- 1Department of Biology, Institute of General Microbiology, Kiel University, Kiel, Germany
- 2omics2view.consulting GbR, Kiel, Germany
- 3Department of Evolutionary Genetics, Max Planck Institute for Evolutionary Biology, Plön, Germany
- 4Marine Ecology Division, Research Unit Experimental Ecology, Benthic Ecology, GEOMAR Helmholtz Centre for Ocean Research Kiel, Kiel, Germany
Due to ocean acidification and global warming, surface seawater of the western Baltic Sea is expected to reach an average of ∼1100 μatm pCO2 and an increase of ∼5°C by the year 2100. In four consecutive experiments (spanning 10–11 weeks each) in all seasons within 1 year, the abiotic factors temperature (+5°C above in situ) and pCO2 (adjusted to ∼1100 μatm) were tested for their single and combined effects on epibacterial communities of the brown macroalga Fucus vesiculosus and on bacteria present in the surrounding seawater. The experiments were set up in three biological replicates using the Kiel Outdoor Benthocosm facility (Kiel, Germany). Phylogenetic analyses of the respective microbiota were performed by bacterial 16S (V1-V2) rDNA Illumina MiSeq amplicon sequencing after 0, 4, 8, and 10/11 weeks per season. The results demonstrate (I) that the bacterial community composition varied in time and (II) that relationships between operational taxonomic units (OTUs) within an OTU association network were mainly governed by the habitat. (III) Neither single pCO2 nor pCO2:Temperature interaction effects were statistically significant. However, significant impact of ocean warming was detected varying among seasons. (IV) An indicator OTU (iOTU) analysis identified several iOTUs that were strongly influenced by temperature in spring, summer, and winter. In the warming treatments of these three seasons, we observed decreasing numbers of bacteria that are commonly associated with a healthy marine microbial community and—particularly during spring and summer—an increase in potentially pathogenic and bacteria related to intensified microfouling. This might lead to severe consequences for the F. vesiculosus holobiont finally affecting the marine ecosystem.
Introduction
Macroalgal hosts and their associated microorganisms, designated as holobionts (Egan et al., 2013; Saha and Weinberger, 2019) or synonymously termed as “metaorganisms” (Bosch and McFall-Ngai, 2011), need to cope with changing environmental conditions like ocean acidification and warming (Qiu et al., 2019; van der Loos et al., 2019). For the adaptation and resilience of a holobiont to an altered environment an optimized host-microbe interaction is predicted to be essential (Dittami et al., 2016). In marine environments macroalgae are important components of benthic communities and essential hosts for a multitude of associated microbes (Wiencke and Bischof, 2012). Due to secreted polysaccharides their nutrient-rich surfaces represent attractive targets for rapid colonization, either by various microbes from other near-by marine organisms (e.g., algae, animals) or from the surrounding seawater resulting in the establishment of an epibiotic biofilm (Armstrong et al., 2001; Steinberg and de Nys, 2002; Dang and Lovell, 2016). Since bacteria are the first colonizers and main components of microbial biofilms, they are often considered as the most diverse microorganisms on marine surfaces with important roles attributed to them (e.g., key metabolic pathways like carbon cycling) (Wahl et al., 2012; Singh and Reddy, 2014; Dang and Lovell, 2016). The epibacterial community and the colonized macroalgal host interact in multiple and complex ways (Goecke et al., 2010; Egan et al., 2013; Dang and Lovell, 2016). Bacteria can provide the algal host with beneficial substances. For example, marine epibacteria and extracellular polymeric substances (EPS) play important roles in the morphological development and healthy growth of the algal host (Singh and Reddy, 2014; Wichard, 2015). Epibacteria benefit from the nutrient-rich algal surface, since the macroalgae provide substances the attached bacteria require for growth and metabolism. For example, synthesized macroalgal polysaccharides can be digested by bacterial enzymes to obtain carbon and energy (Hehemann et al., 2012; Martin et al., 2015). Beside bacteria, also for other marine organisms the nutrient-rich algal surface is an attractive habitat for settlement. In this regard, the epibacterial layer may promote, hinder or selectively control further settlement of prokaryotic and eukaryotic organisms on the algal surface (Nasrolahi et al., 2012; Wahl et al., 2012). Microorganisms associated with macroalgae may exhibit strong antimicrobial and antifouling activities (Satheesh et al., 2016). On the other hand, macroalgae might be able to control their epibacterial layer to a certain degree through the use of attracting or defending substances. For example, for Baltic Fucus vesiculosus it was shown that thallus-associated compounds mediated bacterial surface colonization (Lachnit et al., 2009b, 2013; Saha et al., 2011), whereas surface-attached molecules inhibited bacterial settlement protecting the macroalgal surface against potential pathogens and microfouling (Weinberger, 2007; Wahl et al., 2010; Saha et al., 2012, 2017; Saha and Wahl, 2013; Saha and Weinberger, 2019). In general, bacterial cell densities on nutrient-rich macroalgal surfaces are high (Martin et al., 2014; Dang and Lovell, 2016). On Baltic F. vesiculosus an average bacterial cell density of 5 × 107 per cm2 was reported by Stratil et al. (2013), suggesting highly complex interactions by direct cell-cell communication within the biofilm. The biofilm composition fundamentally depends on many biotic and abiotic conditions such as algal species, age, season and environmental parameters as reviewed by Martin et al. (2014). Previous studies reported that epibacterial communities differ between macroalgal host species and seasons (Lachnit et al., 2009a, 2011). Experimental studies by Stratil et al. (2013, 2014) further indicated a reshaping of native epibacterial communities on Baltic F. vesiculosus along temperature and salinity gradients over time.
In the context of climate change, ocean acidification and global warming are key processes driven by increasing atmospheric CO2 concentrations that affect marine ecosystems worldwide. It is likely that the partial pressure of CO2 (pCO2) will continue to increase over the next ∼100 years causing a decrease in mean pH of the oceans by approximately 0.2–0.3 pH units and global temperature is expected to increase by approximately 3–7°C (Omstedt et al., 2012; IPCC, 2013; Elken et al., 2015) with regional variability. The temperature of coastal shallow waters of the western Baltic Sea is expected to increase by up to 5°C until the year 2100 (Gräwe et al., 2013; Elken et al., 2015; Wahl et al., 2015a). Furthermore, the pH in this region is expected to decrease in average by ∼0.2 units driven by elevated pCO2 reaching an average of ∼1100 μatm (Wahl et al., 2015a). The majority of ocean acidification studies on marine microbes mainly focused on planktonic microbial communities with rather variable and inconsistent outcomes regarding effects on abundance, diversity and community composition. The results reach from changes in planktonic bacterial community composition and function (Beman et al., 2011; Kitidis et al., 2011; Krause et al., 2012; Lomas et al., 2012; Bergen et al., 2016) to mostly stable community compositions without functional changes (Newbold et al., 2012; Lindh et al., 2013; Roy et al., 2013; Oliver et al., 2014) under acidification. Incubation experiments with microbial biofilms and marine sediments resulted either in rapid responses of microbial communities to ocean acidification (Witt et al., 2011; Laverock et al., 2013; Braeckman et al., 2014; Tait et al., 2014), or in minor effects of acidification on community composition and function (Tait et al., 2013; Gazeau et al., 2014). Currently, the response of epibacteria on algae to ocean acidification is scarce. In particular, the response of epibacterial communities on marine macroalgae exposed to combined acidification and warming under near-natural conditions including seasonal and daily fluctuations in seawater parameters, remains unexplored. One exception is our recent study Mensch et al. (2016), that revealed only minor pCO2 but strong temperature effects on the bacterial communities attached to the Wadden Sea F. vesiculosus forma mytili (F. mytili) and in surrounding seawater. This study was performed in the outdoor tidal benthic mesocosms on the island of Sylt, Germany (Pansch et al., 2016), using a similar experimental design as outlined in the current study, however, was limited to one spring experiment (Mensch et al., 2016).
In most of the Baltic Sea, a key habitat-forming species is the perennial brown macroalga F. vesiculosus of the class Fucophyceae (Phaeophyceae) (Torn et al., 2006; Rönnbäck et al., 2007). F. vesiculosus individuals grow on hard substrata, in the western Baltic Sea typically down to a water depth of 2 m (Kautsky et al., 1986; Vogt and Schramm, 1991). Their thalli possess gas vesicles favoring an upright habitus and, thus enhancing light harvest. Together with its associated micro- and macro-organisms, F. vesiculosus represents a benthic community of high ecological importance for the coastal shallow waters of the western Baltic Sea. Thus, it was of special interest to investigate the impacts of future ocean acidification and warming events on the F. vesiculosus holobiont under near-natural experimental conditions across all seasons within 1 year. In this context, the use of mesocosms has become a popular tool in climate change research (Stewart et al., 2013).
Our experiments were conducted in the Kiel Outdoor Benthocosms (KOB) described by Wahl et al. (2015a), followed by phylogenetic analyses by Illumina amplicon sequencing of bacterial 16S rRNA genes. The study addressed the following questions. (I) What is the composition of the bacterial communities on the surface of F. vesiculosus and within the surrounding seawater and how do they restructure throughout all four seasons? (II) Which operational taxonomic units (OTUs) are strongly correlated among the OTUs that were present across the majority (≥60%) of all samples? (III) Do ocean acidification and warming impact the bacterial communities and will this impact vary among seasons? And finally, (IV) which bacterial taxa suffer or benefit from warming, identified by an indicator OTU (iOTU) analysis (Fortunato et al., 2013)?
Materials and Methods
The Kiel Outdoor Benthocosms
The benthocosms were constructed to simulate near-natural Baltic Sea shallow underwater climate scenarios (Supplementary Figure S1). For a detailed description of the KOB facility (located at the Kiel Fjord, Kiel, Germany), the experimental design and the underlying water parameters of the performed seasonal experiments see Wahl et al. (2015a). Briefly, the floating outdoor facility consists of six tanks with two independent subunits resulting in 12 experimental units with a seawater capacity of 1500 L each. Seawater was constantly supplied from the Kiel Fjord via a pipeline located at 1 m depth (with flow-through of ∼1.3 times the tank volume per 24 h), thus natural daily and seasonal fluctuations of seawater parameters (e.g., temperature, pH, salinity, nutrients) were transferred to the benthocosms (units with ambient temperature were adjusted equally to the actual seawater temperature in the Kiel Fjord). Water temperature in the tanks was constantly monitored by internal sensors and automatically controlled via a heating/cooling system. The benthocosms were covered with slanted, translucent hoods resulting in independent gas-tight headspaces per unit. The pCO2 in the headspace was constantly monitored by internal gas sensors and manipulated by injections of pure CO2 to a defined concentration, causing seawater acidification by atmosphere-water gas exchange. The latter was enhanced by wave generators (seawater inflow) and aspirator pumps, installed to mimic water movements similar to Baltic coastal zones and to enlarge the mixing efficiency.
Experimental Setup and Treatments
Within 1 year, four consecutive seasonal experiments were performed during April 2013 to March 2014 as described by Wahl et al. (2015a, 2019) for 10–11 weeks: spring (04/04, 05/02, 05/30, and 06/19/2013, 11 weeks), summer (07/04, 08/01, 08/29, and 09/17/2013, 11 weeks), autumn (10/10, 11/07, 12/05, and 12/17/2013, 10 weeks) and winter (01/16, 02/13, 03/13, and 04/01/2014, 11 weeks). For each experiment, stones bearing at least one F. vesiculosus individual were collected along the Kiel Fjord (at 54°27′14.951′′N 10° 11′ 51.886′′E) at a depth of ∼1 m (for a detailed description of the sampling site see Graiff et al., 2015). Grazers, attached to the F. vesiculosus individuals that were used for biofilm sampling, were carefully removed in seawater to keep the natural microbiota. Each benthocosm unit was populated with 20 (25 for spring experiment) stones carrying F. vesiculosus and several organisms commonly found in the natural habitat of Baltic F. vesiculosus (main mesograzers: the periwinkles Littorina littorea, Idotea spp. isopod crustaceans and Gammarus spp. amphipods, see Werner et al., 2016). The amount of grazers varied between seasons due to their natural abundance fluctuations in the Kiel Fjord (Werner et al., 2016), however, macroalgal and grazer biomass was equally distributed to the 12 units in each experiment. Stones with F. vesiculosus were placed at fixed positions onto the gratings at ∼40 cm water depth. F. vesiculosus individuals were incubated in the benthocosms under one of four different conditions: (1) increased temperature (+5°C temperature at ambient pCO2), (2) elevated pCO2 (1100 μatm pCO2 at ambient temperature), (3) increased temperature and elevated pCO2 (+5°C temperature at 1100 μatm pCO2), and (4) ambient (ambient temperature and ambient pCO2) with three units per treatment.
Temperature and pH/pCO2
As described elsewhere (Graiff et al., 2015; Wahl et al., 2015a) seawater parameters were documented regularly: temperature, pH, salinity, and O2 were constantly logged by sensors, in addition daily hand-held pH measurements were performed, total alkalinity (TA) and nutrients (PO4, NO3) were measured twice per week, and dissolved inorganic carbon (DIC) monthly. The original raw data of constantly logged temperature and pH data per benthocosm unit are available at the PANGAEA® data platform1.
Time courses of temperature and pH values for all treatments of all four benthocosm experiments are shown in Supplementary Figure S2 and in related publications (Graiff et al., 2015; Wahl et al., 2015a). Supplementary Figure S3 shows the salinity of the Kiel Fjord seawater that was constantly supplied to the benthocosms.
Sampling of F. vesiculosus Biofilm and Surrounding Seawater
Seawater samples were taken via side ports at ∼20 cm water depth (while hoods were still closed to keep the adjusted atmosphere during the sampling event) into sterile 2 L bottles, transferred to the laboratory and kept at 4°C in the dark until vacuum-filtered within 1 day. Water was pre-filtered through 10 μm Isopore polycarbonate membrane filters (Millipore, Billerica, MA, United States) to remove particles larger than 10 μm, and subsequently planktonic cells from 1 L were collected by vacuum filtration through 0.22 μm Millipore Express PLUS polyethersulfone membrane filters (Millipore, Billerica, MA, United States) at max. −0.2 bar. Membrane filters were transferred to 2 mL reaction tubes and subsequently frozen in liquid nitrogen.
