- 1Department of Entomology, The Hebrew University of Jerusalem, Rehovot, Israel
- 2Institute of Plant Sciences, Newe-Ya'ar Research Center, Agricultural Research Organization, Ramat-Yishai, Israel
- 3Department of Entomology, Newe-Ya'ar Research Center, Agricultural Research Organization, Volcani Center, Ramat-Yishai, Israel
- 4Institute for Integrative Systems Biology, Universitat de València-CSIC, València, Spain
- 5Unidad Mixta de Investigación en Genómica y Salud, Fundación para el Fomento de la Investigación Sanitaria y Biomédica de la Comunidad Valenciana (FISABIO) and Institute for Integrative Systems Biology, Universitat de València, València, Spain
Insect lineages feeding on nutritionally restricted diets such as phloem sap, xylem sap, or blood, were able to diversify by acquiring bacterial species that complement lacking nutrients. These bacteria, considered obligate/primary endosymbionts, share a long evolutionary history with their hosts. In some cases, however, these endosymbionts are not able to fulfill all of their host's nutritional requirements, driving the acquisition of additional symbiotic species. Phloem-feeding members of the insect family Aleyrodidae (whiteflies) established an obligate relationship with Candidatus Portiera aleyrodidarum, which provides its hots with essential amino acids and carotenoids. In addition, many whitefly species harbor additional endosymbionts which may potentially further supplement their host's diet. To test this hypothesis, genomes of several endosymbionts of the whiteflies Aleurodicus dispersus, Aleurodicus floccissimus and Trialeurodes vaporariorum were analyzed. In addition to Portiera, all three species were found to harbor one Arsenophonus and one Wolbachia endosymbiont. A comparative analysis of Arsenophonus genomes revealed that although all three are capable of synthesizing B vitamins and cofactors, such as pyridoxal, riboflavin, or folate, their genomes and phylogenetic relationship vary greatly. Arsenophonus of A. floccissimus and T. vaporariorum belong to the same clade, and display characteristics of facultative endosymbionts, such as large genomes (3 Mb) with thousands of genes and pseudogenes, intermediate GC content, and mobile genetic elements. In contrast, Arsenophonus of A. dispersus belongs to a different lineage and displays the characteristics of a primary endosymbiont—a reduced genome (670 kb) with ~400 genes, 32% GC content, and no mobile genetic elements. However, the presence of 274 pseudogenes suggests that this symbiotic association is more recent than other reported primary endosymbionts of hemipterans. The gene repertoire of Arsenophonus of A. dispersus is completely integrated in the symbiotic consortia, and the biosynthesis of most vitamins occurs in shared pathways with its host. In addition, Wolbachia endosymbionts have also retained the ability to produce riboflavin, flavin adenine dinucleotide, and folate, and may make a nutritional contribution. Taken together, our results show that Arsenophonus hold a pivotal place in whitefly nutrition by their ability to produce B vitamins.
1. Introduction
Mutualistic, commensal, or parasitic relationships have been described in a diverse array of eukaryotes that are allied with bacterial symbionts (Moya et al., 2008). The class Insecta provides examples of mutualistic associations, and several lineages are known to have lived in intimate relationships with obligate symbionts for millions of years. Such symbionts are usually harbored inside specialized cells, termed bacteriocytes (sometimes organized as a bacteriome), and are vertically transmitted from the mother to her offspring. These bacterial symbionts, living inside cells and transmitted throughout long evolutionary periods, have been denoted primary (or obligatory) endosymbionts. The evolution of these symbioses has had several effects on the gene repertoires of both hosts and endosymbionts, but is most often characterized by drastic reductions in the genome contents of the latter (Latorre and Manzano-Marín, 2016). Host diet complementation frequently initiates the symbiosis, but in some cases, the host has nutritional requirements which the primary endosymbiont is unable to satisfy, and secondary (or facultative) endosymbionts are required for further diet complementation. When these requirements become vital, a secondary endosymbiont might become primary, resulting in a consortium of primary endosymbionts (Lamelas et al., 2011).
Aphids (Aphidoidea), scale insects (Coccoidea), whiteflies (Aleyrodoidea), and psyllids (Psylloidea) are superfamilies composing the suborder Sternorrhyncha, included in the order Hemiptera. All Sternorrhyncha feed on plant phloem sap, a diet poor in nitrogenous compounds, especially essential amino acids, but rich in sugars (Douglas, 2006). Among them, whiteflies and psyllids are two sister lineages whose common ancestor established a long-term association with a bacterium of the family Halomonadaceae (Gammaproteobacteria:Oceanospirillales) (Santos-Garcia et al., 2014a). This bacterial lineage has co-diversified with its hosts and is known as Candidatus Portiera aleyrodidarum in whiteflies and Candidatus Carsonella ruddii in psyllids (hereafter, the term Candidatus is used only the first time a species is mentioned). These two endosymbionts have extremely reduced genomes, ranging from 160 to 174 kb and 182–207 genes in Carsonella, and 280–357 kb and 284–318 genes in Portiera (Sloan and Moran, 2012b; Santos-Garcia et al., 2015). Based on gene repertoires of both Carsonella and its host, the two partners can complement each other to produce all essential amino acids, with the exception of tryptophan and histidine (Sloan et al., 2014). Indeed, tryptophan complementation by Carsonella and a secondary endosymbiont has been only reported in a few psyllid species (Sloan and Moran, 2012b). On the other hand, it was proposed that Portiera collaborates with its hosts in the synthesis of all essential amino acids, and is able to produce carotenoids and lipoate independently (Santos-Garcia et al., 2012, 2015; Sloan and Moran, 2012a; Luan et al., 2015). Neither Carsonella and nor Portiera can produce other vitamins or cofactors, which are deficient in the phloem sieve-element diet of whiteflies and psyllids (Zimmermann and Milburn, 1975). Unlike the generally limited bacterial associates of psyllids, individual whiteflies may carry one or several additional endosymbionts belonging to seven genera: the gammaproteobacteria Hamiltonella (Enterobacterales:Enterobacteriaceae) and Arsenophonus (Enterobacterales:Morganellaceae); the alphaproteobacteria Wolbachia (Rickettsiales:Anaplasmataceae), Rickettsia (Rickettsiales:Rickettsiaceae), and Hemipteriphilus (Rickettsiales:Rickettsiaceae); the bacteroidetes Cardinium (Cytophagales:Amoebophilaceae); and the chlamydiae Fritschea (Parachlamydiales: Simkaniaceae) (Thao et al., 2003; Bing et al., 2013b; Skaljac et al., 2013; Marubayashi et al., 2014; Zchori-Fein et al., 2014). It has been suggested that some of these additional endosymbionts are involved in supplying vitamins and cofactors to their host. For example, Candidatus Hamiltonella defensa from the whitefly Bemisia tabaci potentially produces several B vitamins (riboflavin, pyridoxine, biotin and folic acid) (Rao et al., 2015). Hamiltonella is fixed in the MEAM1 and MED-Q1 species of the B. tabaci complex and is always found sharing bacteriocytes with Portiera (Gottlieb et al., 2008; Zchori-Fein et al., 2014). Interestingly, Arsenophonus is a genus of secondary endosymbiont that has been observed in many whitefly species (Thao and Baumann, 2004; Cass et al., 2014; Marubayashi et al., 2014; Zchori-Fein et al., 2014; Pandey and Rajagopal, 2015; Santos-Garcia et al., 2015). Similar to Hamiltonella, Arsenophonus is restricted to the bacteriocytes and seems to be fixed in the whitefly species/population. In contrast, other endosymbionts such as Candidatus Wolbachia or Candidatus Rickettsia species present different tissue tropisms and are generally not fixed (Gottlieb et al., 2008; Skaljac et al., 2010, 2013; Kapantaidaki et al., 2014; Marubayashi et al., 2014; Zchori-Fein et al., 2014). Despite some potential complementations with Portiera for essential amino acid production detected in Rickettsia, the production of threonine in Wolbachia, or a few intermediate metabolites and cofactors in both endosymbionts, Wolbachia and Rickettsia from B. tabaci have limited metabolic potential and seem to import more resources from the host than they can share with it (Opatovsky et al., 2018). Similarly, Cardinium from B. tabaci demonstrates scarce metabolic potential and seems not to be involved in host diet complementation (Santos-Garcia et al., 2014b). On the other hand, some Arsenophonus and Wolbachia lineages have evolved intimate associations with other insect taxa with different diets, such as blood-sucking insects, where the supplementation of B vitamins and cofactors are their proposed role (Hosokawa et al., 2010; Šochová et al., 2017).
The sole whitefly family, Aleyrodidae, is comprised of two main subfamilies – the Aleurodicinae and the Aleyrodinae. These two subfamilies probably originated between the Jurassic (Shcherbakov, 2000; Drohojowska and Szwedo, 2015) and Middle Cretaceous (Campbell et al., 1994). Whereas the Aleyrodinae contains the largest number of species described to date (140 genera, 1440 species), the Aleurodicinae has a relatively low number of described species (17 genera, 120 species) (Ouvrard and Martin, 2018). Analysis of the genome content of Portiera from two Aleurodicinae species, Aleurodicus dispersus and Aleurodicus floccissimus, and two Aleyrodinae species, Trialeurodes vaporariorum and B. tabaci, suggested that they supply their hosts with essential amino acids and carotenoids but are unable to complement their hosts' diet with essential vitamins and cofactors (Santos-Garcia et al., 2015). Because Hamiltonella potentially supplies B. tabaci with vitamins and cofactors (Rao et al., 2015), the work presented here was initiated to test the prediction that Arsenophonus and/or Wolbachia, are capable of providing their hosts with the lacking components.
2. Materials and Methods
2.1. Sequence Retrieval
Sequences of bacteria other than Portiera were retrieved from a previous shotgun sequencing project of three whitefly species: A. dispersus, A. floccissimus, and T. vaporariorum (Santos-Garcia et al., 2015). Briefly, T. vaporariorum was collected in 2014 near the IRTA Institute of Agrifood Research and Technology (Barcelona, Spain) and identified by Dr. Francisco José Beitia. A. dispersus and A. floccissimus were collected and identified by Dr. Estrella Hernandez Suarez in 2014 from banana fields (Tenerife Island, Spain). All three whiteflies harbored Portiera, Arsenophonus and Wolbachia endosymbionts. Genomic DNA (gDNA) was obtained using an alkaline lysis method from single bacteriomes (dissected with glass microneedles). For each species, 10 single bacteriome gDNA extractions were subjected to whole-genome amplification (GenomiPhi V2, GE Healthcare) to representatively increase the total amount of gDNA (from both host and endosymbionts) before sequencing, and pooled by species. Whole-genome amplified gDNA was sequenced by Illumina HiSeq 2000 using a mate-paired library (100 bpX2, 3 kb insert size). A full description can be found in Santos-Garcia et al. (2015).
