- 1School of Medical Sciences, Faculty of Medicine and Health, The University of Sydney, Camperdown, NSW, Australia
- 2Tuberculosis Research Program, Centenary Institute, Sydney, NSW, Australia
- 3Centre for Inflammation, Centenary Institute, University of Technology, Sydney, NSW, Australia
- 4Faculty of Science, School of Life Sciences, University of Technology, Sydney, NSW, Australia
- 5Sydney Institute for Infectious Diseases and the Charles Perkins Centre, The University of Sydney, Camperdown, NSW, Australia
Mycobacterium abscessus is a member of the non-tuberculous mycobacteria (NTM) group, responsible for chronic infections in individuals with cystic fibrosis (CF) or those otherwise immunocompromised. While viewed traditionally as an opportunistic pathogen, increasing research into M. abscessus in recent years has highlighted its continued evolution into a true pathogen. This is demonstrated through an extensive collection of virulence factors (VFs) possessed by this organism which facilitate survival within the host, particularly in the harsh environment of the CF lung. These include VFs resembling those of other Mycobacteria, and non-mycobacterial VFs, both of which make a notable contribution in shaping M. abscessus interaction with the host. Mycobacterium abscessus continued acquisition of VFs is cause for concern and highlights the need for novel vaccination strategies to combat this pathogen. An effective M. abscessus vaccine must be suitably designed for target populations (i.e., individuals with CF) and incorporate current knowledge on immune correlates of protection against M. abscessus infection. Vaccination strategies must also build upon lessons learned from ongoing efforts to develop novel vaccines for other pathogens, particularly Mycobacterium tuberculosis (M. tb); decades of research into M. tb has provided insight into unconventional and innovative vaccine approaches that may be applied to M. abscessus. Continued research into M. abscessus pathogenesis will be critical for the future development of safe and effective vaccines and therapeutics to reduce global incidence of this emerging pathogen.
Introduction
The Mycobacterium abscessus clade are an emerging group of prominent human pathogens. Comprised of the three subspecies M. abscessus subsp. abscessus, M. abscessus subsp. bolletti, and M. abscessus subsp. massiliense and previously including closely related species Mycobacterium chelonae, these organisms are responsible for severe skin, soft tissue, and pulmonary infections (Adekambi et al., 2017; Ryan and Byrd, 2018). The M. abscessus clade is particularly problematic for individuals with enhanced susceptibility to pulmonary infection, such as those with bronchiectasis or chronic obstructive pulmonary disease (COPD), prior tuberculosis (TB) infection, or individuals with cystic fibrosis (CF; Griffith et al., 2007). The incidence of NTM infection in individuals with non-CF bronchiectasis is particularly high, with an estimated 37% of all patients with this condition having an NTM infection (Mirsaeidi et al., 2013). CF patients are particularly susceptible to NTM infection, with incidence rates in one study reported to be 1,000 times higher than in the general population (Martiniano et al., 2019). CF is a recessive genetic disorder affecting the cystic fibrosis transmembrane conductance receptor (CFTR) and resulting in dysregulated chloride transport, with a wide affect across different organs including the lung. Individuals with CF have dysregulated mucus production and are particularly susceptible to bacterial infections that become chronic and difficult to eradicate (Cantin et al., 2015). Mycobacterium abscessus infection is extremely difficult to treat due to intrinsic, adaptive, and acquired antibiotic resistance traits; these result in poor treatment success rates as low as 30% depending on the subspecies (Nessar et al., 2012; Koh et al., 2014). Furthermore, the establishment of chronic M. abscessus infection is associated with a significant decline in lung function, which has a profound impact on patient quality of life (Esther et al., 2010; Kwak et al., 2019). Despite the significant impact of M. abscessus infection on susceptible populations, there is currently no vaccine available (approved or within clinical trials) for M. abscessus. Given both the difficulty in treating M. abscessus and the poor prognosis following the establishment of persistent and chronic infection, there is an urgent need to develop novel prophylactic interventions to reduce incidence of M. abscessus infections in at-risk populations.
The development of novel vaccines for pathogens, such as M. abscessus, can be guided by the characterization of novel virulence factors (VFs), molecular components which facilitate pathogen survival and persistence in the host. Bacterial VFs promote pathogen resistance to immune defenses, improve adherence or invasion of host cells/tissues or enhance survival through modification of the host environment (Wu et al., 2008). The study of virulence determinants can identify targets for the attenuation of pathogens, or proteins which themselves may be sufficiently immunogenic to be formulated into a vaccine (Ottenhoff and Kaufmann, 2012). For example, M. tuberculosis antigen 85 complex proteins are VFs that promote bacterial entry into host cells; the immunogenic nature of these proteins has led to their inclusion in numerous recombinant, subunit, and viral vectored vaccines (Babaki et al., 2017). Although some VFs are unsuitable for incorporation into vaccines—due to poor immunogenicity or unsuitable cellular location for immune exposure—mechanistic studies of these VFs can shed light onto host responses to infection and thus informs future vaccine studies.
While traditionally viewed as an opportunistic pathogen, there is now compelling evidence that the M. abscessus clade possesses hallmark characteristics of a true pathogen. Work on M. abscessus in recent years has shed light on the unique disease pathogenesis and VFs possessed by this clade. While M. abscessus has multiple well-characterized mycobacterial VFs, it also possesses non-mycobacterial VFs which share high homology with other notable CF pathogens (Ripoll et al., 2009). Given the developments in our understanding of M. abscessus virulence in recent years, the purpose of this review is to summarize our current knowledge on M. abscessus VFs, as well as recent research efforts to develop vaccines against this emerging pathogen. An overview of M. abscessus biology and pathogenesis in the context of non-tuberculous mycobacteria (NTM) can be found in Johansen, Herrmann (Johansen et al., 2020).
Evolution of Mycobacterium abscessus Into a Human Pathogen
Many species of NTM are ubiquitous in both urban and natural environments. NTM are adept at survival in numerous habitats, such as soil, peats, and swamps, where the thick hydrophobic membrane of Mycobacterium spp. facilitates strong adherence to different surfaces and likely promotes survival in hostile environments (Falkinham, 2009). This resilience to different environmental conditions has allowed the permeation of NTM into urban settings, where species, such as M. abscessus, are isolated from potable water sources and plumbing systems (Thomson et al., 2013). Hospital outbreaks of NTM infections are frequently linked to contaminated water supplies (Tam et al., 2014; Guimarães et al., 2016); acquisition of infection from environmental sources, rather than from other infected individuals, is through to be the major transmission route by which M. abscessus gains entry to human hosts (Thomson et al., 2013). The prevalence of M. abscessus in nosocomial environments, combined with high-level resistance to many glutaraldehyde-based hospital-grade disinfectants (Burgess et al., 2017), has facilitated the establishment of opportunistic M. abscessus infections in both immunocompetent and immunocompromised individuals.
Given the incursion of M. abscessus into urban environments, such as plumbing systems and water sources, the occurrence of opportunistic NTM infections is unsurprising. However, the diverse array of VFs possessed by the M. abscessus clade points to a more complex evolution of this organism into a human pathogen. Importantly, NTM including M. abscessus have been isolated from free-living amoeba derived from urban water sources, suggesting a role for amoeba in enabling NTM persistence (Delafont et al., 2014). It has been proposed that early adaptations of M. abscessus to an intracellular amoeba lifestyle may have enhanced M. abscessus virulence and promoted survival in mammalian hosts (Thomas and McDonnell, 2007). Preculture of M. abscessus in Acanthamoeba castellanii amoeba enhances persistence in vivo in a murine model of infection (N’Goma et al., 2015). Mycobacterium abscessus also remain viable when encysted by amoeba, suggestive of both their adaptation to the intracellular lifestyle and the potential for amoeba to act as an environmental reservoir for M. abscessus (da Silva et al., 2018). Dubois et al. (2019) performed extensive transcriptomic analysis to track changes in gene expression induced in M. abscessus during intracellular growth. It was demonstrated that growth of M. abscessus in amoeba and macrophages induced similar patterns of differential gene expression, including an upregulation of genes to cope with intracellular stresses, such as heat shock and oxidative stress (e.g., GroEL-ES and hsp), a switch to slower growth phenotype, and utilization of fatty acids as an energy source. Following selection of M. abscessus genes upregulated in amoeba and subsequent expression of these genes in opportunistic pathogen M. chelonae, the authors showed enhanced growth of complemented M. chelonae in macrophages. This supports the theory that growth of M. abscessus in amoeba facilitates enhanced infection and survival in mammalian macrophages, and also highlights the diverse range of mechanisms M. abscessus uses to persist in the host (Dubois et al., 2019).
Although most NTM infections are acquired from environmental sources, phylogenetic analysis of M. abscessus clinical isolates suggests that CF centers may facilitate indirect person-to-person transmission of M. abscessus clones through fomites (Aitken et al., 2012). Whole genome sequencing of M. abscessus isolated in global CF centers has revealed the presence of dominant M. abscessus clones with diverse geographical distribution. In addition to comprising 70% of total global M. abscessus infections, these dominant isolates display heightened virulence in macrophages and in Severe Combined Immunodeficiency (SCID) mice (Bryant et al., 2016). This is reflective of trends observed with other well-characterized CF pathogens, such as Pseudomonas aeruginosa, where epidemic strains are associated with worse patient outcome (Panagea et al., 2003; Fothergill et al., 2012).
While patterns of evolution in CF pathogens have been extensively described for other species, such as P. aeruginosa, only recently have similar patterns been described in M. abscessus. Bryant et al. recently used single nucleotide polymorphism analysis of global M. abscessus clinical isolates to define the trajectory of M. abscessus evolution into a true pathogen (Bryant et al., 2021). The initial horizontal acquisition of genes from unrelated species (as described by Ripoll et al., 2009) resulted in a significant leap in M. abscessus genomic variation. Following this, the transition of M. abscessus to the preferred infection site of the lung and evolution into a pulmonary pathogen was coupled with a further increase in M. abscessus genomic variation. This is unsurprising given the crucible of selective pressures present within the CF lung, such as antibiotic stressors, interspecific competition, and host immune defenses all encouraging the dominance of favorable traits (Harrison, 2007; Folkesson et al., 2012). Furthermore, the physical separation of different lung lobes coupled with the heterogeneity of CF lung tissue creates numerous ecological niches that facilitate species diversification (Harrison, 2007). Bryant et al. confirmed this hypothesis with the identification of numerous, genetically distinct subclones of M. abscessus isolated from different areas of the lung in individual patients (Bryant et al., 2021). The authors also described the presence of M. abscessus clones with hypermutable phenotypes, capable of greater phenotypic variation likely due to DNA damage from the highly oxidative pulmonary environment (Ciofu et al., 2005; Martina et al., 2014). While these results identified strong evolutionary pressure on M. abscessus genes that promote intramacrophage survival, perhaps the most prominent finding was the apparent fitness cost of enhanced virulence of M. abscessus isolates. Importantly, mutants with virulence mutations display reduced transmission rates, presumably due to their impaired survival on fomites. As such, the “evolutionary potential” or continued gain of virulence of M. abscessus is limited provided direct patient-to-patient spread (through aerosolized droplets) does not occur (Doyle et al., 2020; Bryant et al., 2021). However, whether M. abscessus can spread through aerosols is still a matter that requires clarification (Bryant et al., 2013). Given the demonstrated potential of this species to acquire polymorphisms that promote M. abscessus survival and the possibility for continued species adaptation, there is a significant need for the continued identification of M. abscessus VFs.
Mycobacteria-Specific Virulence Factors
The genus Mycobacterium contains approximately 200 species, many of which inhabit soil or water environments and have occasional interaction with humans (Tortoli et al., 2017; Armstrong and Parrish, 2021). However, some members of this genus, such as M. tb and Mycobacterium leprae, are extremely successful human pathogens, being the causative agents of TB and leprosy, respectively (Gupta et al., 2018). Similarly, Mycobacterium marinum and Mycobacterium ulcerans are NTM infections acquired by environmental exposure but are adept at establishing cutaneous infection in humans (Tan et al., 2020). The success of these species is tied closely with their ability to survive within the host using an extensive collection of Mycobacteria-specific VFs. As our understanding of M. abscessus virulence expands, there is continual discovery of virulence traits resembling other Mycobacterial species. These further contribute to the definition of M. abscessus as a truly pathogenic species.
Mycobacterial Membrane Protein Large Proteins
Mycobacterial membrane protein large (MmpL) proteins are a family of VFs of the Mycobacterium genus, responsible for transport of lipids and siderophores to the periplasmic space (Melly and Purdy, 2019). These include complex lipids that are essential for the integrity of the mycobacterial envelope, which forms a barrier of protection from immune cells and chemotherapeutic agents (Bailo et al., 2015). Many of these lipids play prominent roles in modifying host–pathogen interactions, and as a result mutations within MmpL proteins can lead to alterations in mycobacterial virulence (Chalut, 2016).
The MmpL class of transport proteins is overrepresented in M. abscessus, with 31 identified MmpL proteins in the reference strain compared to 14 within M. tb (Deshayes et al., 2010). MmpL4 proteins have an important contribution to M. abscessus virulence through their transport of glycopeptidolipids (GPL) to the outer mycobacterial surface which gives colonies a glossy and smooth appearance on solid agar. Disruption of MmpL4 proteins results in the conversion from the smooth morphotype to a rough cording morphotype, the latter of which is associated with enhanced virulence due to large serpentine cord formation, triggering pro-inflammatory responses and apoptosis (Pawlik et al., 2013). Disruption of M. abscessus MmpL8 reduces the transport of glycosyl-diacylated-nondecyl-diols (GDND), a novel glycolipid that facilitates bacterial adhesion to macrophages and induces macrophage phagosomal rupture, and thereby reduces mycobacterial virulence (Halloum et al., 2016). MmpL proteins from mycobacterial species, such as Mycobacterium smegmatis, also confer resistance to some antibiotics, such as isoniazid, by acting as an efflux pump (Pasca et al., 2005; Viljoen et al., 2017). Mutations within transcriptional regulator tetR, which controls two MmpS-MmpL gene pairs, promote resistance to clofazimine and bedaquiline in M. abscessus (Richard et al., 2019), while MmpS5-MmpL5 are associated with efflux of thiacetazone derivatives (Halloum et al., 2017). Absence of either MmpL-S pair increases susceptibility of intracellular M. abscessus to bedaquiline, indicating the contribution of this protein to providing antibiotic resistance (Gutiérrez et al., 2019). Given the role of MmpL proteins in enhancing or attenuating virulence and conferring antimicrobial resistance, this a particularly noteworthy area of continued research for the development of M. abscessus vaccine candidates.
ESX Secretion Systems
Members of the Mycobacterium genus possess an outer mycomembrane comprised of components that must be transported across the cell membrane to reach the extracellular space. Early secretory antigenic target (ESAT-6) secretion (ESX) systems, also known as Type VII secretion systems, are important players in facilitating transport of proteins across this outer membrane and are known to play a significant role in mycobacterial virulence, nutrient acquisition, and bacterial conjugation (Lagune et al., 2021). There are five characterized ESX secretion systems spread across the Mycobacterium genus, varying in complexity and in function (Gröschel et al., 2016). However, all ESX systems have a conserved set of genes that include the machinery for substrate secretion, accessory proteins, and ESX secreted proteins (Houben et al., 2014). The most extensively studied ESX system, ESX-1, facilitates M. tb intracellular survival via inhibition of phagosomal acidification and induces phagosome rupture and mycobacterial escape to the cytosol (Sturgill-Koszycki et al., 1994; van der Wel et al., 2007). The contribution of other ESX secretion systems to virulence is less well-characterized; however, the ESX-3 system of M. tb is known to be utilized for iron acquisition in low nutrient environments and the absence of this system attenuates growth in vivo (Siegrist et al., 2009; Tufariello et al., 2016). The role of the ESX-5 secretion system in slow-growing mycobacteria relates to the integrity of the mycobacterial capsule, with disruption of ESX-5 in M. marinum leading to inability of this pathogen to disrupt the cellular membrane (Ates et al., 2016). While attenuation of ESX-5 deficient M. marinum is evident in zebrafish embryos, the same mutant is hypervirulent in adult zebrafish (Weerdenburg et al., 2012). Given that zebrafish embryos solely possess innate immunity, while the adult zebrafish harbors both innate and adaptive immunity, these findings suggest that ESX-5 may play different roles in disease pathogenesis depending on whether innate or adaptive immunity is the major driver of cellular control of mycobacterial infection (Cronan and Tobin, 2014).
There are currently three characterized secretion systems of M. abscessus—ESX-3, ESX-4, and ESX-P. Secretion targets of ESX-3 (EsxG/H proteins) induce pro-inflammatory cytokine production when co-cultured with bone marrow-derived macrophages, and mice infected with ESX-3-deleted-M. abscessus display a reduced inflammatory and granulomatous response coupled with decreased bacterial survival (Kim et al., 2017). However, this work did not elucidate specific mechanisms by which ESX-3 promotes M. abscessus growth in vivo. Furthermore, this study did not include complementation of ESX-3 function in M. abscessus, highlighting the need for further investigation into the role of ESX-3 in M. abscessus virulence. In contrast, the function of ESX-4 within M. abscessus was recently characterized in detail (Laencina et al., 2018). Through generation of an extensive mutagenesis library, Laencina et al. associated disruptions of the ESX-4 locus with a decrease in intramacrophage and amoeba survival. ESX-4 associated ATPase EccB4 was shown to contribute to virulence by limiting phagosomal acidification and facilitating cytosol contact through phagosomal membrane damage. Unlike ESX-3 and ESX-4, ESX-P is a plasmid-borne secretion system unique to a clinical isolate of M. bolletti (Dumas et al., 2016). More recent work has characterized plasmid-borne ESX components within M. abscessus clinical isolates, suggesting a more extensive presence of ESX systems in this species than previously thought (Dedrick et al., 2021). Whether these secretion systems are functional and contribute to M. abscessus virulence is an area of continued research, and may represent an unexplored means by which M. abscessus is able to acquire novel VFs that contribute to evolving pathogenicity.