The hoods were opened shortly before the biofilm sampling. Prior to biofilm sampling, the F. vesiculosus thalli were rinsed with freshly prepared 0.22 μm filtered artificial seawater (17 g/L, Reef Crystals, Aquarium Systems, Sarrebourg, France) with a salinity of 15 practical salinity units (PSU), comparable to the Kiel Fjord seawater salt concentrations, to remove non-attached cells and particles. Importantly, during each experiment biofilm samples were repeatedly taken from the upper, younger parts of 2–3 thalli (∼15 cm2 by visual estimation) of the same F. vesiculosus individuals (one F. vesiculosus per unit at a fixed position where the action of the wave generator mixed the water). For each sampling event biofilm samples were taken from different thallus tips of the same individual. Sampled thallus areas were subsequently labeled with small cable straps to prevent resampling of the same region. The biofilm samples were taken with sterile cotton tips, transferred to 2 mL reaction tubes and subsequently frozen in liquid nitrogen. All shock-frozen samples were stored at −80°C until DNA extraction.
Samples were taken after 0, 4, 8, and 10, or 11 weeks of incubation per seasonal experiment (n = 3 per treatment), with two exceptions: (1) missing biofilm data due to high fouling of F. vesiculosus under warming during summer in week 11 and (2) missing water data due to canceled filtration because of the storm “Xaver” (5.-6.12.2013) during autumn in week 8.
DNA Extraction and PCR Amplification of Bacterial 16S rDNA for Illumina MiSeq Amplicon Sequencing
Genomic DNA was isolated using the Isol-RNA Lysis Reagent (5 PRIME, Gaithersburg, MD, United States) as described by Mensch et al. (2016). The PCR amplification of the hypervariable region V1–V2 of the bacterial 16S rDNA, the subsequent purification and pooling of the amplicons, as well as the Illumina MiSeq sequencing were performed as described in Mensch et al. (2016). Sequences were submitted to the NCBI Sequence Read Archive under accession number SRP075254.
Bioinformatic Processing
Raw data of two MiSeq runs were combined and a quality filter on raw reads was performed using the trimmer program Trimmomatic version 0.33 (Bolger et al., 2014). Briefly, reads were analyzed with a sliding window of 4 bp. Regions were trimmed if the average Phred score (Ewing and Green, 1998) within the window was below 30. Illumina adapters and primer sequences were removed. Trimmed reads were kept within the dataset if the forward and reverse read both survived the quality trimming and were longer than 36 bp. Sequence processing was continued using Mothur version 1.35.1 (Schloss et al., 2009; Kozich et al., 2013) according to Mensch et al. (2016) as follows: trimmed reads were concatenated to 31,838,465 contiguous sequences (contigs) using the command make.contig. Contigs with ambiguous bases or homopolymers longer than 8 bases as well as contigs longer than 552 bases were removed (screen.seqs). The remaining 20,636,961 contigs were screened for redundant sequences (unique.seqs) and clustered into 1,642,619 unique sequences. The sequences were consecutively aligned (align.seqs) to a modified version of the SILVA database release 102 (Pruesse et al., 2007) containing only the hypervariable regions V1 and V2. Sequences not aligning in the expected region were removed from the dataset (screen.seqs). The alignment was condensed by removing gap-only columns (filter.seqs). The final alignment contained 19,311,894 sequences (1,126,879 unique) of lengths between 244 and 401 bases. Command pre.cluster was used to include sequences with up to three positional differences compared to larger sequence clusters into the latter. Chimeric sequences were removed using the Uchime algorithm (Edgar et al., 2011) (chimera.uchime, followed by remove.seqs). This left 12,575,261 sequences (205,946 unique) in the dataset. Sequence classification was performed with classify.seqs using the Wang Method (Wang et al., 2007) on a modified Greengenes database (containing only the hypervariable regions V1 and V2) with a bootstrap threshold of 80%. Sequences belonging to the kingdom archaea, to chloroplasts or mitochondria were removed from the dataset (remove.lineage). OTUs were formed using the average neighbor clustering method with cluster.split. This step was parallelized by taking into account the taxonomic classification at the order level. A sample-by-OTU table containing 57,197 OTUs at the 97% level was generated (make.shared). OTUs were classified taxonomically using the modified Greengenes database mentioned above (classify.otu).
Statistical Analysis of Bacterial 16S rDNA Amplicon Data
Statistical downstream analysis was conducted with custom scripts using R (v.3.3.1) as recently outlined by Mensch et al. (2016). OTUs of very low abundance only increase computation time without contributing useful information thus were removed from the dataset, resulting in a decrease in the number of OTUs from 57,197 to 3,095 retained in the filtered OTU table, while still representing 95% of the overall OTU counts. The median (IQR) overall OTU count per sample was 7605 (4739–12822). A redundancy analysis (RDA) was performed with Hellinger-transformed OTU counts as described by Mensch et al. (2016) to explore the extent of change in relative OTU abundance across samples explained by the experimental factors Temperature, pCO2, Week and habitat Type per Season (see metadata Supplementary Table S1). The latter (subset data “Season”) was performed to distinguish subset RDA models per season, firstly because different F. vesiculosus individuals were used in the four experiments and secondly due to some missing sequencing data excluded from the RDA [summer week 11 (decayed macroalgae) and autumn week 8 (storm)]. The factor pCO2 was removed from the RDA model at an early stage, because the pCO2 effect was not statistically significant (significance threshold p < 0.05). The temperature effects per season and type were visualized using the R package ggplot2 v3.1.1 (Wickham, 2016).
Further analyses were conducted as recently described by Mensch et al. (2016). OTUs significant at an false discovery rate of 5% (q ≤ 0.05) were further subject to indicator analysis with function multipatt of the R package indicspecies v1.7.5 (Cáceres and Legendre, 2009) with 105 permutations. Indicator OTUs (iOTUs) – in analogy to indicator species sensu Cáceres and Legendre (2009) – are OTUs that prevail in a certain sample group (here: a level of Temperature within a certain sample Type and level of Week) while being found only irregularly and at low abundance in other sample groups.
Venn diagrams of OTU shared between sample groups were created with R package VennDiagram v1.6.20 (Chen, 2018). For alpha diversity analysis, effective OTU richness [Shannon numbers equivalent, 1D (Jost, 2006, 2007)] was calculated from the filtered OTU table. Multi-panel alpha diversity and iOTU plots were drawn with R package lattice v0.20-33 (Sarkar, 2008). An OTU association network (incorporating all sequenced samples due to small datasets per season) was inferred with the R package SpiecEasi v0.1 (Kurtz et al., 2015). OTUs were required to be present in ≥60% of all samples to reduce the number of zeros in the data for a robust calculation, resulting in a set of 42 OTUs. Calculations were performed with the Meinshausen and Bühlmann neighborhood selection framework (MB method, Meinshausen and Bühlmann, 2006). Correlations between associated OTUs were determined from the centered log-ratio-transformed counts. The igraph package v1.0.1 (Csárdi and Nepusz, 2006) was employed for visualizing strong OTU associations (absolute correlation ≥ 0.6).
Results
During April 2013 to March 2014 four consecutive seasonal experiments were performed as described in detail in Wahl et al. (2015a, 2019) and the “Materials and Methods” section, to monitor changes in the bacterial communities associated with F. vesiculosus in response to acidification and warming. During the experiments the monthly mean ambient seawater temperature increased from 8.2 ± 1.4 to 16.1 ± 1.3°C in the spring experiment (Supplementary Figure S2; see also Graiff et al., 2015; Wahl et al., 2015a). During summer, maximum temperatures were recorded in late July–August (19.5 ± 1.2, max. 24.8°C), followed by a decrease to 17.4 ± 1.0°C in September. Then, ambient seawater temperature decreased from 13.1 ± 0.5 to 6.9 ± 0.5°C in autumn and slightly increased again from 4.2 ± 1.4 to 6.7 ± 1.1°C in winter. In each season, seawater temperature was approximately +5°C higher in the warming treatments. Importantly, during the summer experiment in late July to August daily temperatures of ∼29°C were reached in the warming treatments. In general, ambient temperature showed a seasonal variation typical for the region with a minimum of about 1°C in late January/early February and a maximum of about 24°C in late July/early August. In addition, daily fluctuations in the order of 1–3°C were observed, reaching extremes of 5°C on sunny summer days (Wahl et al., 2015a). During the experiments the monthly mean ambient seawater pH (NBS scale, Supplementary Figure S2; Graiff et al., 2015; Wahl et al., 2015a) ranged from 8.73 ± 0.11 to 8.26 ± 0.39 in spring, from 8.20 ± 0.19 to 7.91 ± 0.12 in summer, from 7.97 ± 0.09 to 7.87 ± 0.05 in autumn and from 7.96 ± 0.07 to 8.08 ± 0.14 in winter, with the overall tendency of constantly declining pH values over the year. Highest pH values were measured in April at the beginning of the spring experiment and lowest pH values in December at the end of the autumn experiment, then again rising during the winter experiment. Atmospheric CO2 enrichment of elevated pCO2 treatments (1050–1100 μatm) resulted in a pH reduction of the seawater by 0.18 ± 0.08 compared to ambient pCO2 conditions (380–450 μatm, free air-circulation under hood; Wahl et al., 2015a). All benthocosms were constantly supplied with seawater from the Kiel Fjord. The salinity changed during the year from 12.4 ± 0.3 in April to highest 20.7 ± 1.5 PSU in December 2013 (Supplementary Figure S3).
(I) Bacterial Community Composition of F. vesiculosus Biofilm and Seawater
Temporal Development of the Bacterial Communities
The majority of OTUs were shared among biofilm and seawater bacterial communities during all seasons (1717 ± 46 OTUs), however, more OTUs were specific to the surface of F. vesiculosus than were specific for the surrounding seawater (Figure 1). On F. vesiculosus 405, 429, 582, and 427 OTUs were detected during the spring, summer, autumn, and winter experiments, respectively. The Figures 2, 3 provides the numbers of OTUs shared among all seasons within the biofilm and seawater samples, respectively.
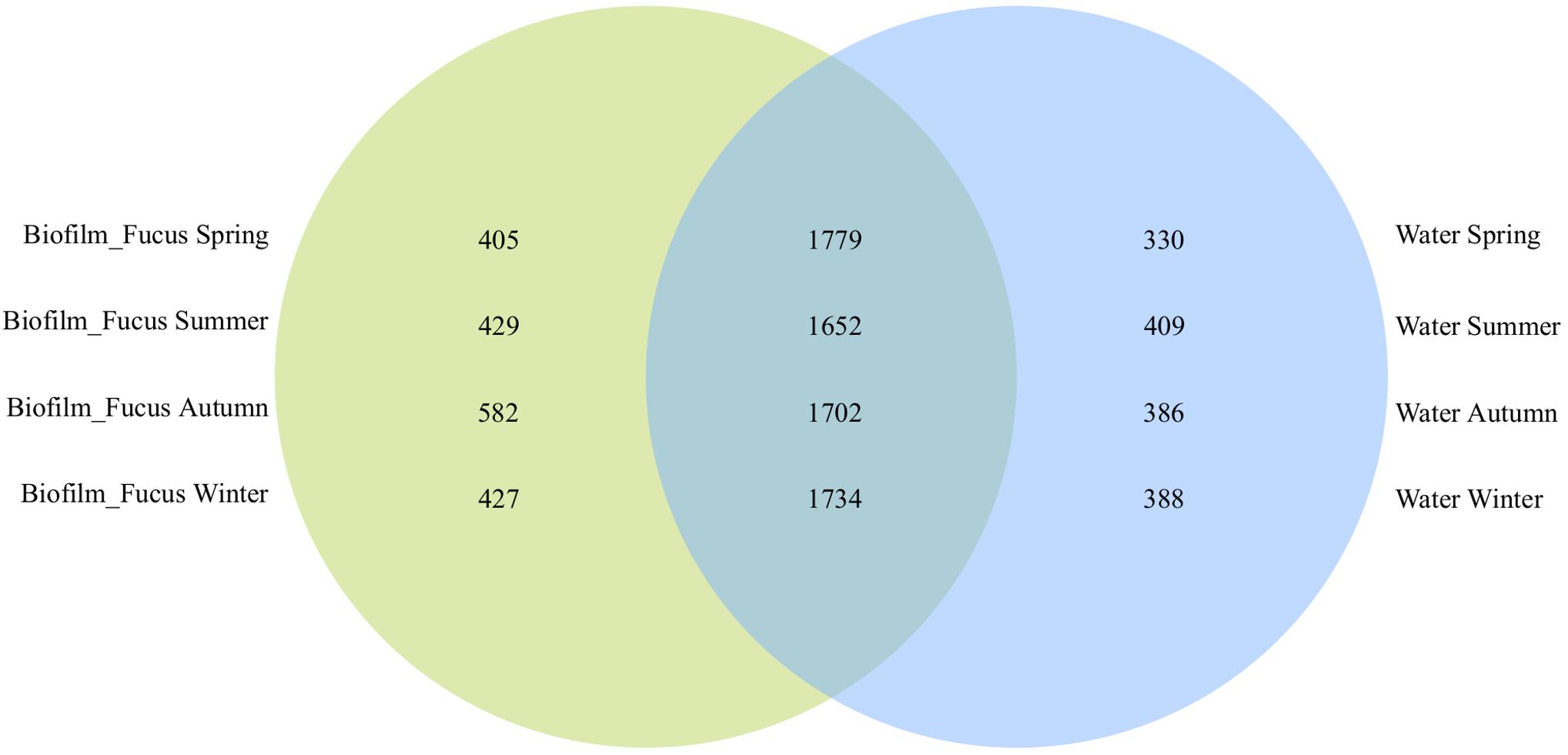
Figure 1. OTU overlaps among biofilm and seawater bacterial communities per season. Venn diagram illustrating the counts of shared OTUs between Fucus vesiculosus biofilm (“Biofilm_Fucus”, green) and seawater (“Water”, blue) bacterial communities during spring, summer, autumn, and winter.
