- 1Laboratório Especial de Micologia, Disciplina de Infectologia, Universidade Federal de São Paulo, São Paulo, Brazil
- 2Central Laboratory Division, Hospital das Clínicas da Faculdade de Medicina da Universidade de São Paulo, São Paulo, Brazil
The cell wall is an essential component in fungal homeostasis. The lack of a covering wall in human cells makes this component an attractive target for antifungal development. The host environment and antifungal stress can lead to cell wall modifications related to drug resistance. Antifungals targeting the cell wall including the new β-D-glucan synthase inhibitor ibrexafungerp and glycosyl-phosphatidyl Inositol (GPI) anchor pathway inhibitor fosmanogepix are promising weapons against antifungal resistance. The fosmanogepix shows strong in vitro activity against the multidrug-resistant species Candida auris, Fusarium solani, and Lomentospora prolificans. The alternative carbon sources in the infection site change the cell wall β-D-glucan and chitin composition, leading to echinocandin and amphotericin resistance. Candida populations that survive echinocandin exposure develop tolerance and show high chitin content in the cell wall, while fungal species such as Aspergillus flavus with a higher β-D-glucan content may show amphotericin resistance. Therefore understanding fungal cell dynamics has become important not only for host-fungal interactions, but also treatment of fungal infections. This review summarizes recent findings regarding antifungal therapy and development of resistance related to the fungal cell wall of the most relevant human pathogenic species.
Introduction
The cell wall is an essential component in homeostasis of fungal cells (Latgé, 2007; Gow et al., 2017). It also has a dual interaction process with the surrounding environment, which either negatively or positively impacts fungal cell survival. Cell wall antigens induce immune recognition by the infected host and facilitate phagocytosis (Roy and Klein, 2012). Some antigens, named pathogen-associated molecular patterns (PAMPs), are recognized by a wide range of pattern-recognition receptors (PRRs) on host cell surfaces (Roy and Klein, 2012). Conversely, environmental stresses lead to cell wall modifications that impede immune recognition (Gow et al., 2017).
Representing approximately 40% of the total fungal cell volume, the fungal cell wall forms a tensile and robust core scaffold to which a variety of proteins and superficial components with fibrous and gel-like carbohydrates form polymers, making a strong but flexible structure (Munro, 2013; Gow et al., 2017). Most cell walls have two layers: (1) the inner layer comprising a relatively conserved structural skeleton and (2) the outer layer which is more heterogeneous and has species-specific peculiarities (Gow et al., 2017). The inner cell wall represents the loadbearing, structural component of the wall that resists the substantial internal hydrostatic pressure exerted on the wall by the cytoplasm and membrane (Latgé, 2007). This layer includes chitin and glucan, in which 50–60% of the dry weight of the cell wall is made up of β-(1-3)-glucan. The outer-layer structure consists of heavily mannosylated glycoproteins with modified N- and O- linked oligosaccharides. The structure of these oligosaccharide side chains differs among fungal species (Shibata et al., 1995; Hobson et al., 2004).
Since human cells do not have a covering wall, antifungals that target the production of cell wall components are more selective and less toxic when compared to azole derivatives and amphotericin B (Patil and Majumdar, 2017). Echinocandins were the first systemic antifungals that targeted the cell wall by disrupting the production of glucans (Patil and Majumdar, 2017). For invasive candidiasis, echinocandins were a great development that lowered the mortality associated with these infections, with low toxicity and few interactions with other medication (Mora-Duarte et al., 2002; Pappas et al., 2016). However, intrinsic and acquired resistance to echinocandins limits its usefulness, leading to research into other targets in the fungal cell wall for antifungal therapy (Hasim and Coleman, 2019).
Cell wall dynamics may play an important role for the development of antifungal resistance and interesting concepts regarding this subject are emerging. Structural and cell wall composition modifications have been investigated in Candida and Aspergillus isolates presenting antifungal resistance (Seo et al., 1999; Mesa-Arango et al., 2016). In echinocandin-tolerant Candida isolates, β-1,3- and β-1,6-glucans crosslinks modifications and higher chitin content have been described (Perlin, 2015), while higher β-D-glucan composition has been found in amphotericin-B-resistant Aspergillus flavus isolates (Seo et al., 1999).
In this manuscript, we review the fungal cell wall as a target for antifungal therapy and, in conjunction, visit cell wall modifications that may be related to antimicrobial resistance.
Fungal Cell Wall Targeting Antifungals
Antifungals targeting the cell wall have been developed in the last years (Walker et al., 2011; Chaudhary et al., 2013; Mutz and Roemer, 2016; Hasim and Coleman, 2019). Most of these drugs act by inhibiting β-D-glucan synthase, but chitin synthase and glycosylphosphatidylinositol (GPI) anchor pathway inhibitors are also under development (Figure 1A).
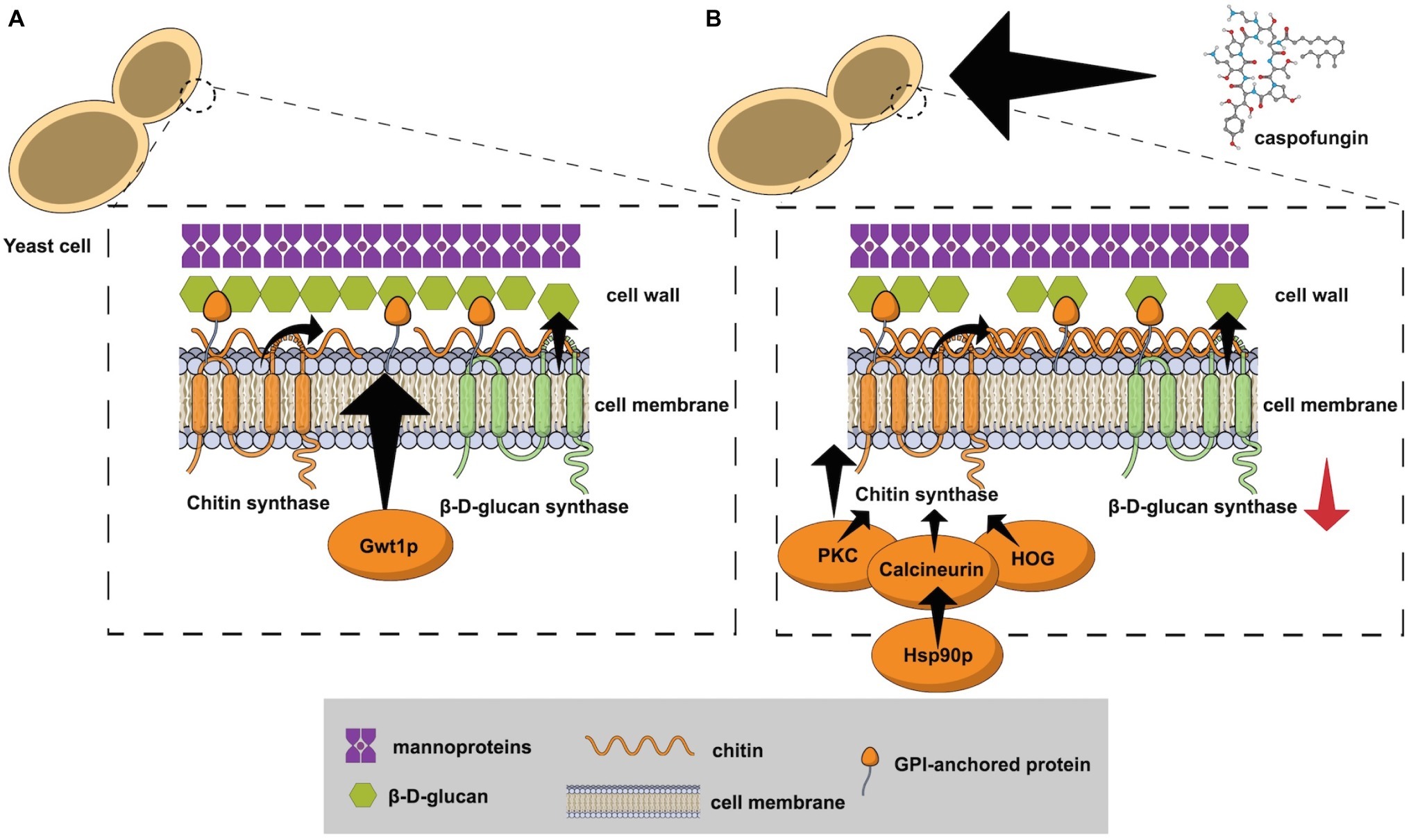
Figure 1. (A) The fungal cell wall and the targets that have been explored for antifungal development: β-D-glucan synthase, chitin synthase, and the enzyme Gwt1 from the GPI anchor pathway; (B) Echinocandin exposure causes cell wall stress by inhibition of the β-D-glucan synthase. The protein kinase C (PKC), high osmolarity glycerol response (HOG), and Ca+2-calcineurin pathways have been implicated in the response to cell wall damage and chitin synthase hyper stimulation. Calcineurin is a client protein for the Hsp90 chaperone and genetic compromise of the gene HSP90 reduces the tolerance mechanism.
