- 1Key Laboratory for Water Quality and Conservation of the Pearl River Delta, Ministry of Education, School of Environmental Science and Engineering, Guangzhou University, Guangzhou, China
- 2Key Laboratory of Biometallurgy of Ministry of Education, School of Minerals Processing and Bioengineering, Central South University, Changsha, China
- 3School of Environmental Science and Engineering, Guangdong University of Technology, Guangzhou, China
- 4Federal Institute for Geosciences and Natural Resources (BGR), Hanover, Germany
- 5College of Environmental Science and Engineering, Donghua University, Shanghai, China
- 6Biofilm Centre, Aquatische Biotechnologie, Universität Duisburg-Essen, Essen, Germany
Sulfobacillus and Leptospirillum occur frequently in leaching systems. Here we investigated the effects of cells of L. ferriphilum on biofilm formation and leaching performance by S. thermosulfidooxidans. The effects were caused by the presence of L. ferriphilum or an addition of pyrite leach liquor from L. ferriphilum. Data show that the number of attached S. thermosulfidooxidans on pyrite increases, if the pyrite had been pre-colonized by living biofilms of L. ferriphilum, while it decreases if the pre-colonized biofilms had been inactivated. Coaggregation between S. thermosulfidooxidans and L. ferriphilum occurs during the dual-species biofilm formation, but different effects on bioleaching were noted, if the preculture of L. ferriphilum had been different. If L. ferriphilum had been pre-colonized on a pyrite, significantly negative effect was shown. However, if the two species were simultaneously inoculated into a sterile leaching system, the bioleaching efficiency was better than that of a pure culture of S. thermosulfidooxidans. The effect might be related to a metabolic preference of S. thermosulfidooxidans. If S. thermosulfidooxidans performed leaching in a filtered pyrite leachate from L. ferriphilum, the cells preferred to oxidize RISCs instead of ferrous ion and the number of attached cells decreased compared with the control. This study gives an indication that in a short-term multi-species leaching system the role of S. thermosufidooxidans may be related to the time of its introduction.
Introduction
Microorganisms catalyze metal ion leaching from insoluble metal sulfides, such as pyrite (FeS2), arsenopyrite (FeAsS), chalcopyrite (CuFeS2), sphalerite (ZnS) or marcasite (FeS2) by biological oxidation via contact and non-contact modes. Non-contact leaching is mainly exerted by planktonic bacteria, while contact leaching requires bacteria attached on the mineral surface. In both cases ferric ion is the most important oxidizing agent. As a consequence of the attack on metal sulfides ferrous ions and reduced inorganic sulfur compounds (RISCs) are produced. Ferrous ion is then re-oxidized to ferric ion by the iron-oxidizing microorganisms, and RISCs are oxidized to sulfate by sulfur-oxidizing bacteria. As a result, minerals get dissolved (Sand et al., 2001). This process is called bioleaching or biomining. In this way it is applied industrially to win metals from low-grade metal sulfides because of low cost and environmentally less harmful than the conventional mineral processing. However, if this process happens in a natural environment, then it may cause serious problems known as acid mine drainage (AMD) with high metal concentrations and extremely low pH (Hoffert, 1947).
Molecular analysis in the leachate of industrially or naturally leaching systems shows that microbial consortia of multiple species occur and the genus Sulfobacillus and Leptospirillum are detected frequently (Okibe et al., 2003; Johnson et al., 2008; Acosta et al., 2014). The genus Sulfobacillus was described by Golovacheva and Karavaiko in 1978 (Golovacheva and Karavaĭko, 1978). Sulfobacilli are Gram-positive, generally non-motile, rod shaped, endospore-forming and moderately thermophilic acidophilic bacteria. The temperature range for their growth is 17–60°C, with an optimum around 40–55°C. The pH range for their growth is 1.1–5.5, with an optimum between 1.7 and 2.4 (Bogdanova et al., 2006). They can oxidize ferrous ion, reduced RISCs such as tetrathionate, thiosulfate and elemental sulfur, as well as sulfide minerals in the presence of 0.02% yeast extract. They always are members of microbial leaching communities because of their versatile metabolism and also because of their high tolerance to low pH and high metal concentrations (Rawlings and Johnson, 2007). Decreased amounts of sulfobacilli even result in reduced bioleaching efficiency for chalcopyrite (Spolaore et al., 2011).
Markosyan isolated Leptospirillum in 1972 and described it as a mesophilic, vibrioid-shaped and iron-oxidizing bacterium (Markosyan, 1972). Leptospirilli are Gram-negative bacteria with a lot of remarkable properties, which allow them to thrive in leaching habitats. Compared with acidithiobacilli, leptospirilli can resist 10 times higher ferric ion concentrations than acidithiobacilli. Their optimum growth temperature and pH is 22–35°C and 1.5–2.3, respectively (Sand et al., 1992). Leptospirilli attach to metal sulfides fast and firmly (Sand et al., 1992; Zhu et al., 2012) and are pivotal for biofilm formation in mixed cultures. They are pre-colonizers helping other microorganisms attach to substrata (Noël et al., 2010).
The understanding of interactions at the strain and at the species or genus level in bioleaching communities under such extreme conditions is still in its infancy but would be very helpful to enhance bioleaching or to control AMD. Okibe et al. (Okibe and Johnson, 2004) investigated the interactions between moderately thermophilic prokaryotes during bioleaching of pyrite. They assume interactions between these acidophiles, because they found that different combinations of these acidophiles had either positive or negative effects on the dissolution of pyrite. Castro et al. (Castro et al., 2016) showed that Sulfolobus metallicus and Acidianus copahuensis negatively influenced each other during initial attachment and pyrite dissolution, and physical contact between the two species was detected. Maulani et al. (2016) found that pre-established biofilms of Leptospirillum ferriphilum inhibited the initial attachment to pyrite by cells of Ferroplasma acidiphilum and did not promote pyrite leaching by F. acidiphilum. In contrast, inactivated biofilm cells of S. thermosulfidooxidans enhanced pyrite bioleaching by F. acidiphilum. Bellenberg et al. (2014) reported that molecular cell-to-cell communication plays a role in regulating biofilm formation in leaching systems, and their data also indicate other still undefined interactions. Liu et al. (2016) investigated the influence of S. thermosulfidooxidans on the Archaeon Acidianus sp. DSM 29099 during leaching of pyrite. They described that leaching by Acidianus sp. DSM 29099 becomes enhanced with either cells or filtered leachates of S. thermosulfidooxidans. However, little work has been done to analyze the interactions between the genus Leptospirillum and Sulfobacillus, although both are often dominant in leaching operations.
In order to investigate such interactions in this study S. thermosulfidooxidans DSM9293T and L. ferriphilum DSM 14647T were chosen. Since S. thermosulfidooxidans DSM9293T is known to be a weak biofilm former (Li et al., 2016), we used L. ferriphilum, either as living cells or inactivated biofilms or filtered leachates to influence biofilm formation and pyrite leaching by S. thermosulfidooxidans. The results show that the number of attached S. thermosulfidooxidans on pyrite increased, if the pyrite was pre-colonized by living biofilms of L. ferriphilum, while it decreased if the biofilms had been inactivated previously. L. ferriphilum facilitates biofilm formation by S. thermosulfidooxidans, if dual-species biofilms can form on pyrite. Physical contact between S. thermosulfidooxidans and L. ferriphilum illustrates that coaggregations between the two species were formed, but with different consequences. If L. ferriphilum, either living or inactivated, had pre-colonized pyrite, the leaching performance of S. thermosulfidooxidans was worse than that of either single culture. However, when the two species were simultaneously inoculated to a sterile leaching system, the leaching performance was much better than before. When S. thermosulfidooxidans performed leaching in filtered leachate from L. ferriphilum, a high percentage of ferrous ion and low pH of the system illustrate it preferred to oxidize RISCs over ferrous ion, and numbers of attached cells decreased compared with the control. The results indicate that in a multi-species leaching system the role of S. thermosufidooxidans might be decided by the time when it is introduced: it is more like an iron oxidizer if added initially with other species, while it prefers to be a sulfur oxidizer if it is not introduced at the beginning of the bioleaching. Our study provides information for investigating species-species interactions in a bioleaching system, which is helpful for researchers to optimize a leaching consortium or to prevent bioleaching happening in natural environment.
Materials and Methods
Strains and Cultivation
Strains S. thermosulfidooxidans DSM 9293 and L. ferriphilum DSM 14647 were purchased from Deutsche Sammlung von Mikroorganismen und Zellkulturen (DSMZ), Germany. The two strains were cultivated in Mackintosh (MAC) medium (Mackintosh, 1978). 0.02% yeast extract was supplemented in the medium for S. thermosulfidooxidans. 2% (m/v) pyrite grains or 1% sulfur prills or sulfur slices were added as energy source for S. thermosulfidooxidans. 4 g/L ferrous ion was added as energy source for L. ferriphilum. The initial pH of the medium was adjusted to 2.5 when the energy source was sulfur, while the pH was adjusted to 1.8 when the energy source was pyrite or ferrous ion. Both strains were cultivated on a rotary shaker at 140 rpm. Culturing temperature for S. thermosulfidooxidans and L. ferriphilum was 45 and 37°C, respectively.
Preparation of Pyrite Grains, Pyrite Slices, Sulfur Prills and Sulfur Slices
All pyrite was museum grade in form of naturally crystalized cubes from Freiberg, Germany. Smooth and shiny pyrite cubes were used for the experiments. The cubes were cut by a diamond saw and slices with one shiny side and one rough side were obtained. Only the shiny side was used for biofilm formation. The remaining pyrite was ground and pyrite grains with a size of 50–100 μm were used for cultivation or bioleaching experiments. Pyrite grains and slices were cleaned and sterilized according to Schippers et al. (1996). Briefly, they were washed with 6 M boiling HCl for 30 min, then rinsed with deionized water till the pH was neutral. After washing with acetone for three times, they were dried at 80°C for 12 h and sterilized for 24 h at 120°C under a nitrogen atmosphere.