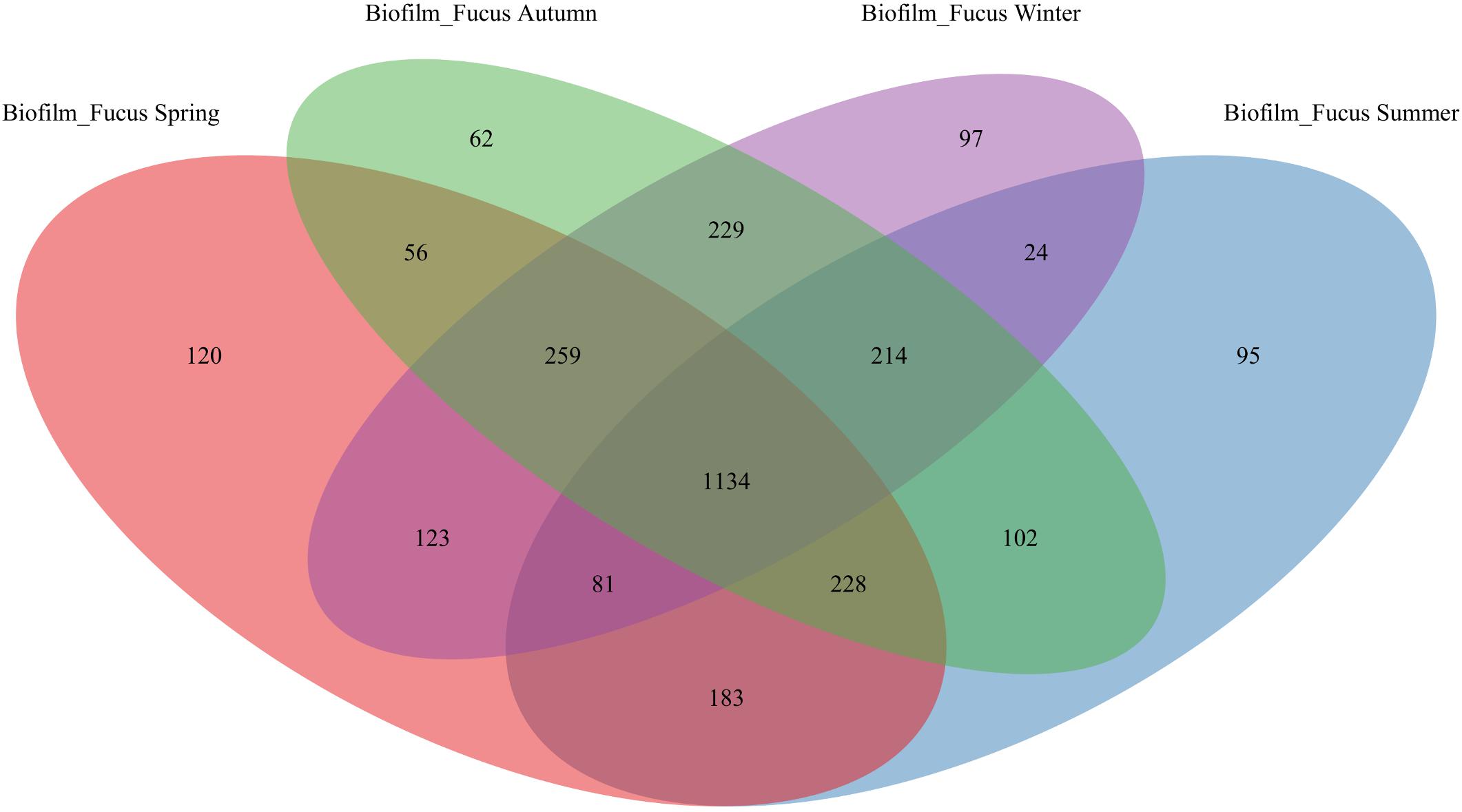
Figure 2. OTU overlap of biofilm bacterial communities among seasons. Venn diagram illustrating the counts of OTUs shared by Fucus vesiculosus biofilm (“Biofilm_Fucus”) bacterial communities among spring, summer, autumn, and winter.
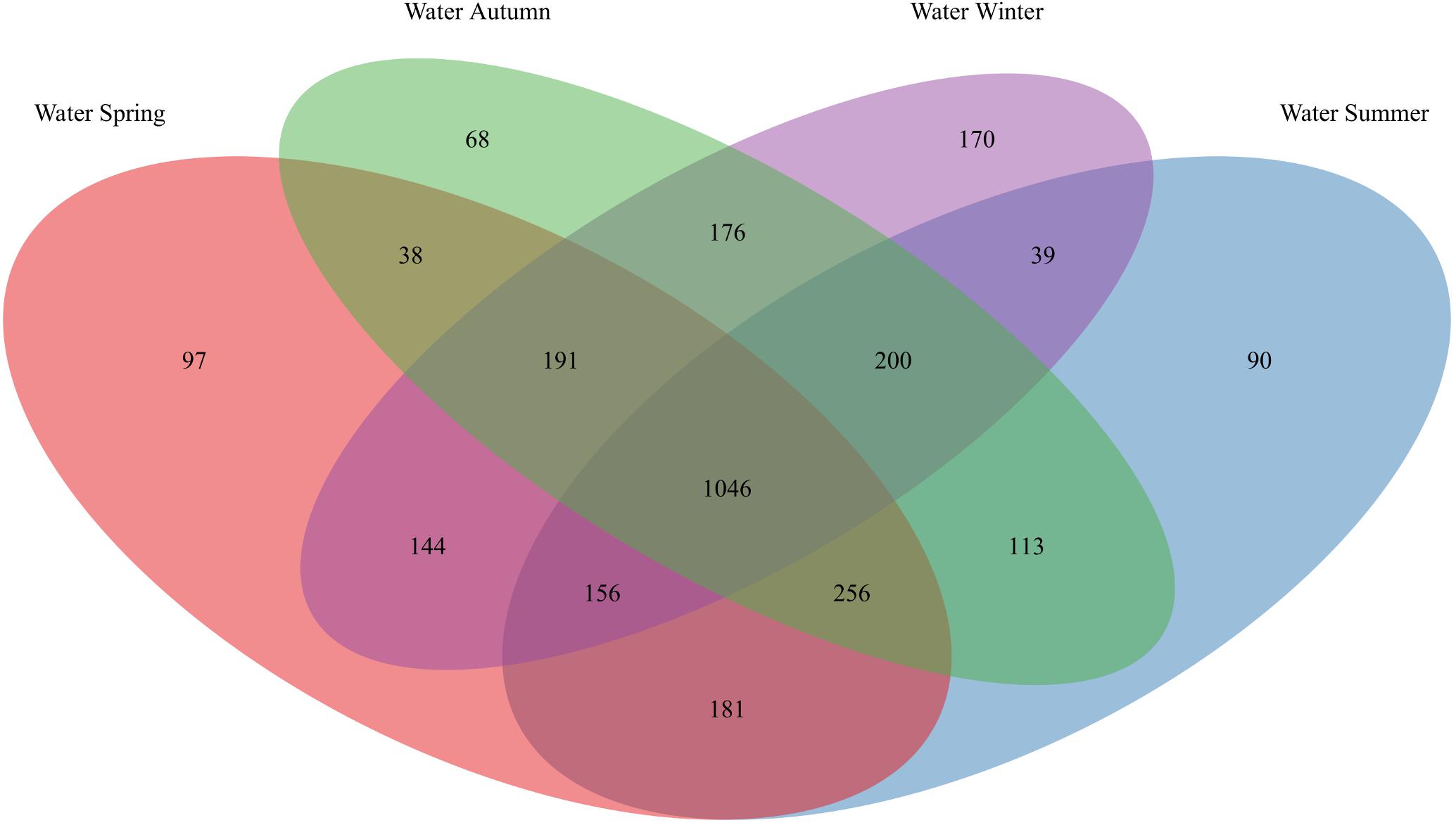
Figure 3. OTU overlap of seawater bacterial communities among seasons. Venn diagram illustrating the counts of OTUs shared by seawater (“Water”) bacterial communities among spring, summer, autumn, and winter.
At the OTU level, the bacterial communities varied in their composition depending on habitat type (biofilm or seawater) and on season (spring, summer, autumn, or winter). Within each season, the bacterial communities on F. vesiculosus and in the surrounding seawater developed over weeks 0, 4, 8, and 10/11 remarkably (F ≥ 2) differing in their community composition as revealed by pairwise F-value clustering (Supplementary Figures S4, S5), respectively. An exception was observed in winter, when differences in biofilm community patterns between weeks were hardly (week 0 vs. 4) or not significant (week 8 vs. 11) (Supplementary Figure S4D).
Phylogenetic Composition of the Bacterial Communities
Across all seasons and treatments, the biofilm attached to the F. vesiculosus surface was generally dominated by Proteobacteria (mainly Alpha- and Gamma-, fewer Delta- and Betaproteobacteria), Bacteroidetes (Flavobacteriia, Saprospirae, and Cytophagia), Actinobacteria (Actinobacteria and Acidimicrobiia), and Cyanobacteria (Oscillatoriophycideae) (Supplementary Figure S6). However, the epibacterial community composition was highly variable between the F. vesiculosus replicates, and the relative abundances of bacterial classes varied between weeks and seasons (n = 3 per treatment; 180 biofilm samples in total).
Under ambient conditions, the majority of the epibacterial community on F. vesiculosus consisted of Alphaproteobacteria, Gammaproteobacteria, Flavobacteriia, and Saprospirae, depending on week and season (spring 61.0 ± 13.5%, summer 49.0 ± 9.1%, autumn 51.1 ± 9.6%, and winter 65.4 ± 6.4%, mean ± SD% relative abundance). During spring, the Alpha- and Gammaproteobacteria together accounted for 43.7 ± 11.8%, whereas their proportion was lower in the other seasons (36.0 ± 6.7% in summer to winter). In contrast to spring to autumn (16.7 ± 10.6%) Flavobacteriia and Saprospirae together tended to be more abundant in the biofilm during winter (25.3 ± 6.7%). During autumn to winter 10.4 ± 4.8% Deltaproteobacteria were present on F. vesiculosus, about twice as much than found in spring to summer (5.6 ± 2.9%). Betaproteobacteria showed irregular pattern in single biofilm samples during spring to summer with 8.6 ± 12.5% and were nearly absent in autumn to winter (1.5 ± 1.8%). Acidimicrobiia were nearly absent in spring (1.4 ± 0.9%), but low abundant through the rest of the year (3.8 ± 2.5%), and Cytophagia were hardly found in winter (0.9 ± 0.5%), however, showed small abundance in the other seasons (3.2 ± 2.3%). Compared to summer and winter (0.5 ± 0.9%) the class Oscillatoriophycideae showed its highest abundances in spring and autumn (3.0 ± 2.4% and 2.0 ± 4.2%, respectively), whereas the Actinobacteria were least abundant through all seasons (0.7 ± 1.1%). At the end of the summer experiment (week 11), only few biofilm samples were taken because water temperatures of ∼24°C and ∼29°C were reached for ambient and increased temperature treatments, respectively, both resulting in the death of the host algae (see “Materials and Methods” section); in particular under the warming treatment F. vesiculosus was completely decayed.
Under ambient conditions, the majority of the free-living bacteria in the surrounding seawater belonged to Alpha-, Gammaproteobacteria, and Flavobacteriia (Supplementary Figure S7). Although varying in proportion, together these three classes accounted for 75.3 ± 11.8% of the total bacterial community composition in spring, summer and winter, contrarily to 55.9 ± 12.7% in autumn. Among them, the Flavobacteriia reached highest prevalence during summer (24.1 ± 14.0%). Furthermore, the proportion of Actinobacteria was markedly higher in autumn (19.2 ± 14.8%) than in all other seasons (4.6 ± 3.1%). Betaproteobacteria were constantly present (5.8 ± 3.8%) throughout all seasons. Saprospirae were nearly absent in summer and reached highest proportion during spring (1.2 ± 1.6% and 8.8 ± 15.0%, respectively), whereas Deltaproteobacteria were constantly low abundant though all seasons (1.3 ± 1.2%) and Acidimicrobiia showed highest percentage during autumn (4.7 ± 2.8%). In week 8 of the autumn experiment, seawater samples were lost due to a severe storm (see “Materials and Methods” section; n = 3 per treatment; 180 water samples in total). In general, contrarily to the high variation in community composition in F. vesiculosus biofilms, the variation in community composition between replicates of the surrounding seawater was smaller.
Alpha Diversity
At the OTU level, the effective OTU richness 1D (or Shannon numbers equivalents, expressing the alpha diversity; Jost, 2006, 2007) of F. vesiculosus biofilm tended to be higher in autumn and winter (up to median values of ∼200) compared to spring and summer (up to median values of ∼150), however, with generally high variability between biofilm replicates (Figure 4). Further, in spring and autumn, biofilm 1D tended to increase over time. In the surrounding water, 1D was not affected by season and showed small variation at constantly low median values (∼25 in spring and summer, and ∼50 in autumn and winter). In general, 1D was much higher in biofilm than in seawater samples. No clear differences in 1D were observed in the single and combined pCO2 or warming treatments for both habitat types in all seasons.
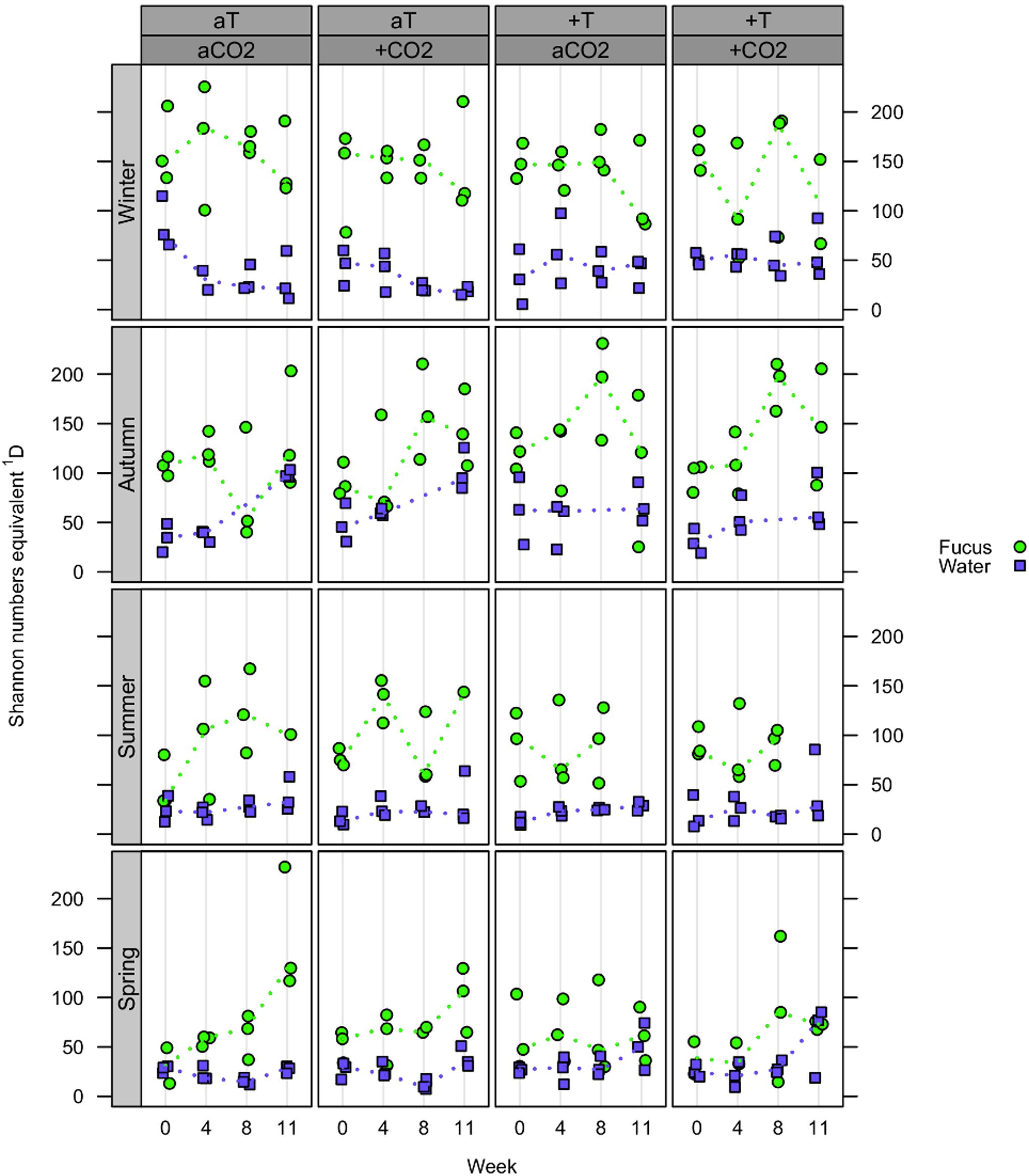
Figure 4. Effective OTU richness (alpha diversity). Distribution of Shannon numbers equivalents 1D at OTU level concerning Fucus vesiculosus biofilm (green circles) and water samples (blue squares) after 0, 4, 8, and 10/11 weeks of treatment during spring, summer, autumn, and winter, respectively, in the Kiel Benthocosms at four different conditions (see section “Materials and Methods”; T, temperature; CO2, pCO2; a, ambient; +, increased). Dots represent 1D of each single sample and dotted lines connect the medians (n = 3) between weeks.
