- 1Alliance for Research on the Mediterranean and North Africa, University of Tsukuba, Tsukuba, Japan
- 2National Institute of Advanced Industrial Science and Technology, Tsukuba, Japan
- 3School of Integrative and Global Majors, University of Tsukuba, Tsukuba, Japan
- 4Department of Gastrointestinal and Hepato-Biliary-Pancreatic Surgery, Faculty of Medicine, University of Tsukuba, Tsukuba, Japan
- 5Faculty of Life and Environmental Sciences, University of Tsukuba, Tsukuba, Japan
In the present study, we conducted microarray gene expression profiling to explore the direction of differentiation of human amnion epithelial cells (hAECs) treated with rosmarinic acid (RA). hAECs have several clinical advantages over other types of stem cells, including availability, low immunogenicity, low rejection rate, non-tumorigenicity, and less ethical constraint. On the other hand, RA is a phenolic compound having several health benefits, including neuroprotective and antidepressant-like activities. In this study, hAECs were isolated from discarded term placenta and were treated with 20 μM RA for 7 days. Microarray gene expression profiling was conducted for three biological replicates of RA-treated and untreated control cells on day 0 and day 7. Gene set enrichment analysis, and gene annotation and pathway analysis were conducted using online data mining tools GSEA and DAVID. Gene expression profiling showed that RA treatment biased hAECs toward ectodermal lineage progression, regulated transcription factors involved in neuronal differentiation, regulated neural specific epigenetic modifiers and several extracellular signaling pathways of neural induction, and significantly inhibited Notch signaling pathway. Gene expression profiling of RA-treated hAECs reveals for the first time a potential role of RA in neural induction and neuronal differentiation of hAECs. Having a naturally occurring compound as differentiation inducer as well as a readily available source of stem cells would have great advantages for the cell-based therapies. Findings from our genome-wide analysis could provide a foundation for further in-depth investigation.
Introduction
Human amnion epithelial cell is gaining interest as a novel alternative source of stem cells in the field of regenerative cellular therapy. hAECs express surface markers of both ESCs and MSCs and have similar functional properties of both of the stem cells. Additionally, hAECs have several advantages over other types of stem cells. Because of their embryonic origin, hAECs retain their pluripotent potential and can be differentiated into all three germ layers under proper condition. However, unlike other stem cells, they do not form teratomas in vivo. Most importantly, hAECs have low immunogenicity and a wide range of immunomodulatory properties. These cells can be obtained from discarded term placenta and are, therefore, readily available and devoid of any ethical constraint. These unique properties of hAECS offer significant practical advantages for potential clinical applications (Miki et al., 2005; Miki and Strom, 2006; De Coppi et al., 2007). In several studies, hAECS were induced to differentiate into specific cell types, including hepatocytes, adipocytes, myocytes, cardiomyocytes, osteocytes, pancreatic cells, as well as neural and glial cells. However, it was reported that hAECs are primarily committed to ectodermal lineage, and can express several neural markers even before the induction of differentiation (Pan et al., 2006). Treatment with retinoic acid and basic fibroblast growth factor was also reported to induce neural markers expression in hAECs (Niknejad et al., 2010). Sakuragawa et al. (1996) reported that under certain conditions, differentiated hAESCs can synthesize and release acetylcholine, catecholamines, dopamine, neurotrophic factors, and noggin. Use of hAECs in cellular therapies of spinal cord injuries has been reported promising because of their ability to induce neuronal differentiation and neurotransmitter secretion. Thus, hAECs have new therapeutic potential for several neurodegenerative disorders that affect the central nervous system (McDonald et al., 2011; Miki, 2011).
Currently, different synthetic and semi-biological cytokines, recombinant growth factors, and proteins are used for stem cell proliferation and differentiation. Most of these substances have several drawbacks. They may show toxic effects, have a possible risk of rejection, and can cause malignancy (Marion and Mao, 2006; Raghavan et al., 2015). These reagents are rapidly degradable and are required to replace continuously, making the whole procedure highly expensive, hence limiting their use in therapeutic tissue engineering. Therefore, a new research stream has been evolving to use natural medicinal plant extracts as stimulants of stem cells because of their high availability, low toxicity, and minimum side effects (Raghavan et al., 2015). Plant-derived extracts are rich in polyphenols, and flavonoids, and have proven health beneficial effects. A few studies have reported the differentiation potential of some herbal extracts on different types of stem cells (Udalamaththa et al., 2016). Additionally, several plant extracts and their active components showed potential effects in neuronal differentiation and proliferation, like phytochemicals from Butea superba in stem cells (Arai et al., 2013), flavonoid component from Scutellaria baicalensis in C17.2 cells (Li et al., 2012), acetic acid extract of Mucuna gigantea in bone marrow-derived hMSCs (Kongros et al., 2012), Salvia miltiorrhiza extract on Wharton jelly derived hMSCs (Ma et al., 2005). However, to our knowledge, there is no study to date reporting the effect of any natural compounds on hAECs differentiation.
As a part of our continued research efforts to explore beneficial effects of naturally occurring compounds of plant origin from the Mediterranean region, we have been investigating the effects of different natural compounds on the direction of hAECs differentiation. Rosmarinic acid is a phenolic compound and an ester of caffeic acid. It is commonly found in several culinary herb species, like Rosmarinus officinalis (rosemary), Ocimum basilicum (basil), Ocimum tenuiflorum (holy basil), and Melissa officinalis (lemon balm). It has several health benefits, like anti-inflammatory, antioxidative, and neuroprotective effects (Alagawany et al., 2017). We have previously reported that ethanolic extract of R. officinalis, as well as RA itself, has neuroprotective and anti-depressant like activities both in vitro and in vivo (Sasaki et al., 2013; Kondo et al., 2015). We also reported neuronal cell differentiation induction effects of essential oil of R. officinalis (Villareal et al., 2017).
Microarray gene expression profiling is a useful tool to explore genome-wide expression patterns that are activated during studied biological conditions and provides a foundation for further examination of molecular mechanism and regulatory pathways. In this study, we treated three dimensional (3D) spheroids of hAEC with 20 μM of RA for 7 days and conducted microarray analysis to explore its effects on the direction of hAECs differentiation.
Materials and Methods
Extraction of Amnion Epithelial Cells
Amnion epithelial cells were isolated from delivered term placenta. The amnion was aseptically separated from the chorion and was washed with 200 mL of Hank’s Basic Salt Solution – Calcium and Magnesium Free (CMF-HBSS) to remove blot clots on the membrane. The amnion was then cut into smaller pieces using surgical scissors and placed into 50 mL conical tubes. A pre-digestion buffer (CMF-HBSS, 0.5 mM EGTA, 20 mL) was added to the tubes. The amnion was gently rocked in the solution for 30 s and was transferred to new 50 mL conical tubes. Twenty mL of pre-digestion buffer was added to the amnion tissue and was incubated at 37°C for 10 min. The pre-digestion buffer was discarded and 20 mL of 0.05% trypsin-EDTA was added to the tissue. The tubes were incubated for 40 min at 37°C and then placed on ice after incubation. Two volumes of Dulbecco’s Modified Eagle Medium (DMEM, 10% FBS, 1% Penn-Strep) was added to the trypsin digest and centrifuged at 200 g for 10 min at 4°C. The supernatant was discarded and the pellet was resuspended in 10 mL of DMEM. Small tissue aggregates were removed by filtration through a 100 μm filter. The cell suspension was collected and the membrane was discarded.
