- 1UMR CNRS 7267, Ecologie et Biologie des Interactions, Université de Poitiers, Poitiers, France
- 2Leibniz Center for Tropical Marine Ecology, Bremen, Germany
- 3Rowland Institute at Harvard, Harvard University, Cambridge, MA, USA
Bacterial symbionts represent essential drivers of arthropod ecology and evolution, influencing host traits such as nutrition, reproduction, immunity, and speciation. However, the majority of work on arthropod microbiota has been conducted in insects and more studies in non-model species across different ecological niches will be needed to complete our understanding of host–microbiota interactions. In this review, we present terrestrial isopod crustaceans as an emerging model organism to investigate symbiotic associations with potential relevance to ecosystem functioning. Terrestrial isopods comprise a group of crustaceans that have evolved a terrestrial lifestyle and represent keystone species in terrestrial ecosystems, contributing to the decomposition of organic matter and regulating the microbial food web. Since their nutrition is based on plant detritus, it has long been suspected that bacterial symbionts located in the digestive tissues might play an important role in host nutrition via the provisioning of digestive enzymes, thereby enabling the utilization of recalcitrant food compounds (e.g., cellulose or lignins). If this were the case, then (i) the acquisition of these bacteria might have been an important evolutionary prerequisite for the colonization of land by isopods, and (ii) these bacterial symbionts would directly mediate the role of their hosts in ecosystem functioning. Several bacterial symbionts have indeed been discovered in the midgut caeca of terrestrial isopods and some of them might be specific to this group of animals (i.e., Candidatus Hepatoplasma crinochetorum, Candidatus Hepatincola porcellionum, and Rhabdochlamydia porcellionis), while others are well-known intracellular pathogens (Rickettsiella spp.) or reproductive parasites (Wolbachia sp.). Moreover, a recent investigation of the microbiota in Armadillidium vulgare has revealed that this species harbors a highly diverse bacterial community which varies between host populations, suggesting an important share of environmental microbes in the host-associated microbiota. In this review, we synthesize our current knowledge on the terrestrial isopod microbiome and identify future directions to (i) fully understand the functional roles of particular bacteria (both intracellular or intestinal symbionts and environmental gut passengers), and (ii) whether and how the host-associated microbiota could influence the performance of terrestrial isopods as keystone species in soil ecosystems.
Introduction
Over the last decade, research on animal–bacterial symbioses has shifted from its historical focus on binary host–symbiont interactions to a more holistic perspective, taking into account that symbioses are shaped by complex multipartite interactions between hosts and diverse symbiotic communities (the microbiome), partly acquired from the environment. This “microbiome revolution” (Blaser, 2014) was mainly driven by the advent of new high-throughput DNA sequencing technologies, which enabled us to appreciate the diversity and ubiquity of animal-associated microbiomes as an essential component of host biology as well as a source of evolutionary novelty (Ley et al., 2008; Fraune and Bosch, 2010; McFall-Ngai et al., 2013). Hence, it is now established that the microbiome can modulate host development (Shin et al., 2011), nutrition (Chandler et al., 2011; He et al., 2013; Wong et al., 2014), immunity (Chu and Mazmanian, 2013), vector competence and susceptibility to pathogen infection (Dong et al., 2009; Koch and Schmid-Hempel, 2012), and speciation (Brucker and Bordenstein, 2012, 2013).
The majority of studies on arthropod microbiomes have been conducted in insects and have made enormous contributions to our understanding of intricate host–symbiont relationships. For instance, many insects feeding on nutrient-deficient diets such as plant sap or vertebrate blood maintain long-lasting associations with heritable obligate mutualistic endosymbionts (primary symbionts) which provide essential nutrients (amino acids and vitamins) lacking from the host’s diet (Thao and Baumann, 2004; Moran et al., 2005; Pais et al., 2008; McCutcheon et al., 2009; McCutcheon and Moran, 2010). These symbionts are usually harbored in specialized cells (the bacteriocytes), which may form an additional host organ, the bacteriome. An even higher number of species harbor facultative intracellular symbionts (secondary symbionts). These are generally not required for host survival and reproduction, but may be beneficial under certain environmental conditions, for instance through increased thermal tolerance, host plant speciation, predator avoidance and defense against natural enemies and parasites (Montllor et al., 2002; Oliver et al., 2003, 2005; Ferrari et al., 2004; Tsuchida et al., 2004, 2010; Scarborough et al., 2005; Jaenike et al., 2010; Lukasik et al., 2013). Other facultative bacteria act as reproductive parasites and manipulate host reproduction in order to promote their own vertical transmission from mother to offspring (Duron et al., 2008; Hurst and Frost, 2015). The most common phenotype is cytoplasmic incompatibility (CI), a reproductive incompatibility between sperm and egg preventing normal mitosis (Tram and Sullivan, 2002; Serbus et al., 2008). Other reproductive manipulations result in female-biased sex-ratios that increase the number of infected females in host populations (via parthenogenesis, male-killing, or the feminization of genetic males), thereby enhancing maternal symbiont transmission (Stouthamer et al., 1993; Hurst et al., 2003; Narita et al., 2007; Bouchon et al., 2008). Bacteria of the genus Wolbachia are by far the most frequently encountered reproductive parasites and manifest the widest spectrum of reproductive manipulations (Hilgenboecker et al., 2008; Werren et al., 2008; Sicard et al., 2014b).
When considering the wider microbial community beyond heritable endosymbionts, many insect microbiotas are of relatively low diversity but highly specialized, such as in bees and flies (Koch and Schmid-Hempel, 2011; Martinson et al., 2011; Wong et al., 2011). At the other end of the spectrum are the termites with their highly diverse hindgut community of bacteria, archaea, and protozoans involved in lignocellulose digestion (Hongoh, 2010; He et al., 2013; Peterson et al., 2015). However, only very few studies have investigated microbiome patterns across ecologically diverse groups of insects, with the exception of Colman et al. (2012). Their study demonstrated that microbiota composition was strongly influenced by host diet in various insects. Specifically, bacterial diversity was highest in detritivorous and xylophagous species from different insect orders (termites and beetles) and their microbiotas were more similar to each other compared to insects with other diets. Overall, however, more studies in diverse non-model arthropods across different ecological niches will be needed to complete our understanding of host–microbiota interactions beyond the insects. In this review, we present terrestrial isopod crustaceans as an emerging model organism to investigate arthropod–bacteria symbioses of evolutionary and ecological relevance.
Terrestrial Isopod Ecology and Evolution
From an evolutionary perspective, oniscidean isopods are the most successful colonizers of terrestrial habitats among the Crustacea, comprising around 3700 species (Schmalfuss, 2003). Originating from the marine littoral zone, they have colonized almost all types of terrestrial environments on Earth, including deserts, except for high alpine and polar regions (Figure 1). This makes them excellent model organisms regarding evolutionary adaptations to a terrestrial lifestyle in terms of reproduction, respiration, nutrition, excretion systems, and protection against desiccation (reviewed in Hornung, 2011). The main morphological and physiological changes compared to marine crustaceans include (i) a reduction in body size, (ii) a water-resistant cuticle, (iii) pleopodal lungs, (iv) a water conducting system, and (v) development of juveniles in a closed brood pouch (marsupium; reviewed in Hornung, 2011). In addition, they had to adapt to the food sources available in terrestrial environments (reviewed in Zimmer, 2002). Extant terrestrial isopods are detritivores, i.e., they feed on dead and decaying organic matter. Therefore, they represent a keystone species for plant litter decomposition in terrestrial ecosystems, contribute to nutrient cycling and regulate soil microbial activity (Zimmer and Topp, 1999; Zimmer, 2002; Crowther et al., 2015).
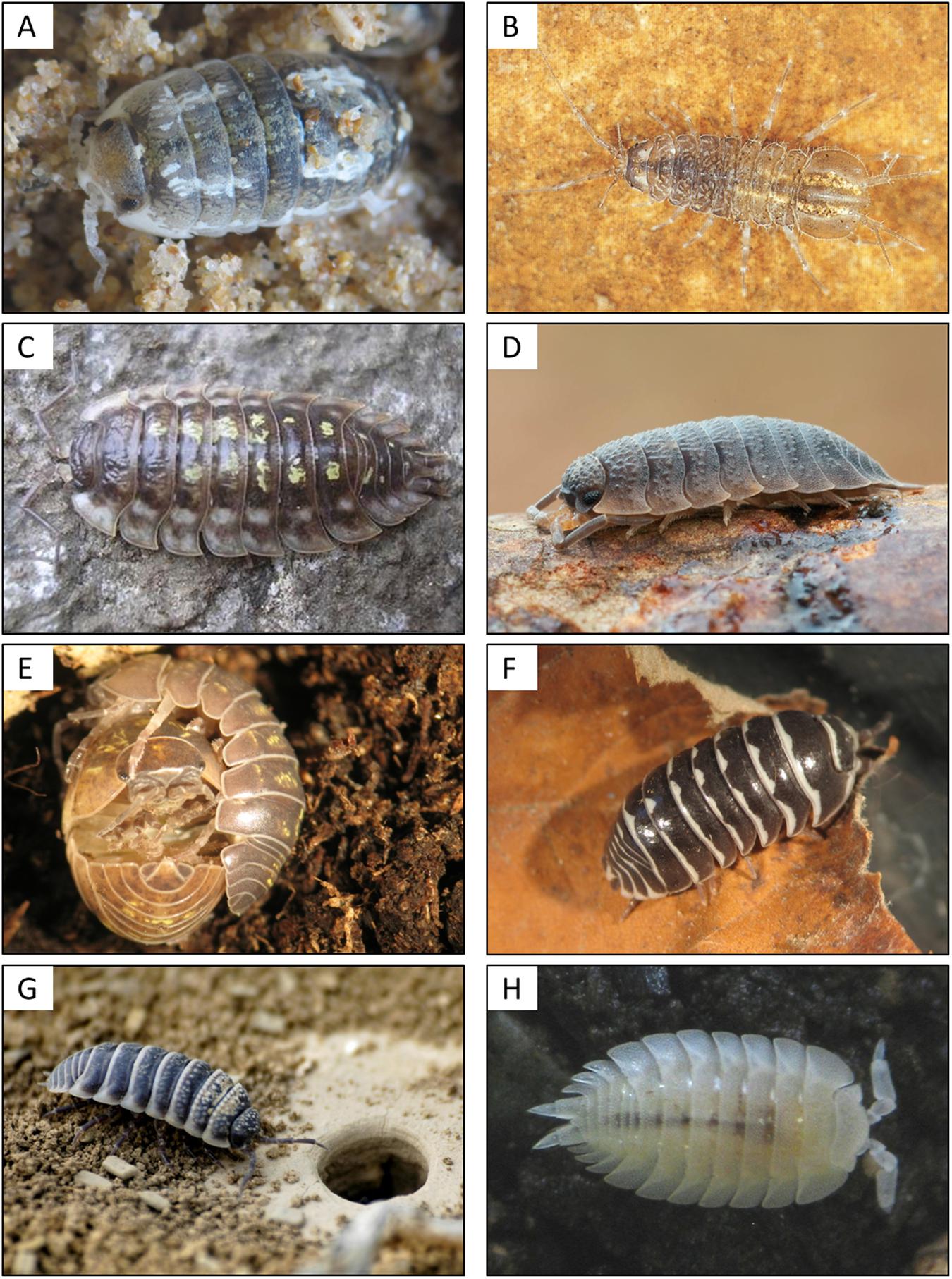
FIGURE 1. Diversity of terrestrial isopods from littoral to desert habitats. (A) The semi-terrestrial Tylos europaeus, inhabiting the littoral zone; (B) the freshwater isopod Asellus aquaticus; (C) the common woodlouse Oniscus asellus; (D) the rough woodlouse Porcellio scaber; (E) the pillbug Armadillidum vulgare during mating; (F) the “zebra pillbug” Armadillidium maculatum; (G) the desert isopod Hemilepistus reaumuri near the entrance of a burrow in Tunisia; and (H) the ant woodlouse Platyarthrus hoffmannseggii. This species is blind and lives in ant nests. Picture credit: Martin Zimmer (B), Sören Franzenburg (D), Giuseppe Montesanto (G), UMR CNRS 7267 (A,C,E,F,H).
While plant material represents one of the most abundant sources of fixed carbon and nitrogen on the planet, it mainly consists of complex lignocellulose polymers that hinder the digestive utilization of this carbon and nitrogen. Obtaining nutrients from these compounds requires a complex set of enzymes allowing the degradation of lignins as well as cellulose. Historically, it has long been believed that the majority of animals do not possess the necessary enzymatic machinery and instead rely on symbiotic microorganisms (e.g., ruminants or termites). However, this view is increasingly challenged by the detection of endogenous cellulases in various animals (reviewed in Watanabe et al., 1998; Davison and Blaxter, 2005; Cragg et al., 2015), including isopod crustaceans (King et al., 2010; Kostanjsek et al., 2010; Kern et al., 2013). Nonetheless, most animal species studied to date still rely on symbiont-derived enzymes for cellulose degradation, either alone or in combination with host-derived endogenous enzymes (Cragg et al., 2015). Therefore, the role of bacteria (both environmental bacteria and symbionts located in the digestive tissues) in terrestrial isopod nutrition and fitness has attracted considerable attention in the last decades. First, isopods are constantly in contact with environmental microbes in the soil or ingested with their plant food sources and/or via feeding on their congeners’ feces (coprophagy; Gunnarsson and Tunlid, 1986; Zimmer and Topp, 2000; Zimmer et al., 2003; Ihnen and Zimmer, 2008). Second, it has long been suspected that bacterial symbionts located in the digestive tissues might play an important role in host nutrition via the provisioning of digestive enzymes (Zimmer and Topp, 1998a,b; Zimmer et al., 2001, 2002; Zimmer and Bartholmé, 2003). If this were the case, then (i) the acquisition of these bacteria might have been an important evolutionary prerequisite for the colonization of land by isopods, and (ii) these bacterial symbionts would directly mediate how their hosts contribute to important ecosystem processes such as decomposition and nutrient cycling. Several bacterial symbionts have indeed been discovered in the midgut caeca and the hindgut of terrestrial isopods and some of them might be specific to this group of animals (i.e., Candidatus Hepatoplasma crinochetorum, Candidatus Hepatincola porcellionum, and Rhabdochlamydia porcellionis; Kostanjsek et al., 2004b, 2007; Wang et al., 2004a,b), while others are well-known intracellular pathogens (Rickettsiella spp.) or reproductive parasites (Wolbachia sp.) infecting various insects as well (Werren et al., 2008; Bouchon et al., 2011; Leclerque and Kleespies, 2012; Kleespies et al., 2014; Sicard et al., 2014b). Moreover, a recent investigation of the microbiota in Armadillidium vulgare has revealed a high bacterial density and diversity in various tissues (Dittmer et al., 2014, 2016). In this review, we synthesize our current knowledge regarding the functional roles of particular bacteria in the terrestrial isopod microbiome (both intracellular or intestinal symbionts and environmental bacteria) and discuss to what extent this microbiome could (i) influence the performance of terrestrial isopods as keystone species of the soil macrofauna and (ii) represent a potential source of adaptive capacity in changing environments.
A Diverse Symbiont Assembly
As for many symbioses, the association between isopods and microorganisms has initially been viewed as highly specific binary interactions between one host and one symbiont. We present the current knowledge of bacterial symbionts described in terrestrial isopods. Most of these bacteria where first discovered through microscopic observations and/or through ecological traits and all of them are intracellular or extracellular symbionts that are facultative for the host. Nicely illustrating the initial concept of symbiosis introduced by de Bary (1879), they represent a large scope of host–symbiont associations, including reproductive parasites (Wolbachia), bacterial pathogens (Rickettsiella and Rhabdochlamydia) and several symbionts specifically associated with digestive tissues, namely Candidatus Hepatincola, Candidatus Hepatoplasma, and Candidatus Bacilloplasma. Although most research has been dedicated to bacterial symbionts of terrestrial isopods, we expand this section to include viruses and acanthocephalan parasites known to infect this group of animals.