(II) OTU Association Network
Bacterial correlations (within the entire dataset of 366 sequenced samples including treatment samples) described by an OTU association network of OTUs that were consistently present across the majority (≥60%, see “Materials and Methods” section) of samples included 42 OTUs with moderate to strong correlations (Figure 5 and Supplementary Table S2). Their strength and type of correlation, as well as environmental (habitat related) and seasonal distribution within the OTU network are shown in Figure 5. The network-forming OTUs belonged to 11 bacterial classes with the majority belonging to Alphaproteobacteria, Flavobacteriia, Beta- and Gammaproteobacteria, complemented by OTUs of Saprospirae, Acidimicrobiia, Actinobacteria, BME43 cluster, Deltaproteobacteria, Mollicutes, and Oscillatoriophycideae (Supplementary Table S2). Apart from few exceptions, all network OTUs were either found in biofilm or water samples (Figure 5A): positive correlations appeared only between OTUs dominating the same habitat, and negative correlations were rare and occurred solely between OTUs of different habitats. The proportional distribution of network-forming OTU counts varied with season (Figure 5B): Largest proportions were found in summer and spring, less in autumn, and smallest in winter. The OTU association network analysis (Figure 5 and Supplementary Table S2) revealed some notable findings described in the following section.
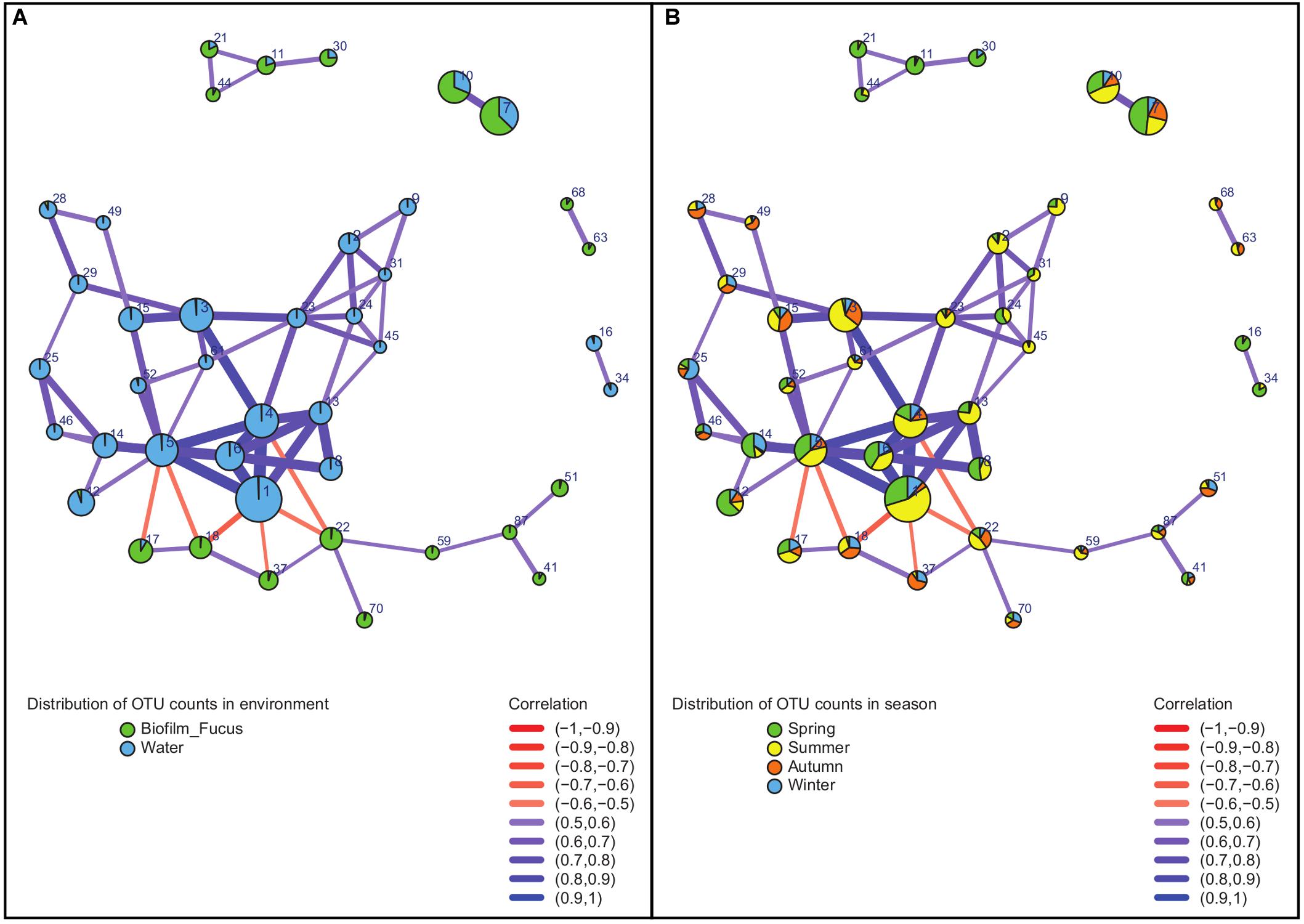
Figure 5. Association network of strongly correlated OTUs. Analysis was performed on sequence data of entire dataset. Vertices represent OTUs and are labeled by OTU number (for details see Supplementary Table S2) and colored according to (A) distribution in environment (habitat) or (B) distribution in season, respectively. Width and color of the edges connecting the vertices vary according to strength and sign (color gradient red to blue describes negative to positive) of the correlation between associated OTUs. Diameters of the vertices vary according to the relative abundance of each OTU.
One of the rare network OTUs present in both habitats, biofilm and seawater, was OTU#30 (Glaciecola punicea) found in spring, and to a small extent in winter. This OTU occurred to ∼25% in the surrounding seawater and to ∼75% associated with F. vesiculosus. OTUs found to be primary attached to F. vesiculosus were the positively correlated OTU#87 (Rubidimonas crustatorum) and OTU#41 [unclassified (uncl.) Maribacter]. Both were present in all seasons, but dominant in summer and spring. Further, OTU#17 (uncl. Erythrobacter) and OTU#18 (uncl. Polyangiaceae) were also positively correlated in biofilm samples. They occurred in all seasons, but OTU#17 was dominant in spring to summer, and OTU#18 was present mainly in summer to winter. Additionally correlated OTUs in the F. vesiculosus biofilm were OTU#37 (uncl. Thiohalorhabdales), OTU#70 (acidimicrobial C111), OTU#59 (uncl. Flavobacteriaceae), and OTU#51 (uncl. Hyphomonadaceae).
Negative correlations between OTUs of different habitats were found, for instance, between both, OTU#17 and OTU#18 (positive correlated uncl. Erythrobacter and uncl. Polyangiaceae) predominantly on F. vesiculosus, and OTU#5 [uncl. “Candidatus (Ca.) Portiera”] in seawater, as well as between OTU#18 (uncl. Polyangiaceae) on F. vesiculosus and OTU#1 (Pelagibacter ubique) in seawater. Moreover, OTU#1 was strongly positive correlated with three OTUs (#4, #6, #13) in water of the same family Pelagibacteraceae underlining the essential role of this abundant family for microbial seawater communities. Additionally, OTU#1 (P. ubique) and OTU#5 (uncl. “Ca. Portiera”) were positively correlated in seawater, both present in all seasons, but mainly in summer and spring. Another example for positively correlated OTUs in water were OTU#31 (“Ca. Aquiluna rubra”) and OTU#24 (uncl. Acholeplasma), both present in spring and summer. Further OTUs predominantly associated with seawater samples were OTU#3 (uncl. Octadecabacter) detected in all seasons, but mainly summer and autumn, as well as OTU#34 (uncl. RS62, Comamonadaceae) mainly found in spring and less in summer.
(III) Temperature and pCO2 Effects on Biofilm and Seawater Bacterial Communities per Season
Redundancy analysis (RDA) was performed as described in the “Materials and Methods” section, showing that a restructuring of bacterial community structures to elevated pCO2 levels was not statistically significant in any season. Further, no additional interaction of elevated pCO2 with increased temperature was observed. However, the impact of increased temperature on the bacterial communities varied between the seasons, but could not be measured in autumn (Figure 6 and Supplementary Table S3).
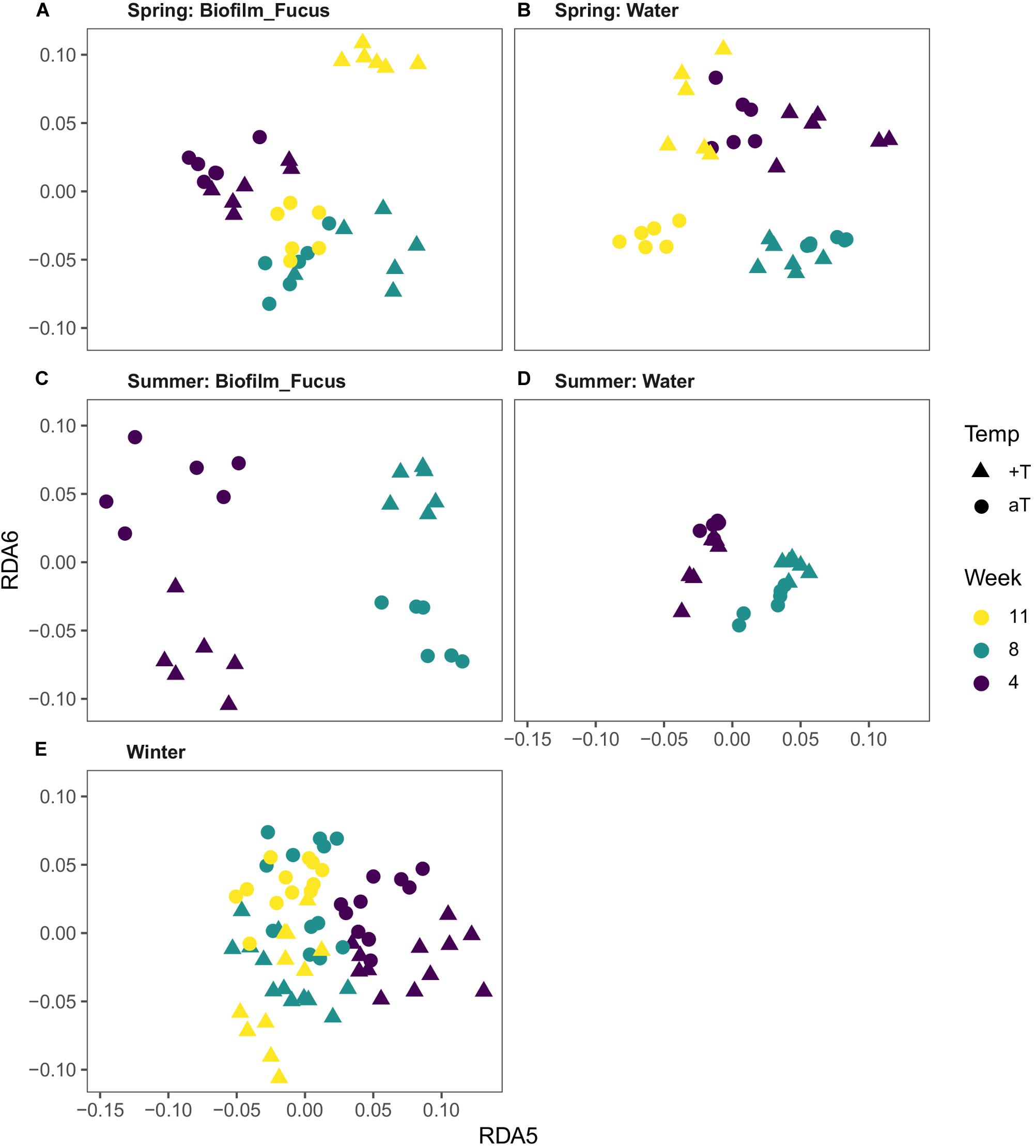
Figure 6. Visualization of the temperature effect on bacterial communities. Distance plots were derived from respective RDA models (see “Materials and Methods” section). Since the temperature effect is rather subtle, higher dimensions (constrained axes “RDA 5” and “RDA 6”) were chosen to achieve optimal visual differentiation between temperature treatment groups (Temp; aT, ambient temperature; +T, increased temperature). In spring (A,B) and summer (C,D), the difference in community composition depends on the origin of the bacterial communities (“Biofilm_Fucus” or “Water”) as well as on the duration of the treatment (Week; note the different directions in which the groups are separated). In winter (E), samples are uniformly separated according to temperature treatment, regardless of duration and origin.
In spring, a week-wise evaluation revealed that biofilm and water communities significantly developed over time while both habitat types were differently affected by increased temperature (Type:Temp:Week interaction, Supplementary Table S3). In weeks 4, 8, and 11, increased temperature significantly restructured the biofilm [F(1,10) = 1.75, p = 0.026; F(1,10) = 2.18, p = 0.005; and F(1,10) = 1.89, p = 0.007] and seawater communities [F(1,10) = 2.84, p = 0.007; F(1,10) = 3.44, p = 0.006; and F(1,10) = 3.04, p = 0.007], see Figures 6A,B. Although the temperature effect on biofilm was statistically weak (indicated by F ≤ 2) in weeks 4 and 11, it was highly significant. The temperature effect in weeks 4, 8, and 11 on biofilm and water samples explained 6, 10, and 7% and 14, 18, and 16% of total variance in spring, respectively.
