- 1División de Ciencias Ambientales, Instituto Potosino de Investigación Científica y Tecnológica, San Luis Potosí, Mexico
- 2División de Biología Molecular, Instituto Potosino de Investigación Científica y Tecnológica, San Luis Potosí, Mexico
- 3Laboratory for Research on Advanced Processes for Water Treatment, Engineering Institute, Campus Juriquilla, Universidad Nacional Autónoma de México, Querétaro, Mexico
Humic substances are redox-active organic molecules, which play pivotal roles in several biogeochemical cycles due to their electron-transferring capacity involving multiple abiotic and microbial transformations. Based on the redox properties of humic substances, and the metabolic capabilities of microorganisms to reduce and oxidize them, we hypothesized that they could mediate the anaerobic oxidation of methane (AOM) coupled to the reduction of nitrous oxide (N2O) in wetland sediments. This study provides several lines of evidence indicating the coupling between AOM and the reduction of N2O through an extracellular electron transfer mechanism mediated by the redox active functional groups in humic substances (e.g., quinones). We found that the microbiota of a sediment collected from the Sisal wetland (Yucatán Peninsula, southeastern Mexico) was able to reduce N2O (4.6 ± 0.5 μmol N2O g sed.–1 day–1) when reduced humic substances were provided as electron donor in a close stoichiometric relationship. Furthermore, a microbial enrichment derived from the wetland sediment achieved simultaneous 13CH4 oxidation (1.3 ± 0.1 μmol 13CO2 g sed.–1 day–1) and N2O reduction (25.2 ± 0.5 μmol N2O g sed.–1 day–1), which was significantly dependent on the presence of humic substances as an extracellular electron shuttle. Taxonomic characterization based on 16S rRNA gene sequencing revealed Acinetobacter (a ɣ-proteobacterium), the Rice Cluster I from the Methanocellaceae and an uncultured archaeon from the Methanomicrobiaceae family as the microbes potentially involved in AOM linked to N2O reduction mediated by humic substances. The findings reported here suggest that humic substances might play an important role to prevent the emission of greenhouse gases (CH4 and N2O) from wetland sediments. Further efforts to evaluate the feasibility of this novel mechanism under the natural conditions prevailing in ecosystems must be considered in future studies.
Introduction
Continuous emissions of greenhouse gases (GHG), such as carbon dioxide (CO2), methane (CH4), and nitrous oxide (N2O) have been associated to several environmental problems that include global warming (GW), alterations of precipitation patterns, changes in groundwater levels and soil conditions, as well as extreme weather events (Kumar et al., 2020). Therefore, intensive research is currently underway to elucidate the microbial and abiotic processes driving these GHG emissions from natural environments.
Wetlands are highly dynamic ecosystems that, collectively, constitute the largest biogenic source of GHG (Turetsky et al., 2014). For instance, the net amount of CH4 released from these environments represents one third of the global CH4 budget (∼164 Tg yr–1) (Bridgham et al., 2013). Regarding N2O, the global emissions estimation from coastal wetlands is up to 4.8 Tg N year–1 and this amount could be further increased due to anthropogenic exacerbation of the N cycle (Murray et al., 2015). Altogether, CH4 and N2O are two of the most hazardous GHG, both because of their high GW potential (25 and 300 times higher than that of CO2, respectively), and because of their long residence time in the Earth’s atmosphere (12 and 114 years, respectively) (Tangen and Bansal, 2019).
Anaerobic degradation of natural organic matter (NOM) by microorganisms, which involves the methanogenesis process, constitutes an important source for CO2 and CH4 emissions from aquatic ecosystems (Gougoulias et al., 2014; Laruelle et al., 2014; Lever, 2016). In the same fashion, N2O is produced by anaerobic microbes in nitrogen-rich environments due to incomplete denitrification, and through the nitrification process occurring in the oxic-anoxic interfaces present above and below oxygen deficient zones (Babbin et al., 2015; Ji et al., 2015; Pajares and Ramos, 2019).
As a counterpart of these microbial sources of GHG emissions, there are several mechanisms for CH4 and N2O microbial uptake, which have been extensively described. Regarding CH4, after assuming for decades that only aerobic microbes could oxidize this very stable compound (via a monooxygenase activation), several inorganic terminal electron acceptors (TEAs) have been reported to support anaerobic methane oxidation (AOM) by specialized anaerobic microorganisms (Gupta et al., 2013; Segarra et al., 2015). These TEAs include sulfate (SO42–), nitrate and nitrite (NO3–, NO2–), as well as metallic oxides of iron [Fe(III)] and manganese [Mn(IV)] (Welte et al., 2016; He et al., 2018; Bhattarai et al., 2019). Recently, the redox-active fraction of the continuously decaying NOM, commonly referred to as humic substances (Lehmann and Kleber, 2015), as well as their structural analogs (e.g., quinones), have also been found to be suitable TEAs for achieving AOM (Scheller et al., 2016; Valenzuela et al., 2017; Bai et al., 2019). Moreover, humic substances can promote AOM not only by acting as TEA, but also by shuttling electrons derived from AOM toward metallic oxides reduction (Smemo and Yavitt, 2011; He et al., 2019; Valenzuela et al., 2019).
Concerning N2O, the only known microbial process responsible for its consumption involves its reduction to molecular nitrogen (N2). This one-step transformation is achieved by nosZ gene (nitrous oxide reductase) bearing microorganisms, which might not be mandatorily denitrifiers (Hallin et al., 2018). Regularly, the source of electrons for this reaction comes from labile molecules in NOM. Additionally, it has recently been reported that CH4 could serve as an electron donor for this reaction, implying the existence of microorganisms capable of coupling the simultaneous consumption of two GHG (Cheng et al., 2019). Despite this, the underlying mechanisms and the main environmental drivers of this process remain unknown. Taking into account the previous evidence showing that reduced humic substances could serve as electron donor for denitrification (Aranda-Tamaura et al., 2007; van Trump et al., 2011), and that oxidized humic substances could support AOM by serving as TEA (Valenzuela et al., 2017), we aimed to decipher if they could mediate AOM linked to N2O reduction via an inter-species electron transfer (IET) process. It has previously been proven that humic substances and other quinone-containing materials (such as biochar or activated carbon) may link the oxidation and reduction of molecules that one single microorganism could not accomplish due to metabolic limitations (Liu et al., 2012; Lovley, 2017; Rotaru et al., 2018).
Materials and Methods
Wetland and Sediment Sampling Description
The Sisal wetland is located in the coastal zone of the Yucatán Peninsula (southeastern Mexico, 21°09′26′′N, 90°03′09′′W). This marsh possesses a semi-arid climate, a high degree of karstification, as well as intermittent saltwater inputs from the ocean causing variable salinity levels (Batllori-Sampedro et al., 1999). Sediment cores were collected from the wetland in January 2016. The cores were sampled under a water column of approximately 70 cm in depth and the length of the cores was 15 cm. All sediment and water samples were stored in tight sealed plastic containers, which were maintained in ice until their arrival to the laboratory where they were then stored at 4°C in a dark room for 18 months before conducting the incubations. Before performing the incubation assays, sediment and its pore water were chemically characterized. Some of the most relevant chemical components found in the collected water column samples and extracted pore water were sulfate and nitrate due to its potential role as electron acceptors for microbial activity. Potential electron donors identified were sulfide and hints of degradable NOM detected as total organic carbon (TOC). Further details on these characteristics have previously been reported elsewhere (Valenzuela et al., 2017, 2019).
Microcosms Set-Up
Kinetics of N2O Reduction
An initial evaluation of the capacity of the wetland sediment biota to employ reduced Pahokee Peat humic substances (PPHS, catalog number from the IHSS: 1S103H) as electron donors for N2O reduction was performed. To this end, serum bottles (25 mL) were inoculated with 1 g of previously homogenized wetland sediment, and 15 mL of inorganic basal medium enriched with PPHS at a concentration of 1 g L–1 was employed. The composition of the basal medium used (in g L–1) was as follows (modified from Cervantes et al., 2000): NaHCO3 (5), NH4Cl (0.3), K2HPO4 (0.2), MgCl2 6H2O (0.03), and CaCl2 (0.1). Trace elements were included in the medium by adding 1 mL L–1 of a solution with the following composition (in mg L–1): FeCl2⋅4H2O (2,000), H2BO3 (50), ZnCl2 (50), CuCl2⋅6H2O (90), MnCl2⋅4H2O (500), AlCl3⋅6H2O (90), CoCl2⋅6H2O (2,000), NiCl⋅6H2O (920), Na2SeO⋅5H2O (162), (NH4)6Mo7O24 (500), EDTA (1,000), Na2WO4⋅H2O (100), and 1 mL L–1 HCl at 36%. NaCl (3 g L–1) was added to the medium to match the salinity level detected in the water column at the moment of the sediment sampling (Valenzuela et al., 2017). The final pH of the medium was 7.2 ± 0.05 and it remained constant throughout the incubation period. Controls lacking PPHS were also included, as well as PPHS enriched sterilized controls, which were prepared by autoclaving (three cycles) and subsequent addition of anhydrous chloroform (99%, Sigma-Aldrich) at a concentration of 10% v/v. All microcosms were incubated under anoxic conditions for 2 months with hydrogen (H2) as the electron donor to achieve PPHS reduction (these treatments are referred to as PPHSred). The headspace of all microcosms was flushed with argon (99.9% purity, Praxair) for 10 min, and then H2 was provided to a partial pressure of 0.67 atm with disposable syringes (supply of H2 was done three times during the reduction process). After this incubation period to achieve PPHS reduction, all bottles were thoroughly flushed with Ar for 1 h to remove the remaining H2, which was confirmed by chromatographic measurements, and then the electrons stored as PPHSred were measured by the ferrozine technique (Lovley et al., 1996; Valenzuela et al., 2017).
Controls containing the same concentration of oxidized PPHS (PPHSox) were prepared as follows: bottles pre-incubated as previously described, but containing only sediment, were provided with PPHSox from a concentrated stock prepared by magnetic stirring using the same inorganic medium previously described. Prior to spiking the microcosms with the PPHSox, dissolved oxygen was flushed away from the stock solution by purging with Ar for 1 h. The purpose of preparing these controls in this manner was to attenuate the sediment intrinsic electron acceptors and donors in the same way, and during the same period, as in the main treatments (those including PPHS and provided with H2 for their reduction). To begin the N2O reduction experiment, 4 mL of N2O were spiked to all microcosms, except to those PPHSred bottles serving as endogenous controls (to verify the re-oxidation of PPHSred by intrinsic TEAs remaining). Afterward, the zero-time gaseous measurements were done, and the incubation period was started by placing all bottles in a dark room at 28°C, which was the temperature prevailing in the wetland at the moment of sampling. The incubation was carried out without mechanical shaking. The number of replicates per treatment was three (detailed description concerning this experimental setting is provided in Supplementary Table S1).
A preliminary incubation was conducted with PPHSred and 15N2O to verify the reduction of 15N2O to 15N2, which was confirmed by GC-MS analysis (see Supplementary Figure S1). Therefore, N2O consumption was referred to as N2O reduction in the present study.