Cell Culture Maintenance
Amnion epithelial cells were maintained in Placenta Epithelial Cell Basal Medium (PromoCell, Cat. #C-26140). Cells were constantly monitored with media change every 2–4 days. To subculture AECs, the plates were first washed twice with 10 mL of PBS. The PBS was discarded and 3 mL of pre-digestion buffer (pre-warmed to 37°C) was added to the plate. The plate was incubated at 37°C for 5 min. Five mL of 0.05% (w/v) trypsin-EDTA (pre-warmed to 37°C) was then added to the plate and incubated at 37°C for 10 min. Five mL of DMEM was added to stop the reaction. The cell suspension was placed into conical tubes and centrifuged at 200 RPM for 4 min at 4°C. The supernatant was discarded and the pellet was resuspended in DMEM. The cell suspension was again centrifuged at 200 RPM for 4 min at 4°C. The supernatant was discarded and the cells were resuspended in the placental cell medium. The cells were then seeded onto new cell culture dishes.
Preparation of 3D Culture Plates
Lipidure TM (NOF Corporation, Cat. #CMS206; 400 μL) solution (50 mg in 10 mL absolute ethanol) was placed into each well of the 3D culture plate (ElplasiaTM, Kuraray Co., Ltd., Cat #RB 500 400 NA 24) and was allowed to sit for 2 min. The Lipidure TM solution was aspirated out and the plate was dried for 3 h. After drying, 400 μL of PBS was placed in each well and the plate was centrifuged at 2000 g for 15 min at room temperature. The plate was observed under the microscope to ensure that there were no bubbles in the wells. The PBS was then discarded and the wells were washed twice with 400 μL of PBS. The plates were then stored in the cell culture incubator until use.
Spheroid Formation and Compound Supplement
From each well of the 3D culture plate, 300 μL of PBS was aspirated. Spheroids were formed by seeding 1 × 106 AECs in Placenta Basal Epithelial Cell Medium into each well of the 24-well plate. After the initial 24 h culture, the medium was changed with 20 μM of RA (FUJIFILM Wako Pure Chemical Corporation, Japan) every 48 h for three times for the treatment samples. Day 0 control (D0) samples were collected before adding the treatment to the cells. Control samples were maintained in the Placental medium that was also changed every 48 h. Finally, we collected the treatment (T7) and control (D7) samples from 1-week culture.
RNA Extraction and Quantification
For RNA extraction, Isogen (Nippon Gene Co., Ltd., Toyama, Japan) was added and the cell suspensions were centrifuged for 5 min at 500 g and the pellet was stored at −80°C until use. The integrity of RNA was quantified using NanoDrop 2000 spectrophotometer (Thermo Fisher Scientific, Wilmington, DE, United States).
Microarray Expression and Data Analysis
We prepared RNA samples for gene expression profiling analysis with GeneChip® 3′ Expression Arrays using 3′ IVT PLUS Reagent Kit (Affymetrix Inc., Santa Clara, CA, United States). Two hundred and fifty ng of total RNA from each sample was used to generate amplified and biotinylated cRNA from poly (A) RNA in a total RNA sample according to the user manual. IVT Incubation time was 16 h. GeneAtlas® Hybridization, Wash and Stain Kit was used for hybridizing 3′ IVT Array Strips according to the user manual (P/N 08-0306). Human genome array strips (HG-U219) were hybridized for 16 h in a 45°C incubator, washed and stained and finally imaging was done with the GeneAtlas Fluidics and Imaging Station. HG 219 array strip can determine the expression profile of 49386 genes. Microarray expression profiling was conducted for two biological replicates between T7 and D0 samples and three biological replicates between T7 and D7 samples.
The raw data were normalized using Expression Console Software provided by the Affymetrix following robust multichip average (RMA) algorithm1. Subsequent analysis of the gene expression data was carried out in the freely available Transcriptome Analysis Console (TAC) version 4 (Thermo Fisher Scientific Inc.). Genes with a fold change > 1.1 (in linear space) and p-Value < 0.05 (one-way between-subject) were considered as DEGs. Further analysis was conducted using an online data mining tool DAVID (Database for Annotation, Visualization, and Integrated Discovery, version 6.8). We used “Functional Annotation” tool of DAVID to identify the most relevant biological terms, including GO terms, biological pathways, tissue expression, and disease associations (Huang da et al., 2009). We also performed gene set enrichment analysis (GSEA) for the top 3000 DEGs between T7 and D0 hAECs and for all DEGs between T7 and D7 hAECs to test whether a priori-defined groups of genes associated with neural differentiation were significantly related to the DEGs between RA-treated and control cells on day 0 and day 72 (Subramanian et al., 2005).
Ethics Approval
The protocol was reviewed and approved by the Ethical Review Committee of the University of Tsukuba. Informed written consent was obtained from the mothers who donated the placenta after delivery.
Results
We treated 3D hAECs with 20 μM of RA for 7 days and compared the gene expression pattern between untreated control cells on day 0 and day 7 and RA-treated cells. We identified DEGs, respectively, in two (T7 vs. D0) and three (T7 vs. D7) biological replicates and explored the biological events that took place during seven days of RA treatment.
Characteristics of AECs and DEGs
Several stem cell markers were expressed highly in the undifferentiated hAECs, like SSEA-4 (99.2%), Tra-1-60 (71.1%), Tra-1-81 (13.7%), EPCAM (75.4%), E-cadherin (99.9%), and Lectin (98.1%) (Supplementary Figure 1). Additionally, AEC spheroids (3D culture) highly expressed the stemness-related genes compared to the 2D counterpart. On the 7th day of culture, 3D spheroids expressed OCT-4, NANOG, LIN28, SOX-2, C-MYC, and KLF4 significantly higher than 2D cells (Supplementary Figure 2).
Although a huge number of genes were differentially expressed between treatment and control cells on day 0 (T7 vs. D0), there was not much change in gene expression between treatment and control cells on day 7 (T7 vs. D7), as we did not add any additional supplements to induce differentiation (Supplementary Figure 3). To understand the integrative response of hAECs, including how they actively adjust transcriptome after RA treatment through GO annotation groups, we assigned the cutoff for DEGs to be >1.1 (fold change); p-Value < 0.05. We found 23 976 genes between T7 and D0 samples and 1740 genes between T7 and D7 samples were consistently differentially expressed. After deleting duplicates and unnamed genes, we identified 13 396 DEGs between T7 and D0 samples, among which 11 930 genes were upregulated and 1466 were downregulated. On the other hand, there were 1572 DEGs between T7 and D7 samples, among which 176 genes were upregulated and 1396 genes were downregulated. Volcano plots show the distribution of fold change and significance levels of the DEGs (Figures 1A,B). We found that nearly 60% of DEGs were brain-specific (Figures 1C,D). We assumed RA treatment could induce, at least in part, neuronal differentiation.
