- 1School of Life Sciences, Shanghai University, Shanghai, China
- 2Shanghai Key Laboratory of Bio-Energy Crops, Shanghai, China
In this study, we evaluated the effects of volatile organic compounds (VOCs) produced by Bacillus subtilis CF-3 in inhibiting Monilinia fructicola in vitro and in vivo. In the in vitro experiments, the effect of VOCs on the growth of the pathogenic fungi was explored by using plate enthalpy test; mycelial morphology was studied by scanning electron and transmission electron microscopy; and fatty acid contents in the cell membrane were assessed by gas chromatography-mass spectrometry (GC-MS). The results indicated that treatment with benzothiazole and CF-3 for 24 h, in the form of a fermentation broth (24hFB), significantly inhibited the germination of fungal spores, modified hyphal and cell morphology, and decreased the cell membrane fluidity and integrity. In the in vivo experiments, the effect of VOCs on the defense mechanism of peach fruit toward M. fructicola was studied, and we found that benzothiazole and CF-3 24hFB inhibited the activity of the pathogenic enzymes (pectinase, cellulase) secreted by M. fructicola to reduce the decomposition of plant tissues, activate the antioxidant enzymes (peroxidase, polyphenol oxidase, catalase, and superoxide dismutase) in the fruit to eliminate excessive reactive oxygen species in order to reduce plant cell damage, and trigger the disease-resistant enzymes (phenylalanine ammonia-lyase, chitinases, and β-1,3-glucanase) to enhance the resistance of peach fruit to M. fructicola and inhibit its growth. This study suggests that CF-3 VOCs could activate disease-resistant enzymes to prevent the invasion of pathogenic fungi and induce resistance in peach, thereby providing a theoretical basis for future applications.
Introduction
Due to the inadequate treatment in each production step, Monilinia fructicola infested on the peach fruit causes decay, resulting in large economic losses worldwide (Liu et al., 2008; Hu et al., 2011). Previously, postharvest fruit diseases were controlled via refrigeration or modified atmosphere storage and fungicides (Zheng et al., 2013). Physical methods may not provide efficient inhibition, whereas chemical methods may cause food safety and environmental pollution issues (Schreinemachers and Tipraqsa, 2012). Thus, Bacillus subtilis is widely studied for fruit preservation due to its rich physiological characteristics and strong environmental adaptability, which has broad application prospects in the control of plant diseases as an extremely important bio-control resource (Ongena et al., 2005).
In recent years, the antifungal effect of B. subtilis has gained immense attention in research. B. subtilis inhibits various plant pathogens and has an antagonistic effect on several plant fungi diseases that affect the plant roots, branches, flowers, and fruits (Senthil et al., 2011; Gava et al., 2019). In the existing report, B. subtilis antagonizes more than 30 types of plant pathogens (Moyne et al., 2004). It is widely distributed in nature and is nontoxic and harmless to humans and children, easy to isolate and cultivate, and has broad-spectrum antifungal activity and strong antireverse ability to produce antibiotics and enzymes such as peptides, lipopeptides, polyenes, and amino acids (Ahimou and Deleu, 1999), thus being extremely beneficial in biological control and fruit preservation.
Moreover, recent studies reported that B. subtilis can produce not only antibiotics and antifungal proteins (Zhang et al., 2019) but also a series of VOCs (Leelasuphakul et al., 2008), which can effectively inhibit the growth of postharvest pathogens of fruits and vegetables. Waewthongrak et al. (2015) reported that the cyclolipopeptides secreted by B. subtilis ABS-S14 had a broad-spectrum antifungal effect, thereby reducing the Penicillium strains exposed to B. subtilis and cell-free culture by 96.2 and 90.9%, respectively. Ambrico and Trupo, (2017) further confirmed that Iturin A produced by B. subtilis ET-1 can effectively inhibit the occurrence of blue and gray molds on lemon and strawberry fruits, respectively.
Compared with the nonvolatile antifungal substances, VOCs are more likely to exert antifungal activity via air and soil. During preservation, the VOCs produced by B. subtilis can be combined with the modified atmosphere storage in order to achieve anticorrosion. Although B. subtilis VOCs are proved to be effective in inhibiting the growth of pathogens (Vespermann et al., 2007), the mechanism of VOCs remains unclear. It was reported that B. subtilis VOCs could destroy the mycelial morphology, exhibiting a strong antimicrobial effect (Zhou et al., 2011), and could prevent the pigment production in pathogenic bacteria (inhibition rate 43–93%; Liu et al., 2008).
Peaches are favorably cultivated in China, and any decay caused by M. fructicola may result in more than 20% yield loss (Zhang et al., 2015a,b). In the previous study, we isolated and identified the B. subtilis CF-3 (Gao et al., 2016) and total 74 potential VOCs in the fermentation process (Gao et al., 2017). And the main VOCs were noted as 1-octanol, benzaldehyde, 3-methylbutanal, benzoic acid, methoxy-phenyl-oxime, anisole, benzothiazole, and 2,4-di-tert-butylthiophenol, which have different antimicrobial effects (Gao et al., 2018). Among them, benzothiazole, a crucial compound that inhibited M. fructicola was assessed. In order to clarify the mode of VOC action both in vivo and in vitro, this article highlights the effect of VOCs in inducing fruit resistance against M. fructicola and attempts to explain how VOCs inhibit pathogen, thereby laying the foundation for future applications.
Materials and Methods
Preparation of Strain and 24hFB
B. subtilis CF-3 was isolated from fermented bean curd and identified by the Laboratory of Food Safety and Quality Control (School of Life Sciences, Shanghai University), which was registered in the China Center for Type Culture Collection, CCTCC M 2016125 (Gao et al., 2016). B. subtilis CF-3 was cultivated for 7 days at 37°C, using LB solid medium (Gao et al., 2017).
M. fructicola (provided by the Beijing University of Agriculture) was cultivated for 7 days at 25°C, using PDA solid medium (Gao et al., 2017).
To obtain 24hFB, B. subtilis CF-3 was cultivated on LB solid medium for 24 h at 37°C, then gently scraped with an inoculating loop and then transferred into 100 ml LB liquid medium in a conical flask and cultivated at 37°C in a rotary shaker at 150 rpm for 24 h, and was eventually diluted to a concentration of about 108 cfu/ml through a hemocytometer. Moreover, a fumigation treatment was performed.
Fumigation Treatment by B. subtilis CF-3 Volatile Organic Compounds
The method described by Arrebola et al. (2010) and Jiang et al. (2014) was used to evaluate the inhibitory effects of VOCs produced by B. subtilis CF-3 against M. fructicola. About 20 μl of B. subtilis CF-3 fermentation broth after 24 h fermentation was aspirated and evenly spread on LB solid medium. Subsequently, a plug (Ø7 mm) from the agar of the fungus, which was incubated for 7 days, was punched and placed at the center of a fresh PDA medium. Eventually, the LB solid medium was inverted on the fungus-attached PDA solid medium and sealed with a parafilm to reduce the loss of VOCs. The sealed petri dish was placed in an electrothermal incubator at 28°C for 7 days.
Effects of Volatile Organic Compounds on M. fructicola Spore Germination
Preparation of pathogenic spore suspension: 5 ml of sterile water containing 0.05% Tween 80 was added to the PDA plate of M. fructicola precultivated for 7 days, and the solution was then filtered through eight layers of sterile cheesecloth, and then, the concentration was adjusted to 1 × 104 conidia/ml using distilled water.
The treatment settings were as follows: blank control group (no treatment, distilled water); positive control group (500 mg/L thymol solution with distilled water); 1 mol/L benzothiazole; and CF-3 24hFB.