Kinetics of Simultaneous N2O and 13CH4 Consumption
One gram of homogenized wetland sediment was inoculated into 25 mL serum bottles containing 15 mL of the anoxic basal medium previously described. Afterward, the headspace of each bottle was flushed with argon gas (Ar) for 10 min. All microcosms were then pre-incubated in a dark room at 28°C for approximately 30 days. The purpose of this initial incubation was to deplete endogenous electron donors and acceptors, such as labile organic molecules, sulfate, nitrate and oxidized metals, which were in fact already subject to attenuation due to the potential microbial activity taking place during the storage period even under the refrigeration temperature (18 months of storage at 4°C from sampling to incubation). After this incubation period, microcosms were taken inside an anoxic chamber (COY 14500; atmosphere composed of N2/H2, 95%/5% v/v) to replace the liquid phase by freshly prepared anoxic basal medium enriched with 500 mg L–1 of PPHS. Control incubations were filled with regular basal medium lacking PPHS. Once the basal medium was replaced, all microcosms were sealed with rubber stoppers and aluminum crimps, taken outside the anaerobic chamber and their atmosphere was flushed with Ar for 10 min. Once these anoxic microcosms were prepared, 2 mL of 13CH4 (99 atom.%, Sigma-Aldrich) and/or 4 mL of N2O (99.9% purity, Sigma-Aldrich) were injected into the bottles’ headspace using plastic disposable syringes. Endogenous controls were left without addition of GHG, while sterile controls were prepared as described above. The number of replicates per treatment was three (detailed description concerning this experimental design is provided in Supplementary Table S2).
The first incubation cycle in which microbial 13CH4 oxidation and N2O reduction was observed lasted 9 days. After this period, microcosms were supplied with new basal medium (including fresh PPHS where appropriate) inside the anaerobic chamber and then flushed with Ar for 10 min. 13CH4 and N2O were spiked again, and a second incubation cycle of 9 days was started.
Analytical Techniques
Sulfate, Sulfide, Nitrate, and Nitrite Measurements
The concentrations of SO42–, dissolved sulfide (HS–), NO3– and NO2– were measured according to standard methodologies previously established (capillary electrophoresis and spectrophotometric detection) (Cord-Ruwisch, 1985; Soga and Ross, 1999; APHA/AWWA/WEF, 2012). A detailed description of these methodologies and their modifications can be found in Rios-Del Toro et al. (2018a).
Isotopic Carbon Dioxide and Nitrous Oxide Determinations
Simultaneous quantification of 13CO2 production from 13CH4 oxidation and N2O consumption was conducted by mass spectrometry (MS) (Agilent Technologies 5977A Series MSD) complemented by 7890B gas chromatograph (GC). Separation was achieved with a HP-PLOT/Q + PT capillary column (30 m × 0.320 mm ID × 0.20 μm) from Agilent Technologies. Helium was used as carrier gas at 0.3 mL min–1. The chromatographic method was as follows: the starting temperature was 70°C, and then a ramp with an increase of 20°C per min was implemented for 3 min. The temperatures of injector and MS source were maintained at 250 and 230°C, respectively. The injection volume was 20 μL and there was only one replicate of injection per analyzed sample. The gas injected into the gas chromatograph was manually taken directly from the headspace of the incubations and immediately injected into the GC port. This chromatographic method was also adequate for the detection of 12CH4, which was also monitored throughout the experiments.
Quantification of Electron-Donating Capacity in Slurry Samples
The reduction of humic material in the form of PPHS or intrinsic NOM was assessed as the amount of ferrous iron produced by the reaction of ferric citrate with slurry taken from the microcosms under anoxic conditions. The ferrous iron released was then measured by the ferrozine technique (Lovley et al., 1996) and corrected for intrinsic ferrous iron detected (Fe2+ measured in samples after acid treatment without addition of ferric citrate) (Stookey, 1970). These measurements were performed in a spectrophotometer located inside the COY 14500 anaerobic chamber previously described. Further details on this methodology have been previously described (Valenzuela et al., 2017).
Molecular Analysis
DNA Extraction
Two replicates for each experimental treatment were sacrificed at the end of the incubation periods for total DNA extraction. Bottles were vigorously shaken and then 500 μL of slurry were taken with sterile disposable syringes to extract DNA using the PowerSoil DNA extraction kit (Mo Bio Laboratories, Carlsbad, CA, United States) according to the protocol described by the manufacturer. The construction of the 16S rRNA genomic libraries was based on DNA samples processed in an independent manner in order to obtain parallel sequencing results for each experimental replicate.
Sequencing and Genomic Libraries Construction
Total DNA isolated from each experimental replicate was amplified using primers targeting the 16S rRNA gene of Bacteria (V3–V4 region, 341F-805R) and Archaea (340F-1000R) (Gantner et al., 2011), both fused with Illumina adapter overhang nucleotide sequences. PCRs for bacterial 16S rRNA region were performed in 25 μL reaction mixtures using Invitrogen HF Platinum Taq Polymerase (Thermo Fisher Scientific, United States) under the following conditions: denaturation at 95°C for 90 s, followed by 30 cycles of amplification at 95°C for 15 s, 57°C for 30 s, 72°C for 30 s, 80°C for 30 s and finished with 95°C for 15 s and 60°C for 10 s. PCRs for archaeal 16S rRNA region were performed under the conditions reported by Gantner et al. (2011). PCR products were indexed using Nextera XT Index Kit v2 (Illumina, San Diego, CA, United States) according to the Illumina’s 16S Metagenomic Sequencing Library Preparation protocol. Libraries were further sequenced by single end with Illumina MiSeq sequencer.
16S rRNA Bioinformatic Analysis
Mothur open source software (v 1.34.4) was used for analysis of 16S rRNA libraries (Kozich et al., 2013). Sequences with a length less than 500 bp, homopolymer runs of eight or more bases, those with more than one mismatch to the sequencing primer and Q-value average below 25 were discarded. The potential occurrence of chimeric sequences was analyzed using UCHIME algorithm. Group membership was determined prior to trimming of the barcode and primer sequence. A distance matrix was calculated across the set of non-redundant sequences and the readings were grouped into operational taxonomic units (OTUs) with a similarity threshold of 97%. Mothur’s Bayesian classifier and the SILVA v.132 reference set were used to taxonomically categorize the sequences using the nearest alignment space termination (NAST) algorithm. Taxonomic assignments were made with a confidence threshold greater than 80% of bootstrap value. The accession numbers of sequences in this work were deposited in the GenBank sequence read archive under the BioProject with PRJNA576687 accession number.
Results
Kinetics of N2O Reduction With PPHSred as Electron Donor
The microbial communities present in the sediments of Sisal wetland have previously been shown to perform AOM linked to PPHS reduction (Valenzuela et al., 2017). However, their capacity to use PPHSred as electron donor for N2O reduction has not been demonstrated. With the purpose of verifying this unexplored process, sediment incubations were conducted including PPHSred and N2O, along with the respective controls (see Supplementary Table S1). In an initial incubation period lasting 12 days, the wetland sediment’s microbiota achieved maximum N2O reducing activities of up to 4.6 ± 0.5 μmol g sed.–1 day–1 [mean ± standard error (SE), n = 3] in microcosms enriched with PPHSred (Figure 1A). The oxidation of 0.8 ± 0.3 milli-equivalents (meq) L–1 (mean ± SE, n = 3) derived from PPHSred occurred in parallel to N2O reduction in this treatment during the whole incubation period (Figure 1A). This PPHSred oxidation activity was calculated by the loss on their EDC through the same incubation period (Figure 1B). Considering quinones/hydroquinones as the main redox groups in humic substances (Scott et al., 1998), the stoichiometry of N2O reduction coupled to hydroquinones oxidation can be considered as follows:
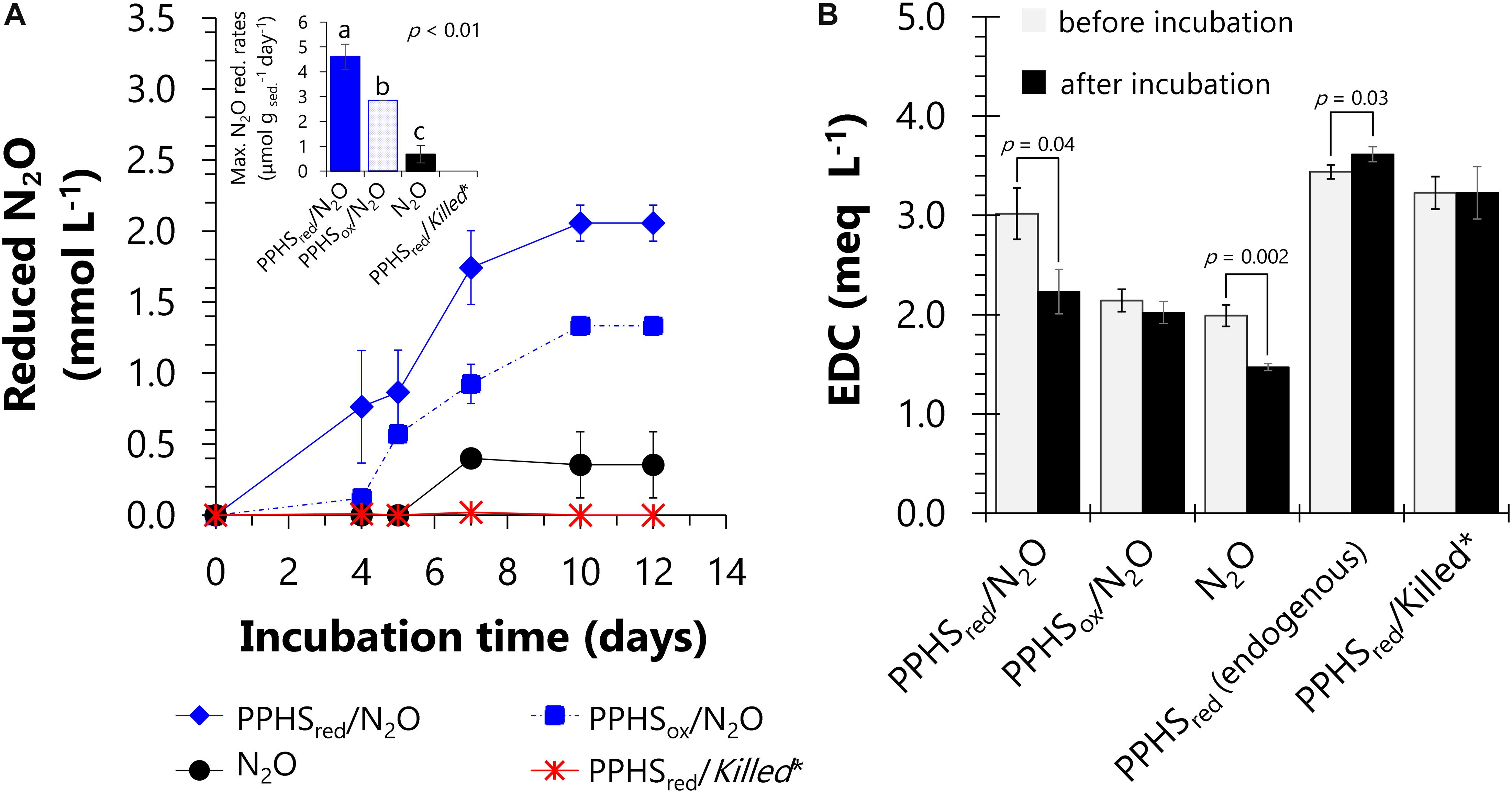
Figure 1. Nitrous oxide reduction linked to re-oxidation of reduced functional groups in reduced Pahokee Peat Humic Substances (PPHSred). Panel (A) depicts the normalized (initial concentration – concentration, Ci–C) kinetics of N2O reduction. The inset shows the maximum rates of N2O reduction based on the linear regressions of at least three sampling points during the period of highest activity. Statistically different groups (rates) are represented with letters obtained via a one-way ANOVA and the Duncan post hoc test (95% percent confidence interval). Panel (B) shows changes on the electron donating capacity (EDC) of PPHS or intrinsic NOM before and after incubation with or without N2O after 12 days of incubation. Significant changes in EDC during incubation time are denoted with p-values (< 0.05), which were evaluated through a Student’s t-test (95% percent confidence interval, degrees of freedom = 4). Data represent the average from triplicate incubations ± standard error. *Killed controls contained the same concentration of N2O as in the main experimental treatments (3.23 ± 0.03 mmol L– 1). Detailed description of the experimental set-up employed in this experiment is shown in Supplementary Table S1.