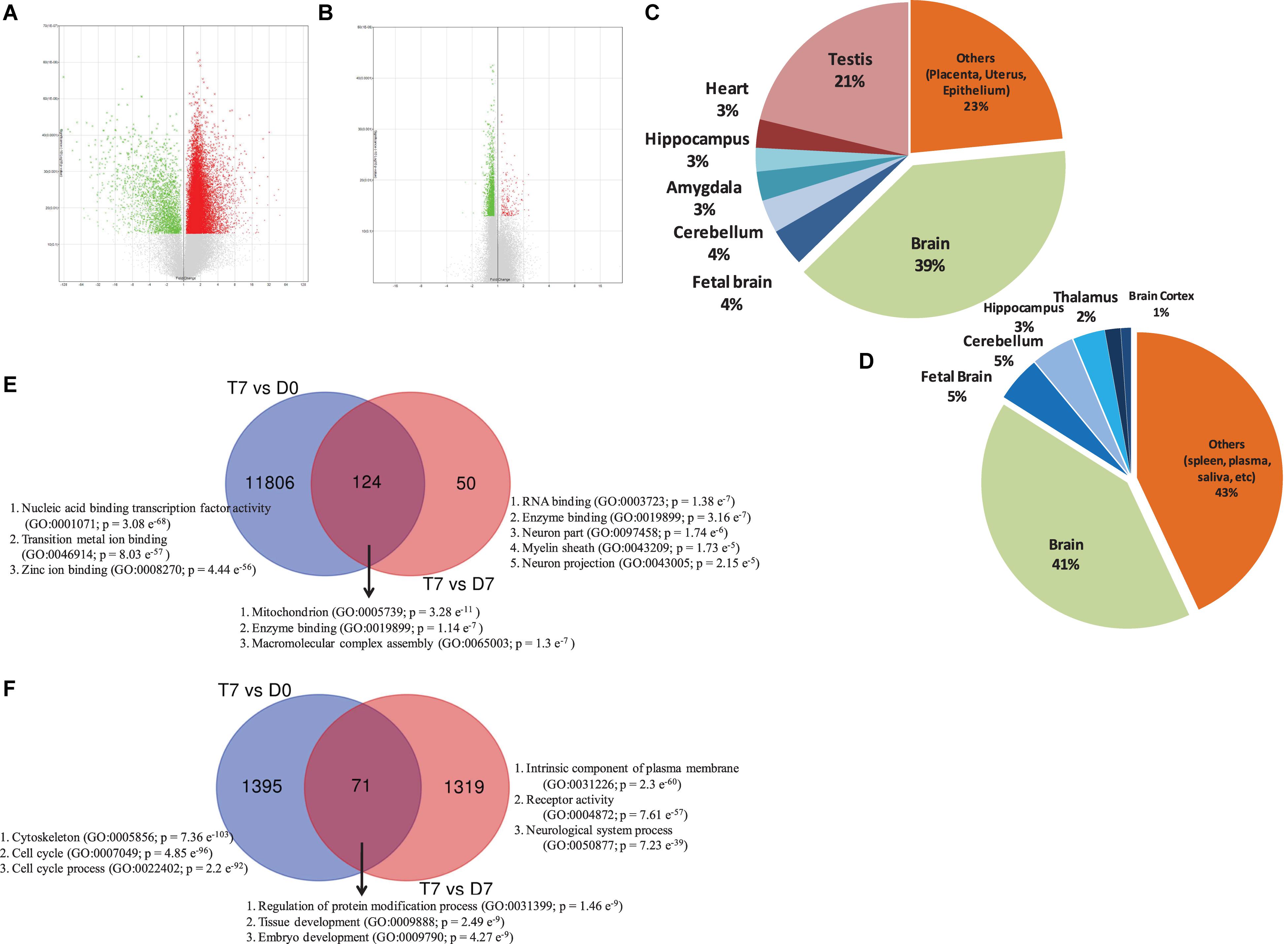
Figure 1. Volcano plot displaying DEGs between (A) RA-treated (T7) and day 0 untreated-control (D0) hAECs, and (B) RA-treated (T7) and day 7 untreated-control (D7) hAECs. The vertical axis (y-axis) corresponds to -log10 p-Value of the ANOVA p-Values, and the horizontal axis (x-axis) displays linear fold change. The red dots represent the up-regulated genes; the green dots represent the downregulated genes. Pie chart showing the enriched (p < 0.05) tissue expressions by the DEGs between (C) RA-treated (T7) and day 0 untreated-control (D0) hAECs, and (D) RA-treated (T7) and day 7 untreated-control (D7) hAECs. Venn diagram showing common and unique sets of DEGs between each exposure. Blue circles denote DEGs between RA-treated (T7) and day 0 untreated-control (D0), red circles denote DEGs between RA-treated (T7) and day 7 untreated-control (D7). (E) Venn diagram for upregulated DEGs. (F) Venn diagram for downregulated DEGs. Significantly enriched gene ontology (GO) have been listed for each set of DEGs.
Significantly Enriched Gene Sets and GO
Gene set enrichment analysis showed that several priori-defined hallmark gene sets associated with cell cycle, such as G2/M checkpoint and E2F targets, along with GO of cell differentiation (GO: 0045595) and neurogenesis (GO: 0022008) were significantly enriched by the DEGs between T7 and D0 cells (Table 1). When compared between T7 and D7 cells, significantly enriched GO were cell differentiation (GO: 0045595), neurogenesis (GO: 0022008), and neuron differentiation (GO: 0030182). Top gene sets by the unique upregulated DEGs between T7 and D0 were nucleic acid binding (GO: 0001071), metal ion binding (GO: 0046914), and Zinc ion binding (GO: 0008270); while top significantly enriched gene sets by the unique upregulated DEGs between T7 and D7 were RNA binding (GO: 0003723), enzyme binding (GO: 0019899), and neuron part (GO: 0097458; Figure 1E). Figure 1F shows the top significantly enriched gene sets by the unique sets of downregulated DEGs between each exposure. Detailed functional analysis of DEGs between T7 and D0 and between T7 and D7 is presented in Figure 2 and Supplementary Figure 4, respectively. Supplementary Table 1 listed the fold changes and p-Values of important DEGs. Top ten upregulated DEGs between T7 and D0 are significantly associated with tissue development (GO: 0009888, p = 2.41 e–7) and epithelial cell differentiation (GO: 0030855, p = 2.65 e–6).
