- 1Centre for Ecology and Evolution in Microbial Model Systems, Linnaeus University, Kalmar, Sweden
- 2Department of Biology and Environmental Science, Linnaeus University, Kalmar, Sweden
- 3Limnology and Science for Life Laboratory, Department of Ecology and Genetics, Uppsala University, Uppsala, Sweden
The continental deep biosphere is suggested to contain a substantial fraction of the earth’s total biomass and microorganisms inhabiting this environment likely have a substantial impact on biogeochemical cycles. However, the deep microbial community is still largely unknown and can be influenced by parameters such as temperature, pressure, water residence times, and chemistry of the waters. In this study, 21 boreholes representing a range of deep continental groundwaters from the Äspö Hard Rock Laboratory were subjected to high-throughput 16S rRNA gene sequencing to characterize how the different water types influence the microbial communities. Geochemical parameters showed the stability of the waters and allowed their classification into three groups. These were (i) waters influenced by infiltration from the Baltic Sea with a “modern marine (MM)” signature, (ii) a “thoroughly mixed (TM)” water containing groundwaters of several origins, and (iii) deep “old saline (OS)” waters. Decreasing microbial cell numbers positively correlated with depth. In addition, there was a stronger positive correlation between increased cell numbers and dissolved organic carbon for the MM compared to the OS waters. This supported that the MM waters depend on organic carbon infiltration from the Baltic Sea while the ancient saline waters were fed by “geogases” such as carbon dioxide and hydrogen. The 16S rRNA gene relative abundance of the studied groundwaters revealed different microbial community compositions. Interestingly, the TM water showed the highest dissimilarity compared to the other two water types, potentially due to the several contrasting water types contributing to this groundwater. The main identified microbial phyla in the groundwaters were Gammaproteobacteria, unclassified sequences, Campylobacterota (formerly Epsilonproteobacteria), Patescibacteria, Deltaproteobacteria, and Alphaproteobacteria. Many of these taxa are suggested to mediate ferric iron and nitrate reduction, especially in the MM waters. This indicated that nitrate reduction may be a neglected but important process in the deep continental biosphere. In addition to the high number of unclassified sequences, almost 50% of the identified phyla were archaeal or bacterial candidate phyla. The percentage of unknown and candidate phyla increased with depth, pointing to the importance and necessity of further studies to characterize deep biosphere microbial populations.
Introduction
Despite being isolated from the photosynthetic fueled surface by time and distance, the continental subsurface is estimated to contain up to 19% of the earth’s total biomass (McMahon and Parnell, 2014). This vast subsurface community is comprised of all three domains of life plus viruses (reviewed in Itavaara et al., 2016), is at least partially active (Schippers et al., 2005; Eydal et al., 2009; Morono et al., 2011; Orsi et al., 2013), and affects global nutrient and energy dynamics including the carbon (Pedersen and Ekendahl, 1992), sulfur (Pedersen et al., 2014), and nitrogen (Nielsen et al., 2006) cycles. In addition, the activity of microorganisms inhabiting the continental deep biosphere is important for, e.g., long-term disposal of nuclear waste where sulfate reduction to sulfide can result in the corrosion of the copper canisters used to store spent fuel (Standish et al., 2016).
Life is always dependent on the presence of water but deep continental microbial communities are also constrained by boundaries including the size of the water filled rock fractures, physical parameters such as temperature and pressure that increase with depth from the surface, and the chemical constituents of the waters such as electron donors and acceptors for microbial energy conservation. Waters present in deep hard bedrock fractures are typically oligotrophic and the amount of dissolved organic carbon (DOC) decreases with the degree of separation from the surface (Magnabosco et al., 2016). This results in higher microbial diversities in waters with more rapid replenishment that are supported by organic carbon compared to older, more isolated waters where the microbial community is maintained by carbon dioxide and hydrogen of geological origin (Purkamo et al., 2015; Wu et al., 2015; Hubalek et al., 2016; Kieft et al., 2018; Lopez-Fernandez et al., 2018b). Due to the difficulty in accessing the deep continental biosphere, many studies are carried out in mines (e.g., Pfiffner et al., 2006) or boreholes (e.g., Itavaara et al., 2011), providing access to waters with a very similar geographical proximity and only a single or minimal range of origins. This results in a limited and likely incomplete picture of the true microbiological diversity in the continental deep sub-surface being presented.
One site that circumvents the problem of accessing groundwaters with a range of characteristics is the Äspö Hard Rock Laboratory (Äspö HRL) situated on the Baltic Sea coast in southeast Sweden. This underground laboratory consists of a 3.6 km long tunnel, partially extending under the Baltic Sea, which descends to a depth of 460 m below sea level (mbsl). The Äspö HRL is built in Paleoproterozoic granitoids, which contain an abundance of mainly vertical to sub-vertical fractures and fracture zones where water of different sources and ages flows (Drake et al., 2012). These aquifers include: old saline (OS) water that resides in and flows up from deep parts of the bedrock system; brackish water that has penetrated from the overlying Baltic Sea and its predecessors (e.g., the Littorina Sea); and fresh waters introduced via rain, melting snow, and retreating glaciers in the Pleistocene (Laaksoharju et al., 2008a; Mathurin et al., 2012, 2014a). Besides providing access to multiple water types, the Äspö HRL alleviates many problems associated with contamination of deep biosphere microbiological studies as the tunnel was constructed over 30 years ago and the groundwaters continually flow toward the tunnel by hydrostatic pressure (as opposed to pumping). However, how the geochemistry of the different groundwaters demarcates the deep biosphere prokaryote diversity, richness, and metabolic strategies has not been evaluated in such a large area.
Many previous investigations have described Äspö HRL microbial communities, including cultivation-based approaches (Pedersen, 2013), ATP analysis (Eydal and Pedersen, 2007), high-throughput 16S rRNA gene inventories (Hubalek et al., 2016), and viability studies (Lopez-Fernandez et al., 2018a), as well as metagenome-based reconstructions of planktonic (Wu et al., 2015) and biofilm (Wu et al., 2017) communities. In addition, a recent metatranscriptome-based study revealed that the three domains of life are active in the deep continental biosphere (Lopez-Fernandez et al., 2018b). These studies highlight a broad range of metabolic strategies including nitrate, sulfate, and sulfur reduction along with simple fermentative microbes with a very small cell size that readily pass through a 0.22 μm membrane filter that is typically used for cell capture (Wu et al., 2015). These past findings emphasize the need to carry out a systematic and broader survey of the microbial communities in all of the different Äspö HRL water types.
In this study, we have sampled 21 boreholes representing a broad range of groundwaters dominated by meteoric, Baltic Sea, and OS origins along with mixtures thereof. The sampled boreholes intersect groundwaters with an approximate underground area of 300 m × 300 m and a depth down to 1000 m making this the first large-scale three-dimensional microbiological investigation of the deep biosphere. Statistical correlations between chemical characteristics and the microbial communities based on high-throughput sequencing of the 16S rRNA gene were used to investigate how the communities are influenced by the different water types.
Materials and Methods
Sampling Site and Boreholes
The Swedish Nuclear Fuel and Waste Management Company (SKB)-operated Äspö HRL is situated on the Baltic Sea coast (Lat N 57° 26′ 4′′ Lon E 16° 39′ 36′′). Twenty-one boreholes (Table 1) were sampled that extend outward from the tunnel walls to cover an approximate total area of 300 × 300 × 1000 m (Figure 1).
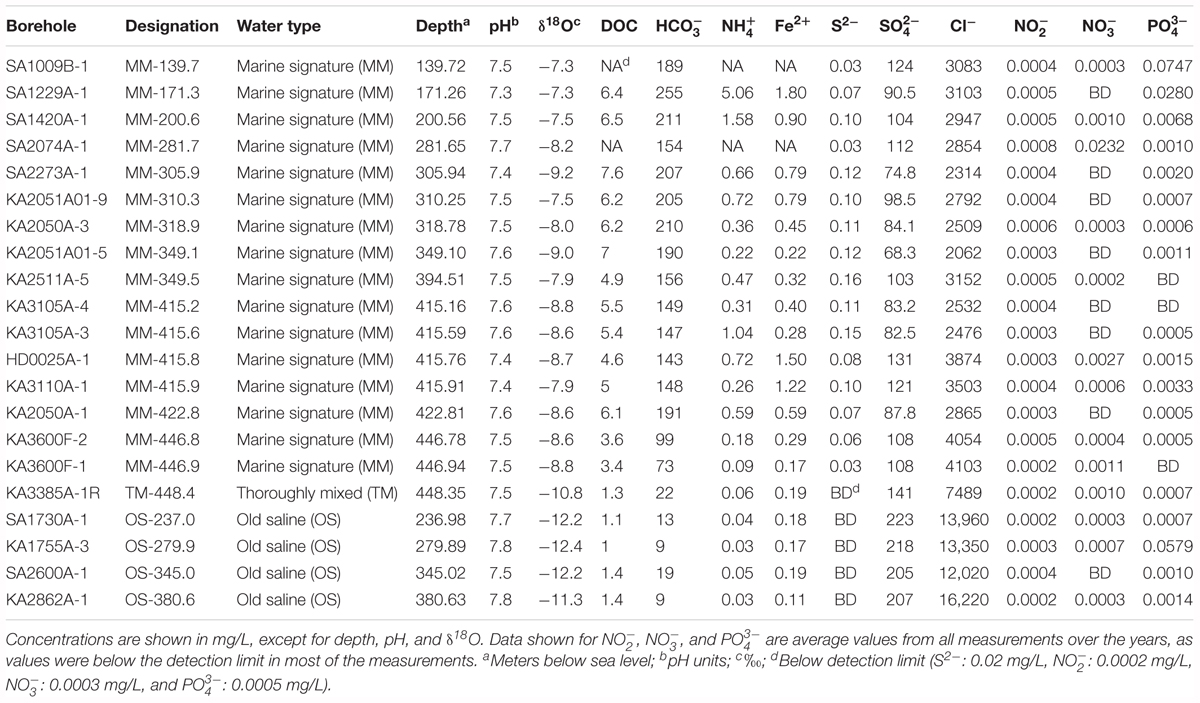
TABLE 1. Type and chemical composition of the sampled groundwaters in May 2016 (November 2015 only for SA2074A-1).
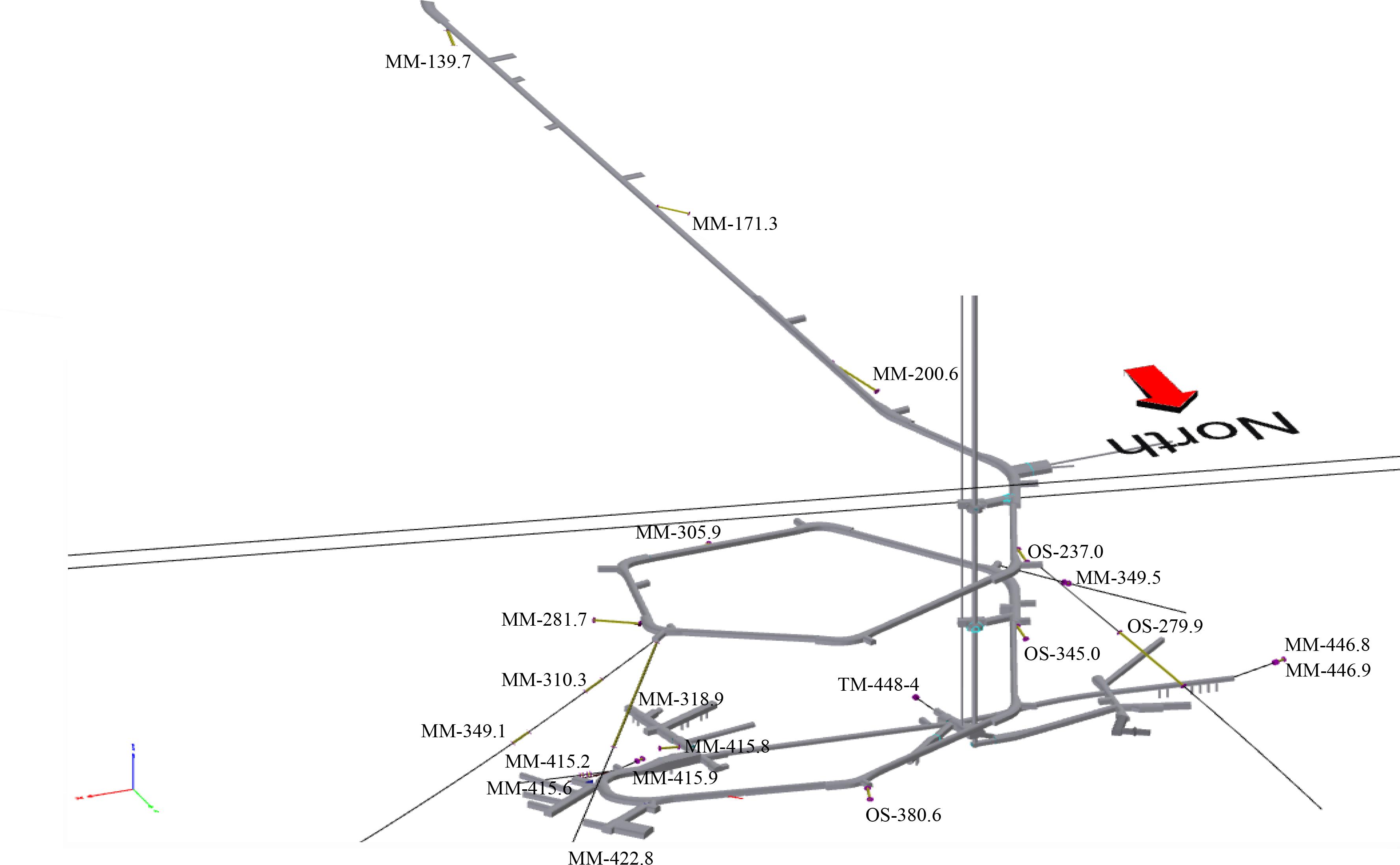
FIGURE 1. Three-dimensional model of the 21 sampled boreholes showing their position along the Äspö HRL tunnel, depth below sea level, and the water types contained therein. The borehole designations (MM, modern marine; TM, thoroughly mixed; and OS, old saline) refer to those given in Table 1.