∗Further details on these thermodynamic calculations are included in Supporting Information (Supplementary Figure S3).
Where QH2-PPHSred refers to reducing equivalents stored as hydroquinones in PPHSred (2 reducing equivalents per hydroquinone moiety) and Q-PPHSox represents quinone equivalents produced as PPHSox during the oxidation of QH2-PPHSred. The ratio of QH2-PPHSred oxidized: N2O reduced obtained at the end of the experiments was approximately 1.08 (corrected for the oxidation of PPHSred quantified in controls lacking N2O and for the N2O reduction measured in controls amended with PPHSox, respectively) (Figure 1 and Table 1), which is in agreement with the expected stoichiometric value (1:1, Eq. 1).
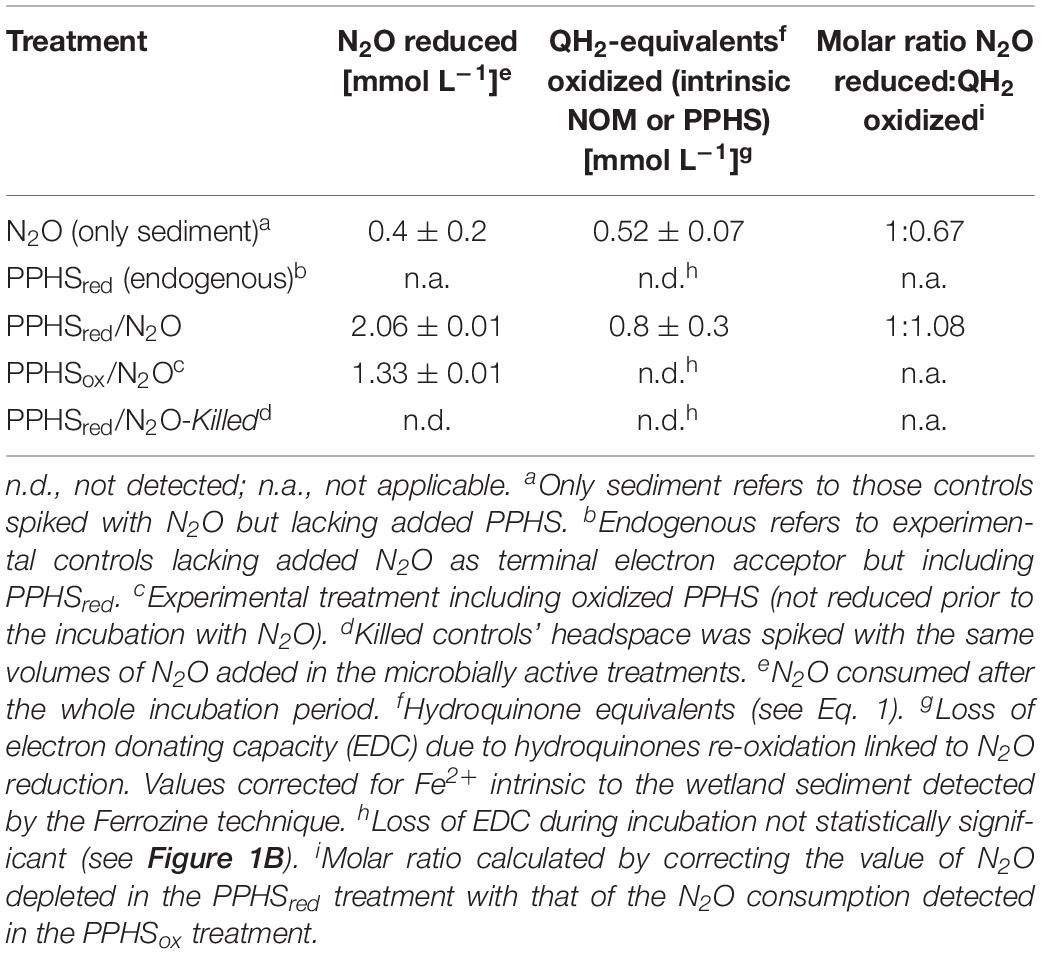
Table 1. Electron balance during the reduction of N2O with reduced Pahokee Peat Humic Substances (PPHSred) as electron donors.
Furthermore, the oxidation of reduced redox groups in the intrinsic NOM present in the wetland sediment (incubated without PPHS), was concomitant to the N2O reduction observed in these controls (the ratio QH2-NOMred oxidized:N2O reduced was 0.67, corrected for the corresponding controls). This confirms the active role of reduced groups in the intrinsic NOM of the wetland sediment to sustain the N2O reducing process (Figure 1 and Table 1). This is in agreement with previous studies showing that the electron accepting capacity (EAC) of the intrinsic NOM in the same sediment partially sustained AOM by serving as TEA (Valenzuela et al., 2017). Namely, redox-active moieties present in intrinsic NOM play an active role in microbial activities related to the consumption of GHG. Additionally, substantial N2O reduction occurred in sediment incubations spiked with PPHSox (2.8 μmol gsed.–1 day–1), which was significantly higher than that measured in the absence of PPHS (0.7 ± 0.3 μmol gsed.–1 day–1) (mean ± SE, n = 3). This suggests that the labile fraction of PPHSox partly fueled N2O reduction. Sediment incubations enriched with PPHSred, but lacking added N2O (endogenous controls) did not show any significant loss on the initial EDC (including both PPHSred and reduced intrinsic NOM), suggesting that N2O was the only TEA fueling microbial PPHSred oxidation (Figure 1B). Sulfate, nitrite and nitrate were not detected during these incubations further confirming that N2O was the most relevant TEA. Abiotic controls (killed microorganisms) including both PPHSred and N2O did not show any activity (Table 1), validating the biological nature of these redox processes.
AOM Linked to N2O Reduction Mediated by Humic Substances
N2O Reduction Rates
After a conditioning pre-incubation cycle, intended to exhaust intrinsic electron donors and acceptors present in the wetland sediment, N2O reduction was documented in two subsequent incubation periods (referred to as 1st and 2nd incubation cycles). During the 1st cycle, high N2O reduction rates were observed only in microcosms enriched with PPHS (0.23 ± 0.03 and 0.15 ± 0.03 mmol N2O gsed.–1 day–1 (mean ± SE, n = 3) in the presence and in the absence of 13CH4, respectively). These treatments displayed nearly complete depletion of the supplied N2O within the first 3 days of incubation (Supplementary Figure S2). However, this activity was mainly fueled by the oxidation of labile organic compounds originally present in the wetland sediment. Thus, the role of PPHS as redox mediator linking AOM to N2O reduction was not conclusive in the 1st cycle (Supplementary Figure S2). Nevertheless, throughout the 2nd incubation cycle (Figure 2), the importance of PPHS fueling the consumption of both GHG was explicitly shown by ∼30% more N2O reduced in the treatment containing 13CH4 as electron donor. The maximum reduction rate observed in this treatment was 25.5 ± 0.5 μmol N2O g sed.–1 day–1 (mean ± SE, n = 3), 15% higher than the rate observed in experimental controls containing PPHS, but lacking 13CH4 (22.1 ± 0.2 μmol N2O g sed.–1 day–1) (mean ± SE, n = 3). Marginal rates of N2O reduction (1.7 ± 0.3 and 1.2 ± 0.6 μmol N2O g sed.–1 day–1) (mean ± SE, n = 3) were observed with and without 13CH4 addition, respectively, in microcosms lacking PPHS, which were in fact very similar to the activity observed in abiotic controls (Figure 2B). Moreover, high N2O reduction was only observed in incubations amended with PPHS or with PPHS/13CH4 (Figure 2A).
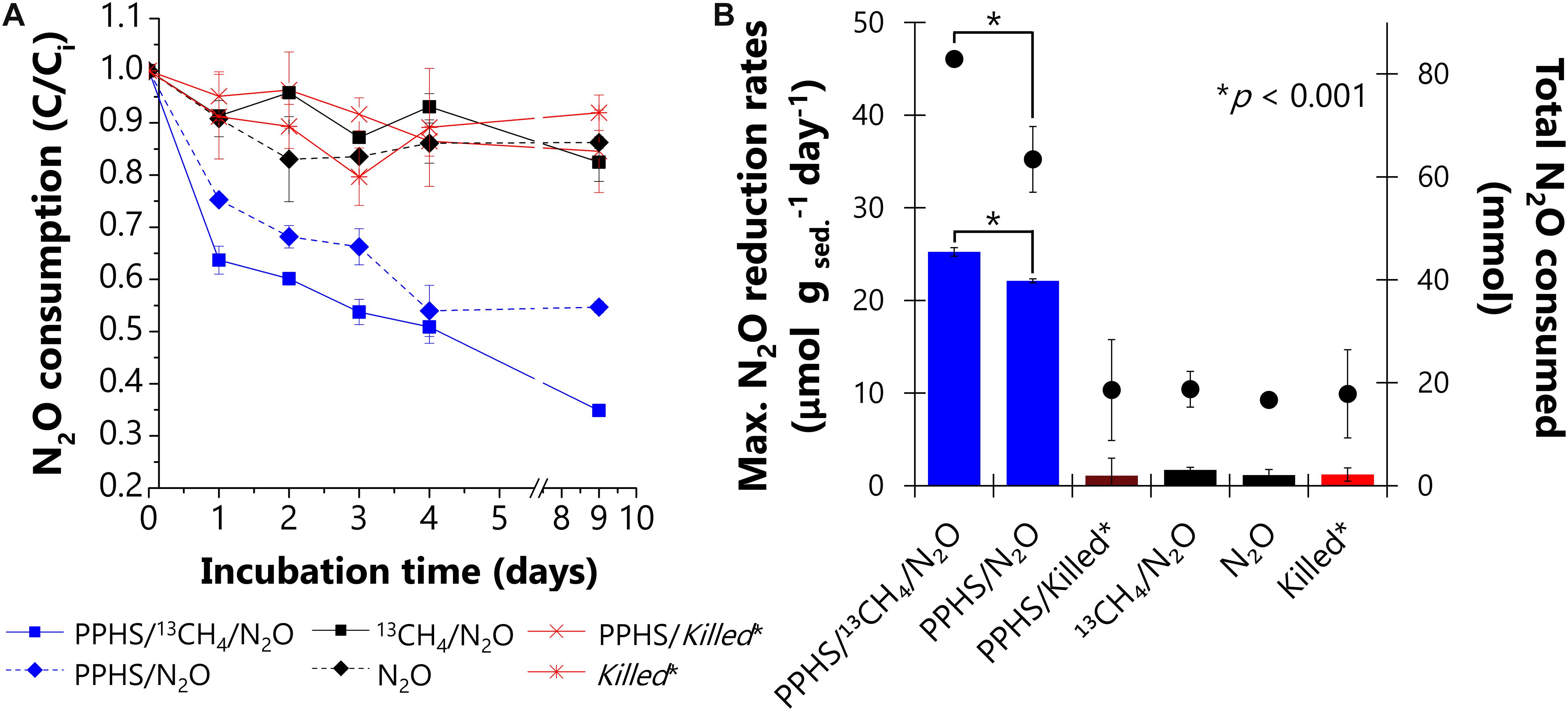
Figure 2. Nitrous oxide reduction promoted by Pahokee Peat Humic Substances (PPHS) acting as electron shuttle and 13CH4 as electron donor during the second cycle of incubation. Panel (A) depicts the normalized (concentration/initial concentration, C/Ci) kinetics of N2O consumption with and without PPHS as electron shuttle. Panel (B) shows the maximum N2O reduction rates (bars, left axis) based on the linear regressions of at least three sampling points during the period of the highest activity. The net amount of N2O depleted after 9 days of incubation is shown in the right axis (⋅ symbols). Data represent the average from triplicate incubations ± standard error. *Killed controls contain the same concentration of 13CH4 (∼4 mmol L– 1) and N2O (6.6 ± 0.4 mmol L– 1) as in the main experimental treatments. Significant differences in the maximum N2O consumption rates and total N2O reduced during incubation time among the treatments containing PPHS are indicated with asterisks denoting p-values (<0.001), which were evaluated through a Student’s t-test (95% percent confidence interval, degrees of freedom = 4).
