine synthetase III) helps recovery from prolonged nitrogen chlorosis. J. Bacteriol. 182, 5615–5619. doi: 10.1128/JB.182.19.5615-5619.2000
Scanlan, D. (2003). Physiological diversity and niche adaptation in marine Synechococcus. Adv. Microb. Physiol. 47, 1–64. doi: 10.1016/S0065-2911(03)47001-X
Scanlan, D., Ostrowski, M., Mazard, S., Dufresne, A., Garczarek, L., Hess, W., et al. (2009). Ecological genomics of marine picocyanobacteria. Microbiol. Mol. Biol. Rev. 73, 249–299. doi: 10.1128/MMBR.00035-08
Strehl, B., Holtzendorff, J., Partensky, F., and Hess, W. (1999). A small and compact genome in the marine cyanobacterium Prochlorococcus marinus CCMP 1375: lack of an intron in the gene for tRNA(Leu)UAA and a sigle copy of the rRNA operon. FEMS Microbiol. Lett. 181, 261–266. doi: 10.1111/j.1574-6968.1999.tb08853.x
Tolonen, A., Aach, J., Lindell, D., Johnson, Z., Rector, T., Steen, R., et al. (2006). Global gene expression of Prochlorococcus ecotypes in response to changes in nitrogen availability. Mol. Syst. Biol. 2:53. doi: 10.1038/msb4100087
Urbach, E., and Chisholm, S. (1998). Genetic diversity in Prochlorococcus populations flow cytometrically sorted from the Sargasso Sea and Gulf Stream. Limnol. Oceanogr. 43, 1615–1630. doi: 10.4319/lo.1998.43.7.1615
Valentine, R. C., Shapiro, B. M., and Stadtman, E. R. (1968). Regulation of glutamine synthetase. XII. Electron microscopy of the enzyme from Escherichia coli. Biochemistry 7, 2143–2152. doi: 10.1021/bi00846a017
Waterhouse, A. M., Procter, J. B., Martin, D. M., Clamp, M., and Barton, G. J. (2009). Jalview Version 2–a multiple sequence alignment editor and analysis workbench. Bioinformatics 25, 1189–1191. doi: 10.1093/bioinformatics/btp033
Whelan, S., and Goldman, N. (2001). A general empirical model of protein evolution derived from multiple protein families using a maximum-likelihood approach. Mol. Biol. Evol. 18, 691–699. doi: 10.1093/oxfordjournals.molbev.a003851
Wyman, M., and Bird, C. (2007). Lack of control of nitrite assimilation by ammonium in an oceanic picocyanobacterium, Synechococcus sp. strain WH 8103. Appl. Environ. Microbiol. 73, 3028–3033. doi: 10.1128/AEM.02606-06
Yamashita, M., Almassy, R., Janson, C., Cascio, D., and Eisenberg, D. (1989). Refined atomic model of glutamine synthetase at 3.5 Å resolution. J. Biol. Chem. 264, 17681–17690.
Zinser, E., Coe, A., Johnson, Z., Martiny, A., Fuller, N., Scanlan, D., et al. (2006). Prochlorococcus ecotype abundances in the north atlantic ocean as revealed by an improved quantitative PCR method. Appl. Environ. Microbiol. 72, 723–732. doi: 10.1128/AEM.72.1.723-732.2006
Keywords: Synechococcus, marine cyanobacteria, glutamine synthetase, physiological regulation, glnA, glnN, gene expression
Citation: Domínguez-Martín MA, Díez J and García-Fernández JM (2016) Physiological Studies of Glutamine Synthetases I and III from Synechococcus sp. WH7803 Reveal Differential Regulation. Front. Microbiol. 7:969. doi: 10.3389/fmicb.2016.00969
Received: 06 April 2016; Accepted: 03 June 2016;
Published: 28 June 2016.
Edited by:
George S. Bullerjahn, Bowling Green State University, USAReviewed by:
David J. Scanlan, University of Warwick, UKRajeshwar P. Sinha, Banaras Hindu University, India
Copyright © 2016 Domínguez-Martín, Díez and García-Fernández. This is an open-access article distributed under the terms of the Creative Commons Attribution License (CC BY). The use, distribution or reproduction in other forums is permitted, provided the original author(s) or licensor are credited and that the original publication in this journal is cited, in accordance with accepted academic practice. No use, distribution or reproduction is permitted which does not comply with these terms.
*Correspondence: José M. García-Fernández, am1nYXJjaWFAdWNvLmVz