2.2. Metagenome-Assembled Genomes
For each species library, RAW Illumina reads were quality checked, and trimmed/clipped if necessary, with FastQC v0.11.3 (Andrews, 2010) and TrimmomaticPE v0.33 (Bolger et al., 2014), respectively. Kraken v0.10.6 (Wood and Salzberg, 2014) was used to classify the RAW Illumina reads with a custom genomic database including: Portiera, Hamiltonella, Rickettsia, Wolbachia, Arsenophonus, Candidatus Cardinium hertigii, several whiteflies' mitochondria, Bemisia tabaci MEAM1, and Acyrthosiphon pisum (Table S1). Reads classified as mitochondrial, insect or Portiera were discarded. The remaining reads were assembled with SPADES v3.11.0 (–meta –careful –mp) (Nurk et al., 2017). The resultant contigs were classified using Kraken and the custom database. Contigs belonging to known whitefly endosymbionts were recovered and added to the custom database. Trimmed/clipped reads were reclassified and reads belonging to known whitefly endosymbionts were reassembled alone with SPADES (–careful –mp) to produce metagenome-assembled genomes (MAGs). Illumina reads were digitally normalized (khmer v1.1) before the reassembly stage (Crusoe et al., 2015). MAG contigs were scaffolded and gap-filled with SSPACE v3 (Boetzer et al., 2011) and Gapfiller v1.10 (Boetzer and Pirovano, 2012), respectively. Finally, a manual iterative mapping approach, using Illumina trimmed/clipped reads, was performed with Bowtie2 v2.2.6 (Langmead and Salzberg, 2012), MIRA v4.9.5 (mapping mode) (Chevreux et al., 1999), and Gap4 (Staden et al., 2000) until no more contigs/reads were joined/recovered for each MAG. Finally, Bowtie2 and Pilon v.1.21 (–jumps) (Walker et al., 2014) with Illumina reads were used to correct MAG contigs.
2.3. MAG Annotation
Initial annotations of MAGs were performed with prokka v1.12 (Seemann, 2014). Enzyme commission numbers were added with PRIAM March-2015 release (Claudel-Renard et al., 2003). Gene Ontology, PFAM, and InterPro terms were added with InterProScan v5.27-66 (Jones et al., 2014). Putative pseudogenes and their positions in the genome were detected with LAST using several bacterium-related proteomes as query (Kiełbasa et al., 2011). Insertion sequences were predicted with ISsaga (Varani et al., 2011). B. tabaci genome annotations (GCA_001854935.1) were downloaded and refined with PRIAM and InterProScan. MAGs, and their corresponding Portiera (Santos-Garcia et al., 2015) and B. tabaci metabolism comparisons were performed on PathwayTools v21.5 (Karp et al., 2002). Pathway completeness was measured as the number of enzymes present in the analyzed genome over the total number of enzymes in the pathway. Heat map and clustering were performed on R (R Core Team, 2018) with ggplots2 (Wickham, 2009).
2.4. Comparative Genomics
OrthoMCL v2.0.9 was used to compute clusters of orthologous proteins (Li et al., 2003). Cluster of orthologous groups (COG) terms were assigned using DIAMOND v0.8.11.73 (July 2016 bacterial RefSeq database, Buchfink et al., 2015) and MEGAN6 (Huson et al., 2016). Average nucleotide identity (ANI) and average amino acid identity (AAI) values were obtained with the Enveomics tools (Rodriguez-R and Konstantinidis, 2016). Synteny between MAGs was checked with Mummer v3 (Kurtz et al., 2004) and Mauve, using Arsenophonus from A. dispersus (ARAD) or Wolbachia from A. dispersus (WBAD) as the reference for contig re-ordering (Darling et al., 2010). GenoPlotR was used to plot the Mauve results (Guy et al., 2010).
The presence of potential orthologs of B. tabaci genes in the other whitefly species was assessed using the Illumina cleaned data and DIAMOND, with a blastx search strategy (minimum alignment length of 25 bp and 1e−3 e-value) against the selected B. tabaci proteins (Table S2). Recovered reads were classified with Kraken, using the custom and mini-Kraken databases, to discard bacterial reads. Finally, non-bacterial reads were used as query for a blastx search against the nr database (last access: March 2018) (Altschul et al., 1990) and their best hit was classified with MEGAN6. Only reads assigned to the phylum Arthropoda were considered to be genomic reads of the screened genes in the other whitefly species.
2.5. Phylogenetics
General phylogenetic position of the newly sequenced Arsenophonus and Wolbachia were assessed using several 16S rRNA genes downloaded from GenBank. Genes were aligned with ssu-align (default masking) (Nawrocki, 2009). IQ-TREE v1.6.2 was used to select the best substitution model (SYM+R3 and TN+F+R2, respectively) and to infer the majority rule maximum likelihood (ML) tree, with its associated support values (Nguyen et al., 2015; Kalyaanamoorthy et al., 2017).
Phylogenomic trees were generated using 82 and 87 conserved proteins, selected using PhyloPhlAn v0.99 (Segata et al., 2013), from Arsenophonus and Wolbachia inferred proteomes, respectively. Protein files were sorted by species name, using fastasort from exonerate v2.2.0 (Slater and Birney, 2005) and aligned with MAFFT v7.215 (Katoh et al., 2002). Protein alignments were concatenated by species index using Geneious v11.1.2 (Kearse et al., 2012). IQ-TREE was used to infer the majority rule ML tree, and associated support values, under the substitution models JTTDCMut+F+R3 for Arsenophonus and JTT+F+I+G4 for Wolbachia.
3. Results
3.1. General Genomic Features
A total of five endosymbiont MAGs were recovered from the three studied whitefly species: Arsenophonus from A. dispersus (ARAD), A. floccissimus (ARAF) and T. vaporariorum (ARTV), and Wolbachia from A. dispersus (WBAD) and A. floccissimus (WBAF) (Figure 1 and Table 1). All MAGs were of draft status but with different assemblage quality. Only ARAD was assembled as a circular scaffold, supported by some mate-paired reads. However, a gap at the contig edges was not closed due to the presence of a dnaX duplication and a long-AT low-complexity region. The assembly of the ARAF genome was also of high quality, with only 11 contigs and a N50 value ≥500 kb. The rest of the recovered MAGs were of different draft statuses, with Wolbachia being the most fragmented. Although Wolbachia had been previously detected by PCR in T. vaporariorum (Santos-Garcia et al., 2015), its genome was impossible to assemble and analyze due to the low amount of recovered reads (Table S3). Indeed, only 21 Wolbachia contigs were recovered. Of these, only one contig was ≥1 kb (2.5X coverage) while the rest were ≤400 nt. This suggests that Wolbachia is present in T. vaporariorum but in low amounts. However, an alternative explanation is that recovered Wolbachia reads/contigs in T. vaporariorum resulted from sequence index misassignments during multiplex sequencing (index switching).
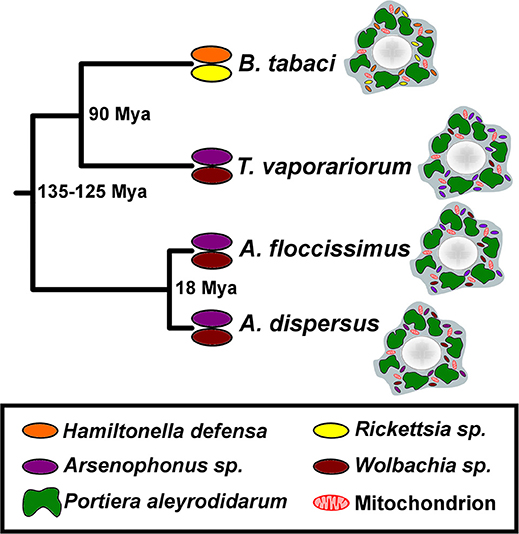
Figure 1. Whiteflies and endosymbiont content. Schematic representation of the phylogeny and endosymbionts content of the Aleurodicus, T. vaporariorum and B. tabaci whiteflies (Santos-Garcia et al., 2015). Sequences from Wolbachia were scarcely detected in T. vaporariorum, suggesting low amounts of this bacterium in the insect. Mya, million years ago.
According to their genomic features, the sequenced Arsenophonus could be divided into two groups: ARAD displayed the typical characteristics of primary endosymbionts, whereas ARAF and ARTV displayed genomic features closer to facultative or secondary endosymbionts (Table 1). This pattern was emphasized by the different genome sizes (0.67 vs. 3 Mb for ARAD vs. ARAF and ARTV), and their respective GC content (32 vs. 37%), numbers of genes (428 vs. around 2000), and presence of insertion sequences and prophages (0 vs. 12). However, both groups had a low coding density (range 41–54%) due to the presence of almost as many pseudogenes as genes. Most of the prophages in ARAF and ARTV were unrelated (based on their ANI values) except the pairs of prophages 2/8 and 3/7 from ARAF and the pair of prophage 9 from ARAF and prophage 7 from ARTV (Figures S1, S2 and Table S4).
Regarding Wolbachia, both recovered MAGs displayed sizes and genomic features similar to other sequenced species from this genus (Ellegaard et al., 2013), with WBAF being more fragmented and lacking two tRNAs. Two incomplete prophages were detected in the genome of WBAD (Figure S1).
3.2. Phylogenetic Placement
The phylogenetic positions of each assembled Arsenophonus within the genus were tested using 16S rRNA phylogeny, phylogenomics and ANI/AAI analyses. Based on the 16S rRNA phylogenetic analysis, Arsenophonus from whiteflies were distributed in at least five supported clusters, with ARAD being in a different lineage than ARAF and ARTV (Figure S3). ARAD was placed in a cluster together with other Arsenophonus from the whiteflies A. dispersus, Aleurodicus dugesii, and Bemisia centroamericana, and Candidatus Arsenophonus triatominarum from several triatomine bugs, among other insects. ARAF and ARTV were close to an Arsenophonus from T. vaporariorum, and included in a low-supported cluster with Arsenophonus symbionts from several whiteflies, bat flies and one ant. The use of a species threshold of 95% ANI (Konstantinidis and Tiedje, 2005) suggested that A. triatominarum, Candidatus Arsenophonus nilaparvata, Arsenophonus of Entylia carinata, ARAF and ARTV belong to the same species, whereas Arsenophonus nasoniae, Candidatus Arsenophonus lipopteni and ARAD form three different species (Table S4 and Figure S4). The AAI analysis showed the presence of two clusters, one for ARAD and A. nasoniae and the other for the remaining species, except the endosymbiont A. lipopteni, with a value ≤80% AAI. The phylogenomics results showed partial congruence with ANI/AAI values, placing A. triatominarum as the closest relative of ARAD (Figure 2A). The two endosymbionts formed a sister monophyletic clade to all other Arsenophonus, which formed a second monophyletic clade. A. nasoniae was placed at the basal position of both clades.