Lsr2
Lsr2 is a histone-like protein possessed by several members of the Mycobacterium genus, with roles in transcriptional regulation and DNA damage protection. In M. tb, Lsr2 is required for normal growth in anaerobic and DNA damage-inducing conditions (Bartek et al., 2014). When functioning as a transcriptional regulator, the binding of Lsr2 to coding sequences (of which VF genes are common) may contribute to the transition to a latent metabolic state that occurs during chronic M. tb infection (Gordon et al., 2010; Bartek et al., 2014). In M. smegmatis, lsr2-knockout strains have decreased persistence within macrophages, an effect which is reversed following scavenger treatment to sequester free radicals (Colangeli et al., 2009). Mycobacterium smegmatis lsr2 knockouts also display a smooth, glossy phenotype and are defective in biofilm and pellicle formation (Chen et al., 2006). This is reflected in M. abscessus, where rough morphotypes display greater levels of Lsr2 expression than smooth morphotypes. While M. abscessus lsr2 knockouts are not affected in their glycopeptidolipids (GPL) profile, lsr2 knockout in the rough morphotype of M. abscessus show greater susceptibility to reactive oxygen intermediates and display reduced survival within macrophages, as well as in the zebrafish and murine model of infection, particularly at later time points during infection (Le Moigne et al., 2019). Given the importance of this transcriptional regulator for pathogenesis of M. abscessus, Lsr2 may be a promising target for drug development (Liu and Gordon, 2012).
Glycopeptidolipids
Mycobacterium abscessus grown on solid agar medium displays distinct colony morphologies characterized as smooth (S) and rough (R) morphotypes, each with unique patterns of virulence. These are linked to the variable expression of GPL on the cell surface of M. abscessus, with expression of GPL within S morphotypes resulting in round, glossy colonies, while absence of GPL leads to R morphotypes resulting in a dry, corded appearance (Nessar et al., 2011). The localization of M. abscessus GPL has been shown to cluster to specific nanodomains on the bacterial surface, ultimately modulating surface hydrophobicity and likely influencing bacterial adhesion and virulence (Nessar et al., 2011; Viljoen et al., 2020). The conversion of S to R occur as a result of mutation within genes located within the GPL locus, often associated with GPL synthesis or secretion (Nessar et al., 2011; Pawlik et al., 2013; Park et al., 2015; Li et al., 2020). To date, the mechanisms responsible for S to R conversion are not known; however, this has been hypothesized to occur in response to stress (e.g., Antibiotic stress) which may manifest during host colonization (Bernut et al., 2016b; Gutiérrez et al., 2018). R morphotypes are more frequently isolated from CF patients with chronic infection, and display heightened virulence compared to smooth morphotypes (Jönsson et al., 2007; Kreutzfeldt et al., 2013). The differences in virulence can be linked to different interactions between S and R morphotypes with immune subsets following infection.
As surface molecules on M. abscessus, the absence of GPL on the surface of R morphotypes alters the initial interaction between bacteria and phagocytic cells. A lack of surface GPL exposes immunostimulatory ligands such phosphatidyl-inositol mannoside (PIM) moieties which activate Toll-like receptor (TLR) signaling, resulting in downstream pro-inflammatory cytokine production (Rhoades et al., 2009). While S morphotypes also express PIMs, the heightened expression of surface GPL masks these TLR ligands which acts to prevent innate cell activation (Davidson et al., 2011). Polar GPL themselves also act in an immunosuppressive manner, dampening pro-apoptotic signals induced by R morphotypes and limiting reactive oxygen species (ROS) production (Whang et al., 2017). It is likely that GPL also play an important role in cell–cell interactions, with post-translational modifications of GPL affecting adherence and invasion of M. abscessus to macrophages (Daher et al., 2020). Remodeling of surface GPL also occurs following growth in artificial CF sputum, although the biological significance of this is unknown (Wiersma et al., 2020). Together, these data point to the prominent role of GPL in defining early infection outcomes, with immunologically “silent” establishment of S morphotype M. abscessus in the upper respiratory tract likely facilitating initial colonization of the lung (Gutiérrez et al., 2018).
The S and R morphologies of M. abscessus interact differently with immune cells, leading to different patterns of growth in vivo. While S morphotypes exist largely as single bacilli, R morphotypes form clumps which are difficult for phagocytic cells to engulf (Roux et al., 2016). These aggregates remain adhered to phagocytic cups or, if small enough, are contained within “social” phagosomes containing multiple bacilli. Within the macrophage, R morphotypes appear resistant to lysosomal degradation, leading to extensive and rapid intracellular growth, followed by macrophage apoptosis (Aguilo et al., 2013; Kim et al., 2019). Following apoptosis, rough bacilli are then released into the extracellular space whereby they replicate freely and aggregate to form large extracellular serpentine cords which resist phagocytosis, causing excessive inflammation and abscess formation (Bernut et al., 2014a). Disruption of M. abscessus cording attenuates virulence in vivo, indicating the importance of this phenotype in dictating the outcome of infection (Bernut et al., 2014a; Halloum et al., 2016).
S morphotypes of M. abscessus also resist intracellular degradation, through more direct manipulation of macrophage effector function. Phagocytosed S bacilli often exist in “loner phagosomes,” and act to limit phagosomal acidification and disrupt phagosome membrane integrity, which may facilitate escape to the cytosol (Roux et al., 2016). While cytosol escape and blocking of phagosome acidification are associated with functional ESX-4 found in M. abscessus, it is unclear what role surface GPL may play in facilitating this effector function (Gutiérrez et al., 2018; Laencina et al., 2018). Given the integral link between mycobacterial virulence and macrophage function, understanding the mechanisms by which M. abscessus interacts with innate immune cells is critical in our understanding of M. abscessus virulence (Feng et al., 2020).
Other Cell Surface Molecules
The structure of the mycobacterial cell wall and outer envelope is comprised of a complex array of lipoproteins, glycolipids, and glycoproteins, many of which take part in host–pathogen interactions (Jackson, 2014). These may directly modulate immune responses, or improve the structural integrity of the mycomembrane which renders M. abscessus more resistant to immune mediators (Karakousis et al., 2004). For example, succinylation of M. abscessus surface polysaccharides alters intracellular survival by an uncharacterized mechanism, possibly in relation to altered cell surface hydrophobicity and charge (Palčeková et al., 2020). Other VFs may arise from proteins associated with the modification or transport of cell surface molecules. Knockout of pmt, a protein-O-mannosyltransferase which is responsible for glycosylation of lipoproteins in the mycobacterial cell envelope, increases cell wall permeability which may enhance susceptibility to innate cellular defenses, such as ROS (Becker et al., 2017). Similarly, probable N-acetyl transferase Eis2 is thought to play a role in cell wall biogenesis or transport of cell wall components; deletion of this VF increases M. abscessus susceptibility to ROS and H2O2 and reduces intracellular survival (Dubois et al., 2019). Expression of some surface components, such as trehalose polyphelates (TPPs), does not directly affect macrophage viability, but promote virulence by facilitating M. abscessus cording (Llorens-Fons et al., 2017). Mycobacterium abscessus cell envelope-derived lipids, such as cardiolipin or I-mannosides, are also effective at neutralizing LL-37, an antimicrobial peptide produced by neutrophils and macrophages (Honda et al., 2015, 2020). It has been established that different growth conditions alters the cell wall composition of M. abscessus; whether this occurs in an infection setting and how this impacts on the outcome of infection remains to be established (Hunt-Serracin et al., 2019).
Non-mycobacteria-Specific Virulence Factors
While M. abscessus was first isolated in 1952, complete sequencing of the M. abscessus genome was completed relatively recently in 2009 (Brown-Elliott and Wallace, 2002; Ripoll et al., 2009). In their genomic analysis of M. abscessus, Ripoll et al. identified regions syntenic with non-mycobacterial species, including Actinobacteria, such as Rhodococcus, as well as opportunistic CF pathogens P. aeruginosa and Burkholderia cepacea (Ripoll et al., 2009; Mahenthiralingam, 2014). The organization of these genes into large clusters was suggestive of en bloc horizontal gene transfer events from distant species, with some of these regions encoding proteins that are known to promote virulence in other species. The functions of these VFs are well defined in other species, with most facilitating survival in harsh or stressful conditions. Some are involved in early P. aeruginosa colonization and/or persistence in CF airways, such as phenazine biosynthesis and homogentisate catabolism genes (Rodriguez-Rojas et al., 2009; Jimenez et al., 2012). VFs involved in iron acquisition and resistance to reactive nitrogen intermediates (RNI) were also identified, with sequence homology to Rhodococcus spp. (Ripoll et al., 2009). Similarly, the M. abscessus genome contained proteins with predicted function in phenylacetic acid degradation; these promote B. cenocepacia survival in the Caenorhabditis elegans models of infection, although their precise mechanism of action is unknown (Law et al., 2008; Loutet and Valvano, 2010). Some of these non-mycobacterial VFs have been examined in detail (Figure 1), however for the most part their function in M. abscessus virulence is yet to be established.
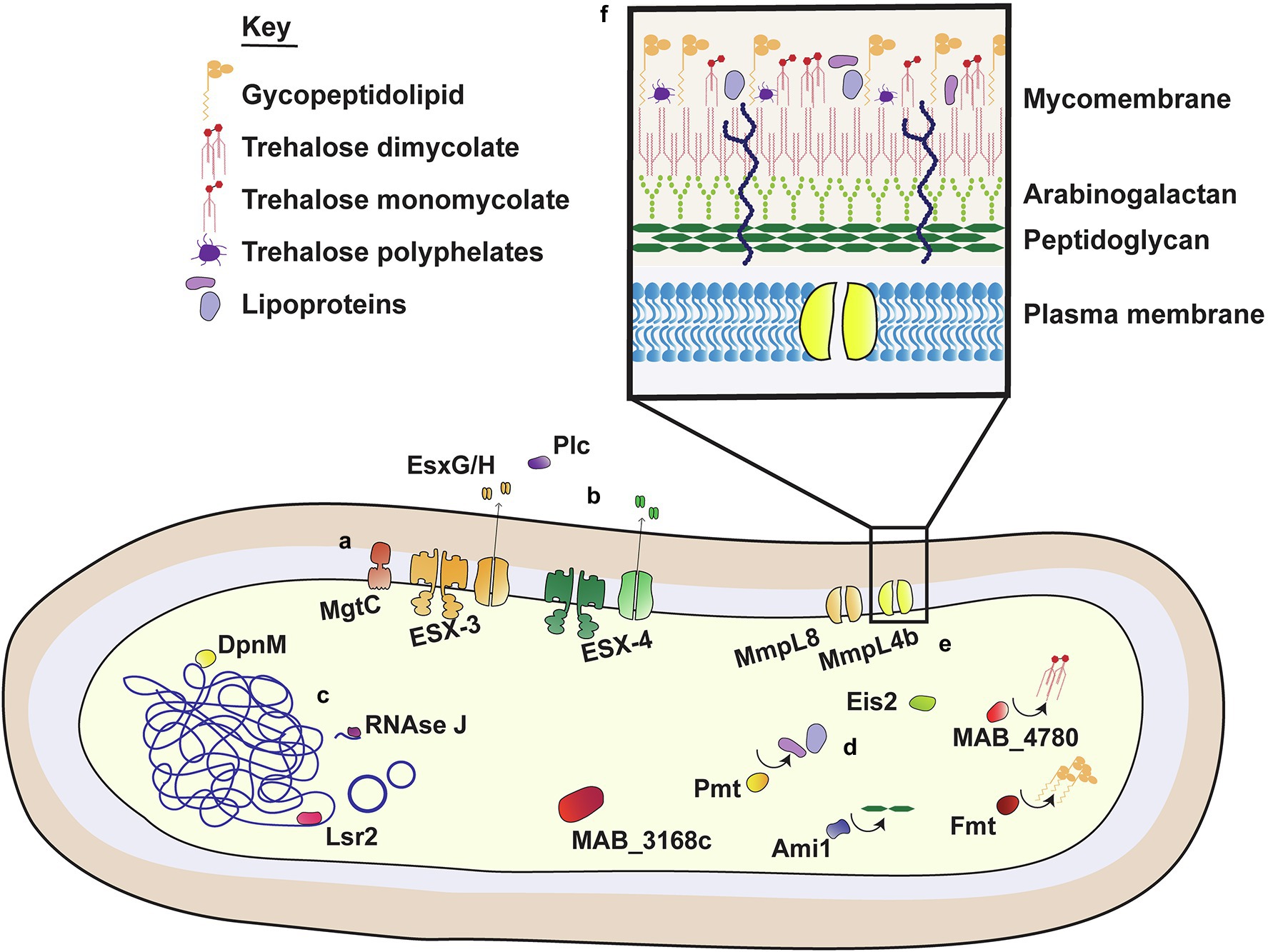
Figure 1. Virulence factors of M. abscessus. M. abscessus uses an extensive range of virulence factors to facilitate survival within host cells. These include membrane bound proteins, pores and secretion systems (i); secreted proteins with a role in virulence (ii); transcriptional regulators and nucleic acid associated proteins (iii); proteins involved in the modification (iv) and transport (v) of cell surface components; and molecules that comprise the outer mycobacterial membrane (vi).
Phospholipase C
Phospholipases are virulence determinants found in a diverse range of mycobacterial and non-mycobacterial species, including P. aeruginosa, Staphylococcus aureus, and Listeria monocytogenes (Ghannoum, 2000). Phospholipases promote virulence by allowing escape from the phagosome and cell-to-cell spread, which is thought to be mediated by cleavage of phospholipids that form part of the cell membrane (Camilli et al., 1991; Smith et al., 1995; Ghannoum, 2000). Expression of plc genes by P. aeruginosa is associated with phosphate limiting conditions, and disruption of plcC results in a reduction of P. aeruginosa virulence in the murine model of infection (Ostroff et al., 1989). Similarly, phospholipase C is upregulated by virulent M. tb in phosphate limiting conditions, such as those that may occur in an intracellular environment (Le Chevalier et al., 2015). PlcC isolated from M. abscessus induces macrophage lysis, and plcC knockout strains display reduced survival within amoeba (N’Goma et al., 2015). Given the sequence similarity between plcC from M. abscessus and other CF pathogens (N’Goma et al., 2015), PlcC is a promising antigenic target for vaccine development. CF patients with pulmonary NTM infection or P. aeruginosa infection have higher titers of anti-PlcC antibodies in serum than uninfected individuals, indicating immune exposure to the PlcC antigen following infection (Le Moigne et al., 2015). Vaccination with M. abscessus plcC DNA induces potent antibody responses and a moderate reduction in bacterial burden in ΔF508 mice, which possess the most common CF gene mutation (Cutting, 2015; Le Moigne et al., 2015). However, the role of PlcC in nutrient acquisition in the lung, and if it enables M. abscessus to maintain long-term infection is unclear (Raynaud et al., 2002).
MgtC
MgtC plays an essential role in the survival of pathogens such Salmonella enterica subsp. typhimurium and CF pathogens B. cenopacea and P. aeruginosa (Alix and Blanc-Potard, 2007; Belon et al., 2015). While the physiological function of this protein has not been fully elucidated, MgtC is a membrane protein that confers survival benefits for both intracellular and extracellular pathogens (Belon et al., 2015). Expression is essential for growth in magnesium poor conditions for P. aeruginosa and B. cenopacea, while upregulation of MgtC following phagocytosis provides enhanced intracellular survival and resistance to low pH for intracellular pathogen Salmonella spp. (Maloney and Valvano, 2006; Rang et al., 2007; Lee and Lee, 2015). MgtC appears to perform a similar role in M. tb, as the protein contributes to improved survival in low magnesium and pH conditions and enhanced virulence in mice (Buchmeier et al., 2000). Mycobacterium abscessus has two sequences encoding proteins homologous to MgtC, MAB_3953, and MAB_0146, both of which bear sufficient sequence similarity to S. typhurmium MgtC, as they can partially restore its function in an mgtC-deficient strain (Le Moigne et al., 2016). Mycobacterium abscessus MgtC is induced in low Mg2+ conditions and within the macrophage, mgtC-encoding DNA provides protection against M. abscessus challenge (Le Moigne et al., 2015, 2016). Of note, M. abscessus lacking functional MgtC does not show a significant impairment in macrophage survival, which may indicate a role of this VF in the extracellular stage of M. abscessus growth (Bernut et al., 2014a).