To produce sulfur prills, elemental sulfur powder was firstly molten in a glass beaker at 130°C and then poured into cold deionized water with an agitation of 250 rpm. Due to rapid cooling sulfur prills with a diameter of 2 mm were formed. To produce sulfur slices, molten sulfur was poured onto glass plates to obtain a sulfur layer after its solidification. Then sulfur slices with a size of 3 cm × 3 cm × 0.2 cm were obtained by manually cutting the sulfur layer. Both sulfur prills and slices were autoclaved at 110°C for 90 min.
Bioleaching of Pyrite by S. thermosulfidooxidans in the Presence of L. ferriphilum
Pyrite grains were first incubated at a pulp density of 2% (m/v) with 108 cells/mL of iron-grown L. ferriphilum in 50 mL MAC medium with a pH of 1.8 for 1 day on a rotary shaker at 37°C. Afterward, the culture supernatants were discarded and the pyrite residues were washed three times with fresh MAC medium. After washing the pyrite residues were processed as following: Resuspended the pyrite residues in 50 mL fresh medium; Resuspended the pyrite residues in 50 mL fresh medium which contained 0.02% yeast extracts and 108 cells/mL of sulfur-grown cells of S. thermosulfidooxidans; Incubated the pyrite residues at 100°C for 2 h to heat-inactivate the biofilms of L. ferriphilum. And after washing, the pyrite residues were resuspended in 50 mL fresh medium which contained 0.02% yeast extracts and 108 cells/mL of sulfur-grown cells of S. thermosulfidooxidans. Two more sets of experiment were set: (1) 5 × 107 cells/mL of sulfur-grown S. thermosulfidooxidans cells and 5 × 107 cells/mL of iron-grown L. ferriphilum cells were inoculated simultaneously to 2% (m/v) freshly ground sterile pyrite; (2) 108 cells/mL of S. thermosulfidooxidans were inoculated to 2% (m/v) sterile freshly ground pyrite. All the experiments were performed at 37°C on a rotary shaker at 140 rpm with an initial pH of 1.8. Each experiment was done in triplicate.
Adhesion Experiments
The pyrite grains with 1 day old living or inactivated biofilms of L. ferriphilum were prepared as described above. Then cell attachment of S. thermosulfidooxidans to the pyrite with living or heat-inactivated biofilms followed. Briefly, 108 cells/mL of S. thermosulfidooxidans were inoculated into flasks filled with 50 mL MAC medium, pH 1.8, and with freshly ground sterile pyrite or with living or inactivated biofilms of L. ferriphilum. Then they were cultivated at 37°C with shaking at 140 rpm. The number of planktonic cells was determined by direct counting within 3 h. The amount of attached cells was calculated by subtracting the planktonic cells from the initial cell number. All the experiments were performed in triplicate.
Bioleaching of Pyrite by S. thermosulfidooxidans With Leachate From L. ferriphilum
The culture supernatants of L. ferriphilum were collected after 3 or 14 days of incubation with pyrite. Then they were filtered through a filter with a pore size of 0.22 μm. Afterward, pyrite-grown cells of S. thermosulfidooxidans were harvested by centrifugation and after washing with fresh MAC medium they were inoculated into the filtered leachate with a final concentration of 108 cells/mL. 2% (m/v) freshly ground sterile pyrite were then added to all the flasks. Considering the importance of ferric ion for leaching, two batches of experiment were set as control, in which the same amount of iron as detected in the leachate was added to the fresh medium. One control got an inoculum of S. thermosulfidooxidans, named as biological control, and an assay without inoculum of S. thermosulfidooxidans functioned as chemical control. The added iron was ferric chloride. The experiments were performed on a rotary shaker at 140 rpm, 37°C. Data were collected from three parallel experiments.
Cell Number, pH and Iron Ion Determination
The planktonic cell density was determined by direct counting with a Thoma counting chamber (Assistant, Germany) under a light microscope (Leica DMLS, Wetzlar GmbH) in phase contrast mode with a magnification of 400 times.
To determine the attached cells on the pyrite grains, samples were stained with SYTO9 which combines with the attached cells and gives fluorescent signals. Then an epifluorescence microscope (Axio Imager A1m, Zeiss, Germany) was used to take images of the pyrite grains. Afterward, the images were analyzed by platform Fiji according to Schindelin et al. (2012) to obtain the number of attached cells. Fiji identifies and enumerates the objects in the stack with a user-defined threshold for voxel intensity value. For counting first the value of the voxel intensity threshold was set manually by visual inspection of the images (Fiji: Image > Adjust > Threshold). Some incomplete cells, caused by the adjustment of the threshold, were then filled by function of fill holes (Fiji: Process > Binary > Fill Holes). To distinguish conjunct cells the watershed function was applied (Fiji: Process > Binary > Watershed). Afterward, the counting process was performed (Fiji: Analyze > Analyze Particles). Each cell number was collected from 25 images.
The pH was determined with a digital pH meter (Model pH 537, WTW, inLab 422 Combination Semi-micro pH Electrode, Mettler Toledo).
Ferrous and total iron ions were quantified by the procedures described by Tamura (Tamura et al., 1974). The principle is that 1,10-Phenanthroline complexes ferrous ions. The complex gives a red color and can be measured spectrophotometrically at 492 nm. Subsequently, the total iron can be quantified by adding hydroxylamine, which reduces ferric to ferrous ions. The samples were measured in triplicate within microtiter plates with a UV-Vis spectrophotometer (TECAN, Infinite pro® 200).
AFM and Epifluorescence Microscopy (EFM) Instrumentation and Performance
A “BioMaterials Workstation” (JPK Instruments, Germany) composed of a NanoWizard II atomic force microscope (JPK Instruments) and an upright epifluorescence microscope (Axio Imager A1m, Zeiss, Germany) was applied to visualize the cells and the biofilms. A sample can be visualized at the same site between the two microscopes with 2 μm deviation (Mangold et al., 2008). In this study all samples were first stained by 6 μM SYTO9. The location was first chosen by EFM, then visualized by AFM. For AFM imaging, the contact mode was used. The applied setpoint was below 1 nN and the scan rate ranged from 0.1 to 0.5 Hz. A CSC38/NO AL (Mikromasch, Tallinn, Estonia) probe was used for imaging and cantilever B with the following parameters were chosen for scanning: length, 350 μm; width, 32.5 μm; thickness, 1.0 μm; resonance frequency, 10 kHz; shape, cone with a full cone angle of 40o; and force constant, 0.03 N/m. Before the experiments the cantilevers were immersed in Piranha solution for 10 min and then washed with sterile MilliQ water. All AFM operations were performed in MAC medium at pH 2.5 at room temperature. The sensitivity of the optical lever system and the cantilever spring constant were calibrated in liquid prior to each experiment. The spring constant for each cantilever was compatible with the manufacturer’s specifications.
Results and Discussion
Biofilm Formation by Pure Cultures
Development of biofilms on pyrite slices by a pure culture of S. thermosulfidooxidans or of L. ferriphilum within 14 days is shown in Figure 1 or Figure 2, respectively. Figure 1A clearly indicates that after 1 day incubation cells of S. thermosulfidooxidans had attached on the pyrite slices either in the form of single cells or flocs. However, the attached cells detached gradually and almost no cells could be observed after 16 days of incubation (Figure 1C). It has been reported that cells of S. thermosulfidooxidans are unable to form stable biofilms on pyrite, if a routine culturing procedure is used. The cells are non-motile, but they prefer to colonize the defect sites of mineral surfaces (Sand et al., 2001). Also the culturing environment deteriorates as the experiment proceeds (e.g., decrease of organic compounds and increase of metabolic end products), which might stimulate formation of endospore (Li et al., 2016). In the assays with L. ferriphilum after 1 day of incubation most cells had attached on the surface of the pyrite slices in the form of single cells (Figure 2A). The cells were observed to grow in colonies after 7 days of incubation (Figure 2B). More robust biofilms of L. ferriphilum were observed after 16 days of incubation (Figure 2C).
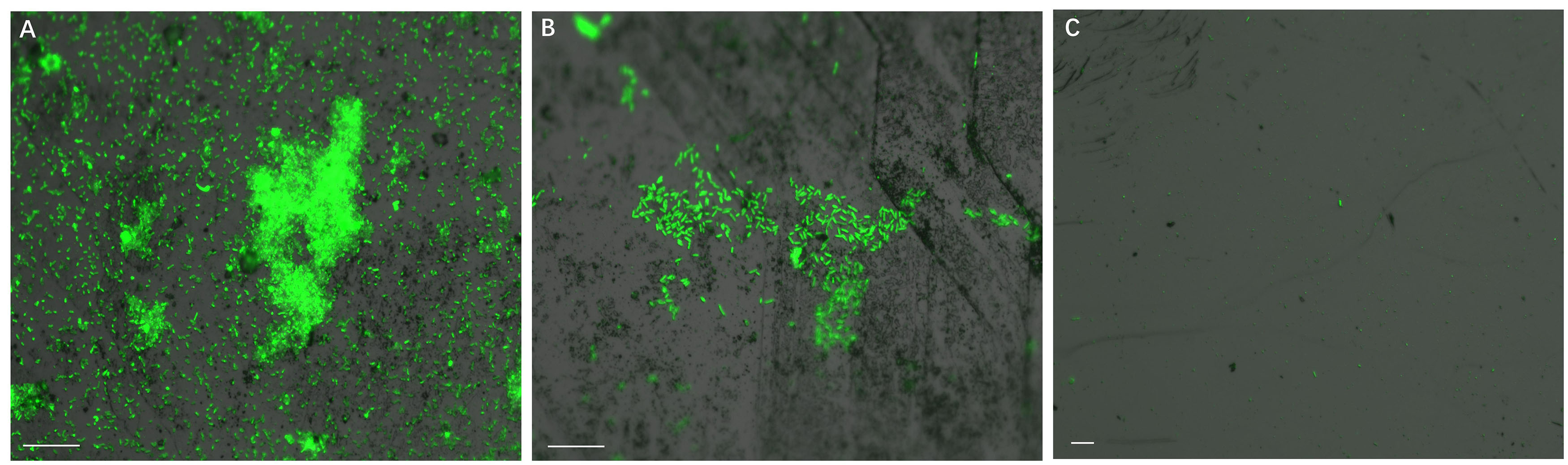
Figure 1. Biofilm formation on pyrite slices by a pure culture of S. thermosulfidooxidans under 37°C with an initial pH value of 1.8. Images (A–C) show 1, 7 and 16 days old biofilms of S. thermosulfidooxidans, respectively. Scale bar, 20 μm.