Wolbachia, A Model to Study Genetic Conflicts
Wolbachia pipientis (hereafter Wolbachia, Figure 2) are obligate intracellular Alphaproteobacteria (order Rickettsiales) that are widespread in arthropods and also found in nematodes (Werren et al., 2008). Their capability to manipulate host reproduction has received considerable attention and has established them as reproductive parasites. Presence of Wolbachia in isopods was first observed in the pill bug A. vulgare as Rickettsia-like bacteria (Martin et al., 1973) that were later formally assigned to Wolbachia (Rigaud et al., 1991; Rousset et al., 1992). Terrestrial isopods have long been known to be prone to reproductive alterations such as intersexuality, sex ratio distortions or incompatible crosses (Legrand et al., 1987). In this context, identifying Wolbachia in A. vulgare emerged from a long series of studies looking for both physiological and genetic factors responsible for sex ratio distortions (reviewed in Bouchon et al., 2008).
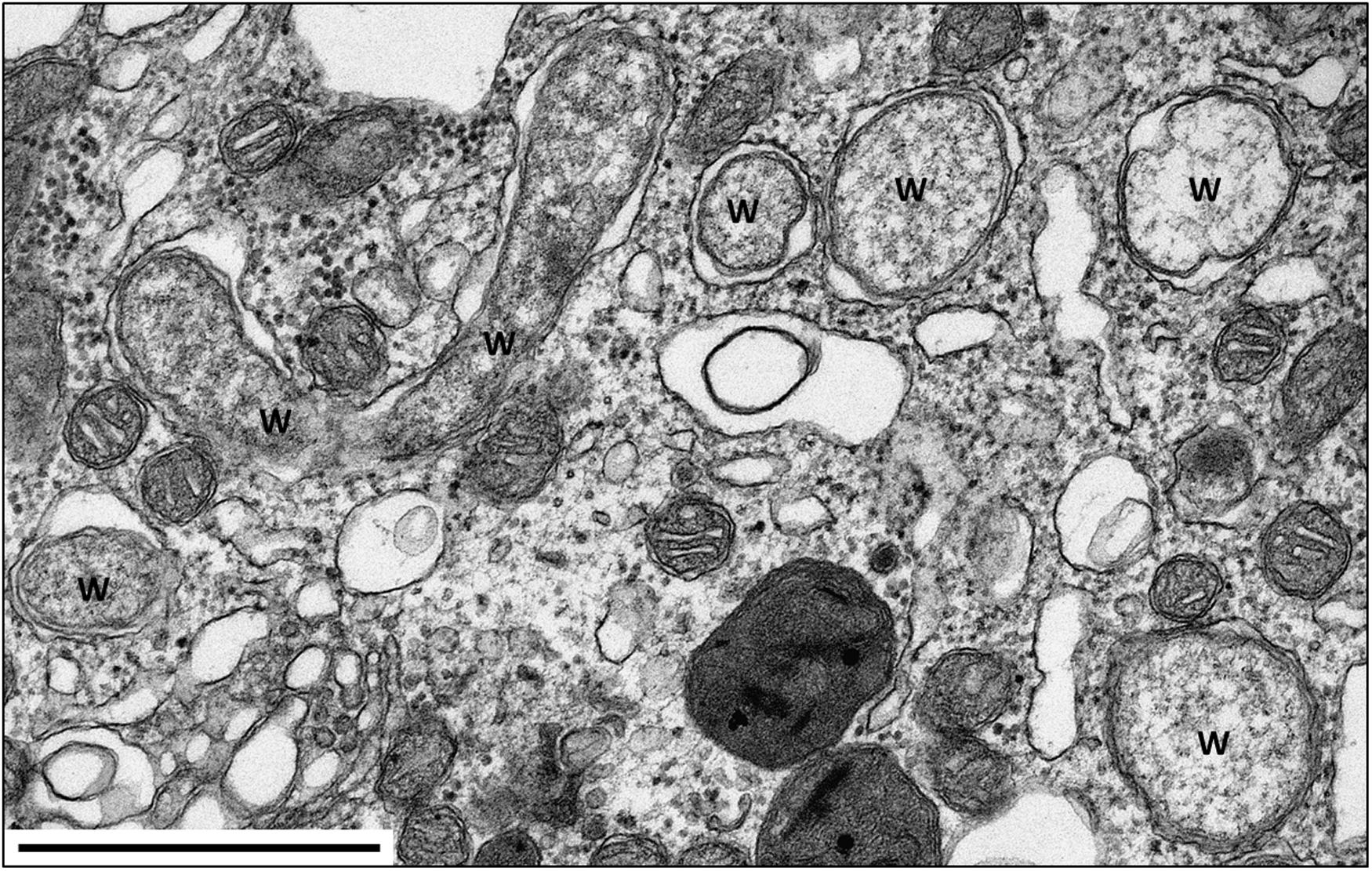
FIGURE 2. TEM of Wolbachia in the nerve cord of A. vulgare. W = Wolbachia. Scale bar: 1 μm. Picture credit: Maryline Raimond.
The most extensive investigations have been performed on A. vulgare where, as observed in numerous terrestrial isopods, unisexual progenies are frequent. Taking advantage of the fact that isopods are highly tractable, especially for grafting experiments, sex reversals can be easily achieved through experimental tissue implants, indicating that sex determination and sex differentiation are very labile. Such experiments were used to demonstrate that A. vulgare females are heterogametic (WZ) and that unusual genetic combinations (WW or ZZ) are viable and fertile (Legrand et al., 1987). The corpus of these investigations led to propose an integrative model of the evolution of sex determination where Wolbachia plays a central role (Rigaud et al., 1997; Cordaux et al., 2011).
To date, three Wolbachia strains (wVulC, wVulM, and wVulP) have been identified in the pill bug (Cordaux et al., 2004; Verne et al., 2007). These Wolbachia are generally present in all tissues including gonads, hemocytes, nervous and digestive tissues (Dittmer et al., 2014; Figures 2 and 5). Interestingly, quantitative investigations also showed that the within-host Wolbachia distribution depends on both the Wolbachia strain and the tissue type, but not on host genotype (Dittmer et al., 2014). While it is expected that Wolbachia are most abundant in the ovaries, the target tissue for transgenerational transmission of obligate endosymbionts, the systemic infection of Wolbachia in terrestrial isopods could also play a role in the association (Sicard et al., 2014b): when transmitted from mothers to offspring, Wolbachia reverse male embryos into functional females, sometimes leading to all-female progenies. This remarkable aptitude to infect offspring may rely on a re-infection of ovaries from somatic tissues instead of direct cellular segregation between oogonia and oocytes (Genty et al., 2014). This manipulation called feminization is a Wolbachia strategy (or extended phenotype) to optimize their vertical transmission. As feminized ZZ males produce females without transmitting any W chromosome, the outcome is the rapid elimination of the W chromosome from infected populations. Therefore, in a population where all individuals are homogametic ZZ, the sex determination is under the control of Wolbachia. Hence, the A. vulgare/Wolbachia interaction is a perfect example of cytoplasmic sex determination (Rigaud et al., 1997; Cordaux et al., 2011). The precise molecular mechanism of feminization in A. vulgare still remains unknown but is achieved by preventing androgenic gland differentiation, the tissue where the androgenic (masculinizing) hormone (AH) is synthetized (Grève et al., 2004; Cerveau et al., 2014). The key role of the AH has been demonstrated by means of androgenic gland implants and injections of AH extract into young females, which were then reversed into functional males. When doing the same experiments with young females infected with Wolbachia, no sex reversal was recorded. Therefore, feminization could result from inhibition of androgenic gland development by targeting the hormonal sex differentiation in A. vulgare.
Apart from terrestrial isopods, Wolbachia-mediated feminization has only been observed in three insects: in Eurema butterflies (Hiroki et al., 2002; Narita et al., 2007; Kern et al., 2015), the leafhopper Zyginidia pullula (Negri et al., 2006, 2009) and the moth Ostrinia scapulalis (Sugimoto and Ishikawa, 2012), although the latter case is more complex in that Wolbachia achieves male-killing through a feminizing factor which induces the female splice variant of the sex determination gene doublesex. This results in a lethal mismatch between genetic and phenotypic sex determination in male embryos (Sugimoto and Ishikawa, 2012). In Eurema butterflies, on the other hand, Wolbachia acts at a later stage and achieves feminization during larval development through an as yet unknown mechanism (Narita et al., 2007), whereas Wolbachia reverses the sex-specific genome methylation pattern in Z. pullula males (Negri et al., 2009). Together, these observations in insects and terrestrial isopods illustrate Wolbachia’s ability to achieve the same phenotype in hosts with diverse sex determination systems.
Feminization is not the only Wolbachia-mediated manipulation observed in terrestrial isopods. To date, CI, a post-zygotic reproductive isolation frequently induced by Wolbachia in insects, has also been demonstrated in the three isopod species Porcellio dilatatus petiti, Porcellio dilatatus dilatatus, and Cylisticus convexus (Legrand et al., 1978; Moret et al., 2001; Sicard et al., 2014a). Wolbachia-induced CI appears when crosses between infected males and uninfected females (or females infected with a different Wolbachia strain) are unfertile. As for feminization, the precise mechanism used by Wolbachia to induce CI has not yet been unraveled. However, the expected consequence of this phenotype is to reduce gene flow between groups of individuals that differ regarding their Wolbachia infection status, possibly playing a role in speciation (Bordenstein et al., 2001; Brucker and Bordenstein, 2012).
Overall, Wolbachia have been identified in a large set of terrestrial isopods with an estimated prevalence of about 60%, revealing a pandemic distribution in this group of animals (Bouchon et al., 2008; Cordaux et al., 2012). Wolbachia form a monophyletic lineage in which 16 phylogenetic supergroups (from A to Q) have been identified to date (Ramírez-Puebla et al., 2015). Until recently, all isopod-borne Wolbachia strains, mostly sampled in temperate regions, belonged to the B supergroup (Cordaux et al., 2004, 2012; Bouchon et al., 2008). However, a recent survey of Wolbachia in neotropical isopod species revealed the presence of strains from A and F supergroups (Zimmermann et al., 2015) and we may expect to uncover an even higher Wolbachia diversity by screening terrestrial isopods in regions that have not been extensively studied thus far. The pandemic infection of Wolbachia in terrestrial isopods might be facilitated by the ability of these bacteria to switch from one host to another (i.e., horizontal transmission). This has been demonstrated in terrestrial isopods where Wolbachia transinfections have been achieved through hemolymph contact (Rigaud and Juchault, 1995), cannibalism or predation (Le Clec’h et al., 2013a). Cannibalism is quite common in terrestrial isopods and these investigations demonstrate that Wolbachia are capable of crossing the intestine barrier and to survive, at least transiently, in a new host. These observations are congruent with the general view inferred from phylogenetic studies that the wide distribution of Wolbachia in terrestrial isopods may result from successful host switching over evolutionary time scales (Bouchon et al., 1998; Cordaux et al., 2001; Zimmermann et al., 2015). Moreover, experimental transinfections have demonstrated that host switching could increase the virulence of Wolbachia (Le Clec’h et al., 2012, 2013b).
Is Wolbachia only a sex parasite? Due to its endocellular lifestyle, Wolbachia has to face the host’s immune defenses (Sicard et al., 2014b). However, live Wolbachia have been observed in the hemocytes (immune cells) of A. vulgare (Braquart-Varnier et al., 2008; Chevalier et al., 2011). Moreover, hematopoietic organs that produce hemocytes are also infected (Chevalier et al., 2011; Braquart-Varnier et al., 2015b), constituting a niche where Wolbachia could potentially initiate and maintain its systemic infection. These intriguing observations raise the question of the efficiency of the host’s immune response. In fact, a negative impact of Wolbachia on host immune competence has been demonstrated in A. vulgare and P. dilatatus, decreasing the survival of infected individuals (Braquart-Varnier et al., 2008; Pigeault et al., 2014). Both cellular (i.e., hemocytes density) and humoral parameters (i.e., phenoloxidase activity) of the host immune response are impacted, leading to septicemia (Sicard et al., 2010). Immune response is also impacted at the molecular level: genes encoding antimicrobial peptides or involved in pathogen recognition, detoxification and autophagy are upregulated in hemocytes of infected individuals, whereas the same gene set is downregulated in ovaries or in whole bodies (Chevalier et al., 2012). These findings underline the fact that each tissue represents a particular niche with specific interactions between the host and Wolbachia. Therefore, hosts should be considered as complex ecosystems composed of a mosaic of microhabitats (Dittmer et al., 2014, 2016; Sicard et al., 2014b). Meanwhile, it has been demonstrated that Wolbachia can also confer protection against pathogenic bacteria by inducing a better resistance to pathogen infection in A. vulgare and P. dilatatus (Braquart-Varnier et al., 2015a), similar to Wolbachia-mediated protection against viruses and Plasmodium parasites in various insects (Hedges et al., 2008; Teixeira et al., 2008; Moreira et al., 2009; Osborne et al., 2009; Hughes et al., 2011; Blagrove et al., 2012; Bian et al., 2013). Taken together, the phenotype of the association between Wolbachia and isopods is the result of a subtle balance between detrimental and beneficial effects on host fitness, depending on how we look at the interactions.
Rickettsiella, A Model to Study Transitions between Pathogenicity and Mutualism?
Bacteria of the genus Rickettsiella are Gammaproteobacteria closely related to Legionella and Coxiella (Roux et al., 1997; Cordaux et al., 2007; Leclerque, 2008). They are facultative endosymbionts that are mainly known as arthropod pathogens (reviewed in Bouchon et al., 2011). Rickettsiella-induced diseases were first reported from the Japanese beetle Popillia japonica (Dutky and Gooden, 1952) and since then have been described from numerous insects encompassing lepidopterans, orthopterans, dipterans, dyctiopterans, coleopterans, hymenopterans and hemipterans, as well as arachnids and crustaceans (reviewed in Bouchon et al., 2011). Vago et al. (1970) first described a Rickettsiella infection in terrestrial isopods in specimens of A. vulgare and therefore proposed the pathotype designation ‘R. armadillidii.’ Since then, Rickettsiella diseases have been reported in numerous terrestrial isopod species (Figure 3), i.e., A. vulgare, A. nasatum, A. granulatum, Eluma purpurascens, Oniscus asellus, Porcellio laevis, and Porcellio gallicus (Yousfi, 1976), Helleria brevicornis and Philoscia muscorum (Cordaux et al., 2007); P. dilatatus (Federici, 1984), Porcellio scaber (Abd and Holdich, 1987; Kleespies et al., 2014) and Atlantoscia floridana (Figure 3B). Rickettsiella have also been detected in the freshwater isopod Asellus aquaticus (Wang et al., 2007). As in other arthropod hosts, Rickettsiella cause a slowly developing but highly contagious disease in terrestrial isopods, resulting in death of infected individuals (Vago et al., 1970; Bouchon et al., 2011). Diseased individuals are easy to recognize in the later stages of infection due to a white substance in the body cavity that is visible through the ventral body surface.
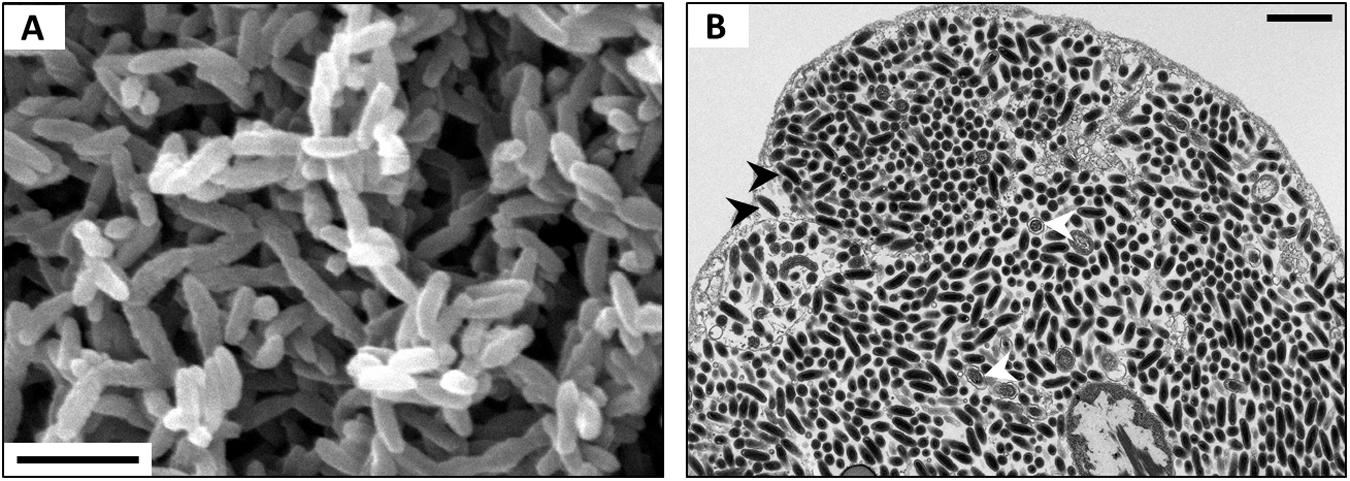
FIGURE 3. (A) SEM of Rickettsiella in the hemolymph of P. scaber. (B) TEM of Rickettsiella in an ovary of Atlantoscia floridana, showing bacterial cells of two different stages: rod-shaped Elementary Bodies (black arrowheads) and replicative Reticulate Bodies (white arrowheads). Scale bar: 1 μm. Picture credit: Maryline Raimond (A,B) and Bianca Lais Zimmermann (B).
