Anesthesia-mediated neuroinflammatory sequelae in post operative cognitive dysfunction: mechanisms and therapeutic implications
- 1Touro College of Osteopathic Medicine, New York, NY, United States
- 2Department of Primary Care, Touro College of Osteopathic Medicine, New York, NY, United States
Post-operative cognitive dysfunction (POCD) is an iatrogenic cognitive decline with unclear etiology. While current hypotheses include surgical and pharmacological-induced neuroinflammatory mechanisms, the growing prevalence, especially amongst the geriatric population, emphasizes the ambiguity of the dysfunction. Recent studies have highlighted the potential role of general and regional anesthesia in the pathogenesis of POCD; these pharmacological effects have been demonstrated to disrupt blood-brain barrier integrity, influence microglial polarization, and have been linked to worsening prognoses in cognitive decline. Moreover, mechanical stress from surgical intervention and reperfusion injury may exacerbate the generation of reactive oxygen species (ROS), thereby increasing oxidative stress to the brain synergistically with blood-brain barrier disruptions. In previous studies, factors for the variable incidence and various risk factors have been explored. In this review, we examine the pharmacological effects of local, regional, and general anesthesia on molecular and cellular glial response, along with its intercellular interactions and previously reported clinical outcomes.
Introduction
Post-operative cognitive dysfunction (POCD) has been reported as early as 1887 but only recently has been shown to be a consequence of complex microglial interactions with the surrounding microenvironment (1, 2). While the guidelines remain unclear, emerging evidence suggests that the interplay of age, gender, administration of anesthesia, and microglial responses may culminate in a post-operative neuropsychiatric change in cognition (3). However, the unclear condition and nature of POCD may partly be due to the variability of POCD assessments that have been previously reported (4). Despite the assessment variabilities, the commonly reported conditions involve decline in cognitive functioning and, more recently, microglial involvement (5).
Microglia are the resident, antigen-presenting, macrophage-like cells of the brain that primarily mediate central nervous system (CNS) inflammation through complex interactions with neurons, endothelial cells, astrocytes, oligodendrocytes, and other resident cells of the CNS (6, 7). The interactions between glial cells and neurons influence how the brain perceives, experiences, and interacts with the world; thus, states of immune dysregulation may compromise this functioning leading to neuropsychiatric pathologies (7, 8). In normal homeostasis, microglia survey their microenvironments and interact with the cellular landscape of the brain, thereby affecting cognition, memory, and learning (7). Thus, aberrant changes to microglial functioning due to anesthesia and surgery may directly impact cognitive functioning, mental health, and emotional regulation through complex interactions within the brain parenchyma (Figure 1, Table 1).
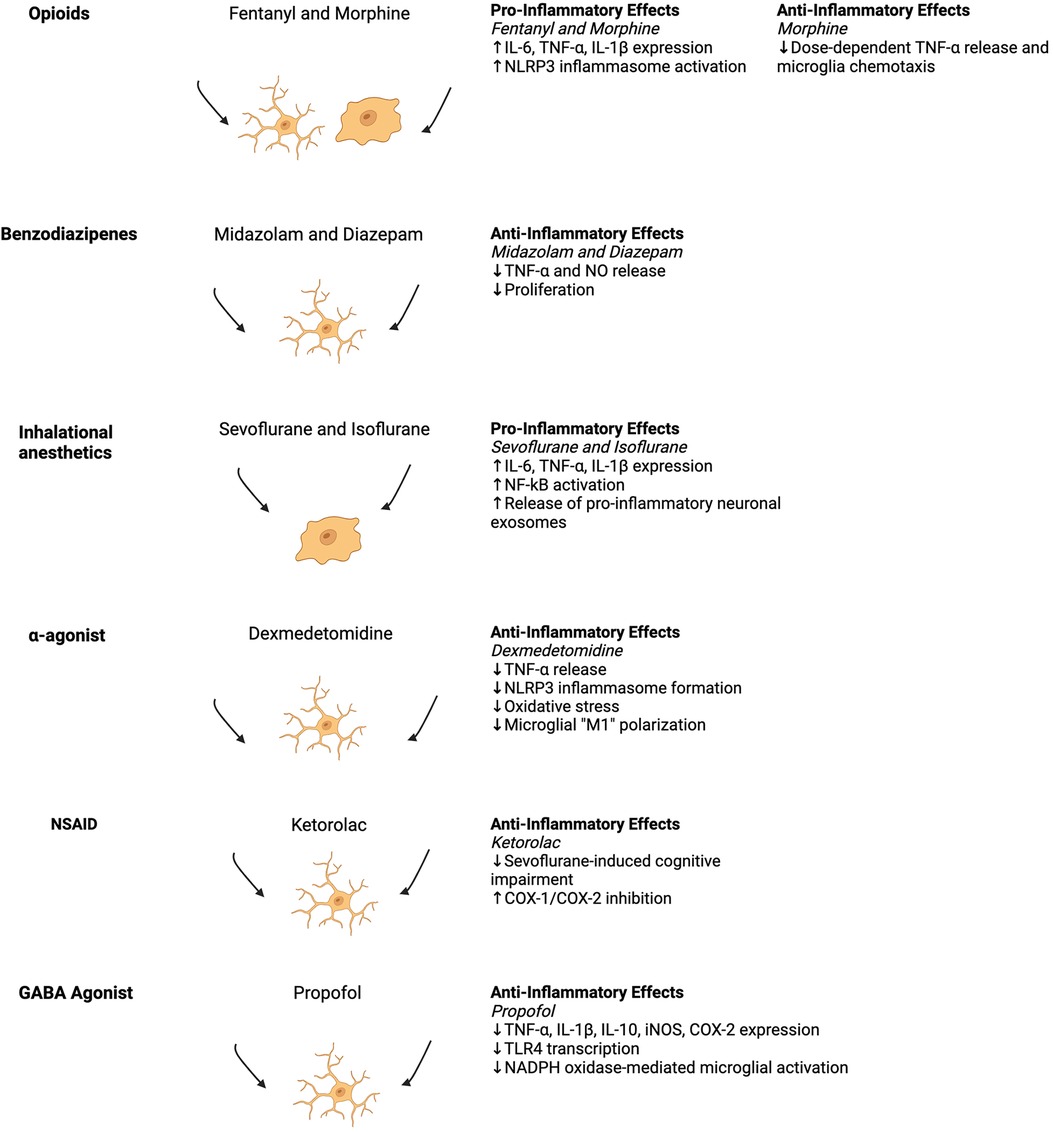
Figure 1. Schematic of the general summary of anesthetic effects on inflammatory phenotypes with associated historical depictions of pro- and anti-inflammatory (ameboid and ramified) microglia morphologies (9). Studies from the figure are cited in Table 1. Created with Biorender.com.
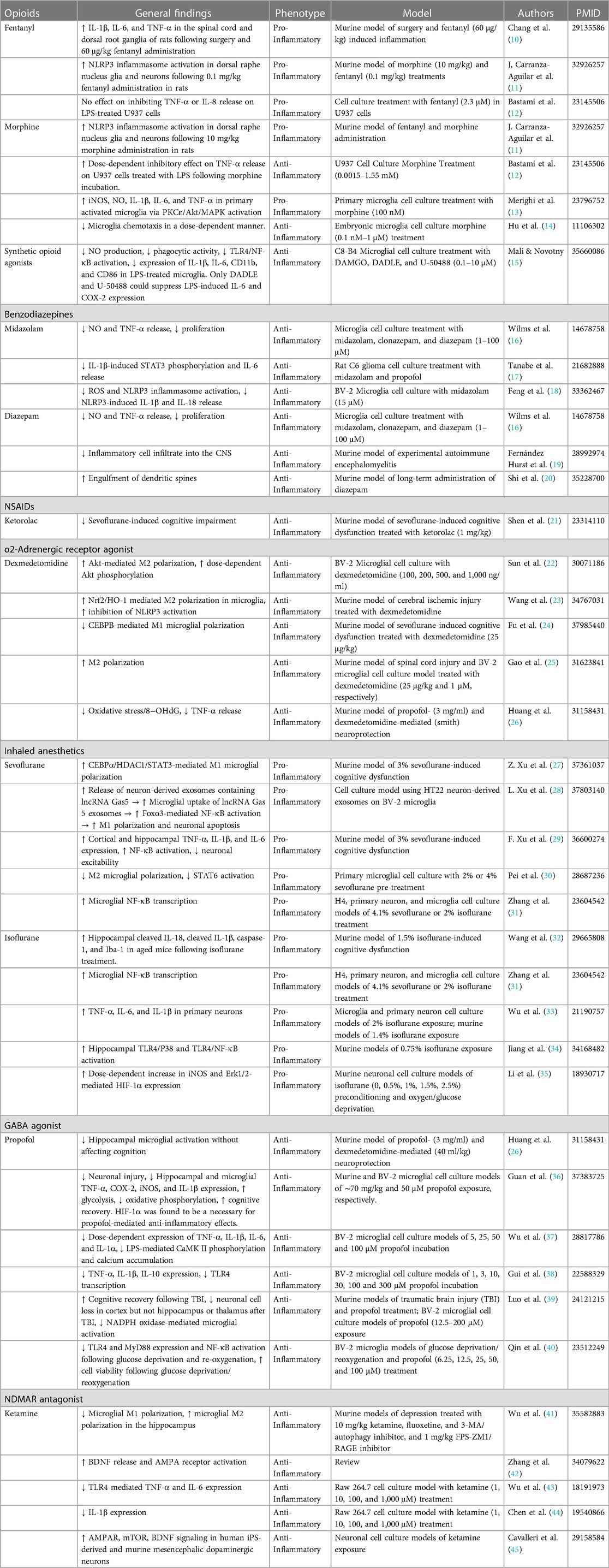
Table 1. General summary of the studies regarding anesthesia-mediated inflammatory phenotype changes.