Porins
Porins are a diverse class of pore proteins that play an important role in the transport of hydrophilic molecules into the bacterial cell. Porins are expressed widely among gram-positive and negative virulence of different species (Achouak et al., 2001). The contribution of porins to the virulence of different species of pathogenic bacteria is extremely diverse and includes facilitating cell adherence, inducing host cell apoptosis and transporting surface proteins associated with virulence (Müller et al., 1999; Azghani et al., 2002; Brunson et al., 2019). Within the context of mycobacteria, M. smegmatis has a collection of pore-forming proteins that regulate nutrient influx which is important for maintaining normal growth rates (Stephan et al., 2005). Deletion of M. smegmatis porin MspA, MspC, and MspD improves M. smegmatis survival in macrophages, by providing greater resistance of M. smegmatis to host-produced nitric oxide (NO; Fabrino et al., 2009). Similarly, deletion of porins mmpA/B in M. abscessus also improves M. abscessus intracellular survival within phagocytic cells and bacterial persistence in SCID mice (de Moura et al., 2021). Porin knockout strain ΔmmpA displays reduced uptake of glucose, but mutants did not display varied uptake by macrophages, susceptibility to NO, or cell cytotoxicity. Of note, sequential samples taken from different early and late M. abscessus infections in CF patients show mutations within the mmp porin genes (Lewin et al., 2021). However, phylogenetic analysis by de Moura et al. could not establish a contributing role of porin mutations to enhanced virulence or transmission of dominant clinical M. abscessus isolates (de Moura et al., 2021). This suggests that while this class of proteins are significant VFs of other species, porins possessed by mycobacterial species including M. abscessus may perform a role unrelated to virulence.
Toward the Development of a Mycobacterium abscessus Vaccine: Possibilities and Challenges
The global incidence of NTM including M. abscessus is increasing, with disease prevalence within CF populations increasing from 9% to 13% in studies conducted after 2000 (Marras et al., 2007; Qvist et al., 2014, 2015; Bar-On et al., 2015; Adjemian et al., 2018). Chronic infection with M. abscessus leads to decreased lung function and is a significant contributor to morbidity and mortality of affected individuals (Esther et al., 2010; Qvist et al., 2015). This combined with the extensive antibiotic resistance and large repertoire of VFs of this pathogen (Figure 1; Table 1) emphasizes the need for prophylactic strategies to limit the global burden of M. abscessus. Given there is currently no vaccine available for M. abscessus and none in clinical development, there is a clear unmet need for the development of vaccines against this pathogen. However, development of a potential M. abscessus vaccine must consider target populations for vaccination, and specific challenges associated with immunizing these populations. This includes an understanding of immune correlates of protection against M. abscessus in both immunocompetent and immunocompromised individuals, in addition to gaining knowledge from efforts to develop a vaccine for M. tb.
Immune Responses to Mycobacterium abscessus Infection
A thorough understanding of the immune correlates of protection against M. abscessus infection is critical for the development of an effective M. abscessus vaccine. This is an area of ongoing research, primarily focused on the use of in vitro, zebrafish, and murine models (Bernut et al., 2015, 2017; Caverly et al., 2015; Meir et al., 2018). Incorporating these different approaches has led to a greater knowledge of immune mechanisms involved in protection against this pathogen.
As with other members of the Mycobacterium genus, early and robust innate immune responses appear to play a critical role in shaping immune control of M. abscessus infection. Monocytes and macrophages are the most common cell subset infected by M. abscessus in human lung tissue (Ganbat et al., 2016). Both S and R morphotypes of M. abscessus are rapidly phagocytosed by macrophages early after infection and depletion of this subset substantially increases bacterial burden in zebrafish (Bernut et al., 2014a 2019). ROS and NO are major bactericidal mechanisms employed by macrophages to kill M. abscessus, with production of NO and ROS strongly correlated with a protective effect across in vitro and animal models of M. abscessus infection (Kim et al., 2014a; Lee et al., 2017). Interestingly, NO production is enhanced by a type I interferon (TI IFN) response and prophylactic administration of rIFN-Β can promote bacterial clearance (Lee et al., 2017). This is contrary to recent work on M. abscessus suggesting that TI IFNs promote macrophage apoptosis and bacterial spread from cell-to-cell (Zhang et al., 2019). In humans, the role of NO and ROS in providing protection against M. abscessus is less clear. When used as an inhaled therapeutic, NO improves lung function and quality of life in CF patients, but this has not been definitively linked to reduction in M. abscessus burden (Bentur et al., 2020). An oxidative environment within the macrophage also appears to enhance M. abscessus growth in an ex vivo setting (Oberley-Deegan et al., 2010). Moreover, NOS2 knockout (Nos2−/−) mice do not display exacerbated bacterial burden compared to wild type (Obregón-Henao et al., 2015). Taken together, these findings suggest a redundant or possibly detrimental role of ROS in protection against M. abscessus infection.
As early responders to pulmonary infection, neutrophils are a prominent component of the immune response against M. abscessus. Neutrophil accumulation is driven by TNF and IL-8 production by macrophages and alveolar epithelial cells, and this subset congregates within murine lungs following infection with both S and R morphotypes (Caverly et al., 2015; Bernut et al., 2016a; Malcolm et al., 2018). Despite the integral role of neutrophils in the formation of the granuloma, the efficacy of this subset in reducing bacterial burden remains unclear. Neutrophils phagocytose bacteria and employ neutrophil extracellular traps (NETs) and ROS production to eliminate M. abscessus, but neutrophil-derived ROS and LL-37 has limited killing activity against M. abscessus (Honda et al., 2015; Malcolm et al., 2018). Extracellular DNA released in response to M. abscessus during NETosis may also contribute to biofilm formation in the CF lung (Malcolm et al., 2013). Pulmonary infection with the more virulent R morphotype of M. abscessus is also accompanied by neutrophil accumulation in the bronchoalveolar lavage fluid, to a greater extent than that induced by the S morphotype (Caverly et al., 2015). Given the inflammatory nature of this immune cell subset, excessive neutrophilia can be extremely damaging to lung tissue and is a primary cause of tissue damage in CF (Downey et al., 2009). How exactly this subset contributes to protection or pathology following chronic infection is an area of continued research.
A cornerstone of the immune response to mycobacterial infection is the formation of the granuloma, a cluster of recruited host immune cell subsets which form a physical structure to restrict bacteria (Figure 2A). In zebrafish, M. abscessus granulomas are primarily comprised of neutrophils and macrophages. The accumulation of these subsets is dependent on TNF, which is required for development of the granuloma structure and the containment of bacterial growth (Bernut et al., 2016a). However, granuloma formation is not always sufficient in containing M. abscessus infection—the induction of macrophage cell death by M. abscessus releases extracellular bacteria, which form large serpentine cords that evade phagocytosis and resist immune defenses (Figure 2B; Bernut et al., 2014a; Halloum et al., 2016). Currently, the specific immunological factors differentiating protective granuloma formation from unrestrained bacterial growth are not known (Johansen et al., 2020). Murine models of M. abscessus infection also display granuloma formation in the lungs and spleen after aerosol or intravenous infection, respectively, and studies using TNF knockout (Tnf−/−) and IFN-γ knockout (Ifngr1−/− and GKO−/−) mice highlight the importance of these cytokines for maintenance of granuloma structure (Rottman et al., 2007; Ordway et al., 2008). Nude, severe combined immunodeficiency (SCID) and GMCSF knockout (GMCSF−/−) mice also develop progressive granulomatous lesions, making these useful models for preclinical drug testing but less suitable for understanding immune factors involved in granuloma formation (Obregón-Henao et al., 2015). While evidence from animal models points to a significant role of this structure in control of M. abscessus infection, the importance of granuloma formation in humans is less clear. Granuloma formation is well documented following cutaneous M. abscessus infection (Bartralot et al., 2000; Kwon et al., 2009), but fewer studies characterize the granulomatous response following pulmonary infection (Jeong et al., 2004; Okazaki et al., 2013). Mycobacterium abscessus results in heterogeneous changes to pulmonary tissue following infection, including inflammatory infiltrate which may be accompanied by the formation of necrotizing or non-necrotizing granulomas (Jeong et al., 2004). Based on what is known about other NTM infections, host immune responses, clinical isolate heterogeneity, and pathogen virulence may all contribute to the extent of granuloma formation and its ability to contain M. abscessus infection (Lammas et al., 2002).
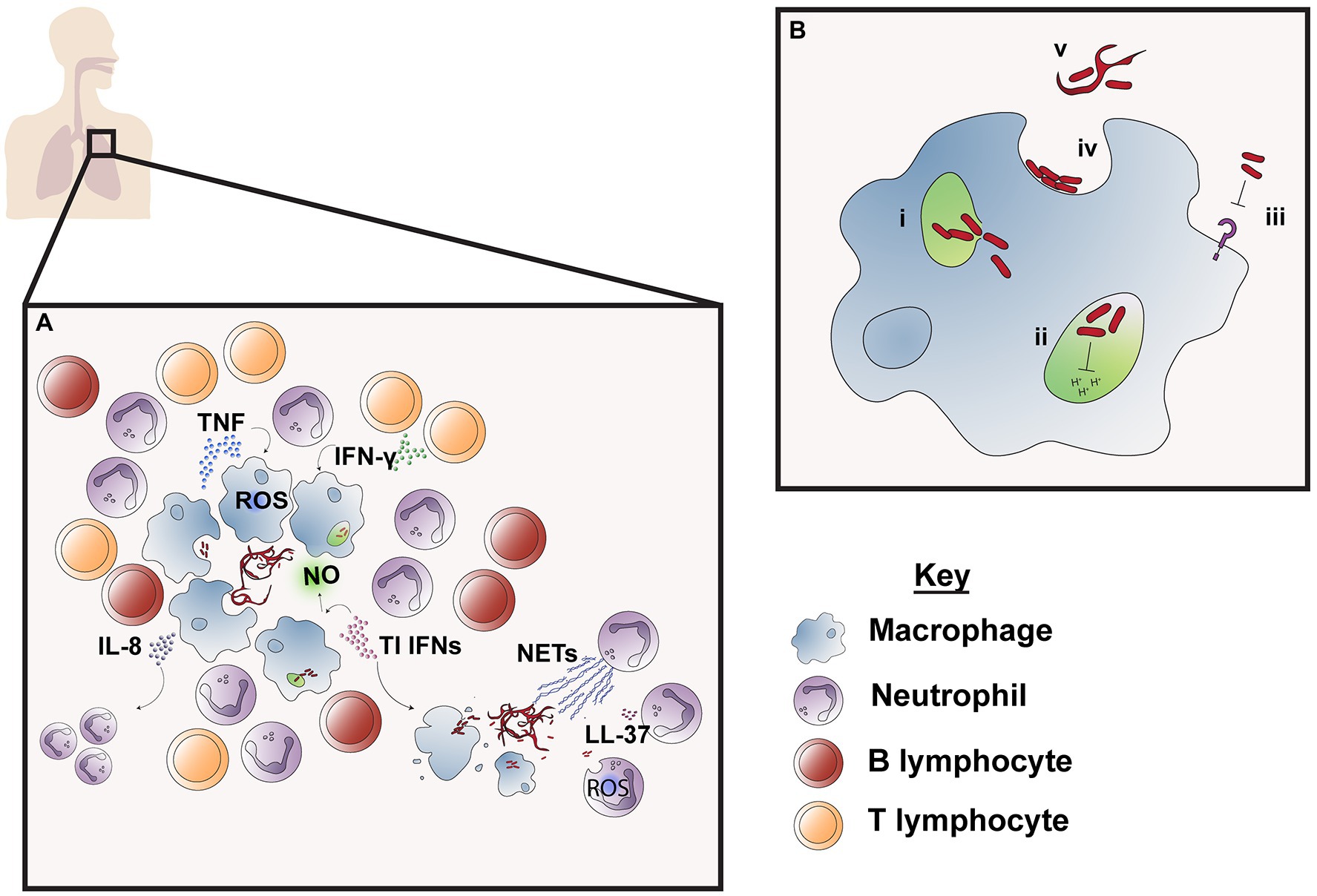
Figure 2. Immune responses to M. abscessus infection. M. abscessus infection is followed by an influx of neutrophils and macrophages surrounded by lymphocytes which work to contain bacteria in the granuloma (A). Cytokines TNF and IFN-γ produced by macrophages and CD4+ T cells are required for granuloma formation and induce macrophage effector function, such as phagosome acidification and reactive oxygen species (ROS) production, while type I IFN production promotes nitric oxide (NO) production. IL-8 attracts neutrophils which are responsible for phagocytosis, NET production and secretion of antimicrobial peptide LL-37. However, release of M. abscessus into the extracellular space as a result of cell death leads to the formation of serpentine cords, which are resistant to innate immune defenses and leads to unchecked bacterial replication. M. abscessus also possesses numerous mechanisms of immune evasion to resist macrophage effector functions (B); these include bacterial escape from the phagosome to the cytosol through interference with the phagosomal membrane (i); prolonged survival within the phagosome by blocking phagosomal acidification and thereby preventing M. abscessus degradation (ii) and inhibition of macrophage TLR signaling which limits downstream immune cell activation and recruitment (iii). M. abscessus also persists in the extracellular environment by avoiding phagocytosis, which is enabled through adherence to macrophage phagocytic cups on the cell surface (iv) and by forming serpentine cords which are too large to be engulfed by macrophages (v).
Most relevant for vaccine design is the contribution of adaptive immune subsets to M. abscessus pulmonary infection. CD4+ T helper (TH) cells appear to be a key component of the protective response, with TH1 responses enabling control of mycobacterial dissemination and granuloma formation through their stimulation of innate immune subsets, such as macrophages and neutrophils (Rottman et al., 2007; Bernut et al., 2016a). In a murine model of M. abscessus infection, bacterial clearance is preceded by an influx of IFN-γ producing CD4+ T cells, and it has been shown that M. abscessus infection persists in IFN-γ knockout GKO−/− mice (Ordway et al., 2008; Obregón-Henao et al., 2015). High-dose infection models in GKO−/− mice display a heightened TH2 response resulting in an immunosuppressive phenotype, supporting the notion that TH1 polarization is ideal for protection against intracellular mycobacteria (Ordway et al., 2008). Similarly, individuals with deficiencies affecting T-cell function appear predisposed to M. abscessus infection (Lutzky et al., 2018). Patients with active M. abscessus infection also display a dampened TH1/TH2 and heightened TH17 cytokine profile compared to healthy controls, suggestive of a link between TH1 polarization and a protective phenotype (Kim et al., 2014b; Lake et al., 2016). This is an especially important consideration in the design of subunit M. abscessus vaccines, where adjuvant choice affects T-cell polarization and thus resulting protection (Knudsen et al., 2016).
While robust T-cell responses are clearly essential for protection against M. abscessus, the contribution of the humoral response to protection against pulmonary M. abscessus infection is less established. Antibodies to M. abscessus are generated following infection or vaccination (Jeon et al., 2009; Le Moigne et al., 2015) and B-cell deficiency in the murine model of infection promotes bacterial growth (Rottman et al., 2007). While humoral responses in healthy individuals are considered a critical components for extracellular pathogens, such as P. aeruginosa, their efficacy appears limited in preventing bacterial colonization or eradicating infection in CF patients (Yonker et al., 2015; Sainz-Mejías et al., 2020). Humoral responses that target surface GPL of M. abscessus S morphotypes may also encourage the conversion to the more virulent R morphotype, thus proving detrimental to the host (Gutiérrez et al., 2018). It is also unclear whether the extracellular stages of M. abscessus growth, such as biofilm formation during early infection, and extracellular cording following phagosome rupture would be effectively disrupted by neutralizing antibodies. Most vaccines currently approved for human use focus on the effective development of humoral responses, and development of vaccines targeting cell-mediated immunity appear more difficult to produce. As such, the contribution of this subset to M. abscessus protection will require continued investigation to inform development of an effective M. abscessus vaccine.
The Quest for a Mycobacterium abscessus Vaccine in Susceptible Populations
Mycobacterium abscessus infection primarily occurs in individuals with reduced pulmonary immune responses, including those with CF, COPD, and non-CF bronchiectasis (Ratnatunga et al., 2020). The development of novel immunization strategies for this pathogen has the potential to dramatically reduce the M. abscessus burden and improve quality of life for these susceptible populations. However, progress toward novel vaccines has been limited by an incomplete understanding of immune responses in these groups that perpetuate M. abscessus infection, and how this may impact the outcome of vaccination. While populations with COPD and non-CF bronchiectasis make up a significant proportion of M. abscessus cases, factors resulting in enhanced susceptibility of these populations to NTM infection have not yet been established (Griffith et al., 2007; Ratnatunga et al., 2020). It is speculated that structural damage within the lung in non-CF bronchiectasis permits bacterial colonization, but immune dysfunction, such as aberrant neutrophil migration and effector function, also perpetuates infection by preventing optimal clearance (Chalmers and Hill, 2013). Populations susceptible to NTM infection with no clear risk factors display reduced IFN in serum and altered adipokine levels, further suggesting an underlying link between immune responses and risk of infection (Kartalija et al., 2013).
Given the frequency with which CF populations become infected with NTM, the consideration of immune responses in CF populations is of utmost importance when developing a vaccine for M. abscessus. As research into the area continues, we are beginning to appreciate the profound impact of CFTR mutations within CF patients on immune cell functionality, and the implications of this for pulmonary bacterial clearance (Hartl et al., 2012). The immune landscape of CF patients includes macrophages with a skewed hyperinflammatory profile (Bruscia and Bonfield, 2016), neutrophils with dysfunctional phagosomal maturation and effector functions (Zhou et al., 2013; Gifford and Chalmers, 2014), and an inflammatory milieu that may limit the ability of innate cells to clear infection (Roghanian et al., 2006; Cockx et al., 2018). Recent work by Bernut et al. also showed impaired ROS production in professional phagocytic cells following M. abscessus infection, using a zebrafish model of CF (Bernut et al., 2019). While less is known about the implications of CFTR mutations on adaptive immune responses, lymphocytes from murine models of CF also display a predilection to the development of TH2 and TH17 responses (Mueller et al., 2011; Tiringer et al., 2013; Mulcahy et al., 2015). Cftr−/− mice also display dysregulated B-cell follicle formation, activation, and accumulation in the lung (Polverino et al., 2019). This adds to the complexity of vaccine development for M. abscessus, as immune responses may be harder to predict in target populations and could potentially be naturally skewed toward non-protective phenotypes (Kartalija et al., 2013). The quality of immune responses within these populations will play a significant role in shaping the outcome of vaccination with an M. abscessus vaccine and is therefore a key area of continued research.