Historically, there has been a lot of confusion regarding taxonomic diversity and phylogenetic relationships within the genus Rickettsiella (Roux et al., 1997; Cordaux et al., 2007; Leclerque, 2008). As such, the entire genus Rickettsiella had been initially misassigned to the order Rickettsiales, a group of intracellular Alphaproteobacteria (Weiss et al., 1984), or alternatively to the Chlamydiales (Federici, 1980b). Indeed, microscopic observations of symptomatic specimens from diverse hosts have described Rickettsiella replication cycles similar in many aspects to the characteristic cycle of the Chlamydiales. Moreover, Rickettsiella species denominations have generally been made based on host species or pathotype, leading to confusing and controversial taxonomic identifications. This is illustrated by the Rickettsiella genome sequencing project (Genome Project AAQJ00000000): while this Rickettsiella strain had been isolated from a terrestrial isopod, it was named after the cricket pathogen Rickettsiella grylli, although these two strains are only distantly related based on molecular markers (Leclerque and Kleespies, 2012; Kleespies et al., 2014). Another case of taxonomic misclassification is exemplified by symbionts of the tick Ixodes ricinus, which were initially identified as representatives of a new genus called ‘Diplorickettsia’ (Diplorickettsia massiliensis; Mediannikov et al., 2010), but later included within the genus Rickettsiella (Leclerque and Kleespies, 2012; Kleespies et al., 2014). Most importantly, D. massiliensis has been identified in three human patients with suspected tick-borne infections, thus establishing its role as a human pathogen (Subramanian et al., 2012). This situation highlights that Rickettsiella is still poorly studied and that our knowledge is based on patchy observations across diverse host organisms.
Over the last decade, however, a growing body of molecular data has been obtained from diverse arthropods as well as environmental samples. Based on the current 16S rRNA gene phylogeny, the most widely used marker gene, the Rickettsiella genus appears monophyletic but highly diverse, including sub-clades that do not follow the pathotypes nor host origin (Kleespies et al., 2014). These data confirm that Rickettsiella are widespread, not only detected in symptomatic hosts but also in healthy individuals and in the environment [e.g., rivers, sea water, and even in aerosol samples (Kleespies et al., 2014)]. In terrestrial isopods, Rickettsiella are observed in genetically diverse hosts (Cordaux et al., 2007; Bouchon et al., 2011; Kleespies et al., 2014), reflecting extensive horizontal transfers that might be facilitated by the ability of these bacteria to survive in the soil outside of their hosts for years (Hurpin and Robert, 1976).
The versatility of the Rickettsiella is also demonstrated regarding their phenotypes and biological traits. Non-pathogenic Rickettsiella strains, phylogenetically distinct from the pathogenic Rickettsiella, have recently been reported in the pea aphid Acyrthosiphon pisum (Tsuchida et al., 2010, 2014). These Rickettsiella are vertically transmitted through transovarial passage, behave as mutualists with no detrimental effects on their hosts and confer protection against predators by changing their host’s body color (Tsuchida et al., 2010, 2014). Vertically transmitted Rickettsiella have also been identified in the leafhopper Orosius albicinctus (Iasur-Kruh et al., 2013). In the same manner, Rickettsiella that are very common and diverse in ticks, are present in eggs laid by infected females, suggesting vertical transmissions (Duron et al., 2016).
In conclusion, the biology of most Rickettsiella strains remains to be characterized but our growing knowledge on diversity and phylogeny, although incomplete, suggests that members of the Rickettsiella genus use diverse strategies to spread and persist in host populations. Overall, Rickettsiella appears as a very promising model to study evolutionary transitions between pathogenicity and mutualism.
Rhabdochlamydia porcellionis, A Model to Study Complex Pathogen Life Cycles
Rhabdochlamydia porcellionis are obligate intracellular bacteria so far only observed in the midgut caeca (hepatopancreas) of P. scaber (Shay et al., 1985; Drobne et al., 1999; Kostanjsek et al., 2004b). Together with the cockroach pathogen Rhabdochlamydia crassificans (Corsaro et al., 2007), R. porcellionis represents a new family (the Rhabdochlamydiacaea) within the phylum Chlamydiae (reviewed in Horn, 2008). The Chlamydiae are obligate intracellular pathogens infecting a wide range of Eukaryotes, including protists (amoebae), insects, fishes, mammals, and humans (reviewed in Horn, 2008). They have probably lived in association with eukaryotic hosts for 100s of millions of years and may even have played an essential role in the establishment of plastids in the primary photosynthetic Eukaryote, despite being absent from extant plants (Huang and Gogarten, 2007). The Rhabdochlamydiacaea are most closely related (87% 16S rRNA gene sequence similarity) to the family Simkaniacaea, comprising the two bacteriocyte-associated insect endosymbionts Candidatus Fritschea bemisiae from whiteflies and Candidatus Fritschea eriococci from scale insects (Thao et al., 2003; Everett et al., 2005; Horn, 2008). In line with this phylogenetic position, R. porcellionis can be successfully cultured in insect cell lines, indicating its capacity to infect diverse arthropod hosts (Sixt et al., 2013).
Rhabdochlamydia porcellionis exhibits the complex developmental cycle that is characteristic for all Chlamydiae, consisting of replicative reticulate bodies (RBs), intermediate bodies (IBs), and infectious elementary bodies (EBs; Drobne et al., 1999; Kostanjsek et al., 2004b). EBs are rod-shaped cells with a dense cytoplasm and a distinctive, five-layered cell wall. After infection of a host cell, they develop into RBs – large, spherical cells with a three-layered cell wall that replicate within intracellular membrane-bound vacuoles. During the advanced stages of infection, aggregations of vacuoles densely packed with bacteria accumulate in the infected cell, leaving visible signs of infection (white spots) on the surface of the midgut caeca (Drobne et al., 1999; Kostanjsek et al., 2004b). At the end of the infection cycle, RBs redifferentiate into a new generation of infectious elementary bodies and vacuoles are released into the lumen of the hepatopancreas, disrupting the cell membrane in the process (Drobne et al., 1999; Kostanjsek et al., 2004b). Based on this infectious life cycle and the damage caused to the host tissue, the relationship between R. porcellionis and its isopod host is clearly parasitic. Moreover, this bacterium has been shown to inhibit apoptosis as a defense mechanism in infected insect cell lines (Sixt et al., 2013). However, many aspects of this symbiosis, including transmission routes and prevalence in other terrestrial isopod species, remain to be elucidated.
Hepatincola and Hepatoplasma, Models to Study Nutritional Symbiosis and Symbiont-Symbiont Interactions?
The hepatopancreas (digestive midgut glands) of terrestrial isopods is responsible for the production and secretion of digestive enzymes and involved in the absorption of digestively released nutrients. Moreover, this organ has been demonstrated to harbor dense populations of bacteria in P. dilatatus (Donadey and Besse, 1972), P. scaber (Wood and Griffiths, 1988; Hames and Hopkin, 1989; Zimmer and Topp, 1998a,b; Zimmer, 1999), Oniscus asellus (Wood and Griffiths, 1988; Hames and Hopkin, 1989), and Ligia pallasii (Zimmer et al., 2001). Based on feeding experiments and correlations between digestive processes and bacterial densities, hepatopancreatic bacteria have been suggested to be involved in the hydrolysis of cellulose (Zimmer and Topp, 1998a; Zimmer et al., 2002) and the oxidative breakdown of lignins (Zimmer and Topp, 1998b) and tannins (Zimmer, 1999). Therefore, hepatopancreatic symbionts of terrestrial isopods might enable isopods to digest leaf litter and contribute directly to a fundamental ecosystem process, namely litter decomposition. If symbionts facilitate the digestion of leaf litter in terrestrial isopods (Zimmer and Topp, 1998a,b), the existence of hepatopancreatic bacteria might have aided the colonization of terrestrial habitats and thus the evolution of terrestrial isopods through subsequent adaptive radiation. The detritivorous freshwater isopod A. aquaticus has been shown to host a relatively diverse hepatopancreatic microbiota (Wang et al., 2007) that seems to contribute to cellulose hydrolysis and phenol oxidation (Zimmer and Bartholmé, 2003). It was only recently that bacterial inhabitants of the hepatopancreas were demonstrated in a marine isopod species, namely Idotea balthica feeding on angiosperm tissue in a low-salinity brackish environment (Zimmer et al., 2001, 2002; Wang et al., 2007; Mattila et al., 2014). Elimination of these bacteria through antibiotic treatment did not affect isopod growth on their angiosperm diet (Mattila et al., 2014), questioning a bacterial role in digestive processes (see above).
Two of these hepatopancreatic symbionts that occur extracellularly in the lumen and attach to the epithelial microvillous brush border of the cells via stalk-like appendages (Figure 4) were identified based on 16S rRNA gene sequences: Candidatus Hepatoplasma crinochetorum (Mollicutes, hereafter ‘Hepatoplasma’; Wang et al., 2004a) and Candidatus Hepatincola porcellionum (Rickettsiales, hereafter ‘Hepatincola’; Wang et al., 2004b). Since then, Hepatoplasma has been detected in all Oniscidean species tested for hepatopancreatic bacteria (except Ligidium hypnorum), whereas Hepatincola has only been observed in species of the Crinocheta (the most terrestrial group; Fraune and Zimmer, 2008). No individual specimen was found to host both symbionts simultaneously (Wang et al., 2007; Fraune and Zimmer, 2008), suggesting some, albeit hitherto unknown, mechanism of mutual exclusion. The intertidal species Ligia oceanica can harbor either Hepatoplasma or Pseudomonas sp. in their hepatopancreas (Wang et al., 2007; Fraune and Zimmer, 2008). Similarly, Hepatoplasma (but not Hepatincola) has been observed in two other intertidal species, Ligia occidentalis and L. pallasii (Eberl, 2010). The evolutionary or phylogenetic relevance of this finding remains unclear. According to highly congruent 16S rRNA- and 18S rRNA-based phylogenetic trees of Hepatoplasma from different isopod species and their isopod hosts, respectively, host–symbiont specificity and co-evolution with little horizontal exchange among host species has been assumed (Fraune and Zimmer, 2008). Taking into account that Hepatoplasma increases its host’s survival on a cellulosic low-quality diet (Fraune and Zimmer, 2008), the acquisition of this symbiont at an early stage of the evolution of terrestrial isopods may be considered a predisposition for the successful colonization of diverse land habitats and the use of terrestrial food sources. In Ligia oceanica, a representative of a prototypal isopod genus, gene sequences that resemble known carbohydrases of the GH45 family could be isolated from individuals harboring hepatopancreatic symbionts, but not from aposymbiotic individuals or from P. scaber (S. Fraune and M. Zimmer, personal communication). The latter species was later shown to possess an endogenous cellulase gene belonging to the GH9 family (Kostanjsek et al., 2010). The recently published genome of Hepatoplasma from A. vulgare (Leclercq et al., 2014) allows the identification of candidate genes that are potentially involved in cellulolytic processes: five coding sequences were assigned to enzyme classes defined by the Carbohydrate-Active Enzyme Database CAZy (Cantarel et al., 2009; Lombard et al., 2014), including the glycoside hydrolase family GH13, two glycosyltransferases and two carbohydrate esterases (Table 1; J. Dittmer, Ph.D. thesis; D. Bouchon, personal communication). However, the transcriptome of A. vulgare (Chevalier et al., 2012) also contains a set of genes of potentially cellulolytic enzymes (J. Dittmer, Ph.D. thesis; D. Bouchon, personal communication), including several orthologs of the endogenous cellulase gene of P. scaber (Kostanjsek et al., 2010). Functional analyses of these candidate genes will determine whether the cellulolytic genes of Hepatoplasma are partly redundant or instead complement endogenous host genes. Despite the fact that P. scaber possesses an endogenous cellulase gene (Kostanjsek et al., 2010), the cellulase activity inside its hindgut lumen correlates with the number of bacterial cells in the hepatopancreas (Zimmer and Topp, 1998a).
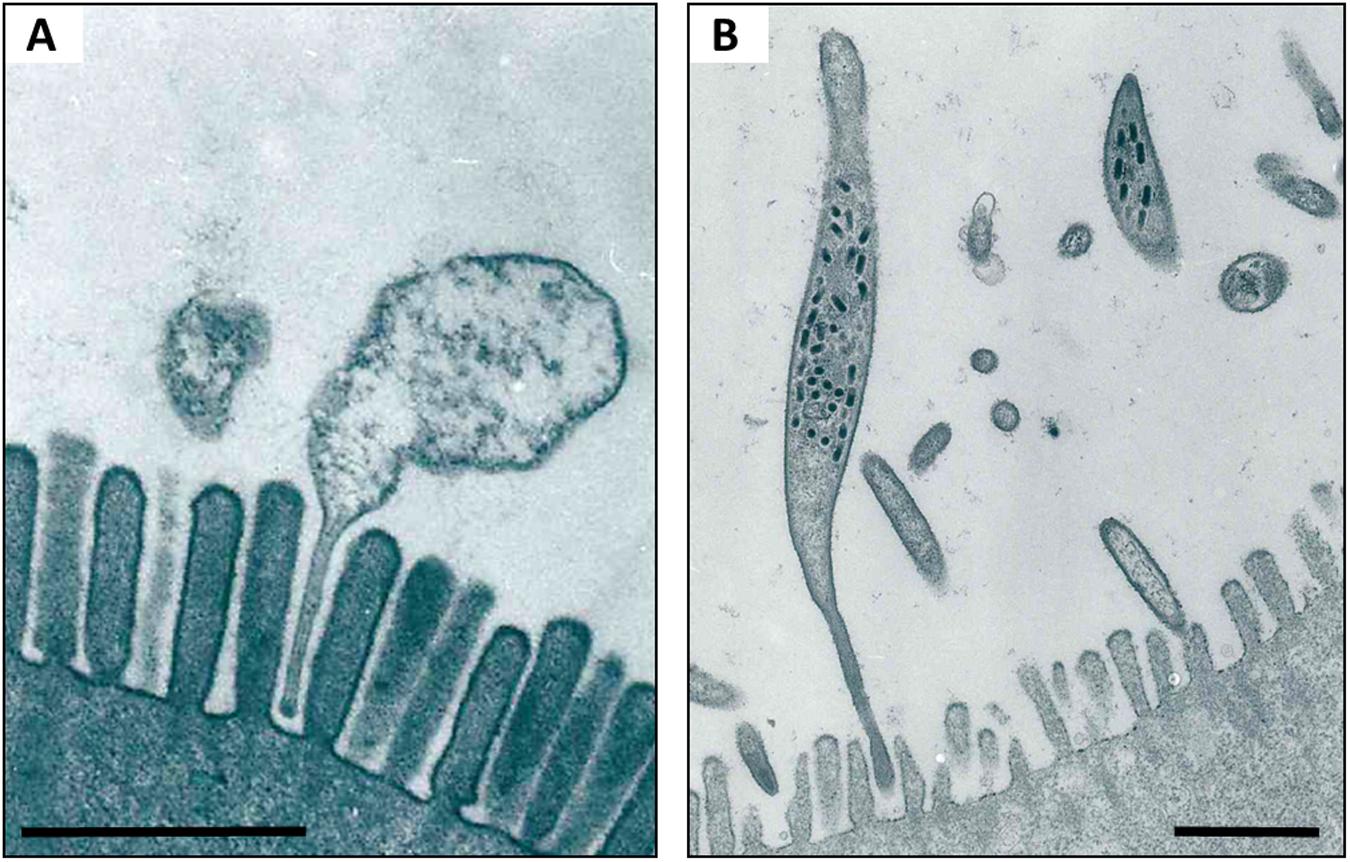
FIGURE 4. TEM of Hepatoplasma (A) and Hepatincola (B) in the lumen of the hepatopancreas (midgut glands) of P. scaber. Note how both bacteria attach to the microvillous border of the host cell via stalk-like appendages. Scale bars: 1 μm. Picture credit: Yongjie Wang.