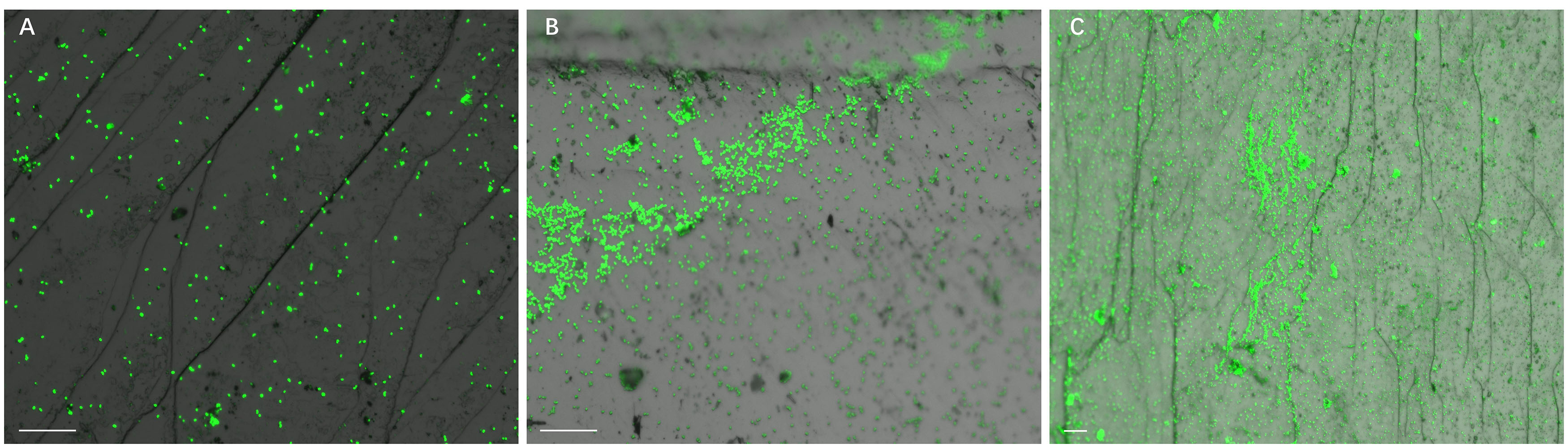
Figure 2. Biofilm formation on pyrite slices by a pure culture of L. ferriphilum under 37°C with an initial pH value of 1.8. Images (A–C) show 1, 7, and 16 days old biofilms of L. ferriphilum, respectively. Scale bar, 20 μm.
Adhesion of Cells of S. thermosulfidooxidans to Pyrite in the Presence of L. ferriphilum
Figure 3 demonstrates that the number of attached cells of S. thermosulfidooxidans on pyrite changes as a function of time. The pyrite was pre-colonized with 1 day old living or inactivated biofilms of L. ferriphilum. In the sterile control after 3 h about 27% of the inoculated cells of S. thermosulfidooxidans had attached on the pyrite. Compared with the sterile control, the percentage of attached cells of S. thermosulfidooxidans increased by around 5%, if the pyrite had been pre-colonized with living cells of L. ferriphilum. However, it decreased by approximately 5%, if the pyrite had been pre-colonized with inactivated cells of L. ferriphilum. It has been reported that bacterial adhesion is influenced by many factors, including surface roughness, type of mineral, hydrophobicity and medium (Dexter et al., 1975; Absolom et al., 1983; Helke et al., 1993; Quirynen et al., 1993; Blackman and Frank, 1996). It is likely that a conditioning film formed on the pyrite surface by excretions of the cells of L. ferriphilum like EPS compounds, which changed surface properties of the pyrite surfaces. This influenced the adhesion of cells of S. thermosulfidooxidans. However, in the assay with the inactivated biofilm of L. ferriphilum the conditioning film and the inactivated cells of L. ferriphilum might have been destroyed or deteriorated and blocked the attaching sites. Consequently, the attached number of cells of S. thermosulfidooxidans decreased.
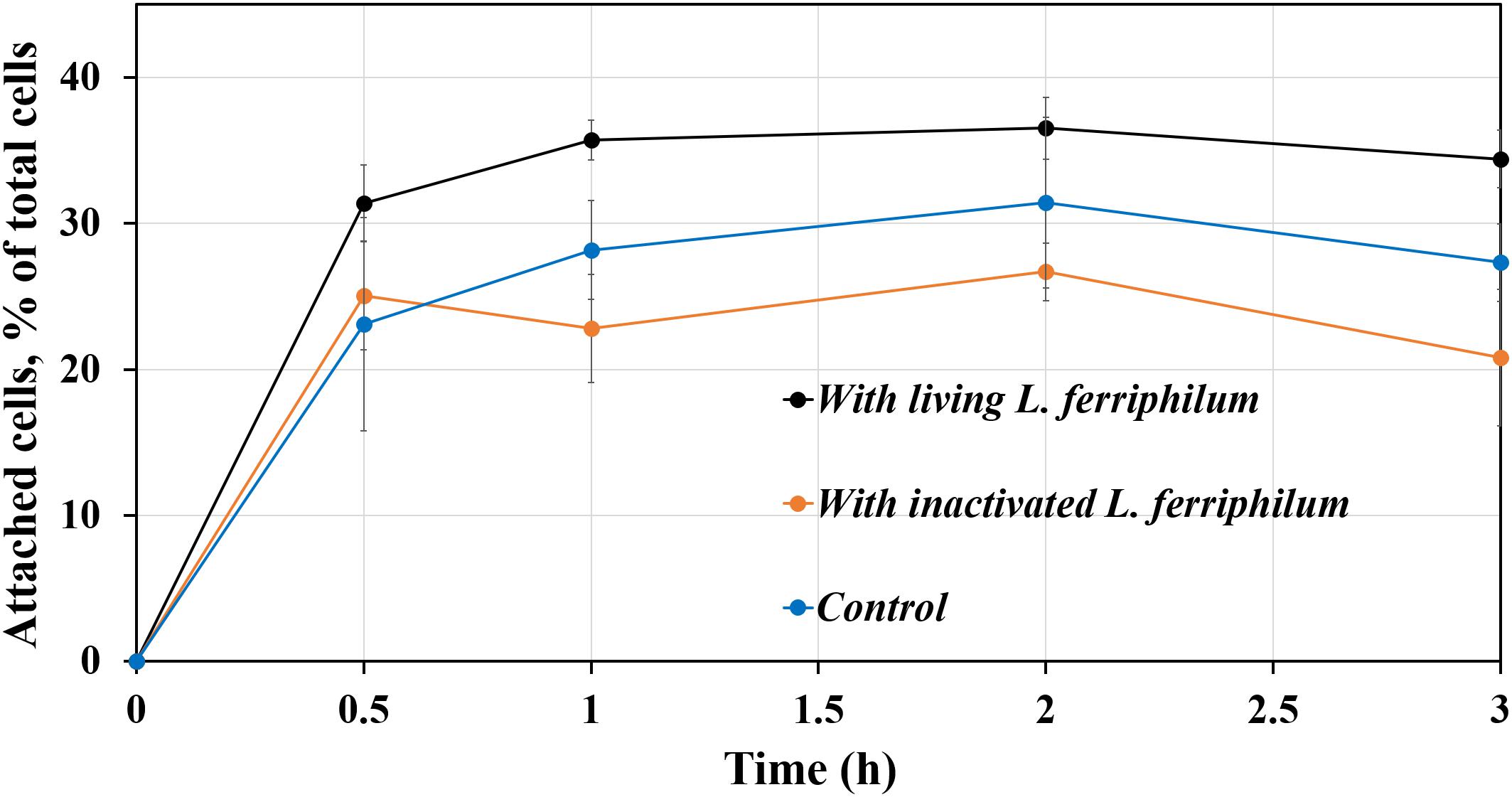
Figure 3. Adhesion of S. thermosulfidooxidans to pyrite pre-colonized by 1 day old biofilms of L. ferriphilum within 3 h under 37°C, pH 1.8.
Biofilm Formation of S. thermosulfidooxidans in the Presence of L. ferriphilum
Figure 4 illustrates biofilm formation on sterile pyrite by a binary culture of S. thermosulfidooxidans with L. ferriphilum within 16 days. The two species were simultaneously inoculated. Figures 5, 6 show the biofilm formation by the culture of S. thermosulfidooxidans on pyrite pre-colonized with living or inactivated biofilms of L. ferriphilum, respectively. It is visible that the cells of S. thermosulfidooxidans are long rod-shaped, while the cells of L. ferriphilum are vibrioid and/or round-shaped and small. The two cell forms can be distinguished well with the microscope. In all three assays after 16 days incubation the biofilms of S. thermosulfidooxidans remained on the pyrite. It indicates that S. thermosulfidooxidans can form biofilms on pyrite in the presence of L. ferriphilum. However, the architecture of the biofilm is different. Biofilms of S. thermosulfidooxidans in the form of single cells are observed on the pyrite pre-colonized with living biofilms of L. ferriphilum (Figure 5). In the other two assays biofilms of S. thermosulfidooxidans grow in the form of colonies with biofilms of L. ferriphilum (Figures 4, 6). In all assays a physical contact between the cells of S. thermosulfidooxidans and L. ferriphilum can be observed, as is visible in the images of EFM and AFM in Figure 7.