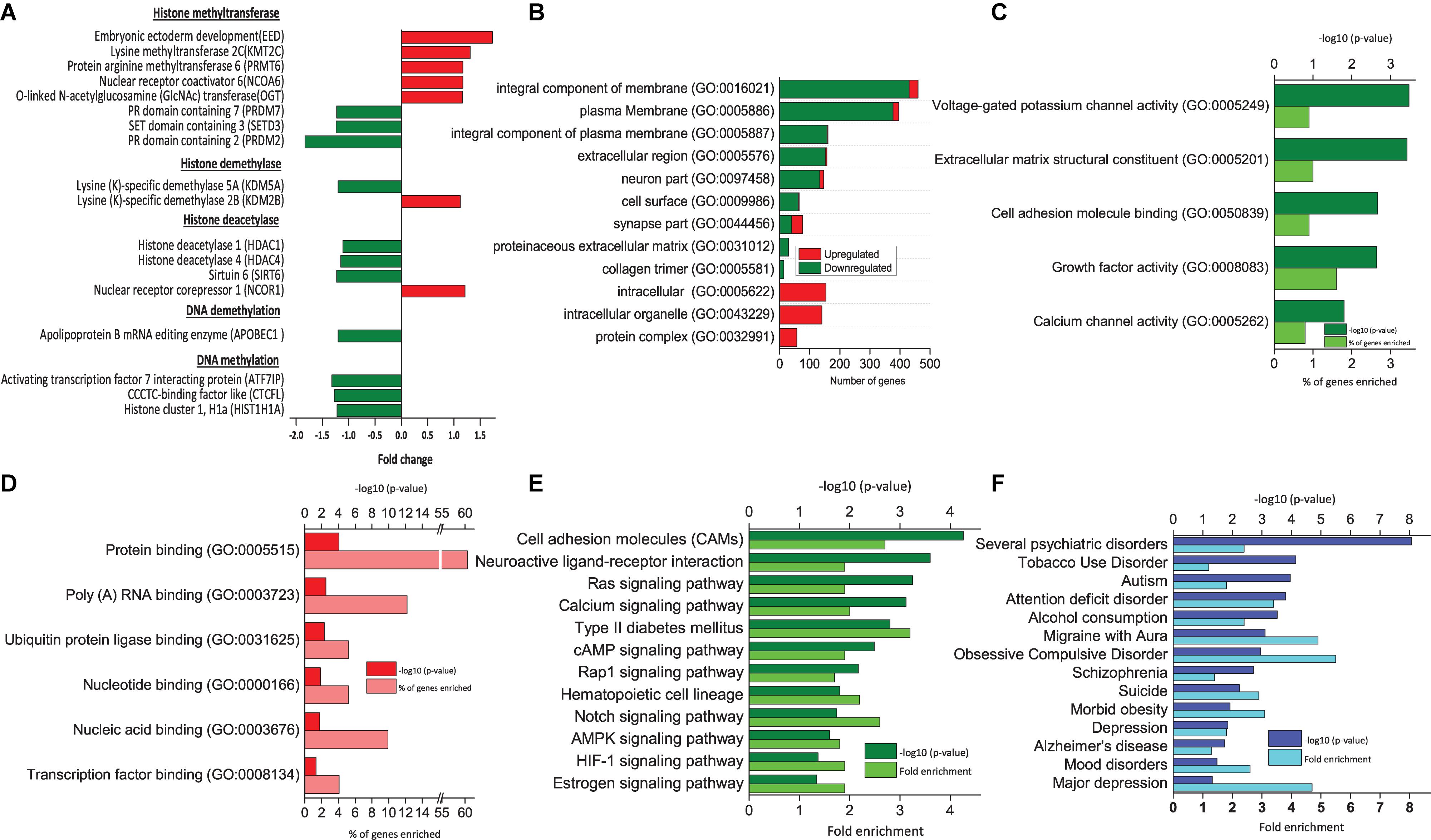
Figure 2. Functional analysis of DEGs between RA-treated (T7) and day 7 untreated control (D7) hAECs. (A) Changes in the expression of epigenetic modifiers by RA treatment (T7) compared with untreated-control on day 7 (D7); (B) Significantly enriched cellular components (p < 0.05; modified Fisher’s exact test); (C) Top molecular functions as per p-Value (modified Fisher’s exact) by downregulated genes. (D) Top molecular functions as per p-Value (modified Fisher’s exact) by upregulated genes. (E) Significantly enriched KEGG pathways by downregulated genes (p < 0.05; modified Fisher’s exact test). (F) Significantly enriched psychological and neurological diseases (p < 0.05; modified Fisher’s exact test).
RA Treatment Biased hAECs Toward Ectodermal Lineage Progression
In the RA-treated cells, there was no expression of stem cell marker genes, indicating that the cells lost their stemness and proceeded toward differentiation. Several endoderm marker genes, like GATA1, HNF4A, NKX3, and SOX17 were significantly upregulated when compared between T7 vs. D0 cells, whereas significantly downregulated when compared between T7 and D7 (Supplementary Table 1). Similarly, some mesoderm marker genes, namely MEST, MEOX1, MESDC1, DLL3, MESP1, and FOXF1 were also downregulated when compared between T7 and D7. The expression of some known ectoderm marker genes, including OTX, SOX1, and PAX6, were significantly upregulated when compared between T7 and D0 hAECs, but no change in expression was observed when compared between T7 and D7 hAECs. However, a less well-known early ectoderm development marker gene EED was expressed significantly higher in both exposures (1.64 and 1.73 fold in T7 vs. D0 and T7 vs. D7, respectively). Eed is a polycomb protein and a core protein of PRC 2. It is expressed by neural stem cells (NSCs) and is necessary for subventricular zone NSC activation, and neurogenesis (Sun et al., 2018). When compared between T7 and D7 hAECs, GSEA showed that 91 DEGs matched the set “PRC2 targets” (p-Value = 6.47 e–33), and 119 DEGs matched the set “Eed targets” (p-Value = 2.17 e–33) identified in human ESCs (Ben-Porath et al., 2008). There was upregulation of BCOR (1.28 fold), a transcriptional corepressor of BCL6 (Huynh et al., 2000). Bcl6 controls neurogenesis through epigenetic repression of selective Notch targets. There was downregulation of DPPA4 (1.32 fold), a possible inhibitor of embryonic cell differentiation into primitive ectoderm lineage.
RA Treatment Regulated TFs Involved in Neuronal Differentiation
Analysis of up- and downregulated TFs between T7 vs. D7 hAECs revealed TFs involved in neuronal differentiation were highly regulated (TCF12, HNRNPD, NLK, and SMAD5). Tcf12 (also known as HEB in human) is a member of bHLH E-proteins that are heterodimeric partners of neural-specific bHLH proteins (Uittenbogaard and Chiaramello, 2002; Mesman and Smidt, 2017). Tcf12 plays an important role both in the early cell-fate commitment of neural progenitors and in subset specification. On the other hand, HELT, a bHLH transcriptional repressor of neuronal differentiation, was downregulated (Nakatani et al., 2004). Nlk is a positive effector of the non-canonical Wnt pathway and enhances neural fate by limiting epidermal choice. Additionally, Smad5 activity is reported to be important for the maintenance of neural progenitor cells. Loss of Smad 5 along with Smad 1 could reduce the number of newly generated neurons and forced cell cycle exit and premature neurogenesis of neural progenitors. On the other hand, ASCL1 that plays a role at early stages of development of specific neural lineages probably through DLL4-NOTCH signaling activation was downregulated.