Geochemical Analyses
The sampled boreholes were included in SKB’s geochemical monitoring of groundwaters at the Äspö HRL site, performed as described in Almén et al. (1986). In most cases, the monitoring started in the 1990s and has been carried out with variable frequency both within and between the boreholes. The rigorously quality controlled chemical and isotopic variables data were retrieved from SKB’s Sicada database (Data delivery SKBdata_17_061). These variables included (references are to analytical techniques and precision): Cl- and δ18O that represent 18O/16O relative to Standard Mean Ocean Water (Mathurin et al., 2012); Ca, Mg, and (Mathurin et al., 2014b); Fe2+, DOC, and S- (Alakangas et al., 2014); and (Rönnback and Åström, 2007); and Mn, , , and (McMahon and Parnell, 2014). The extracted data are from three dates: (i) May 2016, which was as close as possible to the microbial sampling; (ii) May 2013, in order to assess water-quality stability in the short term; and (iii) the first sampling event in each borehole (in most cases in the 1990s) in order to assess long-term water-quality stability. One-way ANOVA post hoc test was used to look for significant differences (p < 0.05) in the chemical parameters between the three different water types. For this comparison, values from the last measurement were used except for nitrate, nitrite, and phosphate where an average of all measurements collected through the years was used as most measurements were close to or below the detection limit.
Cell Numbers, DNA Content, and Size Distribution
To characterize the cell density, DNA content per cell, and cell size distribution, groundwaters were collected at the same time as for microbiological sampling. Triplicates of 1 mL of each of the 21 borehole section groundwaters were collected, fixed with 1% paraformaldehyde for 15 min at 4°C, then 5 mM EDTA and 2 g/L NaCl added, and the samples flash-frozen in liquid nitrogen. Triplicate samples were analyzed by flow cytometry after staining cells with the fluorescent dye Syto 13 (Molecular Probes, Invitrogen, Carlsbad, CA, United States) according to Giorgio et al. (1996). A flow cytometer equipped with a 488-nm blue solid-state laser (Cyflow Space, Partec, Görlitz, Germany) was used to determine cell counts, DNA content per cell, and size distribution. A volume of 50 μL was counted and green fluorescence was used as a trigger during the measurement. The flow cytometry counts were analyzed using Flowing Software version 2.5 (Perttu Terho, Center for Biotechnology, Turku, Finland). Median forward scatter was used as a proxy for average bacterial cell size and DNA content in individual cell samples (Bertilsson et al., 2003). This measurement does not provide information on absolute size without additional calibration, but allows the detection of relative differences in the cellular properties between the different samples. Coefficient of variation was calculated as the standard deviation of the replicates divided by the average. One-way ANOVA test was used to identify significant differences in cell numbers, DNA content per cell, and size distribution (p < 0.05) between the water types. Pearson bivariate correlation test was used to identify the correlation between cell numbers and DOC.
Microbiological Sampling, DNA Extraction, Sequencing, and Bioinformatics
Groundwater sampling was carried out between February and May 2017. Before sampling, five section volumes of groundwater were discarded to avoid contamination from the stagnant water in the borehole pipe. Then, a high pressure, aluminum filter holder (Millipore) with a downstream valve and pressure gauge was directly connected to the borehole. Cell capture (in biological triplicates) was on a single sterile 0.1 μm pore size polyvinylidene fluoride (PVDF), hydrophilic, 25 or 47 mm Durapore Membrane filter (Merck Millipore) per replicate. The filters were aseptically removed from the holders, placed in cryotubes, and immediately frozen in liquid nitrogen for transport to the laboratory where they were stored at -80°C until further processing. The filtered volumes of groundwater per triplicate are shown in Supplementary Table S1. DNA extraction was performed using the MO BIO PowerWater DNA isolation kit following the manufacturer’s instructions except that the final DNA was re-suspended in 50 μL of eluent. The extracted DNA was analyzed by gel electrophoresis and a Qubit 2.0 Fluorometer (Life Technologies) and stored at -20°C until further processing. Then, the V3–V4 region of the 16S rRNA gene was amplified by a two-step PCR using bacterial primers 341F and 805R (Herlemann et al., 2011) and following the PCR protocol described in Hugerth et al. (2014) and followed by high-throughout sequencing at the Science for Life Laboratory, Sweden1 on the Illumina MiSeq platform (2 × 300 bp pair-end reads). Finally, bioinformatic analyses were carried out as previously described (Lopez-Fernandez et al., 2018a). The number of pair-end reads received from the sequencing facility, merged, and quality trimmed reads, and amount of Operational Taxonomic Units (OTUs) clustered can be found in Supplementary Table S1. Final count values were normalized by relative abundance (i.e., % of total sample size) since it has been found to be more accurate than data rarefaction (McMurdie and Holmes, 2013). After normalization of counts, a one-way ANOVA post hoc test was used to (1) test the reproducibility of the biological triplicates per borehole section based on the 16S rRNA relative abundance of phyla and Proteobacteria classes (excluding former Epsilonproteobacteria; p < 0.05) in the three water types; (2) look for significant differences in 16S rRNA relative phyla and Proteobacteria classes abundance (p < 0.05) between the different water types; and (3) find significant differences for 16S rRNA gene relative classified OTUs abundance (p < 0.05) in the three different water types. Alpha diversity (Shannon H index) and Beta diversity (Bray–Curtis dissimilarity) were calculated based on rarefied data to the lowest sample size (9888 counts) and bootstrapped 100 times. Then, a one-way ANOVA test was used to look for significant differences in alpha diversity (p < 0.05) between the 21 different borehole waters. Finally, canonical correspondence analysis (CCA) was performed in PAST 3.17 (Hammer et al., 2001) to visualize the influence of the chemical parameters within the groundwaters and the differences in relative phyla abundance between the different groundwaters.
Results and Discussion
Geochemical Parameters of the Investigated Groundwaters
A time series of chemical parameters showing the stability of the sampled waters is shown in Figure 2 and Supplementary Table S2, while a summary of the geochemical parameters of the different groundwaters is given in Table 1. For 16 groundwaters, the recent (i.e., from 2013 to 2016) chloride concentrations and δ18O values were stable and ranged in total from 2000 to 4500 mg/L and from -7 to -10‰ vs. SMOW, respectively. These values are similar to the corresponding values in the Baltic Sea (3380 mg/L and -6‰ vs. SMOW) reported by Mathurin et al. (2012). Consequently, these groundwaters have a marine signature and are thus most likely composed of Baltic Sea water mixed with minor proportions of meteoric water and/or older more saline water residing in the bedrock fractures (Laaksoharju et al., 2008b). This interpretation was consistent with that the sampled bedrock volume was located under the archipelago on the western shores of the Baltic Sea and is characterized by dominantly sub-vertical fractures that allow for recharge of the overlying marine water (Mathurin et al., 2014b). However, the majority of these 16 groundwaters had higher chloride concentrations during the first sampling event (Figure 2 and Supplementary Table S2). This feature is ascribed to effects caused by the construction of the Äspö HRL tunnel in the 1990s. During the pristine conditions before tunnel construction, many of the fractures in contact with the overlying Baltic Sea were dominated by sea water intrusion during earlier stages of the Baltic Sea when the chloride concentrations were up to 6500 mg/L (Mathurin et al., 2012). After the tunnel construction, water flowing into and pumped away from the tunnel allowed for more efficient marine-water recharge leading to replacement of the ancient more saline waters with current Baltic Sea water (Mathurin et al., 2012). In consequence, at the time of sampling for microbiological analyses much of these groundwaters had recently intruded into the fracture network (within years or decades) and to some extent mixed with the equally young fresh (meteoric) water or older more saline waters previously occupying the fractures and therefore were named modern marine (MM) waters. Four of the groundwaters had considerably higher chloride concentrations and lower δ18O values (Figure 2 and Table 1). These groundwaters represent saline water that typically resides deep in fractured bedrock and are of up to millions of years old (Laaksoharju et al., 2008b), so they were termed OS waters. In contrast to the MM waters, these OS groundwaters increased in salinity since the first sampling (Figure 2). This has been explained by up-flow of very saline deep-lying water as a result of the abundant water discharge via the Äspö HRL tunnel (Laaksoharju et al., 1999). The remaining groundwater had chloride concentrations and δ18O values in-between the groundwaters with marine signature and the saline groundwaters (Figure 2 and Table 1). This groundwater is thus classified as thoroughly mixed (TM) and is composed of unknown proportions of two or more water types such that the age of this groundwater cannot be assessed.