The treatment solution was evenly spread on LB solid medium, and the spore suspension (20 μl) was evenly spread on fresh PDA medium. The reagent-coated medium was inverted on the PDA coated medium with spore suspension and was further sealed with a parafilm and placed in a 28°C incubator for cultivation. Simultaneously, LB solid medium coated with the same volume of distilled water was used as a blank control, and the equal amount of thymol solution was added as a positive control, followed by cultivation under similar conditions. Set up three parallels for each processing group. The germination of spores was, respectively, observed by light microscopy at 2, 4, 6, 8, 10, and 12 h, and the length of the germ tube exceeded half of the maximum diameter of spores as the spore germination standard. When the spore germination rate in the blank control group exceeded 90%, the rate of all treatment groups was measured and calculated. The formula for calculating the spore germination rate is as follows:
where R1 is the spore germination rate; n is the number of spores that have sprouted; and t is the total number of spores.
Effects of Volatile Organic Compounds on M. fructicola Morphology
Preparation of electron microscope fixative (2.5% neutral glutaraldehyde): 10 ml glutaraldehyde solution (25%), 50 ml phosphate buffer solution (0.2 M, 0.588 g NaH2PO4·2H2O, 5.8 g Na2HPO4·12 H2O, dissolved and mixed in 100 ml sterile distilled water, and adjust the pH to 7.2–7.4, standby), and 40 ml sterile distilled water. After mixing, a 2.5% neutral glutaraldehyde solution was prepared.
The scraped hyphae (blank control, single VOC treatment, CF-3 24hFB) were soaked in electron microscope fixative and sent to Shanghai Yuyi Testing Center for electron microscopy.
Effect of Volatile Organic Compounds on the Fatty Acid Content of M. fructicola Cell Membrane
The accurately weighed 0.2 g of the fumigated fungal sample was added in a round bottom flask, with an addition of 100 ml of methanol to reflux for 2 h, and the reflux was steamed 5 ml with a rotary evaporator.
The extracted lipid concentrate was hydrolyzed with potassium hydroxide-methanol solution (11 g/L) at 90°C for 10 min. Total fatty acids were then derived with 2 ml sulfuric acid methanol (10%, V/V) at 90°C for 20 min. After adding 250 μl of the internal standard (1 g/L methyl nonanoate), the fatty acid solution was extracted with 2 × 6 ml of isooctane and occasionally oscillated. The water in the isooctane layer was removed with anhydrous sodium sulfate. Eventually, it was concentrated by rotary evaporation to 1 ml and stored at 20°C for analysis.
According to Tang et al. (2011), an 1 μl sample was injected in HP-5 ms capillary column, and the fatty acid content was analyzed using GC-MS. All samples were analyzed in triplicate, and the standard deviation was calculated.
Effects of Volatile Organic Compounds on the Content of Ergosterol in the M. fructicola Cell Wall
Accurately weighed 0.2 g of the sample was placed in a 40 ml covered vial. About 20 ml NaOH solution (5 g NaOH, 5 ml sterile distilled water, and 95 ml methanol) and 20 μl 1 mg/ml internal standard stock solution [accurately weighed 7-dehydrocholesterol standard (VD3) 100 mg, corrected to 0.1 mg, dissolved in methanol, and diluted to 100 ml, to get 1 mg/ml internal standard stock solution] were hydrolyzed at 80°C for 2 h and then cooled at room temperature. From the above hydrolyzate, 5 ml solution was added in a 15 ml stoppered tube and added 3 ml of n-hexane, and the hydrolyzate was vortexed for 2 min. Next, 1 ml of the supernatant was added into a chromatography bottle, and then, the solvent was dried in a 40°C water bath, and to this 300 μl BSTFA was added at 80°C for 45 min and analyzed by GC-MS after cooling.
According to Saraf et al. (1997), Pasanen et al. (1999), Sha et al. (2008), and Liu et al. (2007), the qualitative analysis was accomplished in selected ion monitoring (SIM) mode with m/z 363 for ergosterol and m/z 351 for internal standard. All samples were analyzed in triplicate, and the standard deviation was calculated.
About 1 ml of ergosterol standard solution with a concentration of 5, 10, 20, 50, and 100 μg/ml, respectively, and 100 μl of 1 mg/ml internal standard stock solution were added in a chromatography bottle. After evaporation of the solvent in a 40°C water bath, 300 μl of BSTFA was added and derived at 80°C for 45 min. The standard series of ergosterol solutions were determined and analyzed by GC-MS in similar conditions. The peak area was analyzed by regression analysis to obtain the integrated peak area of ergosterol and internal standard. The integrated peak area and internal standard of ergosterol were obtained. The ratio of the integrated peak area of ergosterol to the internal standard peak area was used as the ordinate (Y), and the ratio of the ergosterol mass concentration to the internal standard mass concentration was used as the abscissa (X) in order to establish a standard curve of ergosterol.
Effects of CF-3 Volatile Organic Compounds on Related Enzyme Activities and Indicators in Peach Fruit
Peach fruit is the “Zhaohui” variety of Shandong Mengyin, which was purchased from Shanghai Fruit Wholesale Market. We chose fruits that were disease-free, had no obvious wounds, had a neat appearance, and had similar maturity and size. Next, they were pruned and immersed in a 0.1% sodium hypochlorite solution for 1 min and then rinsed with tap water and air-dried for further use.
We took the peach fruit, which was disinfected and air-dried, used a sterilized hole punch with a diameter of 7 mm to make a hole with a diameter of 7 × 5 mm in the center of the largest section of the peach fruit, injected 20 μl of the prepared fungal spore suspension with a syringe of 1 ml, and dried it until further use. Next, we kept 10 peaches on a plastic tray that was sterilized with 75% ethanol (4 × 3 rows of grooves), by placing 1 peach in each groove, and then placed two pieces of filter paper in the remaining two grooves separately (90 mm diameter). Subsequently, 200 μl of reagent or CF-3 24hFB was added to each of the two filter paper sheets (benzothiazole 1 mol/L). Then, it was quickly added into a prepurchased cardboard box (45 cm × 35 cm × 10 cm) and was sealed; the box was wrapped in a gas-conditioned bag and placed at room temperature (25°C). Samples were unpacked every day from Day 1 for a total of 4 days, with three replicates in each treatment. The disease speckle diameters were measured, and the inhibition rate was calculated to contrast treatment effects. Due to the instability of VOCs, they were discarded directly after unpacking and were not resealed.
The peach fruit treatment settings are as follows: (1) blank control group (no treatment); (2) 1 mol/L benzothiazole; and (3) CF-3 24hFB.
We randomly selected 15 peaches from three boxes, five from each box. Namely, there were 15 peaches removed each day for 4 days per treatment. The reduction of the fungal infection in vivo was assessed by inhibition rate, and the formula is as follows:
where R2 is the inhibition rate; d0 is the disease speckle diameter of blank control group; and d is the diameter of treatment group.
The sampling was done by cutting off the outermost 1 cm pulp of the punching part and cutting the 0.5 cm deep pulp at the punching part. The 0.5 cm deep pulp was separated into small pieces, frozen in liquid nitrogen, and stored in a refrigerator at −80°C for further use.
Measurement of Polygalacturonase and Cellulose
To extract polygalacturonase (PG) and cellulose, 10.0 g peach fruit sample was ground in 95% ethanol in an ice bath and centrifuged at 4°C and 12,000 × g for 20 min. Then, it was added in 80% ethanol and centrifuged at 4°C and 12,000 × g for 20 min. Extraction buffer solution was added to the precipitate. After centrifugation, the supernatant was collected and stored at 4°C.
About 0.5 ml PG (or cellulose), extract was mixed with the reaction solution and incubated at 37°C for 1 h. About 1.5 ml 3,5-dinitrosalicylic acid reagent was immediately added dropwise, heated in a boiling water bath for 5 min, diluted to 25 ml, and was shaken. The absorbance was determined at 540 nm. The enzyme extract was boiled for 5 min and was then used as a blank control group.