Several TFs involved in epidermal differentiation (TCF7L1), epithelial cell growth (FOXE3 and EHF), lymphocyte development (ZBTB17), lymphoid cell fate determination (MAML1), erythroid development (GATA1), liver development (HNF4A), hair follicle differentiation (HOXC13), kidney cell differentiation (PAX2), limb development (SP8), hematopoietic stem cell differentiation (EGR1) (Min et al., 2008), and cardiovascular development (HEYL), were downregulated.
RA Treatment Regulated Neural Specific Epigenetic Modifiers
Modification of neural development genes at the DNA level plays an important role in neural induction (Yao and Jin, 2014). We analyzed the effect of RA treatment on the expression pattern of genes that are involved in epigenetic regulation during neural induction (T7 vs. D7). We found that several genes of histone methyltransferases were both up- and downregulated (Figure 2A). Other than EED, a lysine-specific methyltransferase (PRMT6), and an arginine-specific methyltransferase (KMT2C) was upregulated. On the other hand, lysine-dependent three other methyltransferases, namely PRDM2 and 7 and SETD3 were downregulated. Both activating and repressing of histone methylation marks is necessary for the rapid activation of genes during differentiation. Similarly, we found lysine (K)-specific histone demethylase KDM2B was upregulated, whereas KDM5A was downregulated. Most interestingly, we found genes of HDAC complex were downregulated (HDAC1, HDAC4, and SIRT6). It was reported that transcriptional repression mediated by EED involves histone deacetylation (van der Vlag and Otte, 1999). HDACs are involved in the transition from deep layer neuron to upper layer neuron and oligodendrocyte differentiation. However, there was no change in the gene expression of DNA methyltransferases. Usually, DNA methylation takes place from mid-stage to late stage of neuronal differentiation. We assume that as we collected the sample on the 7th day of culture, it was too early to detect any DNA methylation activity directly (Namihira et al., 2008; Murao et al., 2016).
RA Treatment Regulated Neural Specific Epithelial-Mesenchymal Transition
Epithelial-Mesenchymal Transition is a common phenomenon during embryonic development, however, is less explained during neural induction. One of the significantly enriched hallmark gene sets by the DEGs between T7 and D0 is EMT (number of DEGs = 58, p = 1.01 e–24, Table 1). The previous study reported that formation of neuroectoderm from hESCs involves genes of extracellular matrix components (Wang et al., 2012). GO analysis of DEGs between T7 and D7 revealed that significantly enriched cellular components by downregulated genes are an integral component of the plasma membrane, extracellular region, cell surface, and collagen trimer. Significantly enriched cellular components by upregulated genes are intracellular organelle and protein complex (Figure 2B). A similar pattern of gene expression is observed during the early stage of neuronal differentiation. We found downregulation of genes from CADHERIN family (CDH6, CDH16, and CDHR5), COLLAGEN family (COL11A1, COL13A1, COL14A1, COL4A1, COL4A2, and COL9A1). Moreover, genes associated with tight junction (CLDN18 and EPB41L5), desmosome (KAZN), cell surface receptor (CD74, CSPG4, and DCSTAMP), cell polarity (ARHGAP24), and vesicle transport (FYCO1 and SPIRE 1) were consistently downregulated (Supplementary Table 1), indicating potential role of RA treatment in EMT in neural induction (Pankratz et al., 2007; Park et al., 2012).
RA Treatment Regulated Several Extracellular Signaling Pathways of Neural Induction
Several extracellular signaling pathways are well established in a number of proposed models of neural induction. We examined the expression profile of genes related to WNT, TGF-β, and BMP signaling pathways (Doe, 2008). When compared between T7 and D7 hAECs, we found that several genes of the canonical WNT pathway (WNT/β catenin) were downregulated, such as BTRC, CCNY, FZD5, RSPO1, RSPO4, SIX3, WISP3, and WNT5B. On the other hand, a positive effector of the non-canonical WNT (WNT/ calcium) signaling pathway NLK was upregulated. BMP/TGF-β signaling pathway related genes, like ACVRL1, ASPN, CHRD, and SLC39A5 were downregulated. BMP8A, BMP8B, BMPER, and TGF-β2 were also downregulated. On the other hand, the major intracellular BMP signaling pathway component SMAD 5 was upregulated (Leung et al., 2013; Huang et al., 2016).
RA Treatment Significantly Inhibited Notch Signaling Pathway
Notch is considered as a molecular switch in NSCs (Zhou et al., 2010; Imayoshi and Kageyama, 2011). Loss of Notch signaling in NSC leads to increased cell cycle exit and decreased progenitor pool. When compared between T7 and D0 hAECs, several genes associated with cell cycle and notch signaling were significantly upregulated (Table 1 and Supplementary Table 1). However, when compared with T7 and D7 hAECs, we found that RA treatment could significantly downregulate several genes related to the Notch pathway indicating that RA treatment could lead to cell cycle exit and decreased progenitor pool. Notch receptor (NOTCH2), transmembrane ligand of Notch receptors (DLL1 and DLL3), a positive regulator of Notch (DTX1), a downstream effector of Notch signaling (HEYL), transcriptional coactivator for NOTCH (MAML1 and SBNO2), a downstream target of Notch signaling pathway (PKMYT1) were downregulated.
Furthermore, RA might also work on hAECs through downregulating neuroactive ligand-receptor interaction pathways, Ras signaling pathway, the cAMP signaling pathway, and AMPK signaling pathway (Figure 2E). Ras signaling pathway is an upstream pathway for MAPK, Rap 1 pathway. Similarly, cAMP is upstream of the Hedgehog pathway. All of these pathways are known to be regulated at different stages of neuronal differentiation and are balancing between self-renewal and differentiation (Doe, 2008).
RA Treatment Significantly Enriched Several Neurological and Psychological Diseases
We used the functional annotation tool of DAVID for annotating genome-wide data with disease associations using the GAD (Osborne et al., 2009). Several neurological and psychological diseases were significantly enriched, particularly by the downregulated genes, such as Schizophrenia, obsessive-compulsive disorder, migraine with aura, alcohol consumption, bulimia, attention deficit disorder, autism, and tobacco use disorder (Figure 2F). Moreover, major depression, mood disorders, depression, and suicidal tendency related genes were also regulated.
