- 1Dipartimento di Scienze per gli Alimenti, la Nutrizione e l’Ambiente (DeFENS), Università degli Studi di Milano, Milan, Italy
- 2Red Sea Research Center (RSRC), King Abdullah University of Science and Technology (KAUST), Thuwal, Saudi Arabia
The adaptation of sporeformers to extreme environmental conditions is frequently questioned due to their capacity to produce highly resistant endospores that are considered as resting contaminants, not representing populations adapted to the system. In this work, in order to gain a better understanding of bacterial adaptation to extreme habitats, we investigated the phenotypic and genomic characteristics of the halophile Virgibacillus sp. 21D isolated from the seawater-brine interface (SBI) of the MgCl2-saturated deep hypersaline anoxic basin Discovery located in the Eastern Mediterranean Sea. Vegetative cells of strain 21D showed the ability to grow in the presence of high concentrations of MgCl2, such as 14.28% corresponding to 1.5 M. Biolog phenotype MicroArray (PM) was adopted to investigate the strain phenotype, with reference to carbon energy utilization and osmotic tolerance. The strain was able to metabolize only 8.4% of 190 carbon sources provided in the PM1 and PM2 plates, mainly carbohydrates, in accordance with the low availability of nutrients in its habitat of origin. By using in silico DNA-DNA hybridization the analysis of strain 21D genome, assembled in one circular contig, revealed that the strain belongs to the species Virgibacillus dokdonensis. The genome presented compatible solute-based osmoadaptation traits, including genes encoding for osmotically activated glycine-betaine/carnitine/choline ABC transporters, as well as ectoine synthase enzymes. Osmoadaptation of the strain was then confirmed with phenotypic assays by using the osmolyte PM9 Biolog plate and growth experiments. Furthermore, the neutral isoelectric point of the reconstructed proteome suggested that the strain osmoadaptation was mainly mediated by compatible solutes. The presence of genes involved in iron acquisition and metabolism indicated that osmoadaptation was tailored to the iron-depleted saline waters of the Discovery SBI. Overall, both phenomics and genomics highlighted the potential capability of V. dokdonensis 21D vegetative cells to adapt to the environmental conditions in Discovery SBI.
Introduction
Microorganisms inhabit all the habitats present on Earth, including those environments indicated as “extreme” and characterized by strong selective physico-chemical forces, e.g., polar seas, cold and hot deserts, and hydrothermal vents. These extreme environments represent a fascinating source of bacterial diversity and metabolic activities with interesting biotechnological potential (De Vitis et al., 2015; Raddadi et al., 2015, 2018).
Microorganisms living in extreme habitats, namely “extremophiles”, show metabolic and physiological adaptations to their environmental conditions. For instance, they can possess atypical enzymes that are adapted to the extreme conditions (Mapelli et al., 2012; Gunde-Cimerman et al., 2018). The enzymatic adaptation to high-osmolarity stress, included in the so-called “salt-in” strategy, is typical of those microorganisms that adjust their cell turgor pressure in order to avoid any water loss. Examples include the extremely halophilic strains of the Euryarchaeota order of Halobacteriales and the Bacteroidetes bacterium Salinibacter ruber. Conversely, microbial cells can respond to the high-osmolarity stress using a different strategy, known as “low-salt-in” or “compatible solute” strategy, which consists of the intracellular accumulation or uptake of compatible solutes from the surrounding environment in order to balance the cytoplasmic content with the outside (Oren, 2008).
It is generally thought that when unfavorable conditions are present in a specific environment, the inhabiting or transient microbial cells can enter the state of “dormancy” in order to thrive once the stressful conditions are overcome (Lennon and Jones, 2011). Harsh conditions that encourage dormancy include lack of resources, high residence time, presence of predators, occurrence of a perturbation regime and stresses, such as high temperature, desiccation, high UV irradiation, and chemical damage (Lennon and Jones, 2011). Endospores are one of the dormancy expressions, fundamental for the persistence of a certain cell population or genotype with consequent important repercussions at the community and ecosystem levels (Lennon and Jones, 2011). Nevertheless, spore production represents a costly involvement for sporeformers, since it involves not only bacterial genome replication, but also the biosynthesis of cell structures, e.g., protective layers, as well as the death of the mother cell. Moreover, authors have described that under high salinity stress Bacillus subtilis cells can block the entry into the sporulation pathway in order to prevent the osmotically damaged cells committing to a developmental program that they cannot complete (Kunst and Rapoport, 1995; Ruzal et al., 1998; Widderich et al., 2016).
Deep hypersaline anoxic basins (DHABs) have attracted particular interest from researchers and several basins have been discovered in the Eastern Mediterranean Sea, e.g., L’Atalante, Bannock, Discovery, Urania, Thetis, and Kryos (Mapelli et al., 2016; Barozzi et al., 2018; Merlino et al., 2018). These basins are highly saline lakes located on the seafloor, at around 3000 m below sea level, characterized by anoxia, high hydrostatic pressure and a sharp chemocline at the seawater-brine interface (SBI) (van der Wielen et al., 2005; Daffonchio et al., 2006; Yakimov et al., 2007; Borin et al., 2009). Each brine pool has a peculiar chemical composition that selects specialized prokaryotic communities (van der Wielen et al., 2005). For example, the Discovery basin is characterized by the presence of a high concentration of MgCl2 (5 M) and it has been indicated as one of the most extreme environments on Earth (Hallsworth et al., 2007; Lee et al., 2018; Steinle et al., 2018; La Cono et al., 2019). Discovery basin was formed 2000 years ago by the dissolution of evaporates made of bischofite [(MgCl2)⋅H2O] (Wallmann et al., 1997) and its SBI is characterized by a steep MgCl2 gradient ranging from the value of seawater, i.e., 0.48% (0.05 M), to that of the brine, i.e., 48.08% (5.05 M) (Hallsworth et al., 2007). MgCl2 is a remarkably chaotropic salt, able to reduce the enzymatic activity of glucose-6-phosphate dehydrogenase in laboratory conditions by 80 and 95% when present at concentrations of 1.90% (0.2 M) and 3.81% (0.4 M), respectively (Hallsworth et al., 2007). Experiments of enzymatic inactivation, performed using samples from Discovery brine and SBI instead of MgCl2 pure solutions, also displayed similar results (Hallsworth et al., 2007). Due to the chaotropicity of MgCl2 with the absence of other compensating ions, the high MgCl2 concentration has been suggested to create conditions unsuitable for life (Hallsworth et al., 2007), although the recent study on the microbial community thriving in Kryos brine pool extended the chaotropicity limit of life (Yakimov et al., 2015). Furthermore, although DHABs are generally regarded as aggressive environments for cells and macromolecules, naked DNA has been shown to be preserved in this habitat, maintaining its transforming potential (Borin et al., 2008; Yakimov et al., 2015).
The adaptation of bacteria able to produce endospores, i.e., sporeformers, to extreme environmental conditions is frequently questioned, since highly resistant spores are generally considered as resting contaminants, not representing populations adapted to the ecosystem. Here, in order to verify this assumption, we investigated the phenotypic and genomic characteristics of Virgibacillus sp. strain 21D, isolated from the SBI of the DHAB Discovery located in the Eastern Mediterranean Sea (De Vitis et al., 2015).
Materials and Methods
Bacterial Strain and Cultivation Media
Virgibacillus sp. strain 21D was isolated on 246 DSM medium from a SBI sample collected from the Discovery (35°17′ N, 21°41′ E) DHAB (De Vitis et al., 2015). Briefly, serial dilutions of the Discovery interface (1 ml) sample were plated on 246 DSM medium added with cycloheximide 100 μg/ml and incubated at 30°C until the appearance of bacterial colonies. One colony, streaked three times to ensure the purity, was labeled with name 21D and subjected to genotypic characterization and identification, as described by De Vitis et al. (2015). Strain 21D is routinely maintained on marine broth (MB) medium (Conda) by incubation for 48 h at 30°C. Gram staining was performed following the standard Gram procedure. Growth optimum at different NaCl (0, 3, 6, 9, and 12%) and MgCl2 (0, 0.95, 4.76, 9.52, 11.43, 14.28, and 17.14%) concentrations was tested on Plate Count Broth (PCB; supplemented with 6% NaCl in case of the growing tests with MgCl2) by using 96-well microplate. Bacterial growth in 96-well microplate at 30°C was monitored at 610 nm by using a microplate reader (TECAN Infinite Pro200). Elaboration of the retrieved data was performed using Excel software. MgCl2 percentage at which the growth curve showed the higher slope value was considered as optimum MgCl2 concentration. Similarly, the growth of strain 21D in the presence of different osmolytes, i.e., betaine (0.5, 1, 2, 5, and 10%), choline (0.5, 1, 2, 5, and 10%), ethylene glycol (5, 10, 20, and 50%), and potassium chloride (3, 6, 9, and 12%), was monitored in 96-well microplates at 30°C in PCB supplemented with 6% NaCl by using a microplate reader (TECAN Infinite Pro200). Chitinolytic ability of the strain was determined by growth on MB agar plates supplemented with 7% colloidal chitin for 10 days at 30°C (Raddadi et al., 2009).