AOM and Its Coupling With N2O Reduction
Supplementary evidence demonstrating the coupling between AOM and N2O reduction was obtained by quantifying the amount of 13CO2 derived from 13CH4 oxidation in all experimental conditions (Figure 3). During the 2nd incubation cycle, 13CO2 production was only detected in sediment incubations performed with 13CH4/N2O/PPHS (1.3 ± 0.1 μmol 13CO2 g sed.–1 day–1, Figure 3) (mean ± SE, n = 3). Sulfide prevailed at very low concentrations during these incubations (<5 μM), thus its contribution to N2O reduction was negligible. Nevertheless, the labile fraction present in PPHS fueled significant N2O reduction in the treatment N2O/PPHS, which obstructed the accurate assessment of the stoichiometry of the process. At the end of the incubation period, the amount of N2O reduced in the treatment 13CH4/N2O/PPHS was 2.6 ± 0.4 meq L–1 (mean ± SE, n = 3, corrected for the N2O reduction observed in the control treatment, N2O/PPHS) and the produced 13CO2 was equivalent to 6.1 ± 0.6 meq L–1 (mean ± SE, n = 3). Thus, the ratio N2O reduced to 13CO2 produced was 0.43, which means that N2O reduction accounted for 43% of the 13CH4 oxidized (quantified as 13CO2). These calculations were based on the following stoichiometry:
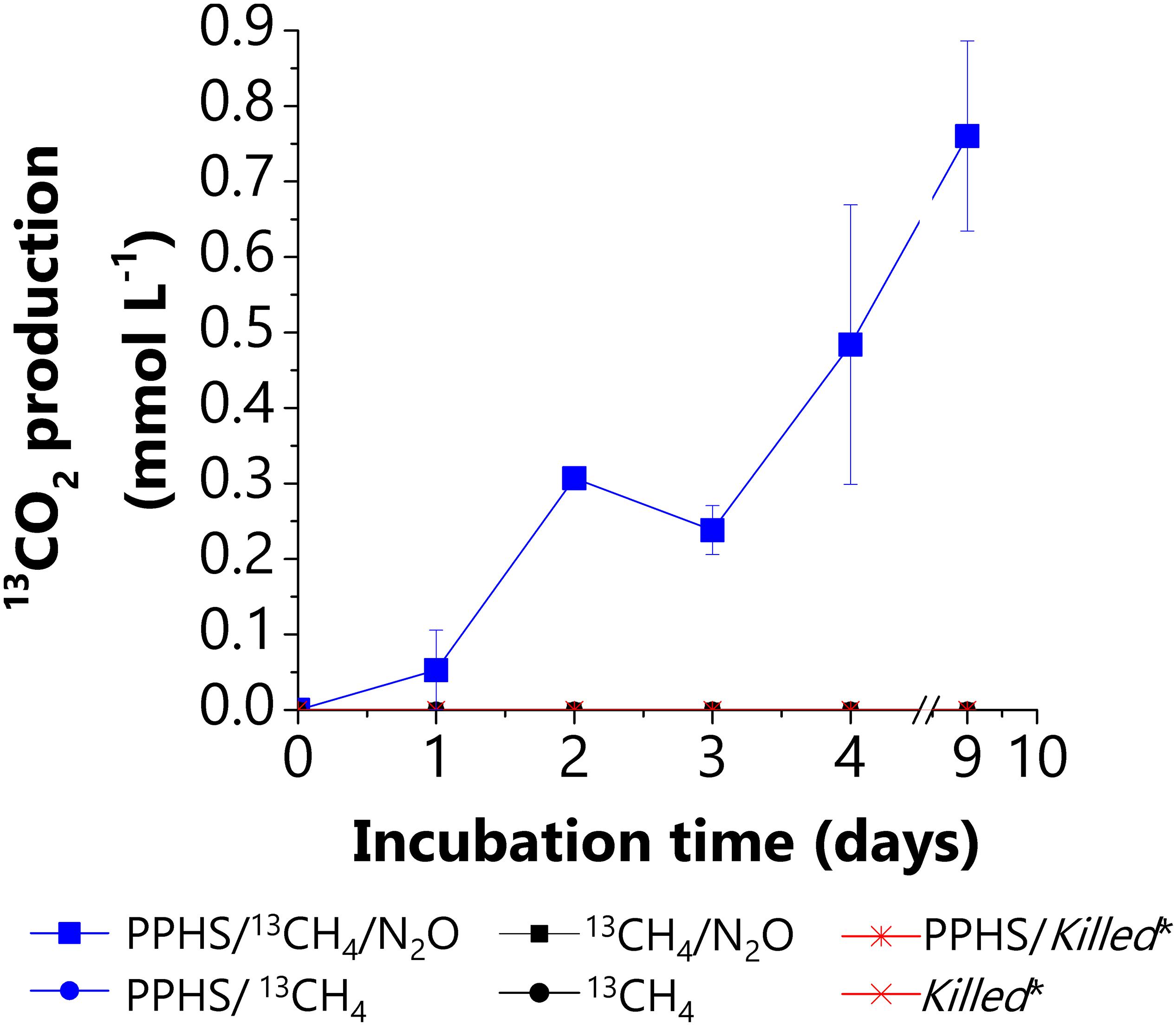
Figure 3. Anaerobic 13CH4 oxidation measured as 13CO2 production from AOM linked to N2O reduction via electron shuttling mediated by PPHS. Data represent the average from triplicate incubations ± standard error.
∗Further details on these thermodynamic calculations are included in Supporting Information (Supplementary Figure S3).
Despite no stoichiometric evidence was collected from these experiments, the parallel occurrence of AOM and N2O reduction prevailing in the 13CH4/N2O/PPHS treatment suggests that PPHS may play an important role on mediating these two microbial processes. No 13CO2 production was observed in experimental treatments lacking PPHS or N2O, further emphasizing the role of humic substances as electron shuttles for coupling AOM to N2O reduction (Figure 3).
PPHS Redox Pattern During AOM Linked to N2O Reduction
The redox state of PPHS was monitored during the observed AOM linked to N2O reduction in the 2nd cycle of incubation (Figure 4). As expected, microcosms containing 13CH4 and PPHS, but lacking N2O, displayed the maximum rates of PPHS reduction (6 ± 0.4 μeq g sed.–1 day–1, Figure 4A) (mean ± SE, n = 3), while endogenous controls incubated in the absence of 13CH4 showed PPHS reduction rates ∼42% lower than the former experimental treatment. These findings confirm the utilization of 13CH4 as electron donor by humus-reducing microorganisms (humus-driven AOM, Eq. 3).
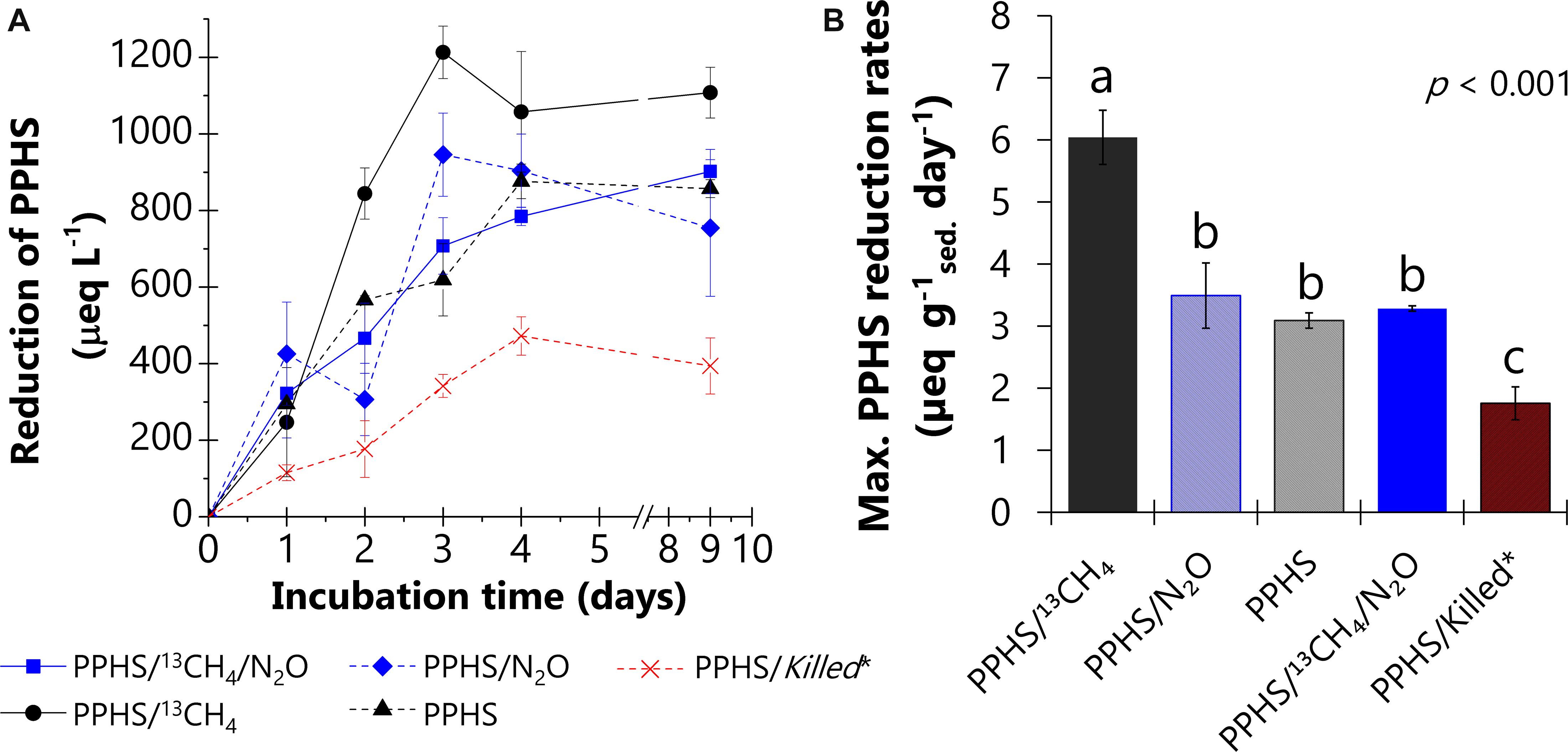
Figure 4. Reduction of Pahokee Peat Humic Substances (PPHS) under the different experimental conditions during the incubation time. PPHS were supplied in the oxidized form at the beginning of the incubation. Panel (A) shows the reduction of PPHS during incubation period. Panel (B) compares the maximum rates of PPHS reduction among the different experimental treatments based on the linear regressions of at least three sampling points during the period of the highest activity. Data represent the average from triplicate incubations ± standard error. *Killed controls contained the same concentrations of 13CH4 (∼4 mmol L– 1) and N2O (6.6 ± 0.4 mmol L– 1) as in the main experimental treatments. Statistically different treatments are represented with letters obtained via a one-way ANOVA and the Duncan post hoc test (95% percent confidence interval).