Research of potential therapeutic agents to protect against M. abscessus infection may greatly benefit from effective animal models that recapitulate the clinical presentation of M. abscessus lung disease. This has been a continual challenge for researchers, as animal genotypes that possess common CF mutations do not display the same pathological symptoms as humans (Guilbault et al., 2007). For example, the ΔF508 mouse model possesses the most common mutation in the CFTR gene in humans but does not recapitulate the disease pathophysiology (Scholte et al., 2004). Although some knockout strains show greater persistence of bacterial load compared to wild-type littermates, none of these models develop spontaneous colonization or persistent infection by CF pathogens in the lung, such as in human patients (McMorran et al., 2001; Guilbault et al., 2005, 2007; Ordway et al., 2008; Bernut et al., 2014b). This problem has been circumvented by the use of immunocompromised strains (Lerat et al., 2014), corticosteroid administration (Maggioncalda et al., 2020), or infection with thrombin and fibrinogen plugs; a strategy similar to the agarose bead infection model used for P. aeruginosa (Facchini et al., 2014; Caverly et al., 2015). In addition to zebrafish and A. castellanni models, these have provided us with snapshots of the immune response to M. abscessus infection; however, an in-depth understanding of correlates of protection is best achieved with immune competent models which recapitulate the immune response to M. abscessus infection in humans.
The discovery of VF acquisition by M. abscessus from other CF pathogens highlights the continued evolutionary potential of this pathogen and reinforces the urgent need for vaccines to provide robust protection to susceptible groups. Suggestions that the CF lung provides an environment that may enhance M. abscessus virulence is particularly worrisome; however, shared VFs across different bacterial species could allow the development of protein vaccine candidates that target multiple CF pathogens (Ripoll et al., 2009; Bryant et al., 2021). For instance, PlcC antibodies from CF patients infected with P. aeruginosa are cross-reactive with M. abscessus PlcC (Le Moigne et al., 2015). Cross-protective vaccines are clearly advantageous in terms of research and development costs; however, no M. abscessus vaccine study to date has confirmed a protective effect of cross-reactive immune responses induced by vaccination (Le Moigne et al., 2016). Given the number of VFs acquired from non-mycobacterial species in the M. abscessus genome, this is a noteworthy area of continued research (Ripoll et al., 2009).
In addition to the development of vaccines for CF pathogens, there is interest in development of an M. abscessus vaccine to provide protection against different mycobacterial species. Lower incidence rates of Mycobacterium avium infection in HIV-positive individuals with prior M. tb infection suggest a degree of protection afforded by mycobacterial exposure (Horsburgh et al., 1996). This has encouraged interest in repurposing the current M. tb vaccine, Mycobacterium bovis Bacille Calmette–Guérin (BCG), to be used for protection against NTM infection in susceptible populations (World Health Organization, 2020). While lower incidences of NTM infection in BCG-vaccinated children support the notion of BCG vaccine-induced cross-protection, there is a paucity of information in the literature specifically referring to protection against M. abscessus (Trnka et al., 1994; Zimmermann et al., 2018). T cells isolated from peripheral blood of latent-TB-infected or BCG-vaccinated individuals produce IFN-γ and granzyme A in response to M. avium restimulation, and these cross-reactive T cells restrict growth of M. avium or M. abscessus in monocytes. BCG vaccination of mice also generates a population of T cells that secrete cytokines upon NTM restimulation; however, the effect of vaccination on bacterial burden was not determined in this study (Abate et al., 2019). As there are clear advantages of repurposing a currently approved and widely distributed vaccine, the role of BCG vaccination in providing protection against M. abscessus infection requires further investigation.
Lessons Learned From TB Vaccination Efforts
Despite being heavily researched since its discovery, M. tb is still a leading cause of death by infectious disease worldwide. In 2020, a quarter of the world’s population was estimated to be infected with M. tb with 10 million new infections in 2019 alone (Chakaya et al., 2021). The protection afforded by the current vaccine available, BCG, is extremely variable across different age groups, latitudes, and to those with previous infection or mycobacterial exposure (Mangtani et al., 2014; Ahmed et al., 2021). While numerous candidates for novel TB vaccines are currently in the developmental pipeline, none have been able to provide sufficient protection to replace BCG. However, we can learn from decades of TB research and vaccine development about factors to consider in the pursuit of an M. abscessus vaccine, in addition to identifying unique challenges specific to this pathogen.
A robust understanding of immune responses required for protection is essential in the development of novel M. abscessus vaccines. In the case of vaccines against M. tb, efforts have been consistently stalled by our incomplete knowledge of immune correlates of protection against M. tb (Counoupas et al., 2019). This was exemplified in the results of the Modified Vaccinia Ankara 85A (MVA85A) vaccine in Phase IIb clinical trials, which had been a leading candidate to replace BCG. While earlier studies had shown strong T-cell-mediated immune responses following vaccination, this vaccine did not show protective efficacy in infants (Scriba et al., 2010; Tameris et al., 2013). TH1 helper subsets and cytokines IFN-γ and TNF also appear to be important for protection against M. abscessus, but whether these subsets are predictive of protection afforded by vaccination has not yet been established (Rottman et al., 2007; Bernut et al., 2016a). The difficulty in determining immune correlates of protection against M. abscessus is exacerbated by the diverse growth stages of this microorganism, with extracellular biofilm formation and colonization preceding the emergence of invasive intracellular variants which form chronic infection in the lung (Howard et al., 2006; Gutiérrez et al., 2018; Bryant et al., 2021). There is currently a paucity of information on how different branches of the immune response (namely, humoral and cell-mediated subsets) contribute to effective bacterial clearance across different infection stages. Diverse vaccination strategies can be aimed at preventing colonization by inducing a strong humoral response or at enhancing clearance of persistent infection by inducing a strong cell-mediated immune response. As with M. tb infection, it is also unclear what impact natural immunity from prior M. abscessus infection has on the risk of reinfection (Verver et al., 2005; McIvor et al., 2017). Recurrence of M. abscessus infection has been attributed to poor antimicrobial efficacy, particularly the inefficacy of macrolide treatments to fully eradicate infection (Pasipanodya et al., 2017). However, it has been noted that reinfection with NTM, such as M. abscessus, frequently occurs with strains of different genotype, suggesting that M. abscessus antigenic variability has an impact on protective immune responses (Koh et al., 2017). A comprehensive understanding of the importance of natural immunity over the duration of M. abscessus infection will be critical to dictate the trajectory of novel vaccine development.
Potential Vaccination Strategies for Mycobacterium abscessus
There is an enormous array of strategies that may be used in the development of a novel M. abscessus vaccine, each with different advantages and drawbacks. These include whole-cell (live attenuated or heat-killed), nucleic acid vaccines, and subunit (viral vectored or protein and adjuvant) vaccines.
Live attenuated vaccines have an enormous advantage in that they possess an extremely diverse repertoire of proteins that may be recognized by the immune system, thereby inducing a more diverse immune response. Beyond attenuation, whole-cell vaccines may also be modified to further enhance antigenic visibility to the immune system. VPM1002, for example, is a modified form of the BCG vaccine containing protein Listeriolysin O, which allows BCG escape into the cytosol (Grode et al., 2013). This facilitates antigen presentation to CD8+ T cells, thereby activating an additional component of the immune response to the vaccine (Grode et al., 2005). However, live attenuated vaccines pose an inherent risk of vaccine-associated disease for immunocompromised individuals or those on immunosuppressants, such as individuals with CF (Burroughs and Moscona, 2000; Malfroot et al., 2005). Heat-killed whole-cell vaccines are advantageous for these populations because there is no risk of vaccine dissemination and have been particularly successful in the TB field. One such example is Vaccae™, a whole-cell vaccine of inactivated environmental mycobacteria Mycobacterium vaccae (Fatima et al., 2020). Given the shared epitopes of M. vaccae with NTM and M. tb, this is a potential vaccine candidate that could be repurposed for M. abscessus.
Novel vaccine candidates with the potential of incorporating VFs also include nucleic acid-based vaccines, such as DNA vaccines. These comprise plasmids encoding antigen/s of interest and employ host cells to synthesize proteins which are recognized by the immune system. Nucleic acid vaccines are advantageous due to the relative ease of design, production and scalability. However, no DNA vaccines have progressed beyond preclinical study within the M. tb field—this may be because of their poorly immunogenic nature, or the more complex requirements for vaccine delivery to enter the nucleus and prevent plasmid degradation (Lee et al., 2018; Sefidi-Heris et al., 2020). Recently however, the use of tetrafunctional block copolymers to enhance cell uptake of exogenous nucleic acid has improved our ability to effectively deliver DNA vaccines (Richard-Fiardo et al., 2015). Both preclinical vaccines for M. abscessus are DNA formulated with tetrafunctional block polymers, one targeting M. abscessus VF PlcC and another targeting MgtC (McIlroy et al., 2009; Le Moigne et al., 2015, 2016). DNA vaccination against M. abscessus PlcC appears to provide marginally superior protection to protein-based vaccination, although the immunological mechanisms driving this trend are unclear (Le Moigne et al., 2015). However, both PlcC and MgtC DNA vaccination have a limited ability to consistently reduce bacterial burden by an appreciable level in both ΔF508 and WT mice across the course of M. abscessus infection (Le Moigne et al., 2015, 2016). Recent successes in mRNA vaccination for the Coronavirus Disease 2019 (COVID-19) has also promoted interest in mRNA as a vaccination strategy. This is due to the ease of manufacturing ability and the ability to induce activation of immune cells by multiple cellular pathways in response to mRNA (Brisse et al., 2020). As this area of vaccine design is relatively new, it is likely that continued research will be required to improve the potential of this formulation before use against M. abscessus.
Subunit vaccines comprise antigenic proteins from the target species, delivered either through viral vector or with an adjuvant to promote induction of an immune response. The selection of appropriate antigen is a critical consideration for subunit vaccines and may determine the protective outcome. Highly expressed VFs are ideal vaccine candidates, and have been targets for multiple TB vaccines in development (Chakaya et al., 2021). Importantly, antigenic expression for M. tb varies over the course of chronic infection and subunit vaccines targeting antigens only expressed at specific stages of infection may lack efficacy (Shi et al., 2004; Rogerson et al., 2006). CF pathogens, such as P. aeruginosa, also show marked change in physiology following adaptation to the CF lung, such as development of a mucoid phenotype, reduced VF expression, and loss of motility, suggesting this is a possibility for M. abscessus (Winstanley et al., 2016). This obstacle may be overcome through the incorporation of “early” and “late” stage antigens to target a pathogen’s full antigenic repertoire. One such example is CysVac2, a subunit vaccine which combines the secreted mycolyltransferase Ag85B with CysD, a component of the sulfate assimilation pathway expressed highly during chronic infection (Pinto et al., 2013; Counoupas et al., 2016). This highlights the importance of understanding M. abscessus antigen expression during infection, which may inform vaccine design.
A particular advantage of subunit vaccines is that the quality and type of the immune response may be more easily modulated through the selection of an adjuvant. Adjuvants, such as these, are most effectively used when their selection is tailored to the variety of immune response generated, which is often independent of the co-administered antigen (Knudsen et al., 2016). A diverse range of adjuvants are currently used in clinical TB vaccine candidates, including TLR agonists and liposomal formulations, such as IC31 and GLA-SE (Stewart et al., 2019; Enriquez et al., 2021). Most of the adjuvants being used in clinical trials for TB encourage the generation of protective TH1 and/or TH17 immune responses (Stewart et al., 2019). Given this is thought to be an immune correlate of protection against M. abscessus, these adjuvants may be useful components of an M. abscessus vaccine. Appropriate selection of adjuvant (such as one with a minimal inflammatory profile) may also facilitate non-parenteral vaccine administration, such as vaccine delivery directly to the respiratory mucosa (Ferrell et al., 2021). There is renewed interest in pulmonary vaccination for TB, whereby delivery of the vaccine directly to the lung induces tissue-resident populations capable of rapid response to M. tb (Counoupas et al., 2020). Similarly, intranasal vaccination with BCG provides superior protection against M. tb infection to subcutaneous vaccination (Perdomo et al., 2016). As our knowledge of immune responses correlated with M. abscessus protection increases, this may be a valuable avenue of investigation.
Reverse Vaccinology for the Development of an Mycobacterium abscessus Vaccine
As the field of vaccinology has advanced, so too has the use of bioinformatics approaches to aid vaccination efforts. Increases in genomic sequencing of pathogenic species has given rise to the field of “reverse vaccinology” whereby potential VFs and antigenic targets are discovered through in silico analysis of a pathogen’s genome and predicted proteome (Rappuoli, 2000). This method has been relatively underutilized for M. tb, likely due to the wealth of in vitro knowledge that has emerged from decades of research. A pathogen, such as M. abscessus, is an excellent candidate for reverse vaccinology approaches to streamline the identification of potential vaccine candidates, given the continual and growing need for a vaccine against this pathogen. There are numerous studies using pangenome analysis to identify shared VFs across clinical isolates of M. abscessus, and this is an ideal starting point for a reverse vaccinology approach (Choo et al., 2014; Davidson et al., 2014; Bryant et al., 2021; Lewin et al., 2021). There is also an extensive range of protein pathway mapping, MHC-II binding prediction, and epitope mapping software currently available which provides greater insight into those proteins likely to yield immunogenic vaccine candidates (Heinson et al., 2015). The reverse vaccinology approach has most recently been used to identify potential VFs and therapeutic targets of M. abscessus by development of a novel hierarchical approach; the development of such workflows is beneficial because they may be applied to other pathogens in future (Dar et al., 2021). There is also enormous advantage in a synergistic approach using wet-lab based and in silico techniques as a starting point for vaccine candidate identification, as this is based upon the confirmed proteome or secretome of different species. This was recently used by Steindor et al. to identify immunogenic proteins across different clinical isolates of M. abscessus and yielded many potential VFs that could be suitable vaccine targets (Steindor et al., 2019). However, current studies on M. abscessus reverse vaccinology lack experimental verification of protein immunogenicity; this will be a critical area of future research to determine the best candidates for progression. As we continue to build on our knowledge of this emerging pathogen, reverse vaccinology will become an essential tool in antigenic discovery for the development of subunit vaccines.
Conclusion
In recent years, we have elevated our understanding of M. abscessus from a relatively innocuous environmental organism to a formidable evolving true pathogen with a range of immune modulatory mechanisms to facilitate its survival within the host. These include VFs with homology to well-known mycobacterial VFs in pathogenic species, such as M. tb, in addition to non-mycobacterial VFs which bear striking resemblance to those present within other CF species, such as B. cenocepacea and P. aeruginosa. These VFs have a range of immune modulatory mechanisms to enhance M. abscessus survival within the host: promoting phagosomal escape, restriction of phagosomal acidification, enhancement of bacterial cording, and immune masking to escape detection. These, in conjunction with the extensive antibiotic resistance of this pathogen, have likely contributed to the increasing global incidence and severity of pulmonary M. abscessus. However, through increased understanding of M. abscessus virulence, we have uncovered potential vaccine candidates and novel drug targets, bringing us closer to novel prevention and eradication strategies for this pathogen. Development of a vaccine may also be guided effectively by lessons learned from decades of research into other pathogenic Mycobacteria, such as M. tb. Continued research into the virulence and immune correlates of protection in M. abscessus pathogenesis using biologically relevant animal models will provide insight into the best strategies to adopt for the development of an M. abscessus vaccine, to improve the quality of life of susceptible populations.
Author Contributions
KF and CC conceptualized the idea of the review article. KF wrote the first draft of the manuscript. KF, MJ, JT, and CC provided revision to the scientific content of the final manuscript. All authors contributed to the article and approved the submitted version.
Funding
JT is supported by the NHMRC Centre of Research Excellence in Tuberculosis Control (1153493).
Conflict of Interest
The authors declare that the research was conducted in the absence of any commercial or financial relationships that could be construed as a potential conflict of interest.
Publisher’s Note
All claims expressed in this article are solely those of the authors and do not necessarily represent those of their affiliated organizations, or those of the publisher, the editors and the reviewers. Any product that may be evaluated in this article, or claim that may be made by its manufacturer, is not guaranteed or endorsed by the publisher.