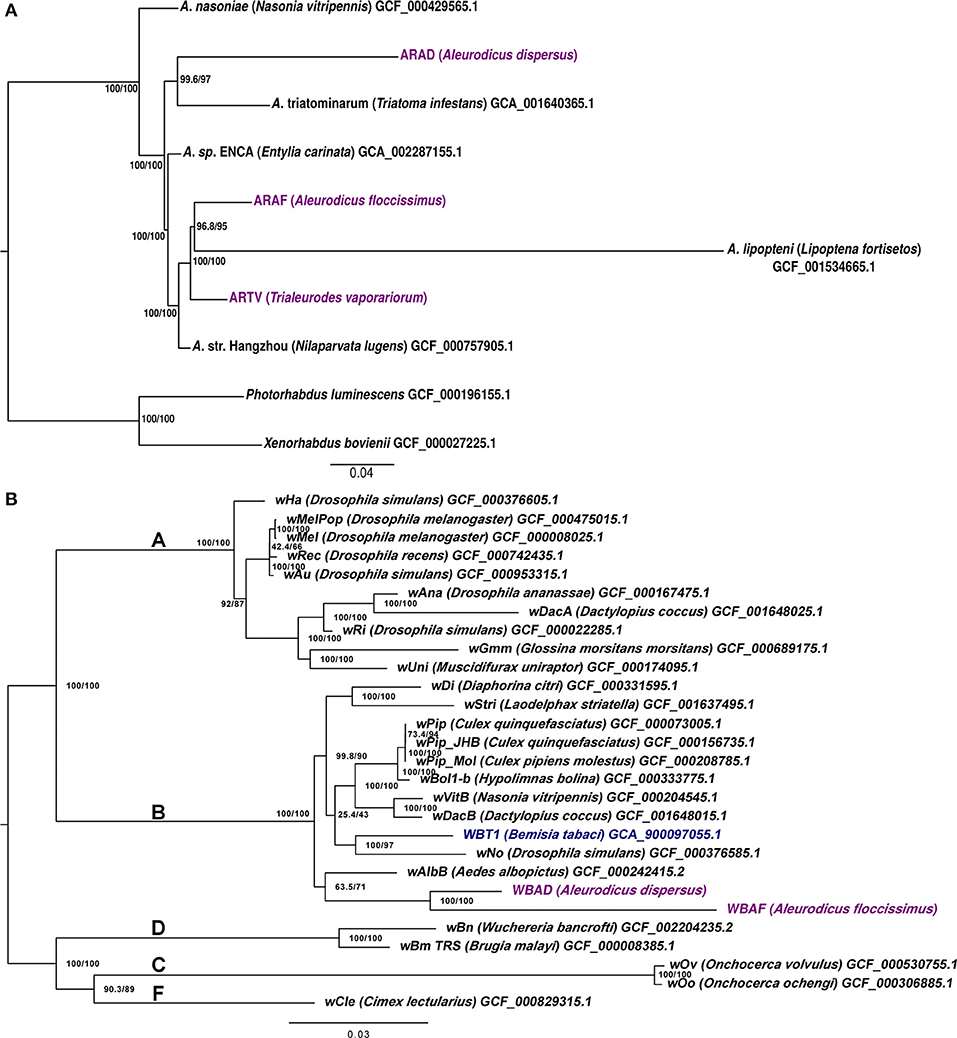
Figure 2. Phylogenomic trees of Arsenophonus and Wolbachia. (A) Arsenophonus (rooted) and (B) Wolbachia (unrooted) ML trees were inferred using 82 and 87 concatenated conserved protein alignments under a JTTDCMut+F+R3 and JTT+F+R3 substitution model, respectively. Support values were obtained with 5000 ultrafast bootstraps (right node labels) and 5000 SH-aLRT (left node labels). Names for the eukaryotic hosts are shown after the strain names in parentheses. Accession numbers are displayed for each of the proteomes used. Arsenophonus ARAD, ARAF, and ARTV and Wolbachia WBAD and WBAF are highlighted in purple. (B) Bold face letters denote the different Wolbachia supergroups. Wolbachia from Bemisia tabaci is highlighted in blue.
The phylogenetic positions of the two Wolbachia were determined with the same analyses. In the 16S rRNA phylogeny, Wolbachia WBAD and WBAF formed a well-supported clade inside a major cluster, which contains Wolbachia strains from other whiteflies and various insect taxa (Figure S5). In addition, based on phylogenomics, WBAD and WBAF were placed as a basal clade relative to the other Wolbachia from the B super group and with a Wolbachia from the mosquito Aedes albopictus as the closest genome included in the analysis (Figure 2B). Although included in the B super group, Wolbachia from B. tabaci was placed in a different cluster, closer to a Wolbachia from the fly Drosophila simulans, and as a sister group of a clade that includes several Wolbachia pipientis strains. Finally, the high nucleotide identity (97.6% ANI) between WBAD and WBAF suggested that these bacteria are strains of the same, recently diverged, species.
3.3. Comparative Genomics: Synteny and Functional Categories
When the inferred proteomes from Arsenophonus ARAD, ARAF, and ARTV were compared (Table S5), a core genome composed of only 289 clusters was obtained (Figure 3B). Interestingly, the core genome included many clusters related to vitamin and cofactor biosynthetic pathways. The small number of shared protein clusters resulted from the reduced proteome of ARAD, which is mainly a subset of the larger ARTV and ARAF proteomes. Only 4 out of the 10 specific ARAD clusters were not hypothetical proteins: pyruvate kinase II (pyruvate kinase I is present in the three proteomes), 3-oxoacyl-[acyl-carrier-protein] synthase (fabF), which is involved in fatty acid and biotin biosynthesis, and proteins encoded by recA and nudE. If only ARAF and ARTV had been compared, the core proteome would have contained more than 1,000 clusters. The number of species-specific clusters of ARTV (997) and ARAF (609) were higher than for ARAD, which is in accordance with their genome sizes. Synteny analysis highlighted the strong genome reduction in ARAD compared to ARAF and ARTV (Figure 3A). This included the loss of macrosynteny (general genome architecture), while maintaining microsynteny (e.g., operons). In contrast, ARTV and ARAD still showed a high level of macrosynteny, although some rearrangements were observed (Figure 3C).
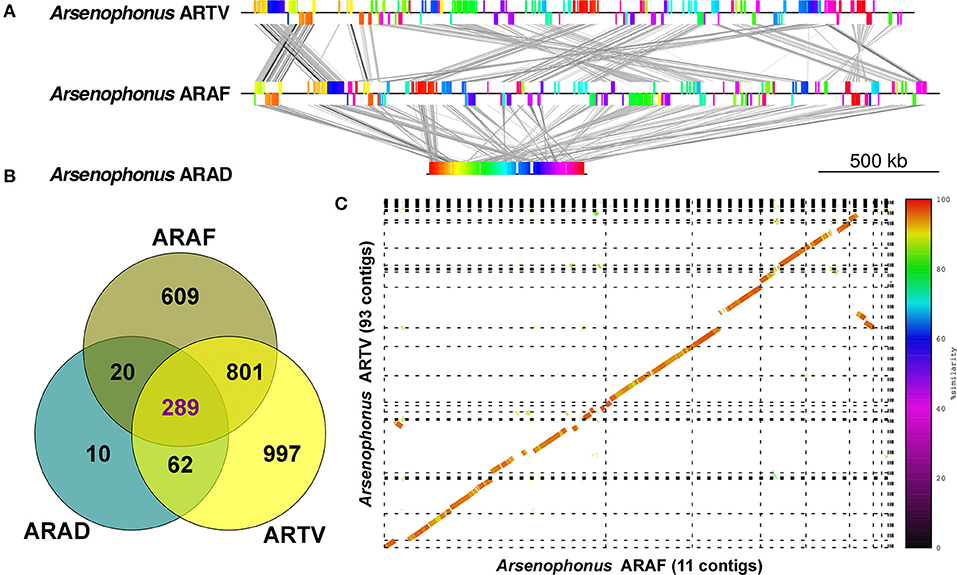
Figure 3. Comparative analyses of the three Arsenophonus MAGs. (A) Graphic linear representation of the genome rearrangements observed comparing ARTV with ARAF and ARAF with ARAD. Synteny blocks display the same color. ARAF and ARTV contigs were ordered according to ARAD before synteny blocks calculations with Mauve for a better visualization. (B) Venn diagram displaying the number of clusters found on each subspace of the Arsenophonus pangenome (Table S5). (C) Synteny between the draft genomes of ARTV and ARAF. Contig edges are displayed as dashed lines. Contigs of ARTV were ordered with Mauve using ARAF as reference for better visualization.
Wolbachia WBAD and WBAF showed a core genome of 686 clusters, and 253 and 169 species-specific clusters, respectively (Figure 4A and Table S6). Although some genomic regions (contigs) were strain-specific, it should be noted that the highly fragmented status of these genomes could produce a large number of artificial specific clusters. Comparison of the two Wolbachia genomes revealed that some of the largest contigs show the same gene order and a high level of nucleotide identity (Figure 4B), suggesting that at least microsynteny is probably kept. However, due to the draft status of the genomes, it is not clear if macrosynteny is conserved.
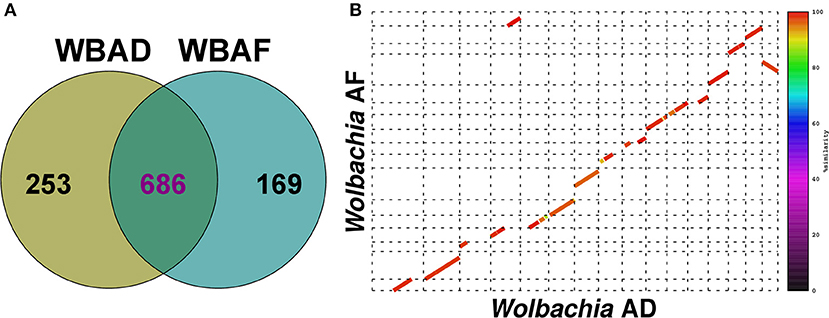
Figure 4. Comparative analyses of the two Wolbachia MAGs. (A) Venn diagram showing the shared and specific coding gene clusters between WBAD and WBAF (Table S6). (B) Synteny between some of the largest contigs from WBAD and WBAF draft genomes. Only contigs larger than 10 kb were used as input, accounting for up to 23% and 25% of the WBAD and WBAF draft genomes. Contigs of WBAF were ordered with Mauve using WBAD as reference for better visualization.
The proteomes of the five endosymbionts were functionally classified according to COGs. Because of the strong reduction of the gene repertoire in ARAD, this endosymbiont showed a smaller number of hits in each functional category, except J (translation and ribosomal structure and biogenesis), where the number of hits was similar to the other endosymbionts (Figure 5 left). In fact, in ARAD, this category had a relative frequency in the proteome ≥0.20 (Figure 5 right). In addition, category J and the other categories related to information storage and processing (A, K, and L) showed higher relative frequencies in ARAD than in the other two Arsenophonus (Figure 5 right). Based on the number of hits and relative frequencies, the loss of the gene repertoire in categories G (carbohydrate metabolism), I (lipid metabolism), and O (post-translational modification and chaperones) was lower in ARAD. As expected, Wolbachia proteomes retained the informational categories but also retained COGs related to, among others, environmental response.
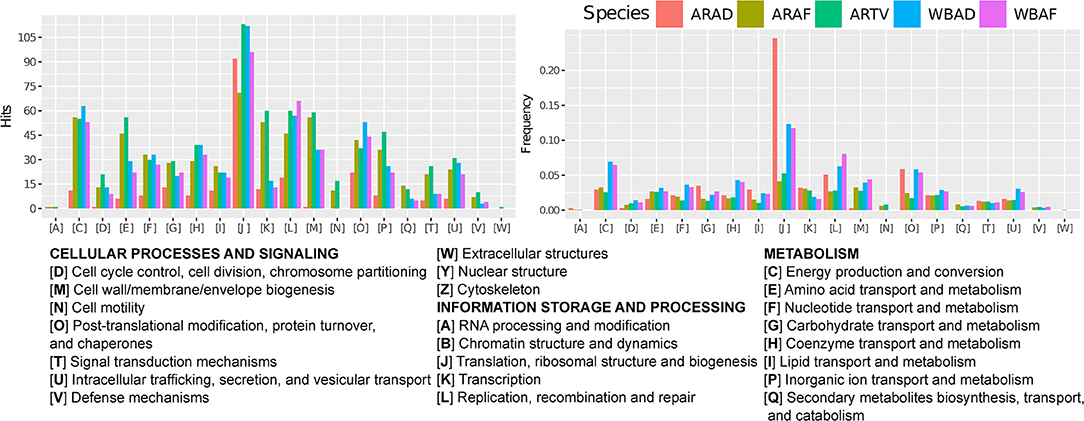
Figure 5. Distribution of the endosymbiont proteomes in functional categories. Bar plots showing the number of hits (left) and their relative frequency (right) in each functional category (COG) in the proteomes of the three analyzed Arsenophonus and the two analyzed Wolbachia MAGs.