Discussion
Placenta-derived hAECs is gaining interest in the field of regenerative medicine to overcome the current challenges of existing cell-based therapies, such as cell resources, invasive extraction procedures, immunological rejection, tumorigenesis and ethical challenges (Miki, 2011). Previous studies reported that cultured human amnion cells were able to express markers characteristic of NSCs (nestin, Musashi-1, and polysialylated neural adhesion molecule), neural cells (TH, AchT, NeuN, MAP-2, and myelin basic protein), astrocytes (GFAP), and oligodendrocytes under optimal differentiation condition (Pan et al., 2006). However, there is no study on the effect of natural compounds on hAECs differentiation. In the present study, we aimed to evaluate microarray gene expression profiling of RA-treated 3D spheroids of hAECs to explore its neuronal differentiation-inducing effect and to provide a foundation for further examination of molecular mechanisms. Altogether, our profiling analysis suggests a potential role of RA in neural induction and neuronal differentiation of hAECs (Figure 3).
Our findings suggest that cultured hAECs are able to express stem cell markers, while RA treatment could direct the differentiation of hAECs toward ectodermal lineage. Although the mechanisms of neuronal cell fate determination are not fully elucidated yet, it is apparent that cell-intrinsic programs, including epigenetic regulations as well as TFs and extracellular cues, are involved in this fate specification of stem cells (Schuldiner et al., 2001). Neuronal differentiation is facilitated through promoting proneural genes of neurogenesis and inhibitory mechanisms that maintain self-renewal and proliferation. Remodeling of chromatin structure through histone modification is an important mechanism of modulating gene expression (Murao et al., 2016). We found that RA treatment could regulate histone modification by simultaneously up and downregulating several histone methyltransferases and histone demethylases. Both the activation and repression of histone methylation marks are necessary for the rapid activation of genes during differentiation. Among the histone methyltransferases that were differentially expressed in the treatment hAECs, Eed is the most important. Eed is an active component of PRC2 and was highly expressed in the treated cells. The epigenetic PRC1 promotes proliferation and self-renewal of NSCs, whereas PRC2-mediated gene silencing enhances NSC activation and neurogenesis through histone methylation (van der Vlag and Otte, 1999). Eed suppresses the expression of Gata6 and thereby increases p21 protein level. Gata6 is essential for primitive gut and heart development. On the other hand, increased p21 leads to inhibition of NSC proliferation and self-renewal, and activation of neurogenesis (Sun et al., 2018). Thus, RA may regulate neuroectodermal lineage progression via epigenetic polycomb protein Eed. PRCs together with histone acetylation also affect layer formation of the cortex. Inhibition of HDACs activity decreases the production of deep layer neurons and increases upper layer neurons, and promotes oligodendrocyte production (Namihira et al., 2008). RA treatment downregulated the expressions of HDAC1 and HDAC4. Furthermore, we found upregulation of BCOR in the RA-treated hAECS. BCoR can function as a corepressor when tethered to DNA and, when overexpressed, can potentiate BCL-6 repression (Huynh et al., 2000). Specific class I and II HDACs interact with BCoR, suggesting that BCoR may functionally link these two classes of HDACs.
Neuronal differentiation is also regulated by many neural specific bHLH family transcriptional activators and repressors, and the balance of activity between these factors is important for the differentiation process. Helt is a member of bHLH-O transcriptional repressors and contains the characteristic Orange domains. Helt is mainly expressed in the neural progenitors and is down-regulated before neuronal differentiation (Nakatani et al., 2004). Tcf12 (also known as Heb) is a member of the E-box protein family, a subfamily of bHLH protein family members. It plays a critical role during embryonic and adult neurogenesis (Uittenbogaard and Chiaramello, 2002; Mesman and Smidt, 2017). Tcf12 is involved in the control of proliferating stem cells and progenitor cells and early cell fate determination depending on the timing and location of TCF12 expression. In this study, we found upregulation of TCF12 expression and downregulation of HELT on the 7th day of RA treatment of hAECs.
Epithelial-mesenchymal transition is a common phenomenon during embryonic development. However, EMT during neural induction is not well characterized yet. GO analysis of DEGs revealed highly enriched molecular functions of extracellular matrix component, collagen trimers as well as enriched cellular components of the plasma membrane, cell surface, protein binding, and proteinaceous extracellular matrix (Wang et al., 2012). Together, our GSEA and gene annotation analysis suggests a potential role of RA in EMT and promoting neural induction (Figure 2B and Table 1).
Signaling pathway analysis allows us to understand the molecular basis of biological events that take place during stem cell differentiation. We found that several extracellular pathways were regulated on the 7th day of RA treatment. RA treatment downregulated the expression of several genes related to the canonical WNT pathway, and BMP/TGF-β pathway, whereas upregulated non-canonical effector NLK. It has been reported that inhibition of the canonical WNT pathway promotes ESCs differentiation toward ectodermal fate, and activation of the non-canonical WNT pathway enhances neural fate by limiting epidermal choice (Huang et al., 2016). We also found the decreased activity of BMP/TGF-β signaling indicating neural differentiation took place (Doe, 2008). However, most SMAD genes were not changed probably because of their association with EMT during neural induction.
Furthermore, we found that RA treatment could significantly inhibit the Notch pathway. Notch signal pathway has multiple critical roles in the regulation of NSC differentiation and is considered as a molecular switch between neurogenic to gliogenic differentiation of NSCs. Previous evidence suggests that Notch inhibition could promote neuronal commitment and neurogenesis, decreased progenitor pool, and inhibit glial fate differentiation (Zhou et al., 2010; Imayoshi and Kageyama, 2011).
Currently, we are using several semi-biological and synthetic stimulants in neuronal differentiation medium, like neurobasal medium, neural cell supplement N2, non-essential amino acid, BDNF, glial cell line-derived neurotrophic factors, cAMP, ascorbic acid, and laminin. We also use inhibitors of GSK3, TGF-β, and AMPK for neural induction medium. Continuous replacement of this medium makes the whole procedure highly expensive. Therefore, RA would have potential as a cost-effective, easily available alternative stimulant of neuronal differentiation.
Additionally, previous studies showed that transplantation of hAECs into the brain of mice with Parkinson’s disease could significantly increase both the duration of rotation and the number of spontaneous movements (Kong et al., 2008). This suggests that the transplanted hAECs were able to promote endogenous neurogenesis in the SVZ and enhance preservation of the nigrostriatal system. We found that RA treatment could significantly enrich several neurological and psychological diseases, suggesting regulation of genes related to brain diseases. Therefore, RA-induced hAECs may have potential as a new therapeutic approach for several neurodegenerative and psychiatric disorders, including Alzheimer’s disease, Parkinson’s disease, spinal cord injuries, and Huntington’s disease. Furthermore, the differentiation potential of hAECs toward a specific direction using a natural compound unveils the great potential of hAECs as a novel tool for drug screening. However, further in-depth investigations on time and dose-dependent effects of RA treatment on morphology, physiology, and gene expression pattern of hAECs are warranted to confirm its neuronal differentiation potential.
Data Availability
All data generated or analyzed during this study are included in this published article and its Supplementary Files. Microarray data are deposited in the Gene Expression Omnibus (GEO) under Accession Number: GSE133277 (https://www.ncbi.nlm.nih.gov/geo/info/linking.html).