Measurement of Antioxidant System and Phenylalanine Ammonia-Lyase
To extract peroxidase (POD), polyphenol oxidase (PPO), catalase (CAT), superoxide dismutase (SOD), superoxide anion ( ), H2O2, malondialdehyde (MDA), and phenylalanine ammonia-lyase (PAL), 5.0 g peach fruit sample was ground in the extraction buffer solution in an ice bath and centrifuged at 4°C and 12,000 × g for 30 min (20 min for , H2O2, and MDA), and the supernatant was used for further measurement.
Started timing as soon as 0.5 ml POD extract (0.1 ml for PPO extract or CAT extract) was mixed rapidly with 3.0 ml guaiacol (25 mmol/L) and 0.2 ml H2O2 (4.0 ml 50 mmol/L pH 5.5 sodium acetate-acetic acid buffer solutions and 1.0 ml catechol for PPO, 2.9 ml 20 mmol/L H2O2 for CAT). The absorbance was measured at 470 nm (420 nm for PPO, 240 nm for CAT), considering distilled water as a reference. The reaction was measured first in 15 s, and it was recorded as the initial value and then recorded at every 1 min (1 min for PPO, 30 s for CAT) and was continuously measured for 6 times (7 times in total).
About 0.1 ml SOD extract and the reaction solution were sequentially added to the measuring tube. The blank control group replaced the supernatant with phosphate buffer solution (0.1 mol/L, pH 7.8). A blank tube was placed in the dark, and the rest were placed under a 4,000 lx fluorescent lamp for 15 min. The reaction was immediately terminated in the dark. The blank tube, which had been placed in the dark, was zero-referenced, and the absorbance value was measured at 560 nm.
About 1.0 ml extract was mixed with 1.0 ml phosphate buffer solution (50 mmol/L, pH 7.8) and 1.0 ml hydroxylamine hydrochloride solution (1 mmol/L) and incubated at 25°C for 1 h. Next, 1.0 ml p-aminobenzenesulfonic acid (17 mmol/L) and 1.0 ml α-naphthylamine (7 mmol/L) were added and incubated at 25°C for 20 min. Eventually, absorbance was immediately measured at 530 nm. The mixture without incubation for 1 h was used as a blank control group.
About 1.0 ml H2O2 extract was mixed with 0.1 ml titanium tetrachloride-hydrochloric acid (10%) and 0.2 ml concentrated ammonia and was centrifuged at 4°C and 12,000 × g for 15 min after reacting for 5 min. Next, the precipitate was washed 2–3 times with the precooled acetone. Eventually, 3.0 ml sulfuric acid (2 mol/L) was added to the precipitate until completely dissolved. The absorbance was determined at 412 nm.
About 2.0 ml MDA extract was mixed with 2.0 ml thiobarbituric acid (6.7 g/L), refrigerated, and centrifuged after boiling for 20 min in a boiling water bath. The absorbance was determined at 450, 532, and 600 nm. The blank control group replaced the supernatant with 2.0 ml of TCA.
About 0.5 ml PAL extract was mixed rapidly with the reaction solution and incubated at 37°C for 1 h. Eventually, 0.1 ml hydrochloric acid (6 mol/L) was added to stop the reaction. The absorbance was determined at 290 nm. The enzyme extract was boiled for 5 min and was used as a blank control group.
Measuring of Superoxide Anion, Chitinase, and β-1,3-Glucanase
The production rate of superoxide anion and the activities of chitinase (CHI) and β-1,3-glucanase (GLU) were measured by superoxide anion kit, CHI kit, and GLU kit, respectively (Comin Biotechnology, Co., Ltd., Suzhou, CHN).
Statistical Analysis
All the experiments were repeated twice. SPSS 18.0 software (SPSS Inc., Chicago, IL, USA) was used to conduct statistical analysis (ANOVA). Duncan’s post hoc test was applied to compare the mean values. A significance level of p < 0.05 was used to determine the significant differences. Mean values with standard deviations were reported. Additionally, Origin Pro 9 (OriginLab Corp., Northampton, MA, USA) was used to produce charts.
Results
In vivo and in vitro Inhibitory Effects of Volatile Organic Compounds on the Growth of M. fructicola
In the spore germination test of M. fructicola, 12 h after treatment, spores that were not subjected to any treatment and those treated with distilled water (blank control group) germinated; hence, spore germination rate in all groups was measured at 12 h. What’s more, thymol was used as positive control, due to the fact that thymol fumigation has serious disintegration of membranes and organelles of M. fructicola spores and mycelia (Svircev et al., 2007). As shown in Figure 1A, the germination rate following the benzothiazole treatment was 4.01 ± 3.24%, and the germination rate with CF-3 24hFB treatment was 19.59 ± 2.12%.
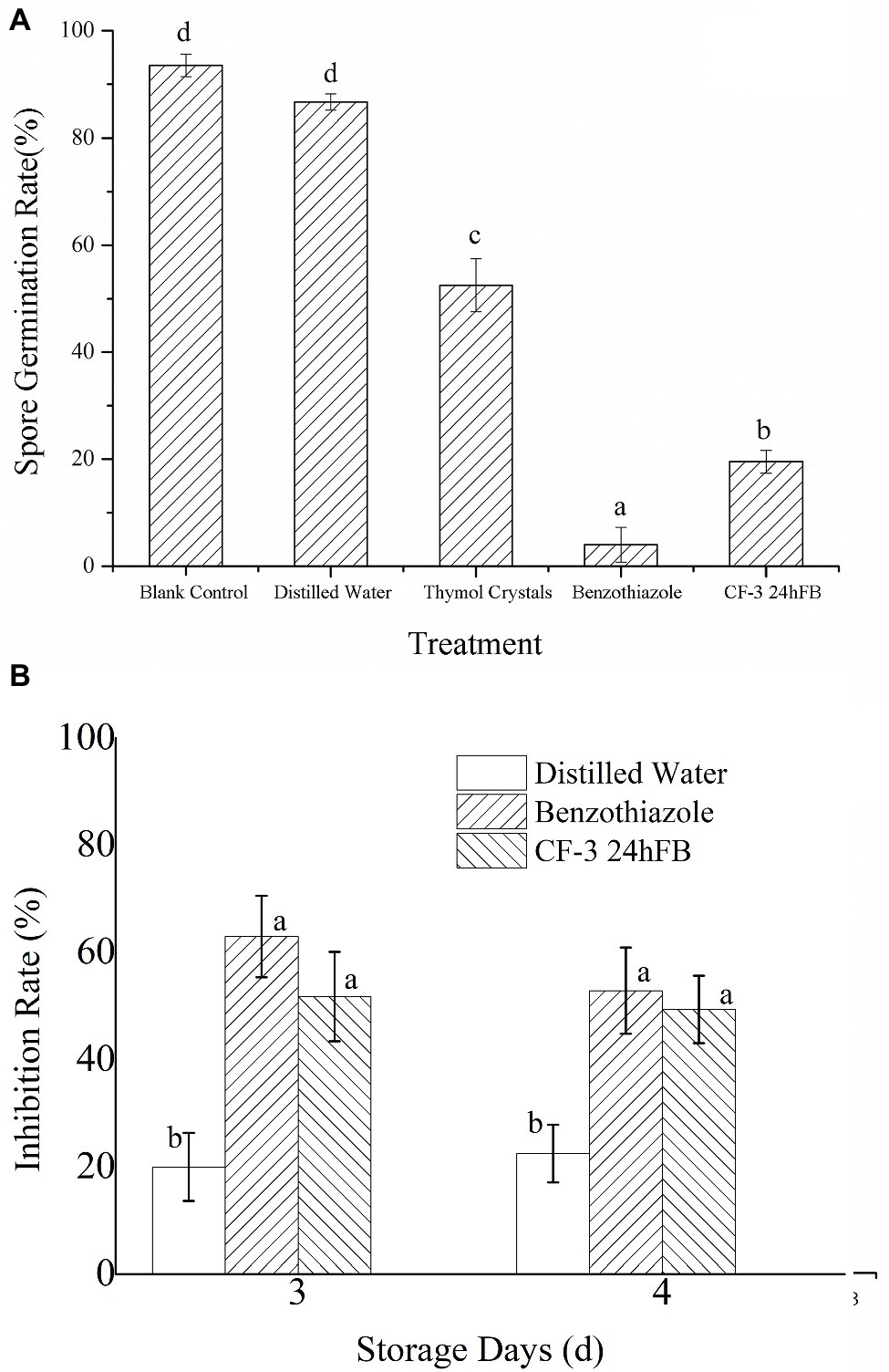
Figure 1. Inhibitory effect of different treatments on spore germination (A) and fungus (B) of M. fructicola. Each column represents the mean value from three independent experiments, and vertical bars represent the standard errors of the means for each treatment. Different letters represent significant differences (p < 0.05).