Scanning Electron Microscopic Analysis
Cells of Virgibacillus sp. strain 21D were filtered onto 0.1 μm polycarbonate Whatman filters (Nucleopore) before fixation with 2.5% glutaraldehyde in 0.1 M cacodylate buffer. Filters were washed in 0.1 M cacodylate buffer and subsequently post-fixed in osmium tetroxide. Samples were washed with an ethanol gradient (20–100% ethanol) and subject to critical point drying (Autosamdri-815B, Tousimis). Filters were coated with a 5 nm layer of Au/Pb using a K575X sputter coater (Quorum) and images were acquired using a Quanta 600 FEG (Thermo Scientific) scanning electron microscope at an acceleration voltage of 5 kV.
Biolog Phenotype MicroArray (PM)
All the materials and reagents used were purchased from Biolog (Hayward, CA, United States). Plates PM1-2, assessing the phenotypes of different carbon sources, and plate PM9, testing for osmotic/ion and pH effects, were used. Strain 21D isolate was streaked on MB agar plates and incubated at 30°C in darkness for 48 h. Cells were scraped from the surface of the plates and inoculated in 20 ml 0.9% NaCl to reach 81–85% transmittance of the cell suspension. For PM1 and PM2, 1.76 ml of cell suspension were added to 20 ml of M9 medium without C sources (12.8 g/L Na2HPO4, 3 g/L KH2PO4, 0.5 g/L NaCl, 1 g/L NH4Cl, 0.24 g/L MgSO4, 0.01 g/L di CaCl2) added with 5% NaCl, together with 0.24 ml of Dye F and 2 ml of PM1-2 additive solution. PM9 was inoculated with an inoculating fluid composed by 10 ml of IF-10b GN/GP, 0.88 ml of cell suspension, 0.12 ml of Dye F and 1 ml of PM additive solution. All the plates were run in duplicates and incubated in the OmniLog incubator for 96 h (PM1–2) or 72 h (PM9) at 30°C. Data was collected every 15 min by the OmniLog incubator and analyzed using Kinetic and Parametric software (Biolog). Phenotype responses were analyzed observing the presence of area under the kinetic curve or reporting the highest point reached by the strain growth curve (PM9). Assays in well A01 for PM1 and PM2 was a negative control.
DNA Preparation
Genomic DNA was prepared from an overnight culture of Virgibacillus sp. strain 21D in MB medium at 30°C (150 rpm) using DNeasy Blood & Tissue Kit (Qiagen, Italy) following the manufacturer’s protocol for Gram-positive bacteria. Quantification and quality control of the DNA was performed by spectrophotometry and agarose (0.8%) gel electrophoresis.
Whole Genome Sequencing, Assembly, and Annotation
The genome of Virgibacillus sp. strain 21D was sequenced using PacBio (Pacific Biosciences, CA, United States) technology at Macrogen (South Korea). Specifically, a library with size of 10 kbp was prepared and 1 SMRT cell used for sequencing. Raw reads were filtered and de novo assembled using FALCON Assembler software (v0.2.2 release, January 2016) (Koren and Phillippy, 2015). After whole genome assembly, the complete genome of Virgibacillus sp. strain 21D was annotated using the pipeline Prokka (Seemann, 2014). The complete genome sequence was deposited in GenBank under the accession number CP018622, BioProject PRJNA354837, BioSample SAMN06052362.
Taxonomic Position of Strain 21D
We used the GTDB-Tk software1 to define the taxonomic position of the strain 21D in the newly described Genome Taxonomy Database (GTDB, Parks et al., 2018). The analysis was performed by concatenating 120 single copy genes present in the reference genomes (accession numbers are reported in Table 1), and in the genome of strain 21D.
In silico DNA–DNA hybridization (digital DDH, dDDH) was computed using the recommended settings of the Genome-to-Genome Distance Calculator (GGDC) web server version 2.1 (Meier-Kolthoff et al., 2013, 2014) available at the website http://ggdc.dsmz.de/. Closest relative species and bacterial species included in Virgibacillus genus were considered.
Determination of the Proteome Isoelectric Point
Genome sequence of Virgibacillus sp. strain 21D was used to infer the isoelectric point (pI) of the proteome by means of the expasy server (Gasteiger et al., 2005). The pI of strains Escherichia coli DSM 30083, V. pantothenticus DSM 26T, V. chiguensis NTU-101T and V. dokdonensis Marseille-P2545, V. halodenitrificans JCM 12304, S. ruber DSM 13855, Desulfohalobium retbaense DSM 5692 were also computed for comparison purposes.
Results
Phenotypic Characterization
Virgibacillus sp. strain 21D is a Gram-variable, motile, and spore-forming bacterium with rod shaped cells (Figure 1A and Supplementary Figure 1). When grown on MB agar plates at 30°C in aerobic conditions, it forms 1–2 mm colonies within 48 h. The isolate can grow at 25, 30, 37, 42, and 50°C. The cells show an average width of 0.4 μm and length of 1.5 μm, as confirmed by scanning electron microscopy (Figure 1A; Heyrman et al., 2003). We observed the capability of cells to form long filamentous chains (Figure 1A), consistent with previous observations of Virgibacillus spp. strains (Yoon et al., 2005; Wang et al., 2008, 2015). Growth was observed in (i) PCB medium containing NaCl in concentrations ranging from 3 to 10% with optimal growth value between 6 and 10% (also considering Biolog experiments); and (ii) in PCB (added with 6% NaCl) containing MgCl2 in concentrations ranging between 0 and 17.14% (Figure 1B). Specifically, the strain 21D was able to grow at high concentrations of MgCl2, i.e., 11.43 and 14.28%, which corresponded to 1.2 and 1.5 M MgCl2. Even in the presence of 17.14% (1.8 M) MgCl2, we verified low growth of the strain 21D, which peaks after 20 h of incubation, followed by a sharp decrease of the optical density (OD) (Figure 1B). Low concentrations of MgCl2, i.e., 0.95 and 4.76% (0.1 and 0.5 M), are required to reach high OD values if compared with 0% MgCl2 growth (Figure 1B).
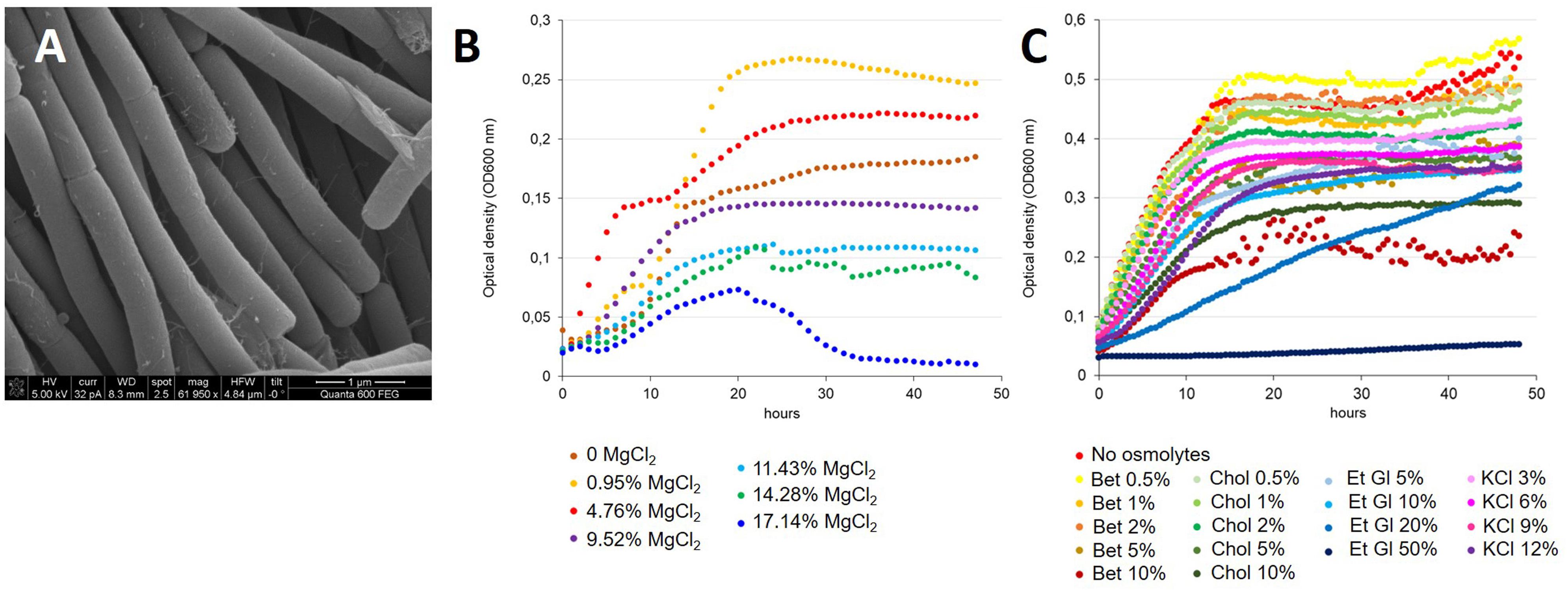
Figure 1. Morphology (A) and growth (B,C) of V. dokdonensis strain 21D. In (A), SEM image of strain 21D. In (B), strain 21D growth in presence of increasing concentrations of MgCl2. In (C), strain 21D growth in presence of increasing concentrations of different osmolytes. Bet, betaine; Chol, choline; Et Gl, ethylene glycol; KCl.