In summer, the bacterial communities of both habitat types significantly developed over time while they were differently affected by the warming treatment (Type:Temp:Week interaction, Supplementary Table S3), shown by a week-wise evaluation. In weeks 4 and 8, increased temperature significantly reshaped the biofilm [F(1,10) = 2.79, p = 0.003; and F(1,10) = 4.15, p = 0.001] and water communities [F(1,10) = 2.00, p = 0.005; and F(1,10) = 2.97, p = 0.002], see Figures 6C,D. Remarkably, the temperature effect on biofilm samples was stronger in summer than in spring, with strongest effect size (F value) in week 8 [Biofilm.Temp.Week08, F(1,10) = 4.15, p = 0.001]. [No data is available for week 11 due to collapsed system during hot summer months (see “Materials and Methods” section).] The temperature effect in weeks 4 and 8 on biofilm and water samples explained roughly 14 and 22% and 8 and 15% of total variance in summer, respectively. A weak, but significant temperature effect on biofilm at the beginning of the summer experiment was measured [Biofilm.Temp.Week00, F(1,10) = 1.68, p = 0.031], however, the iOTU analysis revealed negligible results. This effect might be most likely traced back to a technical artifact of the benthocosm facility, namely to approximately 0.5°C temperature difference between ambient and warm tanks at the beginning of each experiment shortly before treatment start.
No temperature effect could be measured in autumn. However, a week-wise evaluation demonstrated that both types significantly differed in their composition since experimental start, and further developed differently over time (p < 0.001 for each week-wise comparison of weeks 0, 4, and 10, respectively) with the most remarkable differences between start and end of the experiment [Biofilm Week00 – Week10, F(1,22) = 6.53, p < 0.001; Water Week00 – Week10, F(1,22) = 7.38, p < 0.001; Type:Week interaction, Supplementary Table S3]. Roughly, the week effect among biofilm and water samples explained 8–19% and 13–22% of total variance in autumn, respectively.
Although the temperature effect in winter explained only 1% of total variance, the RDA revealed a highly significant superior temperature effect that affected both habitat types similarly in each week [Temp, F(1,85) = 2.17, p = 0.001; Temp + Type:Week interaction (=significant Temperature term and significant Type:Week interaction); Supplementary Table S3], see Figure 6E. An interaction of Temperature with Week was not statistically significant, but was assumed since the pairwise F-value clustering showed that biofilm and water communities markedly (F > 2) differed between weeks thus developed over time [p = 0.001 per week-wise comparison; Biofilm.Week08 ∪ 11 were exceptionally unified (∪) due to high similarity, Supplementary Table S3, see also Supplementary Figures S4, S5]. Similar to autumn, during winter the most remarkable differences in community composition were observed between start and unified weeks 8/11 of the experiment for both types, respectively [Biofilm Week00 – Week08 ∪ 11, F(1,32) = 6.53; Water Week00 – Week08/11, F(1,21) = 7.48/7.24]. On the basis of a week-wise comparison, the week effect among biofilm and water samples explained roughly 6–14% and 7–23% of total variance in winter, respectively.
(IV) Temperature Related iOTUs in Spring, Summer, and Winter
The temperature effects in spring, summer and winter could be traced back to single OTUs that significantly differ in their relative abundance between ambient (aT) and increased (+T) temperature levels (false discovery rate of q ≤ 0.05). According to that, an iOTU for aT is more abundant at ambient temperature conditions and an iOTU for +T shows higher relative abundance at increased temperature. Table 1 summarizes the counts of iOTUs for temperature detected per season, week and type. During summer, the number of iOTUs for +T on F. vesiculosus remarkable increased from 12 to 38 in week 4 to 8. The temperature effect on the bacterial communities was stronger in summer than in spring, supported by higher effect sizes from statistical testing (see section “Results” part III for related F-values) and the observation that on F. vesiculosus roughly twice as much classes were found to harbor iOTUs that were affected by temperature in summer (17 classes) compared to spring (9 classes). To gain more detailed insights into the complex restructuring of bacterial communities in response to warming in spring, summer and winter, Supplementary Tables S4, S5 comprises all iOTUs for aT and +T, respectively, with taxonomic information at least at genus level. The mean differences in relative abundance (Δ‰ = ‰ +T - ‰ aT) underline the negative (–) or positive (+) impacts of the applied warming on a certain iOTU.
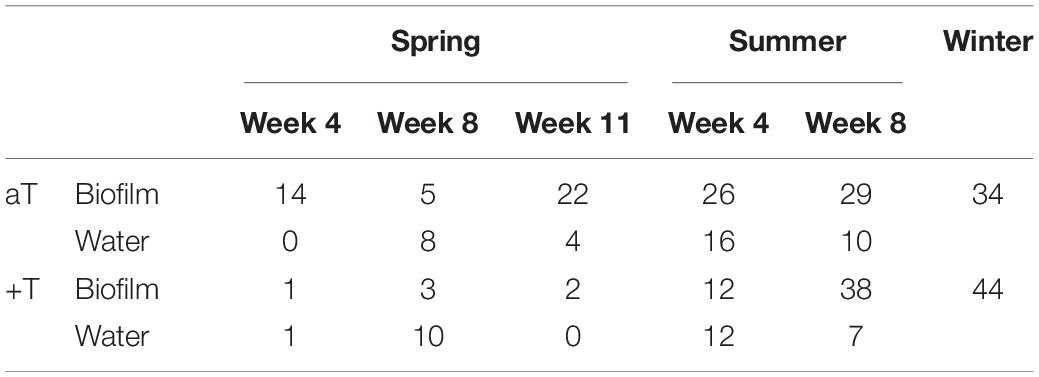
Table 1. Counts of indicator OTUs for temperature. Counts of iOTUs for ambient (aT) and increased temperature (+T; treatment details see “Materials and Methods” section) detected in Fucus vesiculosus biofilm and seawater bacterial communities during spring (weeks 4, 8, and 11), summer (weeks 4 and 8), and winter, respectively.
Selected Indicators Varying Between Both Temperature Levels (Compare Supplementary Tables S4, S5)
On lower taxonomic resolution, many taxa included single iOTUs for both temperature levels (mainly with different OTU numbers) varying with season, week and/or type, because the majority of all iOTUs could not exactly be specified by the performed amplicon sequencing. On F. vesiculosus, the genus Erythrobacter was indicator for aT in spring and summer, and further for +T in winter. Surface attached to F. vesiculosus in summer were iOTUs of the genus Roseivirga as indicators for +T and aT. Indicators for both temperature levels in summer on F. vesiculosus belonged to Crocinitomix, also detected as iOTU for +T in winter. Maribacter was indicator for +T in summer on F. vesiculosus, but indicator for aT in winter. In seawater, the genus Sediminicola was indicator for aT in spring, but for +T in summer. The genus Octadecabacter was indicator for aT in summer in seawater, however, the species O. antarcticus was iOTU for +T in winter. In winter, the genera Polaribacter and Fluviicola were indicators for both +T and aT, respectively. Three species of the genus Glaciecola were identified to be indicators for temperature in different seasons and weeks, associated with both habitat types, while the majority was indicator for aT. G. punicea (iOTU for aT on F. vesiculosus during spring in week 8 with Δ-50.19‰ and in week 11 with Δ-16.52‰), G. mesophila (iOTU for aT in water during summer in week 8 with Δ-2.37‰; but iOTU for +T in winter with Δ+10.89‰) and G. psychrophila (iOTU for aT in winter with Δ-0.69‰). In addition, further different iOTUs belonging to the genus Glaciecola could not be specified, but were indicators for aT in summer for F. vesiculosus (Δ-15.16‰) and in winter for both types, as well as iOTUs for +T and aT in seawater (Δ-20.18‰) during summer
Selected iOTUs for Ambient Temperature (aT) Level (see Supplementary Table S4)
Among the iOTUs for aT on F. vesiculosus in spring were Psychroserpens mesophilus, as well as the genera Luteolibacter and Rickettsia. In summer, Hirschia baltica, Anabaena cylindrica (in week 4 with Δ-9.88‰) and R. crustatorum (in week 8 with Δ-9.66‰) were identified as iOTUs for aT associated with F. vesiculosus. The genus Flavobacterium was detected as iOTUs for aT in spring for both types and during summer in seawater. Lewinella comprised iOTUs for aT in spring on F. vesiculosus and in winter. In seawater P. ubique (in week 8 with Δ-190.14‰ based on 480.14‰ at aT vs. 290.00‰ at +T) and “Ca. Aquiluna rubra” were iOTUs for aT in spring. In summer, iOTUs for aT in water belonged to the genera Coraliomargarita, “Ca. Portiera,” gammaproteobacterial HTCC2207, Microbacterium, Pseudomonas and Yonghaparkia. The genus Persicirhabdus was an indicator for aT in water in summer, and was also detected at aT in winter. In winter, the genus Roseibacillus was also identified as indicator for aT. The higher abundance of these iOTUs at ambient compared to increased temperature in turn means, that they suffered from warming.
Selected iOTUs for Increased Temperature (+T) Level (see Supplementary Table S5)
Although no specified iOTU for +T on F. vesiculosus could be identified in spring, an iOTU for +T attached to F. vesiculosus in summer was Winogradskyella poriferorum (Δ+3.21‰), in addition to iOTUs of the genera Rivularia and Phormidium. Plesiocystis was indicator for +T on F. vesiculosus during summer, and for both types in winter. In spring, iOTUs for +T in seawater belonged to the genera Mycobacterium and Owenweeksia, and in summer to Thalassospira, Acholeplasma and gammaproteobacterial BD2-13. In addition, the genus Arcobacter was indicator for +T in spring in water, and in summer iOTU associated with both F. vesiculosus and water. In winter, several iOTUs for +T belonged to the genera Devosia, Leadbetterella, Formosa, “Ca. Endobugula,” Umboniibacter and Reichenbachiella. In contrast to iOTUs for aT, iOTUs for +T benefited from warming.
Discussion
(I) Seasonal Variations in Epibacterial Communities of F. vesiculosus
The most abundant bacterial phyla attached to Baltic F. vesiculosus under ambient conditions were Proteobacteria, Bacteroidetes and Actinobacteria. In a comparable experimental design on Wadden Sea F. mytili, we observed a similar epibacterial community composition during spring that developed over time (Mensch et al., 2016). This is in agreement with previous field observations that the composition of epibacterial communities depends more on host identity than on habitat (North vs. Baltic Sea; Lachnit et al., 2009a). Although F. mytili and F. vesiculosus differ in many aspects regarding e.g., morphology (Albrecht, 1998) and the habitat conditions they live in (e.g., salinity, tidal ranges, nutrient concentrations), they are closely related macroalgal species. Thus, their surfaces were covered predominantly by the same bacterial classes during the spring experiments and their epibacterial communities only differ at finer phylogenetic resolution. Importantly, the same sampling method, DNA isolation and Illumina MiSeq sequencing technologies were used in our study on F. mytili (Mensch et al., 2016) supporting a reliable comparison of our studies. The epibacterial communities of Baltic F. vesiculosus showed temporal variability, as previously reported in a study using 16S rDNA cloning and Sanger sequencing (Lachnit et al., 2011), showing that biofilms naturally differ in their composition between seasons driven by changes in environmental conditions. Epibacterial communities of Baltic F. vesiculosus alter along temperature and salinity gradients (Stratil et al., 2013, 2014). During our experiments, salinity was monitored, and it changed during the course of the year (Supplementary Figure S3). However, salinity is not a defined experimental factor. While it is likely to have an additional influence on the bacterial communities, this effect was the same for all communities, since all benthocosms/treatments were constantly supplied with the same Fjord seawater. The salinity of the benthocosms and the Fjord were equivalent, shown by Wahl et al. (2015a).
Our findings on the epibacterial community composition of Baltic F. vesiculosus are in agreement with a previous study based on 16S rDNA amplicon pyrosequencing targeting the same hypervariable region V1-V2 (Stratil et al., 2013). Comparable to our previous study on the F. mytili holobiont (Mensch et al., 2016), at any given time point the biofilm replicates generally showed more variability between replicates than water samples, hinting at the influence of individual host physiology (or underlying genetic traits) and a better hydrodynamic homogenization of plankton within tanks and with the fjord water. In contrast, the biofilm community composition appeared more stable over time than seawater communities suggesting that F. vesiculosus controls to some degree its own microbiota via attracting and defending substances (Lachnit et al., 2009b; Wahl et al., 2010, 2012; Saha et al., 2011, 2012, 2017; Saha and Wahl, 2013) and that fluctuations in seawater conditions might affect free-living bacteria more directly (in contrast to matrix-embedded biofilm bacteria) resulting in a faster temporal response.
The alpha diversity (effective OTU richness) of the F. vesiculosus biofilm varied with season in response of epibacteria and/or host to shifts in seawater conditions in the course of the year. Similar to our previous study on Wadden Sea F. mytili (Mensch et al., 2016), the Baltic F. vesiculosus biofilm alpha diversity was about 4 (autumn/winter) to 6 times (spring/summer) higher than in the surrounding seawater, presumably because the macroalgal surface represents an attractive nutrient-rich habitat for settling and dense microbial life resulting in a higher species richness (Egan et al., 2013; Dang and Lovell, 2016).
(II) Correlations Among Associated OTUs Mainly Governed by the Habitat
Positive correlations within OTU association networks suggest mutualistic interactions, while negative correlations often reflect competition (Steele et al., 2011; Faust and Raes, 2012; Fuhrman et al., 2015). However, negative correlations were exclusively found between OTUs dominating in either biofilm or seawater samples, verifying the habitat as most important factor in shaping the bacterial community compositions, as previously observed for F. mytili biofilm and North Sea water (Mensch et al., 2016). Strongest positive correlations were found between OTUs of the Alphaproteobacteria, the most abundant bacterial class on F. vesiculosus in this and other datasets (Stratil et al., 2013; Mensch et al., 2016; Parrot et al., 2019).
(III) Bacterial Communities Were Affected by Temperature With Seasonal Variability, but pCO2 Had No Impact
Although a weak growth stimulating pCO2 effect was observed on Baltic F. vesiculosus hosts (Graiff et al., 2015), no direct pCO2 effects on biofilm and seawater bacterial communities in the single treatment, nor any interaction effect of pCO2 and temperature in the combined treatment was apparent in any seasons. The increase of pCO2 to 1100 μatm in the headspace resulted in a mean decrease of ∼0.2 pH units in the water column (Wahl et al., 2015a), representing only a small change in acidity for bacterial life under environmental conditions since most bacteria show a growth pH range of 2–3 units (e.g., neutrophiles 5.5–8.5 pH) (Madigan et al., 2009). In a similar spring experiment, we observed only a minor role of elevated pCO2 on bacterial communities on Wadden Sea F. mytili and in the surrounding North Sea water (Mensch et al., 2016). The alpha diversity of Baltic F. vesiculosus biofilm and seawater samples appeared not to be affected by the applied single and combined pCO2 and warming treatments across all seasons, consistent with previous results reported by Mensch et al. (2016).