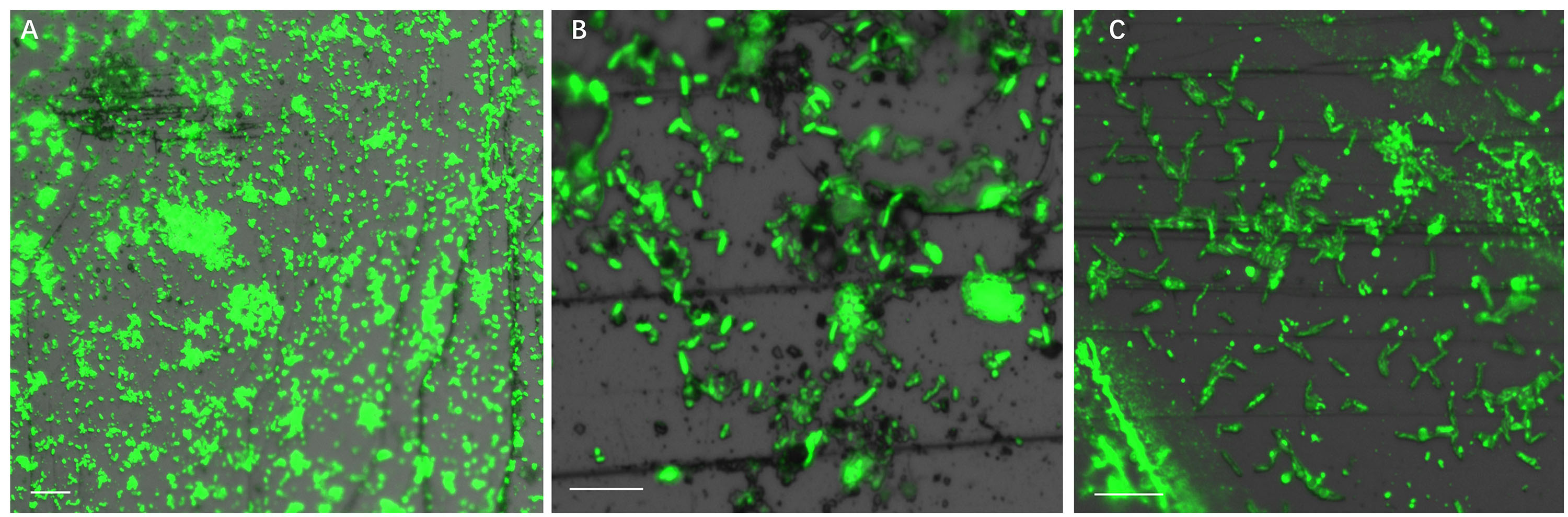
Figure 4. Biofilm formation by cells of S. thermosulfidooxidans and of L. ferriphilum on pyrite under 37°C with an initial pH value of 1.8. The two cells were simultaneously inoculated. Images (A–C) show 1, 7 and 16 days old binary biofilm of S. thermosulfidooxidans and L. ferriphilum, respectively. Scale bar, 10 μm.
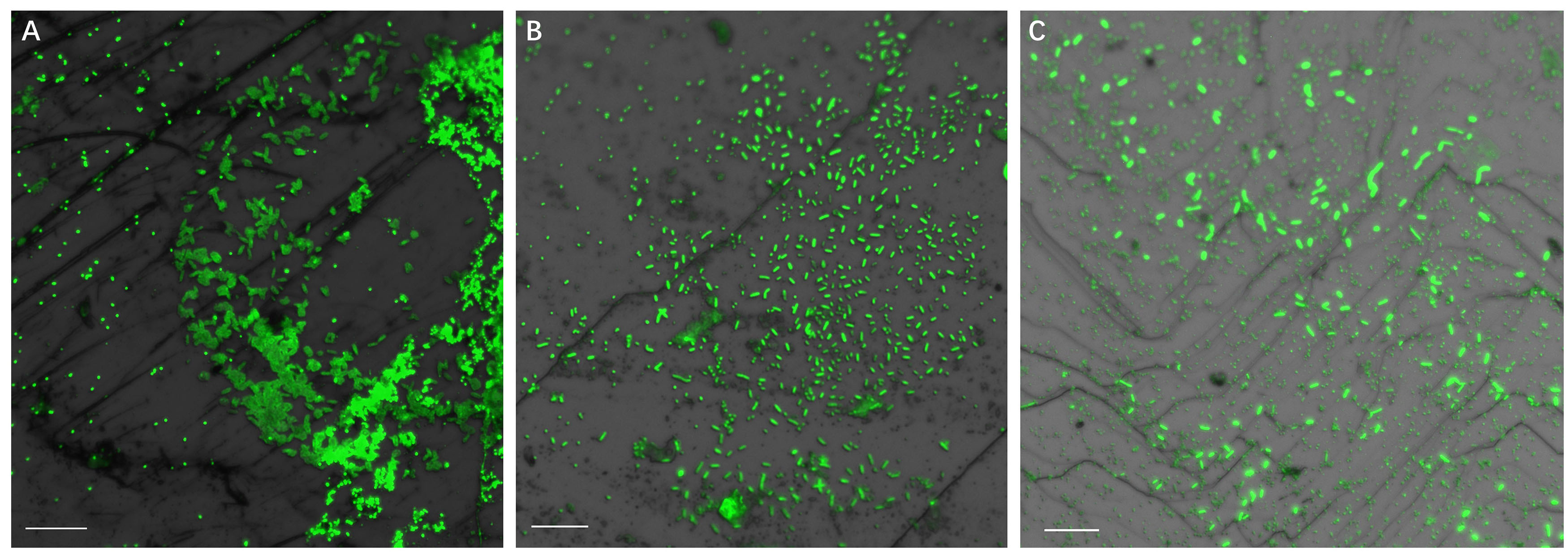
Figure 5. Biofilm formation by S. thermosulfidooxidans on pyrite pre-colonized with 1 day old living biofilms of L. ferriphilum under 37°C with an initial pH value of 1.8. Images (A–C) show the binary biofilm of S. thermosulfidooxidans and L. ferriphilum after 1, 7, and 16 days incubation. Scale bar, 10 μm.
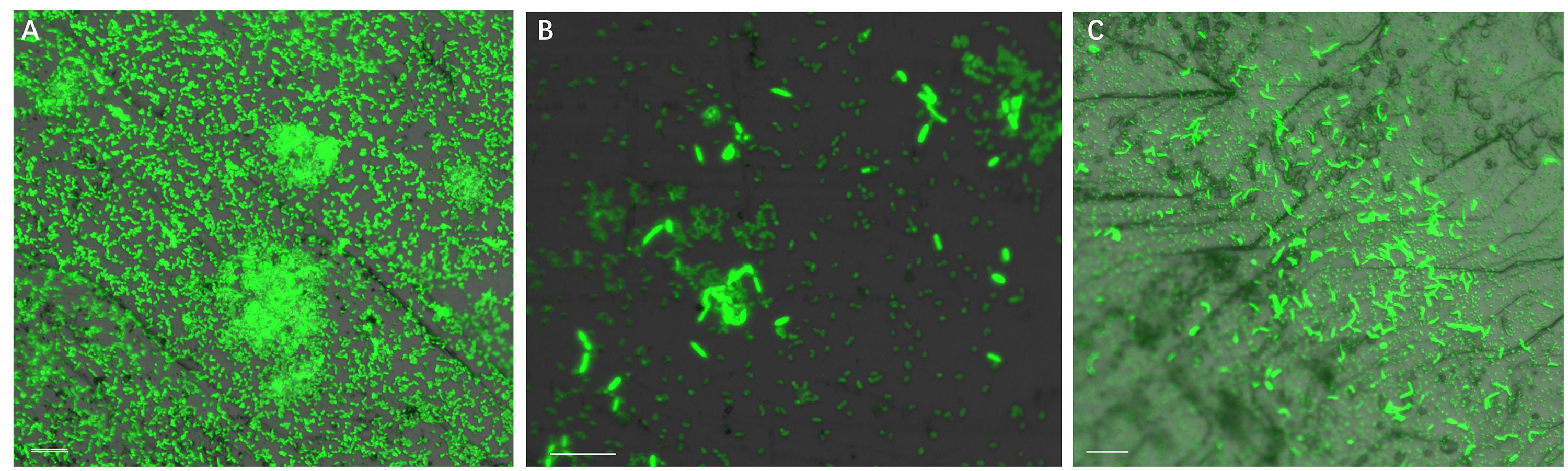
Figure 6. Biofilm formation by S. thermosulfidooxidans on pyrite pre-colonized with 1 day old inactivated biofilm of L. ferriphilum under 37°C with an initial pH value of 1.8. Images (A–C) show the binary biofilm of S. thermosulfidooxidans and L. ferriphilum after 1, 7 and 16 days incubation. Scale bar, 10 μm.