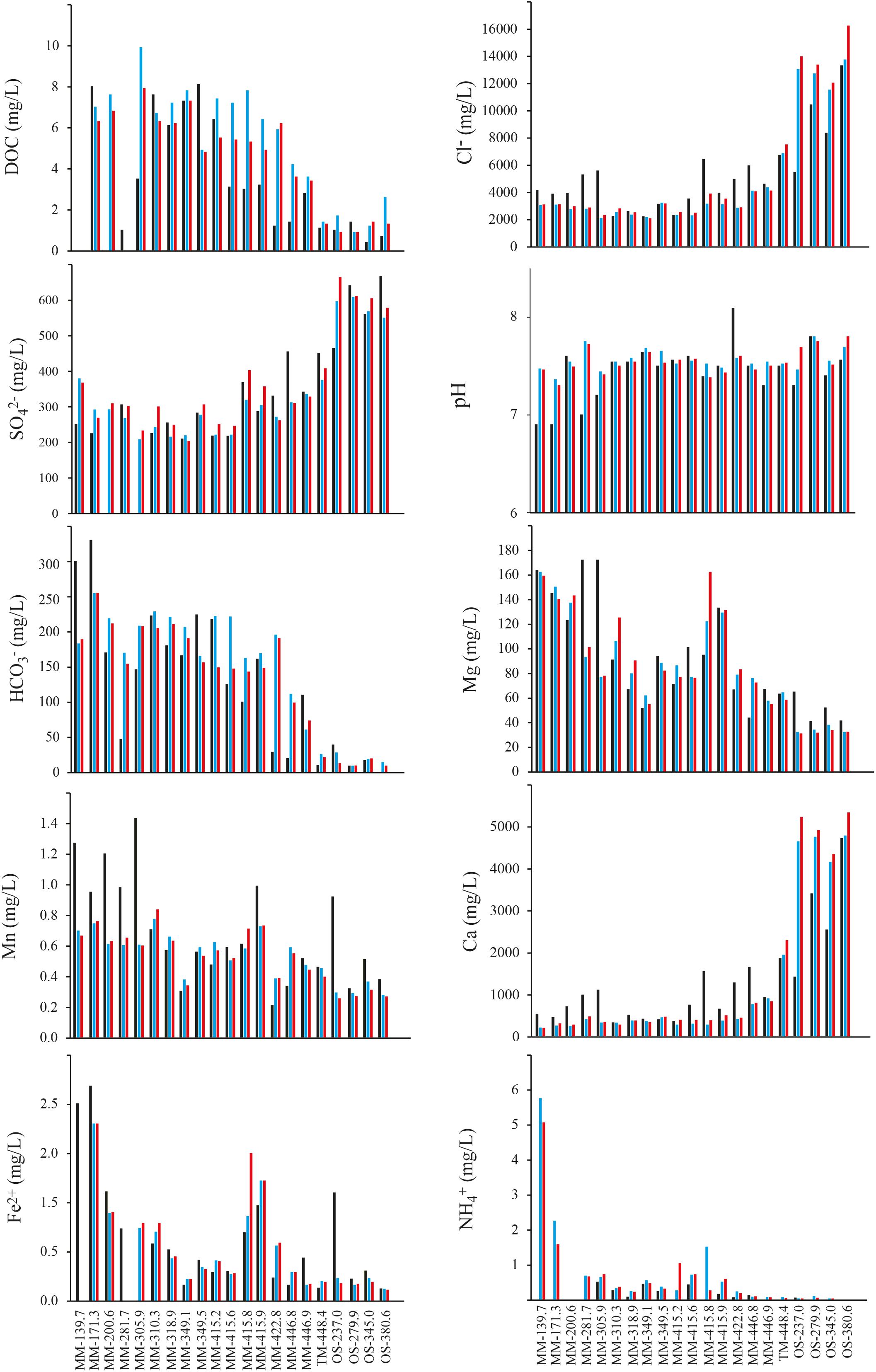
FIGURE 2. (Geo)chemical data for the 21 borehole sections. The black bar shows the first sampling occasion in the section (typically early/mid 1990s), the blue bar gives the value measured in approximately May 2013, and the red bar was measured in May 2016.
The other determined chemical variables are non-conservative and can thus participate in various biogeochemical reactions within the fracture network, including reactions between the water and the fracture walls typically covered with secondary minerals such as calcite, clay minerals, and pyrite (Drake et al., 2013). Despite this, the concentrations of many of them were to a large extent controlled by water source and subsequent water-mixing processes within the fracture network (Gomez et al., 2014). Sulfate and calcium closely matched the chloride concentration and are much higher in the OS groundwaters (Figure 2). In contrast, magnesium, bicarbonate, DOC, and ammonium are higher in marine water than deep saline waters and consequently were higher in the MM groundwaters (Table 1 and Supplementary Table S2). A similar trend was seen for sulfide, which was below the detection limit (0.02 mg/L) in the OS groundwaters but was present in the MM waters at up to nearly 0.2 mg/L. Manganese and ferrous iron varied considerably among the boreholes but were not consistently different between the OS and MM groups (Table 1 and Supplementary Table S2). Finally, nitrite, nitrate, and phosphate occurred in very low concentrations at below (or very close to) the detection limit (Table 1) in the three different water types.
Almost all the tested chemical parameters were significantly different in the three groundwater types (MM, TM, and OS), as shown by the one-way ANOVA test (Supplementary Table S3). For example, Fe2+, DOC, S2-, and were significantly higher in the MM waters compared to the TM and OS boreholes (Supplementary Table S4). In addition, the concentrations of Cl- and were significantly higher in the OS waters compared to those in the MM and TM water types while in turn the values in the TM water were significantly higher compared to the MM waters. Moreover, the values were significantly higher only in the TM water. Finally, was significantly different in all waters with highest values in the MM waters (Supplementary Table S4).
Cell Numbers, DNA Content, and Size Distributions
Cell counts, size distribution, and DNA content per cell are shown in Table 2 and Supplementary Figure S1. However, although cell size and cellular DNA content values were measured, they were close to the detection limit of the flow cytometer and both parameters feature high coefficient of variation. Significant differences were found in cell counts and size within the 21 borehole sections, but not in average cellular DNA content (p = 0.000, 0.004, and 0.100, respectively; n = 21). The flow cytometry analysis showed very low cell numbers compared to marine subsurface sediments with a range of 104–108 cells cm-3 (Morono et al., 2013), continental subsurface counts nearing 107 cells cm-3 at depths down to 500 m below the surface (McMahon and Parnell, 2014), and sub-seafloor sediments (Kallmeyer et al., 2012). The data also revealed a significant positive correlation between decreasing cell numbers and depth (Pearson correlation = 0.50, p = 0.021, n = 21; Figure 3A). This has also been observed in a meta-study of over 100 continental deep biosphere cell density measurements (McMahon and Parnell, 2014). Interestingly, one exception to the trend was groundwater MM-422.81 that had higher cell numbers as well as an increased DOC content (Table 2 and Supplementary Figure S1) that again supports that the microbial cell numbers were linked to depth and DOC content.
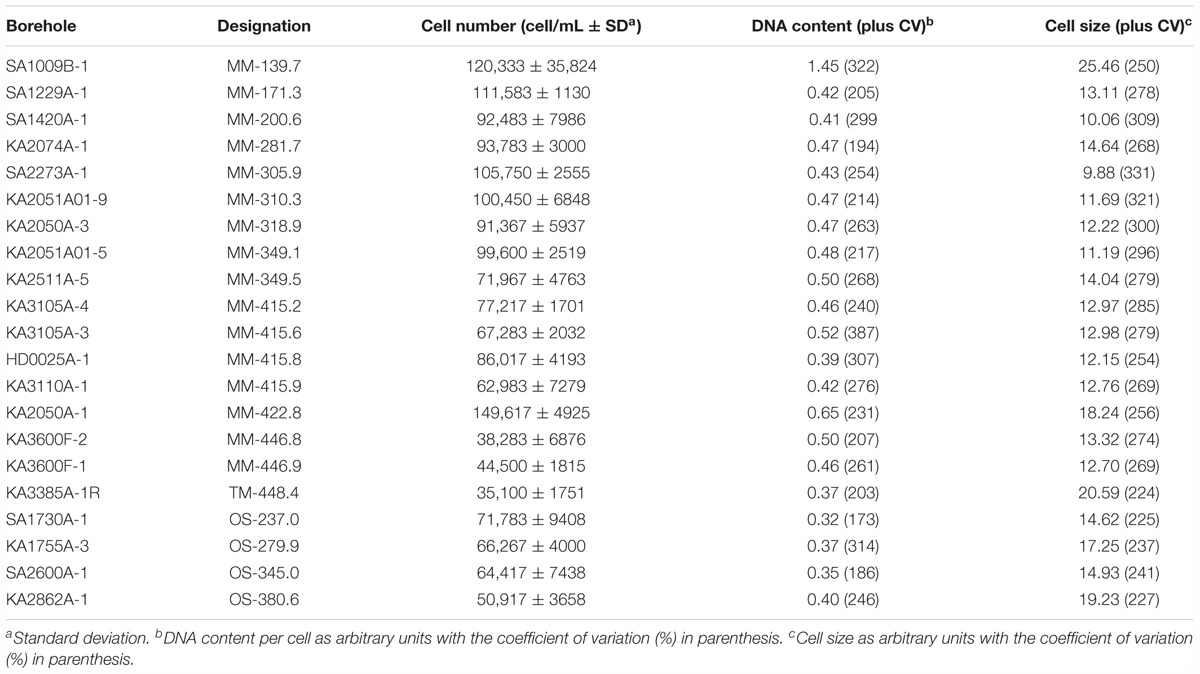
TABLE 2. Average cell counts, DNA content per cell, and size distribution of the 21 sampled groundwaters measured by flow cytometry.
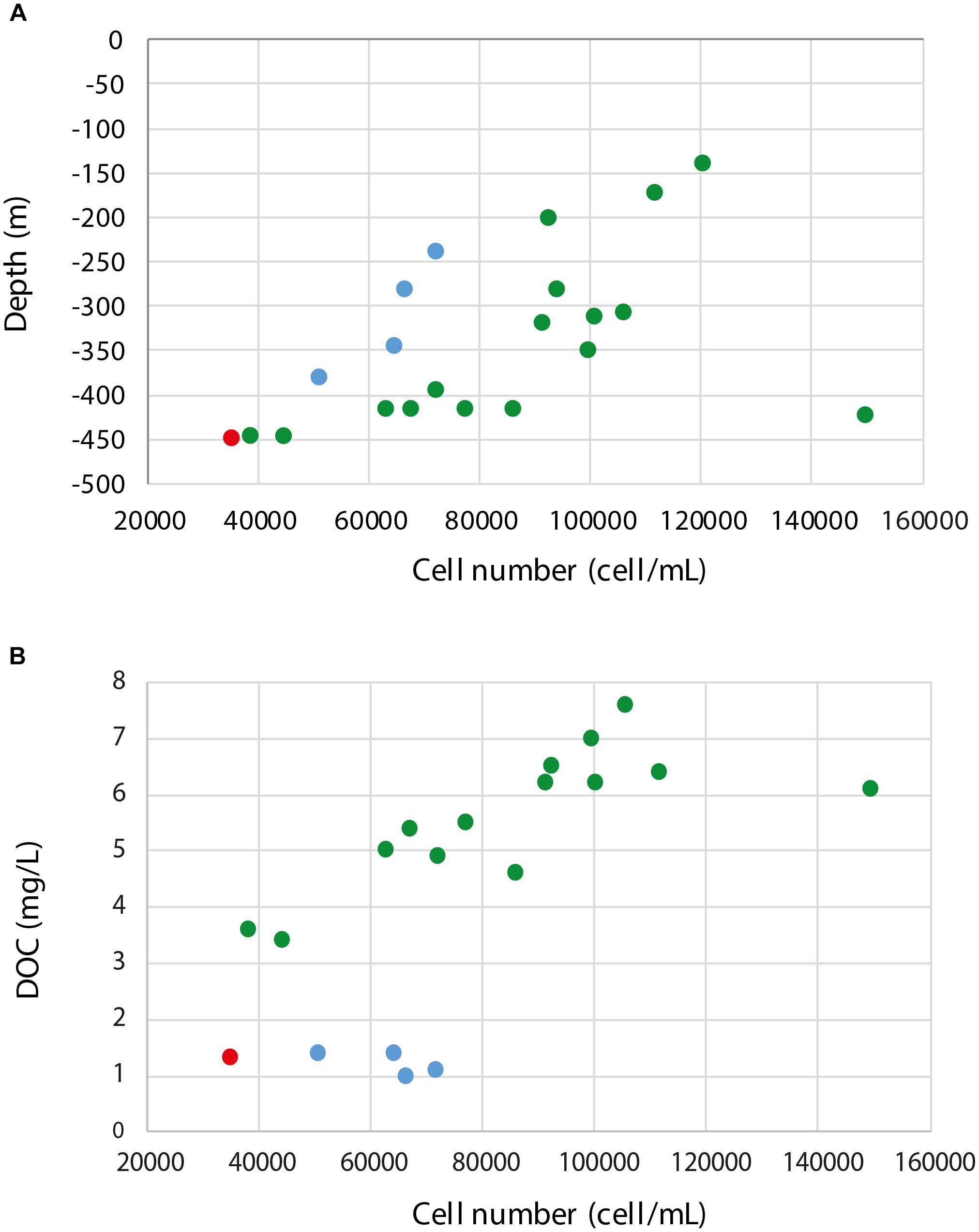
FIGURE 3. Pearson correlation plots (A) between the cell numbers and depth and (B) cell numbers and DOC (boreholes MM-139.7 and MM-281.7 have been omitted as no data for DOC were available) of the sampled borehole sections. MM waters are colored in green, TM in red, and OS in blue.