Another interesting aspect is, that the microenvironment (including various types of microorganisms) of an algal surface is delimited by a diffusive boundary layer that is characterized by a fluctuating pH gradient. Extreme diel fluctuations about 1.5 pH units (i.e., 20 times stronger than the implemented acidification treatment) were measured within the epibacterial layer of Baltic F. vesiculosus (Wahl et al., 2015b). In addition, F. vesiculosus and its associated bacteria are already adapted to high fluctuations in pH/pCO2 conditions along the coasts of the western Baltic Sea including the Kiel Fjord (Melzner et al., 2013; Saderne et al., 2013).
The benthocosm experiments showed, that the macroalgal host F. vesiculosus suffered from warming in summer but was unaffected in the other seasons (also compare related F. vesiculosus tissue data of Graiff et al., 2015), whereas its biotic stressors (grazers and epiphytes) generally benefited from warming, except in summer when the grazers suffered but the epiphytes benefited (Graiff et al., 2015; Werner et al., 2016; Wahl et al., 2019). The bacterial communities on F. vesiculosus and in the surrounding seawater were affected by warming in all seasons but autumn. Since the biofilm is influenced by both its environment and its living host, the warming impacts on the epibacterial communities of F. vesiculosus might be a combination of direct effects on the biofilm and indirect effects via the macroalgal host. It has been shown that microfouling pressure by prokaryotes varied over the year, and chemical fouling control via surface metabolites of Baltic F. vesiculosus is fluctuating seasonally in situ with different abiotic factors (e.g., temperature) influencing the fouling control (Wahl et al., 2010; Saha and Wahl, 2013; Saha et al., 2014; Rickert et al., 2016a, b). In field studies, microfouling pressure was highest during summer (August) coinciding with a strengthened microfouling control observed from F. vesiculosus surface extracts (Rickert et al., 2016a, b). During summer, we noticed a threefold increase of iOTUs for +T on F. vesiculosus. Elevated temperatures during summer might disrupt the microfouling control in thermally stressed F. vesiculosus (Rickert et al., 2016a) resulting in an increase of bacteria related to intensified fouling and potential pathogenic species on the macroalgal surface.
(IV) Several Bacteria of Potential Ecological Importance Suffered or Benefited From Warming
Among the iOTUs for ambient temperature were bacteria that were typically associated with a broad variety of marine hosts. Comparable high (≥Δ-10‰) differences in mean relative abundance between both temperature levels were detected among several iOTUs for ambient temperature, suggesting that these bacteria strongly suffer from warming, since they are dominant at ambient but (nearly) absent at the warming treatment. Among the iOTUs for ambient temperature on F. vesiculosus were the genus Luteolibacter known to include isolates from red algae (Yoon et al., 2008); the genus Rickettsia containing intracellular symbionts of eukaryotes reported for a wide range of eukaryotic hosts (Weinert et al., 2009); Psychroserpens mesophilus once isolated from a marine biofilm (Kwon et al., 2006); the budding bacterium Hirschia baltica originally isolated from brackish surface water of the Kiel Fjord (Schlesner et al., 1990) and R. crustatorum once isolated from a marine crustacean (Yoon et al., 2012). Additionally, A. cylindrica was markedly more abundant at ambient temperature on F. vesiculosus, in particular during summer when up to 29 and 34°C were reached in the ambient and increased temperature treatments, respectively. This in turn might confirm the temperature sensitivity of this nitrogen-fixing heterocystous cyanobacterium since under laboratory conditions increased temperatures of 37–40°C inhibited N2 fixation (Gallon et al., 1993). During summer, “Ca. Portiera” was an indicator for ambient temperature in seawater, consistent with our benthic mesocosm study at the North Sea during spring (Mensch et al., 2016). The genus “Ca. Portiera” (Bing et al., 2013) was discussed as error in the Greengenes database (Campbell et al., 2015). However, our data provide strong evidence that “Ca. Portiera” naturally occurs in marine habitats because it matched within the order Oceanospirillales reported to contain intracellular bacteria (Brenner et al., 2005a; Jensen et al., 2010). Additional iOTUs for ambient temperature belonged to the genera Persicirhabdus that appears nearly ubiquitous in the oceans (Yoon et al., 2008; Freitas et al., 2012), and Roseibacillus containing a strain isolated from a brown alga (Yoon et al., 2008). Together, these observations underline that several naturally occurring bacterial taxa in seawater as well as attached to macroalgal surfaces suffered from warming in this study.
In particular during spring and summer, iOTUs for increased temperature included bacteria that exhibit antifouling activity against macrofoulers, and that might represent potential pathogenic or parasitic bacteria. However, no evidence was found for potential pathogens at increased temperature during winter, possibly because anti-microfouling defense activity by F. vesiculosus was higher during the winter experiment under warming (see related data by Raddatz et al., 2017), and field observations on Baltic F. vesiculosus reported prokaryotic fouling to be lowest during winter months (Rickert et al., 2016a). Nevertheless, during winter, iOTUs that were favored by warming contained genera playing roles in fouling under high temperature and degradation/decomposition processes, like Leadbetterella (Weon et al., 2005) or the flavobacterial genus Formosa known for strains with algal-associated degradation potential (Ivanova et al., 2004; Nedashkovskaya et al., 2006; Mann et al., 2013). Proteobacterial “Ca. Endobugula” bacteria are symbionts of marine bryozoan (Lim and Haygood, 2004; Lim-Fong et al., 2008), in turn implying that warming during winter supported bryozoan growth as detected by increased numbers of their bacterial symbionts. Further, Umboniibacter species living in marine animals are known to be involved in the digestion of plant material and showed favored growth at temperatures above 5°C (Romanenko et al., 2010), consistent with the temperature reached in the warming treatments during the winter experiment (5–15°C range at +T).
Some iOTUs for increased temperature were found in both habitat types during spring and summer, like the epsilonproteobacterial genus Arcobacter that also include animal pathogens, besides non pathogenic free-living species in various habitats (Brenner et al., 2005b; Miller et al., 2007). During summer, an interesting epibacterial iOTU for increased temperature was W. poriferorum (Lau et al., 2005) known for antifouling potential including inhibitory effects on marine biofilm-forming bacteria and larval settlement of macrofoulers (Dash et al., 2009, 2011). That in turn could be important features on the surface of F. vesiculosus to stabilize its microbiota under high fouling pressure due to warming in summer. Some iOTUs for increased temperature belonged to groups containing single species associated with diseases such as pathogenic mycobacteria (uncl. Mycobacterium) known to be distributed also in seawater often causing infections of humans and animals (Dufour, 2004), and Acholeplasma within the class Mollicutes that include surface-associated pathogenic or saprotrophic species (characterized by lysotrophic nutrition, a process of chemoheterotrophic extracellular digestion involved in the processing of dead or decayed organic matter) (Krieg et al., 2010). The higher abundance of Acholeplasma under warming during summer might be connected with the temperature-related decay of F. vesiculosus in our benthocosm experiments (Graiff et al., 2015). As an overall observation, higher abundances of bacteria involved in intensified microfouling processes appear to correlate with a reduced Baltic F. vesiculosus biomass under warming in spring and summer (Graiff et al., 2015). Thus, our benthocosm studies suggest a strong connection between a thermally stressed macroalgal host (along with a reduced defense activity due to dying tissue and probably bacterial infections) with favored growth of bacteria associated with intensified microfouling.
Conclusion
This study shows that increased temperature was a driving abiotic factor in reshaping bacterial communities on Baltic F. vesiculosus and in the surrounding seawater, and that elevated pCO2 had no direct impact, although in nature both climate phenomena – ocean acidification and global warming – are linked. Our detected temperature related iOTUs suggest that warming reduces commonly present and health-associated bacteria, but might also support growth of potential pathogenic bacteria, and favors bacteria that play a role in microfouling processes. This might lead to bacterial community restructuring with potentially severe consequences for the F. vesiculosus holobiont ultimately affecting the entire marine ecosystem.
Data Availability Statement
The datasets generated for this study can be found in the NCBI Sequence Read Archive under accession number SRP075254 (https://www.ncbi.nlm.nih.gov/sra/?term=SRP075254) and at the PANGAEA® data platform (https://doi.pangaea.de/10.1594/PANGAEA.842739).
Author Contributions
BM designed the study, carried out the sampling and sample processing, analyzed and interpreted the data, and wrote the manuscript. SN performed the statistical analyses on the sequencing data. SK sequenced the amplicon samples. RS and MW designed the experiments, contributed the comments on the manuscript, founded, and supervised the work.
Funding
This study was performed in the framework of BIOACID II (Biological Impacts of Ocean Acidification, Phase II, 03F0655M/Schmitz-Streit) and financially supported by the Federal Ministry of Education and Research (BMBF, Germany). This publication was financially supported by the Open Access Publication Fund by the German Research Foundation (DFG), Kiel University.
Conflict of Interest
SN was employed by the company omics2view.consulting GbR, Kiel, Germany.
The remaining authors declare that the research was conducted in the absence of any commercial or financial relationships that could be construed as a potential conflict of interest.
Acknowledgments
We are grateful to all colleagues of the BIOACID II consortium “Benthic Assemblages” for their cooperation and support in the benthocosm experiments. We are grateful to B. Buchholz for recording the water parameters and maintaining technical support of the benthocosm facility. We thank M. A. Fischer for support in raw data processing.
Supplementary Material
The Supplementary Material for this article can be found online at: https://www.frontiersin.org/articles/10.3389/fmicb.2020.01471/full#supplementary-material
Footnotes
References
Albrecht, A. S. (1998). Soft bottom versus hard rock: community ecology of macroalgae on intertidal mussel beds in the Wadden Sea. J. Exp. Mar. Biol. Ecol. 229, 85–109. doi: 10.1016/S0022-0981(98)00044-6
Armstrong, E., Yan, L., Boyd, K. G., Wright, P. C., and Burgess, J. G. (2001). The symbiotic role of marine microbes on living surfaces. Hydrobiologia 461, 37–40. doi: 10.1023/A:1012756913566
Beman, J. M., Chow, C.-E., King, A. L., Feng, Y., Fuhrman, J. A., Andersson, A., et al. (2011). Global declines in oceanic nitrification rates as a consequence of ocean acidification. Proc. Natl. Acad. Sci. U.S.A. 108, 208–213. doi: 10.1073/pnas.1011053108
Bergen, B., Endres, S., Engel, A., Zark, M., Dittmar, T., Sommer, U., et al. (2016). Acidification and warming affect prominent bacteria in two seasonal phytoplankton bloom mesocosms. Environ. Microbiol. 18, 4579–4595. doi: 10.1111/1462-2920.13549
Bing, X.-L., Yang, J., Zchori-Fein, E., Wang, X.-W., and Liu, S.-S. (2013). Characterization of a newly discovered symbiont of the whitefly Bemisia tabaci (Hemiptera Aleyrodidae). Appl. Environ. Microbiol. 79, 569–575. doi: 10.1128/AEM.03030-12
Bolger, A. M., Lohse, M., and Usadel, B. (2014). Trimmomatic: a flexible trimmer for Illumina sequence data. Bioinformatics 30, 2114–2120. doi: 10.1093/bioinformatics/btu170
Bosch, T. C. G., and McFall-Ngai, M. J. (2011). Metaorganisms as the new frontier. Zoology 114, 185–190. doi: 10.1016/j.zool.2011.04.001
Braeckman, U., van Colen, C., Guilini, K., van Gansbeke, D., Soetaert, K., Vincx, M., et al. (2014). Empirical evidence reveals seasonally dependent reduction in nitrification in coastal sediments subjected to near future ocean acidification. PLoS One 9:e108153. doi: 10.1371/journal.pone.0108153
Brenner, D. J., Krieg, N. R., and Staley, J. T. (eds) (2005a). Bergey’s Manual® of Systematic Bacteriology (Second Edition): Volume Two The Proteobacteria, Part B The Gammaproteobacteria. Berlin: Springer.
Brenner, D. J., Krieg, N. R., and Staley, J. T. (eds) (2005b). Bergey’s Manual® of Systematic Bacteriology (Second Edition): Volume Two The Proteobacteria, Part C The Alpha-, Beta-, Delta-, and Epsilonproteobacteria. Berlin: Springer.
Cáceres, M. D., and Legendre, P. (2009). Associations between species and groups of sites: indices and statistical inference. Ecology 90, 3566–3574. doi: 10.1890/08-1823.1
Campbell, A. M., Fleisher, J., Sinigalliano, C., White, J. R., and Lopez, J. V. (2015). Dynamics of marine bacterial community diversity of the coastal waters of the reefs, inlets, and wastewater outfalls of southeast Florida. MicrobiologyOpen 4, 390–408. doi: 10.1002/mbo3.245
Chen, H. (2018). VennDiagram: Generate High-Resolution Venn and Euler Plots. Available online at: https://CRAN.R-project.org/package=VennDiagram
Csárdi, G., and Nepusz, T. (2006). The igraph software package for complex network research. Inter. J. Complex Syst. 1695, 1–9.
Dang, H., and Lovell, C. R. (2016). Microbial surface colonization and biofilm development in marine environments. Microbiol. Mol. Biol. Rev. 80, 91–138. doi: 10.1128/MMBR.00037-15
Dash, S., Jin, C., Lee, O. O., Xu, Y., and Qian, P.-Y. (2009). Antibacterial and antilarval-settlement potential and metabolite profiles of novel sponge-associated marine bacteria. J. Indus. Microbiol. Biotechnol. 36, 1047–1056. doi: 10.1007/s10295-009-0588-x
Dash, S., Nogata, Y., Zhou, X., Zhang, Y., Xu, Y., Guo, X., et al. (2011). Poly-ethers from Winogradskyella poriferorum: antifouling potential, time-course study of production and natural abundance. Bioresour. Technol. 102, 7532–7537. doi: 10.1016/j.biortech.2011.05.034
Dittami, S. M., Duboscq-Bidot, L., Perennou, M., Gobet, A., Corre, E., Boyen, C., et al. (2016). Host–microbe interactions as a driver of acclimation to salinity gradients in brown algal cultures. ISME J. 10, 51–63. doi: 10.1038/ismej.2015.104
Dufour, A. (2004). Pathogenic Mycobacteria in Water: A Guide to Public Health Consequences, Monitoring and Management. Geneva: World Health Organization.