∗Further details on these thermodynamic calculations are included in Supporting Information (Supplementary Figure S3).
Moreover, microcosms amended with both 13CH4 and N2O in the presence of PPHS displayed the lowest PPHS reduction rates among all experimental treatments (3.28 ± 0.04 μeq g sed.–1 day–1) (mean ± SE, n = 3) (Figure 4B). This confirms that PPHSred produced via AOM were re-oxidized to PPHSox coupled to N2O reduction (Figure 5).
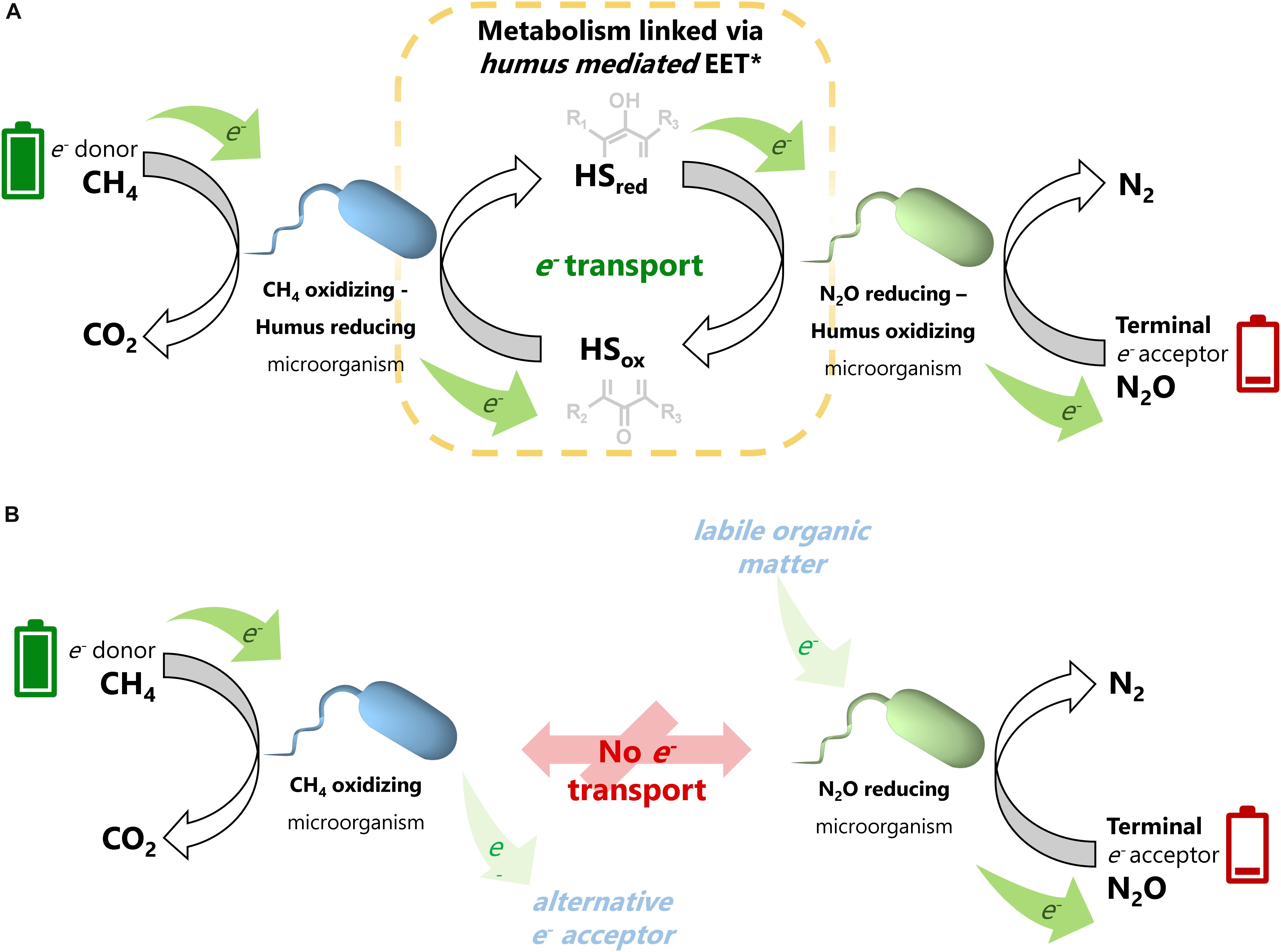
Figure 5. Schematic representation of anaerobic CH4 oxidation linked to N2O reduction mediated by the electron shuttling capacity of humic substances. Panel (A) illustrates the extracellular electron transfer process promoted by humic substances, which links the metabolic capabilities of anaerobic methane oxidizing and nitrous oxide reducing microbes. Full (green) batteries are a representation of the high content of reducing equivalents in CH4, which are taken by anaerobic methane oxidizing-humus reducing microorganisms and then taken from the reduced redox-active moieties (hydroquinones) by humus oxidizing-nitrous oxide-reducing microbes to reduce N2O, represented by an energy depleted (red) battery, into inert N2. In the absence of humic substances (B), each process consuming CH4 and N2O could be independently fueled by an alternative electron donor or electron acceptor present at the wetland sediments (displayed in attenuated colors).
Microbial Communities Potentially Involved in AOM Linked to N2O Reduction
Bacterial Taxa
According to 16S rRNA gene sequences performed at end of the incubation cycles, some bacterial groups considerably changed their relative abundance within the whole bacterial community under the selective conditions established in each experimental treatment (Figure 6). The most noticeable feature in the bacterial community in sediment incubations in which PPHS mediated AOM linked to N2O reduction was the increased relative abundance of a member of the Moraxellaceae family, identified as an Acinetobacter phylotype (accounting for 43% of the bacterial community, Figure 6A). The most predominant taxon observed in the remaining experimental treatments belongs to ɣ-Proteobacteria. However, the percentages of this member of Pseudomonas within each respective microbial community did not show any relationship with the presence of PPHS, CH4 or N2O during the experiments (Figure 6A).
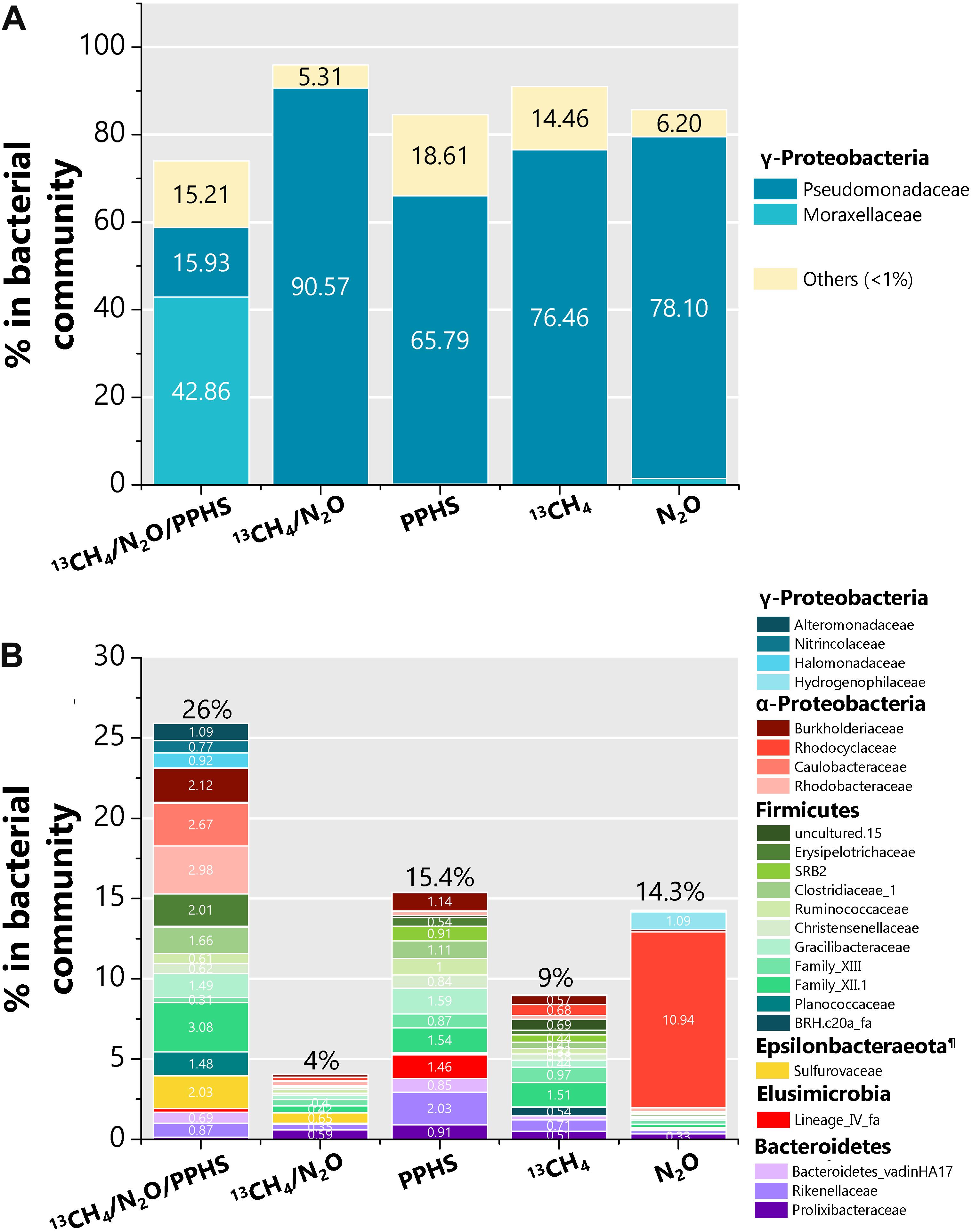
Figure 6. Composition of the bacterial communities found in selected experimental treatments at the end of the incubation period. Panel (A) displays those microbial taxa, which predominated the bacterial communities at the family level (>15% of abundance) as well as the summarized fraction of all families, which percentage in the whole bacterial community was lower than 1%. Panel (B) displays all bacterial families whose percentage in the bacterial community was between 1 and 15%. All data represent the average from two or three (13CH4/N2O treatment) genomic libraries sequenced. Each 16S rRNA library was generated from an independent DNA sample extracted from one biological replicate. ¶ The Epsilonbacteraeota were formerly known as the ε class of the Proteobacteria phylum.
Additional bacterial groups, which relative percentage was noticeably prominent in the 13CH4/N2O/PPHS experimental treatment respect to the controls (Figure 6B) belonged to the families Alteromonadaceae (ɣ-Proteobacteria), Burkholderiaceae, and Caulobacteraceae from the α-Proteobacteria, Erysipelotrichaceae and the family XII from the Firmicutes, as well as Sulfurovaceae from the Epsilonbacteraeota phylum (formerly ε-Proteobacteria).