Moreover, anesthetic mechanisms of action are complex and affect blood-brain-barrier (BBB) permeability, neuronal functioning, and glial responses. Previous studies have proposed neuroinflammation, oxidative stress, BBB integrity, and metabolism dysregulation as mechanisms of POCD. Here, we examine these studies through the intersection of age and anesthetics on the BBB, with the goal of shedding new insight into the pathogenesis of POCD. Moreover, we review the clinical, basic science, and translational studies of POCD, focusing on the neuro-immune axis and interplay with the complex pharmacology of cardiac- and non-cardiac surgery.
POCD incidence discrepancies
POCD has varying reports of prevalence following surgery (46, 47). The reported incidences of POCD following non-cardiac surgery vary between 41%–75% at seven days and 18%–45% after three months postoperatively (46–48). Conversely, POCD incidences following coronary-artery bypass surgery have been reported to be 53%, 36%, and 42% at discharge, six weeks, and five years, respectively (49). In 2005, Gao et al. reported that POCD was observed in more than 40% of patients several months following cardiac surgery. This was also observed in 25% in patients over 60 in non-cardiac surgery one week following surgery with a resolution to 10% after 3 months (46). The discrepancies in prevalence may be due to a lack of previously standardized diagnostic criteria (48).
New nomenclature has been reportedly introduced for POCD in the fifth edition of the Diagnostic and Statistical Manual of Mental Disorders (47, 50). Recent efforts have been made to update the nomenclature from POCD to peri-operative neurocognitive disorders (PND) to encompass delirium and cognitive dysfunction under an umbrella description (47). To date, however, POCD does not appear in DSM-5 and only describes post-operative delirium with an incidence that ranges between 15% and 53% (50).
Clinical risk factors for POCD
Age is widely recognized as a significant risk factor for POCD. Studies have consistently shown that advanced age is associated with a higher incidence of POCD, which refers to a decline in cognitive function following surgery (51). The underlying mechanisms are not fully understood but may involve age-related changes in the brain, increased vulnerability to perioperative stressors, and the presence of pre-existing cognitive impairment (52, 53). Several studies have investigated the impact of age on POCD, highlighting its negative influence on postoperative cognitive outcomes. Moller et al. found that among POCD patients followed for three months, duration of anesthesia, education, multiple surgeries, postoperative infections, and respiratory complications were risk factors for early POCD, but only age was a risk factor for late POCD (54).
Preoperative cognitive impairment or decline could be a potential risk factor for POCD (55, 56). Patients with cognitive impairment before surgery are more vulnerable to experiencing cognitive decline following the procedure (57). Chen et al. conducted a meta-analysis and revealed that preoperative cognitive impairment in older non-cardiac surgical patients significantly increased the risk of delirium, 1-year mortality, discharge to assisted care, 30-day readmission, and postoperative complications (58). Similarly, Au et al. (59) found that in older cardiac surgical patients, preoperative cognitive impairment was associated with an 8-fold increased risk of delirium and an increased hospital and ICU length of stay (59).
The type of surgery is highly influential in the development of POCD (60). Different surgical interventions can impact the incidence and severity of cognitive decline following surgery. Factors such as the invasiveness of the surgery, duration of anesthesia, and exposure to certain anesthetic agents can influence the risk of POCD (4). Cardiac surgery has been given special attention when researching POCD given the higher prevalence of dysfunction following surgical procedures (61). Bhushan et al. (62) found that the incidence of POCD ranged from 9% to 54% in different studies and the highest incidence was observed after open aortic, transcatheter aortic valve implantation, and coronary artery bypass graft surgery (CABG) (62). Understanding the impact of specific surgeries' cognitive outcomes is crucial for optimizing patient care and implementing preventive strategies.
The duration and complexity of surgery may also affect the risk of POCD. Prolonged surgical procedures and complex surgeries are associated with an increased likelihood of cognitive decline in the postoperative period. Extended exposure to anesthesia, physiological stress, and surgical trauma can contribute to cognitive impairment. In an animal study designed to test learning abilities following prolonged disruption after general anesthesia, Culley et al. (63) found a persistent deficit in performance that was not explained by other factors (63). The authors' findings suggest a long-term impairment in learning and memory following an extended duration of anesthesia.
The route of anesthesia administration (intravenous vs. inhalation) may contribute differently to the severity of POCD. Negrini et al. (64) completed a thorough meta-analysis on the rates of POCD incidence following inhalation and total intravenous/injectable anesthesia (TIVA) (64). The researchers defined two objectives: identifying POCD incidence within the first 30 days after the procedure and assessing cognitive function from 30 days to 1 year. With 10 total studies, 1,660 participants were assigned to the TIVA group and 1,730 participants to the inhalation group (64). It was determined for the first objective that the incidence of POCD in the TIVA group was 11.4% compared to the inhalation group at 27.7% (64). Another meta-analysis carried out by Yongbo Di et al. on the outcomes of elderly patients undergoing cancer surgery found no significant difference between intravenous and inhalation anesthetics after one month, but also agreed with the finding that those using inhalation anesthetics have 1.49 times higher risk of having delayed neurocognitive recovery when compared with intravenous agents (65). From these two metanalysis, it seems that the difference between inhaled and intravenous anesthetics is primarily found in the first month after surgery.
Genetic risk factors
One genetic risk factor for POCD is individuals with APOE4 carrier status (66). The longitudinal study performed by Schenning et al. (66) revealed that genetic variations in APOE4 may contribute to POCD pathogenesis. However, conflicting reports are currently present. One study was unable to show a significant association between APOE4 and POCD—whether this is from low statistical power or the pathobiology is unclear (67).
A prospective cohort study utilized genotype data on patients undergoing coronary artery bypass graft (CABG) surgery and showed that minor allelic variations in P-selection and C-reactive protein (CRP) influence POCD outcomes (68). Specifically, the 1059G/C (rs1800947) and 1087G/A (rs6131) minor alleles of CRP and the P-selection-encoding SELP gene, respectively, were associated with a reduction in cognitive dysfunction in 443 European Americans (68). These allelic variations were also associated with reduced perioperative serum CRP and platelet activation, thereby providing a physiological mechanism for the observed genotype variations (68). An additive effect was also demonstrated in patients carried at least one copy of 1059C and 1087A alleles; cognitive deficit incidence rates were 16.7% and 42.9% in patients for carriers of both loci compared to homozygous 1059G and 1087G major allele carriers, respectively (68). While the group examined 37 SNPs in 16 candidate genes including APOE, the major differences were only observed in the previously mentioned CRP and SELP alleles (68). Another study revealed that post-CABG patients who were homozygous for +1444TT had higher CRP levels compared to those who carried a +1444C allele (69).
In the setting of non-emergent cardiac surgery, a gene variant in PH-domain and leucine rich repeat protein phosphatase, PHLPP2 (rs78064607), was identified as having genome-wide significance in a genome-wide association study (GWAS) (70). PHLPP2 has been implicated in the response following ischemia/reperfusion injury and oxidative stress damage to the brain and kidney, respectively (70). In cellular settings PHLPP2 dephosphorylates and inactivates Akt, thereby inhibiting protective pathways following cerebral ischemia/reperfusion injury (70, 71).
Cholinergic gene variants in CHRM2 (rs8191992, rs6962027) and CHRM4 (rs2067482) have also been assessed in the context of post-operative delirium in a candidate-gene association study approach (72). However, no variants in this study reached significance with a GWAS approach (72).
Variations in BDNF have also been identified in Chinese Han populations (73). Xie et al. (73) revealed that an A > G polymorphism at rs6265 locus of BDNF may be protective for POCD development. Patients carrying GG and GA alleles had significantly lower POCD outcomes than those homozygous for the A allele at 7-days post-operatively (73). However no differences were seen 3-months following surgery (73).
Epigenetic involvement
Epigenetic modifications may enhance or protect from POCD-related changes. One group examined DNA methylation changes in the controversial APOE3 and APOE4 variants in a mouse model using isoflurane and surgery (74). The group found hypomethylated regions related to axonogenesis in both isoforms after surgery (74). Conversely, genetic segments relating to synaptic functioning, neurotransmitter trafficking, long term potentiation, and axon guidance in the E3 isoform were significantly hypermethylated following surgery (74). Specifically, Ephrin B2 and Eph A7 receptor gene segments were found to be hypermethylated (74). These cognitive changes and their associated epigenetic modifications were only observed in the E3 allele; this may be partially explained by the lower baseline cognitive performance by mice harboring the E4 allele (74).
Changes in N6-methyladenosine (m6a) methylation patterns have also been studied in the context of POCD mouse models using sevoflurane (75). The study revealed that m6A RNA methylation was significantly elevated in 56 genes and silenced in 1,244 genes in the POCD model compared to controls (75). There was overall a significant reduction in m6A methylation in the mouse hippocampus (75). These reductions could be explained by sevoflurane-mediated suppression of MAPK/ERK activity leading to inhibited methyltransferase activity by METLL3 (75).
Isoflurane rat models of POCD demonstrated abhorrent H3K9 and H4K12 acetylation resulting in dysregulated BDNF signaling (76). These modifications were reversed upon treatment with apigenin (77). Interestingly, sevoflurane models of POCD with exploratory laparotomy in mice resulted in increased histone deacetylase 2 (HDAC2) activity resulting in decreased BDNF and TrkB activity (78). A similar study revealed isoflurane in aged mice upregulated HDAC3 activity in the dorsal hippocampus leading to cognitive impairment (79). These impairments were ameliorated upon HDAC3 inhibition using RGFP966 (79). Overexpression of HDAC3 in young mice resulted in similar patterns of cognitive dysfunction (79). Isoflurane and surgery may lead to activation of HDAC, resulting in decreased acetylation of H3 and H4 histones while leading to a decrease in neuroligin 1 (78, 80, 81). Interestingly, environmental enrichment attenuated these cognitive and epigenetic changes when the environment was enriched two weeks before surgery (81).
miRNA targets
Analysis of preoperative miRNA may also be a promising candid biomarker for predicting POCD outcomes (82). Specifically, miRNA-155 was found to be a strong predictor of POCD progression in patients undergoing laparoscopic surgery for colon cancer (82). Recent studies have unveiled that miRNA is implicated in inflammatory processes and has even been described as a “master regulator of inflammation” (83). The expression of miRNA-155 has been implicated in cancer, asthma, cystic fibrosis, and cardiovascular disease (83, 84). One compound, MLN4924 (pevonedistat), has been demonstrated as a NEDD8-activating enzyme inhibitor that blocks NF-κB from upregulating the miRNA-155 promoter in the context of acute myelogenous leukemia (85).