1,3-β-D-Glucan Synthase Inhibitors
Echinocandins
Echinocandins were first described in the 1970’s as antibiotic polypeptides obtained from Aspergillus nidulans (Nyfeler and Keller-Schierlein, 1974). These molecules are basically hexapeptide antibiotics with N-linked acyl fatty acid chains that intercalates with the phospholipid layer of the cell membrane (Denning, 2003). This antifungal class inhibits the β-D-glucan synthase, which leads to a decrease of the β-D-glucans in the cell wall after noncompetitively binding to the Fksp subunit of the enzyme (Hector, 1993; Denning, 2003; Aguilar-Zapata et al., 2015; Perlin, 2015; Patil and Majumdar, 2017).
The fungal cell wall β-D-glucan synthase complex has two main subunits: Fks1p and Rho1p (Mazur and Baginsky, 1996; Aguilar-Zapata et al., 2015). Fks1p is the catalytic subunit responsible for the production of glycosidic bonds (Schimoler-O’Rourke et al., 2003), while Rho1p is a Ras-like GTP-binding protein that regulates the β-D-glucan synthase activity (Qadota et al., 1996).
Inhibition of β-D-glucan synthase results in the cell death of the Candida species, while echinocandins modify the hyphae morphogenesis and exert a fungistatic effect against Aspergillus species (Bowman et al., 2002). Conversely, species belonging to the order Mucorales and the basidiomycetes are intrinsically resistant to this antifungal class (Espinel-Ingroff, 2003; Aguilar-Zapata et al., 2015).
Currently, there are three echinocandins approved by the FDA for the treatment of invasive fungal infections: caspofungin, anidulafungin, and micafungin (Johnson and Perfect, 2003; Rüping et al., 2008; Pappas et al., 2016). Compared to other antifungal classes, the echinocandins show lower kidney or liver toxicity, fewer drug–drug interactions, and have predominant liver elimination, not requiring dose adjustments during renal failure or dialysis (Aguilar-Zapata et al., 2015). However, echinocandins have pharmacokinetic limitations, such as poor bioavailability upon oral administration, high protein binding, and low central nervous system (CNS) penetration (Wiederhold and Lewis, 2003). New glucan synthase inhibitors with better pharmacokinetics profiles, including oral formulations with high bioavailability, are under investigation (Davis et al., 2019).
Rezafungin (CD101, formerly SP3025, Cidara Therapeutics, San Diego, CA, USA), a next-generation echinocandin, is currently in Phase 3 of clinical trials for the treatment of candidemia and invasive candidiasis1. This antifungal is a structural analog of anidulafungin, but with a choline moiety replacing the hemiaminal group at the C5 ornithine position, resulting in a stable compound with prolonged half-life (Sandison et al., 2017). It is highly soluble in aqueous systems and has a half-life of over 130 h in humans, compared to 24, 9–11, 10–17 h half-lives of anidulafungin, caspofungin, and micafungin, respectively (Kofla and Ruhnke, 2011; Sandison et al., 2017). The long half-life of rezafungin allows an advantageous weekly dosing regimen (Sandison et al., 2017; Sofjan et al., 2018).
Rezafungin has potent in vitro activity against common Candida and Aspergillus species (Wiederhold et al., 2018; Arendrup et al., 2018a,b). Furthermore, this antifungal has strong in vitro antifungal activity against the potential multidrug-resistant species C. auris (Berkow and Lockhart, 2018). Moreover, the in vivo efficacy of rezafungin in neutropenic murine disseminated candidiasis models was demonstrated against C. albicans, C. glabrata, C. parapsilosis (Lepak et al., 2018), and C. auris (Hager et al., 2018a).
Triterpenoids
The triterpenoid class is represented by ibrexafungerp (SCY-078, formerly MK-3118), a new semisynthetic derivate of hemiacetal triterpene glycoside enfumafungin (Synexis Inc., Jersey City, NJ, USA) (Pfaller et al., 2017; Wring et al., 2017; Davis et al., 2019). It is a β-D-glucan synthase inhibitor with similar but not identical biding sites to echinocandins in the catalytic regions Fks1p and Fks2p of the enzyme (Walker et al., 2011; Jiménez-Ortigosa et al., 2017). It has high protein binding and good tissue penetration, although like echinocandins, it has poor CNS penetration (Davis et al., 2019). The pharmacokinetic gain of this new antifungal is its good oral bioavailability (Walker et al., 2011).
Ibrexafungerp has demonstrated good in vitro activity against relevant fungal pathogens such as Candida spp., including multidrug-resistant C. glabrata (Pfaller et al., 2013, 2017; Jiménez-Ortigosa et al., 2017), biofilm producer strains (Marcos-Zambrano et al., 2017b), and C. auris (Larkin et al., 2017). Notably, echinocandin-resistant Candida strains harboring hot spot mutations at the Fksp may retain susceptibility to ibrexafungerp (Pfaller et al., 2017). A more in-depth study analyzing C. glabrata strains with echinocandin resistance and ibrexafungerp susceptibility showed that ibrexafungerp has only partial overlapping at the echinocandins Fksp biding sites in the β-D-glucan synthase enzyme (Jiménez-Ortigosa et al., 2017). Against Aspergillus clinically relevant species, ibrexafungerp has also demonstrated potent in vitro activity (Davis et al., 2019). Moreover, the combination of ibrexafungerp with either voriconazole or amphotericin B has demonstrated synergy against wild-type A. fumigatus strains (Ghannoum et al., 2018). Noteworthy, ibrexafungerp showed some antifungal activity against the multidrug-resistant mold Lomentospora prolificans (Lamoth and Alexander, 2015), and it is highly active against Paecilomyces variotii (Lamoth and Alexander, 2015). However, ibrexafungerp has little activity against Mucorales spp., Fusarium spp., and Purpureocillium lilacinum (Lamoth and Alexander, 2015). The in vitro activity of ibrexafungerp is summarized in Table 1.