Flow cytometry also revealed a positive correlation between increased cell numbers and DOC (Pearson correlation = 0.17, p = 0.487, n = 19; Figure 3B) that was much stronger for the MM (Pearson correlation = 0.20, p = 0.496, n = 14) compared to the OS (Pearson correlation = -0.03, p = 0.973, n = 4) fracture waters. This also supported that MM waters depend on organic carbon sources infiltrated from the surface while the OS waters were fed from below by carbon dioxide and hydrogen of geological origin (Fry et al., 2008; Pedersen, 2013; Wu et al., 2015; Lopez-Fernandez et al., 2018b).
A small cell size of <0.2 μm and a streamlined genome are suggested to be adaptation strategies to the oligotrophic conditions in the deep biosphere (Giovannoni et al., 2014; Wu et al., 2015). Interestingly, the shallowest water included in this survey (groundwater MM-139.7) showed the highest values for DNA content per cell and cell size (Figure 3), which might be related to the proximity of this groundwater to the surface and the influence of the brackish water penetrating from the Baltic Sea. Assuming the limitations of the DNA content measurements and the possibility of multiple genome copies per cell, the varying relative proportions of populations with streamlined genomes between the water types may also explain the lack of a correlation between cell numbers and average cellular DNA content (Supplementary Figure S1).
Microbial Diversity Analyses
Details of the water volume filtered, amount of DNA extracted, and number of 16S rRNA gene amplicons obtained from the sequencing of the 21 boreholes are provided in Supplementary Table S1. The reproducibility of the biological triplicate samples per borehole section was confirmed as differences between each of the triplicates were insignificant (one-way ANOVA test, p > 0.05, n = 3 per borehole section; Supplementary Table S5). No statistically significant differences were found in the alpha diversity (Shannon H index) when comparing all the 21 borehole waters to each other (one-way ANOVA test, p > 0.05, n = 3 per borehole section; Figure 4A). In contrast, significant increases in alpha diversity were found when comparing the 16 MM waters to the single TM and four OS boreholes (one-way ANOVA post hoc test, p = 0.00, n = 48 for MM, n = 3 for TM and n = 12 for OS) supporting a greater diversity in the microbial communities closer to the surface and with more DOC content. However, no significant differences were found when comparing within the four OS waters (Figure 4A). Beta diversity analysis (Bray–Curtis dissimilarity) revealed that the three water types contained different microbial populations, with the highest dissimilarity in the TM water compared to the others (Figure 4B). This was likely due to the special chemical composition of this groundwater type composed by a mix of waters.
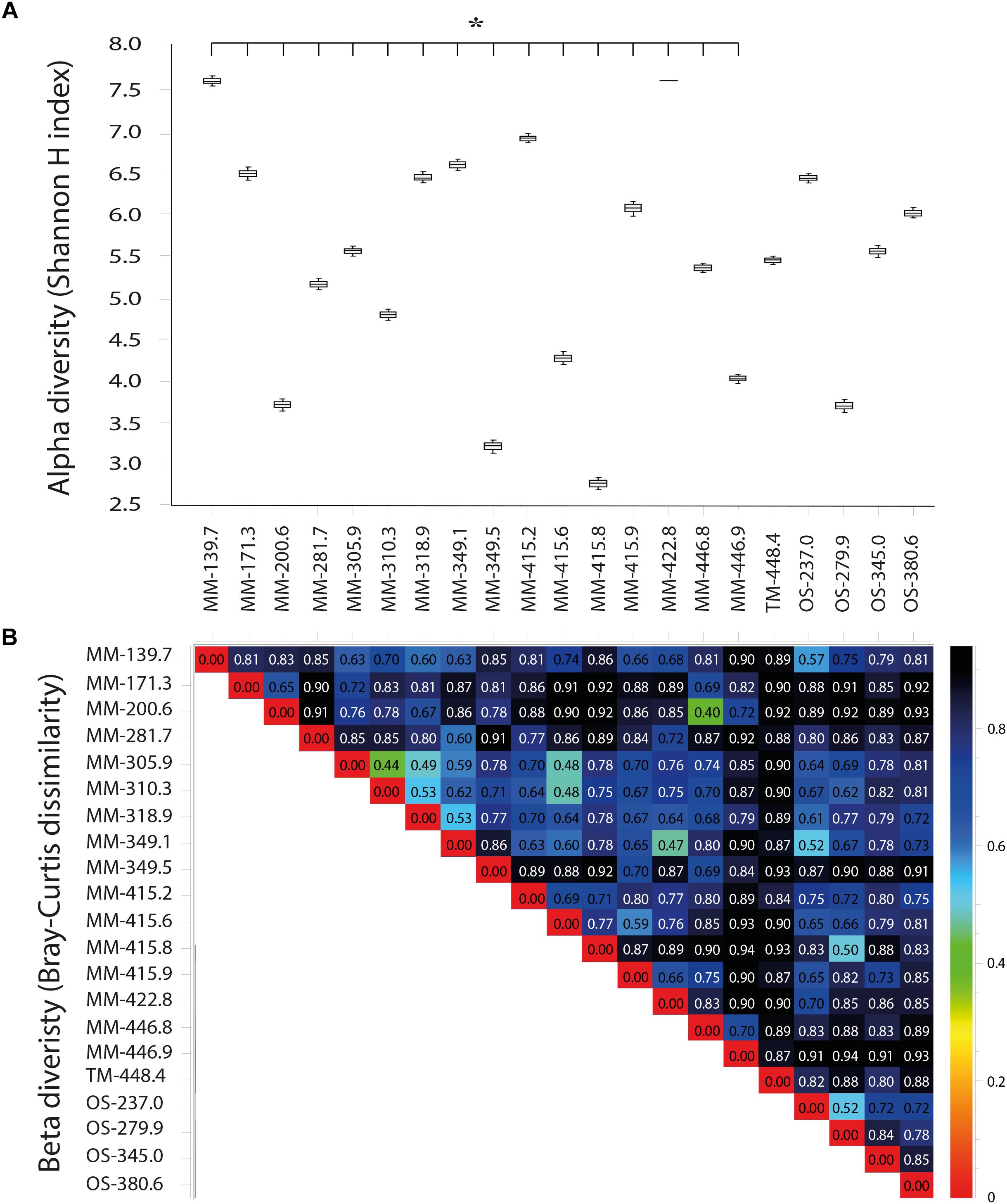
FIGURE 4. Shannon H alpha (A) and Bray–Curtis (B) diversities based on OTUs from the 21 sampled boreholes sections. The statistically significant Shannon H alpha diversity (p < 0.05) is marked with a star.
Microbial Community Composition in the Water Types
The dominant identified microbial taxa in all three groundwater types (Figure 5 and Supplementary Table S6) were Gammaproteobacteria (23% of the total relative abundance), unclassified sequences (22%), the newly proposed Campylobacterota (18%, formerly Epsilonproteobacteria) (Waite et al., 2017, 2018), Patescibacteria (14%), Delta- (7%), and Alphaproteobacteria (6%). At the OTU level, 16S rRNA gene sequences most similar to Sulfurimonas totaled 35.7 and 20.2% in the MM and OS waters, respectively; sequences most similar to the Thiobacillus genus constituted 21.2% of the MM waters; and sequences aligning with the genus Filomicrobium were dominant in the TM water with 34.6%. The deeper OS waters also showed a significantly higher relative abundance of Archaea including Euryarchaeota, Nanoarchaeota, Hadesarchaeaota, and the recently identified Hydrothermarchaeota (one-way ANOVA post hoc test, p < 0.05, n = 48 for MM, n = 3 for TM, and n = 12 for OS). The increased relative proportion of archaea was in agreement with other studies that reveal a dominance of Archaea in deep subsurface sediments (Biddle et al., 2006; Teske and Sørensen, 2008).
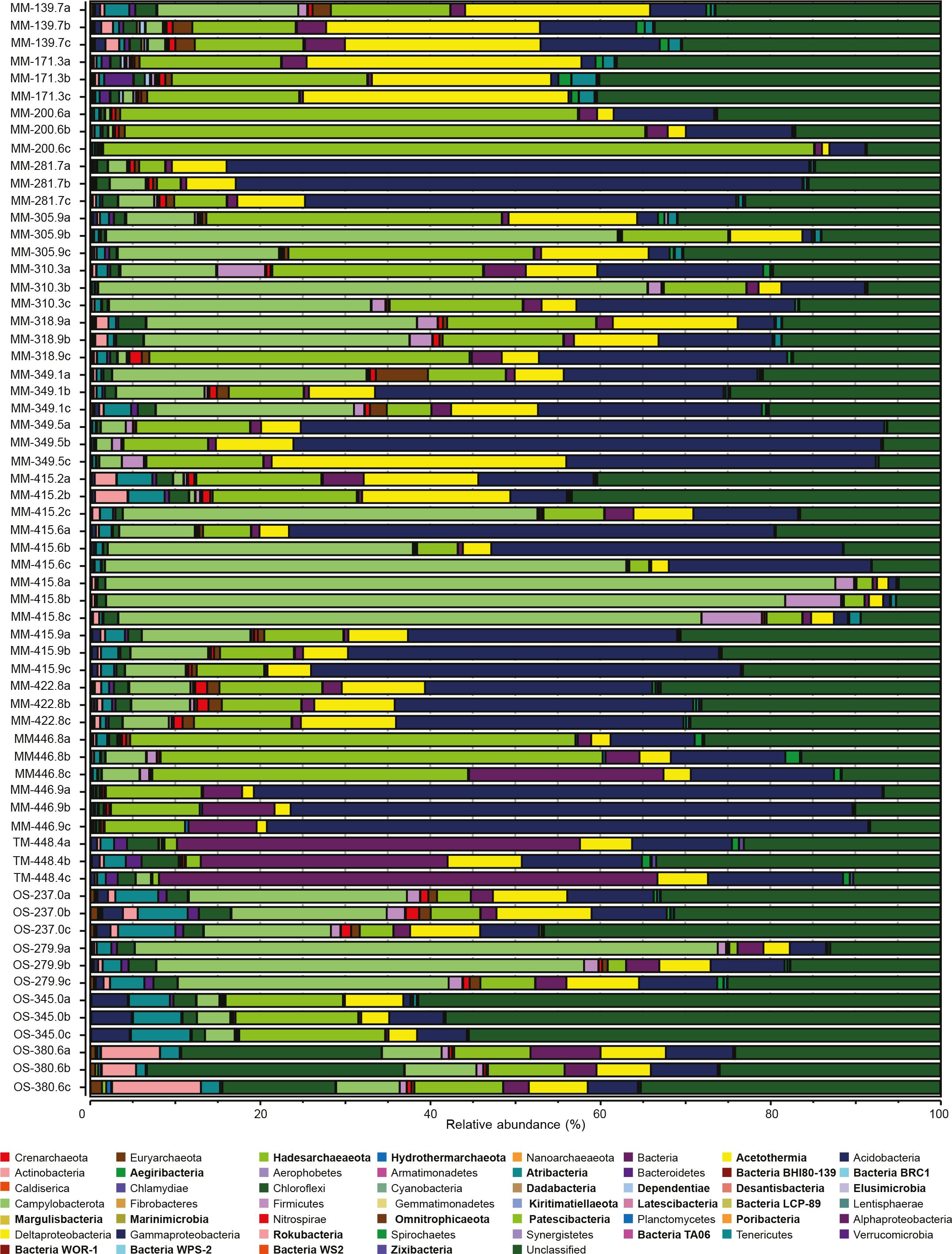
FIGURE 5. Relative abundances of triplicate 16S rRNA gene OTUs sequences from the 21 borehole sections. Microbial community is shown at phylum level (except for Proteobacteria, which have been divided into classes). Candidate phyla are labeled in bold type.
Not surprisingly for the extremely oligotrophic and the largely unexplored deep biosphere, many OTUs were affiliated to candidate phyla and unclassified microorganisms. Almost all candidate phyla that were significantly different between the three different water types showed a higher relative abundance in the OS waters (Figure 5 and Supplementary Table S4). In detail, 11 of the 21 groundwaters had >20% of unclassified OTUs, with the highest representation in borehole OS-345.0 with an average between the triplicate samples of >58% unclassified sequences. In addition, a total of 36 OTUs (10 in the MM, 18 in the OS, and 8 in the TM waters, Supplementary Table S6) had significantly increased relative abundances (one-way ANOVA post hoc test, p < 0.05, n = 48 for MM, n = 3 for TM, and n = 12 for OS) that aligned within the Patescibacteria superphylum. The newly described Patescibacteria may include more than 35 candidate phyla with reduced metabolic capacities (Hug et al., 2016; Parks et al., 2017). Despite that the Tree of Life has recently been expanded with many candidate phyla identified from shallow subsurface waters (Castelle et al., 2015;
Anantharaman et al., 2016, 2018; Hug et al., 2016), the continental deep biosphere contains a large microbial diversity waiting to be characterized and this proportion increases with depth and temporal separation from the surface. In addition, a more thorough study is required to elucidate if those populations placed within known taxa (e.g., the high relative proportion of Proteobacteria, excluding in this study the newly proposed phylum Campylobacterota, formerly Epsilonproteobacteria) are comprised of novel, deep subsurface adapted species.