3.4. Biosynthetic Metabolic Potential
An integrated analysis of the metabolism of the bacteriocytes of the three whitefly species was performed to predict the potential biosynthetic capabilities of the harbored Arsenophonus, Wolbachia and Portiera (Santos-Garcia et al., 2015). That of the insect host was inferred using the B. tabaci genome (GCA_001854935.1). Amino acid biosynthesis in the three whiteflies was mainly conducted by their Portiera strains [(P. aleyrodidarum from A. dispersus (PAAD), A. floccissimus (PAAF) and T. vaporariorum (PATV)], which maintained the ability to produce 4 (PATV) or 5 (PAAF and PAAD) of the 10 essential amino acids, and required some complementation from the host for the synthesis of the others (Figure 6A, cluster A). While some cofactors/vitamins and precursors were produced by almost all of the endosymbionts and the host (cluster B), others were mainly produced by some Arsenophonus (cluster C), both ARAF and ARTV, both Wolbachia and the host (cluster D), or, apparently, none of them (cluster E). In general, both Wolbachia presented lower biosynthetic potential than that of the most reduced Arsenophonus, ARAD. In addition, while both Wolbachia still presented all of the electron transport chain, ARAF and ARTV lost ubiquinol oxidase and ARAD only maintained cytochrome-c oxidase but lost ATP synthase (not shown).
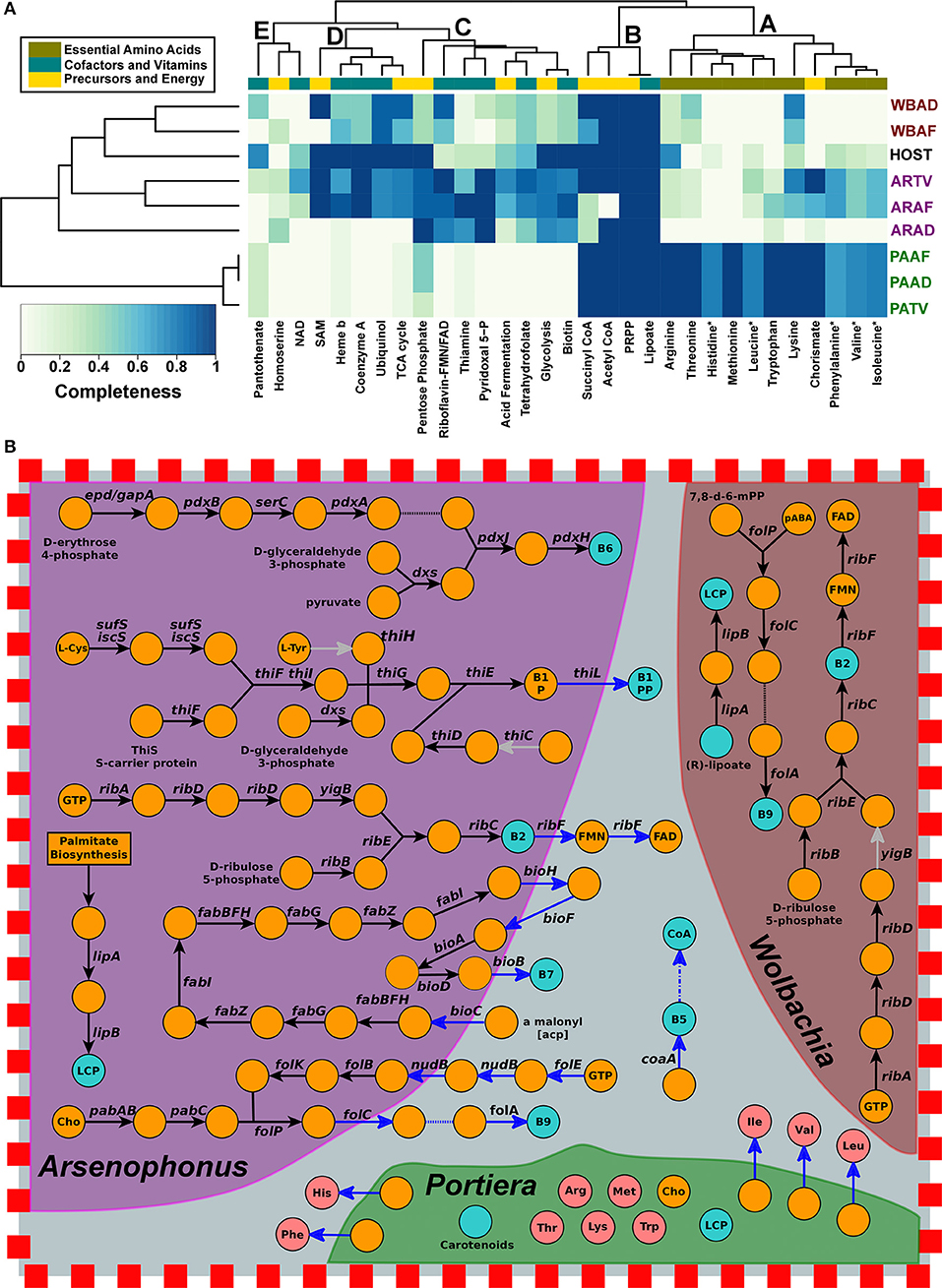
Figure 6. Biosynthetic potential of Arsenophonus, Wolbachia, Portiera and whiteflies. (A) Heat map and hierarchical clustering showing, on a color scale, the degrees of completeness of several compounds' biosynthetic pathways, including essential amino acids, cofactors and vitamins. Arsenophonus (AR), Wolbachia (WB) and Portiera (PA) from A. dispersus (AD), A. floccissimus (AF) and T. vaporariorum (TV) are highlighted in purple, red, and green, respectively. B. tabaci genome was used as a general host representative. (B) Schematic representation of some vitamin/cofactor and precursor metabolic pathways occurring in the bacteriocytes of A. dispersus. Orange, blue, and pink circles represent intermediate metabolites, vitamins/cofactors and essential amino acids, respectively. Black arrows denote enzymes present in the corresponding endosymbiont, blue arrows enzymes present in the host, light gray arrows pseudo/absent genes, dashed arrows simplified pathways, and dashed lines spontaneous reactions. Abbreviations: lipoyl carrier protein (LCP), chorismate (Cho), acyl carrier protein (acp), 4-aminobenzoate (pABA), (7,8-dihydropterin-6-yl)methyl diphosphate (7,8-d-6-mPP), diphosphate (PP).
Although the potential for essential amino acid biosynthesis of Arsenophonus and Wolbachia was limited (Figure 6A), ARAF and ARTV still retained some genes involved in the synthesis of amino acids or their precursors, such as lysine (its precursor, meso-diaminopimelate is also involved in peptidoglycan biosynthesis) and chorismate (the precursor of phenylalanine). In ARTV, the complete pathway, encoded by seven genes, from D-erythrose 4-phosphate and D-ribulose-5-phosphate to chorismate was functional (aroF, aroB, aroQ, aroE, aroL, aroA, and aroC). This, in combination with the presence of tyrA, tyrB, and an additional monofunctional chorismate mutase gene, suggests ARTV's ability to produce tyrosine and its potential collaboration with Portiera, or the host, for the production of phenylalanine. The ability to synthesize amino acids was completely lost in ARAD, suggesting that this not the reason why the lineage of ARAD evolved a close association with A. dispersus.
The contribution of the different partners to the synthesis of vitamins and cofactors was heterogeneous. ARTV and ARAF, the two Arsenophonus with larger genomes, maintained higher capabilities. Although the possibility of missed genes in the process of read recovery and assembly cannot be ruled out, ARTV and ARAF seem to have lost several genes required for some of the pathways. Despite the reductive evolutionary process in ARAD that has drastically reduced its potential, some capabilities are still retained, suggesting that they might be important in the symbiotic relationship (Figures 6A,B). ARAD, but also ARAF and ARTV, encode the complete biosynthesis pathway for pyridoxal 5′-phosphate (vitamin B6) and riboflavin (vitamin B2). However, while ARAF and ARTV are predicted to be able to produce flavin mononucleotide (FMN) and flavin adenine dinucleotide (FAD) because they harbor a functional ribF gene, it is pseudogenized in ARAD. Since this gene is present in all whitefly hosts, both flavin cofactors may be produced in A. dispersus through complementation between ARAD and its host (Figure 6B, Table S2). In addition, considering functions that can be complemented by the host, ARAD is predicted to be able to synthesize thiamine (vitamin B1) and folate (vitamin B9, from imported chorismate) (Figure 6B). Although ARAD has lost thiH, some host-encoded proteins could potentially replace its function (Table S2). ARAF and ARTV also require complementation by their host to produce folate. ARTV has lost most of the thiamine-production pathway, and the missing steps are not complemented by its host. In addition, B. tabaci seems to be able to produce biotin using some horizontally transferred genes (bioA, bioB, and bioD) (Luan et al., 2015), a protein capable of replacing the BioC function, and the insect's own fatty acid biosynthesis pathway. Genes with similar function to bioABCDFH genes were found to be present in T. vaporariorum genomic reads, suggesting that this whitefly is also capable of producing biotin by itself. Because only genes similar to bioBCFH were identified in the genomic reads from both Aleurodicus species (Table S2), the presence of bioA and bioD in ARAD and ARAF is required for the cooperative synthesis of biotin. However, ARAD, unlike ARAF, has only retained the two missing genes in the genomes of both Aleurodicus.
In ARAF, the gene yigB which is part of the riboflavin pathway, was pseudogenized. This gene encodes a Haloacid Dehalogenase (HAD) phosphatase that belongs to a superfamily of enzymes. Therefore, the activity of the protein encoded by this gene can likely be substituted by related genes in the endosymbiont or host genomes (Manzano-Marín et al., 2015). Finally, all Arsenophonus conserved the glycolysis and pentose phosphate pathways to produce the precursors required for riboflavin, thiamine, and pyridoxal. Indeed, in ARAD, the enzyme encoded by gatA from the glycolysis pathway seems to have replaced the epd product in the pyridoxal pathway. The combination of the ilvC-encoded enzyme in Portiera (Price and Wilson, 2014) and the panBC genes that were horizontally transferred to B. tabaci allows this whitefly to produce its own pantothenate (vitamin B5) and coenzyme A. However, panBC genes are not likely to be present in the genomes of A dispersus, A. floccissimus, or T. vaporariorum, where only bacterial hits were recovered, showing no similarity to the ones present in the B. tabaci genome. This suggests that these whiteflies are not able to produce pantothenate and they acquire it from the diet or other sources (Figure 6B and Table S2). However, failure of horizontally transferred gene detection could be an artifact of the methods used, since they were unable to discover new horizontally transferred genes in the screened whiteflies.
The third endosymbiont of A. dispersus and A. floccissimus, Wolbachia WBAD and WBAF, may also contribute to the vitamin/cofactor synthesis of the system. Similar to Arsenophonus ARAD and ARAF, they encode the complete pathway for riboflavin–FMN–FAD synthesis and might be able to produce folate from some intermediate precursors (Figure 6B). In addition, WBAD and WBAF are able to activate lipoate. However, as indicated above, we cannot rule out the presence of other biosynthetic pathways that were lost in the WBAD and WBAF genomes due to the large fragmentation of the assemblages. The same could be happening with respect to incomplete pathways, where gaps may simply reflect genes that were missed during the assembly process.