The antifungal effects of VOCs on M. fructicola are presented in Figure 1B. The inhibition rates of benzothiazole and CF-3 24hFB were reported to be nearly 65 and 50% in 3 days, separately.
In vitro Effects of Volatile Organic Compounds on M. fructicola
Scanning electron microscopy studies showed that the hyphae in the blank group were intact, and the surface was smooth and full (Figure 2). By contrast, the hyphae shrank, twisted, and ruptured, resulting in a creased or wrinkled surface after benzothiazole and CF-3 24hFB treatment. Transmission electron microscopy showed that the blank group hyphae had typical fungal ultrastructure, normal and uniform cell wall thickness, intact cell membrane, and uniform cytoplasm; however, the treated organelles started to shrink, the plasma membrane ruptured and separated from the cell wall, and the intracellular components were severely destroyed and formed an empty shell, indicating that the VOCs of CF-3 24hFB and benzothiazole can act on the cell wall and cell membrane of the fungus, destroying its barrier function.
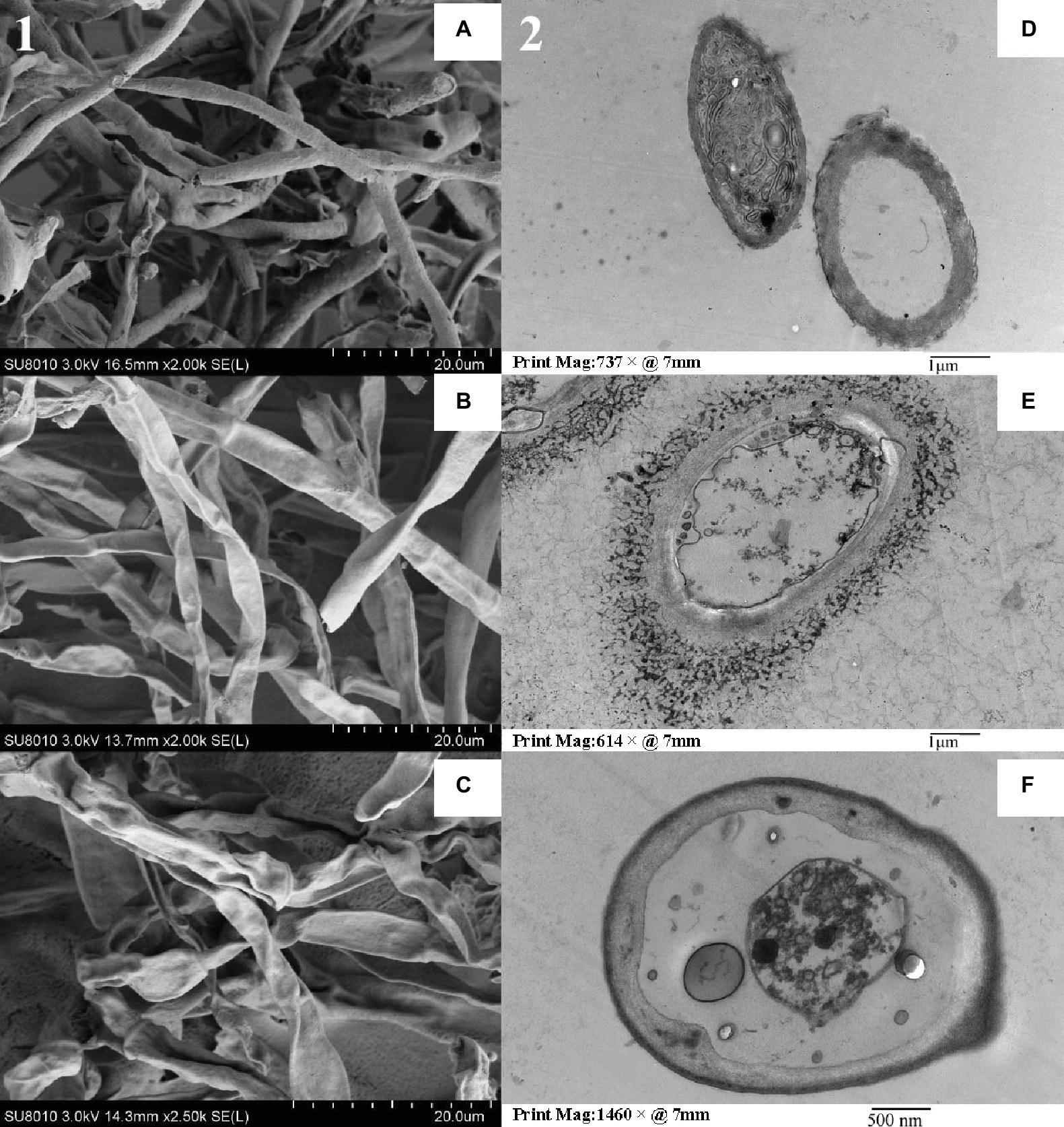
Figure 2. Electron microscope detection for M. fructicola. The left side (1) is a scanning electron micrograph of M. fructicola, and the right side (2) is a transmission electron micrograph. (A) and (D) are blank control, (B) and (E) are benzothiazole treatment, (C) and (F) are CF-3 24hFB treatment.
The results of GC-MS analysis revealed that M. fructicola of the blank control contained more long-chain saturated fatty acids, and the relative content of trans-linoleic acid, oleic acid, and palmitic acid increased after treatment with benzothiazole and CF-3 24hFB (Table 1), indicating that the unsaturation in the cell membrane of M. fructicola decreased, and the fluidity of the membrane gradually weakened, resulting in leakage of contents.
The ergosterol content in the cell wall of M. fructicola treated with benzothiazole and CF-3 24hFB significantly reduced (Table 2), indicating that the treatment with VOCs can reduce ergosterol content in the cell wall, affect the integrity of the fungal cell wall, and thus weaken the cell membrane and material transport, thereby affecting the viability of fungal cells.
In vivo Effects of CF-3 Volatile Organic Compounds on Peach
As can be observed from Figure 3, the polygalacturonase activity in the peach fruit of the blank group and the CF-3 24hFB treatment group increased conspicuously in 3 days then fell back (Figure 3A); however, the magnitude of increase and the enzyme activity in the blank group were much greater than those in the CF-3 24hFB treatment group. And the enzyme activity of the benzothiazole treatment group was lower than that of the other two groups. The cellulase level in peach fruit increased rapidly as the number of storage days increased, whereas the two treatment groups were not obviously different in 2 days, and the activity in the benzothiazole treatment group was the lowest in 3 and 4 days (Figure 3B).