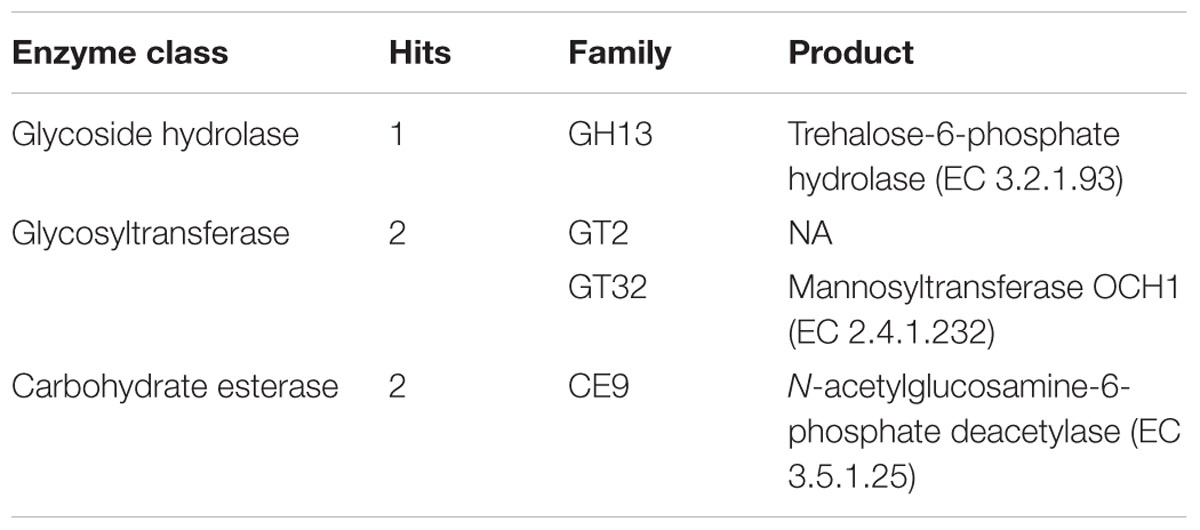
TABLE 1. Carbohydrate-active enzymes in the genome of Hepatoplasma crinochetorum based on the CAZy database (Cantarel et al., 2009; Lombard et al., 2014).
In contrast to Hepatoplasma, Hepatincola reduces its host’s longevity (Fraune and Zimmer, 2008). The relationship between Hepatincola and its isopod hosts is evolutionarily much younger than that of the more ancient Hepatoplasma (Wang et al., 2007). The presence of stalk-like cytoplasmic appendages that both Hepatoplasma and Hepatincola insert into the epithelial brush border of hepatopancreatic cells (Figure 4) has prompted the hypothesis that the evolutionarily young parasite (Hepatincola) invaded the evolutionarily ancient mutualistic relationship of its host with Hepatoplasma through molecular mimicry (Fraune and Zimmer, 2008). As mentioned above, the two symbionts seem to mutually exclude each other, and it is tempting to speculate that the presence of the mutualist prevents the parasite from colonizing the hepatopancreas.
Contradictory to the hypothesis that Hepatoplasma might have facilitated the success of isopods during the early stages of terrestrialization, this symbiont exhibits low prevalence in some populations (Wang et al., 2007). This is potentially due to environmental transmission rather than vertical transmission from mother to offspring (Wang et al., 2007). Hence, Hepatoplasma cells appear to be ingested along with inoculated food sources, through coprophagy or through cannibalism (Brandstädter and Zimmer, 2008). These observations suggest that Hepatoplasma might have become less relevant or redundant, potentially upon the evolutionary acquisition of endogenous cellulases by isopods (see above). Alternatively, this particular symbiosis may not have been significant for the evolutionary success of isopods on land because of its contribution to digestive processes but, if any, because of other beneficial roles, such as preventing pathogenic or parasitic infections.
From an evolutionary point of view, it is interesting to consider the presence of hepatopancreatic bacteria in thus far only one of the screened marine isopod species, and only in a population from a low-salinity brackish environment (Mattila et al., 2014). This observation is in line with Plante et al. (1990), reasoning that the conditions within marine invertebrate guts differ less from the external microbial environment than that in terrestrial or freshwater species, rendering it not necessarily advantageous for a marine bacterium to colonize the gut of an invertebrate instead of living in seawater. Further detailed studies on the suitability of marine and brackish isopod species as hosts for bacterial symbionts are warranted to better understand the evolutionary and ecological background of the symbiotic association of isopods and intestinal symbionts.
Bacilloplasma, A Model to Study Ancient Host–Symbiont Relationships?
Candidatus Bacilloplasma (hereafter ‘Bacilloplasma’) represents a lineage of Mollicutes (phylum Tenericutes) associated with the hindgut cuticle of terrestrial isopods (Kostanjsek et al., 2007). It was first described in P. scaber and Ligidium hypnorum (Drobne, 1995; Kostanjsek et al., 2007) and recently it was also detected in the hindgut of A. vulgare using pyrotag sequencing (Dittmer et al., 2016). Bacilloplasma are wall-less, rod-shaped extracellular bacteria that colonize the hindgut surface in P. scaber via attachment to the cuticular spines protruding into the gut lumen, suggesting a highly specific adaptation to the isopod gut environment (Kostanjsek et al., 2007). While their precise role in the terrestrial isopod hindgut remains unknown, a non-pathogenic commensal relationship seems likely due to the absence of disease symptoms in infected individuals (Kostanjsek et al., 2007). Nonetheless, a detailed investigation of isopod fitness in relation with Bacilloplasma infection is still needed. Likewise, nothing is known regarding symbiont acquisition and transmission. Interestingly, the hindgut cuticle is regularly shed during molting, raising the question how the infection is maintained or reestablished after this disturbance. A possible mechanism might be to reingest the shed cuticle, which is common in terrestrial isopods and assumed to restore minerals lost during the molt (Kostanjsek et al., 2007). Similarly, cannibalism may also allow for horizontal transmission between individuals, as Bacilloplasma has never been detected in soil or associated with plant litter or isopod feces.
The bacterium has not been cultured and its 16S rRNA gene has less than 83% sequence similarity with other Mollicutes clades (Kostanjsek et al., 2007). Candidatus Lumbricincola, a gut symbiont of earthworms, is distantly related to Bacilloplasma based on the 16S rRNA gene (Nechitaylo et al., 2009). Interestingly, isopods and earthworms occupy similar ecological niches within the soil macrofauna. Therefore, it would be highly relevant to obtain more molecular data allowing us to potentially discover past symbiont transfers between the two hosts. Moreover, several close relatives of Bacilloplasma have recently been observed in the mid- and hindguts of several marine decapod crustaceans, namely the shrimps Rimicaris exoculata (Durand et al., 2010) and Litopenaeus vannamei (Zhang et al., 2014), as well as the Chinese Mitten Crab Eriocheir sinensis (Chen et al., 2015; Zhang et al., 2016). In the latter case, symbionts closely related to both Bacilloplasma and Lumbricincola even represent the dominant taxa in the gut, but are absent from gills and the surrounding water, suggesting that these bacteria are specialized resident gut symbionts (Zhang et al., 2016). Moreover, microscopic observations of the Mitten Crab hindgut revealed highly abundant, rod-shaped bacteria attached directly to the epithelium surface (instead of the cuticular spines) using pili-like structures for adherence (Chen et al., 2015). While it is tempting to speculate that Bacilloplasma has evolved from a marine ancestor still common in aquatic crustaceans today, and has therefore maintained a long-lasting symbiotic association with terrestrial isopods, more research is clearly needed in order to better understand this relationship.
Viruses and Acanthocephalan Parasites, Models to Study Host Manipulations by Non-bacterial Symbionts
Animal-associated microbiotas are not limited to bacterial symbionts but can also include other microorganisms, such as viruses, archaea, protists or Eukaryotic parasites, which may have equally strong impacts on host fitness as the bacterial members of the microbiota. A classic example for this is the diverse microbial community in the hindgut of lower termites, consisting of bacteria, archaea and protists, all of which are involved in digestive processes (Hongoh, 2010; He et al., 2013; Peterson et al., 2015). Although our knowledge regarding non-bacterial microbes in terrestrial isopods is still very limited, two other microorganisms are known to manipulate their isopod hosts in various ways: an Invertebrate Iridescent Virus (IIV) and the acantocephalan parasite Plagiorhynchus cylindraceus.
Invertebrate Iridescent Viruses (genus: Iridovirus, family: Iridoviridae) are DNA viruses known to infect diverse invertebrates, mainly terrestrial isopods and dipteran insects, but also molluscs, annelids and nematodes (Williams, 2008). They replicate in the cytoplasm of infected cells and form large, icosahedral virions arranged into paracrystalline arrays (Lupetti et al., 2013). Their characteristic feature is the iridescent color caused by light reflection from the paracrystalline virion arrays in infected tissues. As such, iridovirus infection in terrestrial isopods is manifested by a change in body color, as infected individuals turn purple-blue (Cole and Morris, 1980; Federici, 1980a; Lupetti et al., 2013). This discoloration starts as a blue bloom on the unpigmented ventral side of the animal, which then migrates to the pigmented dorsal cuticle, before the entire body turns a completely iridescent blue (Wijnhoven and Berg, 1999; Lupetti et al., 2013). Infection is also accompanied by behavioral changes in that heavily infected individuals show a decreased response to phototactic stimuli or water contact and produce less feces, indicative of decreased food consumption (Wijnhoven and Berg, 1999; Lupetti et al., 2013). Virion aggregates have been observed in various tissues, such as epidermal, muscle and fat body cells, the hindgut, reproductive tissues, nerve cells and hemocytes (Cole and Morris, 1980; Federici, 1980a; Lupetti et al., 2013). Diseased cells are generally hypertrophied due to viral replication and the accumulation of large numbers of virions in the cytoplasm and infected specimens generally die within 30 days post-infection (Federici, 1980a; Lupetti et al., 2013).
The iridovirus was first isolated from A. vulgare, P. scaber and P. dilatatus, and was initially named Isopod Iridescent Viruses, a term that was later generalized to IIV (Cole and Morris, 1980; Federici, 1980a). Since then, iridovirus infection has been observed in 25 terrestrial isopod species from all over the world (Wijnhoven and Berg, 1999; Williams, 2008; Karasawa et al., 2012; Lupetti et al., 2013). Although the mechanism of infection in the wild is not completely understood, it has been hypothesized that infections can be acquired via wound contact, cannibalism on diseased conspecifics or corpophagy (Cole and Morris, 1980).
Apart from this particular virus, a recent study in A. vulgare indicates that the diversity of viruses in terrestrial isopods may be far greater than anticipated. Indeed, a genome-wide survey revealed no less than 54 endogenous viral elements (EVEs), representing 10 viral lineages from five viral families, integrated in the genome of A. vulgare (Theze et al., 2014). Moreover, many of these endogenized virus particles have uninterrupted Open Reading Frames, indicating the recent endogenization of viruses probably currently present in isopod populations (Theze et al., 2014).
Besides viruses, infection with Eukaryotic parasites can have far-reaching consequences for the host organism. Indeed, many parasites spend parts of their life cycle in an intermediate host that eventually needs to be consumed by a definitive host, in which the parasite can then reach maturity. Consequently, parasites have evolved elaborate strategies to manipulate the behavior of their intermediate host in that it facilitates encounters with potential definitive hosts. An example for such a host manipulation can be observed in terrestrial isopods infected with the acanthocephalan P. cylindraceus, a parasite of birds that uses terrestrial isopods as intermediate hosts (Schmidt and Olsen, 1964). During the parasite’s life cycle, eggs are excreted with the bird’s feces and ingested by terrestrial isopods. The acanthor hatches within the isopod gut, penetrates the gut epithelium and remains there in a state of dormancy for up to 25 days. Then, it migrates through the gut wall into the body cavity, where it develops into an infective cystacanth within 60–65 days post-infection. When an infected isopod is ingested by a bird, the parasite’s definitive host, P. cylindraceus attaches itself to the bird’s gut wall and develops into a mature worm (Schmidt and Olsen, 1964).
Plagiorhynchus cylindraceus has been detected in natural populations of six isopod species, namely A. vulgare, A. versicolour, Hemilepistus fedtschenkoi, H. reductus, P. scaber, and Trachelipus squamuliger (Moore, 1983; Levri and Coppola, 2004; Dimitrova, 2009). Although parasite prevalence and infection intensity are generally very low, P. cylindraceus infection has significant effects on their isopod host. First, acanthocephalan infection alters isopod behavior in that infected individuals spend more time in dry, exposed and unsheltered areas, making them easier to detect by bird predators than their uninfected conspecifics (Moore,. 1983). Second, the parasite reduces female reproductive potential, as ovaries of infected females often fail to develop normally, while the testes of infected males appear unaffected (Schmidt and Olsen, 1964; Moore, 1983; Dimitrova, 2009).
Plagiorhynchus cylindraceus infection generally triggers an immune response by the isopod host, manifested by hemocyte aggregations and melanisation of the parasite at the site of penetration into the gut epithelium (Schmidt and Olsen, 1964; Dappen and Nickol, 1981). However, resistance against P. cylindraceus is age-dependent, as more than 90% of adult A. vulgare (more than 1 year old) successfully encapsulated and eliminated the intruder in laboratory experiments, while more than 70% of isopods under 9 months of age failed to mount an immune response and became infected (Nickol and Dappen, 1982). This high level of resistance observed in adult isopods may explain why parasite prevalence is generally very low in natural populations.
The Big Picture: The Terrestrial Isopod Microbiome
Bacterial Diversity – There is More Than Meets the Eye
Apart from the bacterial endosymbionts described above, all of which are specifically adapted to the within-host or even intracellular environment, the role of more diverse microbial communities in terrestrial isopod ecology has been investigated for several decades (reviewed in Zimmer, 2006). Early molecular studies of isopod microbiota focussed exclusively on digestive tissues, due to the interest in bacteria potentially involved in nutritional processes. Despite being based only on relatively small 16S rRNA gene clone libraries, this work already hinted at a high bacterial diversity: numerous taxa from the phyla Bacteroidetes, Firmicutes, and Proteobacteria (e.g., Bacillus, Bacteroides, Enterococcus, Pseudomonas) have been observed in the hindgut of P. scaber (Kostanjsek et al., 2002, 2004a; Lapanje et al., 2010) and diverse Proteobacteria (Aeromonas, Burkholderia, Ralstonia, Rhodobacter, Vibrio) in the midgut caeca of the freshwater isopod A. aquaticus (Wang et al., 2007) and the semiterrestrial species L. pallasii and Ligia occidentalis (Eberl, 2010). A recent in-depth pyrotag screening of the bacterial community in several tissues of A. vulgare from laboratory-reared lineages as well as populations sampled in the field (Dittmer et al., 2016) provides us with the most detailed picture of the isopod microbiome to date. In total, 208 bacterial genera from 31 classes and 19 phyla were observed in this study (Supplementary Table S1), revealing a high bacterial diversity not just in the gut, but in all tested tissues, including midgut caeca, gonads, nerve cord and hemolymph (Figure 5A). 28 additional genera were detected in the feces of the same individuals but were absent from the tissues. Alphaproteobacteria, Gammaproteobacteria, and Mollicutes together represented 92% of all sequences due to highly predominant taxa (i.e., Wolbachia, Halomonas, Pseudomonas, Rickettsiella, Shewanella, and Hepatoplasma), while Firmicutes, Actinobacteria, and Bacteroidetes represented 2, 1.8, and 1% of all sequences, respectively (Figures 5B,C). However, the phylum Actinobacteria was the second most represented phylum in terms of taxonomic richness, with 54 observed genera, after the Proteobacteria with 88 genera (Supplementary Table S1).