References
Abate, G., Hamzabegovic, F., Eickhoff, C. S., and Hoft, D. F. (2019). BCG vaccination induces M. avium and M. abscessus cross-protective immunity. Front. Immunol. 10:234. doi: 10.3389/fimmu.2019.00234
Achouak, W., Heulin, T., and Pagès, J.-M. (2001). Multiple facets of bacterial porins. FEMS Microbiol. Lett. 199, 1–7. doi: 10.1111/j.1574-6968.2001.tb10642.x
Adekambi, T., Sassi, M., van Ingen, J., and Drancourt, M. (2017). Reinstating Mycobacterium massiliense and mycobacterium bolletii as species of the mycobacterium abscessus complex. Int. J. Syst. Evol. Microbiol. 67, 2726–2730. doi: 10.1099/ijsem.0.002011
Adjemian, J., Olivier, K. N., and Prevots, D. R. (2018). Epidemiology of pulmonary nontuberculous mycobacterial sputum positivity in patients with cystic fibrosis in the United States, 2010–2014. Ann. Am. Thorac. Soc. 15, 817–826. doi: 10.1513/AnnalsATS.201709-727OC
Aguilo, J., Alonso, H., Uranga, S., Marinova, D., Arbues, A., De Martino, A., et al. (2013). ESX-1-induced apoptosis is involved in cell-to-cell spread of mycobacterium tuberculosis. Cell. Microbiol. 15, 1994–2005. doi: 10.1111/cmi.12169
Ahmed, A., Rakshit, S., Adiga, V., Dias, M., Dwarkanath, P., D’Souza, G., et al. (2021). A century of BCG: impact on tuberculosis control and beyond. Immunol. Rev. 301, 98–121. doi: 10.1111/imr.12968
Aitken, M. L., Limaye, A., Pottinger, P., Whimbey, E., Goss, C. H., Tonelli, M. R., et al. (2012). Respiratory outbreak of mycobacterium abscessus subspecies massiliense in a lung transplant and cystic fibrosis center. Am. J. Respir. Crit. Care Med. 185, 231–232. doi: 10.1164/ajrccm.185.2.231
Alix, E., and Blanc-Potard, A.-B. (2007). MgtC: a key player in intramacrophage survival. Trends Microbiol. 15, 252–256. doi: 10.1016/j.tim.2007.03.007
Armstrong, D. T., and Parrish, N. (2021). Current updates on mycobacterial taxonomy, 2018-2019. J. Clin. Microbiol. 59:e0152820. doi: 10.1128/JCM.01528-20
Ates, L. S., van der Woude, A. D., Bestebroer, J., van Stempvoort, G., Musters, R. J., Garcia-Vallejo, J. J., et al. (2016). The ESX-5 system of pathogenic mycobacteria is involved in capsule integrity and virulence through its substrate PPE10. PLoS Pathog. 12:e1005696. doi: 10.1371/journal.ppat.1005696
Azghani, A. O., Idell, S., Bains, M., and Hancock, R. E. (2002). Pseudomonas aeruginosa outer membrane protein F is an adhesin in bacterial binding to lung epithelial cells in culture. Microb. Pathog. 33, 109–114. doi: 10.1006/mpat.2002.0514
Babaki, M. K. Z., Soleimanpour, S., and Rezaee, S. A. (2017). Antigen 85 complex as a powerful mycobacterium tuberculosis immunogene: biology, immune-pathogenicity, applications in diagnosis, and vaccine design. Microb. Pathog. 112, 20–29. doi: 10.1016/j.micpath.2017.08.040
Bailo, R., Bhatt, A., and Aínsa, J. A. (2015). Lipid transport in mycobacterium tuberculosis and its implications in virulence and drug development. Biochem. Pharmacol. 96, 159–167. doi: 10.1016/j.bcp.2015.05.001
Bar-On, O., Mussaffi, H., Mei-Zahav, M., Prais, D., Steuer, G., Stafler, P., et al. (2015). Increasing nontuberculous mycobacteria infection in cystic fibrosis. J. Cyst. Fibros. 14, 53–62. doi: 10.1016/j.jcf.2014.05.008
Bartek, I., Woolhiser, L., Baughn, A., Basaraba, R., Jacobs, W., Lenaerts, A., et al. (2014). Mycobacterium tuberculosis Lsr2 is a global transcriptional regulator required for adaptation to changing oxygen levels and virulence. MBio 5:e01106-14. doi: 10.1128/mBio.01106-14
Bartralot, R., Pujol, R. M., García-Patos, V., Sitjas, D., Martín-Casabona, N., Coll, P., et al. (2000). Cutaneous infections due to nontuberculous mycobacteria: histopathological review of 28 cases. Comparative study between lesions observed in immunosuppressed patients and normal hosts. J. Cutan. Pathol. 27, 124–129. doi: 10.1034/j.1600-0560.2000.027003124.x
Becker, K., Haldimann, K., Selchow, P., Reinau, L. M., Dal Molin, M., and Sander, P. (2017). Lipoprotein glycosylation by protein-O-mannosyltransferase (MAB_1122c) contributes to low cell envelope permeability and antibiotic resistance of mycobacterium abscessus. Front. Microbiol. 8:2123. doi: 10.3389/fmicb.2017.02123
Belon, C., and Blanc-Potard, A.-B. (2016). Intramacrophage survival for extracellular bacterial pathogens: MgtC as a key adaptive factor. Front. Cell. Infect. Microbiol. 6:52. doi: 10.3389/fcimb.2016.00052
Belon, C., Soscia, C., Bernut, A., Laubier, A., Bleves, S., and Blanc-Potard, A.-B. (2015). A macrophage subversion factor is shared by intracellular and extracellular pathogens. PLoS Pathog. 11:e1004969. doi: 10.1371/journal.ppat.1004969
Bentur, L., Gur, M., Ashkenazi, M., Livnat-Levanon, G., Mizrahi, M., Tal, A., et al. (2020). Pilot study to test inhaled nitric oxide in cystic fibrosis patients with refractory mycobacterium abscessus lung infection. J. Cyst. Fibros. 19, 225–231. doi: 10.1016/j.jcf.2019.05.002
Bernut, A., Dupont, C., Ogryzko, N. V., Neyret, A., Herrmann, J.-L., Floto, R. A., et al. (2019). CFTR protects against mycobacterium abscessus infection by fine-tuning host oxidative defenses. Cell Rep. 26, 1828.e4–1840.e4. doi: 10.1016/j.celrep.2019.01.071
Bernut, A., Dupont, C., Sahuquet, A., Herrmann, J. L., Lutfalla, G., and Kremer, L. (2015). Deciphering and imaging pathogenesis and cording of mycobacterium abscessus in zebrafish embryos. J. Vis. Exp. 103:53130. doi: 10.3791/53130
Bernut, A., Herrmann, J.-L., Kissa, K., Dubremetz, J.-F., Gaillard, J.-L., Lutfalla, G., et al. (2014a). Mycobacterium abscessus cording prevents phagocytosis and promotes abscess formation. Proc. Natl. Acad. Sci. 111, E943–E952. doi: 10.1073/pnas.1321390111
Bernut, A., Herrmann, J.-L., Ordway, D., and Kremer, L. (2017). The diverse cellular and animal models to decipher the physiopathological traits of mycobacterium abscessus infection. Front. Cell. Infect. Microbiol. 7:100. doi: 10.3389/fcimb.2017.00100
Bernut, A., Le Moigne, V., Lesne, T., Lutfalla, G., Herrmann, J.-L., and Kremer, L. (2014b). In vivo assessment of drug efficacy against mycobacterium abscessus using the embryonic zebrafish test system. Antimicrob. Agents Chemother. 58, 4054–4063. doi: 10.1128/AAC.00142-14
Bernut, A., Nguyen-Chi, M., Halloum, I., Herrmann, J.-L., Lutfalla, G., and Kremer, L. (2016a). Mycobacterium abscessus-induced granuloma formation is strictly dependent on TNF signaling and neutrophil trafficking. PLoS Pathog. 12:e1005986. doi: 10.1371/journal.ppat.1005986
Bernut, A., Viljoen, A., Dupont, C., Sapriel, G., Blaise, M., Bouchier, C., et al. (2016b). Insights into the smooth-to-rough transitioning in mycobacterium bolletii unravels a functional Tyr residue conserved in all mycobacterial MmpL family members. Mol. Microbiol. 99, 866–883. doi: 10.1111/mmi.13283
Brisse, M., Vrba, S. M., Kirk, N., Liang, Y., and Ly, H. (2020). Emerging concepts and technologies in vaccine development. Front. Immunol. 11:583077. doi: 10.3389/fimmu.2020.583077
Brown-Elliott, B. A., and Wallace, R. J. (2002). Clinical and taxonomic status of pathogenic nonpigmented or late-pigmenting rapidly growing mycobacteria. Clin. Microbiol. Rev. 15, 716–746. doi: 10.1128/CMR.15.4.716-746.2002
Brunson, D. N., Maldosevic, E., Velez, A., Figgins, E., and Ellis, T. N. (2019). Porin loss in Klebsiella pneumoniae clinical isolates impacts production of virulence factors and survival within macrophages. Int. J. Med. Microbiol. 309, 213–224. doi: 10.1016/j.ijmm.2019.04.001
Bruscia, E. M., and Bonfield, T. L. (2016). Cystic fibrosis lung immunity: the role of the macrophage. J. Innate Immun. 8, 550–563. doi: 10.1159/000446825
Bryant, J. M., Brown, K. P., Burbaud, S., Everall, I., Belardinelli, J. M., Rodriguez-Rincon, D., et al. (2021). Stepwise pathogenic evolution of mycobacterium abscessus. Science 372:eabb8699. doi: 10.1126/science.abb8699
Bryant, J. M., Grogono, D. M., Greaves, D., Foweraker, J., Roddick, I., Inns, T., et al. (2013). Whole-genome sequencing to identify transmission of mycobacterium abscessus between patients with cystic fibrosis: a retrospective cohort study. Lancet 381, 1551–1560. doi: 10.1016/S0140-6736(13)60632-7
Bryant, J. M., Grogono, D. M., Rodriguez-Rincon, D., Everall, I., Brown, K. P., Moreno, P., et al. (2016). Population-level genomics identifies the emergence and global spread of a human transmissible multidrug-resistant nontuberculous mycobacterium. Science 354:751. doi: 10.1126/science.aaf8156
Buchmeier, N., Blanc-Potard, A., Ehrt, S., Piddington, D., Riley, L., and Groisman, E. A. (2000). A parallel intraphagosomal survival strategy shared by mycobacterium tuberculosis and salmonella enterica. Mol. Microbiol. 35, 1375–1382. doi: 10.1046/j.1365-2958.2000.01797.x
Burgess, W., Margolis, A., Gibbs, S., Duarte, R. S., and Jackson, M. (2017). Disinfectant susceptibility profiling of glutaraldehyde-resistant nontuberculous mycobacteria. Infect. Control Hosp. Epidemiol. 38:784. doi: 10.1017/ice.2017.75
Burroughs, M., and Moscona, A. (2000). Immunization of pediatric solid organ transplant candidates and recipients. Clin. Infect. Dis. 30, 857–869. doi: 10.1086/313823
Camilli, A., Goldfine, H., and Portnoy, D. A. (1991). Listeria monocytogenes mutants lacking phosphatidylinositol-specific phospholipase C are avirulent. J. Exp. Med. 173, 751–754. doi: 10.1084/jem.173.3.751
Cantin, A. M., Hartl, D., Konstan, M. W., and Chmiel, J. F. (2015). Inflammation in cystic fibrosis lung disease: pathogenesis and therapy. J. Cyst. Fibros. 14, 419–430. doi: 10.1016/j.jcf.2015.03.003
Caverly, L. J., Caceres, S. M., Fratelli, C., Happoldt, C., Kidwell, K. M., Malcolm, K. C., et al. (2015). Mycobacterium abscessus morphotype comparison in a murine model. PLoS One 10:e0117657. doi: 10.1371/journal.pone.0117657
Chakaya, J., Khan, M., Ntoumi, F., Aklillu, E., Fatima, R., Mwaba, P., et al. (2021). Global tuberculosis report 2020–reflections on the global TB burden, treatment and prevention efforts. Int. J. Infect. Dis. 113(Suppl. 1), S7–S12. doi: 10.1016/j.ijid.2021.02.107
Chalmers, J. D., and Hill, A. T. (2013). Mechanisms of immune dysfunction and bacterial persistence in non-cystic fibrosis bronchiectasis. Mol. Immunol. 55, 27–34. doi: 10.1016/j.molimm.2012.09.011
Chalut, C. (2016). MmpL transporter-mediated export of cell-wall associated lipids and siderophores in mycobacteria. Tuberculosis 100, 32–45. doi: 10.1016/j.tube.2016.06.004
Chen, J. M., German, G. J., Alexander, D. C., Ren, H., Tan, T., and Liu, J. (2006). Roles of Lsr2 in colony morphology and biofilm formation of mycobacterium smegmatis. J. Bacteriol. 188, 633–641. doi: 10.1128/JB.188.2.633-641.2006
Choo, S. W., Wee, W. Y., Ngeow, Y. F., Mitchell, W., Tan, J. L., Wong, G. J., et al. (2014). Genomic reconnaissance of clinical isolates of emerging human pathogen mycobacterium abscessus reveals high evolutionary potential. Sci. Rep. 4, 1–10. doi: 10.1038/srep04061
Ciofu, O., Riis, B., Pressler, T., Poulsen, H. E., and Høiby, N. (2005). Occurrence of hypermutable Pseudomonas aeruginosa in cystic fibrosis patients is associated with the oxidative stress caused by chronic lung inflammation. Antimicrob. Agents Chemother. 49, 2276–2282. doi: 10.1128/AAC.49.6.2276-2282.2005
Cockx, M., Gouwy, M., Van Damme, J., and Struyf, S. (2018). Chemoattractants and cytokines in primary ciliary dyskinesia and cystic fibrosis: key players in chronic respiratory diseases. Cell. Mol. Immunol. 15, 312–323. doi: 10.1038/cmi.2017.118
Colangeli, R., Haq, A., Arcus, V. L., Summers, E., Magliozzo, R., McBride, A., et al. (2009). The multifunctional histone-like protein Lsr2 protects mycobacteria against reactive oxygen intermediates. Proc. Natl. Acad. Sci. 106, 4414–4418. doi: 10.1073/pnas.0810126106
Counoupas, C., Ferrell, K. C., Ashhurst, A., Bhattacharyya, N. D., Nagalingam, G., Stewart, E. L., et al. (2020). Mucosal delivery of a multistage subunit vaccine promotes development of lung-resident memory T cells and affords interleukin-17-dependent protection against pulmonary tuberculosis. NPJ Vaccines 5, 1–12. doi: 10.1038/s41541-020-00255-7
Counoupas, C., Pinto, R., Nagalingam, G., Hill-Cawthorne, G. A., Feng, C. G., Britton, W. J., et al. (2016). Mycobacterium tuberculosis components expressed during chronic infection of the lung contribute to long-term control of pulmonary tuberculosis in mice. NPJ Vaccines 1:16012. doi: 10.1038/npjvaccines.2016.12
Counoupas, C., Triccas, J. A., and Britton, W. J. (2019). Deciphering protective immunity against tuberculosis: implications for vaccine development. Expert Rev. Vaccines 18, 353–364. doi: 10.1080/14760584.2019.1585246
Cronan, M. R., and Tobin, D. M. (2014). Fit for consumption: zebrafish as a model for tuberculosis. Dis. Model. Mech. 7, 777–784. doi: 10.1242/dmm.016089
Cutting, G. R. (2015). Cystic fibrosis genetics: from molecular understanding to clinical application. Nat. Rev. Genet. 16, 45–56. doi: 10.1038/nrg3849
Daher, W., Leclercq, L.-D., Viljoen, A., Karam, J., Dufrêne, Y. F., Guérardel, Y., et al. (2020). O-methylation of the glycopeptidolipid acyl chain defines surface hydrophobicity of mycobacterium abscessus and macrophage invasion. ACS Infect. Dis. 6, 2756–2770. doi: 10.1021/acsinfecdis.0c00490
Dar, H. A., Ismail, S., Waheed, Y., Ahmad, S., Jamil, Z., Aziz, H., et al. (2021). Designing a multi-epitope vaccine against Mycobacteroides abscessus by pangenome-reverse vaccinology. Sci. Rep. 11, 1–18. doi: 10.1038/s41598-021-90868-2
da Silva, J. L., Nguyen, J., Fennelly, K. P., Zelazny, A. M., and Olivier, K. N. (2018). Survival of pathogenic mycobacterium abscessus subsp. massiliense in Acanthamoeba castellanii. Res. Microbiol. 169, 56–60. doi: 10.1016/j.resmic.2017.10.001
Davidson, R. M., Hasan, N. A., Reynolds, P. R., Totten, S., Garcia, B., Levin, A., et al. (2014). Genome sequencing of mycobacterium abscessus isolates from patients in the United States and comparisons to globally diverse clinical strains. J. Clin. Microbiol. 52, 3573–3582. doi: 10.1128/JCM.01144-14
Davidson, L. B., Nessar, R., Kempaiah, P., Perkins, D. J., and Byrd, T. F. (2011). Mycobacterium abscessus glycopeptidolipid prevents respiratory epithelial TLR2 signaling as measured by HβD2 gene expression and IL-8 release. PLoS One 6:e29148. doi: 10.1371/journal.pone.0029148
Dedrick, R. M., Aull, H. G., Jacobs-Sera, D., Garlena, R. A., Russell, D. A., Smith, B. E., et al. (2021). The prophage and plasmid mobilome as a likely driver of mycobacterium abscessus diversity. MBio 12, e03441–e03420. doi: 10.1128/mBio.03441-20