In time-to-kill experiments, ibrexafungerp showed mainly fungicidal activity against Candida albicans and non-albicans isolates (Scorneaux et al., 2017). For in vivo murine models of invasive candidiasis caused by C. albicans, C. glabrata, and C. parapsilosis, this drug showed similar concentration-dependent killing of the three Candida species (Lepak et al., 2015).
This antifungal is currently in clinical trials for the treatment of vulvovaginal candidiasis (Phase 3; https://clinicaltrials.gov/ct2/show/NCT03987620), for invasive aspergillosis in combination with voriconazole (Phase 2; https://clinicaltrials.gov/ct2/show/NCT03672292), invasive and mucosal candidiasis (Phase 3; https://clinicaltrials.gov/ct2/show/NCT03059992), and for invasive candidiasis due to C. auris (Phase 3; https://clinicaltrials.gov/ct2/show/NCT03363841).
Chitin Synthase Inhibitors
Chitin is an essential component of the fungal cell wall and compounds that affect its synthesis have been investigated as antifungals, such as nikkomycins, polyoxins, and plagiochin (Chaudhary et al., 2013).
Nikkomycins are peptidyl nucleoside agents that competitively inhibit chitin synthase (CHS). Nikkomycin Z has some in vitro activity against C. parapsilosis, Coccidioides immitis, and Blastomyces dermatitidis (Hector et al., 1990), but its usefulness relies on the synergism with echinocandins for C. albicans, A. fumigatus, and C. immitis (Chiou et al., 2001; Cheung and Hui, 2017). One study using a murine model of invasive candidiasis showed that Nikkomycin Z plus echinocandins were effective for the treatment of infections by echinocandin-resistant C. albicans (Cheung and Hui, 2017).
Glycosylphosphatidyl Inositol Anchor Pathway Inhibitors
Glycosylphosphatidyl inositol (GPI) is a component of the eukaryotes cell wall and is synthesized in the endoplasmic reticulum by a conserved pathway (Ikezawa, 2002). GPI glycolipids anchor different proteins to the cell wall and are essential for its integrity (Yadav and Khan, 2018).
Antifungals targeting GPI anchor synthesis pathway have been developed in the last 15 years (Tsukahara et al., 2003; Mutz and Roemer, 2016). One of the targets of the GPI anchor synthesis pathway is the protein Gwt1 (GPI-anchored wall protein transfer 1), an inositol acyltransferase that catalyzes inositol acylation (Tsukahara et al., 2003; Hata et al., 2011). Inhibition of Gwt1 compromises cell wall integrity, biofilm production, germ tube formation, and produces severe fungal growth defects (Yadav and Khan, 2018). In C. albicans and Saccharomyces cerevisiae, Gwt1 inhibition has been shown to jeopardize the maturation and stabilization of GPI-anchored mannoproteins (McLellan et al., 2012). The first compound used to inhibit the Gwt1 enzyme was the molecule 1-(4-butylbenzyl) isoquinoline (BIQ), described by Tsukahara et al. (2003).
From the BIQ molecule, a new compound with higher antifungal potency was created by the Tsukuba Research Laboratories of Eisai Co., Ltd. (Ibaraki, Japan), the APX001A or manogepix (formerly E1210) (Hata et al., 2011). Later, Amplix Pharmaceuticals Inc. (San Diego, CA, USA) developed the N-phosphonooxymethyl prodrug fosmanogepix (APX001, formerly E1211) with oral and IV formulations. The prodrug is metabolized by phosphatases and converted to manogepix (APX001A, formerly E1210) which inhibits the Gwt1 but not the human homolog Pig-W (Watanabe et al., 2012; Wiederhold et al., 2019). The oral formulation of fosmanogepix presented good bioavailability in murine experiments (Zhao et al., 2018).
The in vitro activity of manogepix has been investigated against yeasts and molds (Miyazaki et al., 2011; Castanheira et al., 2012). Low minimal inhibitory concentrations (MICs) of this new antifungal were found against C. albicans, C. tropicalis, C. glabrata, C. parapsilosis, C. lusitaniae, C. kefyr, (Miyazaki et al., 2011; Pfaller et al., 2019), and also against multidrug-resistant C. auris (Hager et al., 2018a), and echinocandin-resistant C. glabrata (Pfaller et al., 2019). However, in vitro results against C. krusei and C. norvegensis have been described as poor (Arendrup et al., 2018a). Potent in vitro activity of manogepix was also noticed against Cryptococcus neoformans and Cryptococcus gattii strains (Shaw et al., 2018; Pfaller et al., 2019). Regarding in vitro activity against molds, low MICs against Aspergillus species from the Section Fumigati, Flavi, Terrei, and Nigri (Miyazaki et al., 2011; Pfaller et al., 2019), Purpureocillium lilacinum, Cladosporium species, Phialophora species, Rhinocladiella aquaspersa, Fonsecaea pedrosoi (Miyazaki et al., 2011), Scedosporium apiospermum, and Scedosporium aurantiacum (Castanheira et al., 2012), and against the multidrug-resistant species Fusarium solani and L. prolificans (Castanheira et al., 2012). The in vitro activity of manogepix is summarized in Table 1.
The in vivo activity of manogepix/fosmanogepix has been also investigated in murine models of disseminated candidiasis, aspergillosis, fusariosis (Hata et al., 2011; Hager et al., 2018b), and Coccidioides immitis pneumonia (Viriyakosol et al., 2019). In a murine model of disseminated C. albicans infection, it showed similar efficacy to caspofungin, fluconazole, and liposomal amphotericin B (Hata et al., 2011). Another study compared the efficacy of manogepix/fosmanogepix and anidulafungin for the treatment of mouse with disseminated C. auris infection and found higher survival rates in the group treated with the Gwt1 inhibitor (Hager et al., 2018b). In a murine model of invasive Aspergillus flavus infection, mice treated with this new antifungal had similar survival rates when compared to the groups treated with either voriconazole or caspofungin (Hata et al., 2011). In the same study, mice infected by F. solani showed a higher survival rate when treated with fosmanogepix 20 mg/kg compared to the control group without antifungal therapy (Hata et al., 2011).
There is currently a Phase 2, single-arm, and open-label trial of fosmanogepix for the first-line treatment of candidemia2.
Fungal Cell Wall Modifications and Antifungal Resistance
Modifications in fungal cell wall architecture appear after stresses produced by the host microenvironment and antifungal exposure (Ene et al., 2012; Perlin, 2015; Mesa-Arango et al., 2016).
In vitro studies have shown in conditions that mimic the host microenvironment at the infection site that yeast cells may develop wall modifications and antifungal resistance (Ene et al., 2012; Brown et al., 2014). C. albicans cells grown in serum (<0.1% glucose) show major changes in the cell wall architecture, with a decrease in the length of mannan chains, and in the chitin and β-glucan content (Ene et al., 2012). Moreover, growth-challenging conditions with alternative carbon sources, such as lactate, alter cell wall biosynthesis, leading to the production of a leaner but stiffer inner cell wall (Ene et al., 2012). These cell wall-remodeled C. albicans cells become resistant to amphotericin B (AMB) and caspofungin (Ene et al., 2012). Similar results were demonstrated for C. glabrata strains that grown under an alternative carbon microenvironment showed altered cell wall architecture with a lower content of chitin and β-glucan, and with an increased outer mannan layer (Chew et al., 2019). These C. glabrata cells were also resistant to AMB when grown in lactate or oleate (Chew et al., 2019).