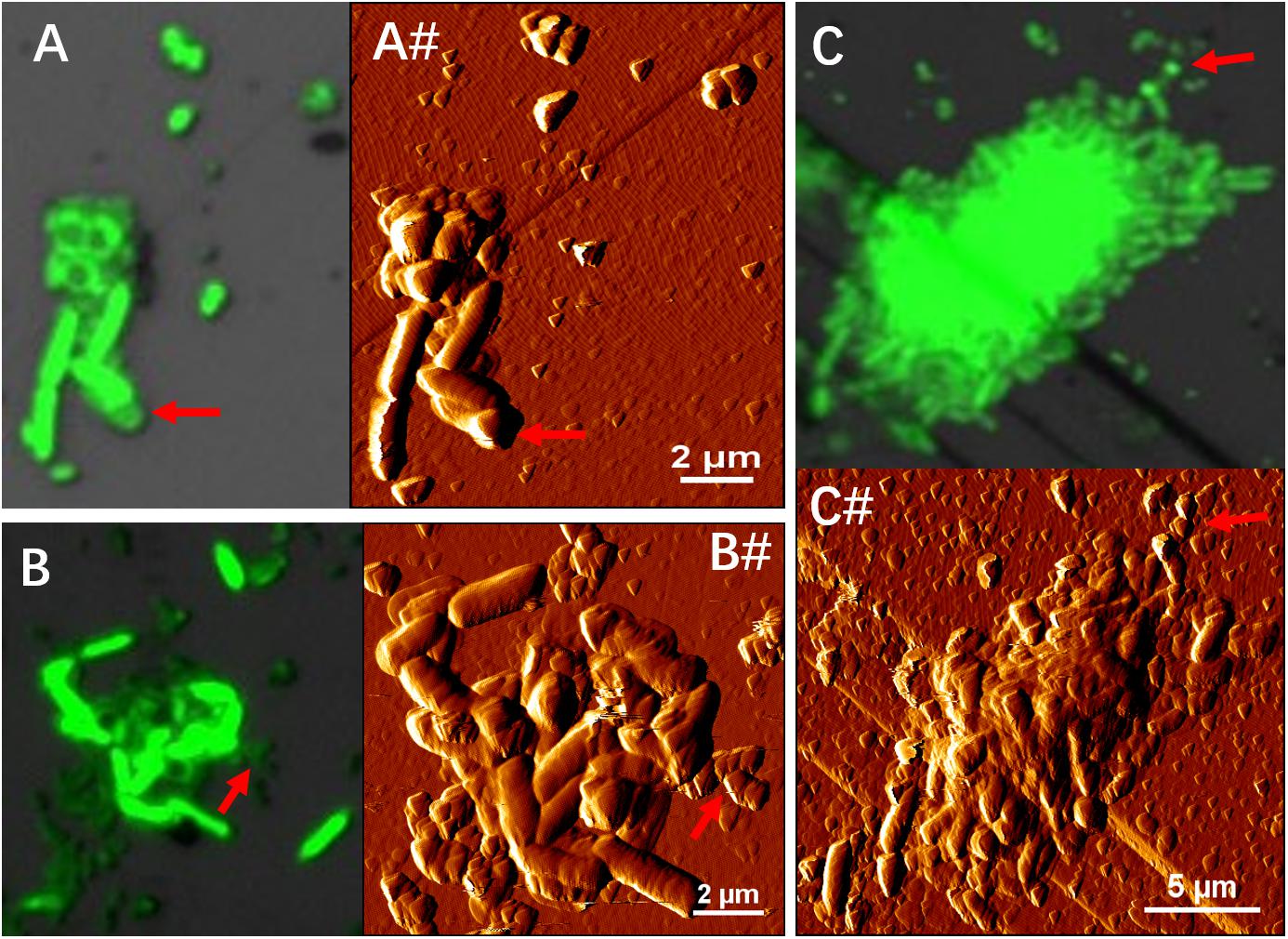
Figure 7. EFM (A–C) combined AFM (A#–C#) images indicate physical contacts between cells of S. thermosulfidooxidans and cells of L. ferriphilum during biofilm formation on pyrite under 37°C with an initial pH value of 1.8. Images (A,A#,B,B#) show the biofilms of S. thermosulfidooxidans on pyrite pre-colonized with 1 day old living and inactivated biofilms of L. ferriphilum. Images C and C# show biofilm developed by culture of S. thermosulfidooxidans and L. ferriphilum on a clean pyrite. All the red arrows in images indicate the cells of L. ferriphilum.
A physical contact has been reported also for other microorganisms and it happens during microbial coaggregation. Coaggregation, the process of adhesion between genetically distinct bacterial partners, was first found between bacteria isolated from human dental plaque in 1970 (Gibbons and Nygaard, 1970). It is an integral process in the development of multispecies biofilms and is caused by specific ligand receptor interactions (Rickard et al., 2003). In a dual-species coaggregation one of the species will benefit from being within a coaggregation, while the other may also gain or not gain advantages as compared to planktonic cells. Katharios-Lanwermeyer et al. (2014) reviewed coaggregation mediated interactions and concluded five potential outcomes for biofilm communities: (1) The interspecies can rapidly form aggregates because of the enhanced cell-cell adhesion; (2) Species within the coaggregation can exchange of metabolites; (3) The coaggregation can aid one species to kill/inactivate another or to protect both species from predation; (4) The coaggregation reduces the distance between cells, thereby facilitating cell-cell signaling; (5) The coaggregation can protect cells from adverse events, enhance their antimicrobial resistance and genetic exchange.
In this study, obviously, coaggregation enhanced the adhesion of S. thermosulfidooxidans. However, how the two species developed the coaggregation or whether it was also caused by specific ligand receptor interactions, and whether this coaggregation could facilitate metabolite exchange or cell-cell signaling remains unclear. More delicate experiments need to be done to answer these questions.
Bioleaching of Pyrite by S. thermosulfidooxidans in the Presence of L. ferriphilum
For an improved understanding the assays are numbered as follows: (1) S. thermosulfidooxidans incubated with pyrite pre-colonized with 1 day old living biofilms of L. ferriphilum; (2) S. thermosulfidooxidans incubated with pyrite pre-colonized with 1 day old inactivated biofilms of L. ferriphilum; (3) S. thermosulfidooxidans and L. ferriphilum were simultaneously inoculated into assays with sterile pyrite; (4) pyrite colonized with 1 day old biofilms of L. ferriphilum (without planktonic cells); (5) S. thermosulfidooxidans incubated with sterile pyrite. Data for an assay with sterile pyrite are not shown, since no dissolution was detected.
The leaching of pyrite by S. thermosulfidooxidans in the presence of L. ferriphilum was calculated and the results are shown in Figure 8. In all assays the pH decreased, which indicates that bioleaching occurred. Since L. ferriphilum can oxidize only ferrous ion for energy but S. thermosulfidooxidans can use both, ferrous ion and RISCs, as energy source, the pH in assay 4 decreased the least. The pH decreased the most in assays 1 and 3, but in the first 14 days the pH in assay 3 was lower than it in assay 1. After 16 days of leaching the highest iron concentration was detected in assay 4 with a final value of 1.5 g/L, followed by the one in assay 3 with 1 g/L. There were 0.5 g/L and 0.3 g/L iron detected in assay 1 and assay 2, respectively. The data indicate that with biofilms of L. ferriphilum at the beginning of leaching, no matter whether living or inactivated, these biofilms exerted a negative effect on leaching by S. thermosulfidooxidans.
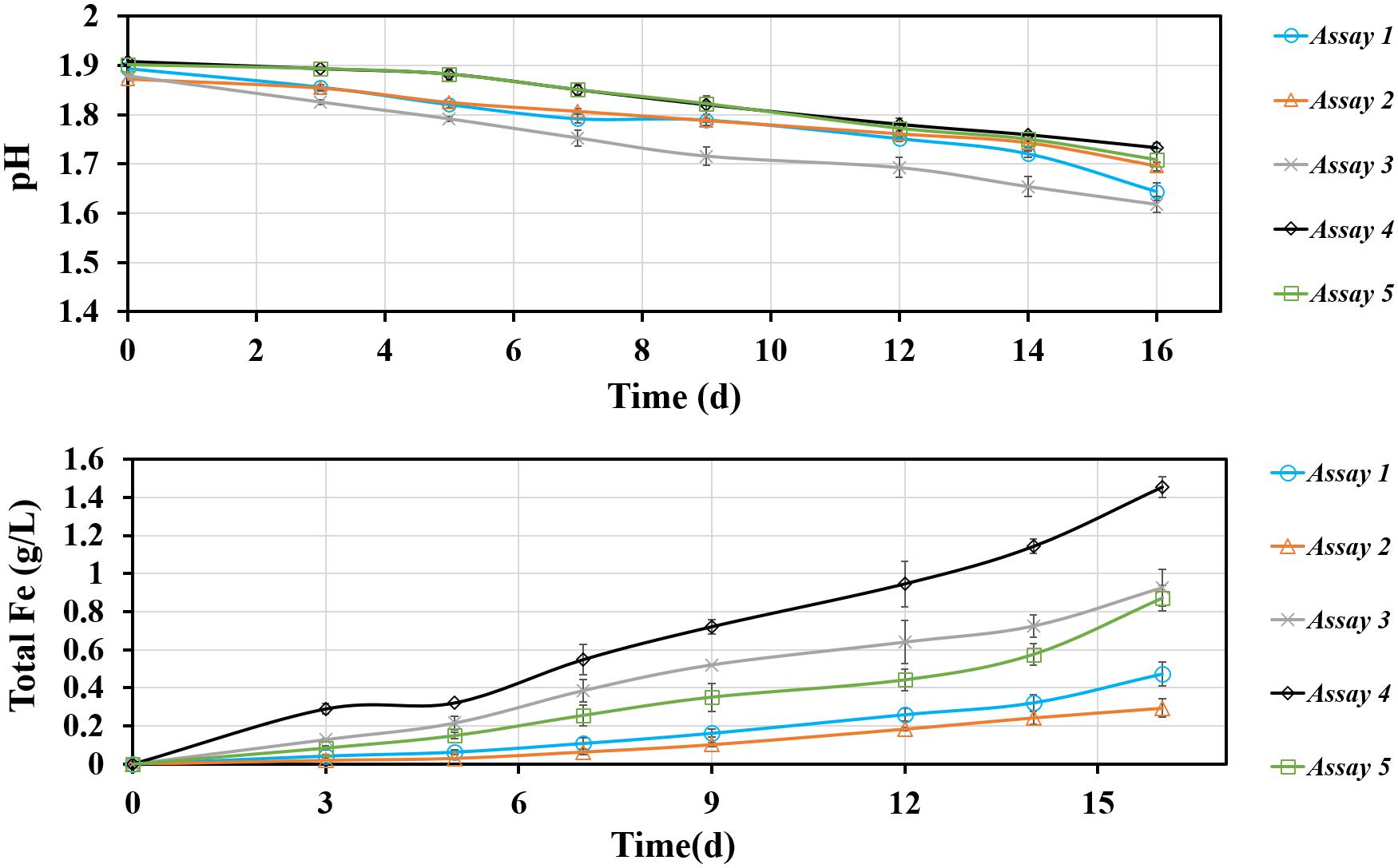
Figure 8. Changes of pH and total iron concentration as a function of time during pyrite bioleaching under 37°C with an initial pH value of 1.8. Assay 1, S. thermosulfidooxidans with 1 day old living biofilms of L. ferriphilum incubated in sterile medium; Assay 2, S. thermosulfidooxidans with 1 day old inactivated biofilms of L. ferrriphilum incubated in sterile medium; Assay 3, S. thermosulfidooxidans and L. ferriphilum were simultaneously inoculated and incubated with sterile pyrite and medium; Assay 4, Pyrite colonize with 1 day old biofilms of L. ferriphilum incubated in sterile medium; Assay 5, S. thermosulfidooxidans incubated in sterile medium with sterile pyrite.