Delafont, V., Mougari, F., Cambau, E., Joyeux, M., Bouchon, D., Héchard, Y., et al. (2014). First evidence of amoebae–mycobacteria association in drinking water network. Environ. Sci. Technol. 48, 11872–11882. doi: 10.1021/es5036255
de Moura, V. C., Verma, D., Everall, I., Brown, K. P., Belardinelli, J. M., Shanley, C., et al. (2021). Increased virulence of outer membrane porin mutants of mycobacterium abscessus. Front. Microbiol. 12:706207. doi: 10.3389/fmicb.2021.706207
Deshayes, C., Bach, H., Euphrasie, D., Attarian, R., Coureuil, M., Sougakoff, W., et al. (2010). MmpS4 promotes glycopeptidolipids biosynthesis and export in mycobacterium smegmatis. Mol. Microbiol. 78, 989–1003. doi: 10.1111/j.1365-2958.2010.07385.x
Downey, D., Bell, S., and Elborn, J. (2009). Neutrophils in cystic fibrosis. Thorax 64, 81–88. doi: 10.1136/thx.2007.082388
Doyle, R. M., Rubio, M., Dixon, G., Hartley, J., Klein, N., Coll, P., et al. (2020). Cross-transmission is not the source of new mycobacterium abscessus infections in a multicenter cohort of cystic fibrosis patients. Clin. Infect. Dis. 70, 1855–1864. doi: 10.1093/cid/ciz526
Dubois, V., Pawlik, A., Bories, A., Le Moigne, V., Sismeiro, O., Legendre, R., et al. (2019). Mycobacterium abscessus virulence traits unraveled by transcriptomic profiling in amoeba and macrophages. PLoS Pathog. 15:e1008069. doi: 10.1371/journal.ppat.1008069
Dubois, V., Viljoen, A., Laencina, L., Le Moigne, V., Bernut, A., Dubar, F., et al. (2018). MmpL8(MAB) controls mycobacterium abscessus virulence and production of a previously unknown glycolipid family. Proc. Natl. Acad. Sci. U. S. A. 115, E10147–E10156. doi: 10.1073/pnas.1812984115
Dumas, E., Christina Boritsch, E., Vandenbogaert, M., Rodríguez de la Vega, R. C., Thiberge, J.-M., Caro, V., et al. (2016). Mycobacterial pan-genome analysis suggests important role of plasmids in the radiation of type VII secretion systems. Genome Biol. Evol. 8, 387–402. doi: 10.1093/gbe/evw001
Enriquez, A. B., Izzo, A., Miller, S. M., Stewart, E. L., Mahon, R. N., Frank, D. J., et al. (2021). Advancing adjuvants for mycobacterium tuberculosis therapeutics. Front. Immunol. 12:740117. doi: 10.3389/fimmu.2021.740117
Esther, C. R. Jr., Esserman, D. A., Gilligan, P., Kerr, A., and Noone, P. G. (2010). Chronic mycobacterium abscessus infection and lung function decline in cystic fibrosis. J. Cyst. Fibros. 9, 117–123. doi: 10.1016/j.jcf.2009.12.001
Fabrino, D. L., Bleck, C. K., Anes, E., Hasilik, A., Melo, R. C., Niederweis, M., et al. (2009). Porins facilitate nitric oxide-mediated killing of mycobacteria. Microbes Infect. 11, 868–875. doi: 10.1016/j.micinf.2009.05.007
Facchini, M., De Fino, I., Riva, C., and Bragonzi, A. (2014). Long term chronic Pseudomonas aeruginosa airway infection in mice. J. Vis. Exp. 85:51019. doi: 10.3791/51019
Falkinham, J. O. (2009). Surrounded by mycobacteria: nontuberculous mycobacteria in the human environment. J. Appl. Microbiol. 107, 356–367. doi: 10.1111/j.1365-2672.2009.04161.x
Fatima, S., Kumari, A., Das, G., and Dwivedi, V. P. (2020). Tuberculosis vaccine: a journey from BCG to present. Life Sci. 252:117594. doi: 10.1016/j.lfs.2020.117594
Feng, Z., Bai, X., Wang, T., Garcia, C., Bai, A., Li, L., et al. (2020). Differential responses by human macrophages to infection with mycobacterium tuberculosis and non-tuberculous mycobacteria. Front. Microbiol. 11:116. doi: 10.3389/fmicb.2020.00116
Ferrell, K. C., Stewart, E. L., Counoupas, C., Ashhurst, T. M., Britton, W. J., Petrovsky, N., et al. (2021). Intrapulmonary vaccination with delta-inulin adjuvant stimulates non-polarised chemotactic signalling and diverse cellular interaction. Mucosal Immunol. 14, 762–773. doi: 10.1038/s41385-021-00379-6
Folkesson, A., Jelsbak, L., Yang, L., Johansen, H. K., Ciofu, O., Høiby, N., et al. (2012). Adaptation of Pseudomonas aeruginosa to the cystic fibrosis airway: an evolutionary perspective. Nat. Rev. Microbiol. 10, 841–851. doi: 10.1038/nrmicro2907
Fothergill, J. L., Walshaw, M. J., and Winstanley, C. (2012). Transmissible strains of Pseudomonas aeruginosa in cystic fibrosis lung infections. Eur. Respir. J. 40, 227–238. doi: 10.1183/09031936.00204411
Ganbat, D., Seehase, S., Richter, E., Vollmer, E., Reiling, N., Fellenberg, K., et al. (2016). Mycobacteria infect different cell types in the human lung and cause species dependent cellular changes in infected cells. BMC Pulm. Med. 16, 1–16. doi: 10.1186/s12890-016-0185-5
Ghannoum, M. A. (2000). Potential role of phospholipases in virulence and fungal pathogenesis. Clin. Microbiol. Rev. 13, 122–143. doi: 10.1128/CMR.13.1.122
Gifford, A. M., and Chalmers, J. D. (2014). The role of neutrophils in cystic fibrosis. Curr. Opin. Hematol. 21, 16–22. doi: 10.1097/MOH.0000000000000009
Gordon, B. R., Li, Y., Wang, L., Sintsova, A., Van Bakel, H., Tian, S., et al. (2010). Lsr2 is a nucleoid-associated protein that targets AT-rich sequences and virulence genes in mycobacterium tuberculosis. Proc. Natl. Acad. Sci. 107, 5154–5159. doi: 10.1073/pnas.0913551107
Griffith, D. E., Aksamit, T., Brown-Elliott, B. A., Catanzaro, A., Daley, C., Gordin, F., et al. (2007). An official ATS/IDSA statement: diagnosis, treatment, and prevention of nontuberculous mycobacterial diseases. Am. J. Respir. Crit. Care Med. 175, 367–416. doi: 10.1164/rccm.200604-571ST
Grode, L., Ganoza, C. A., Brohm, C., Weiner, J., Eisele, B., and Kaufmann, S. H. (2013). Safety and immunogenicity of the recombinant BCG vaccine VPM1002 in a phase 1 open-label randomized clinical trial. Vaccine 31, 1340–1348. doi: 10.1016/j.vaccine.2012.12.053
Grode, L., Seiler, P., Baumann, S., Hess, J., Brinkmann, V., Eddine, A. N., et al. (2005). Increased vaccine efficacy against tuberculosis of recombinant Mycobacterium bovis bacille Calmette-Guerin mutants that secrete listeriolysin. J. Clin. Invest. 115, 2472–2479. doi: 10.1172/JCI24617
Gröschel, M. I., Sayes, F., Simeone, R., Majlessi, L., and Brosch, R. (2016). ESX secretion systems: mycobacterial evolution to counter host immunity. Nat. Rev. Microbiol. 14, 677–691. doi: 10.1038/nrmicro.2016.131
Guilbault, C., Martin, P., Houle, D., Boghdady, M., Guiot, M., Marion, D., et al. (2005). Cystic fibrosis lung disease following infection with Pseudomonas aeruginosa in Cftr knockout mice using novel non-invasive direct pulmonary infection technique. Lab. Anim. 39, 336–352. doi: 10.1258/0023677054306944
Guilbault, C., Saeed, Z., Downey, G. P., and Radzioch, D. (2007). Cystic fibrosis mouse models. Am. J. Respir. Cell Mol. Biol. 36, 1–7. doi: 10.1165/rcmb.2006-0184TR
Guimarães, T., Chimara, E., do Prado, G. V. B., Ferrazoli, L., NGF, C., dos Santos Simeão, F. C., et al. (2016). Pseudooutbreak of rapidly growing mycobacteria due to mycobacterium abscessus subsp bolletii in a digestive and respiratory endoscopy unit caused by the same clone as that of a countrywide outbreak. Am. J. Infect. Control 44, e221–e226. doi: 10.1016/j.ajic.2016.06.019
Gupta, R. S., Lo, B., and Son, J. (2018). Phylogenomics and comparative genomic studies robustly support division of the genus mycobacterium into an emended genus mycobacterium and four novel genera. Front. Microbiol. 9:67. doi: 10.3389/fmicb.2018.00067
Gutiérrez, A. V., Richard, M., Roquet-Banères, F., Viljoen, A., and Kremer, L. (2019). The TetR family transcription factor MAB_2299c regulates the expression of two distinct MmpS-MmpL efflux pumps involved in cross-resistance to clofazimine and bedaquiline in mycobacterium abscessus. Antimicrob. Agents Chemother. 63, e01000–e01019. doi: 10.1128/AAC.01000-19
Gutiérrez, A. V., Viljoen, A., Ghigo, E., Herrmann, J.-L., and Kremer, L. (2018). Glycopeptidolipids, a double-edged sword of the mycobacterium abscessus complex. Front. Microbiol. 9:1145. doi: 10.3389/fmicb.2018.01145
Halloum, I., Carrere-Kremer, S., Blaise, M., Viljoen, A., Bernut, A., Le Moigne, V., et al. (2016). Deletion of a dehydratase important for intracellular growth and cording renders rough mycobacterium abscessus avirulent. Proc. Natl. Acad. Sci. U. S. A. 113, E4228–E4237. doi: 10.1073/pnas.1605477113
Halloum, I., Viljoen, A., Khanna, V., Craig, D., Bouchier, C., Brosch, R., et al. (2017). Resistance to thiacetazone derivatives active against mycobacterium abscessus involves mutations in the MmpL5 transcriptional repressor MAB_4384. Antimicrob. Agents Chemother. 61:e02509-16. doi: 10.1128/AAC.02509-16
Harrison, F. (2007). Microbial ecology of the cystic fibrosis lung. Microbiology 153, 917–923. doi: 10.1099/mic.0.2006/004077-0
Hartl, D., Gaggar, A., Bruscia, E., Hector, A., Marcos, V., Jung, A., et al. (2012). Innate immunity in cystic fibrosis lung disease. J. Cyst. Fibros. 11, 363–382. doi: 10.1016/j.jcf.2012.07.003
Heinson, A. I., Woelk, C. H., and Newell, M.-L. (2015). The promise of reverse vaccinology. Int. Health 7, 85–89. doi: 10.1093/inthealth/ihv002
Honda, J. R., Hess, T., Carlson, R., Kandasamy, P., Nieto Ramirez, L. M., Norton, G. J., et al. (2020). Nontuberculous mycobacteria show differential infectivity and use phospholipids to antagonize LL-37. Am. J. Respir. Cell Mol. Biol. 62, 354–363. doi: 10.1165/rcmb.2018-0278OC
Honda, J. R., Hess, T., Malcolm, K. C., Ovrutsky, A. R., Bai, X., Irani, V. R., et al. (2015). Pathogenic nontuberculous mycobacteria resist and inactivate cathelicidin: implication of a novel role for polar mycobacterial lipids. PLoS One 10:e0126994. doi: 10.1371/journal.pone.0126994
Horsburgh, C. R. Jr., Hanson, D. L., Jones, J. L., and Thompson, S. E. III (1996). Protection from Mycobacterium avium complex disease in human immunodeficiency virus-infected persons with a history of tuberculosis. J. Infect. Dis. 174, 1212–1217. doi: 10.1093/infdis/174.6.1212
Houben, E. N., Korotkov, K. V., and Bitter, W. (2014). Take five—type VII secretion systems of mycobacteria. Biochim. Biophys. Acta 1843, 1707–1716. doi: 10.1016/j.bbamcr.2013.11.003
Howard, S. T., Rhoades, E., Recht, J., Pang, X., Alsup, A., Kolter, R., et al. (2006). Spontaneous reversion of mycobacterium abscessus from a smooth to a rough morphotype is associated with reduced expression of glycopeptidolipid and reacquisition of an invasive phenotype. Microbiology 152, 1581–1590. doi: 10.1099/mic.0.28625-0
Hunt-Serracin, A. C., Parks, B. J., Boll, J., and Boutte, C. C. (2019). Mycobacterium abscessus cells have altered antibiotic tolerance and surface glycolipids in artificial cystic fibrosis sputum medium. Antimicrob. Agents Chemother. 63:e02488-18. doi: 10.1128/AAC.02488-18
Jackson, M. (2014). The mycobacterial cell envelope—lipids. Cold Spring Harb. Perspect. Med. 4:a021105. doi: 10.1101/cshperspect.a021105
Jeon, B.-Y., Kwak, J., Lee, S.-S., Cho, S., Won, C. J., Kim, J. M., et al. (2009). Comparative analysis of immune responses to mycobacterium abscessus infection and its antigens in two murine models. J. Microbiol. 47:633. doi: 10.1007/s12275-009-0139-1
Jeong, Y. J., Lee, K. S., Koh, W.-J., Han, J., Kim, T. S., and Kwon, O. J. (2004). Nontuberculous mycobacterial pulmonary infection in immunocompetent patients: comparison of thin-section CT and histopathologic findings. Radiology 231, 880–886. doi: 10.1148/radiol.2313030833
Jimenez, P. N., Koch, G., Thompson, J. A., Xavier, K. B., Cool, R. H., and Quax, W. J. (2012). The multiple signaling systems regulating virulence in Pseudomonas aeruginosa. Microbiol. Mol. Biol. Rev. 76, 46–65. doi: 10.1128/MMBR.05007-11
Johansen, M. D., Herrmann, J.-L., and Kremer, L. (2020). Non-tuberculous mycobacteria and the rise of mycobacterium abscessus. Nat. Rev. Microbiol. 18, 392–407. doi: 10.1038/s41579-020-0331-1
Jönsson, B. E., Gilljam, M., Lindblad, A., Ridell, M., Wold, A. E., and Welinder-Olsson, C. (2007). Molecular epidemiology of mycobacterium abscessus, with focus on cystic fibrosis. J. Clin. Microbiol. 45, 1497–1504. doi: 10.1128/JCM.02592-06
Karakousis, P. C., Bishai, W. R., and Dorman, S. E. (2004). Mycobacterium tuberculosis cell envelope lipids and the host immune response. Cell. Microbiol. 6, 105–116. doi: 10.1046/j.1462-5822.2003.00351.x
Kartalija, M., Ovrutsky, A. R., Bryan, C. L., Pott, G. B., Fantuzzi, G., Thomas, J., et al. (2013). Patients with nontuberculous mycobacterial lung disease exhibit unique body and immune phenotypes. Am. J. Respir. Crit. Care Med. 187, 197–205. doi: 10.1164/rccm.201206-1035OC
Kim, B.-R., Kim, B.-J., Kook, Y.-H., and Kim, B.-J. (2019). Phagosome escape of rough mycobacterium abscessus strains in murine macrophage via phagosomal rupture can lead to type I interferon production and their cell-to-cell spread. Front. Immunol. 10:125. doi: 10.3389/fimmu.2019.00125
Kim, T. S., Kim, Y. S., Yoo, H., Park, Y. K., and Jo, E.-K. (2014a). Mycobacterium massiliense induces inflammatory responses in macrophages through toll-like receptor 2 and c-Jun N-terminal kinase. J. Clin. Immunol. 34, 212–223. doi: 10.1007/s10875-013-9978-y
Kim, S.-Y., Koh, W.-J., Kim, Y. H., Jeong, B.-H., Park, H. Y., Jeon, K., et al. (2014b). Importance of reciprocal balance of T cell immunity in mycobacterium abscessus complex lung disease. PLoS One 9:e109941. doi: 10.1371/journal.pone.0111352
Kim, Y. S., Yang, C.-S., Nguyen, L. T., Kim, J. K., Jin, H. S., ho Choe, J., et al. (2017). Mycobacterium abscessus ESX-3 plays an important role in host inflammatory and pathological responses during infection. Microbes Infect. 19, 5–17. doi: 10.1016/j.micinf.2016.09.001
Knudsen, N. P. H., Olsen, A., Buonsanti, C., Follmann, F., Zhang, Y., Coler, R. N., et al. (2016). Different human vaccine adjuvants promote distinct antigen-independent immunological signatures tailored to different pathogens. Sci. Rep. 6:19570, doi: 10.1038/srep19570
Koh, W.-J., Jeong, B.-H., Kim, S.-Y., Jeon, K., Park, K. U., Jhun, B. W., et al. (2017). Mycobacterial characteristics and treatment outcomes in mycobacterium abscessus lung disease. Clin. Infect. Dis. 64, 309–316. doi: 10.1093/cid/ciw724
Koh, W., Stout, J., and Yew, W. (2014). Advances in the management of pulmonary disease due to mycobacterium abscessus complex. Int. J. Tuberc. Lung Dis. 18, 1141–1148. doi: 10.5588/ijtld.14.0134
Kreutzfeldt, K. M., McAdam, P. R., Claxton, P., Holmes, A., Seagar, A. L., Laurenson, I. F., et al. (2013). Molecular longitudinal tracking of mycobacterium abscessus spp. during chronic infection of the human lung. PLoS One 8:e63237. doi: 10.1371/journal.pone.0063237
Küssau, T., Van Wyk, N., Johansen, M. D., Alsarraf, H. M., Neyret, A., Hamela, C., et al. (2020). Functional characterization of the N-acetylmuramyl-l-alanine amidase, Ami1, from mycobacterium abscessus. Cell 9:2410. doi: 10.3390/cells9112410