An intermediary step to antifungal resistance is the development of tolerance (Perlin, 2015). Cells surviving drug exposure can respond to selection and evolve resistance (Perlin, 2015). Echinocandin exposure causes cell wall stress by inhibition of the β-D-glucan synthesis, which triggers adaptive cellular factors that stimulate chitin production (Walker et al., 2008, 2010). Protein kinase C (PKC), high osmolarity glycerol response (HOG), and Ca+2-calcineurin pathways have been implicated in the response to cell wall damage and chitin synthesis (Figure 1B; Lagorce et al., 2003; Bermejo et al., 2008; Walker et al., 2008; Fortwendel et al., 2009). The chaperone Hsp90 is another crucial component for echinocandins tolerance after cell wall stress (Singh et al., 2009; O’Meara et al., 2017). Calcineurin is a client protein for the Hsp90 chaperone and genetic compromise of the gene HSP90 reduced the tolerance mechanism in C. albicans (Singh et al., 2009), C. glabrata (Singh-Babak et al., 2012), and Aspergillus fumigatus (Lamoth et al., 2014). Another expression of fungal adaptive mechanisms caused by antifungal stress is called the parodoxal effect, which is the recuperation of fungal growth after exposure to antifungals at increasing concentrations above a certain threshold (Aruanno et al., 2019). This phenomenon has been reported in Candida spp. and Aspergillus spp. after exposure to echinocandins, mainly caspofungin (Rueda et al., 2014; Marcos-Zambrano et al., 2017a; Aruanno et al., 2019). Similar to the tolerance mechanism, the paradoxical effect is related to intracellular signaling pathways that lead to cell wall remodeling with increase of the chitin and loss of β-D-glucan content (Aruanno et al., 2019). In A. fumigatus, caspofungin exposure may also lead to an increase in reactive oxygen species (ROS) production and to modifications of the lipid microenvironment surrounding the β-D-glucan synthase, leading to echinocandins resistance (Satish et al., 2019).
In C. albicans, other relevant components for echinocandin tolerance may be located at chromosome 5 (Ch5), since some tolerant mutants showed either monosomy of the Ch5, or combined monosomy of the left arm and trisomy of the right arm of Ch5 (Yang et al., 2017). Eventually, persistent echinocandin exposure leads to FKS mutations and organisms with marked and stable resistance emerge with a high chitin content in the cell wall (Walker et al., 2013; Perlin, 2015). FKS mutations in Candida species and echinocandin resistance have been extensively reviewed elsewhere (Walker et al., 2010; Perlin, 2015).
AMB resistance may be explained by multiple mechanisms, among them modifications in the cell wall architecture (Seo et al., 1999; Mesa-Arango et al., 2016). Aspergillus flavus isolates with AMB resistance have been related to invasive fungal infections with poor prognosis in neutropenic patients (Koss et al., 2002; Hadrich et al., 2012). Seo, Akiyoshi, and Ohnishi demonstrated that in vitro AMB-resistant mutant strains of A. flavus have similar sterol content in the cell membrane when compared to susceptible strains (Seo et al., 1999). Conversely, the cell wall from the resistant mutants contained more 1,3-β-D-glucan when compared to susceptible strains (Seo et al., 1999). The authors suggest that the higher content of glucans found in the resistant mutants helps to adsorb AMB, making it more difficult for the antifungal to reach the cell membrane (Seo et al., 1999). Comparisons between biofilm (AMB-resistant) and planktonic (AMB-susceptible) C. albicans cells revealed that the cell wall from the biofilm-grown isolates are thicker and have more β-1,3-glucans (Nett et al., 2007). In C. tropicalis, AMB resistance has been linked to several potential mechanisms, such as increase in catalase activity, changes in mitochondrial potential, low accumulation of reactive oxygen species, and deficiency in ergosterol at the cell membrane (Forastiero et al., 2013; Mesa-Arango et al., 2014). More recently, cell wall modifications have also been found in AMB-resistant C. tropicalis isolates (Mesa-Arango et al., 2016). The AMB-resistant isolates showed thicker cell walls with higher volume when compared to susceptible isolates (Mesa-Arango et al., 2016). Besides, these AMB-resistant organisms had a 2- to 3-fold increase of β-1,3-glucans in the cell wall (Mesa-Arango et al., 2016).
Conclusions and Perspectives
Recent advances in the science of the fungal cell wall have opened the doors to new therapeutic modalities for fungal infections, and have helped to better understand the mechanisms of antifungal resistance. New antifungals targeting the cell wall show better safety and PK/PD profiles than the available toxic polyenes and azole derivative molecules. The new β-D-glucan synthase inhibitor ibrexafungerp has potent in vitro activity against multidrug-resistant pathogens such as echinocandin-resistant C. glabrata, C. auris, and Aspergillus species.
Glucan synthase inhibitors such as Nikkomycin Z have strong synergism with echinocandins and may be useful for the treatment of echinocandins-resistant Candida infections and refractory aspergillosis.
The GPI anchor pathway inhibitors APX001/APX001A have good pharmacokinetic profiles and strong in vitro activity against several fungal pathogenic species, including multiresistant C. auris, F. solani, and L. prolificans. This makes these drugs the most promising antifungals to be launched in the future.
The microenvironment at the infection site leads to modification in the fungal cell wall, which may lead to antifungal resistance. Cell wall stress induced by echinocandin exposure leads to the emergence of tolerant cells with high chitin content. The PKC, HOG, and Ca+2-calcineurin pathways, as well as the chaperone Hsp90, are crucial components for the phenomenon of antifungal tolerance and should be explored as future targets for antifungal therapy. A few AMB-resistant A. flavus and C. tropicalis showed higher content of glucans in the cell wall, but further studies analyzing the cell wall modifications and AMB resistance are necessary to increase the strength of this correlation.
Author Contributions
SL, AC, and JA conceived of the manuscript. SL and JA conducted the literature review. SL, AC, and JA wrote the manuscript. AC revised the manuscript.
Funding
The work of SL is supported by CAPES (Grant 88882.430766/2019-01). The work of JA is supported by FAPESP (Grant 2018/18347-4). AC received grants from CNPq (Grant 307510/2015-8) and FAPESP (Grant 2017/02203-7).
Conflict of Interest
The authors declare that the research was conducted in the absence of any commercial or financial relationships that could be construed as a potential conflict of interest.