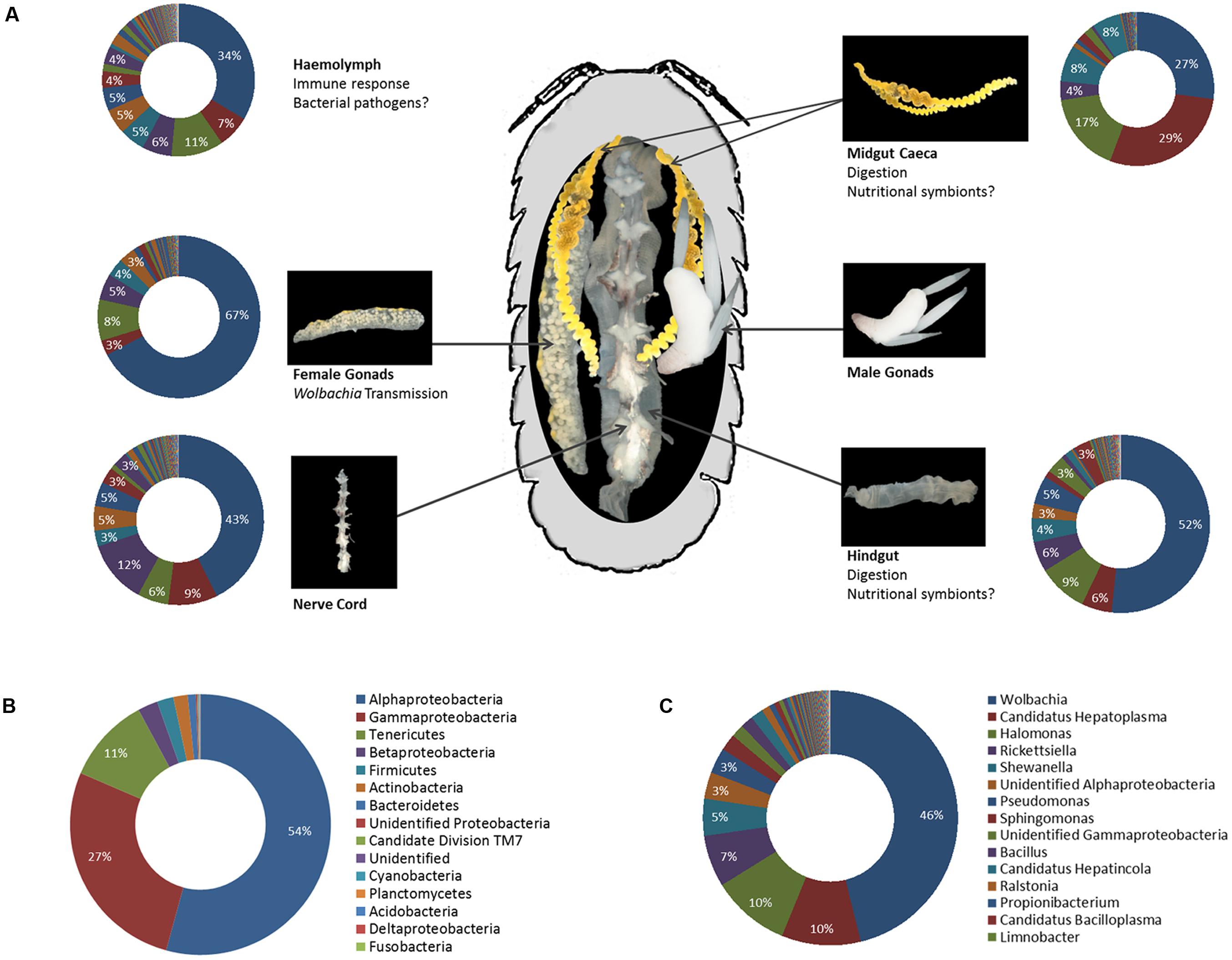
FIGURE 5. (A) Representation of the microbiota in different tissues of A. vulgare (modified from Dittmer et al., 2016). Note that data from males and females are combined, therefore the chart illustrating the microbiota in the gonads represents both male and female gonads. The legend for all charts is the same as in (C). (B,C) The microbiome of A. vulgare for all tissues combined, at the phylum (B) and genus (C) level. Proteobacteria are represented at class level. Legends show the 15 most abundant phyla/classes and genera, respectively.
Another recently published investigation of the hindgut microbiome in P. scaber using Illumina sequencing (Horvathova et al., 2016) enables us for the first time to compare the gut microbiomes of two isopod species: while the gut microbiome of P. scaber was similar to that of A. vulgare in terms of bacterial diversity at the phylum level (20 phyla), microbiome composition was quite different: Proteobacteria represented by far the most abundant phylum in both species (approximately 85%) but Alphaproteobacteria were more abundant than Gammaproteobacteria in A. vulgare, whereas the opposite was the case in P. scaber. Similarly, the Mollicutes Hepatoplasma and Bacilloplasma accounted for 8.5% of the gut microbiome in A. vulgare, while they were negligible in P. scaber (0.72%). In contrast, Bacteroidetes and Actinobacteria were more highly represented in P. scaber than in A. vulgare.
While more microbiomes from ecologically diverse species are clearly needed in order to establish potential species-specific patterns, these two studies already demonstrate that the terrestrial isopod microbiome can be highly diverse and contain 100s of taxa, even in the presence of highly predominant endosymbionts such as Wolbachia or Hepatoplasma. Investigating how these two fractions of the microbiome (i.e., highly abundant heritable endosymbionts and the rare bacterial biosphere) interact, either directly or indirectly (e.g., via regulation of the host immune response), represents an interesting path for future research.
Another intriguing aspect is the fact that all major tissues were found to harbor a high bacterial diversity in A. vulgare (Dittmer et al., 2016). While it is obvious that some bacteria still have their preferred niches (e.g., Hepatoplasma is most abundant in the caeca although it can be present in other tissues as well), it seems that isopods are not only fairly permeable to bacteria but that bacteria can also spread easily from one tissue to another. This is in line with previous findings regarding Wolbachia tissue tropisms in isopods, since (i) Wolbachia is able to cross the gut barrier and infect several other tissues after transfer via cannibalism (Le Clec’h et al., 2013a) and (ii) the cyclically produced oocytes are probably infected with Wolbachia from somatic tissues (Genty et al., 2014). Circulating hemocytes most likely serve as vehicles for Wolbachia between tissues (Braquart-Varnier et al., 2015b). If such inter-tissue transfers are possible for Wolbachia, it is conceivable that other bacteria might be able to adopt similar strategies. In this context, it is of interest that the highest species richness in A. vulgare was observed in the hemolymph (Dittmer et al., 2016). While the precise within-host trajectories of isopod-associated bacteria are far from being understood, it is likely that many of them represent commensal or opportunistic bacteria able to occupy various niches in the host, depending on available nutrients or host immunotolerance. From a technical perspective, the possibility to study host–microbiota dynamics at the tissue level due to the large body size of the host nicely illustrates one of the advantages of isopods as a model system.
Environmental Bacteria – Transient Passengers, Food or Collaborators?
A major finding from the microbiome of A. vulgare is that an important fraction of the bacterial community is most likely acquired from environmental sources, since about 70% of all taxa were also detected in feces and/or soil (Dittmer et al., 2016). Although these taxa represent rare members of the microbiome, they may constitute a source of variability between individuals and populations, depending on the microbes prevailing in the environment (e.g., soil or plant food sources). Indeed, different populations of A. vulgare harbored different bacterial communities, while the microbiomes of several genetically diverse laboratory-reared lineages were more similar to each other (Dittmer et al., 2014, 2016). The latter excludes different host genotypes as the primary driver of microbiome composition in this species and makes a shift due to environmentally acquired bacteria all the more likely. The effect of different food sources on the gut microbiome has been more formally tested in P. scaber, confirming that different food sources shape taxonomic composition of the gut microbiome at the phylum level (Horvathova et al., 2016).
The importance of environmental bacteria for terrestrial isopod health and fitness has attracted considerable attention long before the recent interest in animal microbiomes. Hence, it has been demonstrated that microbial colonization of leaf litter and artificial diets is beneficial for P. scaber, as it improves survival and growth rates of juveniles and adults as well as female reproductive success, especially when feeding on low-quality food sources (Zimmer and Topp, 1997, 2000; Kautz et al., 2002; Horvathova et al., 2015, 2016). However, it has proven challenging to elucidate the precise role of the bacteria in causing this positive effect. Several hypotheses have been put forth: (i) Environmental microbes might themselves represent an easily digestible supplementary food source, providing essential or limiting nutrients (Gunnarsson and Tunlid, 1986; Zimmer and Topp, 1998a; Ihnen and Zimmer, 2008). In line with this hypothesis, P. scaber has been shown to selectively consume and digest Actinomycetes over other bacteria and fungi, independent of their cellulolytic capacity (Ihnen and Zimmer, 2008). Moreover, recent experiments indicate that microbes acquired from leaf litter or feces may enhance juvenile growth via the provisioning of fatty acids and vitamins (Horvathova et al., 2015). (ii) Microbial enzymes contribute to digestion by preconditioning the food prior to ingestion (“external rumen”) or even through continued enzymatic activity after ingestion (Hassall and Jennings, 1975). Although a direct link between bacterial enzymes and isopod digestion has not been demonstrated to date, the isopod gut contains high amounts of cellulolytic enzymes which most animals cannot produce (Zimmer and Topp, 1998a,b). Nonetheless, there is evidence that the marine isopod Limnoria quadripunctata produces numerous endogenous glycosyl hydrolases (King et al., 2010) and two functional cellulases of host origin have been extracted from the midgut caeca in P. scaber (Kostanjsek et al., 2010). However, the presence of endogenous cellulolytic enzymes in terrestrial isopods remains to be investigated in more detail in order to determine whether they could solely account for cellulose digestion by isopods or act in synergy with microbial enzymes produced by symbiotic and/or environmental bacteria. (iii) Microbial colonization acts as an indicator of high-quality food, thereby stimulating consumption (Zimmer et al., 2003). This may be due to microbial activity enhancing palatability and nutritive quality of the leaf litter (e.g., by decreasing the C:N ratio and the content of phenolic compounds) prior to ingestion by isopods (see ii). The truth is most likely a combination of all of these and may depend to some extend on the isopod species and its feeding preferences, the nutritive quality of the food source and the different microbes involved.
Perspective: Relevance of the Microbiome at the Ecosystem Level?
Considering that researchers are now beginning to unravel the true complexity of the terrestrial isopod microbiome, a challenging objective for future research will be to better understand the functional roles of different bacteria in a wider range of isopod species as well as their interaction with the host organism, other members of the symbiotic community and the environment. Fascinating questions that need to be addressed concern the acquisition of different microbes by juveniles – possible transmission pathways include vertical transmission (either via the egg or the marsupial fluid in the brood pouch), horizontal transmission (via contact with conspecifics, coprophagy, or cannibalism) and environmental transmission (from soil or plant food sources). How plastic or variable is the microbiome throughout an individual’s lifespan – do some microbes establish more stable associations (i.e., become residents) throughout the life cycle while others remain transient? Is there a regular turn-over due to molting? Are environmental microbes just randomly acquired from the environment or is there some control or selection by the host over both microbiome composition and microbial density? Most importantly, does the plasticity of the microbiome represent an adaptive advantage? For instance, do population-specific microbiomes provide the host with microbial functions that are best adapted to the prevailing environmental conditions? These may include certain types of food sources or abiotic environmental factors. As such, it has been demonstrated that mercury pollution in the environment induced a shift in the gut microbiome of P. scaber along with an enrichment of mercury-resistant bacteria, which could potentially influence mercury tolerance of the host (Lapanje et al., 2010). Along the same lines, the microbiome may be essential in mediating the functional role of terrestrial isopods as key decomposers of organic matter and regulators of nutrient cycling in soil ecosystems. As such, isopod grazing on fungi has a stabilizing effect on microbe-mediated nutrient cycling in soils that is predicted to remain stable even under global climate change scenarios (Crowther et al., 2015). Therefore, if changes in the microbiome translate into functional differences for the host (e.g., in terms of health, nutrition, and reproduction), they might ultimately interfere with the regulatory function of isopods in terrestrial ecosystems. If this were the case, a better understanding of the complex interplay between isopods and their microbiome could help predict the stability of ecosystem functions under different ecologically relevant conditions, such as global climate change.
Concluding Remarks
This review synthesizes our current knowledge on bacterial endosymbionts as well as the microbiome as a whole in terrestrial isopods. We highlight that terrestrial isopods represent an excellent model system to study diverse symbiotic interactions along the spectrum from parasitism to mutualism. Moreover, this group of animals inhabits virtually all types of terrestrial environments from the marine littoral zone to deserts, thereby allowing the study of host–symbiont interactions across large-scale eco-evolutionary gradients. While many aspects remain to be investigated (e.g., the functional roles of particular bacteria in diverse host species, different environmental conditions or in the transition from marine to terrestrial habitats), the meta-omics era now provides us with the necessary toolkit to address such questions even in non-model organisms.
Data Accessibility
The 16S rRNA gene sequences from the microbiota of Armadillidium vulgare have been deposited in the European Nucleotide Archive under the study accession number PRJEB8160.
Author Contributions
DB, MZ, and JD contributed ideas and figures and wrote the manuscript.
Funding
This research was supported by the Centre National de la Recherche Scientifique (CNRS), the Université de Poitiers and the Région Poitou-Charentes. Research on the microbiome of Armadillidium vulgare was supported by Ph.D. grants from the CNRS and the Région Poitou-Charentes to JD. Transcriptomics of Armadillidium vulgare was supported by a grant from the Agence Nationale de la Recherche (ANR) for the project ImmunSymbArt (ANR-10-BLAN-1701) coordinated by DB.
Conflict of Interest Statement
The authors declare that the research was conducted in the absence of any commercial or financial relationships that could be construed as a potential conflict of interest.
Acknowledgments
The authors would like to thank Sören Franzenburg and Giuseppe Montesanto for pictures of terrestrial isopods as well as Maryline Raimond, Bianca Lais Zimmermann, Yongjie Wang and the ImageUP microscopy platform at the University of Poitiers for the electron microscopy images.
Supplementary Material
The Supplementary Material for this article can be found online at: http://journal.frontiersin.org/article/10.3389/fmicb.2016.01472
References
Abd, E. M., and Holdich, D. M. (1987). The occurrence of a Rickettsial disease in British woodlice (Crustacea, Isopoda, Oniscidea) populations. J. Invertebr. Pathol. 49, 252–258. doi: 10.1016/0022-2011(87)90056-5
Bian, G., Joshi, D., Dong, Y., Lu, P., Zhou, G., Pan, X., et al. (2013). Wolbachia invades Anopheles stephensi populations and induces refractoriness to Plasmodium infection. Science 340, 748–751. doi: 10.1126/science.1236192
Blagrove, M. S., Arias-Goeta, C., Failloux, A. B., and Sinkins, S. P. (2012). Wolbachia strain wMel induces cytoplasmic incompatibility and blocks dengue transmission in Aedes albopictus. Proc. Natl. Acad. Sci. U.S.A. 109, 255–260. doi: 10.1073/pnas.1112021108
Blaser, M. J. (2014). The microbiome revolution. J. Clin. Invest. 124, 4162–4165. doi: 10.1172/JCI78366
Bordenstein, S. R., O’Hara, F. P., and Werren, J. H. (2001). Wolbachia-induced incompatibility precedes other hybrid incompatibilities in Nasonia. Nature 409, 707–710. doi: 10.1038/35055543
Bouchon, D., Cordaux, R., and Grève, P. (2008). “Feminizing Wolbachia and the evolution of sex determination in isopods,” in Insect Symbiosis, eds K. Bourtzis and T. A. Miller (Boca Raton, FL: CRC Press), 273–296.
Bouchon, D., Cordaux, R., and Grève, P. (2011). “Rickettsiella, intracellular pathogens of arthropods,” in Manipulative Tenants: Bacteria Associated with Arthropods, eds E. Zchori-Fein and K. Bourtzis (Boca Raton, FL: CRC Press), 127–148.