Strain 21D was also grown in the presence of different concentrations of the following osmolytes: betaine, choline, ethylene glycol and KCl (Figure 1C). In PCB 6%NaCl, strain 21D showed the ability to grow similarly to the positive control when 0.5, 1, or 2% betaine was added to the medium, whereas at concentrations of 5 and 10% betaine a slight decrease in OD values (more marked with 10% betaine) occurred. A similar trend was found in the presence of choline, whereas KCl addition did allow the strain to grow well, although at lower OD values than in the absence of osmolytes in the growth medium (line in red in Figure 1C). In presence of 20 and 50% ethylene glycol, a reduced growth and a strong inhibition of strain 21D were found respectively, while the addition of 5 and 10% of the osmolyte did slightly affect the strain growth.
Vegetative cells of strain 21D were able to metabolize 8.4% of the tested carbon sources (8 out of 95 in plate PM1 and 8 out of 95 in plate PM2) (Table 2). They showed the capability to metabolize glucose, ribose, arbutin, tagatose, and glucosamine, while they were not able to hydrolyse chitin in the adopted experimental conditions. Plate PM9 was used to test the bacterial metabolic activity in presence of different stress conditions. We confirmed the strain activity in presence of NaCl (with the optimal activity between 6 and 10% NaCl), as well as in presence of different osmolytes and ions (Table 3 and Supplementary Figure 2). We detected a reduction of the bacterial metabolism in certain cases, i.e., in presence of 1–4% NaCl, 2% sodium sulfate, 5–20% ethylene glycol, 1–2% sodium formate, 2% urea, 10–100 mM ammonium sulfate (pH 8.0) and 10–100 mmol/L sodium nitrate. A strong reduction was also detected with 3–7% urea, 20–200 mM sodium benzoate (pH 5.2) and 10–100 mM sodium nitrite (Supplementary Figure 2).
Genome Sequencing and Identification
A total number of 117,330 reads were retrieved with a mean subread length of 8,461 bp and N50 of 11,849 bp. The genome showed a GC% of 36.6, with 3,944 CDS, 63 tRNA, and 18 rRNA. In Table 4 we provide detailed information related to the complete genome of Virgibacillus sp. strain 21D. One circular chromosome of 4,263,520bp in size was obtained after assembly (Figure 2A). Number of genes associated with general COG functional categories are reported in Table 5.
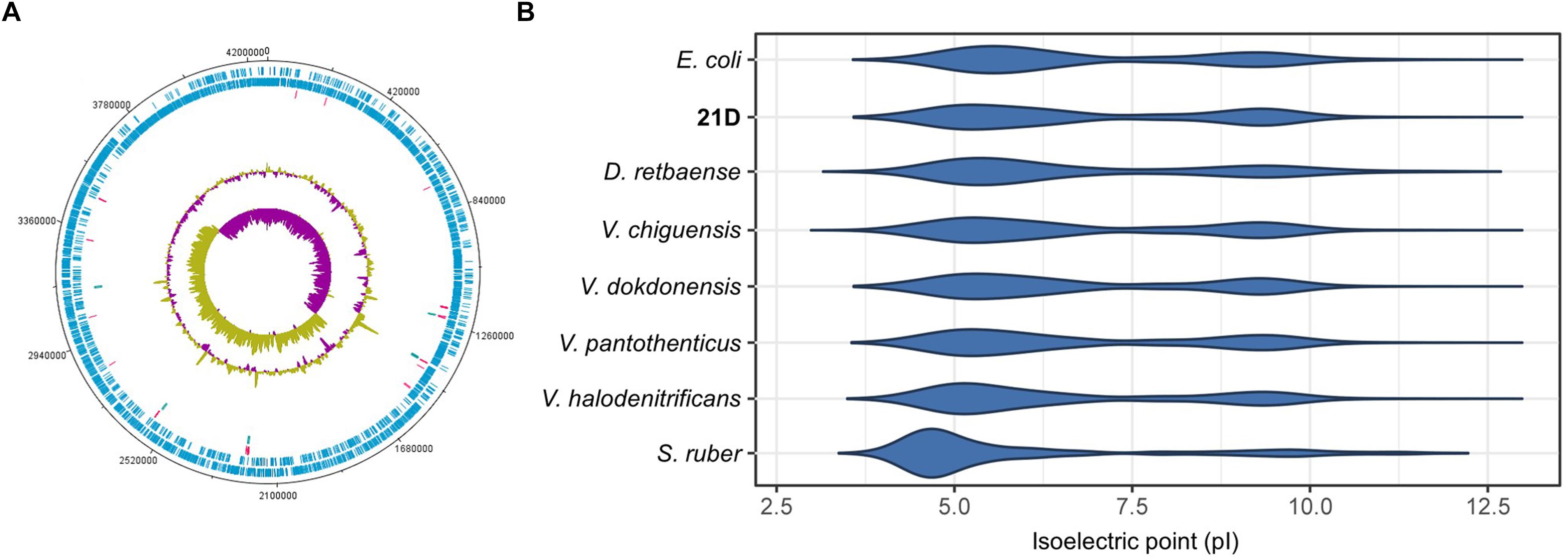
Figure 2. Circular map of the genome (A) and isoelectric point of the proteome (B) of V. dokdonensis strain 21D. In (A), marked characteristics are shown from outside to the center: CDS on forward strand, CDS on reverse strand, tRNA, rRNA, GC content and GC skew. In (B), are reported the isoelectric point of the proteome of E. coli DSM 30083, V. pantothenticus DSM 26T, V. chiguensis NTU-101T, V. dokdonensis Marseille-P2545, V. halodenitrificans JCM 12304, S. ruber DSM 13855, D. retbaense DSM 5692.
By concatenating 120 single copy genes present in the reference genomes (see accession numbers in Table 1), as well as in the genome of strain 21D (GTDB software, Parks et al., 2018) and through in silico DNA–DNA hybridization (dDDH, Table 6), strain 21D was phylogenetically classified as V. dokdonensis (Figure 3). The previous assignment (based on 800 bp-fragment of the 16S rRNA gene) of the strain 21D to the V. pantothenticus species as reported by De Vitis et al. (2015) was not supported. The analysis showed congruency with the taxonomic affiliation of the strain based on almost full-length 16S rRNA gene (Supplementary Figure 3).
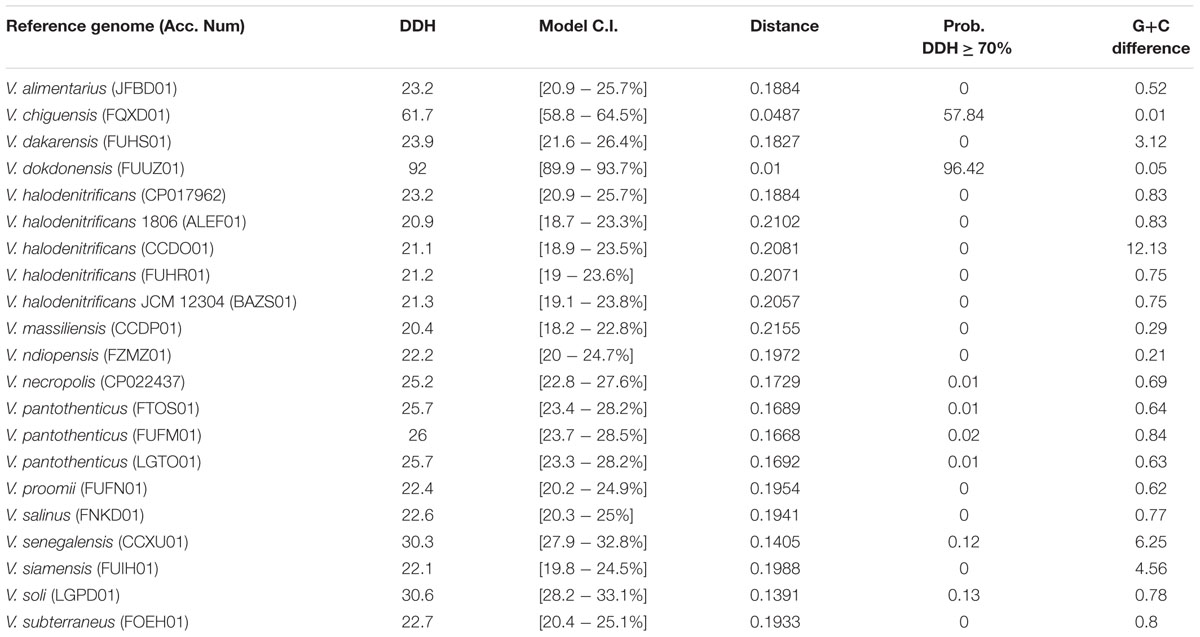
Table 6. In silico DNA–DNA hybridization analysis by using Genome sequence-based (sub-)species delineation (GGDC) server (http://ggdc.dsmz.de/).