Footnotes
References
Aguilar-Zapata, D., Petraitiene, R., and Petraitis, V. (2015). Echinocandins: the expanding antifungal armamentarium. Clin. Infect. Dis. 61(Suppl. 6), S604–S611. doi: 10.1093/cid/civ814
Arendrup, M. C., Chowdhary, A., Astvad, K. M. T., and Jørgensen, K. M. (2018a). APX001A in vitro activity against contemporary blood isolates and Candida auris determined by the EUCAST reference method. Antimicrob. Agents Chemother. 62, pii: e01225-18. doi: 10.1128/AAC.01225-18
Arendrup, M. C., Meletiadis, J., Zaragoza, O., Jørgensen, K. M., Marcos-Zambrano, L. J., Kanioura, L., et al. (2018b). Multicentre determination of rezafungin (CD101) susceptibility of Candida species by the EUCAST method. Clin. Microbiol. Infect. 24, 1200–1204. doi: 10.1016/j.cmi.2018.02.021
Aruanno, M., Glampedakis, E., and Lamoth, F. (2019). Echinocandins for the treatment of invasive aspergillosis: from laboratory to bedside. Antimicrob. Agents Chemother. 63, pii: e00399-19. doi: 10.1128/AAC.00399-19
Berkow, E. L., and Lockhart, S. R. (2018). Activity of CD101, a long-acting echinocandin, against clinical isolates of Candida auris. Diagn. Microbiol. Infect. Dis. 90, 196–197. doi: 10.1016/j.diagmicrobio.2017.10.021
Bermejo, C., Rodríguez, E., García, R., Rodríguez-Peña, J. M., Rodríguez de la Concepción, M. L., Rivas, C., et al. (2008). The sequential activation of the yeast HOG and SLT2 pathways is required for cell survival to cell wall stress. Mol. Biol. Cell 19, 1113–1124. doi: 10.1091/mbc.e07-08-0742
Bowman, J. C., Hicks, P. S., Kurtz, M. B., Rosen, H., Schmatz, D. M., Liberator, P. A., et al. (2002). The antifungal echinocandin caspofungin acetate kills growing cells of Aspergillus fumigatus in vitro. Antimicrob. Agents Chemother. 46, 3001–3012. doi: 10.1128/AAC.46.9.3001-3012.2002
Brown, A. J. P., Budge, S., Kaloriti, D., Tillmann, A., Jacobsen, M. D., Yin, Z., et al. (2014). Stress adaptation in a pathogenic fungus. J. Exp. Biol. 217, 144–155. doi: 10.1242/jeb.088930
Castanheira, M., Duncanson, F. P., Diekema, D. J., Guarro, J., Jones, R. N., and Pfaller, M. A. (2012). Activities of E1210 and comparator agents tested by CLSI and EUCAST broth microdilution methods against Fusarium and Scedosporium species identified using molecular methods. Antimicrob. Agents Chemother. 56, 352–357. doi: 10.1128/AAC.05414-11
Chaudhary, P. M., Tupe, S. G., and Deshpande, M. V. (2013). Chitin synthase inhibitors as antifungal agents. Mini Rev. Med. Chem. 13, 222–236. doi: 10.2174/138955713804805256
Cheung, Y.-Y., and Hui, M. (2017). Effects of echinocandins in combination with Nikkomycin Z against invasive Candida albicans bloodstream isolates and the fks mutants. Antimicrob. Agents Chemother. 61, pii: e00619-17. doi: 10.1128/AAC.00619-17
Chew, S. Y., Ho, K. L., Cheah, Y. K., Sandai, D., Brown, A. J. P., and Than, L. T. L. (2019). Physiologically relevant alternative carbon sources modulate biofilm formation, Cell Wall architecture, and the stress and antifungal resistance of Candida glabrata. Int. J. Mol. Sci. 20, pii: E3172. doi: 10.3390/ijms20133172
Chiou, C. C., Mavrogiorgos, N., Tillem, E., Hector, R., and Walsh, T. J. (2001). Synergy, pharmacodynamics, and time-sequenced ultrastructural changes of the interaction between nikkomycin Z and the echinocandin FK463 against Aspergillus fumigatus. Antimicrob. Agents Chemother. 45, 3310–3321. doi: 10.1128/AAC.45.12.3310-3321.2001
Cordeiro, R. A., Brilhante, R. S. N., Rocha, M. F. G., Fechine, M. A. B., Costa, A. K. F., Camargo, Z. P., et al. (2006). In vitro activities of caspofungin, amphotericin B and azoles against Coccidioides posadasii strains from northeast, Brazil. Mycopathologia 161, 21–26. doi: 10.1007/s11046-005-0177-0
Davis, M. R., Donnelley, M. A., and Thompson, G. R. (2019). Ibrexafungerp: a novel oral glucan synthase inhibitor. Med. Mycol. pii: myz083. doi: 10.1093/mmy/myz083
Denning, D. W. (2003). Echinocandin antifungal drugs. Lancet 362, 1142–1151. doi: 10.1016/S0140-6736(03)14472-8
Ene, I. V., Adya, A. K., Wehmeier, S., Brand, A. C., MacCallum, D. M., Gow, N. A. R., et al. (2012). Host carbon sources modulate cell wall architecture, drug resistance and virulence in a fungal pathogen. Cell. Microbiol. 14, 1319–1335. doi: 10.1111/j.1462-5822.2012.01813.x
Espinel-Ingroff, A. (2003). In vitro antifungal activities of anidulafungin and micafungin, licensed agents and the investigational triazole posaconazole as determined by NCCLS methods for 12,052 fungal isolates: review of the literature. Rev. Iberoam. Micol. 20, 121–136.
Forastiero, A., Mesa-Arango, A. C., Alastruey-Izquierdo, A., Alcazar-Fuoli, L., Bernal-Martinez, L., Pelaez, T., et al. (2013). Candida tropicalis antifungal cross-resistance is related to different azole target (Erg11p) modifications. Antimicrob. Agents Chemother. 57, 4769–4781. doi: 10.1128/AAC.00477-13
Fortwendel, J. R., Juvvadi, P. R., Pinchai, N., Perfect, B. Z., Alspaugh, J. A., Perfect, J. R., et al. (2009). Differential effects of inhibiting chitin and 1,3-{beta}-D-glucan synthesis in ras and calcineurin mutants of Aspergillus fumigatus. Antimicrob. Agents Chemother. 53, 476–482. doi: 10.1128/AAC.01154-08
Ghannoum, M., Long, L., Larkin, E. L., Isham, N., Sherif, R., Borroto-Esoda, K., et al. (2018). Evaluation of the antifungal activity of the novel Oral Glucan synthase inhibitor SCY-078, singly and in combination, for the treatment of invasive aspergillosis. Antimicrob. Agents Chemother. 62, e00244–e00218. doi: 10.1128/AAC.00244-18.