4. Discussion
Some bacterial lineages show predominantly facultative symbiotic lifestyles with insects. In such cases, they can inhabit the cytoplasm of bacteriocytes and coexist with the primary endosymbiont. These bacterial lineages usually have large gene repertoires and genomes, allowing them to be autonomous and capable of infecting individuals from very different taxa. Examples of such clades are the genera Arsenophonus, Sodalis, and Serratia (Nováková et al., 2009; Manzano-Marín et al., 2017; Santos-Garcia et al., 2017). Because their presence may have fitness costs for the host, they do not become fixed in the insect lineage except if their nutritional contribution (or other kind of benefit) is important and is required for multiple generations. Under such a scenario, a facultative endosymbiont may evolve toward a more intimate association, becoming a co-primary symbiont (Lamelas et al., 2011). Although there are several features associated with this new lifestyle, the most relevant is a decrease in genome size. In the lineage Arsenophonus–Riesia, the sizes of several genomes have been reported, ranging from 0.58 Mb for Candidatus Riesia pediculicola (Kirkness et al., 2010) and 0.84 Mb for A. lipopteni (Nováková et al., 2016) to 3.86 Mb for A. triatominarum, but with several genomes of intermediate size (Šochová et al., 2017). Similarly, Sodalis endosymbionts display a large range of genome sizes, from 0.35 Mb for Candidatus Mikella endobia (Husnik and McCutcheon, 2016) to circa 4.5 Mb for Sodalis glossinidius or Candidatus Sodalis pierantonius, and with several genome sizes in the range of 1–2 Mb (Santos-Garcia et al., 2017). This range has also been observed in Serratia symbiotica, with genome sizes from 0.65 to 3.86 Mb (Manzano-Marín et al., 2016). To the best of our knowledge, Arsenophonus ARAD is among the smallest genomes in its genus, between R. pediculicola and A. lipopteni (Šochová et al., 2017). ARAD is still undergoing a genome reduction process that could end up ≤0.4 Mb if the current 54% coding density is considered. The ARAD gene repertoire is clearly a subset of Arsenophonus from other whiteflies'. It is totally dependent on the host environment and, as we discuss below, putatively supplies/complements its host with cofactors/vitamins that are not produced by Portiera and/or the host. Thus, ARAD can be considered a co-primary endosymbiont. On the other hand, ARAF and ARTV are still at an intermediate stage of the genome-reduction process (e.g., high number of pseudogenes, inactivation of mobile genetic elements, and virulence/secretion factors), but they are already showing dependence on the host environment. Therefore, they can be considered relatively recent obligate endosymbionts with the potential to establish a co-primary symbiotic relationship with Portiera and their host (Latorre and Manzano-Marín, 2016).
Based on phylogenetic and molecular methods, Thao and Baumann (2004) indicated that whiteflies have experienced multiple Arsenophonus infections. Nováková et al. (2009) phylogenetic analysis placed these Arsenophonus from whiteflies in five, probably six, lineages in a broader phylogeny that includes several Arsenophonus from different hosts. Despite some topological differences produced by low-supported clades, our results are in accordance with both studies. With respect to the Arsenophonus 16S rRNA phylogenetic relationships, the long branches of the A. dispersus/A. dugesii lineage, which includes ARAD, suggest a more ancient infection compared to Arsenophonus from other insects, including whiteflies (with the exception of B. centroamericana). In addition, it should be noted that a second Arsenophonus from A. dugesii presented a shorter branch and was closer to B. centroamericana. This suggests a potential endosymbiont replacement, closely related to the one in B. centroamericana, in this Aleurodicus species. The ARTV/ARAF cluster contains several whitefly species and some of them may have been infected by the same, or closely related, Arsenophonus strains. In the case of ARAF, the long branch is an artifact of the incompletely recovered copy of the 16S rRNA gene, and not the result of a long-term association with its host. Nevertheless, our phylogenomic and ANI/AAI analyses strongly support ARAD's belonging to a different lineage from the other two whiteflies' Arsenophonus, ARAF and ARTV. Two scenarios can explain the fact that ARAD and ARAF, despite their host belonging to the same genus, are from two different lineages. In the first, the ancestor of ARAD infected the lineage of A. dispersus after its divergence from A. floccissimus and, later, started its shift to a primary symbiosis and its associated genome-reduction process. In this case, the process could have taken place for as long as 18 million years (My), the estimated divergence time between these two whitefly species (Santos-Garcia et al., 2015). An alternative scenario is that this association began prior to the divergence of both Aleurodicus species, but later, the ARAD-type symbiont was replaced in the A. floccissimus lineage by another Arsenophonus lineage (ARAF). Although both scenarios are possible, the large number of pseudogenes in the ARAD genome suggests that the initiation of the process was relatively recent, as suggested by the first scenario. For example, Riesia, which also shows a strong genome reduction, started its symbiosis with Pediculus lice around 13–25 My ago (Mya) (Kirkness et al., 2010). Still, the second scenario cannot be excluded as symbionts, independent of their symbiotic status, can be replaced by other bacteria of the same, or different genera, as long as the newcomers have similar biosynthetic capabilities. Indeed, repeated symbiont replacements have been documented in many insect lineages, including other sternorrhynchans such as aphids and mealybugs (Husnik and McCutcheon, 2016; Manzano-Marín et al., 2017; Meseguer et al., 2017; Russell et al., 2017; Sudakaran et al., 2017). Regardless of whether ARAD was already present in the Aleurodicus ancestor, and taking into account that this genus diverged from the Trialeurodes (Aleyrodinae) ancestor more than 100 Mya (Shcherbakov, 2000; Drohojowska and Szwedo, 2015), it is clear that both ARAF and ARTV originated from independent recent infections by the same, or closely related, Arsenophonus strains. Previous works have demonstrated that horizontal transmission of endosymbionts between whiteflies can be plant and/or parasitoid mediated; however, all reported cases have involved endosymbionts that are not restricted in the bacteriocyte (Chiel et al., 2009; Caspi-Fluger et al., 2012; Ahmed et al., 2015). The fact that all reported Arsenophonus are bacteriocyte-restricted makes this hypothesis less plausible (Gottlieb et al., 2008; Skaljac et al., 2013; Marubayashi et al., 2014; Pandey and Rajagopal, 2015) and gives more support to the idea that an opportunistic Arsenophonus, for example one that was gut-associated or from other insects but with the ability to invade new hosts, was the source of those infections. Later on, during the establishment of this Arsenophonus in the new host's environment, it could derive into an obligate endosymbiont, as in the case of ARAF and ARTV.
Nutritionally poor diets, such as phloem sap or blood, cannot provide insects with all of their dietary requirements, including several vitamins. For example, the essentiality of dietary riboflavin was demonstrated in aposymbiotic aphids, but not in aphids harboring their primary endosymbiont Buchnera aphidicola, which is able to produce riboflavin (Nakabachi and Ishikawa, 1999). Moreover, B. aphidicola of aphids from the subfamily Lachninae had already lost the genes encoding the pathway in the Lachninae ancestor. This led to the establishment in this group of a new association with an additional bacterium, capable of producing riboflavin. In most lineages, this is the co-primary S. symbiotica, a facultative endosymbiont in other aphid lineages (Manzano-Marín and Latorre, 2014). The analyses of the co-symbiosis in species of Lachninae revealed a complex system with S. symbiotica endosymbionts harboring a very small genome (in Tuberolachnus salignus) or intermediate genome sizes (in some species of the genus Cinara), or being replaced by other endosymbionts belonging to different genera, such as Sodalis, Erwinia, or Hamiltonella, among others (Manzano-Marín et al., 2016, 2017; Meseguer et al., 2017). The potentially essential role of riboflavin biosynthesis in establishing symbiotic relationships has also been demonstrated in several blood-feeding invertebrates harboring different endosymbionts, such as the leech Haementeria officinalis and its Candidatus Providencia siddallii endosymbiont (Manzano-Marín et al., 2015), the Wolbachia associated with the bedbug Cimex lectularius (Moriyama et al., 2015), or the Coxiella-like bacteria found in several ticks (Gottlieb et al., 2015). Indeed, the Arsenophonus–Riesia lineage includes several examples of intimate host–symbiont associations with the production of B vitamins as a pivotal role, but also several facultative symbionts of this lineage have the potential to produce vitamins and cofactors. For example, the louse fly Melophagus ovinus is a hematophagous dipteran that relies on Candidatus Arsenophonus melophagi, its primary endosymbiont, to acquire B vitamins that are not present in its diet, i.e, riboflavin, pyridoxine, and biotin (Nováková et al., 2015). A. lipopteni is the primary endosymbiont of another hematophagous louse fly (Lipoptena cervi), and similar to A. melophagi, it has the potential to produce riboflavin, pyridoxine, and biotin (Nováková et al., 2016). In the human louse Pediculus humanus, another hematophagous insect, R. pediculicola is in charge of supplying its host with several B vitamins including pantothenate, riboflavin, and biotin (Kirkness et al., 2010). A. nasoniae from the parasitic wasp Nasonia vitripennis has broad metabolic potential, including the ability to produce riboflavin, biotin, folate, thiamine, and pyridoxine (Darby et al., 2010). The brown planthopper Nilaparvata lugens feeds exclusively on rice phloem and has a yeast-like primary endosymbiont that produces essentials amino acids, and helps its host with nitrogen recycling but also steroids synthesis. However, N. lugens always has Arsenophonus or Wolbachia as secondary endosymbionts. Again, the Arsenophonus from N. lugens has broad metabolic potential and is able to produce riboflavin, biotin, folate, thiamine, and pyridoxine (Xue et al., 2014). Similar biosynthetic potential was found in the Arsenophonus from the treehopper Entylia carinata, another phloem-feeding insect, although in this case Arsenophonus is not fixed in the population (Mao et al., 2017). Interestingly, Arsenophonus seems to be the most common secondary endosymbiont found in whiteflies, always sharing the bacteriocytes with Portiera and in most cases being fixed (or close to it) in the tested populations (Thao and Baumann, 2004; Gottlieb et al., 2008; Skaljac et al., 2013; Cass et al., 2014; Kapantaidaki et al., 2014; Marubayashi et al., 2014; Pandey and Rajagopal, 2015; Santos-Garcia et al., 2015). Therefore, taking into account the metabolic potential of other Arsenophonus infecting different hosts, we hypothesize that the ability to synthesize riboflavin and other vitamins is probably the main reason for the presence of Arsenophonus endosymbionts in whiteflies and for the evolution of ARAD toward a co-primary symbiont in A. dispersus. In such a scenario, the continuous acquisitions/replacements of Arsenophonus in whiteflies could maintain, at the population level, the availability of such vitamins when the concurrent secondary endosymbiont is no longer able to produce them, or when a newcomer with greater metabolic potential and the ability to establish symbiotic relationships arrives.