Epigenetic targeting
As previously described, inhibition of HDAC2 and HDAC3 may ameliorate anesthesia-related cognitive changes following surgery (78, 79). Apigenin, a plant-derived flavonoid, was shown to recover histone acetylation in vivo that reduced isoflurane-induced suppression of BDNF and cognitive deficits while reducing inflammatory cytokines associated with neuroinflammation (76).
Neuroimmune functions
The CNS, composed of the parenchyma proper, ventricles, and meninges, has long been considered “immune privileged” (86). While the meninges, perivascular spaces, and choroid plexus are populated by dendritic cells (DC) and macrophages, the parenchyma is inhabited by microglia, a specialized immune cell that compromises nearly 10% of all glial cells (87). Microglia are vital to the parenchymal milieu for CNS homeostasis, development, and response to insult and trauma. These specialized, multifunctional cells engage in continuous surveillance of the microenvironment through their ramified processes (7, 88).
Morphologically these cells can be characterized as ramified, ameboid, or hypertrophic, depending on the stimuli (88–90). When ramified, microglia engage in surveillance through cell-cell contacts with the vasculature, astrocytes, and neuronal somas (90, 91). In the aging brain, however, dystrophic microglial morphologies have been observed while ameboid phenotypes have been reported to occur in the developing CNS (90, 92).
Historically, these cells have been described to become polarized to an “M1-like” or M2-like” state, which corresponds to a pro- and anti-inflammatory characteristic, respectively (93–95). According to Tang and Le (96), the M1 microglia are associated with sites of injury and inflammation, and are generally considered cytotoxic. Conversely, the M2 microglia are characterized by anti-inflammatory phenotypes and are associated with tissue-repair, phagocytosis, immunosuppression, regeneration, and neuroprotection. The M1 microglia produce pro-inflammatory mediators such as interleukin 1 beta (IL-1β), tumor necrosis factor α (TNF-α), IL-6, reactive oxygen species (ROS), nitric oxide (NO), and superoxide in response to injury through activation of iNOS and NF-κB pathways (95, 96). On the other hand, M2, alternatively-activated microglia are induced by interleukin-4, -10, and -13 (IL-4,-10,13) and transforming growth factor β (TGFβ), which result in phagocytosis, extracellular matrix (ECM) remodeling, and neuroprotection (96). However, current standards are moving towards describing microglia as having multiple states, such as M1 or M2, existing on a spectrum through proteomic, metabolic, transcriptomic, morphological, or epigenetic studies (9).
The functions of microglia-neuron crosstalk include engulfing apoptotic cells and dendritic spines, phagocytosing functional and non-functional synapses, forming axonal tracts, and clearing cellular debris (92, 97). Through these interactions, microglia regulate neuronal activity and synaptic plasticity and by extension, can influence cognition, learning, and memory depending on their physiological state (92). Additionally, microglial interactions with astrocytes contribute to homeostasis and neuroinflammation through complex signaling affecting the neurovascular unit (NVU), thereby affecting the BBB permeability and function (95).
Mechanistically, microglial responses to insult have been shown to be mediated through ATP signaling, neuronal NMDA receptor activation, and extracellular calcium signaling, as reviewed by Tay et al. (92). For example, microglial response to laser injury has been shown to be mediated by through microglial P2RY12 and ATP signaling in vivo (92, 98, 99). Another purinergic signaling mechanism was discovered to be through dendritic NDMA receptor activation, which resulted in the release of ATP, thereby causing an outgrowth of microglial processes (92, 100). Moreover, a decline in cerebral extracellular calcium concentrations has been shown to guide microglial processes towards neuronal dendrites (92, 101).
Like neurons, microglia-astrocyte communication is influenced by the polarization state of microglia (95). Through cytokine, chemokine, and ATP signaling, microglia influence astrocytes to become neurotoxic or neuroprotective, depending on the stimuli (102).
Aging effects on the blood brain barrier
The BBB consists of continuous non-fenestrated endothelial cells (ECs) encompassed by pericytes, smooth muscle cells, astrocytes, microglia, oligodendrocytes, and neurons that are collectively called the neurovascular unit (103). As age progresses, there is natural deterioration of the BBB, allowing unwanted pathogenesis and an overall decrease in global cerebral blood flow (104). The BBB is a fundamental element of normal and healthy brain function and deterioration can be used as a biomarker for the normal aging process (105). Furthermore, the dysregulation of nutrients such as glucose consumption may cause metabolic-associated inflammation (106). As age progresses, a natural resistance to insulin develops and causes a general increase in blood glucose levels (107). Changes in plasma glucose levels are associated with altered BBB transport function, integrity, and oxidative stress in the CNS microcapillaries. An example is the increase in type 1 membrane-protein expression which transports amyloid-beta from the blood into the brain, establishing a link between glucose dysregulation and Alzheimer's disease. Hyperglycemia has been associated with progression to cerebral ischemia and enhancement of secondary brain injuries (108).
POCD, similar to the effects of aging, is also believed to be caused by mechanisms such as neuroinflammation and oxidative events, according to preclinical studies (109). Furthermore, the inflammatory hypothesis has been observed in clinical studies, with patients showing elevated levels of pro-inflammatory cytokines in both the CNS and periphery following surgeries such as primary total hip arthroplasty with spinal anesthesia (110, 111). Therefore, the correlation between the aging effects of the BBB's constituent cells and the impact of anesthesia on those cells can yield mechanistic understandings of the causes and effects of POCD. Cells notable for changes in aging are ECs, pericytes, astrocytes, and microglia that result in dysregulation and expression of extracellular components and tight junction proteins (104).
During aging, oxidative stress induces TNF-α production in EC, thereby causing degradation of the basement membrane and tight junctions (112, 113). Loss of capillary ECs has also been implicated during aging, resulting in elongation of the remaining ECs to maintain coverage (114). The upregulation of apoptotic pathways occurs in pericytes through the activity of caspases 3/7 (115) resulting in decreased cell viability (116). Focal pericyte loss induces capillary dilation and disrupts normal flow (117). Subsets of affected capillaries experience reduced perfusion due to flow steal and others are susceptible to stalls in flow and regression, leading to loss of capillary connectivity (118). Furthermore, senile pericytes produce NO which react with O2 resulting in free radical damage (119). ROS increase results in calcium dysregulation in neurons, repressing calcium-binding proteins and causing further BBB degradation and neuronal loss (120). This oxidative stress triggers astrocytes to upregulate the expression of cytokines and chemokines such as matrix metalloproteinase 3 (MMP3) and p16INK4A (senescence associated secretory phenotype SASP) that induce neuroinflammation, increased permeability, and ultimately BBB disruption (121).
Once aging has impacted the integrity of the BBB, blood-derived proteins such as fibrinogen and plasminogen cross more readily. Pro-inflammatory fibrin aggregates in the brain, binding CD11b/CD18 and repolarizing microglia to a pro-inflammatory M1 phenotype, leading to further inflammation and neurodegeneration (122, 123). Primed microglia produce exaggerated and prolonged IL-6 activity, leading to a positive feedback loop in M1 activated microglia (124). This is a theme seen in aging microglia-hypersensitivity to inflammatory stimuli. This can further be attributed to the limited repopulating capacity of microglia and therefore increased number of aged microglia population and resulting overload of work due to the decreased number (125). Consequently, there are changes in several immune checkpoints resulting in increased immune vigilance along with dysregulated phagocytosis (126, 127). An increase in neurotoxic substances and reduced ability to phagocytose toxic debris and proteins results in apoptotic bodies, misfolded protein aggregates, and myelin—hallmarks of age-related neurogenerative diseases (128–130).
Anesthetic effects on the blood brain barrier
The effects of anesthetics such as isoflurane, sevoflurane, and desflurane have been previously studied (131–133). Globally, aging can cause dysregulation of glucose consumption and metabolism, leading to exacerbation of secondary brain injuries and Alzheimer's disease. Sevoflurane has been shown to induce iron overload and inhibit oxygen and glucose absorption rates by downregulating GLUT1 transporters in the cerebral endothelial cells (134). The combination of the natural insulin resistance seen in aging and the effects of anesthetics such as sevoflurane may lead to cumulative exacerbation of metabolism dysregulation. When looking at the effects of anesthesia on the BBB, it is important to account for the inflammatory effects of surgery itself. Surgery induces inflammatory processes both locally and systemically. The release of cytokines such as IL-1 and TNF-α from ECs and microglial activation following surgery can trigger cascades that increase BBB permeability (135). Earlier, it was discussed that intravenous drugs, such as propofol, may be relatively beneficial with regard to POCD incidence compared to inhaled anesthetics, especially in the first month of recovery. A mechanism through which this may be explained is propofol's phenol-based structure and anti-inflammatory effects (136). These anti-inflammatory effects may be explained in part due to suppression of prostaglandin E2 and leukotriene production, thereby attenuating the probability of POCD pathogenesis (137, 138).
The common initial mechanism believed to initiate damage of the BBB is limited ischemia due to sedation (139). Through scanning electron microscopy, it has been shown that sevoflurane and isoflurane can induce the death of brain ECs resulting in focal BBB breakdown (140). This was especially prevalent in the vicinity of BBB-associated tight junctional folds and appeared to be the first indication of cell death. The integrity of the BBB was studied through immunohistochemistry staining for immunoglobulin G, and recovery after 24 h was not observed. Of note, after 24 h, brain ECs in young and middle-aged animals appeared more comparable to controls than those of older animals, continuing the correlation between age and BBB integrity (140).