Bouchon, D., Rigaud, T., and Juchault, P. (1998). Evidence for widespread Wolbachia infection in isopod crustaceans: molecular identification and host feminization. Proc. Biol. Sci. 265, 1081–1090. doi: 10.1098/rspb.1998.0402
Brandstädter, K., and Zimmer, M. (2008). “Infection of terrestrial isopods with environmentally transmitted bacterial symbionts,” in Proceedings of the International Symposium on Terrestrial Isopod Biology: ISTIB-07, eds M. Zimmer, F. Charfi-Cheikhrouha, and S. Taiti (Aachen: Shaker Verlag).
Braquart-Varnier, C., Altinli, M., Pigeault, R., Chevalier, F. D., Grève, P., Bouchon, D., et al. (2015a). The mutualistic side of Wolbachia-isopod interactions: Wolbachia mediated protection against pathogenic intracellular bacteria. Front. Microbiol. 6:1388. doi: 10.3389/fmicb.2015.01388
Braquart-Varnier, C., Lachat, M., Herbinière, J., Johnson, M., Caubet, Y., Bouchon, D., et al. (2008). Wolbachia mediate variation of host immunocompetence. PLoS ONE 3:e3286. doi: 10.1371/journal.pone.0003286
Braquart-Varnier, C., Raimond, M., Mappa, G., Chevalier, F. D., Le, Clec’h W, and Sicard, M. (2015b). The hematopoietic organ: a cornerstone for Wolbachia propagation between and within hosts. Front. Microbiol. 6:1424. doi: 10.3389/fmicb.2015.01424
Brucker, R. M., and Bordenstein, S. R. (2012). Speciation by symbiosis. Trends Ecol. Evol. 27, 443–451. doi: 10.1016/j.tree.2012.03.011
Brucker, R. M., and Bordenstein, S. R. (2013). The hologenomic basis of speciation: gut bacteria cause hybrid lethality in the genus Nasonia. Science 341, 667–669. doi: 10.1126/science.1240659
Cantarel, B. L., Coutinho, P. M., Rancurel, C., Bernard, T., Lombard, V., and Henrissat, B. (2009). The carbohydrate-active EnZymes database (CAZy): an expert resource for Glycogenomics. Nucleic Acids Res. 37, D233–D238. doi: 10.1093/nar/gkn663
Cerveau, N., Bouchon, D., Berges, T., and Greve, P. (2014). Molecular evolution of the androgenic hormone in terrestrial isopods. Gene 540, 71–77. doi: 10.1016/j.gene.2014.02.024
Chandler, J. A., Lang, J. M., Bhatnagar, S., Eisen, J. A., and Kopp, A. (2011). Bacterial communities of diverse Drosophila species: ecological context of a host-microbe model system. PLoS Genet. 7:e1002272. doi: 10.1371/journal.pgen.1002272
Chen, X., Di, P., Wang, H., Li, B., Pan, Y., Yan, S., et al. (2015). Bacterial community associated with the intestinal tract of Chinese mitten crab (Eriocheir sinensis) farmed in Lake Tai, China. PLoS ONE 10:e0123990. doi: 10.1371/journal.pone.0123990
Chevalier, F., Herbinière-Gaboreau, J., Bertaux, J., Raimond, M., Morel, F., Bouchon, D., et al. (2011). The immune cellular effectors of terrestrial isopod Armadillidium vulgare: meeting with their invaders, Wolbachia. PLoS ONE 6:e18531. doi: 10.1371/journal.pone.0018531
Chevalier, F., Herbinière-Gaboreau, J., Charif, D., Mitta, G., Gavory, F., Wincker, P., et al. (2012). Feminizing Wolbachia: a transcriptomics approach with insights on the immune response genes in Armadillidium vulgare. BMC Microbiol. 12(Suppl. 1):S1. doi: 10.1186/1471-2180-12-S1-S1
Chu, H., and Mazmanian, S. K. (2013). Innate immune recognition of the microbiota promotes host-microbial symbiosis. Nat. Immunol. 14, 668–675. doi: 10.1038/ni.2635
Cole, A., and Morris, T. J. (1980). A new iridovirus of two species of terrestrial isopods, Armadillidium vulgare and Porcellio scaber. Intervirology 14, 21–30. doi: 10.1159/000149158
Colman, D. R., Toolson, E. C., and Takacs-Vesbach, C. D. (2012). Do diet and taxonomy influence insect gut bacterial communities? Mol. Ecol. 21, 5124–5137. doi: 10.1111/j.1365-294X.2012.05752.x
Cordaux, R., Bouchon, D., and Greve, P. (2011). The impact of endosymbionts on the evolution of host sex-determination mechanisms. Trends Genet. 27, 332–341. doi: 10.1016/j.tig.2011.05.002
Cordaux, R., Michel-Salzat, A., and Bouchon, D. (2001). Wolbachia infection in crustaceans: novel hosts and potential routes for horizontal transmission. J. Evol. Biol. 14, 237–243. doi: 10.1046/j.1420-9101.2001.00279.x
Cordaux, R., Michel-Salzat, A., Frelon-Raimond, M., Rigaud, T., and Bouchon, D. (2004). Evidence for a new feminizing Wolbachia strain in the isopod Armadillidium vulgare: evolutionary implications. Heredity 93, 78–84. doi: 10.1038/sj.hdy.6800482
Cordaux, R., Paces-Fessy, M., Raimond, M., Michel-Salzat, A., Zimmer, M., and Bouchon, D. (2007). Molecular characterization and evolution of arthropod-pathogenic Rickettsiella bacteria. Appl. Environ. Microbiol. 73, 5045–5047. doi: 10.1128/AEM.00378-07
Cordaux, R., Pichon, S., Hatira, H. B., Doublet, V., Grève, P., Marcadé, I., et al. (2012). Widespread Wolbachia infection in terrestrial isopods and other crustaceans. Zookeys 176, 123–131. doi: 10.3897/zookeys.176.2284
Corsaro, D., Thomas, V., Goy, G., Venditti, D., Radek, R., and Greub, G. (2007). ‘Candidatus Rhabdochlamydia crassificans’, an intracellular bacterial pathogen of the cockroach Blatta orientalis (Insecta: Blattodea). Syst. Appl. Microbiol. 30, 221–228. doi: 10.1016/j.syapm.2006.06.001
Cragg, S. M., Beckham, G. T., Bruce, N. C., Bugg, T. D., Distel, D. L., Dupree, P., et al. (2015). Lignocellulose degradation mechanisms across the Tree of Life. Curr. Opin. Chem. Biol. 29, 108–119. doi: 10.1016/j.cbpa.2015.10.018
Crowther, T. W., Thomas, S. M., Maynard, D. S., Baldrian, P., Covey, K., Frey, S. D., et al. (2015). Biotic interactions mediate soil microbial feedbacks to climate change. Proc. Natl. Acad. Sci. U.S.A. 112, 7033–7038. doi: 10.1073/pnas.1502956112
Dappen, G. E., and Nickol, B. B. (1981). Unaltered hematokrit values and differential haemocyte counts in acanthocephalan-infected Armadillidum vulgare. J. Invertebr. Pathol. 38, 209–212. doi: 10.1016/0022-2011(81)90124-5
Davison, A., and Blaxter, M. (2005). Ancient origin of glycosyl hydrolase family 9 cellulase genes. Mol. Biol. Evol. 22, 1273–1284. doi: 10.1093/molbev/msi107
de Bary, A. (1879). Die Erscheinung der Symbiose [The phenomenon of symbiosis]. Vortrag gehalten auf der Versammlung der Deutschen Naturforscher und Ärzte zu Cassel. Trübner: Strassburg, 1–30.
Dimitrova, Z. M. (2009). Occurrence of cystacanths of Plagiorhynchus cylindraceus (Acanthocephala) in the terrestrial isopods Trachelipus squamuliger and Armadillidium vulgare (Oniscidea) in Bulgaria. Acta Parasitol. 54, 53–56. doi: 10.2478/s11686-009-0003-9
Dittmer, J., Beltran-Bech, S., Lesobre, J., Raimond, M., Johnson, M., and Bouchon, D. (2014). Host tissues as microhabitats for Wolbachia and quantitative insights into the bacterial community in terrestrial isopods. Mol. Ecol. 23, 2619–2635. doi: 10.1111/mec.12760
Dittmer, J., Lesobre, J., Moumen, B., and Bouchon, D. (2016). Host origin and tissue microhabitat shaping the microbiota of the terrestrial isopod Armadillidium vulgare. FEMS Microbiol. Ecol. 92: fiw063. doi: 10.1093/femsec/fiw063
Donadey, C., and Besse, G. (1972). Étude histologique ultrastructurale et expérimentale des caecums digestifs de Porcellio dilatatus et Ligia oceanica (Crustacea, Isopoda). Téthys 4, 145–162.
Dong, Y., Manfredini, F., and Dimopoulos, G. (2009). Implication of the mosquito midgut microbiota in the defense against malaria parasites. PLoS Pathog. 5:e1000423. doi: 10.1371/journal.ppat.1000423
Drobne, D. (1995). Bacteria adherent to the hindgut of terrestrial isopods. Acta Microbiol. Immunol. Hung. 42, 45–52.
Drobne, D., Strus, J., Znidarsic, N., and Zidar, P. (1999). Morphological description of bacterial infection of digestive glands in the terrestrial isopod Porcellio scaber (Isopoda, Crustacea). J. Invertebr. Pathol. 73, 113–119. doi: 10.1006/jipa.1998.4818
Durand, L., Zbinden, M., Cueff-Gauchard, V., Duperron, S., Roussel, E. G., Shillito, B., et al. (2010). Microbial diversity associated with the hydrothermal shrimp Rimicaris exoculata gut and occurrence of a resident microbial community. FEMS Microbiol. Ecol. 71, 291–303. doi: 10.1111/j.1574-6941.2009.00806.x
Duron, O., Bouchon, D., Boutin, S., Bellamy, L., Zhou, L., Engelstädter, J., et al. (2008). The diversity of reproductive parasites among arthropods: Wolbachia do not walk alone. BMC Biol. 6:27. doi: 10.1186/1741-7007-6-27
Duron, O., Cremaschi, J., and McCoy, K. D. (2016). The high diversity and global distribution of the intracellular bacterium Rickettsiella in the polar Seabird Tick Ixodes uriae. Microb. Ecol. 71, 761–770. doi: 10.1007/s00248-015-0702-8
Dutky, S. R., and Gooden, E. L. (1952). Coxiella popilliae, n. sp., a Rickettsia causing blue disease of Japanese beetle larvae. J. Bacteriol. 63, 743–750.
Eberl, R. (2010). Sea-land transitions in isopods: pattern of symbiont distribution in two species of intertidal isopods Ligia pallasii and Ligia occidentalis in the Eastern Pacific. Symbiosis 51, 107–116. doi: 10.1007/s13199-010-0057-3
Everett, K. D., Thao, M., Horn, M., Dyszynski, G. E., and Baumann, P. (2005). Novel chlamydiae in whiteflies and scale insects: endosymbionts ‘Candidatus Fritschea bemisiae’ strain Falk and ‘Candidatus Fritschea eriococci’ strain Elm. Int. J. Syst. Evol. Microbiol. 55, 1581–1587. doi: 10.1099/ijs.0.63454-0
Federici, B. A. (1980a). Isolation of an Iridovirus from two terrestrial isopods, the Pill Bug, Armadillidium vulgare, and the Sow Bug, Porcellio dilatatus. J. Invertebr. Pathol. 36, 373–381. doi: 10.1016/0022-2011(80)90041-5
Federici, B. A. (1980b). Reproduction and morphogenesis of Rickettsiella chironomi, an unusual intracellular procaryotic parasite of midge larvae. J. Bacteriol. 143, 995–1002.
Ferrari, J., Darby, A. C., Daniell, T. J., Godfray, H. C. J., and Douglas, A. E. (2004). Linking the bacterial community in pea aphids with host-plant use and natural enemy resistance. Ecol. Entomol. 29, 60–65. doi: 10.1111/j.1365-2311.2004.00574.x
Fraune, S., and Bosch, T. C. (2010). Why bacteria matter in animal development and evolution. Bioessays 32, 571–580. doi: 10.1002/bies.200900192
Fraune, S., and Zimmer, M. (2008). Host-specificity of environmentally transmitted Mycoplasma-like isopod symbionts. Environ. Microbiol. 10, 2497–2504. doi: 10.1111/j.1462-2920.2008.01672.x
Genty, L. M., Bouchon, D., Raimond, M., and Bertaux, J. (2014). Wolbachia infect ovaries in the course of their maturation: last minute passengers and priority travellers? PLoS ONE 9:e94577. doi: 10.1371/journal.pone.0094577
Grève, P., Braquart-Varnier, C., Strub, J. M., Félix, C., Van, Dorsselaer A, and Martin, G. (2004). The glycosylated androgenic hormone of the terrestrial isopod Porcellio scaber (Crustacea). Gen. Comp. Endocrinol. 136, 389–397. doi: 10.1016/j.ygcen.2004.01.015
Gunnarsson, T., and Tunlid, A. (1986). Recycling of fecal pellets in isopods: microorganisms and nitrogen compounds as potential food for Oniscus asellus L. Soil Biol. Biochem. 18, 595–600. doi: 10.1016/0038-0717(86)90081-7
Hames, C. A. C., and Hopkin, S. P. (1989). The structure and function of the digestive system of terrestrial isopods. J. Zool. Lond. 217, 599–627. doi: 10.1111/j.1469-7998.1989.tb02513.x
Hassall, M., and Jennings, J. B. (1975). Adaptive features of gut structure and digestive physiology in the terrestrial isopod Philoscia muscorum (scopoli) 1763. Biol. Bull. 149, 348–364. doi: 10.2307/1540531
He, S., Ivanova, N., Kirton, E., Allgaier, M., Bergin, C., Scheffrahn, R. H., et al. (2013). Comparative metagenomic and metatranscriptomic analysis of hindgut paunch microbiota in wood- and dung-feeding higher termites. PLoS ONE 8:e61126. doi: 10.1371/journal.pone.0061126
Hedges, L. M., Brownlie, J. C., O’Neill, S. L., and Johnson, K. N. (2008). Wolbachia and virus protection in insects. Science 322:702. doi: 10.1126/science.1162418
Hilgenboecker, K., Hammerstein, P., Schlattmann, P., Telschow, A., and Werren, J. H. (2008). How many species are infected with Wolbachia? – A statistical analysis of current data. FEMS Microbiol. Lett. 281, 215–220. doi: 10.1111/j.1574-6968.2008.01110.x
Hiroki, M., Kato, Y., Kamito, T., and Miura, K. (2002). Feminization of genetic males by a symbiotic bacterium in a butterfly, Eurema hecabe (Lepidoptera: Pieridae). Naturwissenschaften 89, 167–170.
Hongoh, Y. (2010). Diversity and genomes of uncultured microbial symbionts in the termite gut. Biosci. Biotechnol. Biochem. 74, 1145–1151. doi: 10.1271/bbb.100094
Horn, M. (2008). Chlamydiae as symbionts in eukaryotes. Annu. Rev. Microbiol. 62, 113–131. doi: 10.1146/annurev.micro.62.081307.162818
Hornung, E. (2011). Evolutionary adaptation of oniscidean isopods to terrestrial life: structure, physiology and behaviour. Terr. Arthropod Rev. 4, 95–130. doi: 10.1163/187498311X576262
Horvathova, T., Babik, W., and Bauchinger, U. (2016). Biofilm feeding: microbial colonization of food promotes the growth of a detritivorous arthropod. Zookeys 577, 25–41. doi: 10.3897/zookeys.577.6149
Horvathova, T., Kozlowski, J., and Bauchinger, U. (2015). Growth rate and survival of terrestrial isopods is related to possibility to acquire symbionts. Eur. J. Soil Biol. 69, 52–56. doi: 10.1016/j.ejsobi.2015.05.003
Huang, J., and Gogarten, J. P. (2007). Did an ancient chlamydial endosymbiosis facilitate the establishment of primary plastids? Genome Biol. 8:R99. doi: 10.1186/gb-2007-8-6-r99
Hughes, G. L., Koga, R., Xue, P., Fukatsu, T., and Rasgon, J. L. (2011). Wolbachia infections are virulent and inhibit the human malaria parasite Plasmodium falciparum in Anopheles gambiae. PLoS Pathog. 7:e1002043. doi: 10.1371/journal.ppat.1002043
Hurpin, P., and Robert, P. H. (1976). Conservation dans le sol de trois germes pathogènes pour les larves de Melolontha melolontha [Col.: Scarabaeidae]. Entomophaga 21, 73–80. doi: 10.1007/BF02372017
Hurst, G. D., and Frost, C. L. (2015). Reproductive parasitism: maternally inherited symbionts in a biparental world. Cold Spring Harb. Perspect. Biol. 7, a017699. doi: 10.1101/cshperspect.a017699
Hurst, G. D., Jiggins, F. M., and Majerus, M. E. (2003). “Inherited microorganisms that selectively kill male hosts: the hidden players of insect evolution?,” in Insect Symbiosis, eds K. Bourtzis and T. A. Miller (Boca Raton, FL: CRC Press), 177–197.