Water Chemistry Shapes the Microbial Community Metabolic Capacities
The CCA based on the chemical parameters and 16S rRNA gene relative abundances supported that the water chemistries influenced the microbial diversity and that there were differences between the MM, TM, and OS waters (Figure 6 and Supplementary Table S4). The CCA showed that the higher DOC containing MM waters were separated into two groups based on the influence of nitrate/nitrite or ferrous iron (Figure 6), suggesting that nitrogen and iron cycling were major processes in the MM waters. Nitrite and ammonia were significantly higher in the MM waters compared to the OS and TM, while nitrate was statistically increased only in the TM water (one-way ANOVA post hoc test, p < 0.05, n = 48 for MM, n = 3 for TM, and n = 12 for OS; Supplementary Table S4). Many nitrogen-cycling taxa have been previously identified in Fennoscandian fracture waters (Kutvonen et al., 2015; Sohlberg et al., 2015; Hubalek et al., 2016; Purkamo et al., 2016). In addition, the genetic potential to fix nitrogen by a microbial community in a granitic subsurface mine has been described and that fixed nitrogen from a biological origin may support subsurface biomass (Swanner and Templeton, 2011). In addition, previous reconstruction of metagenome assembled genomes from the Äspö HRL continental deep subsurface showed a higher than anticipated prevalence of nitrate reducing populations (Wu et al., 2015). Those microorganisms present in this study included Sulfurimonas (Han and Perner, 2015) that despite constituting 35.7% of the total MM population did not have any OTUs with a statistically higher relative proportion compared to the OS and TM waters. However, Sulfurimonas are commonly isolated from sulfidic habitats and are also able to use reduced sulfur compounds as electron donor (Han and Perner, 2015). Therefore, the identified OTUs aligning with Sulfurimonas in this study might be involved in both the sulfur and nitrogen cycles. In contrast to the nitrate reducers in the MM waters, 4 Nitrospirae OTUs, 10 Pseudomonas OTUs, and 6 Hadesarchaeaeota OTUs were significantly higher (one-way ANOVA post hoc test, p < 0.05, n = 48 for MM, n = 3 for TM, and n = 12 for OS; Supplementary Table S6) in the OS water compared to the MM waters. Finally, the TM water contained seven OTUs aligning with the Thiobacillus genus that had significantly higher relative proportions compared to one or both of the MM and OS waters (one-way ANOVA post hoc test, p < 0.05, n = 48 for MM, n = 3 for TM, and n = 12 for OS; Supplementary Table S6). A potential product of nitrate reduction is ammonium that can then be assimilated as a nitrogen source within the cell, such as by the newly proposed phylum Campylobacterota (formerly Epsilonproteobacteria). Although there were no statistically significant differences between the three studied groundwater types at the phylum level, this new taxa showed a high relative abundance in the MM waters, especially in the MM-415.8 groundwater where the average relative abundance was greater than 78% (Figure 5). In addition, the TM water had a significantly increased ammonium concentration compared to the other waters (one-way ANOVA post hoc test, p < 0.05, n = 48 for MM, n = 3 for TM, and n = 12 for OS; Supplementary Table S4). The phyla with significantly higher relative abundances in the TM-448.4 included candidate (Ca.) phylum Zixibacteria, formerly RBG-1 (one-way ANOVA post hoc test, p < 0.05, n = 48 for MM, n = 3 for TM, and n = 12 for OS; Supplementary Table S4) that has been identified as the dominant microorganisms in aquifer sediments and has the ability to assimilate ammonium (Castelle et al., 2013). Previously, the deep continental biosphere was suggested to be mainly supported by sulfate reduction (Nielsen et al., 2006; Hallbeck and Pedersen, 2008; Katsuyama et al., 2013). However, although nitrate, nitrite, and ammonium concentrations were very low in this oligotrophic subsurface environment and regardless of their ultimate source (Swanner and Templeton, 2011), this study also supports that nitrogen cycling may be important for the microorganisms inhabiting the deep biosphere (Kutvonen et al., 2015).
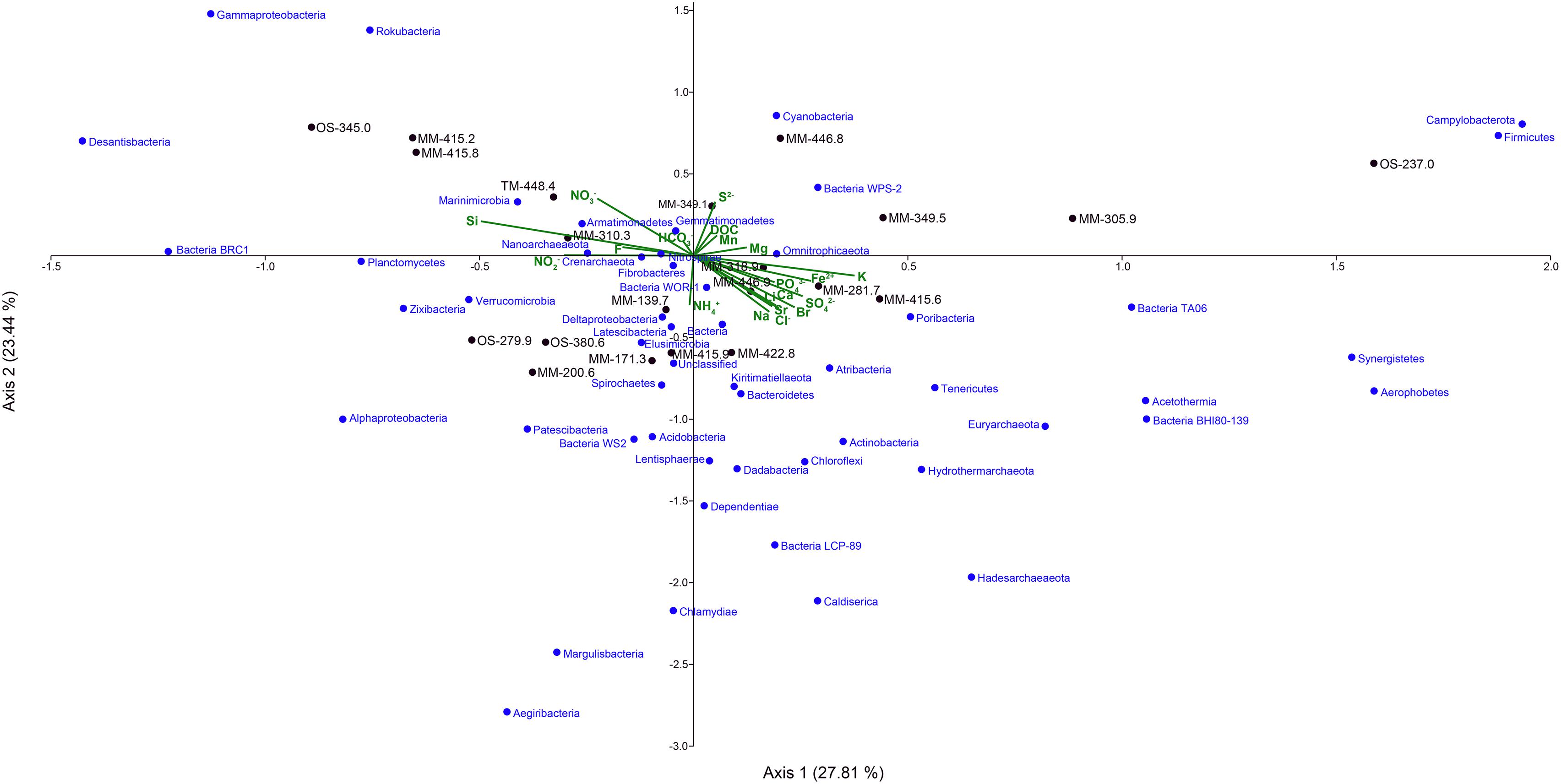
FIGURE 6. Canonical correspondence analysis of the 16S rRNA gene OTUs at phylum level (except for Proteobacteria, which have been divided into classes) combined with the geochemical data from the 21 borehole sections.
Ferrous iron was significantly higher in the MM compared to the other two water types (one-way ANOVA post hoc test, p = 0.001, n = 48 for MM, n = 3 for TM, and n = 12 for OS; Supplementary Table S4), suggesting that anaerobic ferric iron reducing microorganisms contributed to the increased level of ferrous iron in this water type. Microbial ferric iron reduction is phylogenetically scattered throughout the bacterial and archaeal domains, but mainly mediated by the Geobacteraceae family in subsurface environments (Lovley et al., 2004). The presence of ferrous-oxidizing and ferric-reducing microorganisms was identified using flow cells at the Äspö HRL (Ionescu et al., 2015). In this study, taxa that potentially carry out ferric reduction included two OTUs (one of them identified in all three water types) affiliated to Shewanella and with higher but not significantly different relative abundance in the MM waters (Supplementary Table S6); and one OTU from the order Desulfuromonadales (containing the Geobacter genus) identified as significantly higher in the OS compared to MM waters (one-way ANOVA post hoc test, p = 0.012, n = 48 for MM, n = 3 for TM, and n = 12 for OS; Supplementary Table S6). Ferrous iron-oxidizing taxa included the Ferritrophicum genus (Weiss et al., 2007) and Gallionellaceae family (Hallbeck and Pedersen, 2014) with 14 and six OTUs comprising 10.1 and 1.7% of the MM relative populations, respectively. Although the MM groundwaters contained a higher relative proportion of Ferritrophicum, the individual OTUs were not significantly increased compared to the OS and TM waters (Supplementary Table S6). Although the characterized species from these genera are microaerobic, they were identified in the studied anaerobic groundwaters and have also been described to be able to conserve energy under anaerobic conditions (He et al., 2016).