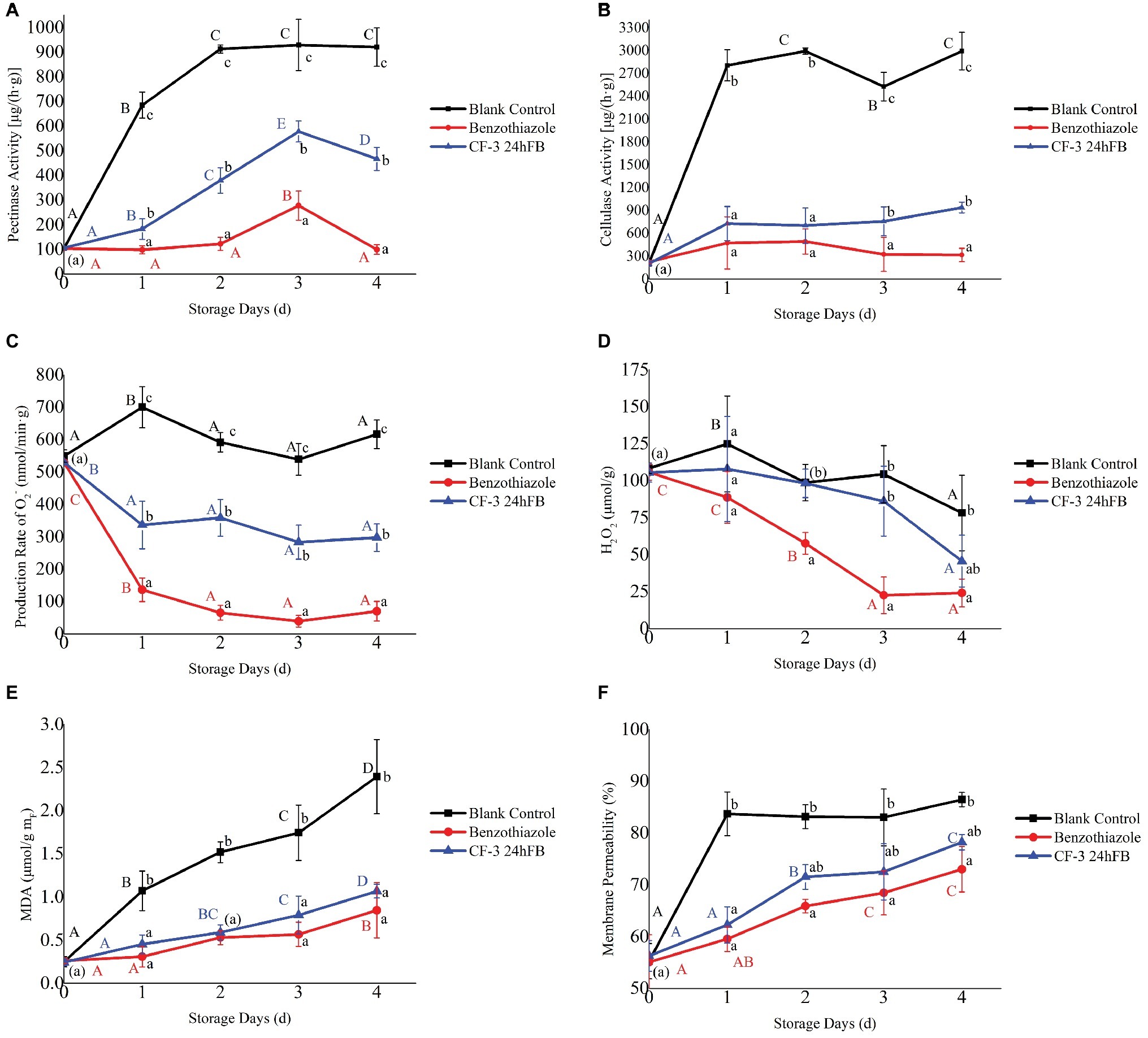
Figure 3. Influence of different treatments on pectinase (A), cellulose (B) activity, the production rate of superoxide anion (C), amount of hydrogen peroxide (D), amount of MDA (E), and membrane permeability (F) in vivo during storage. The mean and standard error in the figure were taken from three replicates, and the different lowercase letters indicated that various treatments during the same storage days differed significantly different, the different uppercase letters indicated that same treatment during different storage days differed significantly different (p < 0.05).
The production rate of superoxide anion and hydrogen peroxide content in peach fruit during storage is presented in Figures 3C,D. During the whole storage process, except for the blank control group, the production rate of superoxide anion in the other two groups fluctuated obviously. The production rate of superoxide anion in the benzothiazole treatment group was overall significantly lower than that in the other groups. Moreover, the general trend of hydrogen peroxide content was decreasing. Except for the benzothiazole treatment group, no significant difference was observed in the hydrogen peroxide content between the other two groups, whereas the hydrogen peroxide content in the benzothiazole treatment group was significantly lower than that in the blank control group.
The changes in MDA content and cell membrane permeability during storage are presented in Figures 3E,F. During the whole storage process, the MDA content in the peach fruit of the two treatment groups increased with storage time, but there was no significant difference between the two groups; however, the MDA content significantly differed from that of the blank control group. During the storage process, cell membrane permeability increased significantly, indicating that the damage of fruit cells became much more serious as the storage days increase, but there was no significant difference in cell permeability between the two treatment groups, and the difference was significantly lower than that in the blank control group. Among these, the MDA content and cell membrane permeability were the lowest in the benzothiazole treatment group.
The activities of four antioxidant-related enzymes (PPO, POD, CAT, and SOD) initially revealed an increasing trend and then decreased during storage (Figure 4). Enzymatic activities in treated peaches were higher than those in the blank control group. Among these, the activities of the four antioxidant enzymes in the benzothiazole treatment group were higher than those in the CF-3 24hFB treatment group, especially in 3 and 4 days, and both peaked on the third day.
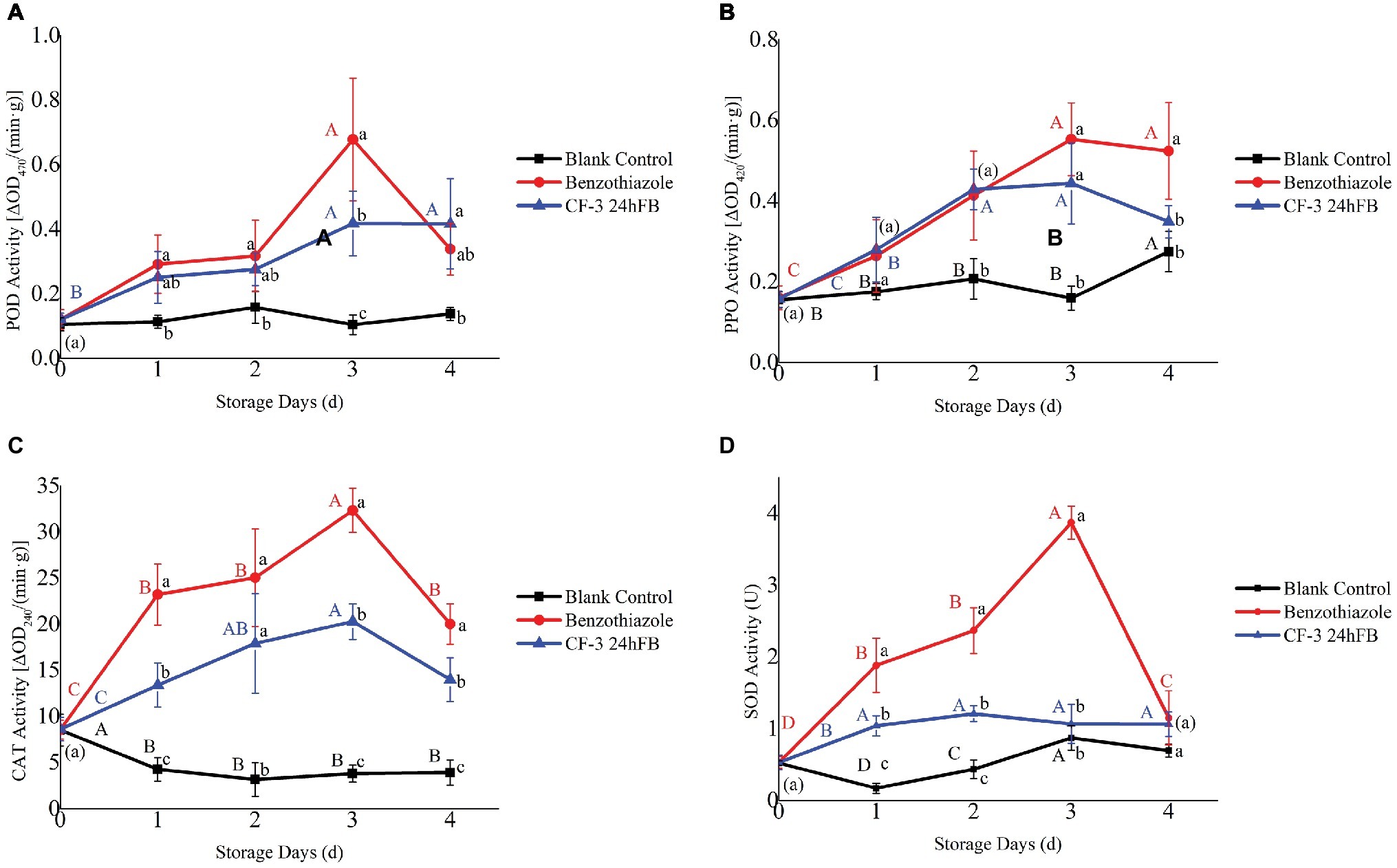
Figure 4. Influence of different treatments on POD (A), PPO (B), CAT (C), and SOD (D) activity in vivo during storage. The mean and standard error in the figure were taken from three replicates, and the different lowercase letters indicated that various treatments during the same storage days differed significantly different, the different uppercase letters indicated that same treatment during different storage days differed significantly different (p < 0.05).