Author Contributions
FF investigated the study, responsible for data curation, carried out the formal analysis, visualized the data, and wrote the original draft of the manuscript. KS investigated the study, and carried out the methodology and data validation. YU investigated the study and carried out the formal analysis. NO and HI conceptualized the study, involved in funding acquisition, and carried out the project administration. NO, Y-WZ, and HI were responsible for the resources, supervised the study, and wrote, reviewed, and edited the manuscript. All authors made the substantial contributions to the manuscript and approved its final version.
Funding
This work was supported by the Japan Science and Technology Agency (JST), Japan International Cooperation Agency (JICA) (SATREPS), and JST-Center of Innovation (COI) project.
Conflict of Interest Statement
The authors declare that the research was conducted in the absence of any commercial or financial relationships that could be construed as a potential conflict of interest.
Supplementary Material
The Supplementary Material for this article can be found online at: https://www.frontiersin.org/articles/10.3389/fnins.2019.00779/full#supplementary-material
Abbreviations
bHLH, basic helix-loop-helix; cRNA, complementary RNA; DEGs, differentially expressed genes; EMT, epithelial-mesenchymal transition; ESCs, embryonic stem cells; GAD, genetic association database; GO, gene ontology; hAECs, human amnion epithelial cells; HDAC, histone deacetylase; iPSCs, induced PSCs; MSCs, mesenchymal stem cells; PRC, polycomb repressive complex; PSCs, pluripotent stem cells; RA, rosmarinic acid.
Footnotes
References
Alagawany, M., El-Hack, M. E. A., Farag, M. R., Gopi, M., Karthik, K., Malik, Y. S., et al. (2017). Rosmarinic acid: modes of action, medicinal values and health benefits. Anim. Health Res. Rev. 18, 167–176. doi: 10.1017/S1466252317000081
Arai, M. A., Koryudzu, K., Koyano, T., Kowithayakorn, T., and Ishibashi, M. (2013). Naturally occurring Ngn2 promoter activators from butea superba. Mol. BioSyst. 9, 2489–2497. doi: 10.1039/c3mb70083f
Ben-Porath, I., Thomson, M. W., Carey, V. J., Ge, R., Bell, G. W., Regev, A., et al. (2008). An embryonic stem cell-like gene expression signature in poorly differentiated aggressive human tumors. Nat. Genet. 40, 499–507. doi: 10.1038/ng.127
De Coppi, P., Bartsch, G., Siddiqui, M. M. jr., Xu, T., Santos, C. C., Perin, L., et al. (2007). I*solation of amniotic stem cell lines with potential for therapy. Nat. Biotechnol. 25:100.
Doe, C. Q. (2008). Neural stem cells: balancing self-renewal with differentiation. Development 135, 1575–1587. doi: 10.1242/dev.014977
Huang, C. T.-L., Tao, Y., Lu, J., Jones, J. R., Fowler, L., Weick, J. P., et al. (2016). Time-course gene expression profiling reveals a novel role of non-canonical WNT signaling during neural induction. Sci. Rep. 6:32600. doi: 10.1038/srep32600
Huang da, W., Sherman, B. T., and Lempicki, R. A. (2009). Systematic and integrative analysis of large gene lists using DAVID bioinformatics resources. Nat. Protoc. 4, 44–57. doi: 10.1038/nprot.2008.211
Huynh, K. D., Fischle, W., Verdin, E., and Bardwell, V. J. (2000). BCoR, a novel corepressor involved in BCL-6 repression. Genes Dev. 14, 1810–1823.
Imayoshi, I., and Kageyama, R. (2011). The role of notch signaling in adult neurogenesis. Mol. Neurobiol. 44, 7–12. doi: 10.1007/s12035-011-8186-0
Kondo, S., El Omri, A., Han, J., and Isoda, H. (2015). Antidepressant-like effects of rosmarinic acid through mitogen-activated protein kinase phosphatase-1 and brain-derived neurotrophic factor modulation. J. Funct. Foods 14, 758–766. doi: 10.1016/j.jff.2015.03.001
Kong, X.-Y., Cai, Z., Pan, L., Zhang, L., Shu, J., Dong, Y.-L., et al. (2008). Transplantation of human amniotic cells exerts neuroprotection in MPTP-induced parkinson disease mice. Brain Res. 1205, 108–115. doi: 10.1016/j.brainres.2008.02.040
Kongros, B., Bunyaratvej, A., Viyoch, J., and Sila-asna, M. (2012). The effects of seed extract of mucuna gigantea on the expression of neural markers in mesenchymal stem cells. J. Med. Plants Res. 6, 1297–1303.
Leung, A. W., Morest, D. K., and Li, J. Y. H. (2013). Differential BMP signaling controls formation and differentiation of multipotent preplacodal ectoderm progenitors from human embryonic stem cells. Dev. Biol. 379, 208–220. doi: 10.1016/j.ydbio.2013.04.023
Li, M., Choi, S. T., Tsang, K. S., Shaw, P. C., and Lau, K. F. (2012). DNA microarray expression analysis of baicalin-induced differentiation of c17. 2 neural stem cells. ChemBioChem 13, 1286–1290. doi: 10.1002/cbic.201200145
Ma, L., Feng, X.-Y., Cui, B.-L., Law, F., Jiang, X.-W., Yang, L.-Y., et al. (2005). Human umbilical cord wharton’s jelly-derived mesenchymal stem cells differentiation into nerve-like cells. Chin. Med. J. 118, 1987–1993.
Marion, N. W., and Mao, J. J. (2006). Mesenchymal stem cells and tissue engineering. Methods Enzymol. 420, 339–361.