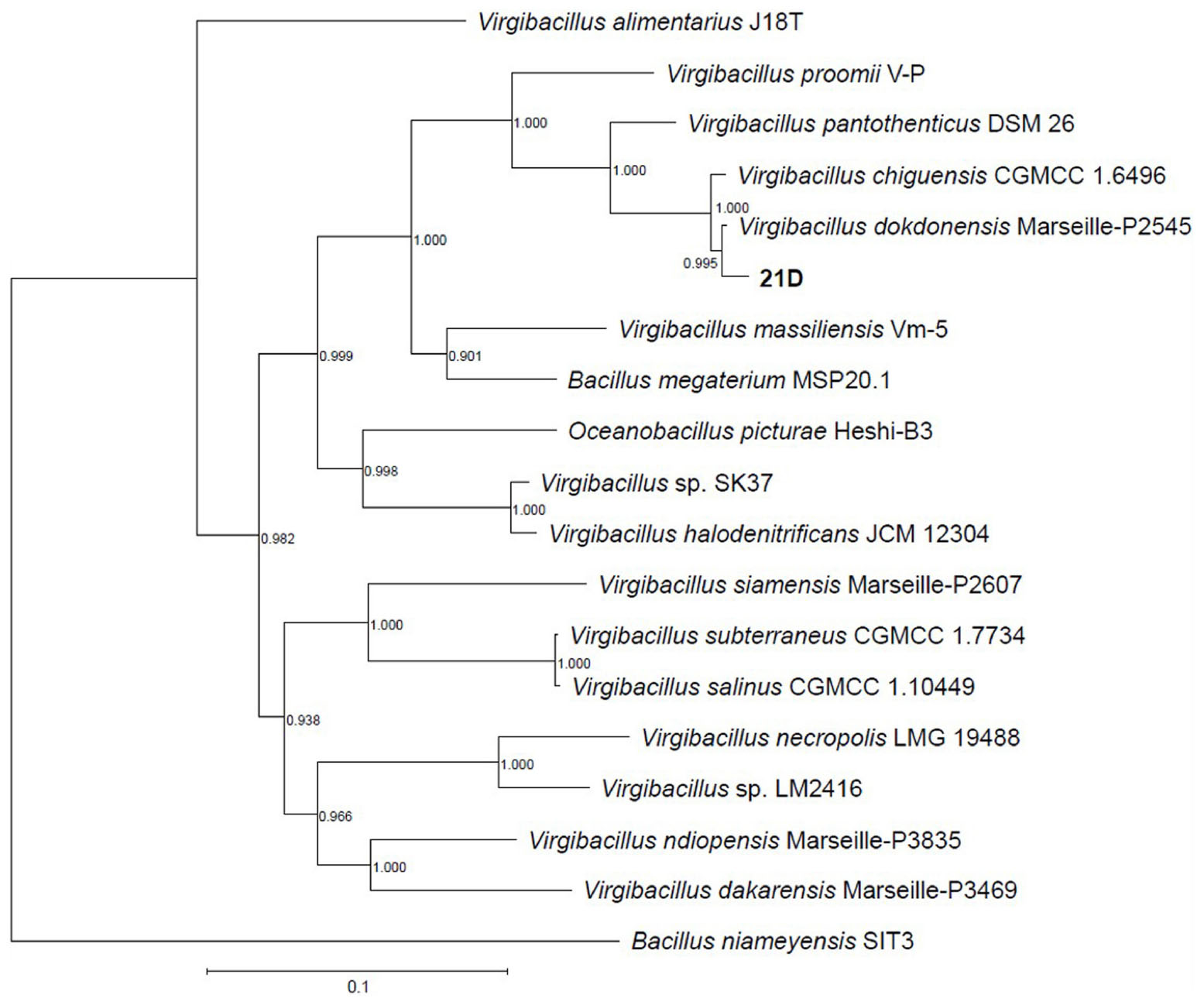
Figure 3. Taxonomic affiliation V. dokdonensis strain 21D. The phylogenetic tree was performed by using GTDB-Tk software, which applies FastTree to a concatenated alignment of 120 ubiquitous single-copy genes. Numbers at the nodes are bootstrap values obtained by repeating the analysis 100 times.
Insights Into Virgibacillus sp. Strain From Genomics
Automatic annotation using “Rapid Annotation using Subsystem Technology” (RAST) platform2 (Aziz et al., 2008; Overbeek et al., 2014; Brettin et al., 2015) showed that V. dokdonensis strain 21D genome contains genes that could help the strain to thrive under the osmotic stress typical of extreme environments. For example, we observed the presence of the glycine betaine transporter OpuD (A21D_00070; A21D_00685; A21D_00747; A21D_00835; A21D_02157; A21D_03789), the glycine betaine/carnitine/choline ABC transporter OpuC (A21D_01624; A21D_01625; A21D_01626; A21D_01627; A21D_03467; A21D_03468) and the glycine betaine/carnitine ABC transporter Gbu (homologous to OpuA of B. subtilis; A21D_02196; A21D_02672; A21D_02673; A21D_02674; A21D_02675) (Hoffmann and Bremer, 2011). Moreover, L-carnitine/gamma-butyrobetaine antiporters (A21D_02627; A21D_02815) and ectoine production CDS (A21D_03919; A21D_03920; A21D_03921) were retrieved. In particular, we compared the nucleotide sequence of ectABC operon of the strain 21D with that of V. pantothenticus DSM 26T, demonstrating an identity of 82% (395/480bp), 81% (1040/1284 bp), and 83% (323/387 bp) with EctA (diaminobutyric acid acetyltransferase, A21D_03921), EctB (diaminobutyric acid transaminase, A21D_03920), and EctC (ectoine synthase, A21D_03919) sequences, respectively. Finally, due to the ability of strain 21D to thrive at high MgCl2 concentrations, the presence of magnesium transporters was investigated and four CDS (A21D_01697, A21D_01947, A21D_02760, and A21D_02928) were unveiled from the genome annotation. The first two CDS encode for CorC protein, whereas the other CDS encode for MgtE transporters.
Taking advantage of RAST function-based comparison tool, we compared the genome of strain 21D with those of the closely related species V. pantothenticus DSM 26T, V. chiguensis NTU-101T and V. dokdonensis Marseille-P2545, available on the NCBI database (Wang et al., 2008, 2015). We found the basic metabolic functions to be conserved between the four genomes. Moreover, all of them showed the presence of genes involved in osmotic stress response, while they differentiated for genes in the category “Iron acquisition and metabolism.” In V. dokdonensis strain 21D genome we found the presence of genes in the subcategories “siderophore metabolism” and “heme, hemin uptake and utilization systems in Gram-positives bacteria” that have not been consistently found in V. pantothenticus, V. dokdonensis, or V. chiguensis genomes. Specifically, genes related to iron uptake mediated by the siderophore petrobactin were only found in the genome of the strain 21D (in 21D: A21D_01225; A21D_01226; A21D_01227; A21D_01228, encoding for petrobactin ABC transporter components). Conversely, genes of the iron-regulated surface determinant system Isd have been only found in V. dokdonensis (21D and Marseille-P2545) genomes, but not in V. pantothenticus and V. chiguensis ones (in 21D: from A21D_01190 to A21D_01200; A21D_01095; A21D_01096; Table 7).
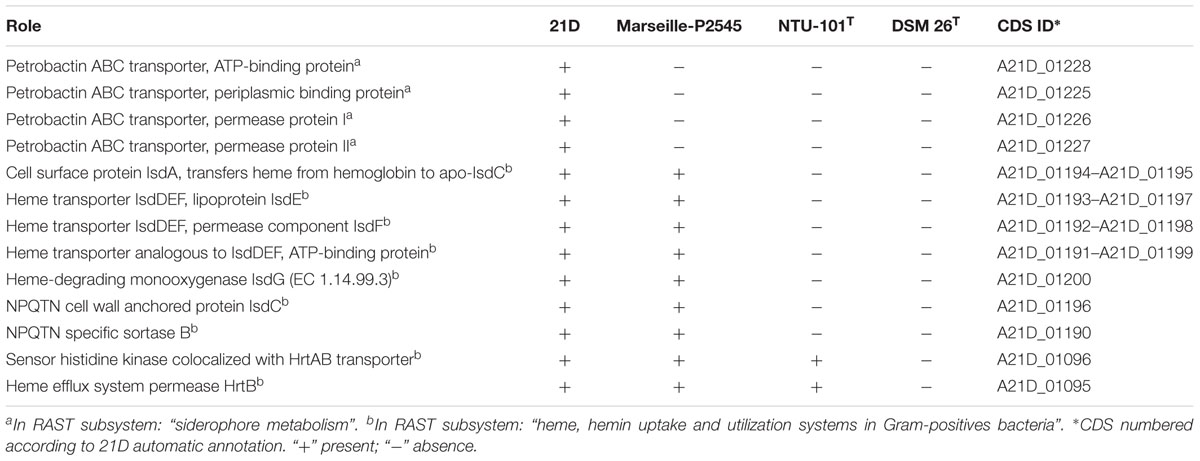
Table 7. Comparison among selected functions in V. dokdonensis 21D, V. dokdonensis Marseille-P2545, V. chiguensis NTU-101T, and V. pantothenticus DSM 26T by using automatic RAST function-based comparison tool.