Archaeal Taxa
Sequencing of archaeal 16S rRNA genomic libraries displayed high percentages of two families of the Euryarchaeota phylum in the 13CH4/N2O/PPHS treatment with respect to the experimental controls (Figure 7). Methanocellaceae and Methanomicrobiaceae families [99.2% and 94.3% dominated by the Rice Cluster I (RC-I) genera and an uncultured phylotype, respectively (Figure 7B)] were highly abundant as compared to the experimental control conditions. In the case of Methanocellaceae family, its abundance was remarkably higher (12.7%) than that found in the controls (0.06 to 1.8%), while Methanomicrobiaceae family approximately doubled its proportion with respect to the controls (22.3% vs. 7.3 to 12.4%, Figure 7A). Furthermore, important members of the archaeal community in all treatments include the Bathyarcheota phylum (∼20%), Marine Benthic Group D and DHVEG family (6–27%) as previously reported for this wetland sediment (Valenzuela et al., 2017). Previously reported AOM-performing archaea were detected at very low percentages in the archaeal communities of the wetland sediment. For instance, ANME-1 was only detected in the 13CH4/N2O/PPHS treatment in a 0.07% proportion of the archaeal community, while the abundance of ANME-3 ranged 0.05 to 0.11% in all experimental treatments. Moreover, Candidatus Methanoperedens nitroreducens, which has been related to AOM under denitrifying conditions (Haroon et al., 2013) was not detected.
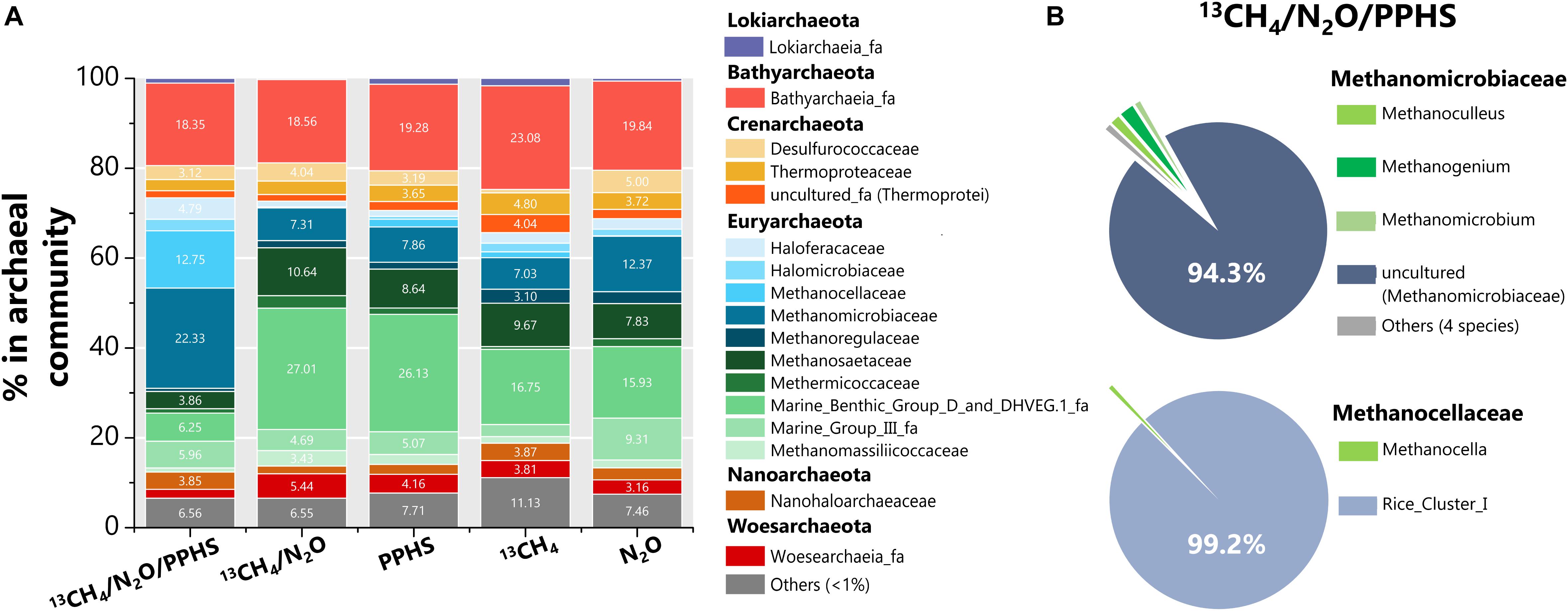
Figure 7. Composition of the archaeal communities found in selected experimental treatments at the end of the incubation period. Panel (A) shows the distribution of the whole archaeal sequences obtained through archaeal 16S rRNA ILLUMINA sequencing at family level amongst the experimental treatments. Panel (B) displays the analysis of Methanomicrobiaceae and Methanocellaceae in the 13CH4/N2O/PPHS treatment at the genus level. All data represent the average from the sequencing of two 16S rRNA libraries. Each library came from an independent DNA sample extracted from an experimental replicate.
Discussion
The purpose of the present study was to evaluate the capacity of humic substances to mediate AOM coupled to the reduction of N2O by the microbiota present in a wetland sediment. The present study provides additional evidence of the important contribution of humic substances to suppress the emission of GHG in wetland sediments (Blodau and Deppe, 2012; Valenzuela et al., 2017, 2019). AOM linked to N2O reduction is a thermodynamically feasible reaction (see Eq. 2). Nevertheless, poor solubility of CH4 [∼19.7 mg CH4 L–1 @ 1 atm and 28°C (Payne, 2017)] in aquatic environments may be an important regulator of the process in natural ecosystems (Serra et al., 2006). The redox mediating capacity of humic substances may help to overcome these limitations by storing reducing equivalents derived from AOM and then channeling them toward the reduction of N2O. This is partly supported by the fact that both soluble and particulate NOM are effective redox mediators fueling several abiotic and microbial processes (Roden et al., 2010; Martinez et al., 2013).
Our results suggested that intrinsic NOM present in the studied wetland sediment promoted AOM linked to N2O reduction. First, there was significant N2O reduction coupled to the loss of the EDC of the NOM present in the wetland sediment when incubations were performed with N2O lacking PPHS and 13CH4 (Figure 1 and Table 1), pointing to the active role of reduced redox groups (e.g., hydroquinones) in the sediment’s NOM sustaining N2O reduction. Additionally, during the 1st cycle of simultaneous AOM and N2O reduction, the treatment lacking PPHS, but including both 13CH4 and N2O, showed higher N2O reduction than that observed in the treatment lacking PPHS and 13CH4 (Supplementary Figure S2). Although this evidence was not stoichiometric probably due to labile organic matter fueling N2O reduction, these initial findings suggest that intrinsic NOM might have played a role in AOM linked to N2O reduction. The role of humic substances in the proposed mechanism was further emphasized in incubations conducted with 13CH4/N2O/PPHS, which showed parallel AOM and N2O reduction (Figures 2, 3). Altogether, these results suggest the theoretical feasibility for this process to occur in nature, which according to our calculations is possible for the whole range of redox potentials (Eo’) proposed for humic substances (−300 to +300 mV, Eq. 1) (Straub et al., 2000; Aeschbacher et al., 2010). Nevertheless, further studies are required to verify if the process prevails under natural conditions since the concentration of 13CH4 and N2O used in our incubations are much higher than those usually occurring in aquatic environments. Moreover, our incubations started several months after the sampling time, thus the inoculum might have not reflected the actual microbial community of the studied wetland sediment. Despite these limitations, our study suggests that humic substances could mediate AOM linked to N2O reduction in wetland sediments, which constitutes a new pathway interconnecting the C and N cycles in these ecosystems. Humic substances have previously been reported to link the C and N biogeochemical cycles by anaerobic ammonium oxidation coupled to microbial reduction of NOM in marine sediments (Rios-Del Toro et al., 2018b).
The current knowledge on anaerobic methanotrophic microorganisms that are able to link the C and the N cycles through AOM driven by oxidized N compounds, describes the mandatory utilization of NO3– (by Candidatus Methanoperedens nitroreducens, an ANME-2d affiliated archaeon) or NO2– (by Candidatus Methylomirabilis oxyfera, a bacterium) as TEA (Ettwig et al., 2010; Zhu et al., 2012; Haroon et al., 2013). Nevertheless, there are no kinetic or genetic studies showing that these microbes could use N2O as TEA, either by themselves or by a syntrophic relationship with another microbial partner to oxidize CH4.
A previous study demonstrating the simultaneous consumption of CH4 and N2O in an artificial wetland proved that this coupled process stimulated the activity of aerobic methanotrophs (Cheng et al., 2019). However, no parallel stimulation of nosZ enzymatic activity could be identified; consequently, the mechanisms for the coupled reaction remained unknown. In the present work, we provide several clues indicating that humic substances can mediate the coupling between the methanotrophs and N2O reducers, which would expand our understanding of the processes driven by the microbial communities in wetland sediments (Figure 5).
Microbial Communities Potentially Involved
The bacterial taxa whose relative abundance increased in the treatment displaying simultaneous 13CH4 and N2O consumption mediated by PPHS, agreed with previously reported denitrifying and non-denitrifying N2O reducing bacteria, which have been described in distinct ecosystems at the genus taxonomical level (Figure 6B) (Conthe et al., 2018; Hallin et al., 2018). Likewise, one bacterial taxum, which stood out due to its important relative abundance within the 16S libraries under the 13CH4/N2O/PPHS conditions was a ɣ-proteobacterial phylotype from the Acinetobacter genus (Figure 6A). Previous studies, have shown the involvement of Acinetobacter species in the N cycle due to their capacity to accomplish heterotrophic nitrification-aerobic denitrification (Chen et al., 2019; Wen et al., 2019), whilst other species have been reported as heterotrophic and autotrophic denitrifiers (Lee et al., 2013; Pishgar et al., 2019). Two works reported by Su et al. (2015, 2016), showed that Acinetobacter sp. strain SZ28 was able to accomplish NO3– and N2O reduction coupled to Mn2+, Fe2+, and S2– oxidation. These authors also documented the capacity of strain SZ28 to employ several organic compounds, including humic substances, as an energy source. Thus, based on the body of evidence demonstrating the respiratory and metabolic versatility of Acinetobacter species, we hypothesize that some species of this microbial taxon could have been potentially involved in N2O reduction using reduced redox groups in PPHSred as electron donor. Nevertheless, we cannot dismiss the potential involvement of other N2O reducers in the process since the diversity of microbes possessing this feature is as wide as the diversity of humus-oxidizing microorganisms (Lovley et al., 1999; Martinez et al., 2013); however, future research must be done in order isolate and characterize the microorganisms conducting this process.
From the archaeal counterpart, only two taxa showed considerable increase within the microbial community in the complete treatment including 13CH4/N2O/PPHS: an uncultured genus of the Methanomicrobiaceae family, and the RC-I genus from the Methanocellaceae (Figures 7A,B). By using H2, formate and CO2 as substrates for methanogenesis (Hunger et al., 2011), the RC-I cluster has been proposed as the most important archaea controlling CH4 emissions from paddy soils (Großkopf et al., 1998; Erkel et al., 2006). Despite this, no methanogenic activity was detected in our incubations under any of the experimental conditions tested. Archaeal members of the RC-I cluster have previously been detected in wetland and marsh ecosystems (Lin et al., 2017; Xiao et al., 2017), as well as in rice paddies. These organotrophic systems contain high amounts of endlessly decomposing NOM, which prevail under anoxic conditions due to flooding, thus creating the proper niche for microbes to perform the redox reactions involved in AOM coupled to N2O reduction, mediated by humic substances (Figure 5). Although to the best of our knowledge, the RC-I archaeal group has not been reported to perform either AOM or humus-reducing activities, Bao et al. (2016) showed that NO3– addition promoted a positive response in RC-I archaea in terms of the expression the mcrA gene (a molecular marker of methanogenesis and/or methanotrophy). This effect was also linked to diminished CH4 production and given the evidence provided in the present study, a possible connection involving the RC-I type of archaea between denitrification and methanotrophy must be addressed in future studies.