Iasur-Kruh, L., Weintraub, P. G., Mozes-Daube, N., Robinson, W. E., Perlman, S. J., and Zchori-Fein, E. (2013). Novel Rickettsiella bacterium in the leafhopper Orosius albicinctus (Hemiptera: Cicadellidae). Appl. Environ. Microbiol. 79, 4246–4252. doi: 10.1128/AEM.00721-13
Ihnen, K., and Zimmer, M. (2008). Selective consumption and digestion of litter microbes by Porcellio scaber (Isopoda: Oniscidea). Pedobiologia 51, 335–342. doi: 10.1016/j.pedobi.2007.06.001
Jaenike, J., Unckless, R., Cockburn, S. N., Boelio, L. M., and Perlman, S. J. (2010). Adaptation via symbiosis: recent spread of a Drosophila defensive symbiont. Science 329, 212–215. doi: 10.1126/science.1188235
Karasawa, S., Takatsuka, J., and Kato, J. (2012). Report on Iridovirus IIV-31 (Iridoviridae, Iridovirus) infecting terrestrial isopods (Isopods, Oniscidea) in Japan. Crustaceana 85, 1269–1278.
Kautz, G., Zimmer, M., and Topp, W. (2002). Does Porcellio scaber (Isopoda: Oniscidea) gain from coprophagy? Soil Biol. Biochem. 34, 1253–1259. doi: 10.1016/S0038-0717(02)00065-2
Kern, M., McGeehan, J. E., Streeter, S. D., Martin, R. N., Besser, K., Elias, L., et al. (2013). Structural characterization of a unique marine animal family 7 cellobiohydrolase suggests a mechanism of cellulase salt tolerance. Proc. Natl. Acad. Sci. U.S.A. 110, 10189–10194. doi: 10.1073/pnas.1301502110
Kern, P., Cook, J. M., Kageyama, D., and Riegler, M. (2015). Double trouble: combined action of meiotic drive and Wolbachia feminization in Eurema butterflies. Biol. Lett. 11, 20150095. doi: 10.1098/rsbl.2015.0095
King, A. J., Cragg, S. M., Li, Y., Dymond, J., Guille, M. J., Bowles, D. J., et al. (2010). Molecular insight into lignocellulose digestion by a marine isopod in the absence of gut microbes. Proc. Natl. Acad. Sci. U.S.A. 107, 5345–5350. doi: 10.1073/pnas.0914228107
Kleespies, R. G., Federici, B. A., and Leclerque, A. (2014). Ultrastructural characterization and multilocus sequence analysis (MLSA) of ‘Candidatus Rickettsiella isopodorum’, a new lineage of intracellular bacteria infecting woodlice (Crustacea: Isopoda). Syst. Appl. Microbiol. 37, 351–359. doi: 10.1016/j.syapm.2014.04.001
Koch, H., and Schmid-Hempel, P. (2011). Bacterial communities in central European bumblebees: low diversity and high specificity. Microb. Ecol. 62, 121–133. doi: 10.1007/s00248-011-9854-3
Koch, H., and Schmid-Hempel, P. (2012). Gut microbiota instead of host genotype drive the specificity in the interaction of a natural host-parasite system. Ecol. Lett. 15, 1095–1103. doi: 10.1111/j.1461-0248.2012.01831.x
Kostanjsek, R., Lapanje, A., Rupnik, M., Strus, J., Drobne, D., and Avgustin, G. (2004a). Anaerobic bacteria in the gut of terrestrial isopod Crustacean Porcellio scaber. Folia Microbiol. (Praha) 49, 179–182. doi: 10.1007/BF02931397
Kostanjsek, R., Milatovic, M., and Strus, J. (2010). Endogenous origin of endo-beta-1,4-glucanase in common woodlouse Porcellio scaber (Crustacea, Isopoda). J. Comp. Physiol. B 180, 1143–1153. doi: 10.1007/s00360-010-0485-7
Kostanjsek, R., Strus, J., and Avgustin, G. (2002). Genetic diversity of bacteria associated with the hindgut of the terrestrial crustacean Porcellio scaber (Crustacea: Isopoda). FEMS Microbiol. Ecol. 40, 171–179. doi: 10.1111/j.1574-6941.2002.tb00950.x
Kostanjsek, R., Strus, J., and Avgustin, G. (2007). “Candidatus Bacilloplasma”, a novel lineage of Mollicutes associated with the hindgut wall of the terrestrial isopod Porcellio scaber (Crustacea: Isopoda). Appl. Environ. Microbiol. 73, 5566–5573. doi: 10.1128/AEM.02468-06
Kostanjsek, R., Strus, J., Drobne, D., and Avgustin, G. (2004b). ‘Candidatus Rhabdochlamydia porcellionis’, an intracellular bacterium from the hepatopancreas of the terrestrial isopod Porcellio scaber (Crustacea: Isopoda). Int. J. Syst. Evol. Microbiol. 54, 543–549. doi: 10.1099/ijs.0.02802-0
Lapanje, A., Zrimec, A., Drobne, D., and Rupnik, M. (2010). Long-term Hg pollution-induced structural shifts of bacterial community in the terrestrial isopod (Porcellio scaber) gut. Environ. Pollut. 158, 3186–3193. doi: 10.1016/j.envpol.2010.07.001
Le Clec’h, W., Braquart-Varnier, C., Raimond, M., Ferdy, J. B., Bouchon, D., and Sicard, M. (2012). High virulence of Wolbachia after host switching: when autophagy hurts. PLoS Pathog. 8:e1002844. doi: 10.1371/journal.ppat.1002844
Le Clec’h, W., Chevalier, F. D., Genty, L., Bertaux, J., Bouchon, D., and Sicard, M. (2013a). Cannibalism and predation as paths for horizontal passage of Wolbachia between terrestrial isopods. PLoS ONE 8:e60232. doi: 10.1371/journal.pone.0060232
Le Clec’h, W., Raimond, M., Guillot, S., Bouchon, D., and Sicard, M. (2013b). Horizontal transfers of feminizing versus non-feminizing Wolbachia strains: from harmless passengers to pathogens. Environ. Microbiol. 15, 2922–2936. doi: 10.1111/1462-2920.12172
Leclercq, S., Dittmer, J., Bouchon, D., and Cordaux, R. (2014). Phylogenomics of “Candidatus Hepatoplasma crinochetorum,” a lineage of mollicutes associated with noninsect arthropods. Genome Biol. Evol. 6, 407–415. doi: 10.1093/gbe/evu020
Leclerque, A. (2008). Whole genome-based assessment of the taxonomic position of the arthropod pathogenic bacterium Rickettsiella grylli. FEMS Microbiol. Lett. 283, 117–127. doi: 10.1111/j.1574-6968.2008.01158.x
Leclerque, A., and Kleespies, R. G. (2012). A Rickettsiella bacterium from the hard tick, Ixodes woodi: molecular taxonomy combining multilocus sequence typing (MLST) with significance testing. PLoS ONE 7:e38062. doi: 10.1371/journal.pone.0038062
Legrand, J., Legrand-Hamelin, E., and Juchault, P. (1987). Sex determination in Crustacea. Biol. Rev. 62, 439–470. doi: 10.1111/j.1469-185X.1987.tb01637.x
Legrand, J., Martin, G., and Artault, J. C. (1978). Corrélation entre la présence d’un symbionte bactérien dans les ovocytes de Porcellio dilatatus petiti, et la stérilité du croisement P. d. petiti mâle x P. d. dilatatus femelle. Arch. Inst. Pasteur Tunis 55, 507–514.
Levri, E. P., and Coppola, B. P. (2004). First report of the acanthocephalan Plagiorhynchus cylindraceus in the terrestrial isopod Porcellio scaber. Comp. Parasitol. 71, 88–90. doi: 10.1654/4085
Ley, R. E., Hamady, M., Lozupone, C., Turnbaugh, P. J., Ramey, R. R., Bircher, J. S., et al. (2008). Evolution of mammals and their gut microbes. Science 320, 1647–1651. doi: 10.1126/science.1155725
Lombard, V., Golaconda Ramulu, H., Drula, E., Coutinho, P. M., and Henrissat, B. (2014). The carbohydrate-active enzymes database (CAZy) in 2013. Nucleic Acids Res. 42, D490–D495. doi: 10.1093/nar/gkt1178
Lukasik, P., van Asch, M., Guo, H., Ferrari, J., and Godfray, H. C. (2013). Unrelated facultative endosymbionts protect aphids against a fungal pathogen. Ecol. Lett. 16, 214–218. doi: 10.1111/ele.12031
Lupetti, P., Montesanto, G., Ciolfi, S., Marri, L., Gentile, M., Paccagnini, E., et al. (2013). Iridovirus infection in terrestrial isopods from Sicily (Italy). Tissue Cell 45, 321–327. doi: 10.1016/j.tice.2013.05.001
Martin, G., Juchault, P., and Legrand, J. J. (1973). Mise en évidence d’un micro-organisme intracytoplasmique symbiote de l’oniscoïde Armadillidium vulgare Latr. dont la présence accompagne l’intersexualité ou la féminisation totale des mâles génétiques de la lignée thélygène. C. R. Acad. Sci. Paris 276, 2313–2316.
Martinson, V. G., Danforth, B. N., Minckley, R. L., Rueppell, O., Tingek, S., and Moran, N. A. (2011). A simple and distinctive microbiota associated with honey bees and bumble bees. Mol. Ecol. 20, 619–628. doi: 10.1111/j.1365-294X.2010.04959.x
Mattila, J. M., Zimmer, M., Vesakoski, O., and Jormalainen, V. (2014). Habitat-specific gut microbiota of the marine herbivore Idotea balthica (Isopoda). J. Exp. Mar. Bio. Ecol. 455, 22–28. doi: 10.1016/j.jembe.2014.02.010
McCutcheon, J. P., McDonald, B. R., and Moran, N. A. (2009). Convergent evolution of metabolic roles in bacterial co-symbionts of insects. Proc. Natl. Acad. Sci. U.S.A. 106, 15394–15399. doi: 10.1073/pnas.0906424106
McCutcheon, J. P., and Moran, N. A. (2010). Functional convergence in reduced genomes of bacterial symbionts spanning 200 My of evolution. Genome Biol. Evol. 2, 708–718. doi: 10.1093/gbe/evq055
McFall-Ngai, M., Hadfield, M. G., Bosch, T. C., Carey, H. V., Domazet-Lošo, T., Douglas, A. E., et al. (2013). Animals in a bacterial world, a new imperative for the life sciences. Proc. Natl. Acad. Sci. U.S.A. 110, 3229–3236. doi: 10.1073/pnas.1218525110
Mediannikov, O., Sekeyova, Z., Birg, M. L., and Raoult, D. (2010). A novel obligate intracellular gamma-proteobacterium associated with ixodid ticks, Diplorickettsia massiliensis, Gen. Nov., Sp. Nov. PLoS ONE 5:e11478. doi: 10.1371/journal.pone.0011478
Montllor, C. B., Maxmen, A., and Purcell, A. H. (2002). Facultative bacterial endosymbionts benefit pea aphids Acyrthosiphon pisum under heat stress. Ecol. Entomol. 27, 189–195. doi: 10.1046/j.1365-2311.2002.00393.x
Moore, J. (1983). Responses of an avian predator and its isopod prey to an acanthocephalan parasite. Ecology 64, 1000–1015. doi: 10.2307/1937807
Moran, N. A., Dunbar, H. E., and Wilcox, J. L. (2005). Regulation of transcription in a reduced bacterial genome: nutrient-provisioning genes of the obligate symbiont Buchnera aphidicola. J. Bacteriol. 187, 4229–4237. doi: 10.1128/JB.187.12.4229-4237.2005
Moreira, L. A., Iturbe-Ormaetxe, I., Jeffery, J. A., Lu, G., Pyke, A. T., Hedges, L. M., et al. (2009). A Wolbachia symbiont in Aedes aegypti limits infection with dengue, Chikungunya, and Plasmodium. Cell 139, 1268–1278. doi: 10.1016/j.cell.2009.11.042
Moret, Y., Juchault, P., and Rigaud, T. (2001). Wolbachia endosymbiont responsible for cytoplasmic incompatibility in a terrestrial crustacean: effects in natural and foreign hosts. Heredity 86, 325–332. doi: 10.1046/j.1365-2540.2001.00831.x
Narita, S., Kageyama, D., Nomura, M., and Fukatsu, T. (2007). Unexpected mechanism of symbiont-induced reversal of insect sex: feminizing Wolbachia continuously acts on the butterfly Eurema hecabe during larval development. Appl. Environ. Microbiol. 73, 4332–4341. doi: 10.1128/AEM.00145-07
Nechitaylo, T. Y., Timmis, K. N., and Golyshin, P. N. (2009). ‘Candidatus Lumbricincola’, a novel lineage of uncultured Mollicutes from earthworms of family Lumbricidae. Environ. Microbiol. 11, 1016–1026. doi: 10.1111/j.1462-2920.2008.01837.x
Negri, I., Franchini, A., Gonella, E., Daffonchio, D., Mazzoglio, P. J., Mandrioli, M., et al. (2009). Unravelling the Wolbachia evolutionary role: the reprogramming of the host genomic imprinting. Proc. Biol. Sci. 276, 2485–2491. doi: 10.1098/rspb.2009.0324
Negri, I., Pellecchia, M., Mazzoglio, P. J., Patetta, A., and Alma, A. (2006). Feminizing Wolbachia in Zyginidia pullula (Insecta, Hemiptera), a leafhopper with an XX/X0 sex-determination system. Proc. Biol. Sci. 273, 2409–2416.
Nickol, B. B., and Dappen, G. E. (1982). Armadillidium vulgare (Isopoda) as an intermediate host of Plagiorhynchus cylindraceus (Acanthocephala) and isopod response to infection. J. Parasitol. 68, 570–575. doi: 10.2307/3280912
Oliver, K. M., Moran, N. A., and Hunter, M. S. (2005). Variation in resistance to parasitism in aphids is due to symbionts not host genotype. Proc. Natl. Acad. Sci. U.S.A. 102, 12795–12800. doi: 10.1073/pnas.0506131102
Oliver, K. M., Russell, J. A., Moran, N. A., and Hunter, M. S. (2003). Facultative bacterial symbionts in aphids confer resistance to parasitic wasps. Proc. Natl. Acad. Sci. U.S.A. 100, 1803–1807. doi: 10.1073/pnas.0335320100
Osborne, S. E., Leong, Y. S., O’Neill, S. L., and Johnson, K. N. (2009). Variation in antiviral protection mediated by different Wolbachia strains in Drosophila simulans. PLoS Pathog. 5:e1000656. doi: 10.1371/journal.ppat.1000656
Pais, R., Lohs, C., Wu, Y., Wang, J., and Aksoy, S. (2008). The obligate mutualist Wigglesworthia glossinidia influences reproduction, digestion, and immunity processes of its host, the tsetse fly. Appl. Environ. Microbiol. 74, 5965–5974. doi: 10.1128/AEM.00741-08
Peterson, B. F., Stewart, H. L., and Scharf, M. E. (2015). Quantification of symbiotic contributions to lower termite lignocellulose digestion using antimicrobial treatments. Insect Biochem. Mol. Biol. 59, 80–88. doi: 10.1016/j.ibmb.2015.02.009
Pigeault, R., Braquart-Varnier, C., Marcadé, I., Mappa, G., Mottin, E., and Sicard, M. (2014). Modulation of host immunity and reproduction by horizontally acquired Wolbachia. J. Insect Physiol. 70, 125–133. doi: 10.1016/j.jinsphys.2014.07.005
Plante, C. J., Jumars, P. A., and Baross, J. A. (1990). Digestive associations between marine invertebrates and bacteria. Ann. Rev. Ecol. Syst. 21, 93–127. doi: 10.1146/annurev.es.21.110190.000521
Ramírez-Puebla, S. T., Servín-Garcidueñas, L. E., Ormeño-Orrillo, E., Vera-Ponce de León, A., Rosenblueth, M., Delaye, L., et al. (2015). Species in Wolbachia? Proposal for the designation of ‘Candidatus Wolbachia bourtzisii’, ‘Candidatus Wolbachia onchocercicola’, ‘Candidatus Wolbachia blaxteri’, ‘Candidatus Wolbachia brugii’, ‘Candidatus Wolbachia taylori’, ‘Candidatus Wolbachia collembolicola’, and ‘Candidatus Wolbachia multihospitum’ for the different species within Wolbachia supergroups. Syst. Appl. Microbiol. 38, 390–399. doi: 10.1016/j.syapm.2015.05.005
Rigaud, T., and Juchault, P. (1995). Success and failure of horizontal transfers of feminizing Wolbachia endosymbionts in woodlice. J. Evol. Biol. 8, 249–255. doi: 10.1046/j.1420-9101.1995.8020249.x
Rigaud, T., Juchault, P., and Mocquard, J. P. (1997). The evolution of sex determination in isopod crustaceans. Bioessays 19, 409–416. doi: 10.1002/bies.950190508
Rigaud, T., Souty-Grosset, C., Raimond, R., Mocquard, J. P., and Juchault, P. (1991). Feminizing endocytobiosis in the terrestrial crustacean Armadillidium vulgare Latr. (Isopoda): recent acquisitions. Endocyt. Cell Res. 7, 259–273.