Although thiamine, pantothenate, coenzyme A, niacin, pyridoxal and biotin can be found in the phloem (free or bound to transporter proteins) (Zimmermann and Milburn, 1975), their concentrations might not be sufficient for many phloem-feeding species. This could explain why the ability to produce some of these compounds has been retained in bacterial endosymbionts whereas it has been lost in others. However, the production of one or several B vitamins (thiamine, riboflavin, pyridoxal, folate, and biotin) and de novo lipoate by whiteflies' Arsenophonus seems to be the main contribution of these endosymbionts to their hosts, allowing them to feed on the limited diet present in the phloem (Dale et al., 2006; Nováková et al., 2015, 2016; Mao et al., 2017). Regarding biotin biosynthesis, the bacterial origin of bioAB and bioD was reported by Luan et al. (2015) and Ankrah et al. (2017). We show here that not all whiteflies seem to have the horizontally transferred bioAD genes, although the rest of the bio operon homologs, including bioB, are present. This suggests that some whiteflies acquired, by several horizontal gene-transfer events, the ability to produce biotin. This is expected to produce a gradual loss of the biotin pathway in secondary endosymbionts of the different whitefly lineages, at least from the Aleyrodinae subfamily, as this group harbors the full bio operon. Indeed, Cardinium infecting B. tabaci has recently lost its ability to produce biotin, raising the possibility that biotin is provided by the co-present Hamiltonella endosymbiont or by the host's metabolic potential (Santos-Garcia et al., 2014b; Rao et al., 2015). As an alternative, this can also lead to intricate complementation patterns as shown in the Arsenophonus from Aleurodicus. It is important to notice that supplementation of vitamins was the role proposed for Hamiltonella in B. tabaci (Rao et al., 2015) and the results reported here support a similar function for Arsenophonus. These two endosymbionts are generally never found together in the same bacteriocyte (Gottlieb et al., 2008; Skaljac et al., 2013; Marubayashi et al., 2014). It could be that Hamiltonella and Arsenophonus compete for the same host resources to produce the same compounds, undermining their unique benefit to the host if harbored together due to the associated cost of maintaining different endosymbionts at high loads (Ferrari and Vavre, 2011). Interestingly, Skaljac et al. (2010) reported that Hamiltonella and Arsenophonus are, apparently, found together in T. vaporariorum bacteriocytes, and present the same sub-cellular localization. However, a worldwide T. vaporariorum population study, conducted by Kapantaidaki et al. (2014), concluded that this whitefly is a single species with low levels of intraspecific variation. In addition, Arsenophonus was almost fixed in all populations, no presence of Hamiltonella was detected, and only sporadic infections of Wolbachia, Cardinium and Rickettsia were reported (a few individuals per population) (Kapantaidaki et al., 2014). Similar results were shown by Marubayashi et al. (2014) in Brazilian T. vaporariorum populations, where Arsenophonus was fixed in the population and no Hamiltonella co-infection was recorded. Indeed, our T. vaporariorum sequencing results support these latter studies, with few reads classified as Hamiltonella (216) and these possibly being misclassifications, and Wolbachia previously detected by PCR (Santos-Garcia et al., 2015) but with few reads assigned to it.
Finally, and in contrast to Arsenophonus, Wolbachia from Aleurodicus species only produces riboflavin de novo and, probably, folate and lipoate from some intermediate metabolites. It has been shown that the bedbug C. lectularius requires the riboflavin produced by Wolbachia for its proper development. Indeed, the riboflavin pathway is conserved among Wolbachia species infecting different invertebrates, suggesting that it may provide a fitness benefit to the infected host (Moriyama et al., 2015). However, it should be noted that while Hamiltonella and Arsenophonus are restricted to the bacteriocytes, Wolbachia is also found in other tissues/cells (Gottlieb et al., 2008; Skaljac et al., 2010, 2013; Bing et al., 2014; Marubayashi et al., 2014). While the first pattern is more characteristic of hemipteran mutualistic endosymbiosis, the second can be found in endosymbionts with a wide range of symbiotic interactions, including parasitism. In addition, these mutualistic endosymbionts are usually fixed, or close to fixed, in different insect populations, as in the case of Arsenophonus in T. vaporariorum or Trialeurodes abutiloneus (Cass et al., 2014; Kapantaidaki et al., 2014), or Hamiltonella in B. tabaci MEAM1 and MED-Q1 species (Zchori-Fein et al., 2014). However, in most reported cases of whiteflies harboring Wolbachia, the individual insects usually also harbor Arsenophonus or Hamiltonella (Skaljac et al., 2010, 2013; Kapantaidaki et al., 2014; Marubayashi et al., 2014; Zchori-Fein et al., 2014). Nevertheless, special attention should be given to some Asian species of B. tabaci and to the ash whitefly Siphoninus phillyreae. While Wolbachia from B. tabaci AsiaII-1 and AsiaII-3 species seem to be fixed at the population level, a mid-to-high prevalence of Arsenophonus can also be observed (Bing et al., 2013a). In addition, although Wolbachia from B. tabaci China1 is fixed in the population, another bacteriocyte-restricted endosymbiont, Candidatus Hemipteriphilus asiaticus, is found in this species (Bing et al., 2013a,b). Whether Wolbachia contributes to the host diet on these B. tabaci species when no other bacteriocyte-restricted secondary endosymbiont is present, or if whether it is the sole source of riboflavin, warrants further investigation. In S. phillyreae, Wolbachia seems to be confined to the bacteriocyte, but it is far from being fixed in the tested populations, undermining the importance of this endosymbiont for whitefly nutrition (Skaljac et al., 2013). Finally, the other few cases in which Wolbachia was found to be the unique secondary endosymbiont should be handled with caution, as they may simply result from failure of general PCR primers to amplify new symbiont species/strains (Augustinos et al., 2011), or the presence of previously unknown endosymbionts such as Hemipteriphilus. In summary, tissue tropism, population infection levels, and genome information suggest that unlike Hamiltonella and Arsenophonus, Wolbachia is not a mutualistic endosymbiont, at least at the metabolic level, for whiteflies.
In conclusion, the loss of genes encoding the enzymes for the synthesis of vitamins in the ancestral Portiera, many millions of years ago, likely generated the requirement of a co-symbiont in whiteflies. Arsenophonus species seem to be the most common co-symbiont. In the lineage of A. dispersus, the harbored Arsenophonus lineage presents a highly reduced genome content. This Arsenophonus lineage is, or is in the process of becoming, a co-primary symbiont which putatively supplies/complements its host with cofactors/vitamins that are not produced by its co-partner Portiera.
Data Availability Statement
The Arsenophonus (ERZ502704-6) and Wolbachia (ERZ502707-8) annotated genomes and the whole genome shotgun libraries (ERR2532344-45 and ERR2534068-71) analyzed for this study have been deposited in the European Nucleotide Archive (ENA) under project number PRJEB26014. The generated Pathway Tools databases and the blastx results of the genomic reads similar to Bemisia tabaci key cofactors/vitamins metabolic genes can be found at https://doi.org/10.6084/m9.figshare.6142307.v1.
Author Contributions
DS-G and FS conceived the study. DS-G and FS performed bioinformatics analysis. KJ performed the phylogenetic analysis. All authors analyzed and discussed the data. DS-G and FS drafted the manuscript with input from AL, AM, EZ-F, SF, and SM. All authors participated in the revision of the manuscript.
Funding
This work was supported by the grants PROMETEOII/2014/065 (Conselleria d'Educació, Generalitat Valenciana, Spain) to AM, BFU2015-64322-C2-1-R (co-financed by FEDER funds and Ministerio de Economía y Competitividad, Spain) to AL, and by the Israel Science Foundation (Israel) grant Nos. 1039/12 to SM and 484/17 to SF and EZ-F.
Conflict of Interest Statement
The authors declare that the research was conducted in the absence of any commercial or financial relationships that could be construed as a potential conflict of interest.
Acknowledgments
DS-G is the recipient of a Golda Meir Postdoctoral Fellowship from the Hebrew University of Jerusalem. The authors acknowledge Francisco J. Beitia and Estrella Hernández Suárez for the whitefly samples, and two reviewers for their suggestions on early drafts of the manuscript.
Supplementary Material
The Supplementary Material for this article can be found online at: http://journal.frontiersin.org/article/10.3389/fmicb.2018.02254/full#supplementary-material
References
Ahmed, M. Z., Li, S.-J., Xue, X., Yin, X.-J., Ren, S.-X., Jiggins, F. M., et al. (2015). The intracellular bacterium Wolbachia uses parasitoid wasps as phoretic vectors for efficient horizontal transmission. PLoS Pathog. 10:e1004672. doi: 10.1371/journal.ppat.1004672
Altschul, S. F., Gish, W., Miller, W., Myers, E. W., and Lipman, D. J. (1990). Basic local alignment search tool. J. Mol. Biol. 215, 403–410. doi: 10.1016/S0022-2836(05)80360-2
Andrews, S. (2010). Fastqc: A Quality Control Tool for High Throughput Sequence Data. Cambridge, UK: Bioinformatics Group, Babraham Institute.
Ankrah, N. Y., Luan, J., and Douglas, A. E. (2017). Cooperative metabolism in a three-partner insect-bacterial symbiosis revealed by metabolic modeling. J. Bacteriol. 199, 1–15. doi: 10.1128/JB.00872-16
Augustinos, A. A., Santos-Garcia, D., Dionyssopoulou, E., Moreira, M., Papapanagiotou, A., Scarvelakis, M., et al. (2011). Detection and characterization of Wolbachia infections in natural populations of aphids: is the hidden diversity fully unraveled? PLoS ONE 6:e28695. doi: 10.1371/journal.pone.0028695
Bing, X.-L., Ruan, Y.-M., Rao, Q., Wang, X.-W., and Liu, S.-S. (2013a). Diversity of secondary endosymbionts among different putative species of the whitefly Bemisia tabaci. Insect Sci. 20, 194–206. doi: 10.1111/j.1744-7917.2012.01522.x
Bing, X.-L., Yang, J., Zchori-Fein, E., Wang, X.-W., and Liu, S.-S. (2013b). Characterization of a newly discovered symbiont of the whitefly Bemisia tabaci (Hemiptera: Aleyrodidae). Appl. Environ. Microbiol. 79, 569–575. doi: 10.1128/AEM.03030-12
Bing, X. L., Xia, W. Q., Gui, J. D., Yan, G. H., Wang, X. W., and Liu, S. S. (2014). Diversity and evolution of the Wolbachia endosymbionts of Bemisia (Hemiptera: Aleyrodidae) whiteflies. Ecol. Evol. 4, 2714–2737. doi: 10.1002/ece3.1126
Boetzer, M., Henkel, C. V., Jansen, H. J., Butler, D., and Pirovano, W. (2011). Scaffolding pre-assembled contigs using SSPACE. Bioinformatics 27, 578–579. doi: 10.1093/bioinformatics/btq683
Boetzer, M., and Pirovano, W. (2012). Toward almost closed genomes with GapFiller. Genome Biol. 13:R56. doi: 10.1186/gb-2012-13-6-r56
Bolger, A. M., Lohse, M., and Usadel, B. (2014). Trimmomatic: a flexible trimmer for Illumina sequence data. Bioinformatics 30, 2114–2120. doi: 10.1093/bioinformatics/btu170
Buchfink, B., Xie, C., and Huson, D. H. (2015). Fast and sensitive protein alignment using DIAMOND. Nat. Methods 12, 59–60. doi: 10.1038/nmeth.3176
Campbell, B. C., Steffen-Campbell, J. D., and Gill, R. J. (1994). Evolutionary origin of whiteflies (Hemiptera: Sternorrhyncha: Aleyrodidae) inferred from 18S rDNA sequences. Insect Mol. Biol. 3, 73–88.
Caspi-Fluger, A., Inbar, M., Mozes-Daube, N., Katzir, N., Portnoy, V., Belausov, E., et al. (2012). Horizontal transmission of the insect symbiont Rickettsia is plant-mediated. Proc. Biol. Sci. 279, 1791–1796. doi: 10.1098/rspb.2011.2095
Cass, B. N., Mozes-Daube, N., Iasur-Kruh, L., Bondy, E. C., Kelly, S. E., Hunter, M. S., et al. (2014). Bacterial endosymbionts in field-collected samples of Trialeurodes sp. nr. abutiloneus (Hemiptera: Aleyrodidae). Res. Microbiol. 165, 77–81. doi: 10.1016/j.resmic.2014.01.005
Chevreux, B., Wetter, T., and Suhai, S. (1999). Genome sequence assembly using trace signals and additional sequence information. Comput. Sci. Biol. 99, 45–56.