Kwak, N., Dalcolmo, M. P., Daley, C. L., Eather, G., Gayoso, R., Hasegawa, N., et al. (2019). Mycobacterium abscessus pulmonary disease: individual patient data meta-analysis. Eur. Respir. J. 54:1801991. doi: 10.1183/13993003.01991-2018
Kwon, Y. H., Lee, G.-Y., Kim, W.-S., and Kim, K. J. (2009). A case of skin and soft tissue infection caused by mycobacterium abscessus. Ann. Dermatol. 21, 84–87. doi: 10.5021/ad.2009.21.1.84
Laencina, L., Dubois, V., Le Moigne, V., Viljoen, A., Majlessi, L., Pritchard, J., et al. (2018). Identification of genes required for mycobacterium abscessus growth in vivo with a prominent role of the ESX-4 locus. Proc. Natl. Acad. Sci. U. S. A. 115, E1002–E1011. doi: 10.1073/pnas.1713195115
Lagune, M., Petit, C., Sotomayor, F. V., Johansen, M. D., Beckham, K. S., Ritter, C., et al. (2021). Conserved and specialized functions of type VII secretion systems in non-tuberculous mycobacteria. Microbiology 167:001054. doi: 10.1099/mic.0.001054
Lake, M. A., Ambrose, L. R., Lipman, M. C., and Lowe, D. M. (2016). “Why me, why now?” using clinical immunology and epidemiology to explain who gets nontuberculous mycobacterial infection. BMC Med. 14, 1–13. doi: 10.1186/s12916-016-0606-6
Lammas, D. A., De Heer, E., Edgar, J., Novelli, V., Ben-Smith, A., Baretto, R., et al. (2002). Heterogeneity in the granulomatous response to mycobacterial infection in patients with defined genetic mutations in the interleukin 12-dependent interferon-gamma production pathway. Int. J. Exp. Pathol. 83, 1–20. doi: 10.1046/j.1365-2613.2002.00216.x
Law, R. J., Hamlin, J. N., Sivro, A., McCorrister, S. J., Cardama, G. A., and Cardona, S. T. (2008). A functional phenylacetic acid catabolic pathway is required for full pathogenicity of Burkholderia cenocepacia in the Caenorhabditis elegans host model. J. Bacteriol. 190, 7209–7218. doi: 10.1128/JB.00481-08
Le Chevalier, F., Cascioferro, A., Frigui, W., Pawlik, A., Boritsch, E. C., Bottai, D., et al. (2015). Revisiting the role of phospholipases C in virulence and the lifecycle of mycobacterium tuberculosis. Sci. Rep. 5:16918. doi: 10.1038/srep16918
Le Moigne, V., Belon, C., Goulard, C., Accard, G., Bernut, A., Pitard, B., et al. (2016). MgtC as a host-induced factor and vaccine candidate against mycobacterium abscessus infection. Infect. Immun. 84, 2895–2903. doi: 10.1128/IAI.00359-16
Le Moigne, V., Bernut, A., Cortès, M., Viljoen, A., Dupont, C., Pawlik, A., et al. (2019). Lsr2 is an important determinant of intracellular growth and virulence in mycobacterium abscessus. Front. Microbiol. 10:905. doi: 10.3389/fmicb.2019.00905
Le Moigne, V., Rottman, M., Goulard, C., Barteau, B., Poncin, I., Soismier, N., et al. (2015). Bacterial phospholipases C as vaccine candidate antigens against cystic fibrosis respiratory pathogens: the Mycobacteriurn abscessus model. Vaccine 33, 2118–2124. doi: 10.1016/j.vaccine.2015.03.030
Lee, J., Kumar, S. A., Jhan, Y. Y., and Bishop, C. J. (2018). Engineering DNA vaccines against infectious diseases. Acta Biomater. 80, 31–47. doi: 10.1016/j.actbio.2018.08.033
Lee, J.-W., and Lee, E.-J. (2015). Regulation and function of the salmonella MgtC virulence protein. J. Microbiol. 53, 667–672. doi: 10.1007/s12275-015-5283-1
Lee, J.-Y., Lee, M.-S., Kim, D.-J., Yang, S.-J., Lee, S.-J., Noh, E.-J., et al. (2017). Nucleotide-binding oligomerization domain 2 contributes to limiting growth of mycobacterium abscessus in the lung of mice by regulating cytokines and nitric oxide production. Front. Immunol. 8:1477. doi: 10.3389/fimmu.2017.01477
Lee, S. J., Shin, S. J., Lee, S. J., Lee, M. H., Kang, T. H., Noh, K. T., et al. (2014). Mycobacterium abscessus MAB2560 induces maturation of dendritic cells via toll-like receptor 4 and drives Th1 immune response. BMB Rep. 47, 512–517. doi: 10.5483/BMBRep.2014.47.9.001
Lerat, I., Cambau, E., Roth dit Bettoni, R., Gaillard, J.-L., Jarlier, V., Truffot, C., et al. (2014). In vivo evaluation of antibiotic activity against mycobacterium abscessus. J. Infect. Dis. 209, 905–912. doi: 10.1093/infdis/jit614
Lewin, A, Kamal, E, Semmler, T, Winter, K, Kaiser, S, Schäfer, H., et al. (2021). Genetic diversification of persistent mycobacterium abscessus within cystic fibrosis patients. Virulence 12, 2415–2429. doi: 10.1080/21505594.2021.1959808
Li, B., Ye, M., Zhao, L., Guo, Q., Chen, J., Xu, B., et al. (2020). Glycopeptidolipid genotype correlates with the severity of mycobacterium abscessus lung disease. J. Infect. Dis. 221(Supplement_2), S257–S262. doi: 10.1093/infdis/jiz475
Liu, J., and Gordon, B. R. (2012). Targeting the global regulator Lsr2 as a novel approach for anti-tuberculosis drug development. Expert Rev. Anti-Infect. Ther. 10, 1049–1053. doi: 10.1586/eri.12.86
Liu, T.-Y., Tsai, S.-H., Chen, J.-W., Wang, Y.-C., Hu, S.-T., and Chen, Y.-Y. (2021). Mab_3083c is a homologue of RNase J and plays a role in colony morphotype, aggregation, and sliding motility of mycobacterium abscessus. Microorganisms 9:676. doi: 10.3390/microorganisms9040676
Llorens-Fons, M., Pérez-Trujillo, M., Julián, E., Brambilla, C., Alcaide, F., Byrd, T. F., et al. (2017). Trehalose polyphleates, external cell wall lipids in mycobacterium abscessus, are associated with the formation of clumps with cording morphology, which have been associated with virulence. Front. Microbiol. 8:1402. doi: 10.3389/fmicb.2017.01402
Loutet, S. A., and Valvano, M. A. (2010). A decade of Burkholderia cenocepacia virulence determinant research. Infect. Immun. 78, 4088–4100. doi: 10.1128/IAI.00212-10
Lutzky, V. P., Ratnatunga, C. N., Smith, D. J., Kupz, A., Doolan, D. L., Reid, D. W., et al. (2018). Anomalies in T cell function are associated with individuals at risk of mycobacterium abscessus complex infection. Front. Immunol. 9:1319. doi: 10.3389/fimmu.2018.01319
Maggioncalda, E. C., Story-Roller, E., Mylius, J., Illei, P., Basaraba, R. J., and Lamichhane, G. (2020). A mouse model of pulmonary Mycobacteroides abscessus infection. Sci. Rep. 10, 1–8. doi: 10.1038/s41598-020-60452-1
Mahenthiralingam, E. (2014). Emerging cystic fibrosis pathogens and the microbiome. Paediatr. Respir. Rev. 15, 13–15. doi: 10.1016/j.prrv.2014.04.006
Malcolm, K. C., Caceres, S. M., Pohl, K., Poch, K. R., Bernut, A., Kremer, L., et al. (2018). Neutrophil killing of mycobacterium abscessus by intra- and extracellular mechanisms. PLoS One 13:e0196120. doi: 10.1371/journal.pone.0196120
Malcolm, K. C., Nichols, E. M., Caceres, S. M., Kret, J. E., Martiniano, S. L., Sagel, S. D., et al. (2013). Mycobacterium abscessus induces a limited pattern of neutrophil activation that promotes pathogen survival. PLoS One 8:e57402. doi: 10.1371/journal.pone.0057402
Malfroot, A., Adam, G., Ciofu, O., Döring, G., Knoop, C., Lang, A. B., et al. (2005). Immunisation in the current management of cystic fibrosis patients. J. Cyst. Fibros. 4, 77–87. doi: 10.1016/j.jcf.2004.10.003
Maloney, K. E., and Valvano, M. A. (2006). The mgtC gene of Burkholderia cenocepacia is required for growth under magnesium limitation conditions and intracellular survival in macrophages. Infect. Immun. 74, 5477–5486. doi: 10.1128/IAI.00798-06
Mangtani, P., Abubakar, I., Ariti, C., Beynon, R., Pimpin, L., Fine, P. E., et al. (2014). Protection by BCG vaccine against tuberculosis: a systematic review of randomized controlled trials. Clin. Infect. Dis. 58, 470–480. doi: 10.1093/cid/cit790
Marras, T. K., Chedore, P., Ying, A. M., and Jamieson, F. (2007). Isolation prevalence of pulmonary non-tuberculous mycobacteria in Ontario, 1997–2003. Thorax 62, 661–666. doi: 10.1136/thx.2006.070797
Martina, P., Feliziani, S., Juan, C., Bettiol, M., Gatti, B., Yantorno, O., et al. (2014). Hypermutation in Burkholderia cepacia complex is mediated by DNA mismatch repair inactivation and is highly prevalent in cystic fibrosis chronic respiratory infection. Int. J. Med. Microbiol. 304, 1182–1191. doi: 10.1016/j.ijmm.2014.08.011
Martiniano, S. L., Nick, J. A., and Daley, C. L. (2019). Nontuberculous mycobacterial infections in cystic fibrosis. Thorac. Surg. Clin. 29, 95–108. doi: 10.1016/j.thorsurg.2018.09.008
McIlroy, D., Barteau, B., Cany, J., Richard, P., Gourden, C., Conchon, S., et al. (2009). DNA/amphiphilic block copolymer nanospheres promote low-dose DNA vaccination. Mol. Ther. 17, 1473–1481. doi: 10.1038/mt.2009.84
McIvor, A., Koornhof, H., and Kana, B. D. (2017). Relapse, re-infection and mixed infections in tuberculosis disease. Pathog. Dis. 75:3. doi: 10.1093/femspd/ftx020
McMorran, B., Palmer, J., Lunn, D., Oceandy, D., Costelloe, E., Thomas, G., et al. (2001). G551D CF mice display an abnormal host response and have impaired clearance of pseudomonas lung disease. Am. J. Phys. Lung Cell. Mol. Phys. 281, L740–L747. doi: 10.1152/ajplung.2001.281.3.L740
Medjahed, H., and Reyrat, J.-M. (2009). Construction of mycobacterium abscessus defined glycopeptidolipid mutants: comparison of genetic tools. Appl. Environ. Microbiol. 75, 1331–1338. doi: 10.1128/AEM.01914-08
Meir, M., Grosfeld, T., and Barkan, D. (2018). Establishment and validation of galleria mellonella as a novel model organism to study mycobacterium abscessus infection, Pathogenesis, and Treatment. Antimicrob. Agents Chemother. 62, e02539–e025317. doi: 10.1128/AAC.02539-17
Melly, G., and Purdy, G. E. (2019). MmpL proteins in physiology and pathogenesis of M. tuberculosis. Microorganisms 7:70. doi: 10.3390/microorganisms7030070
Mirsaeidi, M., Hadid, W., Ericsoussi, B., Rodgers, D., and Sadikot, R. T. (2013). Non-tuberculous mycobacterial disease is common in patients with non-cystic fibrosis bronchiectasis. Int. J. Infect. Dis. 17, e1000–e1004. doi: 10.1016/j.ijid.2013.03.018
Mueller, C., Braag, S. A., Keeler, A., Hodges, C., Drumm, M., and Flotte, T. R. (2011). Lack of cystic fibrosis transmembrane conductance regulator in CD3+ lymphocytes leads to aberrant cytokine secretion and hyperinflammatory adaptive immune responses. Am. J. Respir. Cell Mol. Biol. 44, 922–929. doi: 10.1165/rcmb.2010-0224OC
Mulcahy, E. M., Hudson, J. B., Beggs, S. A., Reid, D. W., Roddam, L. F., and Cooley, M. A. (2015). High peripheral blood th17 percent associated with poor lung function in cystic fibrosis. PLoS One 10:e0120912. doi: 10.1371/journal.pone.0120912
Müller, A., Günther, D., Düx, F., Naumann, M., Meyer, T. F., and Rudel, T. (1999). Neisserial porin (PorB) causes rapid calcium influx in target cells and induces apoptosis by the activation of cysteine proteases. EMBO J. 18, 339–352. doi: 10.1093/emboj/18.2.339
Nessar, R., Cambau, E., Reyrat, J. M., Murray, A., and Gicquel, B. (2012). Mycobacterium abscessus: a new antibiotic nightmare. J. Antimicrob. Chemother. 67, 810–818. doi: 10.1093/jac/dkr578
Nessar, R., Reyrat, J.-M., Davidson, L. B., and Byrd, T. F. (2011). Deletion of the mmpL4b gene in the mycobacterium abscessus glycopeptidolipid biosynthetic pathway results in loss of surface colonization capability, but enhanced ability to replicate in human macrophages and stimulate their innate immune response. Microbiology 157, 1187–1195. doi: 10.1099/mic.0.046557-0
N’Goma, J. C. B., Le Moigne, V., Soismier, N., Laencina, L., Le Chevalier, F., Roux, A.-L., et al. (2015). Mycobacterium abscessus phospholipase C expression is induced during coculture within amoebae and enhances M. abscessus virulence in mice. Infect. Immun. 83, 780–791. doi: 10.1128/IAI.02032-14
Oberley-Deegan, R. E., Rebits, B. W., Weaver, M. R., Tollefson, A. K., Bai, X., McGibney, M., et al. (2010). An oxidative environment promotes growth of mycobacterium abscessus. Free Radic. Biol. Med. 49, 1666–1673. doi: 10.1016/j.freeradbiomed.2010.08.026
Obregón-Henao, A., Arnett, K. A., Henao-Tamayo, M., Massoudi, L., Creissen, E., Andries, K., et al. (2015). Susceptibility of mycobacterium abscessus to antimycobacterial drugs in preclinical models. Antimicrob. Agents Chemother. 59, 6904–6912. doi: 10.1128/AAC.00459-15
Okazaki, A., Takato, H., Ohkura, N., Katayama, N., Kasahara, K., and Fujimura, M. (2013). Successful treatment with chemotherapy and corticosteroids of pulmonary mycobacterium abscessus infection accompanied by pleural effusion. J. Infect. Chemother. 19, 964–968. doi: 10.1007/s10156-012-0539-8
Ordway, D., Henao-Tamayo, M., Smith, E., Shanley, C., Harton, M., Troudt, J., et al. (2008). Animal model of mycobacterium abscessus lung infection. J. Leukoc. Biol. 83, 1502–1511. doi: 10.1189/jlb.1007696
Ostroff, R., Wretlind, B., and Vasil, M. (1989). Mutations in the hemolytic-phospholipase C operon result in decreased virulence of Pseudomonas aeruginosa PAO1 grown under phosphate-limiting conditions. Infect. Immun. 57, 1369–1373. doi: 10.1128/iai.57.5.1369-1373.1989
Ottenhoff, T. H., and Kaufmann, S. H. (2012). Vaccines against tuberculosis: where are we and where do we need to go? PLoS Pathog. 8:e1002607. doi: 10.1371/journal.ppat.1002607
Palčeková, Z., Gilleron, M., Angala, S. K., Belardinelli, J. M., McNeil, M., Bermudez, L. E., et al. (2020). Polysaccharide succinylation enhances the intracellular survival of mycobacterium abscessus. ACS Infect. Dis. 6, 2235–2248. doi: 10.1021/acsinfecdis.0c00361
Panagea, S., Winstanley, C., Parsons, Y. N., Walshaw, M. J., Ledson, M. J., and Hart, C. A. (2003). PCR-based detection of a cystic fibrosis epidemic strain of Pseudomonas aeruginosa. Mol. Diagn. 7, 195–200. doi: 10.1007/BF03260038
Park, I. K., Hsu, A. P., Tettelin, H., Shallom, S. J., Drake, S. K., Ding, L., et al. (2015). Clonal diversification and changes in lipid traits and colony morphology in mycobacterium abscessus clinical isolates. J. Clin. Microbiol. 53, 3438–3447. doi: 10.1128/JCM.02015-15
Pasca, M. R., Guglierame, P., De Rossi, E., Zara, F., and Riccardi, G. (2005). mmpL7 gene of mycobacterium tuberculosis is responsible for isoniazid efflux in mycobacterium smegmatis. Antimicrob. Agents Chemother. 49, 4775–4777. doi: 10.1128/AAC.49.11.4775-4777.2005
Pasipanodya, J. G., Ogbonna, D., Ferro, B. E., Magombedze, G., Srivastava, S., Deshpande, D., et al. (2017). Systematic review and meta-analyses of the effect of chemotherapy on pulmonary mycobacterium abscessus outcomes and disease recurrence. Antimicrob. Agents Chemother. 61, e01206–e01217. doi: 10.1128/AAC.01206-17
Pawlik, A., Garnier, G., Orgeur, M., Tong, P., Lohan, A., Le Chevalier, F., et al. (2013). Identification and characterization of the genetic changes responsible for the characteristic smooth-to-rough morphotype alterations of clinically persistent mycobacterium abscessus. Mol. Microbiol. 90, 612–629. doi: 10.1111/mmi.12387