The activities of three enzymes related to disease resistance in plant (PAL, CHI, β-1,3-GLU) initially revealed an increasing trend and then decreased during storage (Figure 5), and after two treatments, the activities of the three enzymes in the peaches were all higher than those of the blank control group as a whole. Among these, the activities of the three disease-resistant enzymes in the benzothiazole treatment group were higher than those in the CF-3 24hFB, and both peaked on the third day.
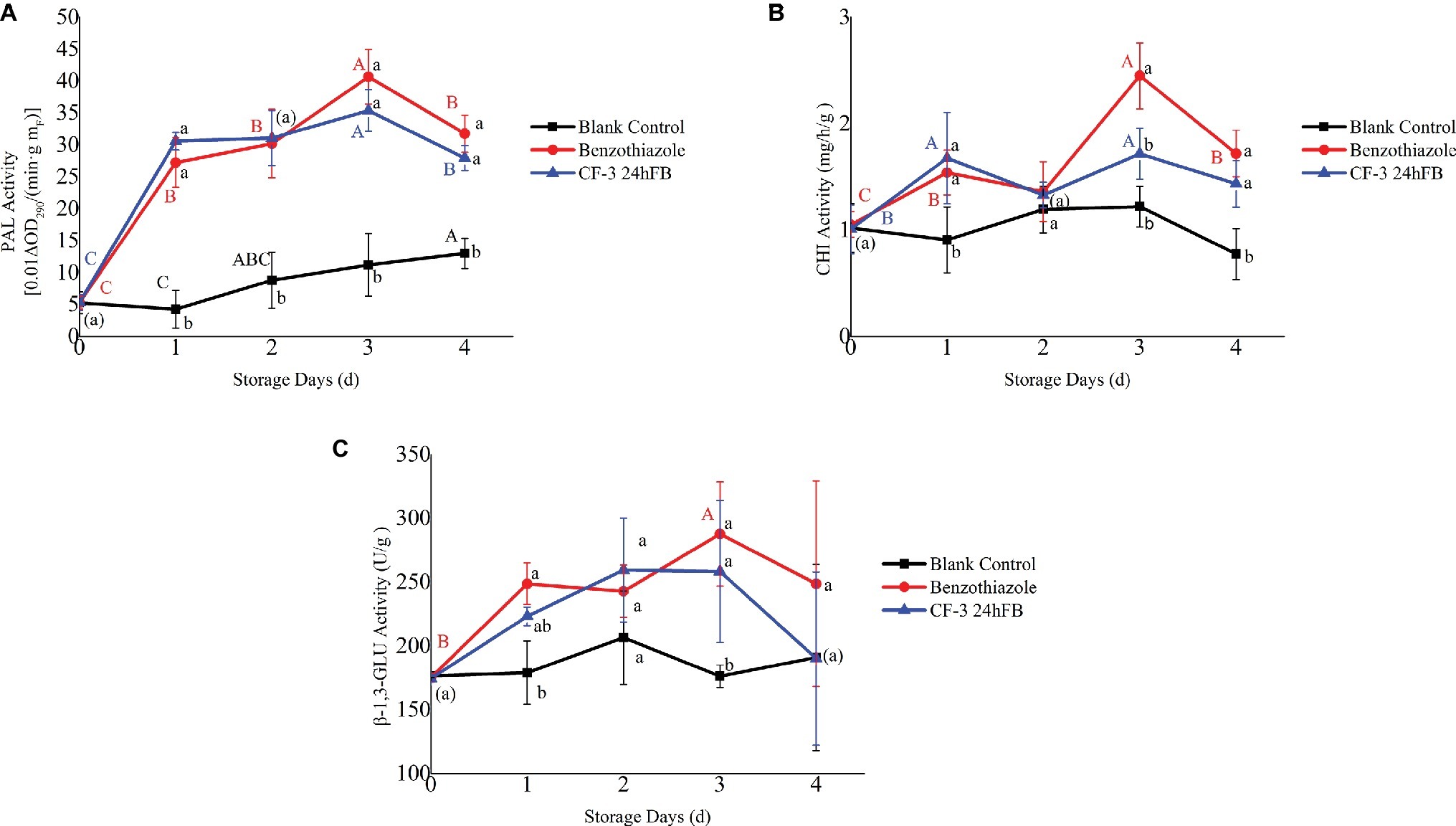
Figure 5. Influence of different treatments on PAL (A), CHI (B), and β-1,3-GLU (C) activity in vivo during storage. The mean and standard error in the figure were taken from three replicates, and the different lowercase letters indicated that various treatments during the same storage days differed significantly different, the different uppercase letters indicated that same treatment during different storage days differed significantly different (p < 0.05).
Discussion
We previously reported by using both physical and flat-panel experiments that VOCs produced by B. subtilis CF-3 can prevent the decay of peaches and significantly inhibit the growth of pathogenic fungi as well as spore germination (Gao et al., 2018). It is further indicated that VOCs can destroy fungal cells in vitro and induce disease resistance in postharvest peaches.
The inhibition rate indicated that the fruits in benzothiazole and 24hFB treatment groups were much better than those in the sterile water and blank groups. Namely, CF-3 VOCs can really prevent decay. In vitro, the lipid composition of the fungal cell membrane and its content are important parameters of the cell membrane, which have several important functions, including increasing cell membrane stability, regulating cell membrane fluidity, and reducing the permeability of water-soluble substances (Heaton and Randall, 2011). If the saturated fatty acid in the cell membrane is at a high level, it will lead to an increase in the cell membrane stiffness, causing cell rupture (Bayer et al., 2000). In this experiment, the unsaturation of fungal cells treated by VOCs decreased to varying degrees, which could lead to the decrease of membrane fluidity, causing cell rupture and leakage of contents as reported (Cronan, 2003). In addition, ergosterol is a major component of the cell wall of filamentous fungi and is helpful for the fluidity and asymmetry and integrity of cell membranes, thus playing an essential role in the growth and function of cells (Lupetti et al., 2002). In this experiment, the content of ergosterol in the wall of fungal cells decreased after treated by VOCs, which could reflect that VOCs destroyed the cell wall of the fungus and interfered with the normal operation of the cell membrane.