It is known that leaching bacteria prefer to colonize the crystal defect sites on a mineral surface and only attached cells can initiate bioleaching (Bellenberg et al., 2015). Obviously the inactivated biofilms of L. ferriphilum in assay 2 (Figure 8) could not perform leaching and to some extent they protected the pyrite surfaces from being attacked by ferric ions. Also the sites for attachment of S. thermosulfidooxidans were blocked. Consequently, the leaching needed longer time to start and the amount of leached iron was the least among all assays (Figure 8). For living biofilms of L. ferriphilum the introduction of S. thermosulfidooxidans might mean introducing a competitor for iron, which can be indicated by the higher iron concentration in assays 4 and 5 (single culture) than in assay 1. Interestingly, also with living L. ferriphilum the pH in assay 3 was much lower and the concentration of iron ion was much higher than in assay 1 (Figure 8). An increased concentration of iron ions mean dissolution of pyrite and a decreased pH means oxidation of RISCs. S. thermosulfidooxidans is the only sulfur-oxidizer in the system. Thus, most likely in assay 1 S. thermosulfidooxidans grew mainly on ferrous ion and the competition led to the bad leaching performance. In assay 3 the decreased pH illustrated the oxidation of RISCs by S. thermosulfidooxidans, but their worse leaching performance than that of the pure culture of L. ferriphilum indicated a negative effect on growth of L. ferriphilum. A possible explanation is that in assay 3 S. thermosulfidooxidans grew on both ferrous ion and RISCs.
Based on the results above two points can be highlighted:
1. The living state of L. ferriphilum at the start has a different influence on leaching performance of the binary culture and this difference might be relate to the metabolic preference of S. thermosulfidooxidans.
2. Contact leaching is important. Inactivated biofilms of L. ferriphilum blocked the attachment sites for S. thermosulfidooxidans on pyrite surfaces, resulting in the lowest leaching efficiency.
Bioleaching of Pyrite by S. thermosulfidooxidans in Spent Leach Liquor
The effects of spent leach liquor from L. ferriphilum on pyrite leaching by S. thermosulfidooxidans was also investigated. In order to reproduce a natural leaching system yeast extract was not added in this section. Firstly, bioleaching of pyrite by S. thermosulfidooxidans without addition of yeast extract was tested and the results are shown in Figure 9. From Figure 9A it can be seen that in the first 12 days the iron concentration increased from 0 to 260 mg/L. Afterward it almost leveled off at 300 mg/L. The iron ions were detected mostly in the form of Fe(III) in the first 9 days. However, from 12 days on Fe(II) became gradually the dominant form. At the end of the experiment the iron ions were almost all in the form of Fe(II). Data in Figure 9B indicate that the pH of the leaching system decreased rapidly from the 6th day on till the 15th day when it did not change any more. The numbers of planktonic cells decreased, from the initial cell density of 1.1 × 108 cells/mL to the final cell density of 4 × 106 cells/mL. The number of attached cells was also monitored. The bar chart (Figure 9C) indicates that the number of attached cells increased during the leaching and, finally, the pyrite surfaces were covered with 5 × 106 cells/cm2 (Figures 9D,E). Our data clearly indicate that after around 14 days of incubation the bioleaching stopped and the cells of S. thermosulfidooxidans started to adhere to pyrite surfaces. It remains, however, unclear, why these events occurred. A similar phenomenon has been reported, where adhesion of Acidithiobacillus ferrooxidans to pyrite was enhanced if no phosphate was added to the growth medium (Bellenberg et al., 2012). In our study lack of nutrition, such as yeast extract, might account for the observed events.
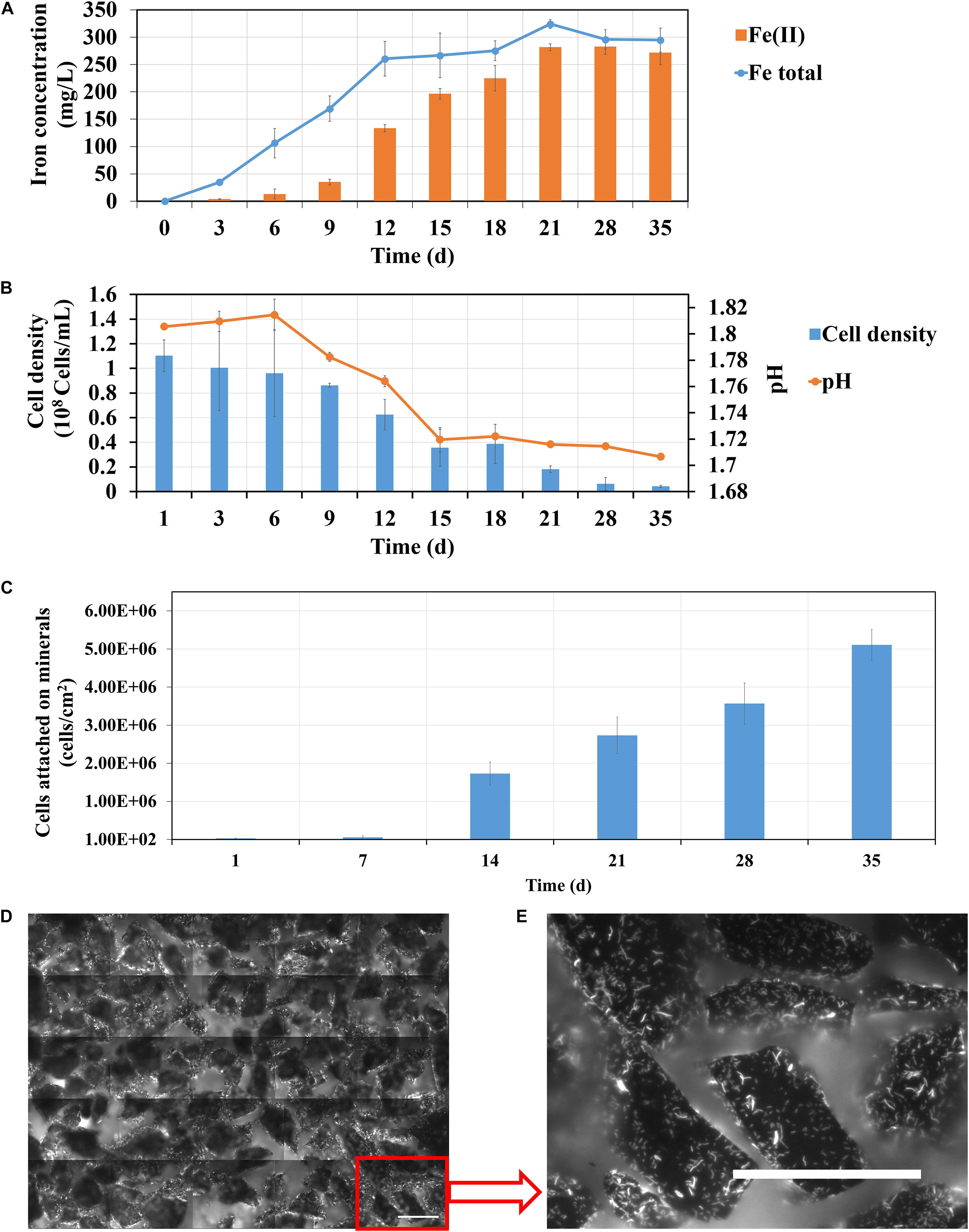
Figure 9. Changes of iron concentration (A), cell density (B) and pH (C) during pyrite leaching by S. thermosulfidooxidans in basal salt medium without yeast extract addition under 37°C with an initial pH value of 1.8. (D,E) are EFM images showing the biofilms formed on pyrite after 35 days of leaching. Scale bar, 100 μm.
Bioleaching of pyrite by S. thermosulfidooxidans with pyrite leachate from L. ferriphilum was then investigated. Figure 10 shows, how each parameter changes during bioleaching, if the bioleaching was performed in 3 days or 14 days old pyrite leachate from L. ferriphilum. Although the leachates were of different age, the results of the three assays were similar: (1) The bioleaching ran better in the biological control than with the leachate; (2) A large amount of Fe(II) was detected in all leaching assays at the end of the experiment (Figures 10A,C); (3) Except for the chemical control the pH in all leaching assays decreased and the planktonic cell densities of S. thermosulfidooxidans also decreased (Figures 10B,D); (4) The number of attached cells increased in all leaching assays (Figure 10E).