Calculation of the isoelectric point (pI) of strain 21D proteome showed that the strain possesses a proteome pI different to the acid one shown by S. ruber, which was included in our analysis as a reference strain of the “salt-in” strategy microorganisms (Figure 2B; Oren and Mana, 2002; Oren, 2008). The pI of 21D proteome is congruent with that of E. coli or the halophilic strain D. retbaense (Spring et al., 2010). Moreover, we observed that the Virgibacillus strains included in our analysis showed highly similar and neutral proteome pIs (Figure 2B).
Discussion
The ability of the strain 21D to grow in the presence of MgCl2 concentrations as high as 11.43–14.28% (1.2–1.5 M) is indicative of its peculiar origin of isolation, i.e., the Discovery SBI, characterized by a steep MgCl2 gradient ranging from the value of seawater (0.05 M) to that of the brine (5.05 M) (Hallsworth et al., 2007). Authors have previously reported the inability to isolate and grow bacterial strains from different Discovery SBI sample fractions by using media containing above 12% (1.26 M) MgCl2 (Hallsworth et al., 2007) and this underlines a characteristic behavior of strain 21D. Moreover, a slight (even if poor) growth of the strain was even reported at 17.14% (1.8 M) MgCl2. There are several examples of haloarchaeal strains that require high concentrations of magnesium for their growth in the literature, including Halobacterium salinarum DSM 3754T, Halobaculum gomorrense DS2807T, Haloferax volcanii DS2T and Halobaculum magnesiiphilum MGY-184T (Mullakhanbhai and Larsen, 1975; Oren et al., 1995; Grant, 2001; Shimoshige et al., 2013). For instance, authors reported that H. magnesiiphilum is able to grow in media with 30% (1.48M) MgCl2.6H2O (Shimoshige et al., 2013).
Three different classes of Mg2+ transporters have been identified in bacteria, namely CorA, MgtE, and MgtA (Groisman et al., 2013). Bacterial genomes can generally encode multiple Mg2+ transporters that belong to either the same or different classes. CorA and MgtE are the primary magnesium transporters in bacteria and archaea (likely being channels rather than transporters, Groisman et al., 2013) and they, indeed, show a wide phylogenetic distribution. Conversely, MgtA proteins occur only in some bacteria and are typically induced in low Mg2+ environments. In the genome of strain 21D, no mgtA genes were annotated, whereas two CDS for both CorA and MgtE were retrieved. Since Mg2+ plays several essential roles, e.g., acting as a cofactor in enzymatic reactions and stabilizing macromolecular complexes and membranes, the maintenance of a correct Mg2+ homeostasis is fundamental for cell functioning: several studies have shown that bacteria assess the levels of Mg2+ present in their surrounding environment or inside the cytoplasm to regulate Mg2+ at the required levels (Groisman et al., 2013).
Phenotypic analysis of 21D suggested a limited metabolic capability of the strain, likely underlying a narrow adaptation of the bacterium to the available C sources present in Discovery SBI. In particular, besides the simple carbon molecules of D-mannose, D-ribose, D-fructose, D-glucose, D-tagatose, and glycerol which are substrates for conserved core metabolic pathways, strain 21D showed an active metabolism on oligomers/polymers with linked α-(1→4) or α-(1→6) glycosidic bonds, i.e., dextrin, maltose, and maltotriose, and β-(1→3) or β-(1→6) glycosidic ones, specifically laminarin. Laminarin is a storage polysaccharide produced by different algal species and one of the most abundant polysaccharides present in the marine environment. It has been estimated that diatoms alone can produce about 5–15 Gt of laminarin per year, playing a pivotal role in the marine carbon cycle (Alderkamp et al., 2007). Laminarin has been detected in shallow waters, being typically associated with microalgal blooms (Unfried et al., 2018), as well as in deeper waters and sediments (Keith and Arnosti, 2001; Arnosti et al., 2005; Teske et al., 2011), but there is no literature concerning the typical depth of the Mediterranean Sea’s DHABs. This might suggest that sinking material from the upper seawater layer can reach Discovery SBI and be available to strain 21D. Furthermore, the strain showed active metabolism on pectin (a component of algal cell walls; Domozych et al., 2012), on the amino-sugar D-glucosamine, but not on chitin (a polymer present in crustacean exoskeletons). We also observed positive results for arbutin, a glycosylated hydroquinone, which is typically extracted from terrestrial plants, likely due to a broad, non-specific enzymatic activity of the strain on this substrate. Finally, strain 21D was active on inositol, a compatible solute that can be accumulated by other extremophiles (Goncalves et al., 2008), and on acetoin, a fermentation product that can be also produced by marine cognates, representing a C source for bacterial growth under unfavorable conditions (Dai et al., 2015).
Regarding carbon metabolism, we retrieved the presence of two β-glucosidases in multiple copies from the genome annotation, i.e., EC 3.2.1.86 (A21D_00222, A21D_00423, A21D_00900, A21D_02186, and A21D_02527) and EC 3.2.1.21 (A21D_00998, A21D_01912; A21D_02532), which catalyze the hydrolysis of phospho-β-D-glucosides and terminal, non-reducing β-D-glucosyl residues, respectively. As well, α-glucosidases, specifically maltose-6-phosphate α-glucosidases (EC 3.2.1.122), oligo-1.6 α-glucosidases (EC 3.2.1.10), and α-glucosidases (EC3.2.1.20), were automatically annotated, underlining the strain capability to metabolize α-linked or β-linked glycosidic bonds.
The genome of strain 21D encodes for genes that could be helpful under the osmotic stress typically encountered in DHAB extreme environments and, indeed, analysis with PM9 plates and growth experiments in the presence of osmolytes unveiled the osmoadaptation capacity of the strain. In order to adjust the cell turgor pressure, the majority of halophilic bacteria generally synthetize or uptake different osmoprotectants from the surroundings, such as ectoine, choline, carnitine and betaine (Oren, 2008). In B. subtilis glycine betaine has a very important role in osmoprotection, accumulating via uptake or being synthetized from the precursor choline (Hoffmann and Bremer, 2011). Specifically, three osmotically inducible transport systems have been depicted in B. subtilis: the ABC transporters OpuA and OpuC and the carrier OpuD, which is a member of the betaine-choline-carnitine transporter (BCCT) family. These transporters can also function for the acquisition of different osmoprotectants other than glycine betaine (Hoffmann and Bremer, 2011). Genome annotation of strain 21D revealed the presence of the abovementioned transporter systems. Interestingly, in B. subtilis Opu transporters have been described to contribute to glycine betaine (and other osmoprotectants such as carnitine) accumulation in cold conditions (Hoffmann and Bremer, 2011; Meadows and Wargo, 2015). Moreover, we also found the presence of the highly conserved ectABC gene cluster (Kuhlmann et al., 2008) responsible for ectoine production, which showed high identity with the gene cluster identified in V. pantothenticus DSM 26T. Specifically, authors underlined that the production of ectoine in DSM 26T is activated when an increase of salinity or a reduction of the growth temperature occur (Kuhlmann et al., 2008). Further experiments are needed to verify and elucidate ectoine production by strain 21D in response to osmotic stress. The strain V. halodenitrificans PDB-F2 also showed the ability to synthesize or uptake ectoine, hydroxyectoine, trehalose, glutamic acid and betaine in presence of 12% (w/v) NaCl (Zhou et al., 2017). Although no reports are available on compatible solutes produced or acquired by strains belonging to V. dokdonensis species and considering our results and the available literature, we can hypothesize that this is a shared feature of V. dokdonensis–V. pantothenticus related species. Growth experiments of strain 21D with ethylene glycol and KCl showed the same trend of Biolog microarray results with good growth performances in the presence of KCl and a slight growth reduction with 20% of ethylene glycol. Interestingly, 50% ethylene glycol strongly inhibited strain 21D, whereas the high concentrations of 9 and 12% KCl did slightly influenced the strain growth.