Sulfate was significantly higher in the OS compared to the other two water types while sulfide was significantly higher in the MM waters (one-way ANOVA post hoc test, p < 0.05, n = 48 for MM, n = 3 for TM, and n = 12 for OS; Supplementary Table S4). The higher concentration of sulfate and sulfide in the OS and MM waters, respectively, likely reflects the relative availability of electron donors in the two groundwater types. Deltaproteobacteria have been previously described as sulfate reducers in deep biosphere environments (e.g., Orsi et al., 2013). Via their ability to also grow by disproportionation of inorganic sulfur compounds (i.e., using them as both electron donor and acceptor) they generate hydrogen sulfide and sulfate (Finster, 2008), and this suggests sulfur cycling was occurring in this extremely oligotrophic environment. Deltaproteobacteria were highly represented in the three different water types and had the highest average relative abundances in MM-139.7 and MM-173.3 with 23.3 and 21.1%, respectively (Figure 5). Only a few OTUs assigned to the Deltaproteobacteria had significantly increased relative abundances in the MM waters (Supplementary Table S6) and these included 16S rRNA gene sequences that were assigned to the Desulfobacteraceae family and Desulfatiglans genus (one-way ANOVA post hoc test, p < 0.05, n = 48 for MM, n = 3 for TM, and n = 12 for OS). Members of the Desulfatiglans genus (Suzuki et al., 2014) and most of the Desulfobacteraceae (Kuever, 2014) are heterotrophs that match the reliance of the MM waters on organic carbon infiltration. That all four OS waters clustered with low DOC and high sulfate concentration (Figure 6) support the previous paradigm of a hydrogen-fueled, sulfate reducing deep biosphere community (Hallbeck and Pedersen, 2008; Wu et al., 2017) that is fed by organic carbon of a microbial origin (Purkamo et al., 2015; Kieft et al., 2018). This is supported by three Desulfocarbo OTUs suggested to be able to grow autotrophically with hydrogen (Kuever, 2014), two Desulfovibrio OTUs that potentially use hydrogen as electron donor (Kushwaha et al., 2018), three Desulfurivibrio OTUs that may use hydrogen (among others) as electron donor coupled to thiosulfate/polysulfide as electron acceptor (Sorokin et al., 2008), four Sulfurimonas OTUs that are described for their versatile chemoautotrophic metabolism (Han and Perner, 2015) that were significantly higher in the OS waters (one-way ANOVA post hoc test, p < 0.05, n = 48 for MM, n = 3 for TM, and n = 12 for OS; Supplementary Table S6). Sulfur oxidizing bacteria and archaea are also present in subsurface habitats (Ghosh and Dam, 2009). Sulfide ion (predominantly H2S/HS-) was significantly higher in the MM waters (one-way ANOVA post hoc test, p = 0.05, n = 48 for MM, n = 3 for TM, and n = 12 for OS; Supplementary Table S4) where the relative abundance of Gammaproteobacteria was also significantly higher (one-way ANOVA post hoc test, p = 0.006, n = 48 for MM, n = 3 for TM, and n = 12 for OS; Figure 5 and Supplementary Table S4). Some Gammaproteobacteria have been highlighted for their role in sulfur oxidation in deep sea hydrothermal vents (Sievert et al., 2008). One highly represented genus from the Gammaproteobacteria was the sulfur compound-oxidizing Thiobacillus (Robertson and Kuenen, 2006) that totaled 21.2% of the MM relative population but only one OTU was significantly increased compared to the OS water (one-way ANOVA post hoc test, p = 0.007, n = 48 for MM, n = 3 for TM, and n = 12 for OS; Supplementary Table S6). Moreover, Ca. phylum Marinimicrobia (SAR406), recently described for its implication in the ocean sulfur cycling via the polysulfide reductase ABC complex that can use H2S as an auxiliary electron donor (Hawley et al., 2017), was significantly higher in the MM waters (one-way ANOVA post hoc test, p = 0.048, n = 48 for MM, n = 3 for TM, and n = 12 for OS; Figure 5 and Supplementary Table S4). This supports that cryptic sulfur cycling was occurring in the three water types and that microbial sulfur disproportionation might be an option to deal with the limitation of electron donor, which may also explain the lack of accumulation of reduced sulfur compounds.
Sources of methane in the deep crystalline bedrock can be both abiotic (e.g., Ischer–Tropsch-type methane production) or biotic via methanogenesis (Kietäväinen and Purkamo, 2015). Methanogenesis is solely carried out by the Archaea and these microbes have been identified in groundwaters from the Fennoscandian Shield (Pande et al., 2014; Wu et al., 2015). In this study, three OTUs that align within the family Methermicoccaceae (Oren, 2014b) and one OTU from the Methanobacteriaceae (Oren, 2014a) had higher relative abundances in the OS water compared to MM groundwaters (one-way ANOVA post hoc test, p < 0.05, n = 48 for MM, n = 3 for TM, and n = 12 for OS; Supplementary Table S6). In addition, methanogenic Archaea from the Methanomassiliicoccales (Soellinger et al., 2015) were present in all waters, but no statistically significant differences were observed between the three different groundwaters. Although methane measurements were not available and therefore, its cycling cannot be confirmed the presence of OTUs that aligned with methane oxidizers and reducers suggested it played a role in the Äspö HRL groundwaters. Anaerobic oxidation of methane can be linked to nitrate reduction by the Ca. genus Methanoperedens (Vaksmaa et al., 2017) that was present in all three groundwaters with a low relative abundance. A second method of anaerobic methane oxidation is via syntrophic associations between microorganisms degrading organic compounds and methanogens. This is the case for one OTU from the genus Syntrophorhabdus (Qiu et al., 2008), 15 OTUs from the family Anaerolineaceae (McIlroy et al., 2017), and three OTUs similar to the genus Syntrophus (Siddique et al., 2011) that were significantly increased in the MM compared to the other two groundwaters (one-way ANOVA post hoc test, p < 0.05, n = 48 for MM, n = 3 for TM, and n = 12 for OS; Supplementary Table S6). These syntrophic relationships of symbiotic taxa that grow alongside methanogens supported that the MM waters have a community with more varied metabolic strategies compared to the OS waters (Wu et al., 2015; Lopez-Fernandez et al., 2018b). In addition, methanogens were represented by one OTU aligning to Methylobacterium genus (Stein, 2018) that was significantly higher in the OS waters, and three OTUs from the genus Methylomicrobium (Akberdin et al., 2018) significantly higher in the TM water (one-way ANOVA post hoc test, p < 0.05, n = 48 for MM, n = 3 for TM, and n = 12 for OS; Supplementary Table S6). Finally, five OTUs were significantly higher in the OS waters compared to the MM and TM (one-way ANOVA post hoc test, p < 0.05, n = 48 for MM, n = 3 for TM, and n = 12 for OS; Supplementary Table S6) aligning to the Ca. phylum Atribacteria (OP9) (Carr et al., 2015), which can potentially produce fermentation products that may also support methanogens. That OTUs assigned to archaeal and bacterial taxa carrying out methanogenesis had significantly higher relative abundance in the OS groundwaters supports the extreme oligotrophic conditions in these waters fed by geogases coming from below (Wu et al., 2015; Lopez-Fernandez et al., 2018b).
Conclusion
The 16S rRNA gene relative abundance of the 21 studied groundwaters showed dissimilar microbial community compositions, with significant differences between the three different water types. Specifically, the microbial diversity within the waters with MM signature was higher in the groundwaters with more DOC content. The TM water showed the highest dissimilarity compared to the other two water types, probably due to the more different chemical composition of this water type. Based on the chemical composition of the three classified water types, the identified microbial phyla might be involved in cycling of nitrogen, iron, and sulfur. Finally, the relative proportion of unclassified microorganisms and candidate phyla increased with depth. This indicates the importance and necessity of further studies to characterize the deep biosphere microbial populations and their influence in the global nutrients and energy cycles.
Data Availability
16S rRNA gene sequences are available at NCBI database with the Bioproject accession number PRJNA434543.
Author Contributions
ML-F, MD, and SB conceived the study. ML-F collected samples, carried out laboratory work, and analyzed biological data. MÅ analyzed geochemical data. ML-F drafted the manuscript and all authors gave final approval for publication.
Funding
The authors acknowledge the Swedish Research Council (2014-4398, 2012-3892, and 2017-04422) and SKB for access to the Äspö HRL and the Sicada database. MD thanks the Nova Center for University Studies, Research and Development, The Hellman Family Foundation, and The Crafoord Foundation.
Conflict of Interest Statement
The authors declare that the research was conducted in the absence of any commercial or financial relationships that could be construed as a potential conflict of interest.
Acknowledgments
We thank Christoffer Bergvall for flow cytometric analyses of bacterial cells. Sequencing was carried out at the National Genomics Infrastructure hosted by Science for Life Laboratory. Bioinformatics utilized the Uppsala Multidisciplinary Center for Advanced Computational Science resource (project b201 3127).
Supplementary Material
The Supplementary Material for this article can be found online at: https://www.frontiersin.org/articles/10.3389/fmicb.2018.02880/full#supplementary-material
FIGURE S1 | Average cell numbers including error bars (A), DNA content per cell (B), and size distribution (C) of triplicate measurements, including standard deviation and coefficient of variation, of the microbial community from the 21 borehole sections. DNA content and size distribution are shown in arbitrary units.
TABLE S1 | Sampling and sequencing information of 21 borehole sections representing the borehole and sample name, water type, total volume of water sampled, DNA extracted, number of read pairs obtained from the sequencing facility, after merging and quality trimming, and the amount of OTUs.
TABLE S2 | Detailed geochemical composition of the 21 groundwaters since the monitoring started in early/mid-1990s until the last measurement in May 2016 (November 2015 only for SA2074A-1).
TABLE S3 | One-way ANOVA of the difference in the chemical values between the three water types (p-values are shown in the table). Average values (mg/L, except for pH in units) for the chemical data in each water type are included. Differences in values that are statistically significant (p < 0.05) are marked in color.
TABLE S4 | One-way ANOVA post hoc test of the difference in chemical values and 16S rRNA relative abundance for individual phyla and proteobacterial classes between the three water types (p-values are shown in the table). Statistically significant differences (p < 0.05) are marked in color with the water type with higher values noted.
TABLE S5 | One-way ANOVA post hoc test of the difference in 16S rRNA gene relative abundance for individual phyla and proteobacterial classes between the triplicate samples per borehole section (p-values are shown in the table).
TABLE S6 | Average relative abundance of the OTUs (97% similarity) for the three water types plus one-way ANOVA post hoc test of the difference in 16S rRNA gene relative abundance for all comparisons between the water types (i.e., MM vs. OS, MM vs. TM, and TM vs. OS). Significant differences are colored for the water type where the OTU has a significantly increased relative abundance (MM, green; TM, red; and OS, blue).
Footnotes
References
Akberdin, I. R., Thompson, M., Hamilton, R., Desai, N., Alexander, D., Henard, C. A., et al. (2018). Methane utilization in Methylomicrobium alcaliphilum 20Z R: a systems approach. Sci. Rep. 8:2512. doi: 10.1038/s41598-018-20574-z
Alakangas, L. J., Mathurin, F. A., Faarinen, M., Wallin, B., and Åström, M. E. (2014). Sampling and characterizing rare earth elements in groundwater in deep-lying fractures in granitoids under in situ high-pressure and low-redox conditions. Aquat. Geochem. 20, 405–418. doi: 10.1007/s10498-014-9225-z
Almén, K.-E., Andersson, O., Fridh, B., Johansson, B.-E., Sehlstedt, M., Gustafsson, E., et al. (1986). Site Investigation-Equipment for Geological, Geophysical, Hydrogeological and Hydrochemical Characterization. Stockholm: Swedish Nuclear Fuel and Waste Management Co.