By studying the effects of VOCs produced by B. subtilis CF-3 on the antioxidant system and disease-resistant enzymes in vivo, the effect of VOCs inducing peach fruit resistance to M. fructicola was explored. Initially, pectinases play significant roles in the firmness and cell structure (Prade et al., 1999), and cellulase degrades the cellulose and the β-1,4-glucan backbone of xyloglucan (Sethu Priya et al., 1996), which are two important pathogenic enzymes for postharvest harvesting of fruits and vegetables. The pectinase and cellulase activities in peach fruit were significantly lower than those in the blank control group after VOCs fumigation, explaining that the structural damage was alleviated. Moreover, the production rate of superoxide anion and hydrogen peroxide content, two main oxygen-derived species, was significantly lower than the blank control group, indicating that the excessive peroxide production was effectively inhibited. As the final product of lipid peroxidation, MDA is often used as an indicator of cellular oxidative damage under stress (Lu et al., 2014). MDA content and cell membrane permeability in the treatment groups were significantly lower than the blank control group, proving that the oxidative damage of plant cell membrane was smaller. By contrast, the enzyme activities of the four antioxidant enzymes (POD, PPO, CAT, and SOD) were significantly increased. It is reported that benzo-(1,2,3)-thiadiazole-7-carbothioic acid S-methyl ester (BTH) can increase the activity of defense enzymes, including PPO, POD, and CAT in strawberries, enhancing the biocontrol efficacy (Zhang et al., 2015a,b), which provides evidence that the peach fruit treated by VOCs can activate defense enzymes in 3 day, and combined ROS index, it can be seen that VOCs effectively produce antioxidant activity to prevent oxidative damage of plant cells. Eventually, CHI and β-1,3-GLU can catalyze the hydrolysis of chitin and β-1,3-glucan on fungal cell walls (Meng et al., 2010). The previously reported studies revealed that 2,3-butanediol treatment could significantly increase the activity of PAL and CHI and induce Agrostis stolonifera for resistance against Rhizoctonia solani (Shi et al., 2018). In our study, the activity changes of the three pathogenic enzymes (PAL, CHI, and β-1,3-GLU) are similar to the antioxidant enzymes, which can act on the fungal cell well and effectively prevent infection by fungi.
In summary, the main results of this study are as follows: in vitro, CF-3 VOCs can inhibit spore germination of pathogenic fungi, cause abnormalities in cell morphology, and decrease cell membrane fluidity and cell wall integrity. In vivo, VOCs can reduce enzyme activities of pathogenic fungi that are involved in decomposition of plant tissues, activate antioxidant enzymes in fruits to remove excess ROS, thus preventing plant cell damage, and activate disease-resistant enzymes to prevent the invasion of pathogenic fungi, thereby inducing resistance to the fungi. CF-3 VOCs reduce fruit decay, and we may establish a foundation for broad applications in production.
Data Availability
The raw data supporting the conclusions of this manuscript will be made available by the authors, without undue reservation, to any qualified researcher.
Author Contributions
HG, PL, and MZ designed the experiments. PL, MZ, and PZ performed the experiments. PL, MZ, SW, and PZ analyzed the data. HG, MZ, PL, and SW drafted the manuscript. All authors read and approved the final manuscript.
Funding
This research was supported by the project of Science and Technology Innovation Action Plan of Shanghai Science and Technology Commission of China (No. 18391901300) and National Science-technology Support Plan of China (No. 2015BAD16B02).
Conflict of Interest Statement
The authors declare that the research was conducted in the absence of any commercial or financial relationships that could be construed as a potential conflict of interest.
References
Ahimou, F., and Deleu, M. J. P. (1999). Surfactin and Iturin A effects on Bacillus subtilis surface hydrophobicity. Enzym. Microb. Technol. 27, 749–754. doi: 10.1016/S0141-0229(00)00295-7
Ambrico, A., and Trupo, M. (2017). Efficacy of cell free supernatant from Bacillus subtilis ET-1, an Iturin A producer strain, on biocontrol of green and gray mold. Postharvest Biol. Technol. 134, 5–10. doi: 10.1016/j.postharvbio.2017.08.001
Arrebola, E., Sivakumar, D., and Korsten, L. (2010). Effect of volatile compounds produced by Bacillus strains on postharvest decay in citrus. Biol. Control 53, 122–128. doi: 10.1016/j.biocontrol.2009.11.010
Bayer, A. S., Presad, R., Chandra, J., Koul, A., Smriti, M., Varma, A., et al. (2000). In vitro resistance of Staphylococcus aureus to thrombin-induced platelet microbicidal protein is associated with alterations in cytoplasmic membrane fluidity. Infect. Immun. 68, 3548–3553. doi: 10.1128/IAI.68.6.3548-3553.2000
Cronan, J. E. (2003). Bacterial membrane lipids: where do we stand? Annu. Rev. Microbiol. 57, 203–224. doi: 10.1146/annurev.micro.57.030502.090851
Gao, H. Y., Xu, X. X., Dai, Y. W., and He, H. X. (2016). Isolation, identification and characterization of Bacillus subtilis CF-3, a bacterium from fermented bean curd for controlling postharvest diseases of peach fruit. Food Sci. Technol. Res. 22, 377–385. doi: 10.3136/fstr.22.377
Gao, H. Y., Xu, X. X., Zeng, Q., and Li, P. Z. (2017). Optimization of headspace solid-phase microextraction for GC-MS analysis of volatile compounds produced by biocontrol strain Bacillus subtilis CF-3 using response surface methodology. Food Sci. Technol. Res. 23, 583–593. doi: 10.3136/fstr.23.583
Gao, H. Y., Li, P. Z., Xu, X. X., Zeng, Q., and Guan, W. Q. (2018). Research on volatile organic compounds from Bacillus subtilis CF-3: biocontrol effects on fruit fungal pathogens and dynamic changes during fermentation. Front. Microbiol. 9:456. doi: 10.3389/fmicb.2018.00456
Gava, C. A. T., Alves, Í. L. S., and Duarte, C. D. (2019). Timing the application of Bacillus subtilis QST 713 in the integrated management of the postharvest decay of mango fruits. Crop Port. 121, 51–56. doi: 10.1016/j.cropro.2019.03.013
Heaton, N. S., and Randall, G. (2011). Multifaceted roles for lipids in viral infection. Trends Microbiol. 19, 368–375. doi: 10.1016/j.tim.2011.03.007
Hu, M. J., Cox, K. D., Schnabel, G., and Luo, C. X. (2011). Monilinia species causing brown rot of peach in China. PLoS One 6:e24990. doi: 10.1371/journal.pone.0024990
Jiang, C. M., Shi, J. L., Liu, Y. L., and Zhu, C. Y. (2014). Inhibition of Aspergillus carbonarius and fungal contamination in table grapes using Bacillus subtilis. Food Control 35, 41–48. doi: 10.1016/j.foodcont.2013.06.054
Leelasuphakul, W., Hemmanee, P., and Chuenchitt, S. (2008). Growth inhibitory properties of Bacillus subtilis strains and their metabolites against the green mold pathogen (Penicillium digitatum Sacc.) of citrus fruit. Postharvest Biol. Technol. 48, 113–121. doi: 10.1016/j.postharvbio.2007.09.024
Liu, W. H., Ding, B., Ruan, X. M., Xu, H. T., Yang, J., and Liu, S. M. (2007). Analysis of free and conjugated phytosterols in tobacco by an improved method using gas chromatography-flame ionization detection. J. Chromatogr. A 1163, 304–311. doi: 10.1016/j.chroma.2007.06.043
Liu, W., Wei, M., Zhu, B., and Liu, F. (2008). Antifungal activities and components of VOCs produced by Bacillus subtilis G8. Cur. Res. Bacteriol. 5, 28–34. doi: 10.3923/crb.2008.28.34
Lu, H. P., Lu, L. F., Zeng, L. Z., Fu, D., Xiang, H. L., Yu, T., et al. (2014). Effect of chitin on the antagonistic activity of Rhodosporidium paludigenum against Penicillium expansum in apple fruit. Postharvest Biol. Technol. 92, 9–15. doi: 10.1016/j.postharvbio.2014.01.009
Lupetti, A., Danesi, R., Campa, M., Del Tacca, M., and Kelly, S. (2002). Molecular basis of resistance to azole antifungals. Trends Mol. Med. 8, 76–81. doi: 10.1016/S1471-4914(02)02280-3
Meng, X., Yang, L., Kennedy, J. F., and Tian, S. (2010). Effects of chitosan and oligochitosan on growth of two fungal pathogens and physiological properties in pear fruit. Carbohydr. Polym. 81, 70–75. doi: 10.1016/j.carbpol.2010.01.057
Moyne, A. L., Cleveland, T. E., and Tuzun, S. (2004). Molecular characterization and analysis of the operon encoding the antifungal lipopeptide bacillomycin D. FEMS Microbiol. Lett. 234, 43–49. doi: 10.1111/j.1574-6968.2004.tb09511.x
Ongena, M., Jacques, P., Touré, Y., Destain, J., Jabrane, A., and Thonart, P. (2005). Involvement of fengycin-type lipopeptides in the multifaceted biocontrol potential of Bacillus subtilis. Appl. Microbiol. Biotechnol. 69, 29–38. doi: 10.1007/s00253-005-1940-3
Pasanen, A. L., Yli-pietil, K., Pasanen, P., Kalliokoski, P., and Tarhanen, J. (1999). Ergosterol content in various fungal species and biocontaminated building materials. Appl. Environ. Microbiol. 65, 138–142.