The comparison of Virgibacillus spp. genome sequences suggests the ability of strain 21D to overcome the iron depletion typical of marine environments (Butler, 2005). Iron is a fundamental element for cell functioning, since it is an essential cofactor in enzymes that catalyze key processes, such as photosynthesis, respiration, and nitrate reduction (Hogle et al., 2014). Given its low concentration in seawater (Butler, 2005), marine bacteria have evolved different strategies to uptake this important element. They can, indeed, secrete or uptake siderophores (including those that are produced by other organisms), i.e., low molecular weight molecules with high affinity for insoluble Fe3+ ions, able to form complexes that are further absorbed by cells (de Carvalho and Fernandes, 2010). Genome analysis suggests that the strain 21D has a siderophore-mediated iron acquisition system, based on ABC transporter components and other membrane-associated proteins (de Carvalho and Fernandes, 2010). Among the bacterial genomes analyzed, only that of strain 21D possessed CDS encoding for this ability. On the other hand, genomes of both V. dokdonensis strains 21D and Marseille-P2545 have a gene cluster encoding for the iron-regulated surface determinant (Isd), which is one of the most studied iron uptake systems used by Gram positive bacteria to obtain iron from heme (Mazmanian et al., 2003). Heme and related molecules may be important sources of iron for marine organisms (Hogle et al., 2014). So far, some studies have investigated the abundance of iron protoporphyrin IX (heme b) in marine organisms and environments, i.e., phytoplankton, particulate material sampled in the North Atlantic Ocean and a mesocosm experiment set up in a Sweden fjord (Honey et al., 2013; Bellworthy et al., 2017). Specifically, heme b may represent 20% of the cellular iron pool of marine phytoplankton (Honey et al., 2013). Heme uptake systems have been thus searched and retrieved from genomes of marine strains (Hopkinson et al., 2008; Roe et al., 2013; Hogle et al., 2014), but the attention has been mainly devoted to the groups Proteobacteria, Bacteroidetes and Cyanobacteria, as well as a few Archaea (Hopkinson and Barbeau, 2012). No reports are available on the ability of marine Gram positive bacteria to utilize the iron contained in heme and heme-related molecules, even though this is a well-known ability exerted by Gram positive bacterial pathogens, such as Staphylococcus aureus (Mazmanian et al., 2003). This could suggest that in its original iron-depleted environment, the strain 21D could utilize heme to sustain its iron requirement. However, experimental verifications are needed to verify the ability of strain 21D to obtain iron both through iron-siderophore complexes and by utilizing heme.
The genus Virgibacillus was established in 1998 (Heyndrickx et al., 1998), while the amended description appeared in 2003 (Heyrman et al., 2003). To date, several Virgibacillus spp. genomes are available in the public databases, including the draft genomes of strain 21D-closely related species, i.e., V. pantothenticus DSM 26T (Wang et al., 2015), V. chiguensis CGMCC 1.6496T ( = NTU-101T) (Wang et al., 2008) and V. dokdonensis Marseille-P2545. Our analysis can thus contribute to enlarge the actual pool of genomic sequences available for the genus Virgibacillus, especially in the perspective to exploit the biotechnological potential associated with deep-sea microorganisms (Huang et al., 2018). Indeed, we have recently explored the bacterial diversity of DHABs located in the Eastern Mediterranean Sea, investigating the capability of different cultivable strains to resolve a racemic mixture of propyl ester of anti-2-oxotricyclo[2.2.1.0]heptan-7-carboxylic acid (R,S), a key intermediate for the synthesis of D-cloprostenol. Interestingly, strain 21D showed reduction of the substrate with medium/high enantioselectivity accordingly to NaCl concentration (De Vitis et al., 2015). A new transaminase was also found in strain 21D and verified to be secreted in a soluble form after the introduction of a single-mutation and the codon optimization for the expression in E. coli (Guidi et al., 2018).
Conclusion
Both phenomics and genomics highlight the potential capability of V. dokdonensis 21D vegetative cells to adapt to the environmental conditions occurring in Discovery SBI, revealing, in particular, a high degree of consistency for C source utilization and osmoadaptation. The presence of the genetic determinants involved in siderophore-mediated iron uptake and heme utilization further suggests the ability of the strain to thrive in iron-depleted marine habitats. In conclusion, our analysis supports the evidence that cells of V. dokdonensis 21D are equipped with genetic and phenotypic determinants to overcome the stressful conditions of Discovery SBI, possibly avoiding their entrance in the dormancy state, which could result in spore production.
Data Availability
The datasets generated for this study can be found in GenBank, CP018622.
Author Contributions
EC designed the study. ZZ, RM, JB, EP, FMa, MC, and MF carried out the experiments. ZZ, EC, RM, FMo, and GM analyzed the data. EC, SB, and DD supported the research. EC wrote the manuscript. All authors contributed to manuscript revision, read, and approved the final version of the manuscript for submission.
Funding
The research leading to these results received funding from the European Community’s Seventh Framework Programme (FP7/2007-2013) under grant agreement no. 311975 (MACUMBA) and from the EU Horizon 2020 Project contract number 634486 (INMARE). King Abdullah University of Science and Technology supported the study through the baseline research funds to DD.
Conflict of Interest Statement
The authors declare that the research was conducted in the absence of any commercial or financial relationships that could be construed as a potential conflict of interest.
Acknowledgments
EC acknowledges personal support from “Piano di Sostegno della Ricerca 2015-2017: Linea 2 – Dotazione annuale per attività istituzionali” in the project “Genetic manipulation of Marine bacteria to reveal specific genE function (GAME).”
Supplementary Material
The Supplementary Material for this article can be found online at: https://www.frontiersin.org/articles/10.3389/fmicb.2019.01304/full#supplementary-material
Footnotes
References
Alderkamp, A. C., van Rijssel, M., and Bolhuis, H. (2007). Characterization of marine bacteria and the activity of their enzyme systems involved in degradation of the algal storage glucan laminarin. FEMS Microbiol. Ecol. 59, 108–117. doi: 10.1111/j.1574-6941.2006.00219.x
Arnosti, C., Durkin, S., and Jeffrey, W. (2005). Patterns of extracellular enzyme activities among pelagic marine microbial communities: implications for cycling of dissolved organic carbon. Aquat. Microb. Ecol. 38, 135–145. doi: 10.3354/ame038135
Aziz, R. K., Bartels, D., Best, A. A., DeJongh, M., Disz, T., Edwards, R. A., et al. (2008). The RAST server: rapid annotations using subsystems technology. BMC Genomics 9:75. doi: 10.1186/1471-2164-9-75
Barozzi, A., Mapelli, F., Michoud, G., Crotti, E., Merlino, G., Molinari, F., et al. (2018). “Microbial diversity and biotechnological potential of microorganisms thriving in the deep-sea brine pools,” in Extremophiles. From Biology to Biotechnology, eds R. D. Durvasula and D. V. Subba Rao (Boca Raton, FL: CRC Press).
Bellworthy, J., Gledhill, M., Esposito, M., and Achterberg, E. P. (2017). Abundance of the iron containing biomolecule, heme b, during the progression of a spring phytoplankton bloom in a mesocosm experiment. PLoS One 12:e0176268. doi: 10.1371/journal.pone.0176268
Borin, S., Brusetti, L., Mapelli, F., D’Auria, G., Brusa, T., Marzorati, M., et al. (2009). Sulfur cycling and methanogenesis primarily drive microbial colonization of the highly sulfidic Urania deep hypersaline basin. Proc. Natl. Acad. Sci. U.S.A. 106, 9151–9156. doi: 10.1073/pnas.0811984106
Borin, S., Crotti, E., Mapelli, F., Tamagnini, I., Corselli, C., and Daffonchio, D. (2008). DNA is preserved and maintains transforming potential after contact with brines of the deep anoxic hypersaline lakes of the Eastern Mediterranean Sea. Saline Syst. 4:10. doi: 10.1186/1746-1448-4-10
Brettin, T., Davis, J. J., Disz, T., Edwards, R. A., Gerdes, S., Olsen, G. J., et al. (2015). RASTtk: a modular and extensible implementation of the RAST algorithm for building custom annotation pipelines and annotating batches of genomes. Sci. Rep. 5:8365. doi: 10.1038/srep08365
Butler, A. (2005). Marine siderophores and microbial iron mobilization. Biometals 18, 369–374. doi: 10.1007/s10534-005-3711-0
Daffonchio, D., Borin, S., Brusa, T., Brusetti, L., van der Wielen, P. W., Bolhuis, H., et al. (2006). Stratified prokaryote network in the oxic-anoxic transition of a deep sea halocline. Nature 440, 203–207. doi: 10.1038/nature04418
Dai, J.-Y., Cheng, L., He, Q.-F., and Xiu, Z.-L. (2015). High acetoin production by a newly isolated marine Bacillus subtilis strain with low requirement of oxygen supply. Process. Biochem. 50, 1730–1734. doi: 10.1016/j.procbio.2015.07.010
de Carvalho, C. C. C. R., and Fernandes, P. (2010). Production of metabolites as bacterial responses to the marine environment. Mar. Drugs 8, 705–727. doi: 10.3390/md8030705
De Vitis, V., Guidi, B., Contente, M. L., Granato, T., Conti, P., Molinari, F., et al. (2015). Marine microorganisms as source of stereoselective esterases and ketoreductases: kinetic resolution of a prostaglandin intermediate. Mar. Biotechnol. 17, 144–152. doi: 10.1007/s10126-014-9602-z
Domozych, D. S., Ciancia, M., Fangel, J. U., Mikkelsen, M. D., Ulvskov, P., and Willats, W. G. (2012). The cell walls of green algae: a journey through evolution and diversity. Front. Plant Sci. 3:82. doi: 10.3389/fpls.2012.00082
Gasteiger, E., Hoogland, C., Gattiker, A., Wilkins, M. R., Appel, R. D., and Bairoch, A. (2005). “Protein identification and analysis tools on the ExPASy server,” in The Proteomics Protocols Handbook, ed. J. M. Walker (New York, NY: Humana press), 571–607. doi: 10.1385/1-59259-890-0:571
Goncalves, L. G., Lamosa, P., Huber, R., and Santos, H. (2008). Di-myo-inositol phosphate and novel UDP-sugars accumulate in the extreme hyperthermophile Pyrolobus fumarii. Extremophiles 12, 383–389. doi: 10.1007/s00792-008-0143-0
Grant, W. D. (2001). “Genus I. Halobacterium Elazari-Volcani 1957, 207,AL emend. Larsen and Grant 1989, 2222,” in Bergey’s Manual of Systematic Bacteriology, 2nd Edn, Vol. 1, eds D. R. Boone, R. W. Castenholz, and G. M. Garrity (New York, NY: Springer), 301–305.