Goldberg, J., Connolly, P., Schnizlein-Bick, C., Durkin, M., Kohler, S., Smedema, M., et al. (2000). Comparison of Nikkomycin Z with amphotericin B and itraconazole for treatment of histoplasmosis in a murine model. Antimicrob. Agents Chemother. 44, 1624–1629. doi: 10.1128/AAC.44.6.1624-1629.2000
Gow, N. A. R., Latge, J.-P., and Munro, C. A. (2017). The fungal cell wall: structure, biosynthesis, and function. Microbiol. Spectrum 5. doi: 10.1128/microbiolspec.FUNK-0035-2016
Hadrich, I., Makni, F., Neji, S., Cheikhrouhou, F., Bellaaj, H., Elloumi, M., et al. (2012). Amphotericin B in vitro resistance is associated with fatal Aspergillus flavus infection. Med. Mycol. 50, 829–834. doi: 10.3109/13693786.2012.684154
Hage, C. A., Connolly, P., Horan, D., Durkin, M., Smedema, M., Zarnowski, R., et al. (2011). Investigation of the efficacy of micafungin in the treatment of histoplasmosis using two north American strains of Histoplasma capsulatum. Antimicrob. Agents Chemother. 55, 4447–4450. doi: 10.1128/AAC.01681-10
Hager, C. L., Larkin, E. L., Long, L. A., and Ghannoum, M. A. (2018a). Evaluation of the efficacy of rezafungin, a novel echinocandin, in the treatment of disseminated Candida auris infection using an immunocompromised mouse model. J. Antimicrob. Chemother. 73, 2085–2088. doi: 10.1093/jac/dky153
Hager, C. L., Larkin, E. L., Long, L., Zohra Abidi, F., Shaw, K. J., and Ghannoum, M. A. (2018b). In vitro and in vivo evaluation of the antifungal activity of APX001A/APX001 against Candida auris. Antimicrob. Agents Chemother. 62, pii: e02319-17. doi: 10.1128/AAC.02319-17
Hasim, S., and Coleman, J. J. (2019). Targeting the fungal cell wall: current therapies and implications for development of alternative antifungal agents. Future Med. Chem. 11, 869–883. doi: 10.4155/fmc-2018-0465
Hata, K., Horii, T., Miyazaki, M., Watanabe, N.-A., Okubo, M., Sonoda, J., et al. (2011). Efficacy of oral E1210, a new broad-spectrum antifungal with a novel mechanism of action, in murine models of candidiasis, aspergillosis, and fusariosis. Antimicrob. Agents Chemother. 55, 4543–4551. doi: 10.1128/AAC.00366-11
Hector, R. F. (1993). Compounds active against cell walls of medically important fungi. Clin. Microbiol. Rev. 6, 1–21. doi: 10.1128/CMR.6.1.1
Hector, R. F., Zimmer, B. L., and Pappagianis, D. (1990). Evaluation of nikkomycins X and Z in murine models of coccidioidomycosis, histoplasmosis, and blastomycosis. Antimicrob. Agents Chemother. 34, 587–593. doi: 10.1128/AAC.34.4.587
Hobson, R. P., Munro, C. A., Bates, S., MacCallum, D. M., Cutler, J. E., Heinsbroek, S. E. M., et al. (2004). Loss of cell wall mannosylphosphate in Candida albicans does not influence macrophage recognition. J. Biol. Chem. 279, 39628–39635. doi: 10.1074/jbc.M405003200
Ikezawa, H. (2002). Glycosylphosphatidylinositol (GPI)-anchored proteins. Biol. Pharm. Bull. 25, 409–417. doi: 10.1248/bpb.25.409
Jiménez-Ortigosa, C., Perez, W. B., Angulo, D., Borroto-Esoda, K., and Perlin, D. S. (2017). De novo Acquisition of Resistance to SCY-078 in Candida glabrata involves FKS mutations that both overlap and are distinct from those conferring echinocandin resistance. Antimicrob. Agents Chemother. 61, e00833–e00817. doi: 10.1128/AAC.00833-17
Johnson, M. D., and Perfect, J. R. (2003). Caspofungin: first approved agent in a new class of antifungals. Expert. Opin. Pharmacother. 4, 807–823. doi: 10.1517/14656566.4.5.807
Kofla, G., and Ruhnke, M. (2011). Pharmacology and metabolism of anidulafungin, caspofungin and micafungin in the treatment of invasive candidosis - review of the literature. Eur. J. Med. Res. 16, 159–166. doi: 10.1186/2047-783X-16-4-159
Koss, T., Bagheri, B., Zeana, C., Romagnoli, M. F., and Grossman, M. E. (2002). Amphotericin B-resistant Aspergillus flavus infection successfully treated with caspofungin, a novel antifungal agent. J. Am. Acad. Dermatol. 46, 945–947. doi: 10.1067/mjd.2002.120627
Lagorce, A., Hauser, N. C., Labourdette, D., Rodriguez, C., Martin-Yken, H., Arroyo, J., et al. (2003). Genome-wide analysis of the response to cell wall mutations in the yeast Saccharomyces cerevisiae. J. Biol. Chem. 278, 20345–20357. doi: 10.1074/jbc.M211604200
Lamoth, F., and Alexander, B. D. (2015). Antifungal activities of SCY-078 (MK-3118) and standard antifungal agents against clinical non-Aspergillus mold isolates. Antimicrob. Agents Chemother. 59, 4308–4311. doi: 10.1128/AAC.00234-15
Lamoth, F., Juvvadi, P. R., Gehrke, C., Asfaw, Y. G., and Steinbach, W. J. (2014). Transcriptional activation of heat shock protein 90 mediated via a proximal promoter region as trigger of caspofungin resistance in Aspergillus fumigatus. J. Infect. Dis. 209, 473–481. doi: 10.1093/infdis/jit530
Larkin, E., Hager, C., Chandra, J., Mukherjee, P. K., Retuerto, M., Salem, I., et al. (2017). The emerging pathogen Candida auris: growth phenotype, virulence factors, activity of antifungals, and effect of SCY-078, a novel glucan synthesis inhibitor, on growth morphology and biofilm formation. Antimicrob. Agents Chemother. 61, pii: e02396-16. doi: 10.1128/AAC.02396-16
Latgé, J.-P. (2007). The cell wall: a carbohydrate Armour for the fungal cell. Mol. Microbiol. 66, 279–290. doi: 10.1111/j.1365-2958.2007.05872.x
Lepak, A. J., Marchillo, K., and Andes, D. R. (2015). Pharmacodynamic target evaluation of a novel oral glucan synthase inhibitor, SCY-078 (MK-3118), using an in vivo murine invasive candidiasis model. Antimicrob. Agents Chemother. 59, 1265–1272. doi: 10.1128/AAC.04445-14
Lepak, A. J., Zhao, M., VanScoy, B., Ambrose, P. G., and Andes, D. R. (2018). Pharmacodynamics of a long-acting echinocandin, CD101, in a Neutropenic invasive-candidiasis murine model using an extended-interval dosing design. Antimicrob. Agents Chemother. 62, pii: e01572-18. doi: 10.1128/AAC.01572-18
Li, R. K., and Rinaldi, M. G. (1999). In vitro antifungal activity of nikkomycin Z in combination with fluconazole or itraconazole. Antimicrob. Agents Chemother. 43, 1401–1405. doi: 10.1128/AAC.43.6.1401
Marcos-Zambrano, L. J., Escribano, P., Sánchez-Carrillo, C., Bouza, E., and Guinea, J. (2017a). Frequency of the paradoxical effect measured using the EUCAST procedure with Micafungin, Anidulafungin, and Caspofungin against Candida species isolates causing Candidemia. Antimicrob. Agents Chemother. 61, pii: e01584-16. doi: 10.1128/AAC.01584-16
Marcos-Zambrano, L. J., Gómez-Perosanz, M., Escribano, P., Bouza, E., and Guinea, J. (2017b). The novel oral glucan synthase inhibitor SCY-078 shows in vitro activity against sessile and planktonic Candida spp. J. Antimicrob. Chemother. 72, 1969–1976. doi: 10.1093/jac/dkx010