Regarding the uncultured Methanomicrobiaceae phylotype, which comprised ∼20% of the total archaeal community, in addition to the numerous reports on its role as CH4 producer (Kröber et al., 2009), a recent study showed that the Methanobacterium genera, which also belongs to the Methanomicrobiaceae family, drove AOM coupled to ferrihydrite reduction with humic substances as electron shuttle (He et al., 2019). These authors demonstrated how this microorganism oxidized CH4 to propionate and proposed that this intermediate was then taken by a bacterial partner (potentially a Desulfovibrio species) to produce siderite (a ferrous iron carbonate). Previous reports have described the syntrophic activity of anaerobic methanotrophic archaea in partnership with bacteria to reduce TEAs, such as SO42– (Boetius et al., 2000). Here, we propose a novel syntrophic process mediated by humic substances in which the RC-I cluster and/or an uncultured member of the Methanomicrobiaceae family could have coupled metabolic capabilities with a bacterial member, such as Acinetobacter, to perform AOM linked to N2O reduction via an EET mechanism mediated by humic substances (Figure 5).
Conclusion
The present study showed several lines of evidence indicating that humic substances mediate an EET process in which AOM is linked to N2O reduction in microcosms derived from coastal wetland sediments. These results further emphasize the relevant role that humic substances could play to prevent the emission of GHG from organotrophic environments and provide insights into the potential of their redox active groups as a metabolic linking agent for connecting the C and N cycles. However, further studies are needed to verify if this process prevails under natural conditions.
Data Availability Statement
The accession numbers of the sequences in this work (SAMN13002101 to SAMN13002126) were deposited in the GenBank Sequence Read Archive under BioProject number PRJNA576687.
Author Contributions
EV and FC conceived the research, designed the experimental set-up, and wrote the manuscript with input from all the authors. CP-L and EV performed the experiments. EV, SC-F, and NL-L coordinated the molecular biology research, which was technically performed by EV, NG-H, and CP-L. NL-L performed the bioinformatic analyses and interpreted the results along with EV.
Funding
This research was financially supported by the National Council of Science and Technology of Mexico (CONACYT, Program Frontiers in Science, Grant 1289 to FC) and by Universidad Nacional Autónoma de México (UNAM, project 9407).
Conflict of Interest
The authors declare that the research was conducted in the absence of any commercial or financial relationships that could be construed as a potential conflict of interest.
Acknowledgments
The authors thank Sonia Arriaga, César Nieto-Delgado, and Fred Thalasso for insightful comments and discussions on the manuscript. The authors also thank to Alejandra Prieto-Davó for sediment sampling, as well as Ma. Carmen Rocha-Medina, Mario Delgado, Elizabeth Cortez-Cedillo, and Juan Pablo Rodas for their technical support. The use of infrastructure at the National laboratory, LANBAMA, is greatly acknowledged, particularly for the technical assistance of Guadalupe Ortega-Salazar and Verónica Zárate-Chávez.
Supplementary Material
The Supplementary Material for this article can be found online at: https://www.frontiersin.org/articles/10.3389/fmicb.2020.00587/full#supplementary-material
References
Aeschbacher, M., Sander, M., and Schwarzenbach, R. P. (2010). Novel electrochemical approach to assess the redox properties of humic substances. Environ. Sci. Technol. 44, 87–93. doi: 10.1021/es902627p
APHA/AWWA/WEF (2012). Standard Methods for the Examination of Water and Wastewater. Washington, DC: America Public Health Association.
Aranda-Tamaura, C., Estrada-Alvarado, M. I., Texier, A. C., Cuervo, F., Gómez, J., and Cervantes, F. J. (2007). Effects of different quinoid redox mediators on the removal of sulphide and nitrate via denitrification. Chemosphere 69, 1722–1727. doi: 10.1016/j.chemosphere.2007.06.004
Babbin, A. R., Bianchi, D., Jayakumar, A., and Ward, B. B. (2015). Rapid nitrous oxide cycling in the suboxic ocean. Science 348, 1127–1129. doi: 10.1126/science.aaa8380
Bai, Y.-N., Wang, X.-N., Wu, J., Lu, Y.-Z., Fu, L., Zhang, F., et al. (2019). Humic substances as electron acceptors for anaerobic oxidation of methane driven by ANME-2d. Water Res. 164:114935. doi: 10.1016/j.watres.2019.114935
Bao, Q., Huang, Y., Wang, F., Nie, S., Nicol, G. W., Yao, H., et al. (2016). Effect of nitrogen fertilizer and/or rice straw amendment on methanogenic archaeal communities and methane production from a rice paddy soil. Appl. Microbiol. Biotechnol. 100, 5989–5998. doi: 10.1007/s00253-016-7377-z
Batllori-Sampedro, E., Febles-Patron, J. L., and Diaz-Sosa, J. (1999). Landscape change in Yucatan’s northwest coastal wetlands (1984-1991). Hum. Ecol. Rev. 6, 8–20.
Bhattarai, S., Cassarini, C., and Lens, P. N. L. (2019). Physiology and distribution of archaeal methanotrophs that couple anaerobic oxidation of methane with sulfate reduction. Microbiol. Mol. Biol. Rev. 83:e00074-18. doi: 10.1128/MMBR.00074-18
Blodau, C., and Deppe, M. (2012). Humic acid addition lowers methane release in peats of the Mer Bleue bog. Canada. Soil Biol. Biochem. 52, 96–98. doi: 10.1016/j.soilbio.2012.04.023
Boetius, A., Ravenschlag, K., Schubert, C. J., Rickert, D., Widdel, F., Gieseke, A., et al. (2000). A marine microbial consortium apparently mediating anaerobic oxidation of methane. Nature 407, 623–626. doi: 10.1038/35036572
Bridgham, S. D., Cadillo-Quiroz, H., Keller, J. K., and Zhuang, Q. (2013). Methane emissions from wetlands: biogeochemical, microbial, and modeling perspectives from local to global scales. Glob. Chang. Biol. 19, 1325–1346. doi: 10.1111/gcb.12131
Cervantes, F. J., Velde, S., Lettinga, G., and Field, J. A. (2000). Competition between methanogenesis and quinone respiration for ecologically important substrates in anaerobic consortia. FEMS Microbiol. Ecol. 34, 161–171. doi: 10.1111/j.1574-6941.2000.tb00766.x
Chen, S., He, S., Wu, C., and Du, D. (2019). Characteristics of heterotrophic nitrification and aerobic denitrification bacterium Acinetobacter sp. T1 and its application for pig farm wastewater treatment. J. Biosci. Bioeng. 127, 201–205. doi: 10.1016/j.jbiosc.2018.07.025
Cheng, C., Shen, X., Xie, H., Hu, Z., Pavlostathis, S. G., and Zhang, J. (2019). Coupled methane and nitrous oxide biotransformation in freshwater wetland sediment microcosms. Sci. Total Environ. 648, 916–922. doi: 10.1016/j.scitotenv.2018.08.185
Conthe, M., Wittorf, L., Kuenen, J. G., Kleerebezem, R., Van Loosdrecht, M. C. M., and Hallin, S. (2018). Life on N2O: deciphering the ecophysiology of N2O respiring bacterial communities in a continuous culture. ISME J. 12, 1142–1153. doi: 10.1038/s41396-018-0063-67
Cord-Ruwisch, R. (1985). A quick method for the determination of dissolved and precipitated sulfides in cultures of sulfate-reducing bacteria. J. Microbiol. Methods 4, 33–36. doi: 10.1016/0167-7012(85)90005-90003
Erkel, C., Kube, M., Reinhardt, R., and Liesack, W. (2006). Genome of rice cluster i archaea—the key methane producers in the rice rhizosphere. Science 313, 370–372. doi: 10.1126/science.1127062
Ettwig, K. F., Butler, M. K., Le Paslier, D., Pelletier, E., Mangenot, S., Kuypers, M. M. M., et al. (2010). Nitrite-driven anaerobic methane oxidation by oxygenic bacteria. Nature 464, 543–548. doi: 10.1038/nature08883
Gantner, S., Andersson, A. F., Alonso-Sáez, L., and Bertilsson, S. (2011). Novel primers for 16S rRNA-based archaeal community analyses in environmental samples. J. Microbiol. Methods 84, 12–18. doi: 10.1016/j.mimet.2010.10.001
Gougoulias, C., Clark, J. M., and Shaw, L. J. (2014). The role of soil microbes in the global carbon cycle: tracking the below-ground microbial processing of plant-derived carbon for manipulating carbon dynamics in agricultural systems. J. Sci. Food Agric. 94, 2362–2371. doi: 10.1002/jsfa.6577
Großkopf, R., Stubner, S., and Liesack, W. (1998). Novel euryarchaeotal lineages detected on rice roots and in the anoxic bulk soil of flooded rice microcosms. Appl. Environ. Microbiol. 64, 4983–4989. doi: 10.1128/aem.64.12.4983-4989.1998
Gupta, V., Smemo, K. A., Yavitt, J. B., Fowle, D., Branfireun, B., and Basiliko, N. (2013). Stable isotopes reveal widespread anaerobic methane oxidation across latitude and peatland type. Environ. Sci. Technol. 47, 8273–8279. doi: 10.1021/es400484t
Hallin, S., Philippot, L., Sanford, R. A., and Jones, C. M. (2018). Genomics and ecology of novel N2O-Reducing microorganisms. Trends Microbiol. 26, 43–55. doi: 10.1016/j.tim.2017.07.003
Haroon, M. F., Hu, S., Shi, Y., Imelfort, M., Keller, J., Hugenholtz, P., et al. (2013). Anaerobic oxidation of methane coupled to nitrate reduction in a novel archaeal lineage. Nature 500, 567–570. doi: 10.1038/nature12375
He, Q., Yu, L., Li, J., He, D., Cai, X., and Zhou, S. (2019). Electron shuttles enhance anaerobic oxidation of methane coupled to iron(III) reduction. Sci. Total Environ. 688, 664–672. doi: 10.1016/j.scitotenv.2019.06.299
He, Z., Zhang, Q., Feng, Y., Luo, H., Pan, X., and Michael, G. (2018). Microbiological and environmental significance of metal-dependent anaerobic oxidation of methane. Sci. Total Environ. 61, 759–768. doi: 10.1016/j.scitotenv.2017.08.140
Hunger, S., Schmidt, O., Hilgarth, M., Horn, M. A., Kolb, S., Conrad, R., et al. (2011). Competing formate- and carbon dioxide-utilizing prokaryotes in an anoxic methane-emitting fen soil. Appl. Environ. Microbiol. 77, 3773–3785. doi: 10.1128/AEM.00282-211
Ji, Q., Babbin, R. A., Jayakumar, A., Olenyk, S., and Ward, B. B. (2015). Nitrous oxide production by nitrification and denitrification in the Eastern Tropical South Pacific oxygen minimum zone. Geophys. Res. Lett. 42, 10755–10764. doi: 10.1002/2015GL066853
Kozich, J. J., Westcott, S. L., Baxter, N. T., Highlander, S. K., and Schloss, P. D. (2013). Development of a dual-index sequencing strategy and curation pipeline for analyzing amplicon sequence data on the miseq illumina sequencing platform. Appl. Environ. Microbiol 79, 5112–5120. doi: 10.1128/AEM.01043-1013
Kröber, M., Bekel, T., Diaz, N. N., Goesmann, A., Jaenicke, S., Krause, L., et al. (2009). Phylogenetic characterization of a biogas plant microbial community integrating clone library 16S-rDNA sequences and metagenome sequence data obtained by 454-pyrosequencing. J. Biotechnol. 142, 38–49. doi: 10.1016/j.jbiotec.2009.02.010
Kumar, P., Adelodun, A. A., Khan, M. F., Krisnawati, H., and Garcia-Menendez, F. (2020). Towards an improved understanding of greenhouse gas emissions and fluxes in tropical peatlands of Southeast Asia. Sustain. Cities. Soc. 53:101881. doi: 10.1016/j.scs.2019.101881
Laruelle, G. G., Lauerwald, R., Pfeil, B., and Regnier, P. (2014). Regionalized global budget of the CO2 exchange at the air-water interface in continental shelf seas. Global Biogeochem. Cycles 28, 1199–1214. doi: 10.1002/2014GB004832
Lee, D. J., Pan, X., Wang, A., and Ho, K. L. (2013). Facultative autotrophic denitrifiers in denitrifying sulfide removal granules. Bioresour. Technol. 132, 356–360. doi: 10.1016/j.biortech.2012.10.105
Lehmann, J., and Kleber, M. (2015). The contentious nature of soil organic matter. Nature 528, 60–68. doi: 10.1038/nature16069
Lever, M. A. (2016). A new era of methanogenesis research. Trends Microbiol. 24, 84–86. doi: 10.1016/j.tim.2015.12.005
Lin, Y., Liu, D., Yuan, J., Ye, G., and Ding, W. (2017). Methanogenic community was stable in two contrasting freshwater marshes exposed to elevated atmospheric CO2. Front. Microbiol. 8:932. doi: 10.3389/fmicb.2017.00932
Liu, F., Rotaru, A. E., Shrestha, P. M., Malvankar, N. S., Nevin, K. P., and Lovley, D. R. (2012). Promoting direct interspecies electron transfer with activated carbon. Energy Environ. Sci. 5, 8982–8989. doi: 10.1039/c2ee22459c
Lovley, D. R. (2017). Happy together: microbial communities that hook up to swap electrons. ISME J. 11, 327–336. doi: 10.1038/ismej.2016.136
Lovley, D. R., Coates, J. D., Blunt-Harris, E. L., Phillips, E. J. P., and Woodward, J. C. (1996). Humic substances as electron acceptors for microbial respiration. Nature 382, 445–448. doi: 10.1038/382445a0
Lovley, D. R., Fraga, J. L., Coates, J. D., and Blunt-Harris, E. L. (1999). Humics as an electron donor for anaerobic respiration. Environ. Microbiol. 1, 89–98. doi: 10.1046/j.1462-2920.1999.00009.x
Martinez, C. M., Alvarez, L. H., Celis, L. B., and Cervantes, F. J. (2013). Humus-reducing microorganisms and their valuable contribution in environmental processes. Appl. Microbiol. Biotechnol. 97, 10293–10308. doi: 10.1007/s00253-013-5350-5357
Murray, R. H., Erler, D. V., and Eyre, B. D. (2015). Nitrous oxide fluxes in estuarine environments: response to global change. Glob. Change. Biol. 21, 3219–3245. doi: 10.1111/gcb.12923
Pajares, S., and Ramos, R. (2019). Processes and microorganisms involved in the marine nitrogen cycle: knowledge and gaps. Front. Mar. Sci. 6:739. doi: 10.3389/fmars.2019.00739
Payne, B. (2017). Henry’s Law and Monitoring Methane in Groundwater Wells, 1st Edn. Amsterdam: Elsevier Inc.