Employing dextran-tracer injection to reveal BBB formation and function, laparotomy under 1.4% isoflurane anesthesia was shown to induce BBB dysfunction as evidenced by increased permeability to 10-kDa dextran postoperatively in the brain tissues of 18-month-old mice compared to 9-month-old mice (141). The above findings were shown in conjunction with increased IL-6 signaling and attenuation with IL-6 knock-out, indicating an IL-6 dependent pathway. This highlights the significance of IL-6 increase in the brain with age and its effects on microglia (124). Regarding IL-6, microglia are the most potent secretory cells and targets for cytokines (130). This study demonstrated that anesthetics modulate microglial activation in a time and dose-dependent manner. Data show that MHC-II plays a role in microglial hypersensitivity and indicates that IL-6 significantly contributes to the microglia-induced exaggeration in neuroinflammation in aged mice following peripheral immune stimulation (142, 143). IL-6 is the main cytokine in producing classically activated M1 microglia, shown to induce inflammation and destruction of the BBB (124). In an established sevoflurane-induced neurotoxicity (SIN) animal model, prolonged anesthesia triggered the activation of NF-kB inflammatory pathway, neuroinflammation, inhibition of neuronal excitability, cognitive dysfunction, and anxiety-like behaviors (29). Specifically, RNA sequencing focused on the neuromorphopathological factors of SIN showed microglial migration, activation, and phagocytosis to be enhanced. Feng et al. found that C1qa and C3 were increased, and C1qa tagging of synapses was also elevated. These activated microglia are shown to be engulfing the hippocampus after prolonged anesthesia, destroying synapses in the area. A specific consequence was the reduction of dendritic spines and a downregulation of genes coding for glutamatergic, cholinergic, dopaminergic, and GABAergic synapses. Propofol as an intravenous anesthetic may be beneficial compared to inhalation anesthetics in offsetting these events as exposure has been shown to not be cytotoxic to macrophages and ELISA revealed significantly reduced levels of LPS-induced TNF-α, IL-1β, NO, and IL-6 biosynthesis by these same cells (144).
A study using two- and six-month-old mice showed that anesthesia and surgery could exacerbate and mirror the results of aging on pericytes (116). CD13- and lectin-positive brain capillary distribution was examined using immunostaining to visualize brain pericytes. The results were decreased hippocampal pericyte coverage and capillary length in old mice compared to younger mice. A Pearson correlation analysis showed degeneration of brain capillaries in the hippocampus of aged mice was closely related to insufficient numbers of pericytes.
The few studies on anesthetics and their effects on astrocytes have been contradictory. Astrocytes are crucial to the emergence from anesthesia through mitochondrial complex 1 function (145). Loss of function of the complex I gene, Ndufs3, confers a profound hypersensitivity to volatile anesthetics (145). Further studies utilizing transgenic in vitro and in vivo models can shed light on anesthetic hypersensitivity involving astrocytes.
Reactive oxygen species effects on the blood brain barrier
Free radicals can be a causative factor in weakening BBB integrity (104). Following surgery, reperfusion injury in tandem with anesthesia-mediated ROS release may further weaken the BBB (146). Furthermore, calcium dysregulation in aging neurons leads to elevated ROS levels (120). Inflammation in the CNS is a major driver of local production of oxidative species, primarily through activity of the microglial proinflammatory phenotype (147). The result is a cumulative BBB insult and leakiness that may promote POCD pathogenesis (148). Thus, damage to the BBB may be a common denominator when examining the effects of ROS in aging and the incidence of POCD due to anesthesia and reperfusion injury.
During sedation, acute hypoxia in ECs and the consequent reperfusion and re-oxygenation produce active metabolites and resulting chemotactic mobilization (139). There is recruitment of activated neutrophils which coagulate with the ECs. More neutrophils adhere to the endothelium of post-capillary venules, causing damage with the release of proteolytic enzymes such as elastase, collagenase, β-glucuronidase, N-acetyl-β-glycosamindase, free radicals, and leukotrienes (149–151). These enzymes have been shown to contribute to general injury of the central and peripheral nervous system postoperatively in addition to more specific pathologies associated with POCD such as Alzheimer's Disease (AD), multiple sclerosis, and dementia after HIV-1 infection (152, 153).
Anesthesia and aging regarding age-related disorders
POCD may have a role in aggravating age-related disorders such as dementia, anticholinergic crisis, and AD. About 8.5 million AD patients need surgery each year (154). In those greater than 80 years of age, general anesthesia was associated with a greater risk of developing AD (155). An inverse correlation has been shown between the age of AD onset and cumulative exposure to general and spinal anesthesia before the age of 50 (156). Research has shown significant links between POCD and delirium, central anticholinergic syndrome, dementia, and akinetic crisis (4). The central cholinergic system plays a role in regular cognitive functioning; inhibition of these muscarinic and nicotinic receptors has been shown to contribute to learning and memory deficits associated with delirium (157). General anesthetics may potentially modulate cognitive function, especially by inhibiting the function of nAChRs. Imaging studies have shown that age-related increases in BBB disruption occur especially in regions most vulnerable to age-related deterioration, indicating that BBB disruption is an underlying mechanism of normal age-related decline (105, 158). Significantly increased BBB permeability along with mild cognitive impairments is often considered the transition state to AD (159). Nation et al. demonstrated that older patients showing early cognitive dysfunction had increased BBB permeability compared to those with no cognitive impairment, even in the absence of any diagnosed neurological or psychiatric condition (160).
The most significant risk factor for vascular-based neurocognitive disorders is aging, with more than 50% of the US population over the age of 80 suffering from AD or vascular disease (VaD) (106). In vitro and in vivo studies have shown that pro-inflammatory cytokines TNF-α and IL-1β down regulate the expression of tight junction proteins occluding-1, claudin-5, ZO-1, and ZO-2 (161). In natural aging, human brain ECs produce complement regulatory proteins, which become elevated during CNS injury or increased permeability/damage of the BBB (162). Normally, the BBB prevents complement protein and components from entering the CNS, but as age progresses, permeability increases, and complement alters the function of microglia, oligodendrocytes, and neurons (163). Again, this permeability can also be seen in POCD. Byproducts of the complement system, C3a and C6a, increase recruitment of inflammatory cells into the brain and the induction of cytokine cascades (IL-1, TNF-α, IL-6, IL-8, IL-17) (135, 164). This has been shown to be associated with AD pathogenesis, as complement binding to amyloid-beta (Aβ) results in pathological amyloid plaques (165). Another blood protein mentioned earlier that is allowed to pass the BBB is fibrinogen (123). Fibrinogen has been shown to contribute to the formation of amyloid plaques along with the cytokines mentioned above.
It has been proposed that the pathological mechanisms underlying POCD mimic that of AD disease (116, 124, 139). The previously reviewed mechanisms behind aging and AD have shown that many of the mechanisms are not only similar, but cumulative. Aggregated Aβ is the main constituent of amyloid plaques found in the brain of AD patients. Different studies have shown the ability of anesthetics to promote the oligomerization of Aβ peptide, supporting a potential link between anesthesia and the acceleration of Aβ-related toxicity (155).
Additionally, isoflurane has been shown to cause mitochondrial dysfunction through opening the mitochondrial permeability transition pores (mPTP) (154). When the mPTP are more readily opened through isoflurane, Zhang et al. (154) observed memory and learning deficits corroborated with a reduction in ATP and mitochondrial membrane potential and an increase in apoptosis marker caspase–3 (154). These mitochondrial dysfunctions have further been associated with AD pathogenesis (154). Isoflurane has also been shown to decrease cognitive performance and responsiveness in wild type animals compared to control, and increase mortality, apoptosis, and Aβ aggregation (166).
Mechanistic effects of anesthesia on neuroinflammation
Regional and local anesthesia
Regional and local techniques are utilized to induce analgesia and hypoesthesia at a surgical site without affecting the level of consciousness in a patient. They differ mainly in the site of application, with regional anesthesia being applied at a distance from the surgical site and local anesthesia being directly at a nearby nerve (167). The main types of regional anesthesia include neuraxial anesthesia, which includes epidural and subarachnoid blocks, peripheral nerves blocks and intravenous regional anesthesia (168). Local anesthesia is generally administered topically or subcutaneously, however it may be given orally in certain procedures as well (169). Drugs commonly used in local and regional anesthesia include amino amides and amino esters, both which act on voltage-gated sodium channels to block nerve signal transmission. They differ most significantly in their metabolism such that amino amides are hydrolyzed in the liver and amino esters are metabolized by plasma cholinesterases (169).
Amino amides frequently used in practice such as lidocaine, bupivacaine and ropivacaine have been shown to exhibit direct suppressive effects on microglia and astrocyte related inflammation. In one in vitro study of microglia clone cells from rat cerebella, researchers induced damage through incubation with LPS and IFN-γ in the presence or absence of lidocaine (170). The researchers found attenuated levels of LDH in the samples treated with lidocaine, which was correlated with decreased microglial activation and injury (170). Su et al. also performed an in vitro study on rat microglia, however they instead highlighted the effect of lidocaine on the production of inflammatory cytokines in response extracellular ATP-mediated activation of p38 MAPK. Lidocaine was shown to attenuate the transcription and serum levels of TNF-α, IL-1β and IL-6 in the two hours post-administration (171). Studies of bupivacaine yielded similar results, with one animal trial performed by Zhang and Deng also showing a significant reduction of TNF-α, IL-1β and IL-6 production in 32 rats administered complete Freund's adjuvant, a known mediator of inflammation. Additionally, the researchers also found that bupivacaine treatment significantly decreased the expression of the spinal microglial marker OX42 and the astrocyte marker GFAP (172). Lastly, ropivacaine was also found to suppress the activation of microglia and astrocytes through measurements of CD11, a marker of microglial activation, and GFAP in mouse models (173).