Mazur, P., and Baginsky, W. (1996). In vitro activity of 1,3-β-D-Glucan synthase requires the GTP-binding protein Rho1. J. Biol. Chem. 271, 14604–14609. doi: 10.1074/jbc.271.24.14604
McLellan, C. A., Whitesell, L., King, O. D., Lancaster, A. K., Mazitschek, R., and Lindquist, S. (2012). Inhibiting GPI anchor biosynthesis in fungi stresses the endoplasmic reticulum and enhances immunogenicity. ACS Chem. Biol. 7, 1520–1528. doi: 10.1021/cb300235m
Mesa-Arango, A. C., Rueda, C., Román, E., Quintin, J., Terrón, M. C., Luque, D., et al. (2016). Cell Wall changes in amphotericin B-resistant strains from Candida tropicalis and relationship with the immune responses elicited by the host. Antimicrob. Agents Chemother. 60, 2326–2335. doi: 10.1128/AAC.02681-15
Mesa-Arango, A. C., Trevijano-Contador, N., Román, E., Sánchez-Fresneda, R., Casas, C., Herrero, E., et al. (2014). The production of reactive oxygen species is a universal action mechanism of amphotericin B against pathogenic yeasts and contributes to the fungicidal effect of this drug. Antimicrob. Agents Chemother. 58, 6627–6638. doi: 10.1128/AAC.03570-14
Miyazaki, M., Horii, T., Hata, K., Watanabe, N.-A., Nakamoto, K., Tanaka, K., et al. (2011). In vitro activity of E1210, a novel antifungal, against clinically important yeasts and molds. Antimicrob. Agents Chemother. 55, 4652–4658. doi: 10.1128/AAC.00291-11
Mora-Duarte, J., Betts, R., Rotstein, C., Colombo, A. L., Thompson-Moya, L., Smietana, J., et al. (2002). Comparison of caspofungin and amphotericin B for invasive candidiasis. N. Engl. J. Med. 347, 2020–2029. doi: 10.1056/NEJMoa021585
Munro, C. A. (2013). Chitin and glucan, the yin and yang of the fungal cell wall, implications for antifungal drug discovery and therapy. Adv. Appl. Microbiol. 83, 145–172. doi: 10.1016/B978-0-12-407678-5.00004-0
Mutz, M., and Roemer, T. (2016). The GPI anchor pathway: a promising antifungal target? Future Med. Chem. 8, 1387–1391. doi: 10.4155/fmc-2016-0110
Nakai, T., Uno, J., Ikeda, F., Tawara, S., Nishimura, K., and Miyaji, M. (2003). In vitro antifungal activity of Micafungin (FK463) against dimorphic fungi: comparison of yeast-like and mycelial forms. Antimicrob. Agents Chemother. 47, 1376–1381. doi: 10.1128/AAC.47.4.1376-1381.2003
Nett, J., Lincoln, L., Marchillo, K., Massey, R., Holoyda, K., Hoff, B., et al. (2007). Putative role of beta-1,3 glucans in Candida albicans biofilm resistance. Antimicrob. Agents Chemother. 51, 510–520. doi: 10.1128/AAC.01056-06
Nyfeler, R., and Keller-Schierlein, W. (1974). Metabolites of microorganisms. 143. Echinocandin B, a novel polypeptide-antibiotic from Aspergillus nidulans var. echinulatus: isolation and structural components. Helv. Chim. Acta 57, 2459–2477. doi: 10.1002/hlca.19740570818
O’Meara, T. R., Robbins, N., and Cowen, L. E. (2017). The Hsp90 chaperone network modulates Candida virulence traits. Trends Microbiol. 25, 809–819. doi: 10.1016/j.tim.2017.05.003
Pappas, P. G., Kauffman, C. A., Andes, D. R., Clancy, C. J., Marr, K. A., Ostrosky-Zeichner, L., et al. (2016). Clinical practice guideline for the management of Candidiasis: 2016 update by the infectious diseases society of America. Clin. Infect. Dis. 62, e1–e50. doi: 10.1093/cid/civ933
Patil, A., and Majumdar, S. (2017). Echinocandins in antifungal pharmacotherapy. J. Pharm. Pharmacol. 69, 1635–1660. doi: 10.1111/jphp.12780
Perlin, D. S. (2015). Echinocandin resistance in Candida. Clin. Infect. Dis. 61(Suppl 6), S612–S617. doi: 10.1093/cid/civ791
Pfaller, M. A., Huband, M. D., Flamm, R. K., Bien, P. A., and Castanheira, M. (2019). In vitro activity of APX001A (Manogepix) and comparator agents against 1,706 fungal isolates collected during an international surveillance program (2017). Antimicrob. Agents Chemother. 63, pii: e00840-19. doi: 10.1128/AAC.00840-19
Pfaller, M. A., Messer, S. A., Motyl, M. R., Jones, R. N., and Castanheira, M. (2013). In vitro activity of a new oral glucan synthase inhibitor (MK-3118) tested against Aspergillus spp. by CLSI and EUCAST broth microdilution methods. Antimicrob. Agents Chemother. 57, 1065–1068. doi: 10.1128/AAC.01588-12
Pfaller, M. A., Messer, S. A., Rhomberg, P. R., Borroto-Esoda, K., and Castanheira, M. (2017). Differential activity of the oral glucan synthase inhibitor SCY-078 against wild-type and echinocandin-resistant strains of Candida species. Antimicrob. Agents Chemother. 61, e00161–e00117. doi: 10.1128/AAC.00161-17
Qadota, H., Python, C. P., Inoue, S. B., Arisawa, M., Anraku, Y., Zheng, Y., et al. (1996). Identification of yeast Rho1p GTPase as a regulatory subunit of 1,3-β-Glucan synthase. Science 272, 279–281. doi: 10.1126/science.272.5259.279
Roy, R. M., and Klein, B. S. (2012). Dendritic cells in anti-fungal immunity and vaccine design. Cell Host Microbe 11, 436–446. doi: 10.1016/j.chom.2012.04.005
Rueda, C., Cuenca-Estrella, M., and Zaragoza, O. (2014). Paradoxical growth of Candida albicans in the presence of caspofungin is associated with multiple cell wall rearrangements and decreased virulence. Antimicrob. Agents Chemother. 58, 1071–1083. doi: 10.1128/AAC.00946-13
Rüping, M. J., Vehreschild, J. J., Farowski, F., and Cornely, O. A. (2008). Anidulafungin: advantage for the newcomer? Expert. Rev. Clin. Pharmacol. 1, 207–216. doi: 10.1586/17512433.1.2.207
Sandison, T., Ong, V., Lee, J., and Thye, D. (2017). Safety and pharmacokinetics of CD101 IV, a novel echinocandin, in healthy adults. Antimicrob. Agents Chemother. 61, e01627–e01616. doi: 10.1128/AAC.01627-16
Satish, S., Jiménez-Ortigosa, C., Zhao, Y., Lee, M. H., Dolgov, E., Krüger, T., et al. (2019). Stress-induced changes in the lipid microenvironment of β-(1,3)-D-glucan synthase cause clinically important echinocandin resistance in Aspergillus fumigatus. mBio 10:e00779-19. doi: 10.1128/mBio.00779-19
Schimoler-O’Rourke, R., Renault, S., Mo, W., and Selitrennikoff, C. P. (2003). Neurospora crassa FKS protein binds to the (1,3)beta-glucan synthase substrate, UDP-glucose. Curr. Microbiol. 46, 408–412. doi: 10.1007/s00284-002-3884-5
Scorneaux, B., Angulo, D., Borroto-Esoda, K., Ghannoum, M., Peel, M., and Wring, S. (2017). SCY-078 is fungicidal against Candida species in time-kill studies. Antimicrob. Agents Chemother. 61, pii: e01961-16. doi: 10.1128/AAC.01961-16
Seo, K., Akiyoshi, H., and Ohnishi, Y. (1999). Alteration of cell wall composition leads to amphotericin B resistance in Aspergillus flavus. Microbiol. Immunol. 43, 1017–1025. doi: 10.1111/j.1348-0421.1999.tb01231.x