McDonald, C., Siatskas, C., and Bernard, C. C. A. (2011). The emergence of amnion epithelial stem cells for the treatment of multiple sclerosis. Inflamm. Regen. 31, 256–271. doi: 10.2492/inflammregen.31.256
Mesman, S., and Smidt, M. P. (2017). Tcf12 is involved in early cell-fate determination and subset specification of midbrain dopamine neurons. Front. Mol. Neurosci. 10:353. doi: 10.3389/fnmol.2017.00353
Miki, T. (2011). Amnion-derived stem cells: in quest of clinical applications. Stem Cell Res. Ther. 2:25. doi: 10.1186/scrt66
Miki, T., Lehmann, T., Cai, H., Stolz, D. B., and Strom, S. C. (2005). Stem cell characteristics of amniotic epithelial cells. Stem Cells 23, 1549–1559. doi: 10.1634/stemcells.2004-0357
Miki, T., and Strom, S. C. (2006). Amnion-derived pluripotent/multipotent stem cells. Stem Cell Rev. 2, 133–141. doi: 10.1007/s12015-006-0020-0
Min, I. M., Pietramaggiori, G., Kim, F. S., Passegué, E., Stevenson, K. E., and Wagers, A. J. (2008). The transcription factor EGR1 controls both the proliferation and localization of hematopoietic stem cells. Cell Stem Cell 2, 380–391. doi: 10.1016/j.stem.2008.01.015
Murao, N., Noguchi, H., and Nakashima, K. (2016). Epigenetic regulation of neural stem cell property from embryo to adult. Neuroepigenetics 5, 1–10. doi: 10.1016/j.nepig.2016.01.001
Nakatani, T., Mizuhara, E., Minaki, Y., Sakamoto, Y., and Ono, Y. (2004). Helt, a novel basic-helix-loop-helix transcriptional repressor expressed in the developing central nervous system. J. Biol. Chem. 279, 16356–16367. doi: 10.1074/jbc.m311740200
Namihira, M., Kohyama, J., Abematsu, M., and Nakashima, K. (2008). Epigenetic mechanisms regulating fate specification of neural stem cells. Philos. Trans. R. Soc. Lond. B Biol. Sci. 363, 2099–2109. doi: 10.1098/rstb.2008.2262
Niknejad, H., Peirovi, H., Ahmadiani, A., Ghanavi, J., and Jorjani, M. (2010). Differentiation factors that influence neuronal markers expression in vitro from human amniotic epithelial cells. Eur. Cell Mater 19, 22–29. doi: 10.22203/ecm.v019a03
Osborne, J. D., Flatow, J., Holko, M., Lin, S. M., Kibbe, W. A., Zhu, L. J., et al. (2009). Annotating the human genome with disease ontology. BMC Genomics 10:S6. doi: 10.1186/1471-2164-10-S1-S6
Pan, L., Shu, J., and Cai, Z. (2006). The morphologic study of the characteristics of neurobiology of the amniotic membrane. Chin. J. Rehabil. Med. 21, 46–49.
Pankratz, M. T., Li, X. J., LaVaute, T. M., Lyons, E. A., Chen, X., and Zhang, S. C. (2007). Directed neural differentiation of human embryonic stem cells via an obligated primitive anterior stage. Stem Cells 25, 1511–1520. doi: 10.1634/stemcells.2006-0707
Park, J. H., Choi, M. R., Park, K. S., Kim, S. H., Jung, K. H., and Chai, Y. G. (2012). The characterization of gene expression during mouse neural stem cell differentiation in vitro. Neurosci. Lett. 506, 50–54. doi: 10.1016/j.neulet.2011.10.046
Raghavan, R. N., Vignesh, G., Kumar, B. S., Selvaraj, R., and Dare, B. J. (2015). Phytochemicals: do they hold the future in stem cell differentiation. Int. J. Res. Pharma 6, 379–381. doi: 10.7897/2277-4343.06374
Sakuragawa, N., Thangavel, R., Mizuguchi, M., Hirasawa, M., and Kamo, I. (1996). Expression of markers for both neuronal and glial cells in human amniotic epithelial cells. Neurosci. Lett. 209, 9–12. doi: 10.1016/0304-3940(96)12599-4
Sasaki, K., El Omri, A., Kondo, S., Han, J., and Isoda, H. (2013). Rosmarinus officinalis polyphenols produce anti-depressant like effect through monoaminergic and cholinergic functions modulation. Behav. Brain Res. 238, 86–94. doi: 10.1016/j.bbr.2012.10.010
Schuldiner, M., Eiges, R., Eden, A., Yanuka, O., Itskovitz-Eldor, J., Goldstein, R. S., et al. (2001). Induced neuronal differentiation of human embryonic stem cells. Brain Res. 913, 201–205.
Subramanian, A., Tamayo, P., Mootha, V. K., Mukherjee, S., Ebert, B. L., Gillette, M. A., et al. (2005). Gene set enrichment analysis: a knowledge-based approach for interpreting genome-wide expression profiles. Proc. Nat. Acad. Sci. 102:15545. doi: 10.1073/pnas.0506580102
Sun, B., Chang, E., Gerhartl, A., and Szele, F. G. (2018). Polycomb protein eed is required for neurogenesis and cortical injury activation in the subventricular zone. Cereb. Cortex 28, 1369–1382. doi: 10.1093/cercor/bhx289
Udalamaththa, V. L., Jayasinghe, C. D., and Udagama, P. V. (2016). Potential role of herbal remedies in stem cell therapy: proliferation and differentiation of human mesenchymal stromal cells. Stem Cell Res. Ther. 7:110. doi: 10.1186/s13287-016-0366-4
Uittenbogaard, M., and Chiaramello, A. (2002). Expression of the bHLH transcription factor Tcf12 (ME1) gene is linked to the expansion of precursor cell populations during neurogenesis. Gene Exp. Patterns 1, 115–121. doi: 10.1016/s1567-133x(01)00022-9
van der Vlag, J., and Otte, A. P. (1999). Transcriptional repression mediated by the human polycomb-group protein EED involves histone deacetylation. Nat. Genetics 23:474. doi: 10.1038/70602
Villareal, M. O., Ikeya, A., Sasaki, K., Arfa, A. B., Neffati, M., and Isoda, H. (2017). Anti-stress and neuronal cell differentiation induction effects of Rosmarinus officinalis L. essential oil. BMC Comp. Altern. Med. 17:549. doi: 10.1186/s12906-017-2060-1
Wang, K., Wang, H., Wang, J., Xie, Y., Chen, J., Yan, H., et al. (2012). System approaches reveal the molecular networks involved in neural stem cell differentiation. Protein Cell 3, 213–224. doi: 10.1007/s13238-012-0014-4
Yao, B., and Jin, P. (2014). Unlocking epigenetic codes in neurogenesis. Genes Dev. 28, 1253–1271. doi: 10.1101/gad.241547.114
Keywords: human amnion epithelial cell, rosmarinic acid, microarray analysis, system biology, neural induction, neuronal differentiation, natural compound
Citation: Ferdousi F, Sasaki K, Uchida Y, Ohkohchi N, Zheng Y-W and Isoda H (2019) Exploring the Potential Role of Rosmarinic Acid in Neuronal Differentiation of Human Amnion Epithelial Cells by Microarray Gene Expression Profiling. Front. Neurosci. 13:779. doi: 10.3389/fnins.2019.00779
Received: 01 April 2019; Accepted: 11 July 2019;
Published: 24 July 2019.
Edited by:
Xiaogang Wu, University of Nevada, Las Vegas, United StatesReviewed by:
Nicola Bernabò, University of Teramo, ItalyDebashis Sahoo, University of California, San Diego, United States
Copyright © 2019 Ferdousi, Sasaki, Uchida, Ohkohchi, Zheng and Isoda. This is an open-access article distributed under the terms of the Creative Commons Attribution License (CC BY). The use, distribution or reproduction in other forums is permitted, provided the original author(s) and the copyright owner(s) are credited and that the original publication in this journal is cited, in accordance with accepted academic practice. No use, distribution or reproduction is permitted which does not comply with these terms.
*Correspondence: Hiroko Isoda, aXNvZGEuaGlyb2tvLmdhQHUudHN1a3ViYS5hYy5qcA==