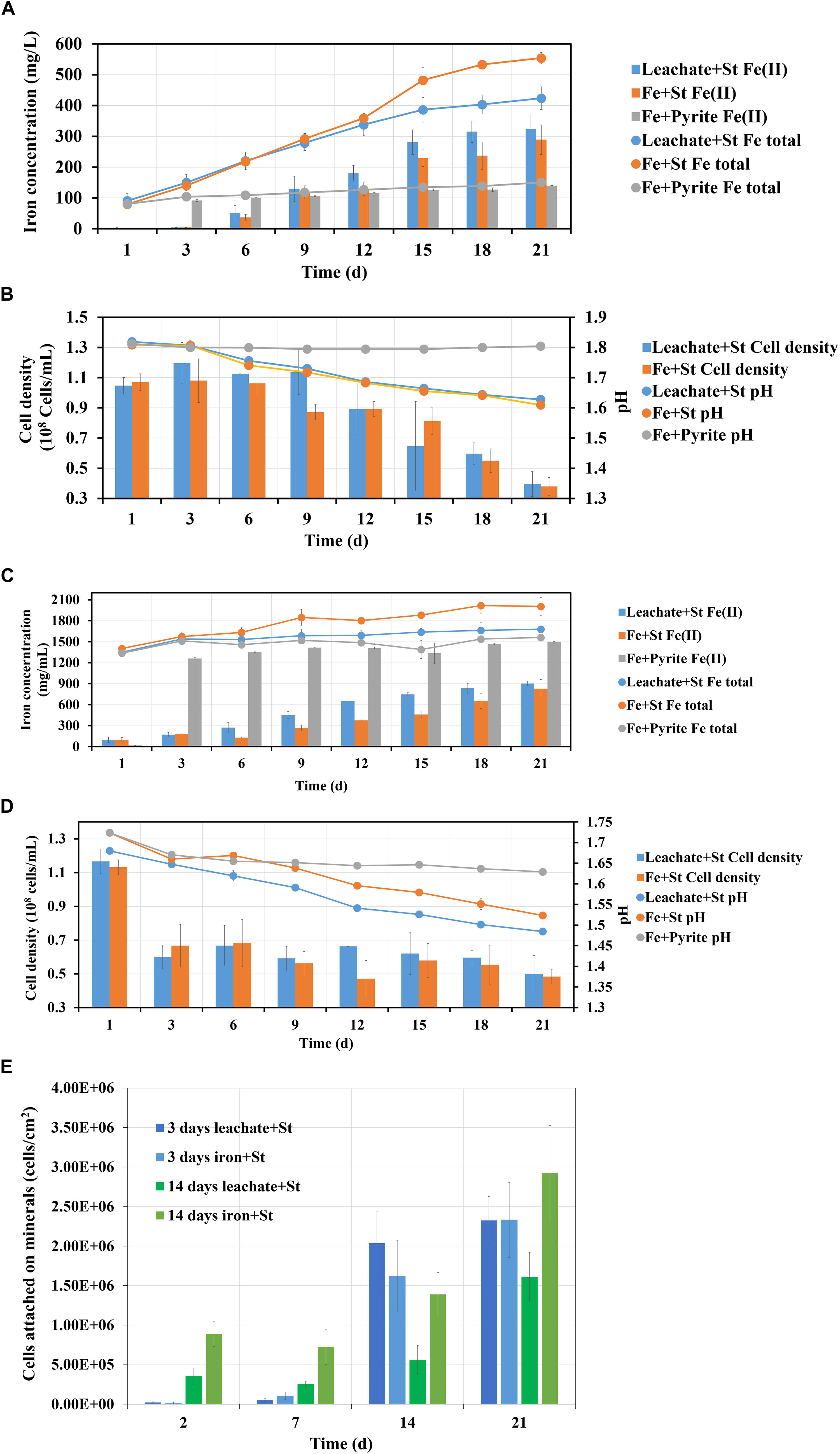
Figure 10. Images (A–E) demonstrate the changes of iron concentration, cell density and pH during pyrite leaching by S. thermosulfidooxidans with 3 days (A,B) and 14 days (C,D) pyrite leachate collected from L. ferriphilum under 37°C. Image (E) shows the number of attached cells of S. thermosulfidooxidans on pyrite. Leachate + St, S. thermosulfidooxidans performs pyrite leaching in pyrite leachate collected from L. ferriphilum; Fe + St, S. thermosulfidooxidans performs pyrite leaching in MAC medium with initial addition of same amount of iron detected in the pyrite leachate collected from L. ferriphilum; Fe + pyrite, pyrite leaching in MAC medium only with initial addition of same amount of iron detected in the pyrite leachate collected from L. ferriphilum.
There are also differences: (1) The age of the leachate influenced the time until the iron concentration started to differ. Specifically, if S. thermosulfidooxidans was incubated with 3 days old leachate, only after 12 days the total iron concentration became lower than in the biological control (Figure 10A). If the cells were incubated in 14 days old leachate, the concentration of total iron differed between the test assays and the biological control from the 3rd day on (Figure 10C). Also due to the age of the leachate the percentage of Fe(II) was different. With 3 days old leachate after 21 days of bioleaching the Fe(II) concentration amounted to 76%. In the biological control the percentage of Fe(II) was 52% (Figure 10A). If the leachate was 14 days old, the assays after 21 days contained 53% of Fe(II), whereas 41% of Fe(II) was measured in the biological control (Figure 10C). (2) The time, until a decrease of the pH occurred, differed also. In the assays with 3 days old leachate the pH started to decrease from the 3rd day on in both assays (Figure 10B). In the assays with 14 days old leachate the pH in both groups decreased right from the start (Figure 10D). (3) For adhesion differences were noted. In the assays with 3 days old leachate in all assays from the 14th day on the cells were detected mostly attached to pyrite surfaces, while in the assays with 14 days old leachate from the very beginning the cells of both assays were detected largely attached on pyrite surfaces. However, in the assays with 14 days old leachate the numbers of attached cells were always lower than it in the biological control, which should be related to the organic products produced by L. ferriphilum (Figure 10E).
From these results three points can be concluded:
1. Autotrophic (control) or rare organic (leachate) nutrition would lead S. thermosulfidooxidans to adhere on pyrite after a certain time of bioleaching, but the adhesion does not show positive effects on bioleaching. With the adhesion a large amount of Fe(II) is detected in the leaching system.
2. Iron in the bioleaching system at the very beginning would affect the bioleaching by S. thermosulfidooxidans under autotrophic or rare organic nutrition conditions: when there is no iron, the bioleaching would level off after a certain time (Figure 9); however, when there is iron, the bioleaching would continue or at least the oxidization of RISCs would continue (Figure 10). Most likely biofilms of S. thermosulfidooxidans grow mainly on RISCs (if enough exist) especially under the condition of rare organic nutrition and/or of high concentration of iron ion. Before we cultivated S. thermosulfidooxidans with sulfur slices and we found that they could develop biofilms although the yeast extract in medium was consumed as time went by (Figure 11). However, it could not develop biofilms on pyrite slices (Figure 1).
3. Based on differences 1 and 3 described above, it can be concluded that the leachate from L. ferriphilum affects iron oxidization and adhesion of S. thermosulfidooxidans (Figure 10).
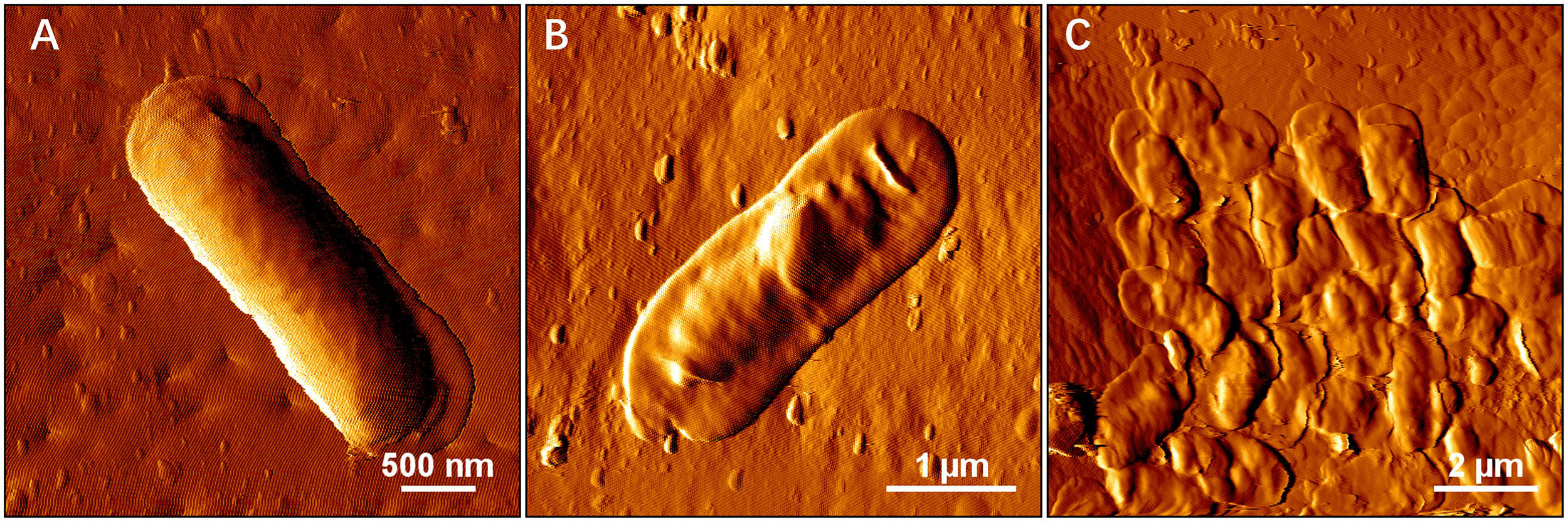
Figure 11. AFM images show biofilm formation on sulfur slices by S. thermosulfidooxidans. Images (A–C) show biofilms after 4 h, 2 and 5 days incubation with sulfur slices in MAC medium with initially addition of 0.02% yeast extracts, respectively.
Conclusion
Adhesion to and biofilm formation on mineral surfaces are critical steps in bioleaching. Understanding the interactions between two important species is helpful to design a microbial community for bioleaching or to prevent AMD. In this study we checked the effects from L. ferriphilum, including its planktonic cells, its living biofilms, its inactivated biofilms or its metabolic substances, on bioleaching of pyrite by S. thermosulfidooxidans. Our studies indicate the importance of contact leaching. Coaggregation of L. ferriphilum and S. thermosulfidooxidans can enhance the biofilm formation by S. thermosulfidooxidans on pyrite surfaces. However, the way of addition of the two species shows different effects on bioleaching, which might be related to metabolic preference of S. thermosulfidooxidans. If the minerals are pre-colonized with biofilms of L. ferriphilum, S. thermosulfidooxidans prefers to grow on iron. Consequently, a competition between the two species lead to a worse leaching performance than that of either pure culture. However, if the cultures of S. thermosulfidooxidans and of L. ferriphilum are simultaneously inoculated to a leaching system, S. thermosulfidooxidans grows on both iron and RISCs, resulting in an improved leaching efficiency compared with the leaching performed by pure S. thermosulfidooxidans. In summary the leaching performance can be ranked as follows: biofilms of L. ferriphilum (Lf) > mixtures of Lf + S. thermosulfidooxidans (St) > St > St + living biofilms of Lf > St + inactivated biofilms of Lf. Thus, the role of S. thermosulfidooxidans in a short-term leaching system is most likely decided by the time/sequence of introduction into the habitat.
Data Availability Statement
All datasets generated for this study are included in the article/supplementary material.
Author Contributions
QL, TX, and WS designed the experiments. QL, JZ, SL, and RZ performed the experiments and analyzed the data. QL, TX, and WS prepared the manuscript. All authors read and approved the final manuscript.
Funding
This work was supported by the National Natural Science Foundation of China (Nos. 41903068, 41773089, U1612442, and 41673138) and the German Federation of Industrial Research Associations (AiF Project IGF 18352 N).