Groisman, E. A., Hollands, K., Kriner, M. A., Lee, E. J., Park, S. Y., and Pontes, M. H. (2013). Bacterial Mg2+ homeostasis, transport, and virulence. Annu. Rev. Genet. 47, 625–646. doi: 10.1146/annurev-genet-051313-051025
Guidi, B., Planchestainer, M., Contente, M. L., Laurenzi, T., Eberini, I., Gourlay, L. J., et al. (2018). Strategic single point mutation yields a solvent- and salt-stable transaminase from Virgibacillus sp. in soluble form. Sci. Rep. 8:16441. doi: 10.1038/s41598-018-34434-3
Gunde-Cimerman, N., Plemenitaš, A., and Oren, A. (2018). Strategies of adaptation of microorganisms of the three domains of life to high salt concentrations. FEMS Microbiol. Rev. 42, 353–375. doi: 10.1093/femsre/fuy009
Hallsworth, J. E., Yakimov, M. M., Golyshin, P. N., Gillion, J. L., Auria, D., de Lima, G., et al. (2007). Limits of life in MgCl2-containing environments: chaotropicity defines the window. Environ. Microbiol. 9, 801–813. doi: 10.1111/j.1462-2920.2006.01212.x
Heyndrickx, M., Lebbe, L., Kersters, K., De Vos, P., Forsyth, G., and Logan, N. A. (1998). Virgibacillus: a new genus to accommodate Bacillus pantothenticus (Proom and Knight 1950). Emended description of Virgibacillus pantothenticus. Int. J. Syst. Evol. Microbiol. 48, 99–106. doi: 10.1099/00207713-48-1-99
Heyrman, J., Logan, N. A., Busse, H. J., Balcaen, A., Lebbe, L., Rodriguez-Diaz, M., et al. (2003). Virgibacillus carmonensis sp. nov., Virgibacillus necropolis sp. nov. and Virgibacillus picturae sp. nov., three novel species isolated from deteriorated mural paintings, transfer of the species of the genus Salibacillus to Virgibacillus, as Virgibacillus marismortui comb. nov. and Virgibacillus salexigens comb. nov., and emended description of the genus Virgibacillus. Int. J. Syst. Evol. Microbiol. 53(Pt 2), 501–511. doi: 10.1099/ijs.0.02371-0
Hoffmann, T., and Bremer, E. (2011). Protection of Bacillus subtilis against cold stress via compatible-solute acquisition. J. Bacteriol. 193, 1552–1562. doi: 10.1128/JB.01319-10
Hogle, S. L., Barbeau, K. A., and Gledhill, M. (2014). Heme in the marine environment: from cells to the iron cycle. Metallonics 6, 1107–1120. doi: 10.1039/c4mt00031e
Honey, D. J., Gledhill, M., Bibby, T. S., Legiret, F.-E., Pratt, N. J., Hickman, A., et al. (2013). Heme b in marine phytoplankton and particulate material from the North Atlantic Ocean. Mar. Ecol. Prog. Ser. 483, 1–17. doi: 10.3354/meps10367
Hopkinson, B. M., and Barbeau, K. A. (2012). Iron transporters in marine prokaryotic genomes and metagenomes. Environ. Microbiol. 14, 114–128. doi: 10.1111/j.1462-2920.2011.02539.x
Hopkinson, B. M., Roe, K. L., and Barbeau, K. A. (2008). Heme uptake by Microscilla marina and evidence for heme uptake systems in the genomes of diverse marine bacteria. Appl. Environ. Microbiol. 74, 6263–6270. doi: 10.1128/AEM.00964-08
Huang, D., Shao, Z.-Z., Yu, Y., Cai, M.-M., Zheng, L.-Y., Li, G.-Y., et al. (2018). Identification, characteristics and mechanism of 1-Deoxy-N-acetylglucosamine from deep-sea Virgibacillus dokdonensis MCCC 1A00493. Mar. Drugs 16:52. doi: 10.3390/md16020052
Keith, S., and Arnosti, C. (2001). Extracellular enzyme activity in a river-bayshelf transect: variations in polysaccharide hydrolysis rates with substrate and size class. Aquat. Microb. Ecol. 24, 243–253. doi: 10.3354/ame024243
Koren, S., and Phillippy, A. M. (2015). One chromosome, one contig: complete microbial genomes from long-read sequencing and assembly. Curr. Opin. Microbiol. 23, 110–120. doi: 10.1016/j.mib.2014.11.014
Kuhlmann, A. U., Bursy, J., Gimpel, S., Hoffmann, T., and Bremer, E. (2008). Synthesis of the compatible solute ectoine in Virgibacillus pantothenticus is triggered by high salinity and low growth temperature. Appl. Environ. Microbiol. 74, 4560–4563. doi: 10.1128/AEM.00492-08
Kunst, F., and Rapoport, G. (1995). Salt stress is an environmental signal affecting degradative enzyme synthesis in Bacillus subtilis. J. Bacteriol. 177, 2403–2501.
La Cono, V., Bortoluzzi, G., Messina, E., La Spada, G., Smedile, F., Giuliano, L., et al. (2019). The discovery of Lake hephaestus, the youngest athalassohaline deep-sea formation on Earth. Sci. Rep. 9:1679. doi: 10.1038/s41598-018-38444-z
Lee, C. J. D., McMullan, P. E., O’Kane, C. J., Stevenson, A., Santos, I. C., Roy, C., et al. (2018). NaCl-saturated brines are thermodynamically moderate, rather than extreme, microbial habitats. FEMS Microbiol. Rev. 42, 672–693. doi: 10.1093/femsre/fuy026
Lennon, J. T., and Jones, S. E. (2011). Microbial seed banks: the ecological and evolutionary implications of dormancy. Nat. Rev. Microbiol. 9, 119–130. doi: 10.1038/nrmicro2504
Mapelli, F., Crotti, E., Molinari, F., Daffonchio, D., and Borin, S. (2016). “Extreme marine environments (brines, seeps, and smokers),” in The Marine Microbiome. An Untapped Source of Biodiversity and Biotechnological Potential, eds L. J. Stal and M. S. Cretoiu (Berlin: Springer), 251–282. doi: 10.1007/978-3-319-33000-6_9
Mapelli, F., Marasco, R., Balloi, A., Rolli, E., Cappitelli, F., Daffonchio, D., et al. (2012). Mineral–microbe interactions: biotechnological potential of bioweathering. J. Biotechnol. 157, 473–481. doi: 10.1016/j.jbiotec.2011.11.013
Mazmanian, S. K., Skaar, E. P., Gaspar, A. H., Humayun, M., Gornicki, P., Jelenska, J., et al. (2003). Passage of heme-iron across the envelope of Staphylococcus aureus. Science 299, 906–909. doi: 10.1126/science.1081147
Meadows, J. A., and Wargo, M. J. (2015). Carnitine in bacterial physiology and metabolism. Microbiology 161, 1161–1174. doi: 10.1099/mic.0.000080
Meier-Kolthoff, J. P., Auch, A. F., Klenk, H.-P., and Göker, M. (2013). Genome sequence-based species delimitation with confidence intervals and improved distance functions. BMC Bioinformatics 14:60. doi: 10.1186/1471-2105-14-60
Meier-Kolthoff, J. P., Klenk, H. P., and Göker, M. (2014). Taxonomic use of DNA G+C content and DNA-DNA hybridization in the genomic age. Int. J. Syst. Evol. Microbiol. 64(Pt 2), 352–356. doi: 10.1099/ijs.0.056994-0
Merlino, G., Barozzi, A., Michoud, G., Ngugi, D. K., and Daffonchio, D. (2018). Microbial ecology of deep-sea hypersaline anoxic basins. FEMS Microbiol. Ecol. 94:fiy085. doi: 10.1093/femsec/fiy085
Mullakhanbhai, M. F., and Larsen, H. (1975). Halobacterium volcanii spec. nov., a Dead Sea halobacterium with a moderate salt requirement. Arch. Microbiol. 104, 207–214. doi: 10.1007/bf00447326
Oren, A. (2008). Microbial life at high salt concentrations: phylogenetic and metabolic diversity. Saline Syst. 15:2. doi: 10.1186/1746-1448-4-2
Oren, A., Gurevich, P., Gemmell, R. T., and Teske, A. (1995). Halobaculum gomorrense gen. nov., sp. nov., a novel extremely halophilic archaeon from the Dead Sea. Int. J. Syst. Bacteriol. 45, 747–754. doi: 10.1099/00207713-45-4-747
Oren, A., and Mana, L. (2002). Amino acid composition of bulk protein and salt relationships of selected enzymes of Salinibacter ruber, an extremely halophilic bacterium. Extremophiles 6, 217–223. doi: 10.1007/s007920100241