Perdomo, C., Zedler, U., Kühl, A. A., Lozza, L., Saikali, P., Sander, L. E., et al. (2016). Mucosal BCG vaccination induces protective lung-resident memory T cell populations against tuberculosis. MBio 7, e01686–e01616. doi: 10.1128/mBio.01686-16
Pinto, R., Leotta, L., Shanahan, E. R., West, N. P., Leyh, T. S., Britton, W., et al. (2013). Host cell–induced components of the sulfate assimilation pathway are major protective antigens of mycobacterium tuberculosis. J. Infect. Dis. 207, 778–785. doi: 10.1093/infdis/jis751
Polverino, F., Lu, B., Quintero, J. R., Vargas, S. O., Patel, A. S., Owen, C. A., et al. (2019). CFTR regulates B cell activation and lymphoid follicle development. Respir. Res. 20, 1–13. doi: 10.1186/s12931-019-1103-1
Qvist, T., Gilljam, M., Jönsson, B., Taylor-Robinson, D., Jensen-Fangel, S., Wang, M., et al. (2015). Epidemiology of nontuberculous mycobacteria among patients with cystic fibrosis in Scandinavia. J. Cyst. Fibros. 14, 46–52. doi: 10.1016/j.jcf.2014.08.002
Qvist, T., Pressler, T., Høiby, N., and Katzenstein, T. L. (2014). Shifting paradigms of nontuberculous mycobacteria in cystic fibrosis. Respir. Res. 15, 1–6. doi: 10.1186/1465-9921-15-41
Rang, C., Alix, E., Felix, C., Heitz, A., Tasse, L., and Blanc-Potard, A. B. (2007). Dual role of the MgtC virulence factor in host and non-host environments. Mol. Microbiol. 63, 605–622. doi: 10.1111/j.1365-2958.2006.05542.x
Rappuoli, R. (2000). Reverse vaccinology. Curr. Opin. Microbiol. 3, 445–450. doi: 10.1016/s1369-5274(00)00119-3
Ratnatunga, C. N., Lutzky, V. P., Kupz, A., Doolan, D. L., Reid, D. W., Field, M., et al. (2020). The rise of non-tuberculosis mycobacterial lung disease. Front. Immunol. 11:303. doi: 10.3389/fimmu.2020.00303
Raynaud, C., Guilhot, C., Rauzier, J., Bordat, Y., Pelicic, V., Manganelli, R., et al. (2002). Phospholipases C are involved in the virulence of mycobacterium tuberculosis. Mol. Microbiol. 45, 203–217. doi: 10.1046/j.1365-2958.2002.03009.x
Rhoades, E. R., Archambault, A. S., Greendyke, R., Hsu, F.-F., Streeter, C., and Byrd, T. F. (2009). Mycobacterium abscessus glycopeptidolipids mask underlying cell wall phosphatidyl-myo-inositol mannosides blocking induction of human macrophage TNF-α by preventing interaction with TLR2. J. Immunol. 183, 1997–2007. doi: 10.4049/jimmunol.0802181
Richard, M., Gutiérrez, A. V., Viljoen, A., Rodriguez-Rincon, D., Roquet-Baneres, F., Blaise, M., et al. (2019). Mutations in the MAB_2299c TetR regulator confer cross-resistance to clofazimine and bedaquiline in mycobacterium abscessus. Antimicrob. Agents Chemother. 63:e01316-18. doi: 10.1128/AAC.01316-18
Richard-Fiardo, P., Hervouet, C., Marsault, R., Franken, P. R., Cambien, B., Guglielmi, J., et al. (2015). Evaluation of tetrafunctional block copolymers as synthetic vectors for lung gene transfer. Biomaterials 45, 10–17. doi: 10.1016/j.biomaterials.2014.12.051
Ripoll, F., Pasek, S., Schenowitz, C., Dossat, C., Barbe, V., Rottman, M., et al. (2009). Non mycobacterial virulence genes in the genome of the emerging pathogen mycobacterium abscessus. PLoS One 4:e5660. doi: 10.1371/journal.pone.0005660
Rodriguez-Rojas, A., Mena, A., Martin, S., Borrell, N., Oliver, A., and Blazquez, J. (2009). Inactivation of the hmgA gene of Pseudomonas aeruginosa leads to pyomelanin hyperproduction, stress resistance and increased persistence in chronic lung infection. Microbiology 155, 1050–1057. doi: 10.1099/mic.0.024745-0
Rogerson, B. J., Jung, Y. J., LaCourse, R., Ryan, L., Enright, N., and North, R. J. (2006). Expression levels of mycobacterium tuberculosis antigen-encoding genes versus production levels of antigen-specific T cells during stationary level lung infection in mice. Immunology 118, 195–201. doi: 10.1111/j.1365-2567.2006.02355.x
Roghanian, A., Drost, E. M., MacNee, W., Howie, S. E., and Sallenave, J.-M. (2006). Inflammatory lung secretions inhibit dendritic cell maturation and function via neutrophil elastase. Am. J. Respir. Crit. Care Med. 174, 1189–1198. doi: 10.1164/rccm.200605-632OC
Rottman, M., Catherinot, E., Hochedez, P., Emile, J.-F., Casanova, J.-L., Gaillard, J.-L., et al. (2007). Importance of T cells, gamma interferon, and tumor necrosis factor in immune control of the rapid grower mycobacterium abscessus in C57BL/6 mice. Infect. Immun. 75, 5898–5907. doi: 10.1128/IAI.00014-07
Roux, A.-L., Viljoen, A., Bah, A., Simeone, R., Bernut, A., Laencina, L., et al. (2016). The distinct fate of smooth and rough mycobacterium abscessus variants inside macrophages. Open Biol. 6:160185. doi: 10.1098/rsob.160185
Ryan, K., and Byrd, T. F. (2018). Mycobacterium abscessus: shapeshifter of the mycobacterial world. Front. Microbiol. 9:2642. doi: 10.3389/fmicb.2018.02642
Sainz-Mejías, M., Jurado-Martín, I., and McClean, S. (2020). Understanding Pseudomonas aeruginosa–host interactions: the ongoing quest for an efficacious vaccine. Cell 9:2617. doi: 10.3390/cells9122617
Scholte, B. J., Davidson, D. J., Wilke, M., and De Jonge, H. R. (2004). Animal models of cystic fibrosis. J. Cyst. Fibros. 3, 183–190. doi: 10.1016/j.jcf.2004.05.039
Scriba, T. J., Tameris, M., Mansoor, N., Smit, E., van der Merwe, L., Isaacs, F., et al. (2010). Modified vaccinia Ankara-expressing Ag85A, a novel tuberculosis vaccine, is safe in adolescents and children, and induces polyfunctional CD4+ T cells. Eur. J. Immunol. 40, 279–290. doi: 10.1002/eji.200939754
Sefidi-Heris, Y., Jahangiri, A., Mokhtarzadeh, A., Shahbazi, M.-A., Khalili, S., Baradaran, B., et al. (2020). Recent progress in the design of DNA vaccines against tuberculosis. Drug Discov. Today 25, 1971–1987. doi: 10.1016/j.drudis.2020.09.005
Shi, L., North, R., and Gennaro, M. L. (2004). Effect of growth state on transcription levels of genes encoding major secreted antigens of mycobacterium tuberculosis in the mouse lung. Infect. Immun. 72, 2420–2424. doi: 10.1128/IAI.72.4.2420-2424.2004
Siegrist, M. S., Unnikrishnan, M., McConnell, M. J., Borowsky, M., Cheng, T.-Y., Siddiqi, N., et al. (2009). Mycobacterial Esx-3 is required for mycobactin-mediated iron acquisition. Proc. Natl. Acad. Sci. 106, 18792–18797. doi: 10.1073/pnas.0900589106
Smith, G. A., Marquis, H., Jones, S., Johnston, N. C., Portnoy, D. A., and Goldfine, H. (1995). The two distinct phospholipases C of listeria monocytogenes have overlapping roles in escape from a vacuole and cell-to-cell spread. Infect. Immun. 63, 4231–4237. doi: 10.1128/iai.63.11.4231-4237.1995
Steindor, M., Nkwouano, V., Stefanski, A., Stuehler, K., Ioerger, T. R., Bogumil, D., et al. (2019). A proteomics approach for the identification of species-specific immunogenic proteins in the mycobacterium abscessus complex. Microbes Infect. 21, 154–162. doi: 10.1016/j.micinf.2018.10.006
Stephan, J., Bender, J., Wolschendorf, F., Hoffmann, C., Roth, E., Mailänder, C., et al. (2005). The growth rate of mycobacterium smegmatis depends on sufficient porin-mediated influx of nutrients. Mol. Microbiol. 58, 714–730. doi: 10.1111/j.1365-2958.2005.04878.x
Stewart, E., Triccas, J. A., and Petrovsky, N. (2019). Adjuvant strategies for more effective tuberculosis vaccine immunity. Microorganisms 7:255. doi: 10.3390/microorganisms7080255
Sturgill-Koszycki, S., Schlesinger, P. H., Chakraborty, P., Haddix, P. L., Collins, H. L., Fok, A. K., et al. (1994). Lack of acidification in mycobacterium phagosomes produced by exclusion of the vesicular proton-ATPase. Science 263, 678–681. doi: 10.1126/science.8303277
Tam, P.-Y. I., Kline, S., Wagner, J. E., Guspiel, A., Streifel, A., Ward, G., et al. (2014). Rapidly growing mycobacteria among pediatric hematopoietic cell transplant patients traced to the hospital water supply. Pediatr. Infect. Dis. J. 33, 1043–1046. doi: 10.1097/INF.0000000000000391
Tameris, M. D., Hatherill, M., Landry, B. S., Scriba, T. J., Snowden, M. A., Lockhart, S., et al. (2013). Safety and efficacy of MVA85A, a new tuberculosis vaccine, in infants previously vaccinated with BCG: a randomised, placebo-controlled phase 2b trial. Lancet 381, 1021–1028. doi: 10.1016/S0140-6736(13)60177-4
Tan, L. T.-H., Raghunath, P., Ming, L. C., and Law, J. W.-F. (2020). Mycobacterium ulcerans and Mycobacterium marinum: pathogenesis, diagnosis and treatment. Prog. Microbes Mol. Biol. 3:1. doi: 10.36877/pmmb.a0000114
Thomas, V., and McDonnell, G. (2007). Relationship between mycobacteria and amoebae: ecological and epidemiological concerns. Lett. Appl. Microbiol. 45, 349–357. doi: 10.1111/j.1472-765X.2007.02206.x
Thomson, R., Tolson, C., Sidjabat, H., Huygens, F., and Hargreaves, M. (2013). Mycobacterium abscessus isolated from municipal water-a potential source of human infection. BMC Infect. Dis. 13, 1–7. doi: 10.1186/1471-2334-13-241
Tiringer, K., Treis, A., Fucik, P., Gona, M., Gruber, S., Renner, S., et al. (2013). A Th17-and Th2-skewed cytokine profile in cystic fibrosis lungs represents a potential risk factor for Pseudomonas aeruginosa infection. Am. J. Respir. Crit. Care Med. 187, 621–629. doi: 10.1164/rccm.201206-1150OC
Tortoli, E., Fedrizzi, T., Meehan, C. J., Trovato, A., Grottola, A., Giacobazzi, E., et al. (2017). The new phylogeny of the genus mycobacterium: the old and the news. Infect. Genet. Evol. 56, 19–25. doi: 10.1016/j.meegid.2017.10.013
Trnka, L., Daňková, D., and Švandová, E. (1994). Six years’ experience with the discontinuation of BCG vaccination: 4. Protective effect of BCG vaccination against the Mycobacterium avium intracellulare complex. Tuber. Lung Dis. 75, 348–352. doi: 10.1016/0962-8479(94)90080-9
Tsai, S. H., Shen, G. H., Lin, C. H., Liau, J. R., Lai, H. C., and Hu, S. T. (2013). Mab_3168c, a putative acetyltransferase, enhances adherence, intracellular survival and antimicrobial resistance of mycobacterium abscessus. PLoS One 8:e67563. doi: 10.1371/journal.pone.0067563
Tufariello, J. M., Chapman, J. R., Kerantzas, C. A., Wong, K.-W., Vilchèze, C., Jones, C. M., et al. (2016). Separable roles for mycobacterium tuberculosis ESX-3 effectors in iron acquisition and virulence. Proc. Natl. Acad. Sci. 113, E348–E357. doi: 10.1073/pnas.1523321113
van der Wel, N., Hava, D., Houben, D., Fluitsma, D., van Zon, M., Pierson, J., et al. (2007). M. Tuberculosis and M. leprae translocate from the phagolysosome to the cytosol in myeloid cells. Cell 129, 1287–1298. doi: 10.1016/j.cell.2007.05.059
Verver, S., Warren, R. M., Beyers, N., Richardson, M., Van Der Spuy, G. D., Borgdorff, M. W., et al. (2005). Rate of reinfection tuberculosis after successful treatment is higher than rate of new tuberculosis. Am. J. Respir. Crit. Care Med. 171, 1430–1435. doi: 10.1164/rccm.200409-1200OC
Viljoen, A., Dubois, V., Girard-Misguich, F., Blaise, M., Herrmann, J. L., and Kremer, L. (2017). The diverse family of M mp L transporters in mycobacteria: from regulation to antimicrobial developments. Mol. Microbiol. 104, 889–904. doi: 10.1111/mmi.13675
Viljoen, A., Viela, F., Kremer, L., and Dufrêne, Y. F. (2020). Fast chemical force microscopy demonstrates that glycopeptidolipids define nanodomains of varying hydrophobicity on mycobacteria. Nanoscale Horiz. 5, 944–953. doi: 10.1039/C9NH00736A
Weerdenburg, E. M., Abdallah, A. M., Mitra, S., De Punder, K., Van Der Wel, N. N., Bird, S., et al. (2012). ESX-5-deficient Mycobacterium marinum is hypervirulent in adult zebrafish. Cell. Microbiol. 14, 728–739. doi: 10.1111/j.1462-5822.2012.01755.x
Whang, J., Back, Y. W., Lee, K. I., Fujiwara, N., Paik, S., Choi, C. H., et al. (2017). Mycobacterium abscessus glycopeptidolipids inhibit macrophage apoptosis and bacterial spreading by targeting mitochondrial cyclophilin D. Cell Death Dis. 8:e3012. doi: 10.1038/cddis.2017.420
Wiersma, C. J., Belardinelli, J. M., Avanzi, C., Angala, S. K., Everall, I., Angala, B., et al. (2020). Cell surface remodeling of mycobacterium abscessus under cystic fibrosis airway growth conditions. ACS Infect. Dis. 6, 2143–2154. doi: 10.1021/acsinfecdis.0c00214
Winstanley, C., O’Brien, S., and Brockhurst, M. A. (2016). Pseudomonas aeruginosa evolutionary adaptation and diversification in cystic fibrosis chronic lung infections. Trends Microbiol. 24, 327–337. doi: 10.1016/j.tim.2016.01.008
World Health Organization (2020). Global tuberculosis report 2020: executive summary. Available at: https://www.who.int/teams/global-tuberculosis-programme/tb-reports/global-tuberculosis-report-2021 (Accessed December 12, 2021).
Wu, H.-J., Wang, A. H., and Jennings, M. P. (2008). Discovery of virulence factors of pathogenic bacteria. Curr. Opin. Chem. Biol. 12, 93–101. doi: 10.1016/j.cbpa.2008.01.023
Yonker, L. M., Cigana, C., Hurley, B. P., and Bragonzi, A. (2015). Host-pathogen interplay in the respiratory environment of cystic fibrosis. J. Cyst. Fibros. 14, 431–439. doi: 10.1016/j.jcf.2015.02.008
Zhang, C., Asif, H., Holt, G. E., Griswold, A. J., Campos, M., Bejarano, P., et al. (2019). Mycobacterium abscessus—bronchial epithelial cells cross-talk through type I interferon signaling. Front. Immunol. 10:2888. doi: 10.3389/fimmu.2019.02888
Zhou, Y., Song, K., Painter, R. G., Aiken, M., Reiser, J., Stanton, B. A., et al. (2013). Cystic fibrosis transmembrane conductance regulator recruitment to phagosomes in neutrophils. J. Innate Immun. 5, 219–230. doi: 10.1159/000346568
Keywords: Mycobacterium abscessus, cystic fibrosis, vaccines, virulence factors, reverse vaccinology
Citation: Ferrell KC, Johansen MD, Triccas JA and Counoupas C (2022) Virulence Mechanisms of Mycobacterium abscessus: Current Knowledge and Implications for Vaccine Design. Front. Microbiol. 13:842017. doi: 10.3389/fmicb.2022.842017
Edited by:
Axel Cloeckaert, Institut National de Recherche pour l’Agriculture, l’Alimentation et l’Environnement (INRAE), FranceReviewed by:
Audrey Bernut, Université de Versailles Saint-Quentin-en-Yvelines, FranceKenneth C. Malcolm, National Jewish Health, United States
Thomas F. Byrd, University of New Mexico, United States
Copyright © 2022 Ferrell, Johansen, Triccas and Counoupas. This is an open-access article distributed under the terms of the Creative Commons Attribution License (CC BY). The use, distribution or reproduction in other forums is permitted, provided the original author(s) and the copyright owner(s) are credited and that the original publication in this journal is cited, in accordance with accepted academic practice. No use, distribution or reproduction is permitted which does not comply with these terms.
*Correspondence: Kia C. Ferrell, kia.ferrell@sydney.edu.au; Claudio Counoupas, claudio.counoupas@sydney.edu.au