Edgar, R. C., Haas, B. J., Clemente, J. C., Quince, C., and Knight, R. (2011). UCHIME improves sensitivity and speed of chimera detection. Bioinformatics 27, 2194–2200. doi: 10.1093/bioinformatics/btr381
Egan, S., Harder, T., Burke, C., Steinberg, P., Kjelleberg, S., and Thomas, T. (2013). The seaweed holobiont: understanding seaweed–bacteria interactions. FEMS Microbiol. Rev. 37, 462–476. doi: 10.1111/1574-6976.12011
Elken, J., Lehmann, A., and Myrberg, K. (2015). “Recent change—marine circulation and stratification,” in Second Assessment of Climate Change for the Baltic Sea Basin, Ed. The BACC II Author Team (Berlin: Springer), 131–144. doi: 10.1007/978-3-319-16006-1_7
Ewing, B., and Green, P. (1998). Base-calling of automated sequencer traces using phred. II. Error probabilities. Genome Res. 8, 186–194. doi: 10.1101/gr.8.3.186
Faust, K., and Raes, J. (2012). Microbial interactions: from networks to models. Nat. Rev. Microbiol. 10:538. doi: 10.1038/nrmicro2832
Fortunato, C. S., Eiler, A., Herfort, L., Needoba, J. A., Peterson, T. D., and Crump, B. C. (2013). Determining indicator taxa across spatial and seasonal gradients in the Columbia River coastal margin. ISME J. 7, 1899–1911. doi: 10.1038/ismej.2013.79
Freitas, S., Hatosy, S., Fuhrman, J. A., Huse, S. M., Welch, D. B. M., Sogin, M. L., et al. (2012). Global distribution and diversity of marine Verrucomicrobia. ISME J. 6, 1499–1505. doi: 10.1038/ismej.2012.3
Fuhrman, J. A., Cram, J. A., and Needham, D. M. (2015). Marine microbial community dynamics and their ecological interpretation. Nat. Rev. Microbiol. 13, 133–146. doi: 10.1038/nrmicro3417
Gallon, J. R., Pederson, D. M., and Smith, G. D. (1993). The effect of temperature on the sensitivity of nitrogenase to oxygen in the cyanobacteria Anabaena cylindrica (Lemmermann) and Gloeothece (Nägeli). New Phytol. 124, 251–257. doi: 10.1111/j.1469-8137.1993.tb03814.x
Gazeau, F., van Rijswijk, P., Pozzato, L., and Middelburg, J. J. (2014). Impacts of ocean acidification on sediment processes in shallow waters of the Arctic Ocean. PLoS One 9:e94068. doi: 10.1371/journal.pone.0094068
Goecke, F., Labes, A., Wiese, J., and Imhoff, J. F. (2010). Chemical interactions between marine macroalgae and bacteria. Mar. Ecol. Progr. Ser. 409, 267–299. doi: 10.3354/meps08607
Graiff, A., Bartsch, I., Ruth, W., Wahl, M., and Karsten, U. (2015). Season exerts differential effects of ocean acidification and warming on growth and carbon metabolism of the seaweed Fucus vesiculosus in the western Baltic Sea. Front. Mar. Sci 2:112. doi: 10.3389/fmars.2015.00112
Gräwe, U., Friedland, R., and Burchard, H. (2013). The future of the western Baltic Sea: two possible scenarios. Ocean Dyn. 63, 901–921. doi: 10.1007/s10236-013-0634-0
Hehemann, J.-H., Smyth, L., Yadav, A., Vocadlo, D. J., and Boraston, A. B. (2012). Analysis of keystone enzyme in Agar hydrolysis provides insight into the degradation (of a polysaccharide from) red seaweeds. J. Biol. Chem. 287, 13985–13995. doi: 10.1074/jbc.M112.345645
IPCC (2013). Climate Change 2013: The Physical Science Basis. Contribution of Working Group I to the Fifth Assessment Report of the Intergovernmental Panel on Climate Change, eds T. F. Stocker, D. Qin, G.-K. Plattner, M. Tignor, S. K. Allen, J. Boschung, et al. (Cambridge: Cambridge University Press).
Ivanova, E. P., Alexeeva, Y. V., Flavier, S., Wright, J. P., Zhukova, N. V., Gorshkova, N. M., et al. (2004). Formosa algae gen. nov., sp. nov., a novel member of the family Flavobacteriaceae. Int. J. Syst. Evol. Microbiol. 54, 705–711. doi: 10.1099/ijs.0.02763-0
Jensen, S., Duperron, S., Birkeland, N.-K., and Hovland, M. (2010). Intracellular Oceanospirillales bacteria inhabit gills of Acesta bivalves. FEMS Microbiol. Ecol. 74, 523–533. doi: 10.1111/j.1574-6941.2010.00981.x
Jost, L. (2007). Partitioning diversity into independent alpha and beta components. Ecology 88, 2427–2439. doi: 10.1890/06-1736.1
Kautsky, N., Kautsky, H., Kautsky, U., and Waern, M. (1986). Decreased depth penetration of Fucus vesiculosus (L.) since the 1940’s indicates eutrophication of the Baltic Sea. Mar. Ecol. Prog. Ser. 28, 1–8. doi: 10.3354/meps028001
Kitidis, V., Laverock, B., McNeill, L. C., Beesley, A., Cummings, D., Tait, K., et al. (2011). Impact of ocean acidification on benthic and water column ammonia oxidation. Geophys. Res. Lett. 38:L21603. doi: 10.1029/2011GL049095
Kozich, J. J., Westcott, S. L., Baxter, N. T., Highlander, S. K., and Schloss, P. D. (2013). Development of a dual-index sequencing strategy and curation pipeline for analyzing amplicon sequence data on the MiSeq Illumina Sequencing Platform. Appl. Environ. Microbiol. 79, 5112–5120. doi: 10.1128/Aem.01043-13
Krause, E., Wichels, A., Giménez, L., Lunau, M., Schilhabel, M. B., and Gerdts, G. (2012). Small changes in pH have direct effects on marine bacterial community composition: a microcosm approach. PLoS One 7:e47035. doi: 10.1371/journal.pone.0047035
Krieg, N. R., Staley, J. T., Brown, D. R., Hedlund, B. P., Paster, B. J., and Ward, N. L., et al. (eds) (2010). Bergey’s Manual® of Systematic Bacteriology (Second Edition): Volume Four The Bacteroidetes, Spirochaetes, Tenericutes (Mollicutes), Acidobacteria, Fibrobacteres, Fusobacteria, Dictyoglomi, Gemmatimonadetes, Lentisphaerae, Verrucomicrobia, Chlamydiae, and Planctomycetes. New York, NY: Springer.
Kurtz, Z. D., Müller, C. L., Miraldi, E. R., Littman, D. R., Blaser, M. J., and Bonneau, R. A. (2015). Sparse and compositionally robust inference of microbial ecological networks. PLoS Comput. Biol. 11:e1004226. doi: 10.1371/journal.pcbi.1004226
Kwon, K. K., Lee, S. J., Park, J. H., Ahn, T.-Y., and Lee, H. K. (2006). Psychroserpens mesophilus sp. nov., a mesophilic marine bacterium belonging to the family Flavobacteriaceae isolated from a young biofilm. Int. J. Syst. Evol. Microbiol. 56, 1055–1058. doi: 10.1099/ijs.0.64171-0
Lachnit, T., Blümel, M., Imhoff, J. F., and Wahl, M. (2009a). Specific epibacterial communities on macroalgae: phylogeny matters more than habitat. Aquatic Biol. 5, 181–186. doi: 10.3354/ab00149
Lachnit, T., Wahl, M., and Harder, T. (2009b). Isolated thallus-associated compounds from the macroalga Fucus vesiculosus mediate bacterial surface colonization in the field similar to that on the natural alga. Biofouling 26, 247–255. doi: 10.1080/08927010903474189
Lachnit, T., Fischer, M., Künzel, S., Baines, J. F., and Harder, T. (2013). Compounds associated with algal surfaces mediate epiphytic colonization of the marine macroalga Fucus vesiculosus. FEMS Microbiol. Ecol. 84, 411–420. doi: 10.1111/1574-6941.12071
Lachnit, T., Meske, D., Wahl, M., Harder, T., and Schmitz, R. (2011). Epibacterial community patterns on marine macroalgae are host-specific but temporally variable. Environ. Microbiol. 13, 655–665. doi: 10.1111/j.1462-2920.2010.02371.x
Lau, S. C. K., Tsoi, M. M. Y., Li, X., Plakhotnikova, I., Dobretsov, S., Lau, K. W. K., et al. (2005). Winogradskyella poriferorum sp. nov., a novel member of the family Flavobacteriaceae isolated from a sponge in the Bahamas. Int. J. Syst. Evol. Microbiol. 55, 1589–1592. doi: 10.1099/ijs.0.63661-0
Laverock, B., Kitidis, V., Tait, K., Gilbert, J. A., Osborn, A. M., and Widdicombe, S. (2013). Bioturbation determines the response of benthic ammonia-oxidizing microorganisms to ocean acidification. Philos. Trans. R. Soc. B 368:20120441. doi: 10.1098/rstb.2012.0441
Lim, G. E., and Haygood, M. G. (2004). “Candidatus Endobugula glebosa,” a specific bacterial symbiont of the marine bryozoan Bugula simplex. Appl. Environ. Microbiol. 70, 4921–4929. doi: 10.1128/AEM.70.8.4921-4929.2004
Lim-Fong, G. E., Regali, L. A., and Haygood, M. G. (2008). Evolutionary relationships of “Candidatus Endobugula” bacterial symbionts and their Bugula bryozoan hosts. Appl. Environ. Microbiol. 74, 3605–3609. doi: 10.1128/AEM.02798-07
Lindh, M. V., Riemann, L., Baltar, F., Romero-Oliva, C., Salomon, P. S., Granéli, E., et al. (2013). Consequences of increased temperature and acidification on bacterioplankton community composition during a mesocosm spring bloom in the Baltic Sea. Environ. Microbiol. Rep. 5, 252–262. doi: 10.1111/1758-2229.12009
Lomas, M. W., Hopkinson, B. M., Ryan, D. E., Shi, D. L., Xu, Y., and Morel, F. M. M. (2012). Effect of ocean acidification on cyanobacteria in the subtropical North Atlantic. Aquatic Microb. Ecol. 66, 211–222. doi: 10.3354/ame01576
Madigan, M. T., Martinko, J. M., Dunlap, P. V., and Clark, D. P. (2009). BROCK Biology of Microorganisms, 12th Edn. San Francisco, CA: Pearson Education, Inc.