There have been mixed reports on the efficacy of lidocaine attenuating POCD incidence rates during cardiac surgery (174–176). However, a potential benefit of lidocaine has been published in the context of laparoscopic colorectal surgery (177). Moreover, regional anesthesia alone or in conjunction with general anesthesia has not been shown to significantly attenuate the incidence of POCD in meta-analyses. Interestingly, one clinical trial including 438 elderly patients found no significant difference in POCD incidence rates three months post-surgery following general or regional anesthesia (178). One meta-analysis of 16 studies found that regional anesthesia showed no difference in cognitive function as opposed to general anesthesia in 13 of the studies, while 3 of the studies produced results slightly favoring regional anesthesia (179). Similarly, another meta-analysis of 7 studies found that regional anesthesia had no significant impact on MMSE testing results at 24 h, three days or seven days post-surgery as opposed to general anesthesia in elderly patients undergoing hip fracture surgery (180). Lastly, a third meta-analysis of 13 studies by Viderman et al., found that regional anesthesia produced no significant difference in cognitive testing at one week and three months post-surgery as opposed to general anesthesia (181). The authors of these meta-analysis highlight several limitations in the literature, such that more research is required for a better understanding of the complex pathogenesis of POCD.
Inhalational anesthetics
Inhalational anesthetics such as isoflurane and sevoflurane have been associated with pro-inflammatory phenotypes in vitro and in vivo (27–35) (Table 1). However, one study revealed a low POCD incidence (1.22%) and no difference in patients who received sevoflurane or desflurane during low-risk surgery (182).
When isoflurane and sevoflurane were compared, it was found that isoflurane depletes calcium from the endoplasmic reticulum (ER) in cerebrocortical and hippocampal neurons while sevoflurane increased calcium concentrations in cortical neurons (183–185). Wei et al. (185) further showed that only isoflurane induced cytotoxicity mediated through a decrease in the Bcl-2/Bax ratio when compared to sevoflurane.
However, the balance of cytotoxicity and cytoprotection may vary in the literature, depending on the experimental conditions. Isoflurane was reported to confer neuroprotection in models of stroke (186). Specifically, isoflurane was shown to protect against oxygen/glucose-deprivation in neurons while increasing transcription of hypoxia inducible factor 1α (HIF1α), Erk1/2, and iNOS (35). Both isoflurane and halothane were even shown to reduce brain damage from focal ischemia in rodent models (187).
Despite the evidence for neuroprotection with isoflurane, sevoflurane, and halothane in ischemia, there is emerging data supporting the association between isoflurane and cognitive impairment in an age-dependent fashion (32). Isoflurane was shown to induce the assembly of a NOD-like receptor protein 3 (NLRP3), forming an inflammasome composed of NLRP3, a caspase recruitment domain (ACS), and caspase-1 (32). As reviewed, this inflammasome mediates the secretion of pro-inflammatory cytokines such as IL-1β and IL-18. Similarly, exposure to sevoflurane has been shown to result in hippocampal microglial activation with elevated IL-1β, IL-6, and TNF-α, mediated through HCN2 channel inhibition (188). Specifically, sevoflurane resulted in downregulation in PEX5R/Trip8b-HCN2 channels in microglia and neurons while causing neuroinflammation in the rodent cortex and hippocampus. A gene enrichment analysis of HCN2 revealed to be associated with “learning and memory, positive regulation of glutamate secretion, regulation of nervous system processes, synaptic processes and transmission, behavior, membrane potential, and negative regulation of sodium ion transport”. Moreover, sevoflurane has been shown to promote microglia M1 polarization through suppression of STAT-6 and inhibition of IL-4 induced M2 polarization (30). Sevoflurane has been shown to induce microglial M1 polarization through release and transfer of neuron exosomes containing lncRNA Gas5 to microglia, which upregulates Foxo3 and activates the inflammatory NF-κB pathway (28). Another mechanism implicated has been increased transcription factor activity of CEBPα which activates the pro-inflammatory HDAC1/STAT3 pathway (27) (Table 1).
Likewise, studies of isoflurane have been demonstrated to upregulate IL-6, TNF-α, IL-1β, along with microglial NF-kB activation (31, 33). The isoflurane-induced model of neuro-inflammation may also be partially explained through activation of TLR4/P38 and TLR4/NF-kB pathways (34) (Table 1).
Ketamine
Ketamine is an NMDA receptor antagonist used as an induction agent for dissociative anesthesia and has been shown to prevent bronchospasms, act as an ionotrope, and even treat major depressive disorder (189–192). The anti-inflammatory characteristics of the (R,S) racemate have been described as early as 1998 (193, 194).
In 1988, Roytblat et al. performed a clinical trial on preoperative 0.25 mg/kg ketamine administration in patients undergoing elective CABG/coronary bypass surgery. The group revealed that patients undergoing CAPG had significantly lower serum levels of pro-inflammatory IL-6 seven days post-surgery with insignificant findings after day seven. Many years later, Beilin et al. (195) studied the effects of ex vivo ketamine administration in the early postoperative period on peripheral blood mononuclear cells (PBMCs). The clinical trial groups either received 0.15 mg/kg of I.V. ketamine or isotonic saline prior to general anesthesia induction. The ketamine-group PBMCs exhibited significantly reduced concentrations of IL-6 4 h post-operatively when compared to the control. IL-2 concentrations appeared unchanged, while TFN-α was significantly elevated in the group 4 h post-operatively (195). However, ketamine-related effects on POCD outcomes have been conflicting from clinical trials performed during cardiac surgery (196, 197). In non-cardiac studies, pre-treatment of sub-anesthetic (0.15 mg/kg) esketamine before gastrointestinal surgery did not result in differences in POCD outcomes at 3-months compared to controls (198).
As reviewed by Zanos et al. (194), the anti-inflammatory characteristics of ketamine were unveiled to result in a reduction of serum NF-κB, C-reactive protein (CRP), iNOS, and IL-6. The anti-inflammatory characteristics of ketamine have also been studied within the context of microglia (42, 41). Specifically, ketamine administration has been shown to induce anti-inflammatory phenotypes in vitro and in vivo (41, 43–45). In one study, Verdonk et al. (199) revealed that ketamine administration to mice caused an upregulation of neuroprotective gene expression along with decreased microglial activation when exposed to pro-inflammatory LPS, as seen through a decrease in the microglial cell body area (199) (Table 1). Interestingly, these morphological changes were only seen in pre-frontal, not hippocampal regions. The mechanism of the anti-inflammatory effects on microglia was revealed to be through a downregulation of heme oxygenase-1 (HO-1) gene expression along with a complex interplay with quinolinic acid, tryptophan, and kynurenine metabolism.
Recent research on ketamine in the prevention of POCD has revealed an overall benefit of ketamine utilization on attenuation of negative effects of anesthesia. One randomized, placebo controlled, double-blind clinical trial sought to study the utilization of ketamine in preventing POCD and delirium in 182 adult patients (200). The researchers found that neither ketamine, haloperidol, or a combination of the two provided superior results over the placebo group in preventing POCD and delirium via measurements of serum cortisol, neuron specific enolase and S100beta (200). Another randomized controlled trial studied the neuroprotective effects of ketamine and dexmedetomidine in reducing POCD in 90 patients aged 65–85 years old undergoing cataract extraction surgeries. In comparison to the placebo group, ketamine and dexmedetomidine groups experienced a significant reduction in POCD development (p < 0.0001) via neuropsychological testing at 1 week and 3 months post-surgery (201).
The research does show some mixed results, however. One single-center randomized placebo-control trial studied the effect of the intraoperative infusion of S-ketamine in 120 patients aged 45–65 undergoing thoracoscopic surgery (202). The patients were split into three groups: patients receiving general anesthesia (C), patients receiving general anesthesia and ultrasound-guided paravertebral block (TP) and the patients receiving general anesthesia, ultrasound-guided paravertebral block, and perioperative s-ketamine. In comparison to the C and TS groups, researchers found that the TP group scored higher (p < 0.05) on a Mini-Mental State Examination (MMSE) at 1-day post-operation and that there was no significant difference in the three groups at 3 months post-operation. This led researchers to conclude that intraoperative ketamine did not significantly benefit neurocognitive function postoperatively (202). Lastly, one meta-analysis on intraoperative ketamine administration to prevent POCD reviewed six randomized controlled trials studying its beneficial effects (203). Only three trials showed any significant reduction of risk from ketamine administration, leading researchers to conclude that the quality of evidence for ketamine as an agent to prevent POCD (203).
Propofol
Propofol, a commonly used GABAA agonist, increases Cl− conduction, hyperpolarizes neurons, decreases cerebral metabolic rate, and has been shown to inhibit in vitro and in vivo microglial M1 polarization (36, 204). In 2023, Guan et al. discovered that propofol is neuroprotective and prevents hippocampal inflammation and microglial metabolic reprogramming through HIF-1α regulation and ROS/PI3K/Akt/mTOR/HIF-1α signaling septic mouse models. Moreover, Huang et al. (26, 205) found that prophylactic propofol before LPS administration reduced microglial M1 polarization. However, TNF-α remained elevated in the hippocampus during the experiments. As reviewed, the anti-inflammatory effects of propofol in microglia may be mediated through GSK-3β inactivation, NADPH oxidase, and NMDA receptor inhibition, and TLR4 downregulation (36–40). Moreover, propofol has been shown to inhibit microglia reprogramming and reduce LPS-induced expression of iNOS, NO, TNF-α, IL-1β, and COX-2 in microglia (36) (Table 1).
There are varying reports on the effects of propofol on POCD outcomes during cardiac and non-cardiac surgery, as described in Table 2 (197, 206–210). Evidence suggests that propofol administration might induce a lower incidence of POCD in comparison to other anesthetics and may offer some neuroprotective benefits during non-cardiac surgery (208, 209). Conversely, propofol used in combination with etomidate has been shown to lower POCD incidence while other studies have shown no difference in outcomes when propofol is compared to sevoflurane (210, 213, 217). During cardiac surgery, however, propofol was shown to be associated with a higher incidence rate of cognitive dysfunction when compared to desflurane and sevoflurane (206, 207).