Rousset, F., Bouchon, D., Pintureau, B., Juchault, P., and Solignac, M. (1992). Wolbachia endosymbionts responsible for various alterations of sexuality in arthropods. Proc. Biol. Sci. 250, 91–98. doi: 10.1098/rspb.1992.0135
Roux, V., Bergoin, M., Lamaze, N., and Raoult, D. (1997). Reassessment of the taxonomic position of Rickettsiella grylli. Int. J. Syst. Bacteriol. 47, 1255–1257. doi: 10.1099/00207713-47-4-1255
Scarborough, C. L., Ferrari, J., and Godfray, H. C. (2005). Aphid protected from pathogen by endosymbiont. Science 310:1781. doi: 10.1126/science.1120180
Schmalfuss, H. (2003). World catalog of terrestrial isopods (Isopoda: Oniscidea). Stuttg. Beitr. Nat. A Biol. 638:341.
Schmidt, G. D., and Olsen, O. W. (1964). Life cycle and development of Prosthorhynchus Formosus (Van Cleave, 1918) Travassos, 1926, an acanthocephalan parasite of birds. J. Parasitol. 50, 721–730. doi: 10.2307/3276191
Serbus, L. R., Casper-Lindley, C., Landmann, F., and Sullivan, W. (2008). The genetics and cell biology of Wolbachia-host interactions. Annu. Rev. Genet. 42, 683–707. doi: 10.1146/annurev.genet.41.110306.130354
Shay, M. T., Bettica, A., Vernon, G. M., and Witkus, E. R. (1985). Chlamydia isopodii sp. n., an obligate intracellular parasite of Porcellio scaber. Exp. Cell Biol. 53, 115–120.
Shin, S. C., Kim, S. H., You, H., Kim, B., Kim, A. C., Lee, K. A., et al. (2011). Drosophila microbiome modulates host developmental and metabolic homeostasis via insulin signaling. Science 334, 670–674. doi: 10.1126/science.1212782
Sicard, M., Bouchon, D., Ceyrac, L., Raimond, R., Thierry, M., Le, Clec’h W, et al. (2014a). Bidirectional cytoplasmic incompatibility caused by Wolbachia in the terrestrial isopod Porcellio dilatatus. J. Invertebr. Pathol. 121, 28–36. doi: 10.1016/j.jip.2014.06.007
Sicard, M., Chevalier, F., De Vlechouver, M., Bouchon, D., Grève, P., and Braquart-Varnier, C. (2010). Variations of immune parameters in terrestrial isopods: a matter of gender, aging and Wolbachia. Naturwissenschaften 97, 819–826. doi: 10.1007/s00114-010-0699-2
Sicard, M., Dittmer, J., Greve, P., Bouchon, D., and Braquart-Varnier, C. (2014b). A host as an ecosystem: Wolbachia coping with environmental constraints. Environ. Microbiol. 16, 3583–3607. doi: 10.1111/1462-2920.12573
Sixt, B. S., Kostanjsek, R., Mustedanagic, A., Toenshoff, E. R., and Horn, M. (2013). Developmental cycle and host interaction of Rhabdochlamydia porcellionis, an intracellular parasite of terrestrial isopods. Environ. Microbiol. 15, 2980–2993. doi: 10.1111/1462-2920.12252
Stouthamer, R., Breeuwert, J. A., Luck, R. F., and Werren, J. H. (1993). Molecular identification of microorganisms associated with parthenogenesis. Nature 361, 66–68. doi: 10.1038/361066a0
Subramanian, G., Mediannikov, O., Angelakis, E., Socolovschi, C., Kaplanski, G., Martzolff, L., et al. (2012). Diplorickettsia massiliensis as a human pathogen. Eur. J. Clin. Microbiol. Infect. Dis. 31, 365–369. doi: 10.1007/s10096-011-1318-7
Sugimoto, T. N., and Ishikawa, Y. (2012). A male-killing Wolbachia carries a feminizing factor and is associated with degradation of the sex-determining system of its host. Biol. Lett. 8, 412–415. doi: 10.1098/rsbl.2011.1114
Teixeira, L., Ferreira, A., and Ashburner, M. (2008). The bacterial symbiont Wolbachia induces resistance to RNA viral infections in Drosophila melanogaster. PLoS Biol. 6:e2. doi: 10.1371/journal.pbio.1000002
Thao, M. L., Baumann, L., Hess, J. M., Falk, B. W., Ng, J. C., Gullan, P. J., et al. (2003). Phylogenetic evidence for two new insect-associated Chlamydia of the family Simkaniaceae. Curr. Microbiol. 47, 46–50. doi: 10.1007/s00284-002-3953-9
Thao, M. L., and Baumann, P. (2004). Evolutionary relationships of primary prokaryotic endosymbionts of whiteflies and their hosts. Appl. Environ. Microbiol. 70, 3401–3406. doi: 10.1128/AEM.70.6.3401-3406.2004
Theze, J., Leclercq, S., Moumen, B., Cordaux, R., and Gilbert, C. (2014). Remarkable diversity of endogenous viruses in a crustacean genome. Genome Biol. Evol. 6, 2129–2140. doi: 10.1093/gbe/evu163
Tram, U., and Sullivan, W. (2002). Role of delayed nuclear envelope breakdown and mitosis in Wolbachia-induced cytoplasmic incompatibility. Science 296, 1124–1126. doi: 10.1126/science.1070536
Tsuchida, T., Koga, R., Fujiwara, A., and Fukatsu, T. (2014). Phenotypic effect of “Candidatus Rickettsiella viridis,” a facultative symbiont of the pea aphid (Acyrthosiphon pisum), and its interaction with a coexisting symbiont. Appl. Environ. Microbiol. 80, 525–533. doi: 10.1128/AEM.03049-13
Tsuchida, T., Koga, R., and Fukatsu, T. (2004). Host plant specialization governed by facultative symbiont. Science 303:1989. doi: 10.1126/science.1094611
Tsuchida, T., Koga, R., Horikawa, M., Tsunoda, T., Maoka, T., Matsumoto, S., et al. (2010). Symbiotic bacterium modifies aphid body colour. Science 330, 1102–1104. doi: 10.1126/science.1195463
Vago, C., Meynadier, G., Juchault, P., Legrand, J. J., Amargier, A., and Duthoit, J. L. (1970). A rickettsial disease of isopod crustaceans. C. R. Acad. Sci. Hebd. Seances Acad Sci. D 271, 2061–2063.
Verne, S., Johnson, M., Bouchon, D., and Grandjean, F. (2007). Evidence for recombination between feminizing Wolbachia in the isopod genus Armadillidium. Gene 397, 58–66. doi: 10.1016/j.gene.2007.04.006
Wang, Y., Brune, A., and Zimmer, M. (2007). Bacterial symbionts in the hepatopancreas of isopods: diversity and environmental transmission. FEMS Microbiol. Ecol. 61, 141–152. doi: 10.1111/j.1574-6941.2007.00329.x
Wang, Y., Stingl, U., Anton-Erxleben, F., Geisler, S., Brune, A., and Zimmer, M. (2004a). “Candidatus Hepatoplasma crinochetorum,” a new, stalk-forming lineage of Mollicutes colonizing the midgut glands of a terrestrial isopod. Appl. Environ. Microbiol. 70, 6166–6172. doi: 10.1128/AEM.70.10.6166-6172.2004
Wang, Y., Stingl, U., Anton-Erxleben, F., Zimmer, M., and Brune, A. (2004b). ‘Candidatus Hepatincola porcellionum’ gen. nov., sp. nov., a new, stalk-forming lineage of Rickettsiales colonizing the midgut glands of a terrestrial isopod. Arch. Microbiol. 181, 299–304. doi: 10.1007/s00203-004-0655-7
Watanabe, H., Noda, H., Tokuda, G., and Lo, N. (1998). A cellulase gene of termite origin. Nature 394, 330–331. doi: 10.1038/28527
Weiss, E., Dasch, G. A., and Chang, K.-P. (1984). “Genus VIII. Rickettsiella Philip 1956, 267AL,” in Bergey’s Manual of Systematic Bacteriology, eds N. R. Krieg and J. G. Holt (Baltimore, MD: Williams and Wilkins), 713–717.
Werren, J. H., Baldo, L., and Clark, M. E. (2008). Wolbachia: master manipulators of invertebrate biology. Nat. Rev. Microbiol. 6, 741–751. doi: 10.1038/nrmicro1969
Wijnhoven, H., and Berg, M. P. (1999). Some notes on the distribution and ecology of Iridovirus (Iridovirus, Iridoviridae) in Terrestrial Isopods (Isopoda, Oniscidae). Crustaceana 72, 145–156.
Williams, T. (2008). Natural invertebrate hosts of iridoviruses (Iridoviridae). Neotrop. Entomol. 37, 615–632. doi: 10.1590/S1519-566X2008000600001
Wong, A. C., Dobson, A. J., and Douglas, A. E. (2014). Gut microbiota dictates the metabolic response of Drosophila to diet. J. Exp. Biol. 217, 1894–1901. doi: 10.1242/jeb.101725
Wong, C. N., Ng, P., and Douglas, A. E. (2011). Low-diversity bacterial community in the gut of the fruitfly Drosophila melanogaster. Environ. Microbiol. 13, 1889–1900. doi: 10.1111/j.1462-2920.2011.02511.x
Wood, S., and Griffiths, B. S. (1988). Bacteria associated with the hepatopancreas of the woodlice Oniscus asellus and Porcellio scaber (Crustacea, Isopoda). Pedobiologia 31, 89–94.
Yousfi, A. (1976). Recherches sur la Pathologie des Crustacés Isopodes Oniscoïdes. Ph.D. thesis, University of Montpellier, Montpellier.
Zhang, M., Sun, Y., Chen, K., Yu, N., Zhou, Z., Chen, L., et al. (2014). Characterization of the intestinal microbiota in pacific white shrimp, Litopenaeus vannamei, fed diets with different lipid sources. Aquaculture 434, 449–455. doi: 10.1016/j.aquaculture.2014.09.008
Zhang, M., Sun, Y., Chen, L., Cai, C., Qiao, F., Du, Z., et al. (2016). Symbiotic bacteria in gills and guts of Chinese mitten crab (Eriocheir sinensis) differ from the free-living bacteria in water. PLoS ONE 11:e0148135. doi: 10.1371/journal.pone.0148135
Zimmer, M. (1999). The fate and effects of ingested hydrolysable tannins in Porcellio scaber. J. Chem. Ecol. 25, 611–628. doi: 10.1023/A:1020962105931
Zimmer, M. (2002). Nutrition in terrestrial isopods (Isopoda: Oniscidea): an evolutionary-ecological approach. Biol. Rev. Camb. Philos. Soc. 77, 455–493. doi: 10.1017/S1464793102005912
Zimmer, M. (2006). The role of animal-microbe interactions in isopod ecology and evolution. Acta Biol. Benr. 13, 127–168.
Zimmer, M., and Bartholmé, S. (2003). Bacterial endosymbionts in Asellus aquaticus (Isopoda) and Gammarus pulex (Amphipoda) and their contribution to digestion. Limnol. Oceanogr. 48, 2208–2213. doi: 10.4319/lo.2003.48.6.2208
Zimmer, M., Danko, J. P., Pennings, S. C., Danford, A. R., Carefoot, T. H., Ziegler, A., et al. (2002). Cellulose digestion and phenol oxidation in coastal isopods (Crustacea: Isopoda). Mar. Biol. 140, 1207–1213. doi: 10.1007/s00227-002-0800-2
Zimmer, M., Danko, J. P., Pennings, S. C., Danford, A. R., Ziegler, A., and Uglow, R. F. (2001). Hepatopancreatic endosymbionts in coastal isopods (Crustacea: Isopoda), and their contribution to digestion. Mar. Biol. 138, 955–963. doi: 10.1007/s002270000519
Zimmer, M., Kautz, G., and Topp, W. (2003). Leaf litter-colonizing microbiota: supplementary food source or indicator of food quality for Porcellio scaber (Isopoda: Oniscidea)? Eur. J. Soil Biol. 39, 209–216. doi: 10.1016/j.ejsobi.2003.07.001
Zimmer, M., and Topp, W. (1997). Does leaf litter quality influence population parameters of the common woodlouse, Porcellio scaber (Crustacea: Isopoda)? Biol. Fertil. Soils 24, 435–441.
Zimmer, M., and Topp, W. (1998a). Microorganisms and cellulose digestion in the gut of the woodlouse Porcellio scaber. J. Chem. Ecol. 24, 1397–1408. doi: 10.1023/A:1021235001949
Zimmer, M., and Topp, W. (1998b). Nutritional biology of terrestrial isopods (Isopoda: Oniscidea): copper revisited. Israel J. Zool. 44, 453–462.
Zimmer, M., and Topp, W. (1999). Relationships between woodlice (Isopoda: Oniscidea) and microbial density and activity in the field. Biol. Fertil. Soils 30, 117–123. doi: 10.1007/s003740050597
Zimmer, M., and Topp, W. (2000). Species-specific utilization of food sources by sympatric woodlice (Isopoda: Oniscidea). J. Anim. Ecol. 69, 1071–1082. doi: 10.1111/j.1365-2656.2000.00463.x
Keywords: bacterial diversity, endosymbionts, microbiota, symbiosis, ecological interactions, terrestrial isopods
Citation: Bouchon D, Zimmer M and Dittmer J (2016) The Terrestrial Isopod Microbiome: An All-in-One Toolbox for Animal–Microbe Interactions of Ecological Relevance. Front. Microbiol. 7:1472. doi: 10.3389/fmicb.2016.01472
Received: 26 June 2016; Accepted: 05 September 2016;
Published: 23 September 2016.
Edited by:
Ute Hentschel, GEOMAR – Helmholtz Centre for Ocean Research Kiel, GermanyReviewed by:
Pepijn Wilhelmus Kooij, Royal Botanic Gardens, UKYuval Gottlieb, Hebrew University of Jerusalem, Israel
Yongjie Wang Wang, Shanghai Ocean University, China
Copyright © 2016 Bouchon, Zimmer and Dittmer. This is an open-access article distributed under the terms of the Creative Commons Attribution License (CC BY). The use, distribution or reproduction in other forums is permitted, provided the original author(s) or licensor are credited and that the original publication in this journal is cited, in accordance with accepted academic practice. No use, distribution or reproduction is permitted which does not comply with these terms.
*Correspondence: Didier Bouchon, ZGlkaWVyLmJvdWNob25AdW5pdi1wb2l0aWVycy5mcg==