Chiel, E., Zchori-Fein, E., Inbar, M., Gottlieb, Y., Adachi-Hagimori, T., Kelly, S. E., et al. (2009). Almost there: transmission routes of bacterial symbionts between trophic levels. PLoS ONE 4:e4767. doi: 10.1371/journal.pone.0004767
Claudel-Renard, C., Chevalet, C., Faraut, T., and Kahn, D. (2003). Enzyme-specific profiles for genome annotation: PRIAM. Nucleic Acids Res. 31, 6633–6639. doi: 10.1093/nar/gkg847
Crusoe, M. R., Alameldin, H. F., Awad, S., Boucher, E., Caldwell, A., Cartwright, R., et al. (2015). The khmer software package: enabling efficient nucleotide sequence analysis. F1000Res 4:900. doi: 10.12688/f1000research.6924.1
Dale, C., Beeton, M., Harbison, C., Jones, T., and Pontes, M. (2006). Isolation, pure culture, and characterization of “Candidatus Arsenophonus arthropodicus,” an intracellular secondary endosymbiont from the hippoboscid louse fly Pseudolynchia canariensis. Appl. Environ. Microbiol. 72, 2997–3004. doi: 10.1128/AEM.72.4.2997-3004.2006
Darby, A. C., Choi, J. H., Wilkes, T., Hughes, M. A., Werren, J. H., Hurst, G. D. D., et al. (2010). Characteristics of the genome of Arsenophonus nasoniae, son-killer bacterium of the wasp Nasonia. Insect Mol. Biol. 19(Suppl. 1), 75–89. doi: 10.1111/j.1365-2583.2009.00950.x
Darling, A. E., Mau, B., and Perna, N. T. (2010). progressiveMauve: multiple genome alignment with gene gain, loss and rearrangement. PLoS ONE 5:e11147. doi: 10.1371/journal.pone.0011147
Douglas, A. E. (2006). Phloem-sap feeding by animals: problems and solutions. J. Exp. Bot. 57, 747–754. doi: 10.1093/jxb/erj067
Drohojowska, J., and Szwedo, J. (2015). Early cretaceous Aleyrodidae (Hemiptera: Sternorrhyncha) from the Lebanese amber. Cretac. Res. 52, 368–389. doi: 10.1016/j.cretres.2014.03.015
Ellegaard, K. M., Klasson, L., Näslund, K., Bourtzis, K., and Andersson, S. G. E. (2013). Comparative genomics of Wolbachia and the bacterial species concept. PLoS Genet. 9:e1003381. doi: 10.1371/journal.pgen.1003381
Ferrari, J., and Vavre, F. (2011). Bacterial symbionts in insects or the story of communities affecting communities. Philos. Trans. R. Soc. Lond. B Biol. Sci. 366, 1389–1400. doi: 10.1098/rstb.2010.0226
Gottlieb, Y., Ghanim, M., Gueguen, G., Kontsedalov, S., Vavre, F., Fleury, F., et al. (2008). Inherited intracellular ecosystem: symbiotic bacteria share bacteriocytes in whiteflies. FASEB J. 22, 2591–2599. doi: 10.1096/fj.07-101162
Gottlieb, Y., Lalzar, I., and Klasson, L. (2015). Distinctive genome reduction rates revealed by genomic analyses of two Coxiella-like endosymbionts in ticks. Genome Biol. Evol. 7, 1779–1796. doi: 10.1093/gbe/evv108
Guy, L., Kultima, J. R., and Andersson, S. G. E. (2010). genoPlotR: comparative gene and genome visualization in R. Bioinformatics 26, 2334–2335. doi: 10.1093/bioinformatics/btq413
Hosokawa, T., Koga, R., Kikuchi, Y., Meng, X.-Y., and Fukatsu, T. (2010). Wolbachia as a bacteriocyte-associated nutritional mutualist. Proc. Natl. Acad. Sci. U.S.A. 107, 769–774. doi: 10.1073/pnas.0911476107
Husnik, F., and McCutcheon, J. P. (2016). Repeated replacement of an intrabacterial symbiont in the tripartite nested mealybug symbiosis. Proc. Natl. Acad. Sci. U.S.A. 113, E5416–E5424. doi: 10.1073/pnas.1603910113
Huson, D. H., Beier, S., Flade, I., Górska, A., El-Hadidi, M., Mitra, S., et al. (2016). MEGAN Community Edition - interactive exploration and analysis of large-scale microbiome sequencing data. PLoS Comput. Biol. 12, 1–12. doi: 10.1371/journal.pcbi.1004957
Jones, P., Binns, D., Chang, H.-Y., Fraser, M., Li, W., McAnulla, C., et al. (2014). InterProScan 5: genome-scale protein function classification. Bioinformatics 30, 1236–1240. doi: 10.1093/bioinformatics/btu031
Kalyaanamoorthy, S., Minh, B. Q., Wong, T. K. F., von Haeseler, A., and Jermiin, L. S. (2017). ModelFinder: fast model selection for accurate phylogenetic estimates. Nat. Methods 14, 587–589. doi: 10.1038/nmeth.4285
Kapantaidaki, D. E., Ovčarenko, I., Fytrou, N., Knott, K. E., Bourtzis, K., and Tsagkarakou, A. (2014). Low levels of mitochondrial DNA and symbiont diversity in the worldwide agricultural pest, the greenhouse whitefly Trialeurodes vaporariorum (Hemiptera: Aleyrodidae). J. Hered. 106, 80–92. doi: 10.1093/jhered/esu061
Karp, P. D., Paley, S., and Romero, P. (2002). The pathway tools software. Bioinformatics 18(Suppl. 1), S225–S232. doi: 10.1093/bioinformatics/18.suppl_1.S225
Katoh, K., Misawa, K., Kuma, K.-i., and Miyata, T. (2002). MAFFT: a novel method for rapid multiple sequence alignment based on fast Fourier transform. Nucleic Acids Res. 30, 3059–3066. doi: 10.1093/nar/gkf436
Kearse, M., Moir, R., Wilson, A., Stones-Havas, S., Cheung, M., Sturrock, S., et al. (2012). Geneious Basic: an integrated and extendable desktop software platform for the organization and analysis of sequence data. Bioinformatics 28, 1647–1649. doi: 10.1093/bioinformatics/bts199
Kiełbasa, S. M., Wan, R., Sato, K., Horton, P., and Frith, M. C. (2011). Adaptive seeds tame genomic sequence comparison. Genome Res. 21, 487–493. doi: 10.1101/gr.113985.110
Kirkness, E. F., Haas, B. J., Sun, W., Braig, H. R., Perotti, M. A., Clark, J. M., et al. (2010). Genome sequences of the human body louse and its primary endosymbiont provide insights into the permanent parasitic lifestyle. Proc. Natl. Acad. Sci. U.S.A. 107, 12168–12173. doi: 10.1073/pnas.1003379107
Konstantinidis, K. T., and Tiedje, J. M. (2005). Genomic insights that advance the species definition for prokaryotes. Proc. Natl. Acad. Sci. U.S.A. 102, 2567–2572. doi: 10.1073/pnas.0409727102
Kurtz, S., Phillippy, A., Delcher, A. L., Smoot, M., Shumway, M., Antonescu, C., and Salzberg, S. L. (2004). Versatile and open software for comparing large genomes. Genome Biol. 5:R12. doi: 10.1186/gb-2004-5-2-r12
Lamelas, A., Gosalbes, M. J., Manzano-Marín, A., Peretó, J., Moya, A., and Latorre, A. (2011). Serratia symbiotica from the aphid Cinara cedri: a missing link from facultative to obligate insect endosymbiont. PLoS Genet. 7:e1002357. doi: 10.1371/journal.pgen.1002357
Langmead, B., and Salzberg, S. L. (2012). Fast gapped-read alignment with Bowtie 2. Nat. Methods 9, 357–359. doi: 10.1038/nmeth.1923
Latorre, A., and Manzano-Marín, A. (2016). Dissecting genome reduction and trait loss in insect endosymbionts. Ann. N. Y. Acad. Sci., 1389, 1–23. doi: 10.1111/nyas.13222
Li, L., Stoeckert, C. J., and Roos, D. S. (2003). OrthoMCL: identification of ortholog groups for eukaryotic genomes. Genome Res. 13, 2178–2189. doi: 10.1101/gr.1224503
Luan, J.-B., Chen, W., Hasegawa, D. K., Simmons, A. M., Wintermantel, W. M., Ling, K.-S., et al. (2015). Metabolic coevolution in the bacterial symbiosis of whiteflies and related plant sap-feeding insects. Genome Biol. Evol. 7, 2635–2647. doi: 10.1093/gbe/evv170
Manzano-Marín, A., and Latorre, A. (2014). Settling down: The genome of Serratia symbiotica from the aphid Cinara tujafilina zooms in on the process of accommodation to a cooperative intracellular life. Genome Biol. Evol. 6, 1683–1698. doi: 10.1093/gbe/evu133
Manzano-Marín, A., Oceguera-Figueroa, A., Latorre, A., Jiménez-García, L. F., and Moya, A. (2015). Solving a bloody mess: B-Vitamin independent metabolic convergence among gammaproteobacterial obligate endosymbionts from blood-feeding arthropods and the leech Haementeria officinalis. Genome Biol. Evol. 7, 2871–2884. doi: 10.1093/gbe/evv188
Manzano-Marín, A., Simon, J.-C., and Latorre, A. (2016). Reinventing the wheel and making it round again: evolutionary convergence in Buchnera Serratia symbiotic consortia between the distantly related Lachninae aphids Tuberolachnus salignus and Cinara cedri. Genome Biol. Evol. 8, 1440–1458. doi: 10.1093/gbe/evw085
Manzano-Marín, A., Szabó, G., Simon, J.-C., Horn, M., and Latorre, A. (2017). Happens in the best of subfamilies: establishment and repeated replacements of co-obligate secondary endosymbionts within Lachninae aphids. Environ. Microbiol. 19, 393–408. doi: 10.1111/1462-2920.13633
Mao, M., Yang, X., Poff, K., and Bennett, G. (2017). Comparative genomics of the dual-obligate symbionts from the treehopper, Entylia carinata (Hemiptera: Membracidae), provide insight into the origins and evolution of an ancient symbiosis. Genome Biol. Evol. 9, 1803–1815. doi: 10.1093/gbe/evx134
Marubayashi, J. M., Kliot, A., Yuki, V. A., Rezende, J. A. M., Krause-Sakate, R., Pavan, M. A., et al. (2014). Diversity and localization of bacterial endosymbionts from whitefly species collected in Brazil. PLoS ONE 9:e108363. doi: 10.1371/journal.pone.0108363
Meseguer, A. S., Manzano-Marín, A., Coeur d'Acier, A., Clamens, A. L., Godefroid, M., and Jousselin, E. (2017). Buchnera has changed flatmate but the repeated replacement of co-obligate symbionts is not associated with the ecological expansions of their aphid hosts. Mol. Ecol. 26, 2363–2378. doi: 10.1111/mec.13910
Moriyama, M., Nikoh, N., Hosokawa, T., and Fukatsu, T. (2015). Riboflavin provisioning underlies Wolbachia's fitness contribution to its insect host. MBio 6, e01732-15–e01732-15. doi: 10.1128/mBio.01732-15
Moya, A., Peretó, J., Gil, R., and Latorre, A. (2008). Learning how to live together: genomic insights into prokaryote-animal symbioses. Nat. Rev. Genet. 9, 218–229. doi: 10.1038/nrg2319
Nakabachi, A., and Ishikawa, H. (1999). Provision of riboflavin to the host aphid, Acyrthosiphon pisum, by endosymbiotic bacteria, Buchnera. J. Insect. Physiol. 45, 1–6. doi: 10.1016/S0022-1910(98)00104-8
Nawrocki, E. P. (2009). Structural RNA Homology Search and Alignment Using Covariance Models, Electronic theses and dissertations, Washington, DC: University School of Medicine, 256.