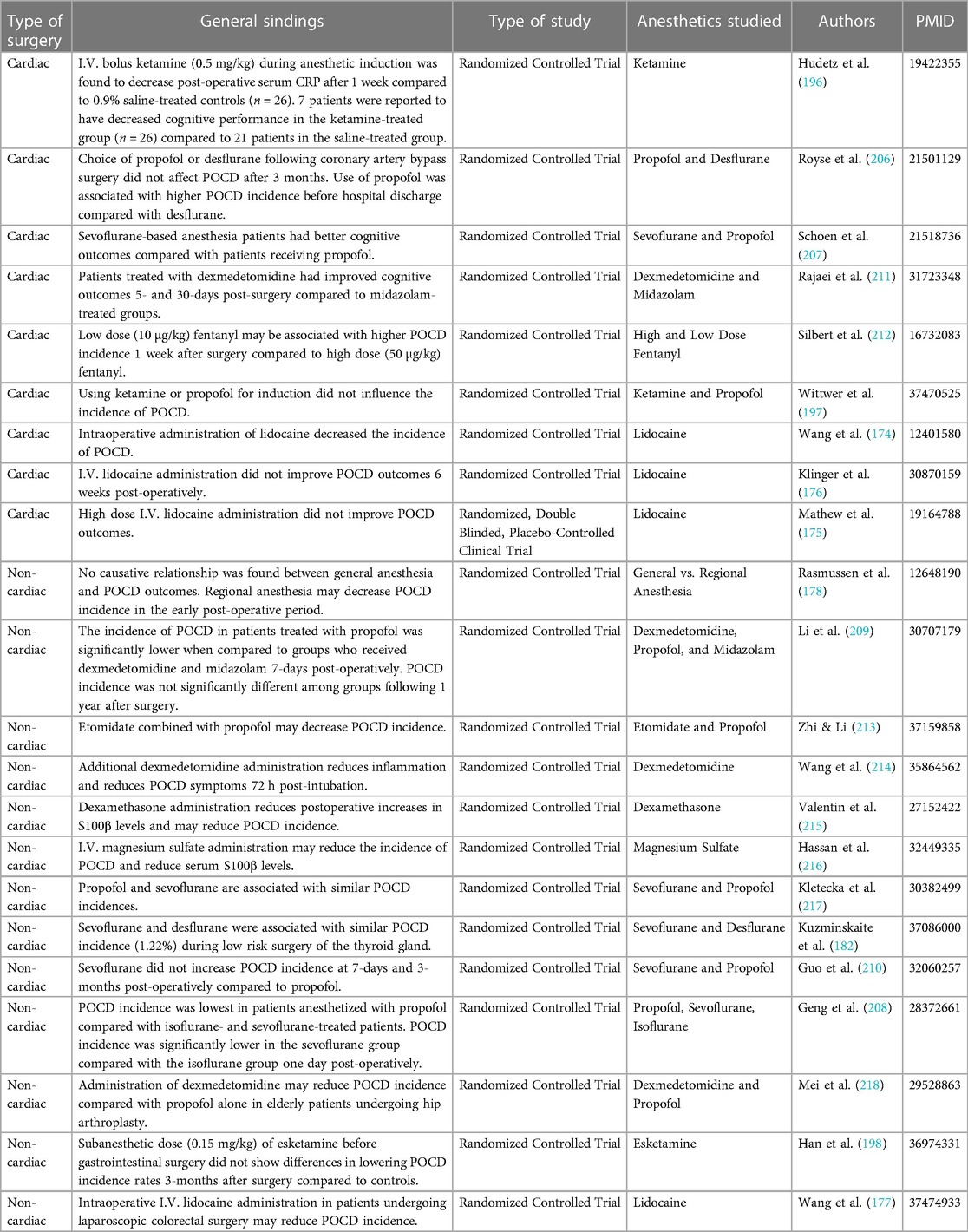
Table 2. Clinical POCD studies on the effects of various anesthetics during cardiac- and non-cardiac surgery.
One randomized controlled preliminary trial researched the effects of propofol, dexmedetomidine, and midazolam on POCD in elderly patients. The study of 164 patients aged 65 years or older undergoing hip or knee arthroplasty split the subjects into three groups given one of the three anesthetic agents with combined spinal-epidural anesthesia. Subjects were asked to complete neuropsychological tests before, 5 days after, and 1 year after surgery. The results showed that propofol had an advantage as compared to the other groups in terms of POCD incidence (209). Another double-blind, randomized control trial researched the incidence of POCD in patients given propofol, isoflurane or sevoflurane. This study of 150 patients aged 60 and older undergoing laparoscopic cholecystectomy found that the incidence of POCD was lower in the propofol group (208). One meta-analysis unveiled propofol as an optimal treatment option for lowering POCD incidence rates in non-cardiac surgery when compared to inhalational anesthetics (219). POCD in non-cardiac surgical patients given inhalational and propofol anesthetics. They reviewed 15 randomized control trials with 1,854 patients while examining MMSE scores, TNF-α levels, and IL-6 levels in patients post-surgery. The researchers found that propofol was superior to inhalational anesthesia at attenuating POCD (219). Lastly, one research group employed a meta-analysis to review 34 clinical trials in 4,314 patients to study the POCD incidence rates with a variety of anesthetic agents in a geriatric, noncardiac surgery cohort (220). Specifically, propofol, dexmedetomidine, sufentanil, and placebo POCD incidence rates were found to be 16.8%, 12.9%, 6.3%, and 27.7%, respectively (220). These rates appear starkly different when compared to sevoflurane (24.0%), desflurane (28.3%), and fentanyl (23.9%) (220). The researchers concluded that propofol, along with dexmedetomidine and sufentanil, attenuated incidence of POCD (220).
Opioids
Opioid administration may provide selective neuroprotection with anti-inflammatory effects on microglia (15, 221, 222) (Table 1). However, studies on the direct effects on glia have been inconsistent (223).
In 2000, Zhang & Xia revealed that opioid agonism at the δ-receptor, but not μ- and κ-opioid receptors, resulted in neuroprotection against models of neuronal excitotoxicity. Rat neocortical neurons were cultured, exposed to glutamate or co-administrations with glutamate and opioid agonists, and were assayed for markers of cellular injury using lactate dehydrogenase. Interestingly, the glutamate-mediated excitotoxicity appeared age-dependent, as eight-to-ten-day old neurons displayed markers of cell injury whereas the four-day neurons did not. Iwata et al. (222) corroborated these findings but found that δ-receptor agonism promoted survival in hippocampal CA3 and dentate gyrus (DG) neurons but not CA1 neurons.
In 2022, Mali & Novotny reviewed the efficacy of μ-, δ-, and κ-opioid receptor agonists on microglial polarization. The review indicated that opioid agonism may result in an M2 polarization in microglia in vitro, evidenced through inhibition of NO production in microglia exposed to pro-inflammatory lipopolysaccharide (LPS). The authors highlighted the work of Merighi et al. (13) focused on the mechanism of opioid-mediated M1 polarization (13). The study revealed that the phenotype change is mediated through TLR4/NF-κB inhibition and TREM2 activation. Addition of the opioid agonists also contributed to morphological changes such as lengthening of microglial processes, indicative of a “resting” phenotype (15). Within the study of hyperalgesia, it was revealed that morphine activated μ-receptors in spinal cord-derived microglia which resulted in BDNF/P2X4 disinhibition (223, 224). Moreover, cell culture models of dose-dependent morphine exposure (0.1 nM–1 µM) resulted in anti-inflammatory phenotypes such as a decrease in microglia chemotaxis (14) (Table 1).
However, it should be noted that studies of chronic opioid administration resulted in the opposite effects (225–227). M1 microglia exposed to opioid agonists after LPS treatment were shown to enhance the release of pro-inflammatory NO, IL-6, TNF-α, and IL-1β via activation of the Akt pathway (13). Specifically, the morphine was mediating the phosphorylation and translocation of PKCε isoforms which regulated Akt and ERK1/2 phosphorylation. However, the experimental groups did not include an “opioid-only” administered group and only included no drugs, LPS, and LPS co-administered with morphine. The studies demonstrating both neuroprotection and inflammation in microglia may be dose dependent, but nonetheless shed light on models of opioid administration to already-inflamed microglia.
Basic science investigations into the effects of fentanyl revealed pro-inflammatory physiological consequences in vitro and in vivo (10–12). Pro-inflammatory IL-1β, TNF-α, and IL-6 were found to be upregulated in spinal cord of rats following surgery and 60 μg/kg fentanyl administration (10). Higher doses of fentanyl (0.1 mg/kg) along with 10 mg/kg morphine administration were found to cause pro-inflammatory phenotype changes resulting in NLRP3 inflammasome formation in dorsal raphe nucleus glia and neurons in rats (11). Cell culture models in U937 cells revealed that morphine, but not fentanyl, inhibited TNF-α release following LPS incubation (12) (Table 1).
Clinical investigations into fentanyl have shed light on its use and associations with POCD. One randomized controlled trial researched the effect of high and low dose fentanyl anesthesia on POCD in the elderly (212). The study of 350 patients aged 55 and older undergoing coronary artery bypass graft surgery were randomized into either a low dose fentanyl group (10 µg/kg) or a high dose fentanyl group (50 µg/kg). Each group completed a group of 8 neuropsychological tests during the week before surgery, 1 week after surgery, 3 months after surgery and 12 months after. The researchers concluded that there was no evidence of an association between high-dose fentanyl and POCD at 3 or 12 months after surgery. However, low dose fentanyl was associated with increased incidence of POCD at 1 week after surgery. One meta-analysis investigated anesthetic drugs and their ability to prevent POCD in elderly patients (220). This study involved a total of 34 trials with over 4,314 patients undergoing noncardiac surgery. The researchers found that fentanyl significantly increased the incidence of POCD when compared to sufentanil, however it showed non-significant ability to reduce the incidence of POCD when compared with a placebo group (220).
Moreover, there appears to be a difference in opioid-related POCD outcomes; these observed incidences may be due, in part, to opioid dosing and dose scheduling in acute and chronic settings. The difference in observed outcomes may also be due to the difference in binding affinities for the various opioid receptors.