Prade, R. A., Zhan, D., Ayoubi, P., and Mort, A. J. (1999). Pectins, pectinases and plant-microbe interactions. Biotechnol. Genet. Eng. Rev. 16, 361–392. doi: 10.1080/02648725.1999.10647984
Saraf, A., Larsson, L., Burge, H., and Milton, D. (1997). Quantification of ergosterol and 3-hydroxy fatty acids in settled house dust by gas chromatography-mass spectrometry: comparison with fungal culture and determination of endotoxin by a limulus amebocyte lysate assay. Appl. Environ. Microbiol. 63, 2554–2559.
Schreinemachers, P., and Tipraqsa, P. (2012). Agricultural pesticides and land use intensification in high, middle and low income countries. Food Policy 37, 616–626. doi: 10.1016/j.foodpol.2012.06.003
Senthil, R., Prabakar, K., Rajendran, L., and Karthikeyan, G. (2011). Efficacy of different biological control agents against major postharvest pathogens of grapes under room temperature storage conditions. Phytopathol. Mediterr. 50, 55–65. doi: 10.14601/Phytopathol_Mediterr-3115
Sethu Priya, K. M., Parbha, T. N., and Tharanathan, R. N. (1996). Postharvest biochemical changes associated with the softening phenomenon in Capsicum annuum fruits. Phytochemistry 42, 961–966. doi: 10.1016/0031-9422(96)00057-X
Sha, Y. F., Deng, C. H., and Liu, B. Z. (2008). Development of C18-functionalized magnetic silica nanoparticles as sample preparation technique for the determination of ergosterol in cigarettes by microwave-assisted derivatization and gas chromatography/mass spectrometry. J. Chromatogr. A 1198-1199, 27–33. doi: 10.1016/j.chroma.2008.05.049
Shi, Y., Liu, X. J., Fang, Y. Y., Tian, Q., Jiang, H. Y., and Ma, H. L. (2018). 2, 3-Butanediol activated disease-resistance of creeping bentgrass by inducing phytohormone and antioxidant responses. Plant Physiol. Biochem. 129, 244–250. doi: 10.1016/j.plaphy.2018.06.010
Svircev, A. M., Smith, R. J., Zhou, T., Hernadez, M., Liu, W. T., and Chu, C. L. (2007). Effects of thymol fumigation on survival and ultrastracture of Monilinia fructicola. Postharvest Biol. Technol. 45, 228–233. doi: 10.1016/j.postharvbio.2006.12.021
Tang, Y., Li, Y. Y., Li, H. M., Wan, D. J., and Tang, Y. J. (2011). Comparison of lipid content and fatty acid composition between tuber fermentation mycelia and natural fruiting bodies. J. Agric. Food Chem. 59, 4736–4742. doi: 10.1021/jf200141s
Vespermann, A., Kai, M., and Piechulla, B. (2007). Rhizobacterial volatiles affect the growth of fungi and Arabidopsis thaliana. Appl. Environ. Microbiol. 73, 5639–5641. doi: 10.1128/AEM.01078-07
Waewthongrak, W., Pisuchpen, S., and Leelasuphakul, W. (2015). Effect of Bacillus subtilis and chitosan applications on green mold (Penicilium digitatum Sacc.) decay in citrus fruit. Postharvest Biol. Technol. 99, 44–49. doi: 10.1016/j.postharvbio.2014.07.016
Zhang, B., Li, Y., Zhang, Y. Y., Qiao, H. T., He, J. T., Yuan, Q., et al. (2019). High-cell-density culture enhances the antimicrobial and freshness effects of Bacillus subtilis S1702 on table grapes (Vitis vinifera cv. Kyoho). Food Chem. 286, 541–549. doi: 10.1016/j.foodchem.2019.02.050
Zhang, X. Y., Sun, Y., Yang, Q. Y., Chen, L. L., Li, W. H., and Zhang, H. Y. (2015a). Control of postharvest black rot caused by Alternaria alternata in strawberries by the combination of Cryptococcus laurentii and Benzo-(1,2,3)-thiadiazole-7-carbothioic acid S-methyl ester. Biol. Control 90, 96–101. doi: 10.1016/j.biocontrol.2015.05.018
Zhang, Y. Y., Zeng, L. Z., Yang, J. L., Zheng, X. D., and Yu, T. (2015b). 6-Benzylaminopurine inhibits growth of Monilinia fructicola and induces defense-related mechanism in peach fruit. Food Chem. 187, 210–217. doi: 10.1016/j.foodchem.2015.04.100
Zheng, M., Shi, J. Y., Shi, J., Wang, Q. G., and Li, Y. H. (2013). Antimicrobial effects of volatiles produced by two antagonistic Bacillus strains on the anthracnose pathogen in postharvest mangos. Biol. Control 65, 200–206. doi: 10.1016/j.biocontrol.2013.02.004
Keywords: Bacillus subtilis CF-3, volatile organic compounds, Monilinia fructicola, biological control, antifungal effect
Citation: Zhou M, Li P, Wu S, Zhao P and Gao H (2019) Bacillus subtilis CF-3 Volatile Organic Compounds Inhibit Monilinia fructicola Growth in Peach Fruit. Front. Microbiol. 10:1804. doi: 10.3389/fmicb.2019.01804
Edited by:
Javier Carballo, University of Vigo, SpainReviewed by:
Iuliia Khomenko, Fondazione Edmund Mach, ItalyFrédérique Reverchon, Instituto de Ecología (INECOL), Mexico
Copyright © 2019 Zhou, Li, Wu, Zhao and Gao. This is an open-access article distributed under the terms of the Creative Commons Attribution License (CC BY). The use, distribution or reproduction in other forums is permitted, provided the original author(s) and the copyright owner(s) are credited and that the original publication in this journal is cited, in accordance with accepted academic practice. No use, distribution or reproduction is permitted which does not comply with these terms.
*Correspondence: Haiyan Gao, aHlnYW8xMTExQDEyNi5jb20=
†These authors have contributed equally to this work