Overbeek, R., Olson, R., Pusch, G. D., Olsen, G. J., Davis, J. J., Disz, T., et al. (2014). The SEED and the Rapid annotation of microbial genomes using subsystems technology (RAST). Nucleic Acids Res. 42, D206–D214. doi: 10.1093/nar/gkt1226
Parks, D. H., Chuvochina, M., Waite, D. W., Rinke, C., Skarshewski, A., Chaumeil, P.-A., et al. (2018). A standardized bacterial taxonomy based on genome phylogeny substantially revises the tree of life. Nat. Biotechnol. 36, 996–1004. doi: 10.1038/nbt.4229
Raddadi, N., Belaouis, A., Tamagnini, I., Hansen, B. M., Hendriksen, B., Abdellatif Boudabous, A., et al. (2009). Characterization of polyvalent and safe Bacillus thuringiensis strains with potential use for biocontrol. J. Bas. Microbiol. 49, 293–303. doi: 10.1002/jobm.200800182
Raddadi, N., Cherif, A., Daffonchio, D., Neifar, M., and Fava, F. (2015). Biotechnological applications of extremophiles, extremozymes and extremolytes. Appl. Microbiol. Biotechnol. 99, 7907–7913. doi: 10.1007/s00253-015-6874-9
Raddadi, N., Giacomucci, L., Marasco, R., Daffonchio, D., Cherif, A., and Fava, F. (2018). Bacterial polyextremotolerant bioemulsifiers from arid soils improve water retention capacity and humidity uptake in sandy soil. Microb. Cell. Fact. 17:83. doi: 10.1186/s12934-018-0934-7
Roe, K. L., Hogle, S. L., and Barbeau, K. (2013). Utilization of heme as an iron source by marine Alphaproteobacteria in the Roseobacter clade. Appl. Environ. Microbiol. 79, 5753–5762. doi: 10.1128/AEM.01562-13
Ruzal, S. M., López, C., Rivas, E., and Sánchez-Rivas, C. (1998). Osmotic strength blocks sporulation at stage II by impeding activation of early sigma factors in Bacillus subtilis. Curr. Microbiol. 36, 75–79. doi: 10.1007/s002849900282
Seemann, T. (2014). Prokka: rapid prokaryotic genome annotation. Bioinformatics 30, 2068–2069. doi: 10.1093/bioinformatics/btu153
Shimoshige, H. I., Yamada, T., Minegishi, H., Echigo, A., Shimane, Y., Kamekura, M., et al. (2013). Halobaculum magnesiiphilum sp. nov., a magnesium-dependent haloarchaeon isolated from commercial salt. Int. J. Syst. Evol. Microbiol. 63(Pt 3), 861–866. doi: 10.1099/ijs.0.037432-0
Spring, S., Nolan, M., Lapidus, A., Glavina Del Rio, T., Copeland, A., Tice, H., et al. (2010). Complete genome sequence of Desulfohalobium retbaense type strain (HR100T). Stand. Genomic Sci. 2, 38–48. doi: 10.4056/sigs.581048
Steinle, L., Knittel, K., Felber, N., Casalino, C., de Lange, G., Tessarolo, C., et al. (2018). Life on the edge: active microbial communities in the Kryos MgCl2-brine basin at very low water activity. ISME J. 12, 1414–1426. doi: 10.1038/s41396-018-0107-z
Teske, A., Durbin, A., Ziervogel, K., Cox, C., and Arnosti, C. (2011). Microbial community composition and function in permanently cold seawater and sediments from an Arctic fjord of Svalbard. Appl. Environ. Microbiol. 77, 2008–2018. doi: 10.1128/AEM.01507-10
Unfried, F., Becker, S., Robb, C. S., Hehemann, J. H., Markert, S., Heiden, S. E., et al. (2018). Adaptive mechanisms that provide competitive advantages to marine bacteroidetes during microalgal blooms. ISME J. 12, 2894–2906. doi: 10.1038/s41396-018-0243-245
van der Wielen, P. W., Bolhuis, H., Borin, S., Daffonchio, D., Corselli, C., Giuliano, L., et al. (2005). The enigma of prokaryotic life in deep hypersaline anoxic basins. Science 307, 121–123. doi: 10.1126/science.1103569
Wallmann, K., Suess, E., Westbrook, G. H., Winckler, G., and Cita, M. B. (1997). Salty brines on the Mediterranean sea floor. Nature 387, 31–32. doi: 10.1038/387031a0
Wang, C. Y., Chang, C. C., Ng, C. C., Chen, T. W., and Shyu, Y. T. (2008). Virgibacillus chiguensis sp. nov., a novel halophilic bacterium isolated from Chigu, a previously commercial saltern located in southern Taiwan. Int. J. Syst. Evol. Microbiol. 58(Pt 2), 341–345. doi: 10.1099/ijs.0.64996-0
Wang, J.-P., Liu, B., Liu, G.-H., Chen, D.-J., Zhu, Y.-J., Chen, Z., et al. (2015). Genome sequence of Virgibacillus pantothenticus DSM 26T (ATCC14576), a mesophilic and halotolerant bacterium isolated from soil. Genome Announc. 3:e01064-15. doi: 10.1128/genomeA.01064-15
Widderich, N., Rodrigues, C. D. A., Commichau, F. M., Fischer, K. E., Ramirez-Guadiana, F. H., Rudner, D. Z., et al. (2016). Salt-sensitivity of σH and Spo0A prevents sporulation of Bacillus subtilis at high osmolarity avoiding death during cellular differentiation. Mol. Microbiol. 100, 108–124. doi: 10.1111/mmi.13304
Yakimov, M. M., La Cono, V., Denaro, R., D’Auria, G., Decembrini, F., Timmis, K. N., et al. (2007). Primary producing prokaryotic communities of brine, interface and seawater above the halocline of deep anoxic lake L’Atalante Eastern Mediterranean Sea. ISME J. 1, 743–755. doi: 10.1038/ismej.2007.83
Yakimov, M. M., La Cono, V., Spada, G. L., Bortoluzzi, G., Messina, E., Smedile, F., et al. (2015). Microbial community of the deep-sea brine Lake Kryos seawater-brine interface is active below the chaotropicity limit of life as revealed by recovery of mRNA. Environ. Microbiol. 17, 364–382. doi: 10.1111/1462-2920.12587
Yoon, J. H., Kang, S. J., Lee, S. Y., Lee, M. H., and Oh, T. K. (2005). Virgibacillus dokdonensis sp. nov., isolated from a Korean island, Dokdo, located at the edge of the East Sea in Korea. Int. J. Syst. Evol. Microbiol. 55(Pt 5), 1833–1837. doi: 10.1099/ijs.0.63613-0
Keywords: brine pools, osmoadaptation, salt stress, magnesium chloride, spore
Citation: Zeaiter Z, Marasco R, Booth JM, Prosdocimi EM, Mapelli F, Callegari M, Fusi M, Michoud G, Molinari F, Daffonchio D, Borin S and Crotti E (2019) Phenomics and Genomics Reveal Adaptation of Virgibacillus dokdonensis Strain 21D to Its Origin of Isolation, the Seawater-Brine Interface of the Mediterranean Sea Deep Hypersaline Anoxic Basin Discovery. Front. Microbiol. 10:1304. doi: 10.3389/fmicb.2019.01304
Received: 13 February 2019; Accepted: 27 May 2019;
Published: 12 June 2019.
Edited by:
Masahiro Ito, Toyo University, JapanReviewed by:
Erhard Bremer, University of Marburg, GermanyMasahiro Kamekura, Halophiles Research Institute, Japan
Copyright © 2019 Zeaiter, Marasco, Booth, Prosdocimi, Mapelli, Callegari, Fusi, Michoud, Molinari, Daffonchio, Borin and Crotti. This is an open-access article distributed under the terms of the Creative Commons Attribution License (CC BY). The use, distribution or reproduction in other forums is permitted, provided the original author(s) and the copyright owner(s) are credited and that the original publication in this journal is cited, in accordance with accepted academic practice. No use, distribution or reproduction is permitted which does not comply with these terms.
*Correspondence: Elena Crotti, ZWxlbmEuY3JvdHRpQHVuaW1pLml0
†Present address: Zahraa Zeaiter, Department of Biotechnology and Biosciences, University of Milano-Bicocca, Milan, Italy; Erica M. Prosdocimi, Dental Institute, King’s College London, London, United Kingdom