Paralytics
Succinylcholine, vecuronium, rocuronium, and cisatracurium are commonly used paralytics during surgery. However, neuromuscular paralytic agents normally do not cross the BBB—except in certain circumstances (228, 229). In 1977, Matteo et al. found d-tubocurarine in the CSF of patients undergoing craniotomy for arteriovenous malformations, pituitary tumors, and cerebral aneurysms post-IV administration of the neuromuscular blocker (229, 230). Similar findings were reported, where atracurium and its metabolite, laudanosine, were found in the CSF of patients undergoing intracranial surgery (229, 231). As reviewed, neuromuscular blockers may cross the BBB in a dose-dependent fashion in critically ill patients. Neurotoxic effects may ensue if the neuromuscular blockers cross the BBB; the resulting effects involve excitotoxicity through an increase in intracellular calcium (229, 232, 233).
Benzodiazepines
Benzodiazepines are GABA-ergic compounds used as sedative agents used that can also reduce myoclonic movements and anxiety while promoting amnesia (204, 234). Midazolam is preferred perioperatively with later use of diazepam and lorazepam (204). Chronic models of diazepam treatment in mice have been shown to target mitochondrial 18 kDa translocator protein (TSPO) in microglia which results in the clearance of synaptic material and dendritic spines and by extension, contributes to cognitive dysfunction (20). However, in more acute models, midazolam has been shown to have anti-inflammatory effects on microglia exposed to LPS (235) (Table 1).
Through interactions at TPSO receptors, midazolam treatment has been shown to reduce LPS-induced NO and TNF-α release from rat microglia (16). Midazolam has also been shown to reduce IL1-B-induced IL-6 release in rat C6 glioma cells through the suppression of STAT3 activation and inhibit IL-1β and IL-18 release in BV-2 mouse microglial cells through the inactivation of the NLRP3 inflammasome (17, 18) (Table 1). Human data regarding the association of midazolam with POCD provides mixed results. In one randomized control trial by Li et al., the researchers found that midazolam impaired subjects' ability to complete a battery of neuropsychological testing compared to a control group at one-week and one-year post-surgery (210). Another randomized control trial by Mansouri et al. found that midazolam improved subjects' scores on an MMSE as compared to a control group undergoing general anesthetic at one-week post-surgery (236). The limitation of this study is that researchers only examined the short-term effects of cognitive function, hence more data is required to know if the reduction in POCD is long-lasting. One meta-analysis reviewed 34 studies involving elderly patients undergoing noncardiac surgery with varying anesthetics and found that midazolam conferred no significant benefit over placebo groups in reducing the incidence of POCD (220).
Diazepam is another benzodiazepine that is also utilized for its sedative and hypnotic properties for the induction of anesthesia. Diazepam acts at TSPO receptors similarly to midazolam in that it reduces the release of proinflammatory cytokines, NO and CD40 cell expression when exposed to inflammatory stimuli such as LPS (19). The exact mechanism of diazepam's reduction of neuroinflammation is unknown, however Fernandez et al. cites an increased production of glucocorticoids from the adrenal cortex, increased synthesis of neurosteroids from microglia and direct actions on peripheral immune cells as possibilities to be explored. Interestingly, studies in mouse models found that acute diazepam administration had no impact on the number and morphology of microglia or the density and formation of dendritic spines. Shi et al. also found that chronic models of diazepam treatment actually impaired the clearance of synaptic material and the structural plasticity of dendritic spines, which may contribute to impairments in spatial memory and long-term potentiation (20, 237) (Table 1). From this data, it seems plausible that the use of diazepam in surgical procedures would not be sustained enough to elicit substantial negative effects on cognition. Regardless, there is a lack of clinical human data regarding the effect of diazepam on POCD. One study conducted by Rasmussen et al., found that neither diazepam nor its metabolites were responsible for any deficits in cognitive functioning at one-week post-surgery (238). This study may be limited, however, due to a small sample size (n = 35).
Ketorolac
Ketorolac, a non-steroidal anti-inflammatory drug (NSAID), elicits a response of analgesia through inhibiting both cyclooxygenases 1 & 2 (COX1/2) and by extension, inhibition of prostaglandin, prostacyclin, and thromboxane synthesis (239, 240). While studies are limited, Shen et al. (21) showed that ketorolac treatment can prevent sevoflurane-induced cognitive dysfunction in young mouse models of anesthesia-mediated cognitive dysfunction (21) (Table 1).
Dexmedetomidine
Dexmedetomidine is a selective α2A adrenergic receptor agonist utilized as an anesthetic adjuvant for the induction and maintenance of anesthesia (241). Its sedative-hypnotic effects are believed to be mediated by pre- and post-synaptic α2A receptor activity in the locus coeruleus (242).
Cell culture and animal models of dexmedetomidine administration revealed anti-inflammatory phenotype effects (22–25, 243). Experiments utilizing in vitro BV-2 microglia revealed that dexmedetomidine incubation resulted in an increase in M2 polarization mediated through an increase in Akt phosphorylation (22). Another identified mechanism of anti-inflammatory microglial polarization has been through an increase in Nrf2/HO-1 signaling resulting in inhibition of NLRP3 inflammasome activation (23). When treating mice exposed to sevoflurane, 25 μg/kg dexmedetomidine administration was found to attenuate CEBPB-mediated microglial inflammatory phenotypes (24). In a similar study, mice treated with 40 ml/kg dexmedetomidine (3.3 μg/ml) prior to LPS injection resulted in a decrease in microglial activation and in oxidative stress markers when compared to 10 ml/kg of propofol (3 mg/ml) (26). Combined in vivo spinal cord injury and in vitro models of dexmedetomidine exposure showed an increase in microglial anti-inflammatory phenotypes following treatment (25) (Table 1).
The current research suggests the dexmedetomidine may attenuate perioperative neuroinflammation and immunosuppression, thereby potentially reducing the incidence of POCD. In 2019, Wang et al. performed a meta-analysis of 67 studies, which demonstrated that dexmedetomidine is associated with a reduced secretion of epinephrine, norepinephrine, cortisol, and blood glucose via suppression of the hypothalamic-pituitary-adrenal axis. The study also highlighted a significant decrease in the concentration of pro-inflammatory cytokines such as IL-6, TNF-α, CRP, IL-b and Il-8, which may be attributed to direct modification of cytokine production from macrophages and monocytes (241). Lastly, dexmedetomidine attenuated immunosuppression following surgery through increased activity of natural killer cells and a higher ratio of CD4 to CD8 T cells and Th1 to Th2. During cardiac surgery, dexmedetomidine was associated with improved POCD outcomes when compared with midazolam-only groups (211). Similar outcomes were observed in the context of non-cardiac surgery (214). Dexmedetomidine has also been shown to be associated with lower POCD incidence rates compared with elderly patients treated with propofol during hip arthroplasty (218). Zeng et al. performed a similar meta-analysis of dexmedetomidine in 2023, which also included data on MMSE testing of post-operative patients to evaluate cognitive functioning (220). The researchers found that there was a significant reduction in POCD occurrence at 12 h, 24 h and 72 h post-surgery (244). Another meta-analysis focused solely on cognitive function, which was evaluated through MMSE testing of post-operative elderly patients, found that dexmedetomidine had a preventative effect on the incidence of POCD (245). While these studies are promising, the evaluation of dexmedetomidine on POCD and neuroinflammation is generally limited to the immediate post-surgical period; therefore more research needs to be done to elicit if these effects are more long-lasting.
Targeting the neuro-immune axis
Several groups have examined pharmacotherapies for POCD in basic science models. Peng et al. (246) utilized metformin as a potential treatment option for isoflurane-induced cognitive dysfunction in vivo (246). The research group utilized a sub-minimal alveolar concentration (MAC) of 1.3% isoflurane to induce cognitive dysfunction in murine models (246). Intraperitoneal injections of metformin resulted in inhibition of M1 polarization in both hippocampal microglia and A1-like astrocytes and subsequent alleviation of cognitive dysfunction (246).
Cibelli et al. probed treatment of experimental POCD using minocycline, an antibiotic with anti-inflammatory properties (247, 248). The group demonstrated that intraperitoneal minocycline injections (40 mg/kg) prevented surgery- and anesthesia-induced behavioral impairment, ameboid morphological changes in microglia, and upregulation of hippocampal IL-1β in mouse models (248).
Celecoxib, a COX-2 inhibitor, has been shown to decrease early incidence of POCD in a geriatric cohort (249). Compared to the control group, patients receiving celecoxib also had decreased plasma levels of COX-2, IL-1β, IL-6, TNF-α, and S100β (249). Wang et al. also demonstrated that inhibiting COX-2 using parecoxib resulted in diminished hippocampal IL-1β, IL-6, TNF-α, and PGE-2 in rodent models (250). A meta-analysis on parecoxib efficacy revealed that treatment with the COX2 inhibitor resulted in lower levels of IL-6 and S100β within 2 days of surgery (205). Kamer et al. (251) studied another COX2 inhibitor, meloxicam, in a mouse model of surgery-induced neuroinflammation. This group found that 60 mg/kg i.p. administration of meloxicam 24 h post-splenectomy using 2.5% and 3.0% isoflurane in rats resulted in decreased glial activation and improved object recognition compared to controls (251). While studying the effect of steroids and general anesthesia on POCD and S100β, Valentin et al. (215) revealed that a single low dose of dexamethasone before noncardiac/non-neurological surgery can attenuate POCD in elderly patients (215). A similar study showed similar results using magnesium sulfate in tandem with general anesthesia in patients undergoing elective laparoscopic cholecystectomy (216).