Mann, A. J., Hahnke, R. L., Huang, S., Werner, J., Xing, P., Barbeyron, T., et al. (2013). The genome of the alga-associated marine flavobacterium Formosa agariphila KMM 3901T reveals a broad potential for degradation of algal polysaccharides. Appl. Environ. Microbiol. 79, 6813–6822. doi: 10.1128/AEM.01937-13
Martin, M., Barbeyron, T., Martin, R., Portetelle, D., Michel, G., and Vandenbol, M. (2015). The cultivable surface microbiota of the brown alga Ascophyllum nodosum is enriched in macroalgal-polysaccharide-degrading bacteria. Front. Microbiol. 6:1487. doi: 10.3389/fmicb.2015.01487
Martin, M., Portetelle, D., Michel, G., and Vandenbol, M. (2014). Microorganisms living on macroalgae: diversity, interactions, and biotechnological applications. Appl. Microbiol. Biotechnol. 98, 2917–2935. doi: 10.1007/s00253-014-5557-2
Meinshausen, N., and Bühlmann, P. (2006). High-dimensional graphs and variable selection with the Lasso. Ann. Statist. 34, 1436–1462. doi: 10.1214/009053606000000281
Melzner, F., Thomsen, J., Koeve, W., Oschlies, A., Gutowska, M. A., Bange, H. W., et al. (2013). Future ocean acidification will be amplified by hypoxia in coastal habitats. Mar. Biol. 160, 1875–1888. doi: 10.1007/s00227-012-1954-1
Mensch, B., Neulinger, S. C., Graiff, A., Pansch, A., Künzel, S., Fischer, M. A., et al. (2016). Restructuring of epibacterial communities on fucus vesiculosus forma mytili in response to elevated pCO2 and increased temperature levels. Front. Microbiol. 7:434. doi: 10.3389/fmicb.2016.00434
Miller, W. G., Parker, C. T., Rubenfield, M., Mendz, G. L., Wösten, M. M. S. M., Ussery, D. W., et al. (2007). The complete genome sequence and analysis of the epsilonproteobacterium Arcobacter butzleri. PLoS One 2:e1358. doi: 10.1371/journal.pone.0001358
Nasrolahi, A., Stratil, S. B., Jacob, K. J., and Wahl, M. (2012). A protective coat of microorganisms on macroalgae: inhibitory effects of bacterial biofilms and epibiotic microbial assemblages on barnacle attachment. FEMS Microbiol. Ecol. 81, 583–595. doi: 10.1111/j.1574-6941.2012.01384.x
Nedashkovskaya, O. I., Kim, S. B., Vancanneyt, M., Snauwaert, C., Lysenko, A. M., Rohde, M., et al. (2006). Formosa agariphila sp. nov., a budding bacterium of the family Flavobacteriaceae isolated from marine environments, and emended description of the genus Formosa. Int. J. Syst. Evol. Microbiol. 56, 161–167. doi: 10.1099/ijs.0.63875-0
Newbold, L. K., Oliver, A. E., Booth, T., Tiwari, B., DeSantis, T., Maguire, M., et al. (2012). The response of marine picoplankton to ocean acidification. Environ. Microbiol. 14, 2293–2307. doi: 10.1111/j.1462-2920.2012.02762.x
Oliver, A. E., Newbold, L. K., Whiteley, A. S., and van der Gast, C. J. (2014). Marine bacterial communities are resistant to elevated carbon dioxide levels. Environ. Microbiol. Rep, 6, 574–582. doi: 10.1111/1758-2229.12159
Omstedt, A., Edman, M., Claremar, B., Frodin, P., Gustafsson, E., Humborg, C., et al. (2012). Future changes in the Baltic Sea acid–base (pH) and oxygen balances. Tellus B Chem. Phys. Meteorol. 64:19586. doi: 10.3402/tellusb.v64i0.19586
Pansch, A., Winde, V., Asmus, R., and Asmus, H. (2016). Tidal benthic mesocosms simulating future climate change scenarios in the field of marine ecology. Limnol. Oceanogr. 14, 257–267. doi: 10.1002/lom3.10086
Parrot, D., Blümel, M., Utermann, C., Chianese, G., Krause, S., Kovalev, A., et al. (2019). Mapping the surface microbiome and metabolome of brown seaweed Fucus vesiculosus by amplicon sequencing, integrated metabolomics and imaging techniques. Sci. Rep. 9:1061. doi: 10.1038/s41598-018-37914-8
Pruesse, E., Quast, C., Knittel, K., Fuchs, B. M., Ludwig, W. G., Peplies, J., et al. (2007). SILVA: a comprehensive online resource for quality checked and aligned ribosomal RNA sequence data compatible with ARB. Nucleic Acids Res. 35, 7188–7196. doi: 10.1093/nar/gkm864
Qiu, Z., Coleman, M. A., Provost, E., Campbell, A. H., Kelaher, B. P., Dalton, S. J., et al. (2019). Future climate change is predicted to affect the microbiome and condition of habitat-forming kelp. Proc. R. Soc. B 286:20181887. doi: 10.1098/rspb.2018.1887
Raddatz, S., Guy-Haim, T., Rilov, G., and Wahl, M. (2017). Future warming and acidification effects on anti-fouling and anti-herbivory traits of the brown alga Fucus vesiculosus (Phaeophyceae). J. Phycol. 53, 44–58. doi: 10.1111/jpy.12473
Rickert, E., Lenz, M., Barboza, F. R., Gorb, S. N., and Wahl, M. (2016a). Seasonally fluctuating chemical microfouling control in Fucus vesiculosus and Fucus serratus from the Baltic Sea. Mar. Biol. 163:203. doi: 10.1007/s00227-016-2970-3
Rickert, E., Wahl, M., Link, H., Richter, H., and Pohnert, G. (2016b). Seasonal Variations in surface metabolite composition of Fucus vesiculosus and Fucus serratus from the Baltic Sea. PLoS One 11:e0168196. doi: 10.1371/journal.pone.0168196
Romanenko, L. A., Tanaka, N., and Frolova, G. M. (2010). Umboniibacter marinipuniceus gen. nov., sp. nov., a marine gammaproteobacterium isolated from the mollusc Umbonium costatum from the Sea of Japan. Int. J. Syst. Evol. Microbiol. 60, 603–609. doi: 10.1099/ijs.0.010728-0
Rönnbäck, P., Kautsky, N., Pihl, L., Troell, M., Söderqvist, T., and Wennhage, H. (2007). Ecosystem goods and services from Swedish coastal habitats: identification, valuation, and implications of ecosystem shifts. AMBIO 36, 534–545. doi: 10.1579/0044-7447200736[534:EGASFS]2.0.CO;2
Roy, A.-S., Gibbons, S. M., Schunck, H., Owens, S., Caporaso, J. G., Sperling, M., et al. (2013). Ocean acidification shows negligible impacts on high-latitude bacterial community structure in coastal pelagic mesocosms. Biogeosciences 10, 555–566. doi: 10.5194/bg-10-555-2013
Saderne, V., Fietzek, P., and Herman, P. M. J. (2013). Extreme variations of pCO2 and pH in a macrophyte meadow of the Baltic Sea in summer: evidence of the effect of photosynthesis and local upwelling. PLoS One 8:e62689. doi: 10.1371/journal.pone.0062689
Saha, M., Goecke, F., and Bhadury, P. (2017). Minireview: algal natural compounds and extracts as antifoulants. J. Appl. Phycol. 30, 1859–1874. doi: 10.1007/s10811-017-1322-0
Saha, M., Rempt, M., Gebser, B., Grueneberg, J., Pohnert, G., and Weinberger, F. (2012). Dimethylsulphopropionate (DMSP) and proline from the surface of the brown alga Fucus vesiculosus inhibit bacterial attachment. Biofouling 28, 593–604. doi: 10.1080/08927014.2012.698615
Saha, M., Rempt, M., Grosser, K., Pohnert, G., and Weinberger, F. (2011). Surface-associated fucoxanthin mediates settlement of bacterial epiphytes on the rockweed Fucus vesiculosus. Biofouling 27, 423–433. doi: 10.1080/08927014.2011.580841
Saha, M., Rempt, M., Stratil, S. B., Wahl, M., Pohnert, G., and Weinberger, F. (2014). Defence chemistry modulation by light and temperature shifts and the resulting effects on associated epibacteria of Fucus vesiculosus. PLoS One 9:e105333. doi: 10.1371/journal.pone.0105333
Saha, M., and Wahl, M. (2013). Seasonal variation in the antifouling defence of the temperate brown alga Fucus vesiculosus. Biofouling 29, 661–668. doi: 10.1080/08927014.2013.795953
Saha, M., and Weinberger, F. (2019). Microbial “gardening” by a seaweed holobiont: surface metabolites attract protective and deter pathogenic epibacterial settlement. J. Ecol. 107, 2255–2265. doi: 10.1111/1365-2745.13193
Sarkar, D. (2008). Lattice: Multivariate data visualization with R. New York, NY: Springer Science & Business Media.
Satheesh, S., Ba-akdah, M. A., and Al-Sofyani, A. A. (2016). Natural antifouling compound production by microbes associated with marine macroorganisms: a review. Electr. J. Biotechnol. 19, 26–35. doi: 10.1016/j.ejbt.2016.02.002
Schlesner, H., Bartels, C., Sittig, M., Dorsch, M., and Stackebrandt, E. (1990). Taxonomic and Phylogenetic Studies on a New Taxon of Budding. Hyphal Proteobacteria, Hirschia baltica gen. nov., sp. nov. Int. J. Syst. Evol. Microbiol. 40, 443–451. doi: 10.1099/00207713-40-4-443
Schloss, P. D., Westcott, S. L., Ryabin, T., Hall, J. R., Hartmann, M., Hollister, E. B., et al. (2009). Introducing mothur: open-source, platform-independent, community-supported software for describing and comparing microbial communities. Appl. Environ. Microbiol. 75, 7537–7541. doi: 10.1128/Aem.01541-09
Singh, R. P., and Reddy, C. R. K. (2014). Seaweed–microbial interactions: key functions of seaweed-associated bacteria. FEMS Microbiol. Ecol. 88, 213–230. doi: 10.1111/1574-6941.12297
Steele, J. A., Countway, P. D., Xia, L., Vigil, P. D., Beman, J. M., Kim, D. Y., et al. (2011). Marine bacterial, archaeal and protistan association networks reveal ecological linkages. ISME J. 5:1414. doi: 10.1038/ismej.2011.24
Steinberg, P. D., and de Nys, R. (2002). Chemical mediation of colonization of seaweed surfaces. J. Phycol. 38, 621–629. doi: 10.1046/j.1529-8817.2002.02042.x
Stewart, R. I. A., Dossena, M., Bohan, D. A., Jeppesen, E., Kordas, R. L., Ledger, M. E., et al. (2013). “Mesocosm experiments as a tool for ecological climate-change research,” in Advances in Ecological Research, Ed. H. Caswell (Amsterdam: Elsevier), 71–181. doi: 10.1016/b978-0-12-417199-2.00002-1
Stratil, S. B., Neulinger, S. C., Knecht, H., Friedrichs, A. K., and Wahl, M. (2013). Temperature-driven shifts in the epibiotic bacterial community composition of the brown macroalga Fucus vesiculosus. MicrobiologyOpen 2, 338–349. doi: 10.1002/mbo3.79
Stratil, S. B., Neulinger, S. C., Knecht, H., Friedrichs, A. K., and Wahl, M. (2014). Salinity affects compositional traits of epibacterial communities on the brown macroalga Fucus vesiculosus. FEMS Microbiol. Ecol. 88, 272–279. doi: 10.1111/1574-6941.12292
Tait, K., Laverock, B., Shaw, J., Somerfield, P. J., and Widdicombe, S. (2013). Minor impact of ocean acidification to the composition of the active microbial community in an Arctic sediment. Environ. Microbiol. Rep. 5, 851–860. doi: 10.1111/1758-2229.12087
Tait, K., Laverock, B., and Widdicombe, S. (2014). Response of an Arctic sediment nitrogen cycling community to increased CO2. Estuar. Coasts 37, 724–735. doi: 10.1007/s12237-013-9709-x
Torn, K., Krause-Jensen, D., and Martin, G. (2006). Present and past depth distribution of bladderwrack (Fucus vesiculosus) in the Baltic Sea. Aquatic Bot. 84, 53–62. doi: 10.1016/j.aquabot.2005.07.011
van der Loos, L. M., Eriksson, B. K., and Falcão Salles, J. (2019). The macroalgal holobiont in a changing Sea. Trends Microbiol. 27, 635–650. doi: 10.1016/j.tim.2019.03.002
Vogt, H., and Schramm, W. (1991). Conspicuous decline of Fucus in Kiel Bay (western Baltic): what are the causes? Mar. Ecol. Progr. Ser. 69, 189–194. doi: 10.3354/meps069189
Wahl, M., Buchholz, B., Winde, V., Golomb, D., Guy-Haim, T., Müller, J., et al. (2015a). A mesocosm concept for the simulation of near-natural shallow underwater climates: the Kiel Outdoor Benthocosms (KOB). Limnol. Oceanogr. 13, 651–663. doi: 10.1002/lom3.10055
Wahl, M., Saderne, V., and Sawall, Y. (2015b). How good are we at assessing the impact of ocean acidification in coastal systems? Limitations, omissions and strengths of commonly used experimental approaches with special emphasis on the neglected role of fluctuations. Mar. Freshw. Res. 67, 25–36. doi: 10.1071/MF14154
Wahl, M., Goecke, F., Labes, A., Dobretsov, S., and Weinberger, F. (2012). The second skin: ecological role of epibiotic biofilms on marine organisms. Front. Microbiol. 3:292. doi: 10.3389/fmicb.2012.00292
Wahl, M., Shahnaz, L., Dobretsov, S., Saha, M., Symanowski, F., David, K., et al. (2010). Ecology of antifouling resistance in the bladder wrack Fucus vesiculosus: patterns of microfouling and antimicrobial protection. Mar. Ecol. Progr. Ser. 411, 33–48. doi: 10.3354/meps08644
Wahl, M., Werner, F. J., Buchholz, B., Raddatz, S., Graiff, A., Matthiessen, B., et al. (2019). Season affects strength and direction of the interactive impacts of ocean warming and biotic stress in a coastal seaweed ecosystem. Limnol. Oceanogr. 9999, 1–21. doi: 10.1002/lno.11350
Wang, Q., Garrity, G. M., Tiedje, J. M., and Cole, J. R. (2007). Naive Bayesian classifier for rapid assignment of rRNA sequences into the new bacterial taxonomy. Appl. Environ. Microbiol. 73, 5261–5267. doi: 10.1128/Aem.00062-07
Weinberger, F. (2007). Pathogen-induced defense and innate immunity in macroalgae. Biol. Bull. 213, 290–302. doi: 10.2307/25066646
Weinert, L. A., Werren, J. H., Aebi, A., Stone, G. N., and Jiggins, F. M. (2009). Evolution and diversity of Rickettsia bacteria. BMC Biol. 7:6. doi: 10.1186/1741-7007-7-6
Weon, H.-Y., Kim, B.-Y., Kwon, S.-W., Park, I.-C., Cha, I.-B., Tindall, B. J., et al. (2005). Leadbetterella byssophila gen. nov., sp. nov., isolated from cotton-waste composts for the cultivation of oyster mushroom. Int. J. Syst. Evol. Microbiol. 55, 2297–2302. doi: 10.1099/ijs.0.63741-0
Werner, F. J., Graiff, A., and Matthiessen, B. (2016). Temperature effects on seaweed-sustaining top-down control vary with season. Oecologia 180, 889–901. doi: 10.1007/s00442-015-3489-x
Wichard, T. (2015). Exploring bacteria-induced growth and morphogenesis in the green macroalga order Ulvales (Chlorophyta). Front. Plant Sci. 6:86. doi: 10.3389/fpls.2015.00086
Wiencke, C., and Bischof, K. (2012). Seaweed Biology: Novel Insights into Ecophysiology, Ecology and Utilization. Berlin: Springer Berlin Heidelberg.
Witt, V., Wild, C., Anthony, K. R. N., Diaz-Pulido, G., and Uthicke, S. (2011). Effects of ocean acidification on microbial community composition of, and oxygen fluxes through, biofilms from the Great Barrier Reef. Environ. Microbiol. 13, 2976–2989. doi: 10.1111/j.1462-2920.2011.02571.x
Yoon, J., Katsuta, A., and Kasai, H. (2012). Rubidimonas crustatorum gen. nov., sp. nov., a novel member of the family Saprospiraceae isolated from a marine crustacean. Antonie van Leeuwenhoek 101, 461–467. doi: 10.1007/s10482-011-9653-3
Yoon, J., Matsuo, Y., Adachi, K., Nozawa, M., Matsuda, S., Kasai, H., et al. (2008). Description of Persicirhabdus sediminis gen. nov., sp. nov., Roseibacillus ishigakijimensis gen. nov., sp. nov., Roseibacillus ponti sp. nov., Roseibacillus persicicus sp. nov., Luteolibacter pohnpeiensis gen. nov., sp. nov. and Luteolibacter algae sp. nov., six marine members of the phylum ‘Verrucomicrobia’, and emended descriptions of the class Verrucomicrobiae, the order Verrucomicrobiales and the family Verrucomicrobiaceae. Int. J. Syst. Evol. Microbiol. 58, 998–1007. doi: 10.1099/ijs.0.65520-0
Keywords: bacterial community structure, 16S rDNA amplicon sequencing, temperature, pCO2, benthocosm, macroalgal holobiont
Citation: Mensch B, Neulinger SC, Künzel S, Wahl M and Schmitz RA (2020) Warming, but Not Acidification, Restructures Epibacterial Communities of the Baltic Macroalga Fucus vesiculosus With Seasonal Variability. Front. Microbiol. 11:1471. doi: 10.3389/fmicb.2020.01471
Received: 18 December 2019; Accepted: 05 June 2020;
Published: 26 June 2020.
Edited by:
Anne Bernhard, Connecticut College, United StatesReviewed by:
Daniel P. R. Herlemann, Estonian University of Life Sciences, EstoniaXosé Anxelu G. Morán, King Abdullah University of Science and Technology, Saudi Arabia
Copyright © 2020 Mensch, Neulinger, Künzel, Wahl and Schmitz. This is an open-access article distributed under the terms of the Creative Commons Attribution License (CC BY). The use, distribution or reproduction in other forums is permitted, provided the original author(s) and the copyright owner(s) are credited and that the original publication in this journal is cited, in accordance with accepted academic practice. No use, distribution or reproduction is permitted which does not comply with these terms.
*Correspondence: Ruth A. Schmitz, cnNjaG1pdHpAaWZhbS51bmkta2llbC5kZQ==