Pishgar, R., Dominic, J. A., Sheng, Z., and Tay, J. H. (2019). Denitrification performance and microbial versatility in response to different selection pressures. Bioresour. Technol. 281, 72–83. doi: 10.1016/j.biortech.2019.02.061
Rios-Del Toro, E. E., Valenzuela, E. I., López-Lozano, N. E., Cortés-Martínez, M. G., Sánchez-Rodríguez, M. A., Calvario-Martínez, O., et al. (2018a). Anaerobic ammonium oxidation linked to sulfate and ferric iron reduction fuels nitrogen loss in marine sediments. Biodegradation 29, 429–442. doi: 10.1007/s10532-018-9839-9838
Rios-Del Toro, E. E., Valenzuela, E. I., Ramírez, J. E., López-Lozano, N. E., and Cervantes, F. J. (2018b). Anaerobic ammonium oxidation linked to microbial reduction of natural organic matter in marine sediments. Environ. Sci. Technol. Lett. 5, 571–577. doi: 10.1021/acs.estlett.8b00330
Roden, E. E., Kappler, A., Bauer, I., Jiang, J., Paul, A., Stoesser, R., et al. (2010). Extracellular electron transfer through microbial reduction of humic substances. Nat. Geosci. 3, 417–421. doi: 10.1038/NGEO870
Rotaru, A.-E., Calabrese, F., Stryhanyuk, H., Musat, F., Shrestha, P. M., Weber, H. S., et al. (2018). Conductive particles enable syntrophic acetate oxidation between Geobacter and Methanosarcina from coastal sediments. mBio 9:e0226-218. doi: 10.1128/mBio.00226-218
Scheller, S., Yu, H., Chadwick, G. L., McGlynn, S. E., and Orphan, V. J. (2016). Artificial electron acceptors decouple archaeal methane oxidation from sulfate reduction. Science 351, 703–707. doi: 10.1126/science.aad7154
Scott, D. T., Mcknight, D. M., Blunt-Harris, E. L., Kolesar, S. E., and Lovley, D. R. (1998). Quinone moieties act as electron acceptors in the reduction of humic substances by humics-reducing microorganisms. Environ. Sci. Technol. 32, 2984–2989. doi: 10.1021/es980272q
Segarra, K. E. A., Schubotz, F., Samarkin, V., Yoshinaga, M. Y., Hinrichs, K.-U., and Joye, S. B. (2015). High rates of anaerobic methane oxidation in freshwater wetlands reduce potential atmospheric methane emissions. Nat. Commun. 6:7477. doi: 10.1038/ncomms8477
Serra, M. C. C., Pessoa, F. L. P., and Palavra, A. M. F. (2006). Solubility of methane in water and in a medium for the cultivation of methanotrophs bacteria. J. Chem. Thermodyn. 38, 1629–1633. doi: 10.1016/j.jct.2006.03.019
Smemo, K. A., and Yavitt, J. B. (2011). Anaerobic oxidation of methane: an underappreciated aspect of methane cycling in peatland ecosystems? Biogeosciences 8, 779–793. doi: 10.5194/bg-8-779-2011
Soga, T., and Ross, G. A. (1999). Simultaneous determination of inorganic anions, organic acids and metal cations by capillary electrophoresis. J. Chromatogr. A 834, 65–71. doi: 10.1016/S0021-9673(98)00692-X
Stookey, L. L. (1970). Ferrozine—a new spectrophotometric reagent for iron. Anal. Chem. 42, 779–781. doi: 10.1021/ac60289a016
Straub, K. L., Benz, M., and Schink, B. (2000). Iron metabolism in anoxic environments at near neutral pH. FEMS Microbiol. Ecol. 34, 181–186. doi: 10.1016/S0168-6496(00)00088-X
Su, J. F., Zheng, S. C., Huang, T. L., Ma, F., Shao, S. C., Yang, S. F., et al. (2015). Characterization of the anaerobic denitrification bacterium Acinetobacter sp. SZ28 and its application for groundwater treatment. Bioresour. Technol. 192, 654–659. doi: 10.1016/j.biortech.2015.06.020
Su, J. F., Zheng, S. C., Huang, T. L., Ma, F., Shao, S. C., Yang, S. F., et al. (2016). Simultaneous removal of Mn(II) and nitrate by the manganese-oxidizing bacterium Acinetobacter sp. SZ28 in anaerobic conditions. Geomicrobiol. J. 33, 586–591. doi: 10.1080/01490451.2015.1063024
Tangen, B. A., and Bansal, S. (2019). Hydrologic lag effects on wetland greenhouse gas fluxes. Atmosphere 10:269. doi: 10.3390/atmos10050269
Turetsky, M. R., Kotowska, A., Bubier, J., Dise, N. B., Crill, P., Hornibrook, E. R., et al. (2014). A synthesis of methane emissions from 71 northern, temperate, and subtropical wetlands. Glob. Change. Biol. 20, 2183–2197. doi: 10.1111/gcb.12580
Valenzuela, E. I., Avendaño, K. A., Balagurusamy, N., Arriaga, S., Nieto-Delgado, C., Thalasso, F., et al. (2019). Electron shuttling mediated by humic substances fuels anaerobic methane oxidation and carbon burial in wetland sediments. Sci. Total Environ. 650, 2674–2684. doi: 10.1016/j.scitotenv.2018.09.388
Valenzuela, E. I., Prieto-Davó, A., López-Lozano, N. E., Hernández-Eligio, A., Vega-Alvarado, L., Juárez, K., et al. (2017). Anaerobic methane oxidation driven by microbial reduction of natural organic matter in a tropical wetland. Appl. Environ. Microbiol. 83, AEM.645–AEM.617. doi: 10.1128/aem.00645-617
van Trump, I. J., Wrighton, K. C., Thrash, J. C., Weber, K. A., Andersen, G. L., and Coates, J. D. (2011). Humic acid-oxidizing, nitrate-reducing bacteria in agricultural soils. MBio 2:e044-11. doi: 10.1128/mBio.00044-11
Welte, C. U., Rasigraf, O., Vaksmaa, A., Versantvoort, W., Arshad, A., and Op, et al. (2016). Nitrate- and nitrite-dependent anaerobic oxidation of methane. Environ. Microbiol. Rep. 8, 941–955. doi: 10.1111/1758-2229.12487
Wen, G., Wang, T., Li, K., Wang, H., Wang, J., and Huang, T. (2019). Aerobic denitrification performance of strain Acinetobacter johnsonii WGX-9 using different natural organic matter as carbon source: effect of molecular weight. Water Res. 164, 114956. doi: 10.1016/j.watres.2019.114956
Xiao, L., Xie, B., Liu, J., Zhang, H., Han, G., Wang, O., et al. (2017). Stimulation of long-term ammonium nitrogen deposition on methanogenesis by Methanocellaceae in a coastal wetland. Sci. Total Environ. 595, 337–343. doi: 10.1016/j.scitotenv.2017.03.27
Keywords: greenhouse gases, anaerobic methanotrophy, wetlands, nitrous oxide, extracellular electron transfer, natural organic matter, archaea, denitrification
Citation: Valenzuela EI, Padilla-Loma C, Gómez-Hernández N, López-Lozano NE, Casas-Flores S and Cervantes FJ (2020) Humic Substances Mediate Anaerobic Methane Oxidation Linked to Nitrous Oxide Reduction in Wetland Sediments. Front. Microbiol. 11:587. doi: 10.3389/fmicb.2020.00587
Received: 07 November 2019; Accepted: 18 March 2020;
Published: 15 April 2020.
Edited by:
Amelia-Elena Rotaru, University of Southern Denmark, DenmarkReviewed by:
Bo Thamdrup, University of Southern Denmark, DenmarkJennifer Glass, Georgia Institute of Technology, United States
Bo Barker Jørgensen, Aarhus University, Denmark
Copyright © 2020 Valenzuela, Padilla-Loma, Gómez-Hernández, López-Lozano, Casas-Flores and Cervantes. This is an open-access article distributed under the terms of the Creative Commons Attribution License (CC BY). The use, distribution or reproduction in other forums is permitted, provided the original author(s) and the copyright owner(s) are credited and that the original publication in this journal is cited, in accordance with accepted academic practice. No use, distribution or reproduction is permitted which does not comply with these terms.
*Correspondence: Francisco J. Cervantes, ZmNlcnZhbnRlc2NAaWluZ2VuLnVuYW0ubXg=