Conflict of Interest
The authors declare that the research was conducted in the absence of any commercial or financial relationships that could be construed as a potential conflict of interest.
References
Absolom, D. R., Lamberti, F. V., Policova, Z., Zingg, W., Van Oss, C. J., and Neumann, A. W. (1983). Surface thermodynamics of bacterial adhesion. Appl. Environ. Microbiol. 46, 90–97. doi: 10.1128/aem.46.1.90-97.1983
Acosta, M., Galleguillos, P., Ghorbani, Y., Tapia, P., Contador, Y., Velásquez, A., et al. (2014). Variation in microbial community from predominantly mesophilic to thermotolerant and moderately thermophilic species in an industrial copper heap bioleaching operation. Hydrometallurgy 150, 281–289. doi: 10.1016/j.hydromet.2014.09.010
Bellenberg, S., Barthen, R., Boretska, M., Zhang, R., Sand, W., and Vera, M. (2015). Manipulation of pyrite colonization and leaching by iron-oxidizing Acidithiobacillus species. Appl. Microbiol. Biotechnol. 99, 1435–1449. doi: 10.1007/s00253-014-6180-y
Bellenberg, S., Díaz, M., Noël, N., Sand, W., Poetsch, A., Guiliani, N., et al. (2014). Biofilm formation, communication and interactions of leaching bacteria during colonization of pyrite and sulfur surfaces. Res. Microbiol. 165, 773–781. doi: 10.1016/j.resmic.2014.08.006
Bellenberg, S., Leon-Morales, C.-F., Sand, W., and Vera, M. (2012). Visualization of capsular polysaccharide induction in Acidithiobacillus ferrooxidans. Hydrometallurgy 129, 82–89. doi: 10.1016/j.hydromet.2012.09.002
Blackman, I. C., and Frank, J. F. (1996). Growth of Listeria monocytogenes as a biofilm on various food-processing surfaces. J. Food Prot. 59, 827–831. doi: 10.4315/0362-028X-59.8.827
Bogdanova, T. I., Tsaplina, I. A., Kondrat’eva, T. F., Duda, V. I., Suzina, N. E., Melamud, V. S., et al. (2006). Sulfobacillus thermotolerans sp. nov., a thermotolerant, chemolithotrophic, chemolithotrophic bacterium. Int. J. Syst. Evol. Microbiol. 56, 1039–1042. doi: 10.1099/ijs.0.64106-0
Castro, C., Zhang, R., Liu, J., Bellenberg, S., Neu, T. R., Donati, E., et al. (2016). Biofilm formation and interspecies interactions in mixed cultures of thermo-acidophilic archaea Acidianus spp. and Sulfolobus metallicus. Res. Microbiol. 167, 604–612. doi: 10.1016/j.resmic.2016.06.005
Dexter, S., Sullivan, J., Williams, J., and Watson, S. (1975). Influence of substrate wettability on the attachment of marine bacteria to various surfaces. Appl. Microbiol. 30, 298–308. doi: 10.1128/aem.30.2.298-308.1975
Gibbons, R. J., and Nygaard, M. (1970). Interbacterial aggregation of plaque bacteria. Arch. Oral. Biol. 15, 1397–1400.
Golovacheva, R., and Karavaĭko, G. (1978). Sulfobacillus, a new genus of Thermophilic sporulating bacteria. Mikrobiologiia 47, 815–822.
Helke, D. M., Somers, E. B., and Wong, A. C. (1993). Attachment of Listeria monocytogenes and Salmonella typhimurium to stainless steel and Buna-N in the presence of milk and individual milk components. J. Food Prot. 56, 479–484. doi: 10.4315/0362-028X-56.6.479
Johnson, D. B., Joulian, C., D’hugues, P., and Hallberg, K. B. (2008). Sulfobacillus benefaciens sp. nov., an acidophilic facultative anaerobic Firmicute isolated from mineral bioleaching operations. Extremophiles 12, 789–798. doi: 10.1007/s00792-008-0184-4
Katharios-Lanwermeyer, S., Xi, C., Jakubovics, N., and Rickard, A. (2014). Mini-review: microbial coaggregation: ubiquity and implications for biofilm development. Biofouling 30, 1235–1251. doi: 10.1080/08927014.2014.976206
Li, Q., Sand, W., and Zhang, R. (2016). Enhancement of biofilm formation on pyrite by Sulfobacillus thermosulfidooxidans. Minerals 6:71. doi: 10.3390/min6030071
Liu, J., Li, Q., Sand, W., and Zhang, R. (2016). Influence of Sulfobacillus thermosulfidooxidans on initial attachment and pyrite leaching by Thermoacidophilic Archaeon Acidianus sp. DSM 29099. Minerals 6:76. doi: 10.3390/min6030076
Mackintosh, M. E. (1978). Nitrogen fixation by Thiobacillus ferrooxidans. Microbiology 105, 215–218. doi: 10.1099/00221287-105-2-215
Mangold, S., Harneit, K., Rohwerder, T., Claus, G., and Sand, W. (2008). Novel combination of atomic force microscopy and epifluorescence microscopy for visualization of leaching bacteria on pyrite. Appl. Environ. Microbiol. 74, 410–415. doi: 10.1128/aem.01812-07
Markosyan, G. (1972). A new iron-oxidizing bacterium, Leptospirillum ferrooxidans gen. Biol. Zh. Arm. 25:26.
Maulani, N., Li, Q., Sand, W., Vera, M., and Zhang, R. (2016). Interactions of the extremely acidophilic archaeon Ferroplasma acidiphilum with acidophilic bacteria during pyrite bioleaching. Appl. Environ. Biotechnol. 1, 43–55.
Noël, N., Florian, B., and Sand, W. (2010). AFM & EFM study on attachment of acidophilic leaching organisms. Hydrometallurgy 104, 370–375.
Okibe, N., Gericke, M., Hallberg, K. B., and Johnson, D. B. (2003). Enumeration and characterization of acidophilic microorganisms isolated from a pilot plant stirred-tank bioleaching operation. Appl. Environ. Microbiol. 69, 1936–1943. doi: 10.1128/aem.69.4.1936-1943.2003
Okibe, N., and Johnson, D. B. (2004). Biooxidation of pyrite by defined mixed cultures of moderately Thermophilic acidophiles in pH-controlled bioreactors: significance of microbial interactions. Biotechnol. Bioeng. 87, 574–583. doi: 10.1002/bit.20138
Quirynen, M., Van Der Mei, H., Bollen, C., Schotte, A., Marechal, M., Doornbusch, G., et al. (1993). An in vivo study of the influence of the surface roughness of implants on the microbiology of supra-and subgingival plaque. J. Dent. Res. 72, 1304–1309. doi: 10.1177/00220345930720090801
Rawlings, D. E., and Johnson, D. B. (2007). The microbiology of biomining: development and optimization of mineral-oxidizing microbial consortia. Microbiology 153, 315–324. doi: 10.1099/mic.0.2006/001206-0
Rickard, A. H., Gilbert, P., High, N. J., Kolenbrander, P. E., and Handley, P. S. (2003). Bacterial coaggregation: an integral process in the development of multi-species biofilms. Trends Microbiol. 11, 94–100. doi: 10.1016/s0966-842x(02)00034-3
Sand, W., Gehrke, T., Jozsa, P.-G., and Schippers, A. (2001). Biochemistry of bacterial leaching-direct vs. indirect bioleaching. Hydrometallurgy 59, 159–175. doi: 10.1016/s0304-386x(00)00180-8
Sand, W., Rohde, K., Sobotke, B., and Zenneck, C. (1992). Evaluation of Leptospirillum ferrooxidans for leaching. Appl. Environ. Microbiol. 58, 85–92. doi: 10.1128/aem.58.1.85-92.1992
Schindelin, J., Arganda-Carreras, I., Frise, E., Kaynig, V., Longair, M., Pietzsch, T., et al. (2012). Fiji: an open-source platform for biological-image analysis. Nat. Methods 9:676. doi: 10.1038/nmeth.2019
Schippers, A., Jozsa, P., and Sand, W. (1996). Sulfur chemistry in bacterial leaching of pyrite. Appl. Environ. Microbiol. 62, 3424–3431.
Spolaore, P., Joulian, C., Gouin, J., Morin, D., and D’hugues, P. (2011). Relationship between bioleaching performance, bacterial community structure and mineralogy in the bioleaching of a copper concentrate in stirred-tank reactors. Appl. Microbiol. Biotechnol. 89, 441–448. doi: 10.1007/s00253-010-2888-5
Tamura, H., Goto, K., Yotsuyanagi, T., and Nagayama, M. (1974). Spectrophotometric determination of iron (II) with 1, 10-phenanthroline in the presence of large amounts of iron (III). Talanta 21, 314–318.
Keywords: Sulfobacillus thermosulfidooxidans, Leptospirillum ferriphilum, bioleaching, adhesion, biofilm
Citation: Li Q, Zhu J, Li S, Zhang R, Xiao T and Sand W (2020) Interactions Between Cells of Sulfobacillus thermosulfidooxidans and Leptospirillum ferriphilum During Pyrite Bioleaching. Front. Microbiol. 11:44. doi: 10.3389/fmicb.2020.00044
Received: 25 September 2019; Accepted: 09 January 2020;
Published: 29 January 2020.
Edited by:
Eric D. van Hullebusch, Université de Paris, FranceReviewed by:
Cecilia Susana Demergasso, Catholic University of the North, ChileSandeep Panda, Süleyman Demirel University, Turkey
Pedro Antonio Galleguillos, Centro de Investigación Científico y Tecnológico para la Minería (CICITEM), Chile
Copyright © 2020 Li, Zhu, Li, Zhang, Xiao and Sand. This is an open-access article distributed under the terms of the Creative Commons Attribution License (CC BY). The use, distribution or reproduction in other forums is permitted, provided the original author(s) and the copyright owner(s) are credited and that the original publication in this journal is cited, in accordance with accepted academic practice. No use, distribution or reproduction is permitted which does not comply with these terms.
*Correspondence: Tangfu Xiao, tfxiao@gzhu.edu.cn; Wolfgang Sand, wolfgang.sand@uni-due.de