Nguyen, L.-T., Schmidt, H. A., von Haeseler, A., and Minh, B. Q. (2015). IQ-TREE: A fast and effective stochastic algorithm for estimating maximum-likelihood phylogenies. Mol. Biol. Evol. 32, 268–274. doi: 10.1093/molbev/msu300
Nováková, E., Husník, F., Šochová, E., and Hypša, V. (2015). Arsenophonus and Sodalis symbionts in louse flies: An analogy to the Wigglesworthia and Sodalis system in tsetse flies. Appl. Environ. Microbiol. 81, 6189–6199. doi: 10.1128/AEM.01487-15
Nováková, E., Hypsa, V., and Moran, N. a. (2009). Arsenophonus, an emerging clade of intracellular symbionts with a broad host distribution. BMC Microbiol 9:143. doi: 10.1186/1471-2180-9-143
Nováková, E., Hypša, V., Nguyen, P., Husník, F., and Darby, A. C. (2016). Genome sequence of Candidatus Arsenophonus lipopteni, the exclusive symbiont of a blood sucking fly Lipoptena cervi (Diptera: Hippoboscidae). Stand Genomic Sci. 11, 72. doi: 10.1186/s40793-016-0195-1
Nurk, S., Meleshko, D., Korobeynikov, A., and Pevzner, P. A. (2017). metaSPAdes: a new versatile metagenomic assembler. Genome Res. 27, 824–834. doi: 10.1101/gr.213959.116
Opatovsky, I., Santos-Garcia, D., Ruan, Z., Lahav, T., Ofaim, S., Mouton, L., et al. (2018). Modeling trophic dependencies and exchanges among insects' bacterial symbionts in a host-simulated environment. BMC Genomics 19:402. doi: 10.1186/s12864-018-4786-7
Ouvrard, D., and Martin, J. H. (2018). The White-files: Taxonomic Checklist of the World's Whiteflies (Insecta: Hemiptera: Aleyrodidae). Available online at: http://www.hemiptera-databases.org/whiteflies/ (Accessed September 12, 2018).
Pandey, N., and Rajagopal, R. (2015). Molecular characterization and diversity analysis of bacterial communities associated with Dialeurolonga malleswaramensis (Hemiptera: Aleyrodidae) adults using 16S rDNA amplicon pyrosequencing and FISH. Insect Sci. 23, 1–8. doi: 10.1111/1744-7917.12220
Price, D. R. G., and Wilson, A. C. C. (2014). A substrate ambiguous enzyme facilitates genome reduction in an intracellular symbiont. BMC Biol. 12:110. doi: 10.1186/s12915-014-0110-4
R Core Team (2018). R: A Language and Environment for Statistical Computing. R Foundation for Statistical Computing, Vienna.
Rao, Q., Rollat-Farnier, P.-A., Zhu, D.-T., Santos-Garcia, D., Silva, F. J., Moya, A., et al. (2015). Genome reduction and potential metabolic complementation of the dual endosymbionts in the whitefly Bemisia tabaci. BMC Genomics 16:226. doi: 10.1186/s12864-015-1379-6
Rodriguez-R, L. M., and Konstantinidis, K. T. (2016). The enveomics collection : a toolbox for specialized analyses of microbial genomes and metagenomes. Peer J. Preprints 4:e1900v1. doi: 10.7287/peerj.preprints.1900v1
Russell, J. A., Oliver, K. M., and Hansen, A. K. (2017). Band-aids for Buchnera and B vitamins for all. Mol. Ecol. 26, 2199–2203. doi: 10.1111/mec.14047
Santos-Garcia, D., Farnier, P.-A., Beitia, F., Zchori-Fein, E., Vavre, F., Mouton, L., et al. (2012). Complete genome sequence of "Candidatus Portiera aleyrodidarum" BT-QVLC, an obligate symbiont that supplies amino acids and carotenoids to Bemisia tabaci. J. Bacteriol. 194, 6654–6655. doi: 10.1128/JB.01793-12
Santos-Garcia, D., Latorre, A., Moya, A., Gibbs, G., Hartung, V., Dettner, K., et al. (2014a). Small but powerful, the primary endosymbiont of moss bugs, Candidatus Evansia muelleri, holds a reduced genome with large biosynthetic capabilities. Genome Biol. Evol. 6, 1875–1893. doi: 10.1093/gbe/evu149
Santos-Garcia, D., Rollat-Farnier, P.-A., Beitia, F., Zchori-Fein, E., Vavre, F., Mouton, L., et al. (2014b). The genome of Cardinium cBtQ1 provides insights into genome reduction, symbiont motility, and its settlement in Bemisia tabaci. Genome Biol. Evol. 6, 1013–1030. doi: 10.1093/gbe/evu077
Santos-Garcia, D., Silva, F. J., Morin, S., Dettner, K., and Kuechler, S. M. (2017). The all-rounder Sodalis-a new bacteriome-associated endosymbiont of the lygaeoid bug Henestaris halophilus (Heteroptera: Henestarinae) and a critical examination of its evolution. Genome Biol. Evol. 9, 1–54. doi: 10.1093/gbe/evx202
Santos-Garcia, D., Vargas-Chavez, C., Moya, A., Latorre, A., and Silva, F. J. (2015). Genome evolution in the primary endosymbiont of whiteflies sheds light on their divergence. Genome Biol. Evol. 7, 873–888. doi: 10.1093/gbe/evv038
Seemann, T. (2014). Prokka: rapid prokaryotic genome annotation. Bioinformatics 30, 2068–2069. doi: 10.1093/bioinformatics/btu153
Segata, N., Börnigen, D., Morgan, X. C., and Huttenhower, C. (2013). PhyloPhlAn is a new method for improved phylogenetic and taxonomic placement of microbes. Nat. Commun. 4:2304. doi: 10.1038/ncomms3304
Shcherbakov, D. E. (2000). The most primitive whiteflies (Hemiptera; Aleyrodidae; Bernaeinae subfam. nov.) from the Mesozoic of Asia and Burmese amber, with an overview of Burmese amber hemipterans. Bull. Nat. Hist. Mus. Lond. 56, 29–37.
Skaljac, M., Zanic, K., Ban, S. G., Kontsedalov, S., and Ghanim, M. (2010). Co-infection and localization of secondary symbionts in two whitefly species. BMC Microbiol. 10:142. doi: 10.1186/1471-2180-10-142
Skaljac, M., Zanić, K., Hrnčić, S., Radonjić, S., Perović, T., and Ghanim, M. (2013). Diversity and localization of bacterial symbionts in three whitefly species (Hemiptera: Aleyrodidae) from the east coast of the Adriatic Sea. Bull. Entomol. Res. 103, 48–59. doi: 10.1017/S0007485312000399
Slater, G. S. C., and Birney, E. (2005). Automated generation of heuristics for biological sequence comparison. BMC Bioinformatics 6, 1–11. doi: 10.1186/1471-2105-6-31
Sloan, D. B., and Moran, N. A. (2012a). Endosymbiotic bacteria as a source of carotenoids in whiteflies. Biol. Lett. 8, 986–989. doi: 10.1098/rsbl.2012.0664
Sloan, D. B., and Moran, N. a. (2012b). Genome reduction and co-evolution between the primary and secondary bacterial symbionts of psyllids. Mol. Biol. Evol. 29, 3781–3792. doi: 10.1093/molbev/mss180
Sloan, D. B., Nakabachi, A., Richards, S., Qu, J., Murali, S. C., Gibbs, R. A., et al. (2014). Parallel histories of horizontal gene transfer facilitated extreme reduction of endosymbiont genomes in sap-feeding insects. Mol. Biol. Evol. 31, 857–871. doi: 10.1093/molbev/msu004
Šochová, E., Husník, F., Nováková, E., Halajian, A., and Hypša, V. (2017). Arsenophonus and Sodalis replacements shape evolution of symbiosis in louse flies. PeerJ 5:e4099. doi: 10.7717/peerj.4099
Staden, R., Beal, K. F., and Bonfield, J. K. (2000). The Staden package, 1998. Methods Mol. Biol. 132, 115–310. doi: 10.1385/1-59259-192-2:115
Sudakaran, S., Kost, C., and Kaltenpoth, M. (2017). Symbiont acquisition and replacement as a source of ecological innovation. Trends Microbiol. 24, 932–976. doi: 10.1016/j.tim.2017.02.014
Thao, M. L., Baumann, L., Hess, J. M., Falk, B. W., Ng, J. C. K., Gullan, P. J., et al. (2003). Phylogenetic evidence for two new insect-associated Chlamydia of the family Simkaniaceae. Curr. Microbiol. 47, 46–50. doi: 10.1007/s00284-002-3953-9
Thao, M. L., and Baumann, P. (2004). Evidence for multiple acquisition of Arsenophonus by whitefly species (Sternorrhyncha: Aleyrodidae). Curr. Microbiol. 48, 140–144. doi: 10.1007/s00284-003-4157-7
Varani, A. M., Siguier, P., Gourbeyre, E., Charneau, V., and Chandler, M. (2011). ISsaga is an ensemble of web-based methods for high throughput identification and semi-automatic annotation of insertion sequences in prokaryotic genomes. Genome Biol. 12:R30. doi: 10.1186/gb-2011-12-3-r30
Walker, B. J., Abeel, T., Shea, T., Priest, M., Abouelliel, A., Sakthikumar, S., et al. (2014). Pilon: an integrated tool for comprehensive microbial variant detection and genome assembly improvement. PLoS ONE 9:e112963. doi: 10.1371/journal.pone.0112963
Wood, D. E., and Salzberg, S. L. (2014). Kraken: ultrafast metagenomic sequence classification using exact alignments. Genome Biol. 15:R46. doi: 10.1186/gb-2014-15-3-r46
Xue, J., Zhou, X., Zhang, C.-X., Yu, L.-L., Fan, H.-W., Wang, Z., et al. (2014). Genomes of the rice pest brown planthopper and its endosymbionts reveal complex complementary contributions for host adaptation. Genome Biol. 15, 521. doi: 10.1186/s13059-014-0521-0
Zchori-Fein, E., Lahav, T., and Freilich, S. (2014). Variations in the identity and complexity of endosymbiont comations in whitefly hosts. Front. Microbiol. 5:310.
Keywords: whitefly, symbiosis, vitamins, genome reduction, Arsenophonus, Wolbachia, metabolic complementation, riboflavin
Citation: Santos-Garcia D, Juravel K, Freilich S, Zchori-Fein E, Latorre A, Moya A, Morin S and Silva FJ (2018) To B or Not to B: Comparative Genomics Suggests Arsenophonus as a Source of B Vitamins in Whiteflies. Front. Microbiol. 9:2254. doi: 10.3389/fmicb.2018.02254
Received: 23 May 2018; Accepted: 04 September 2018;
Published: 25 September 2018.
Edited by:
Martin G. Klotz, Washington State University Tri-Cities, United StatesReviewed by:
David Baltrus, University of Arizona, United StatesPiotr Lukasik, University of Montana, United States
Copyright © 2018 Santos-Garcia, Juravel, Freilich, Zchori-Fein, Latorre, Moya, Morin and Silva. This is an open-access article distributed under the terms of the Creative Commons Attribution License (CC BY). The use, distribution or reproduction in other forums is permitted, provided the original author(s) and the copyright owner(s) are credited and that the original publication in this journal is cited, in accordance with accepted academic practice. No use, distribution or reproduction is permitted which does not comply with these terms.
*Correspondence: Diego Santos-Garcia, ZGllZ28uc2FudG9zQG1haWwuaHVqaS5hYy5pbA==
Francisco J. Silva, ZnJhbmNpc2NvLnNpbHZhQHV2LmVz
†These authors have contributed equally to this work