Anantharaman, K., Brown, C. T., Hug, L. A., Sharon, I., Castelle, C. J., Probst, A. J., et al. (2016). Thousands of microbial genomes shed light on interconnected biogeochemical processes in an aquifer system. Nat. Commun. 7:13219. doi: 10.1038/ncomms13219
Anantharaman, K., Hausmann, B., Jungbluth, S. P., Kantor, R. S., Lavy, A., Warren, L. A., et al. (2018). Expanded diversity of microbial groups that shape the dissimilatory sulfur cycle. ISME J. 12, 1715–1728. doi: 10.1038/s41396-018-0078-0
Bertilsson, S., Berglund, O., Karl, D. M., and Chisholm, S. W. (2003). Elemental composition of marine Prochlorococcus and Synechococcus: implications for the ecological stoichiometry of the sea. Limnol. Oceanogr. 48, 1721–1731. doi: 10.4319/lo.2003.48.5.1721
Biddle, J. F., Lipp, J. S., Lever, M. A., Lloyd, K. G., Sørensen, K. B., Anderson, R., et al. (2006). Heterotrophic Archaea dominate sedimentary subsurface ecosystems off Peru. Proc. Natl. Acad. Sci. U.S.A. 103, 3846–3851. doi: 10.1073/pnas.0600035103
Carr, S. A., Orcutt, B. N., Mandernack, K. W., and Spear, J. R. (2015). Abundant Atribacteria in deep marine sediment from the Adélie Basin. Antarctica. Front. Microbiol. 6:872. doi: 10.3389/fmicb.2015.00872
Castelle, C. J., Hug, L. A., Wrighton, K. C., Thomas, B. C., Williams, K. H., Wu, D., et al. (2013). Extraordinary phylogenetic diversity and metabolic versatility in aquifer sediment. Nat. Commun. 4:2120. doi: 10.1038/ncomms3120
Castelle, C. J., Wrighton, K. C., Thomas, B. C., Hug, L. A., Brown, C. T., Wilkins, M. J., et al. (2015). Genomic expansion of domain archaea highlights roles for organisms from new phyla in anaerobic carbon cycling. Curr. Biol. 25, 690–701. doi: 10.1016/j.cub.2015.01.014
Drake, H., Åström, M. E., Tullborg, E.-L., Whitehouse, M., and Fallick, A. E. (2013). Variability of sulphur isotope ratios in pyrite and dissolved sulphate in granitoid fractures down to 1 km depth–evidence for widespread activity of sulphur reducing bacteria. Geochim. Cosmochim. Acta 102, 143–161. doi: 10.1016/j.gca.2012.10.036
Drake, H., Tullborg, E.-L., Hogmalm, K. J., and Åström, M. E. (2012). Trace metal distribution and isotope variations in low-temperature calcite and groundwater in granitoid fractures down to 1 km depth. Geochim. Cosmochim. Acta 84, 217–238. doi: 10.1016/j.gca.2012.01.039
Eydal, H. S., Jagevall, S., Hermansson, M., and Pedersen, K. (2009). Bacteriophage lytic to Desulfovibrio aespoeensis isolated from deep groundwater. ISME J. 3, 1139–1147. doi: 10.1038/ismej.2009.66
Eydal, H. S., and Pedersen, K. (2007). Use of an ATP assay to determine viable microbial biomass in Fennoscandian Shield groundwater from depths of 3-1000 m. J. Microbiol. Methods 70, 363–373. doi: 10.1016/j.mimet.2007.05.012
Finster, K. (2008). Microbiological disproportionation of inorganic sulfur compounds. J. Sulfur Chem. 29, 281–292. doi: 10.1080/17415990802105770
Fry, J. C., Parkes, R. J., Cragg, B. A., Weightman, A. J., and Webster, G. (2008). Prokaryotic biodiversity and activity in the deep subseafloor biosphere. FEMS Microbiol. Ecol. 66, 181–196. doi: 10.1111/j.1574-6941.2008.00566.x
Ghosh, W., and Dam, B. (2009). Biochemistry and molecular biology of lithotrophic sulfur oxidation by taxonomically and ecologically diverse bacteria and archaea. FEMS Microbiol. Rev. 33, 999–1043. doi: 10.1111/j.1574-6976.2009.00187.x
Giorgio, P. A. D., Bird, D. F., Prairie, Y. T., and Planas, D. (1996). Flow cytometric determination of bacterial abundance in lake plankton with the green nucleic acid stain SYTO 13. Limnol. Oceanogr. 41, 783–789. doi: 10.4319/lo.1996.41.4.0783
Giovannoni, S. J., Thrash, J. C., and Temperton, B. (2014). Implications of streamlining theory for microbial ecology. ISME J. 8, 1553–1565. doi: 10.1038/ismej.2014.60
Gomez, J. B., Gimeno, M. J., Auque, L. F., and Acero, P. (2014). Characterisation and modelling of mixing processes in groundwaters of a potential geological repository for nuclear wastes in crystalline rocks of Sweden. Sci. Total Environ. 468, 791–803. doi: 10.1016/j.scitotenv.2013.09.007
Hallbeck, L., and Pedersen, K. (2008). Characterization of microbial processes in deep aquifers of the Fennoscandian Shield. Appl. Geochem. 23, 1796–1819. doi: 10.1016/j.apgeochem.2008.02.012
Hallbeck, L., and Pedersen, K. (2014). “The family Gallionellaceae,” in The Prokaryotes, eds E. Rosenberg, E. F. DeLong, S. Lory, E. Stackebrandt, and F. Thompson (Berlin: Springer), 853–858.
Hammer, O., Harper, D., and Ryan, P. (2001). PAST: paleontological statistics software package for education and data analysis. Palaeontol. Electron. 4, 1–9.
Han, Y., and Perner, M. (2015). The globally widespread genus Sulfurimonas: versatile energy metabolisms and adaptations to redox clines. Front. Microbiol. 6:989. doi: 10.3389/fmicb.2015.00989
Hawley, A. K., Nobu, M. K., Wright, J. J., Durno, W. E., Morgan-Lang, C., Sage, B., et al. (2017). Diverse Marinimicrobia bacteria may mediate coupled biogeochemical cycles along eco-thermodynamic gradients. Nat. Commun. 8:1507. doi: 10.1038/s41467-017-01376-9
He, S., Tominski, C., Kappler, A., Behrens, S., and Roden, E. E. (2016). Metagenomic analyses of the autotrophic Fe(II)-oxidizing, nitrate-reducing enrichment culture KS. Appl. Environ. Microbiol. 82, 2656–2668. doi: 10.1128/AEM.03493-15
Herlemann, D. P., Labrenz, M., Jürgens, K., Bertilsson, S., Waniek, J. J., and Andersson, A. F. (2011). Transitions in bacterial communities along the 2000 km salinity gradient of the Baltic Sea. ISME J. 5, 1571–1579. doi: 10.1038/ismej.2011.41
Hubalek, V., Wu, X., Eiler, A., Buck, M., Heim, C., Dopson, M., et al. (2016). Connectivity to the surface determines diversity patterns in subsurface aquifers of the Fennoscandian shield. ISME J. 10, 2447–2458. doi: 10.1038/ismej.2016.36
Hug, L. A., Baker, B. J., Anantharaman, K., Brown, C. T., Probst, A. J., Castelle, C. J., et al. (2016). A new view of the tree of life. Nat. Microbiol. 1:16048. doi: 10.1038/nmicrobiol.2016.48
Hugerth, L. W., Wefer, H. A., Lundin, S., Jacobsson, H. E., Lindberg, M., Rodin, S., et al. (2014). DegePrime: a program for degenerate primer design for broad taxonomic-range PCR for microbial ecology studies. Appl. Environ. Microbiol. 80, 5116–5123. doi: 10.1128/AEM.01403-14
Ionescu, D., Heim, C., Polerecky, L., Ramette, A., Haeusler, S., Bizic-Ionescu, M., et al. (2015). Diversity of iron oxidizing and reducing bacteria in flow reactors in the Äspö Hard Rock Laboratory. Geomicrobiol. J. 32, 207–220. doi: 10.1080/01490451.2014.884196
Itavaara, M., Nyyssonen, M., Kapanen, A., Nousiainen, A., Ahonen, L., and Kukkonen, I. (2011). Characterization of bacterial diversity to a depth of 1500 m in the Outokumpu deep borehole, Fennoscandian Shield. FEMS Microbiol. Ecol. 77, 295–309. doi: 10.1111/j.1574-6941.2011.01111.x
Itavaara, M., Salavirta, H., Marjamaa, K., and Ruskeeniemi, T. (2016). “Geomicrobiology and metagenomics of terrestrial deep subsurface microbiomes,” in Advances in Applied Microbiology, Vol. 94, eds S. Sariaslani and G. M. Gadd (Amsterdam: Elsevier), 1–77.
Kallmeyer, J., Pockalny, R., Adhikari, R. R., Smith, D. C., and D’hondt, S. (2012). Global distribution of microbial abundance and biomass in subseafloor sediment. Proc. Natl. Acad. Sci. U.S.A. 109, 16213–16216. doi: 10.1073/pnas.1203849109
Katsuyama, C., Nashimoto, H., Nagaosa, K., Ishibashi, T., Furuta, K., Kinoshita, T., et al. (2013). Occurrence and potential activity of denitrifiers and methanogens in groundwater at 140 m depth in Pliocene diatomaceous mudstone of northern Japan. FEMS Microbiol. Ecol. 86, 532–543. doi: 10.1111/1574-6941.12179
Kieft, T. L., Walters, C. C., Higgins, M. B., Mennito, A. S., Clewett, C. F., Heuer, V., et al. (2018). Dissolved organic matter compositions in 0.6–3.4 km deep fracture waters, Kaapvaal Craton, South Africa. Organ. Geochem. 118, 116–131. doi: 10.1016/j.orggeochem.2018.02.003
Kietäväinen, R., and Purkamo, L. (2015). The origin, source, and cycling of methane in deep crystalline rock biosphere. Front. Microbiol. 6:725. doi: 10.3389/fmicb.2015.00725
Kuever, J. (2014). “The Family Desulfarculaceae,” in The Prokaryotes, eds E. Rosenberg, E. F. DeLong, S. Lory, E. Stackebrandt, and F. Thompson (Berlin: Springer), 41–44.
Kushwaha, S., Marcus, A. K., and Rittmann, B. E. (2018). pH-dependent speciation and hydrogen (H2) control U(VI) respiration by Desulfovibrio vulgaris. Biotechnol. Bioeng. 115, 1465–1474. doi: 10.1002/bit.26579
Kutvonen, H., Rajala, P., Carpén, L., and Bomberg, M. (2015). Nitrate and ammonia as nitrogen sources for deep subsurface microorganisms. Front. Microbiol. 6:1079. doi: 10.3389/fmicb.2015.01079
Laaksoharju, M., Gascoyne, M., and Gurban, I. (2008a). Understanding groundwater chemistry using mixing models. Appl. Geochem. 23, 1921–1940. doi: 10.1016/j.apgeochem.2008.02.018
Laaksoharju, M., Smellie, J., Tullborg, E. L., Gimeno, M., Molinero, J., Gurban, I., et al. (2008b). Hydrogeochemical evaluation and modelling performed within the Swedish site investigation programme. Appl. Geochem. 23, 1761–1795. doi: 10.1016/j.apgeochem.2008.02.015
Laaksoharju, M., Skarman, C., and Skarman, E. (1999). Multivariate mixing and mass balance (M3) calculations, a new tool for decoding hydrogeochemical information. Appl. Geochem. 14, 861–871. doi: 10.1016/S0883-2927(99)00024-4
Lopez-Fernandez, M., Broman, E., Turner, S., Wu, X., Bertilsson, S., and Dopson, M. (2018a). Investigation of viable taxa in the deep terrestrial biosphere suggests high rates of nutrient recycling. FEMS Microbiol. Ecol. 94:fiy121. doi: 10.1093/femsec/fiy121
Lopez-Fernandez, M., Simone, D., Wu, X., Soler, L., Nilsson, E., Holmfeldt, K., et al. (2018b). Metatranscriptomes reveal all three domains of life are active, but are dominated by bacteria in the Fennoscandian crystalline granitic continental deep biosphere. mBio (in press).
Lovley, D. R., Holmes, D. E., and Nevin, K. P. (2004). Dissimilatory Fe(III) and Mn(IV) reduction. Adv. Microb. Physiol. 49, 219–286. doi: 10.1016/S0065-2911(04)49005-5
Magnabosco, C., Ryan, K., Lau, M. C., Kuloyo, O., Lollar, B. S., Kieft, T. L., et al. (2016). A metagenomic window into carbon metabolism at 3 km depth in Precambrian continental crust. ISME J. 10, 730–741. doi: 10.1038/ismej.2015.150
Mathurin, F. A., Åström, M. E., Drake, H., Maskenskaya, O. M., and Kalinowski, B. E. (2014a). REE and Y in groundwater in the upper 1.2 km of Proterozoic granitoids (Eastern Sweden) – Assessing the role of composition and origin of groundwaters, geochemistry of fractures, and organic/inorganic aqueous complexation. Geochim. Cosmochim. Acta 144, 342–378. doi: 10.1016/j.gca.2014.08.004
Mathurin, F. A., Drake, H., Tullborg, E. L., Berger, T., Peltola, P., Kalinowski, B. E., et al. (2014b). High cesium concentrations in groundwater in the upper 1.2 km of fractured crystalline rock - Influence of groundwater origin and secondary minerals. Geochim. Cosmochim. Acta 132, 187–213. doi: 10.1016/j.gca.2014.02.001
Mathurin, F. A., Astrom, M. E., Laaksoharju, M., Kalinowski, B. E., and Tullborg, E. L. (2012). Effect of tunnel excavation on source and mixing of groundwater in a coastal granitoidic fracture network. Environ. Sci. Technol. 46, 12779–12786. doi: 10.1021/es301722b
McIlroy, S. J., Kirkegaard, R. H., Dueholm, M. S., Fernando, E., Karst, S. M., Albertsen, M., et al. (2017). Culture-independent analyses reveal novel anaerolineaceae as abundant primary fermenters in anaerobic digesters treating waste activated sludge. Front. Microbiol. 8:1134. doi: 10.3389/fmicb.2017.01134
McMahon, S., and Parnell, J. (2014). Weighing the deep continental biosphere. FEMS Microbiol. Ecol. 87, 113–120. doi: 10.1111/1574-6941.12196
McMurdie, P. J., and Holmes, S. (2013). phyloseq: An R package for reproducible interactive analysis and graphics of microbiome census data. PLoS One 8:e61217. doi: 10.1371/journal.pone.0061217
Morono, Y., Terada, T., Kallmeyer, J., and Inagaki, F. (2013). An improved cell separation technique for marine subsurface sediments: applications for high-throughput analysis using flow cytometry and cell sorting. Environ. Microbiol. 15, 2841–2849. doi: 10.1111/1462-2920.12153
Morono, Y., Terada, T., Nishizawa, M., Ito, M., Hillion, F., Takahata, N., et al. (2011). Carbon and nitrogen assimilation in deep subseafloor microbial cells. Proc. Natl. Acad. Sci. U.S.A. 108, 18295–18300. doi: 10.1073/pnas.1107763108
Nielsen, M. E., Fisk, M. R., Istok, J. D., and Pedersen, K. (2006). Microbial nitrate respiration of lactate at in situ conditions in ground water from a granitic aquifer situated 450 m underground. Geobiology 4, 43–52. doi: 10.1111/j.1472-4669.2006.00068.x
Oren, A. (2014a). “The family Methanobacteriaceae,” in The Prokaryotes, eds E. Rosenberg, E. F. DeLong, S. Lory, E. Stackebrandt, and F. Thompson (Berlin: Springer), 165–193.