Shaw, K. J., Schell, W. A., Covel, J., Duboc, G., Giamberardino, C., Kapoor, M., et al. (2018). In vitroandin vivoevaluation of APX001A/APX001 and otherGwt1 inhibitors against Cryptococcus. Antimicrob. Agents Chemother. 62:e00523-18. doi: 10.1128/AAC.00523-18
Shibata, N., Ikuta, K., Imai, T., Satoh, Y., Satoh, R., Suzuki, A., et al. (1995). Existence of branched side chains in the cell wall mannan of pathogenic yeast, Candida albicans. Structure-antigenicity relationship between the cell wall mannans of Candida albicans and Candida parapsilosis. J. Biol. Chem. 270, 1113–1122. doi: 10.1074/jbc.270.3.1113
Singh, S. D., Robbins, N., Zaas, A. K., Schell, W. A., Perfect, J. R., and Cowen, L. E. (2009). Hsp90 governs echinocandin resistance in the pathogenic yeast Candida albicans via Calcineurin. PLoS Pathog. 5:e1000532. doi: 10.1371/journal.ppat.1000532
Singh-Babak, S. D., Babak, T., Diezmann, S., Hill, J. A., Xie, J. L., Chen, Y.-L., et al. (2012). Global analysis of the evolution and mechanism of echinocandin resistance in Candida glabrata. PLoS Pathog. 8:e1002718. doi: 10.1371/journal.ppat.1002718
Sofjan, A. K., Mitchell, A., Shah, D. N., Nguyen, T., Sim, M., Trojcak, A., et al. (2018). Rezafungin (CD101), a next-generation echinocandin: a systematic literature review and assessment of possible place in therapy. J. Glob. Antimicrob. Resist. 14, 58–64. doi: 10.1016/j.jgar.2018.02.013
Stevens, D. A. (2000). Drug interaction studies of a Glucan synthase inhibitor (LY 303366) and a chitin synthase inhibitor (Nikkomycin Z) for inhibition and killing of fungal pathogens. Antimicrob. Agents Chemother. 44, 2547–2548. doi: 10.1128/AAC.44.9.2547-2548.2000
Thompson, G. R., Barker, B. M., and Wiederhold, N. P. (2017). Large-scale evaluation of in vitro amphotericin B, Triazole, and echinocandin activity against Coccidioides species from US. Institutions. Antimicrob. Agents Chemother. 61, e02634–e02616. doi: 10.1128/AAC.02634-16
Tsukahara, K., Hata, K., Nakamoto, K., Sagane, K., Watanabe, N.-A., Kuromitsu, J., et al. (2003). Medicinal genetics approach towards identifying the molecular target of a novel inhibitor of fungal cell wall assembly. Mol. Microbiol. 48, 1029–1042. doi: 10.1046/j.1365-2958.2003.03481.x
Viriyakosol, S., Kapoor, M., Okamoto, S., Covel, J., Soltow, Q. A., Trzoss, M., et al. (2019). APX001 and other Gwt1 inhibitor Prodrugs are effective in experimental Coccidioides immitis pneumonia. Antimicrob. Agents Chemother. 63, pii: e01715-18. doi: 10.1128/AAC.01715-18
Walker, L. A., Gow, N. A. R., and Munro, C. A. (2010). Fungal echinocandin resistance. Fungal Genet. Biol. 47, 117–126. doi: 10.1016/j.fgb.2009.09.003
Walker, L. A., Gow, N. A. R., and Munro, C. A. (2013). Elevated chitin content reduces the susceptibility of Candida species to caspofungin. Antimicrob. Agents Chemother. 57, 146–154. doi: 10.1128/AAC.01486-12
Walker, L. A., Munro, C. A., de Bruijn, I., Lenardon, M. D., McKinnon, A., and Gow, N. A. R. (2008). Stimulation of chitin synthesis rescues Candida albicans from echinocandins. PLoS Pathog. 4:e1000040. doi: 10.1371/journal.ppat.1000040
Walker, S. S., Xu, Y., Triantafyllou, I., Waldman, M. F., Mendrick, C., Brown, N., et al. (2011). Discovery of a novel class of orally active antifungal β-1,3-d-Glucan synthase inhibitors. Antimicrob. Agents Chemother. 55, 5099–5106. doi: 10.1128/AAC.00432-11
Watanabe, N.-A., Miyazaki, M., Horii, T., Sagane, K., Tsukahara, K., and Hata, K. (2012). E1210, a new broad-spectrum antifungal, suppresses Candida albicans hyphal growth through inhibition of glycosylphosphatidylinositol biosynthesis. Antimicrob. Agents Chemother. 56, 960–971. doi: 10.1128/AAC.00731-11
Wiederhold, N. P., and Lewis, R. E. (2003). The echinocandin antifungals: an overview of the pharmacology, spectrum and clinical efficacy. Expert Opin. Investig. Drugs 12, 1313–1333. doi: 10.1517/13543784.12.8.1313
Wiederhold, N. P., Locke, J. B., Daruwala, P., and Bartizal, K. (2018). Rezafungin (CD101) demonstrates potent in vitro activity against Aspergillus, including azole-resistant Aspergillus fumigatus isolates and cryptic species. J. Antimicrob. Chemother. 73, 3063–3067. doi: 10.1093/jac/dky280
Wiederhold, N. P., Najvar, L. K., Shaw, K. J., Jaramillo, R., Patterson, H., Olivo, M., et al. (2019). Efficacy of delayed therapy with Fosmanogepix (APX001) in a murine model of Candida auris invasive candidiasis. Antimicrob. Agents Chemother. 63:e01120-19. doi: 10.1128/AAC.01120-19
Wring, S. A., Randolph, R., Park, S., Abruzzo, G., Chen, Q., Flattery, A., et al. (2017). Preclinical pharmacokinetics and Pharmacodynamic target of SCY-078, a first-in-class orally active antifungal Glucan synthesis inhibitor, in murine models of disseminated candidiasis. Antimicrob. Agents Chemother. 61:e02068-16. doi: 10.1128/AAC.02068-16
Yadav, U., and Khan, M. A. (2018). Targeting the GPI biosynthetic pathway. Pathog. Global Health 112, 115–122. doi: 10.1080/20477724.2018.1442764
Yang, F., Zhang, L., Wakabayashi, H., Myers, J., Jiang, Y., Cao, Y., et al. (2017). Tolerance to Caspofungin in Candida albicans is associated with at least three distinctive mechanisms that govern expression of FKS genes and Cell Wall remodeling. Antimicrob. Agents Chemother. 61, e00071–e00017. doi: 10.1128/AAC.00071-17
Zhao, Y., Lee, M. H., Paderu, P., Lee, A., Jimenez-Ortigosa, C., Park, S., et al. (2018). Significantly improved pharmacokinetics enhances in vivo efficacy of APX001 against echinocandin- and multidrug-resistant Candida isolates in a mouse model of invasive candidiasis. Antimicrob. Agents Chemother. 62, pii: e00425-18. doi: 10.1128/AAC.00425-18
Keywords: fungal cell wall, antifungals, therapy, resistance, 1,3-β-D-Glucan Synthase Inhibitors, ibrexafungerp, manogepix
Citation: Lima SL, Colombo AL and de Almeida Junior JN (2019) Fungal Cell Wall: Emerging Antifungals and Drug Resistance. Front. Microbiol. 10:2573. doi: 10.3389/fmicb.2019.02573
Edited by:
Joshua D. Nosanchuk, Albert Einstein College of Medicine, United StatesReviewed by:
Jeniel E. Nett, University of Wisconsin-Madison, United StatesEmma Camacho, Johns Hopkins University, United States
Copyright © 2019 Lima, Colombo and de Almeida Junior. This is an open-access article distributed under the terms of the Creative Commons Attribution License (CC BY). The use, distribution or reproduction in other forums is permitted, provided the original author(s) and the copyright owner(s) are credited and that the original publication in this journal is cited, in accordance with accepted academic practice. No use, distribution or reproduction is permitted which does not comply with these terms.
*Correspondence: João N. de Almeida Junior, am5hajk5QGdtYWlsLmNvbQ==