Oren, A. (2014b). “The Family Methermicoccaceae,” in The Prokaryotes, eds E. Rosenberg, E. F. DeLong, S. Lory, E. Stackebrandt, and F. Thompson (Berlin: Springer), 307–309.
Orsi, W. D., Edgcomb, V. P., Christman, G. D., and Biddle, J. F. (2013). Gene expression in the deep biosphere. Nature 499, 205–208. doi: 10.1038/nature12230
Pande, S., Merker, H., Bohl, K., Reichelt, M., Schuster, S., De Figueiredo, L. F., et al. (2014). Fitness and stability of obligate cross-feeding interactions that emerge upon gene loss in bacteria. ISME J. 8, 953–962. doi: 10.1038/ismej.2013.211
Parks, D. H., Rinke, C., Chuvochina, M., Chaumeil, P.-A., Woodcroft, B. J., Evans, P. N., et al. (2017). Recovery of nearly 8,000 metagenome-assembled genomes substantially expands the tree of life. Nat. Microbiol. 2, 1533–1542. doi: 10.1038/s41564-017-0012-7
Pedersen, K. (2013). Metabolic activity of subterranean microbial communities in deep granitic groundwater supplemented with methane and H2. ISME J. 7, 839–849. doi: 10.1038/ismej.2012.144
Pedersen, K., Bengtsson, A. F., Edlund, J. S., and Eriksson, L. C. (2014). Sulphate-controlled diversity of subterranean microbial communities over depth in deep groundwater with opposing gradients of sulphate and methane. Geomicrobiol. J. 31, 617–631. doi: 10.1080/01490451.2013.879508
Pedersen, K., and Ekendahl, S. (1992). Assimilation of CO2 and introduced organic compounds by bacterial communities in groundwater from southeastern Sweden deep crystalline bedrock. Microb. Ecol. 23, 1–14. doi: 10.1007/BF00165903
Pfiffner, S. M., Cantu, J. M., Smithgall, A., Peacock, A. D., White, D. C., Moser, D. P., et al. (2006). Deep subsurface microbial biomass and community structure in Witwatersrand Basin mines. Geomicrobiol. J. 23, 431–442. doi: 10.1080/01490450600875712
Purkamo, L., Bomberg, M., Kietäväinen, R., Salavirta, H., Nyyssönen, M., Nuppunen-Puputti, M., et al. (2016). Microbial co-occurrence patterns in deep Precambrian bedrock fracture fluids. Biogeosciences 13, 3091–3108. doi: 10.5194/bg-13-3091-2016
Purkamo, L., Bomberg, M., Nyyssönen, M., Kukkonen, I., Ahonen, L., and Itävaara, M. (2015). Heterotrophic communities supplied by ancient organic carbon predominate in deep Fennoscandian bedrock fluids. Microb. Ecol. 69, 319–332. doi: 10.1007/s00248-014-0490-6
Qiu, Y.-L., Hanada, S., Ohashi, A., Harada, H., Kamagata, Y., and Sekiguchi, Y. (2008). Syntrophorhabdus aromaticivorans gen. nov., sp. nov., the first cultured anaerobe capable of degrading phenol to acetate in obligate syntrophic associations with a hydrogenotrophic methanogen. Appl. Environ. Microbiol. 74, 2051–2058. doi: 10.1128/AEM.02378-07
Robertson, L. A., and Kuenen, J. G. (2006). “The genus Thiobacillus,” in The Prokaryotes, eds M. Dworkin, S. Falkow, E. Rosenberg, K. H. Schleifer, and E. Stackebrandt (New York, NY: Springer), 812–827.
Rönnback, P., and Åström, M. (2007). Hydrochemical patterns of a small lake and a stream in an uplifting area proposed as a repository site for spent nuclear fuel, Forsmark, Sweden. J. Hydrol. 344, 223–235. doi: 10.1016/j.jhydrol.2007.07.013
Schippers, A., Neretin, L. N., Kallmeyer, J., Ferdelman, T. G., Cragg, B. A., Parkes, R. J., et al. (2005). Prokaryotic cells of the deep sub-seafloor biosphere identified as living bacteria. Nature 433, 861–864. doi: 10.1038/nature03302
Siddique, T., Penner, T., Semple, K., and Foght, J. M. (2011). Anaerobic biodegradation of longer-chain n-alkanes coupled to methane production in oil sands tailings. Environ. Sci. Technol. 45, 5892–5899. doi: 10.1021/es200649t
Sievert, S. M., Hügler, M., Taylor, C. D., and Wirsen, C. O. (2008). Sulfur Oxidation at Deep-Sea Hydrothermal Vents. Berlin: Springer, 238–258. doi: 10.1007/978-3-540-72682-1_19
Soellinger, A., Schwab, C., Weinmaier, T., Loy, A., Tveit, A. T., Schleper, C., et al. (2015). Phylogenetic and genomic analysis of Methanomassiliicoccales in wetlands and animal intestinal tracts reveals clade-specific habitat preferences. FEMS Microbiol. Ecol. 92:fiv149. doi: 10.1093/femsec/fiv149
Sohlberg, E., Bomberg, M., Miettinen, H., Nyyssönen, M., Salavirta, H., Vikman, M., et al. (2015). Revealing the unexplored fungal communities in deep groundwater of crystalline bedrock fracture zones in Olkiluoto, Finland. Front. Microbiol. 6:573. doi: 10.3389/fmicb.2015.00573
Sorokin, D. Y., Tourova, T., Mußmann, M., and Muyzer, G. (2008). Dethiobacter alkaliphilus gen. nov. sp. nov., and Desulfurivibrio alkaliphilus gen. nov. sp. nov.: two novel representatives of reductive sulfur cycle from soda lakes. Extremophiles 12, 431–439. doi: 10.1007/s00792-008-0148-8
Standish, T., Chen, J., Jacklin, R., Jakupi, P., Ramamurthy, S., Zagidulin, D., et al. (2016). Corrosion of copper-coated steel high level nuclear waste containers under permanent disposal conditions. Electrochim. Acta 211, 331–342. doi: 10.1016/j.electacta.2016.05.135
Stein, L. Y. (2018). “Proteobacterial methanotrophs, methylotrophs, and nitrogen,” in Methane Biocatalysis: Paving the Way to Sustainability, eds M. Kalyuzhnaya and X. H. Xing (Cham: Springer), 57–66.
Suzuki, D., Li, Z., Cui, X., Zhang, C., and Katayama, A. (2014). Reclassification of Desulfobacterium anilini as Desulfatiglans anilini comb. nov. within Desulfatiglans gen. nov., and description of a 4-chlorophenol-degrading sulfate-reducing bacterium, Desulfatiglans parachlorophenolica sp. nov. Int. J. Syst. Evol. Microbiol. 64, 3081–3086. doi: 10.1099/ijs.0.064360-0
Swanner, E., and Templeton, A. (2011). Potential for nitrogen fixation and nitrification in the granite-hosted subsurface at Henderson mine. CO. Front. Microbiol. 2:254. doi: 10.3389/fmicb.2011.00254
Teske, A., and Sørensen, K. B. (2008). Uncultured archaea in deep marine subsurface sediments: have we caught them all? ISME J. 2, 3–18. doi: 10.1038/ismej.2007.90
Vaksmaa, A., Guerrero-Cruz, S., Van Alen, T. A., Cremers, G., Ettwig, K. F., Lüke, C., et al. (2017). Enrichment of anaerobic nitrate-dependent methanotrophic ‘Candidatus Methanoperedens nitroreducens’ archaea from an Italian paddy field soil. Appl. Microbiol. Biotechnol. 101, 7075–7084. doi: 10.1007/s00253-017-8416-0
Waite, D. W., Vanwonterghem, I., Rinke, C., Parks, D. H., Zhang, Y., Takai, K., et al. (2017). Comparative genomic analysis of the class Epsilonproteobacteria and proposed reclassification to Epsilonbacteraeota (phyl. nov.). Front. Microbiol. 8:682. doi: 10.3389/fmicb.2017.00682
Waite, D. W., Vanwonterghem, I., Rinke, C., Parks, D. H., Zhang, Y., Takai, K., et al. (2018). Addendum: comparative genomic analysis of the class Epsilonproteobacteria and proposed reclassification to Epsilonbacteraeota (phyl. nov.). Front. Microbiol. 9:772. doi: 10.3389/fmicb.2018.00772
Weiss, J. V., Rentz, J. A., Plaia, T., Neubauer, S. C., Merrill-Floyd, M., Lilburn, T., et al. (2007). Characterization of neutrophilic Fe(II)-oxidizing bacteria isolated from the rhizosphere of wetland plants and description of Ferritrophicum radicicola gen. nov. sp. nov., and Sideroxydans paludicola sp. nov. Geomicrobiol. J. 24, 559–570. doi: 10.1080/01490450701670152
Wu, X., Holmfeldt, K., Hubalek, V., Lundin, D., Åström, M., Bertilsson, S., et al. (2015). Microbial metagenomes from three aquifers in the Fennoscandian shield terrestrial deep biosphere reveal metabolic partitioning among populations. ISME J. 10, 1192–1203. doi: 10.1038/ismej.2015.185
Wu, X., Pedersen, K., Edlund, J., Eriksson, L., Åström, M., Andersson, A. F., et al. (2017). Potential for hydrogen-oxidizing chemolithoautotrophic and diazotrophic populations to initiate biofilm formation in oligotrophic, deep terrestrial subsurface waters. Microbiome 5:37. doi: 10.1186/s40168-017-0253-y
Keywords: 16S rRNA gene, amplicon sequencing, deep subsurface, groundwaters, chemistry, microbial diversity
Citation: Lopez-Fernandez M, Åström M, Bertilsson S and Dopson M (2018) Depth and Dissolved Organic Carbon Shape Microbial Communities in Surface Influenced but Not Ancient Saline Terrestrial Aquifers. Front. Microbiol. 9:2880. doi: 10.3389/fmicb.2018.02880
Received: 11 September 2018; Accepted: 09 November 2018;
Published: 27 November 2018.
Edited by:
Axel Schippers, Bundesanstalt für Geowissenschaften und Rohstoffe (BGR), GermanyReviewed by:
Nia Lyn Blackwell, University of Tubingen, GermanyCarsten Vogt, Helmholtz-Zentrum für Umweltforschung UFZ, Germany
Takuro Nunoura, Japan Agency for Marine-Earth Science and Technology, Japan
Copyright © 2018 Lopez-Fernandez, Åström, Bertilsson and Dopson. This is an open-access article distributed under the terms of the Creative Commons Attribution License (CC BY). The use, distribution or reproduction in other forums is permitted, provided the original author(s) and the copyright owner(s) are credited and that the original publication in this journal is cited, in accordance with accepted academic practice. No use, distribution or reproduction is permitted which does not comply with these terms.
*Correspondence: Margarita Lopez-Fernandez, margarita.lopezfernandez@lnu.se Mark Dopson, mark.dopson@lnu.se
†Present address: Margarita Lopez-Fernandez, Institute of Resource Ecology, Helmholtz-Zentrum Dresden-Rossendorf, Dresden, Germany