NDMA receptor antagonism
Overactivation of NDMARs can contribute to POCD through dendritic spine loss and apoptosis mediated through activated calpain, a calcium dependent protease, and dysregulated BDNF/TrkB signaling (252). Memantine and MDL-28170, an NDMAr antagonist and calcium-dependent protease inhibitor, respectively, have also been shown to decrease elements of postoperative cognitive dysfunction in murine models (252). Qiu et al. administered intraperitoneal injections of 20 mg/kg of memantine or MDL-28170 to mice prior to isoflurane exposure and post-exposure daily for 5 days (252). Treatment with memantine attenuated isoflurane and surgery-induced microglial and astrocyte inflammation in the hippocampus (252). IL-1β and IL-6 were also decreased in 1- and 8-days post-surgery analysis of hippocampal proinflammatory cytokines following memantine treatment (252). Dendritic spine loss in the CA1 region was also attenuated following the memantine and MDL-28170 administration (252). Surgery- and anesthesia-induced cognitive impairments and cell apoptosis were further attenuated by administration of either the NDMAR or calpain inhibitor (252).
Calcium channel antagonism
Wei et al. (185) utilized dantrolene, a ryanodine receptor antagonist, to suppress isoflurane-mediated cytotoxicity in vitro (185). In this experimental model, isoflurane was shown to deplete calcium from the muscle sarcoplasmic reticulum (185). Similarly, isoflurane was shown to induce calcium release from the endoplasmic reticulum (ER) in hippocampal and cerebrocortical neurons (184). However, cytotoxicity and apoptosis were significantly decreased when cortical neuronal cultures were pretreated with 30 µM dantrolene before 24 h exposure to 2.4% isoflurane (185).
Nimodipine, a second generation 1,4-dihydropyridine calcium channel blocker, has also been shown to have therapeutic effects in rodent models of POCD (253–255). Nimodipine administration partially restored abhorrent expression of IL-6, IL-8, caspase-3, and TNF-α when administered with sevoflurane (253).
α2-Adrenergic receptor agonism
Dexmedetomidine has been shown to have neuroprotective effects in models of sevoflurane-induced cognitive decline through targeting CCAAT/enhancer binding protein beta (CEPBPB) and activating the c-Jun N-terminal kinase (JNK)/p-38 (24). Through JNK/p-38 activation, dexmedetomidine suppresses M1 microglial polarization and reverses neuronal injury in the setting of sevoflurane-exposure (24).
Dexmedetomidine has also demonstrated anti-inflammatory properties in microglial polarization and in patients with resections of gastric cancer (22, 23, 25, 256). In a randomized control study, dexmedetomidine lowered serum levels of IL-6, IL-8, IL-10, TNF-α, and C-reactive protein (CRP) in patients undergoing intestinal surgery (77). These intestinal surgery patients also had improved cognitive recovery and an improved stress response following intravenous dexmedetomidine administration (77). Interestingly, dexmedetomidine has also been shown to serve as a potential “glympathic enhancer” by increasing clearance of rodent intraparenchymal glymphatic tracers (257). This is of emerging importance because reduced sleep quality hinders CSF flow, which can lead to neurodegenerative changes through a reduction in clearing metabolic waste such as lactate and amyloid-β (257). It may be possible that dexmedetomidine may have a therapeutic role by enhancing CSF flow and glymphatic clearance following surgery and anesthesia—especially given the evidence of isoflurane impairing glymphatic flow (257, 258). The lack of GABAergic or cholinergic property are particularly beneficial since this may carry an increased risk of developing delirium (242, 257).
Conclusions and future directions
While the definition of POCD appears to vary, it may be defined as an iatrogenic post-operative neuro-inflammatory state affecting primarily older individuals. The diverse pharmacological arsenal available in the operating theater especially requires attention to agents with potential pro-inflammatory effects. The role of polypharmacy of anesthetic cocktails should also be investigated further. While polypharmacy experimental investigations may be complex, in vitro experiments may provide insight into the complex drug-drug interactions of cell-cell interactions, morphologies, and gene expression patterns. The synergistic effects on known inflammatory and anti-inflammatory pathways should be assessed with a focus on a drug's binding affinity if common drug targets are shared. In all, it appears there may be pro-inflammatory consequences from inhalational anesthetics while agents such as ketamine, opioids, propofol, ketorolac, and benzodiazepines may confer protection against inflammatory phenotypes within the CNS. Experiments regarding prophylaxis and post-operative pharmacotherapy should be explored further within the context of cardiac and non-cardiac surgery.
Author contributions
GS: Conceptualization, Investigation, Writing – original draft, Writing – review & editing. NS: Conceptualization, Investigation, Writing – original draft, Writing – review & editing. TC: Conceptualization, Investigation, Writing – original draft, Writing – review & editing. BA: Conceptualization, Investigation, Writing – original draft, Writing – review & editing. JG: Supervision, Writing – review & editing.
Funding
The author(s) declare financial support was received for the research, authorship, and/or publication of this article.
This study was funded by the Touro College of Osteopathic Medicine Harlem Campus Department of Research.
Conflict of interest
The authors declare that the research was conducted in the absence of any commercial or financial relationships that could be construed as a potential conflict of interest.
Publisher's note
All claims expressed in this article are solely those of the authors and do not necessarily represent those of their affiliated organizations, or those of the publisher, the editors and the reviewers. Any product that may be evaluated in this article, or claim that may be made by its manufacturer, is not guaranteed or endorsed by the publisher.
References
1. Kannan S, Saadani-Makki F, Muzik O, Chakraborty P, Mangner TJ, Janisse J, et al. Microglial activation in perinatal rabbit brain induced by intrauterine inflammation: detection with 11C-(R)-PK11195 and small-animal PET. J Nucl Med. (2007) 48:946–54. doi: 10.2967/jnumed.106.038539
2. Safavynia SA, Goldstein PA. The role of neuroinflammation in postoperative cognitive dysfunction: moving from hypothesis to treatment. Front Psychiatry. (2018) 9:752. doi: 10.3389/fpsyt.2018.00752
3. Li Z, Zhu Y, Kang Y, Qin S, Chai J. Neuroinflammation as the underlying mechanism of postoperative cognitive dysfunction and therapeutic strategies. Front Cell Neurosci. (2022) 16:843069. doi: 10.3389/fncel.2022.843069
4. Rundshagen I. Postoperative cognitive dysfunction. Dtsch Arztebl Int. (2014) 111(8):119–25. doi: 10.3238/arztebl.2014.0119
5. Subramaniyan S, Terrando N. Neuroinflammation and perioperative neurocognitive disorders. Anesth Analg. (2019) 128:781–8. doi: 10.1213/ANE.0000000000004053
6. Prinz M, Priller J. Microglia and brain macrophages in the molecular age: from origin to neuropsychiatric disease. Nat Rev Neurosci. (2014) 15:300–12. doi: 10.1038/nrn3722
7. Kokkosis AG, Tsirka SE. Neuroimmune mechanisms and sex/gender-dependent effects in the pathophysiology of mental disorders. J Pharmacol Exp Ther. (2020) 375:175–92. doi: 10.1124/jpet.120.266163
8. Blank T, Prinz M. Microglia as modulators of cognition and neuropsychiatric disorders. Glia. (2013) 61:62–70. doi: 10.1002/glia.22372
9. Paolicelli RC, Sierra A, Stevens B, Tremblay ME, Aguzzi A, Ajami B, et al. Microglia states and nomenclature: a field at its crossroads. Neuron. (2022) 110:3458–83. doi: 10.1016/j.neuron.2022.10.020
10. Chang L, Ye F, Luo Q, Tao Y, Shu H. Increased hyperalgesia and proinflammatory cytokines in the spinal cord and dorsal root ganglion after surgery and/or fentanyl administration in rats. Anesth Analg. (2018) 126:289–97. doi: 10.1213/ANE.0000000000002601
11. Carranza-Aguilar CJ, Hernandez-Mendoza A, Mejias-Aponte C, Rice KC, Morales M, Gonzalez-Espinosa C, et al. Morphine and fentanyl repeated administration induces different levels of NLRP3-dependent pyroptosis in the dorsal raphe nucleus of male rats via cell-specific activation of TLR4 and opioid receptors. Cell Mol Neurobiol. (2022) 42:677–94. doi: 10.1007/s10571-020-00957-5
12. Bastami S, Norling C, Trinks C, Holmlund B, Walz TM, Ahlner J, et al. Inhibitory effect of opiates on LPS mediated release of TNF and IL-8. Acta Oncol. (2013) 52:1022–33. doi: 10.3109/0284186X.2012.737932
13. Merighi S, Gessi S, Varani K, Fazzi D, Stefanelli A, Borea PA. Morphine mediates a proinflammatory phenotype via mu-opioid receptor-PKCvarepsilon-Akt-ERK1/2 signaling pathway in activated microglial cells. Biochem Pharmacol. (2013) 86:487–96. doi: 10.1016/j.bcp.2013.05.027
14. Hu S, Chao CC, Hegg CC, Thayer S, Peterson PK. Morphine inhibits human microglial cell production of, and migration towards, RANTES. J Psychopharmacol. (2000) 14:238–43. doi: 10.1177/026988110001400307
15. Mali AS, Novotny J. Opioid receptor activation suppresses the neuroinflammatory response by promoting microglial M2 polarization. Mol Cell Neurosci. (2022) 121:103744. doi: 10.1016/j.mcn.2022.103744
16. Wilms H, Claasen J, Rohl C, Sievers J, Deuschl G, Lucius R. Involvement of benzodiazepine receptors in neuroinflammatory and neurodegenerative diseases: evidence from activated microglial cells in vitro. Neurobiol Dis. (2003) 14:417–24. doi: 10.1016/j.nbd.2003.07.002
17. Tanabe K, Kozawa O, Iida H. Midazolam suppresses interleukin-1beta-induced interleukin-6 release from rat glial cells. J Neuroinflammation. (2011) 8:68. doi: 10.1186/1742-2094-8-68
18. Feng H, Liu Y, Zhang R, Liang Y, Sun L, Lan N, et al. TSPO ligands PK11195 and midazolam reduce NLRP3 inflammasome activation and proinflammatory cytokine release in BV-2 cells. Front Cell Neurosci. (2020) 14:544431. doi: 10.3389/fncel.2020.544431
19. Fernandez Hurst N, Zanetti SR, Baez NS, Bibolini MJ, Bouzat C, Roth GA. Diazepam treatment reduces inflammatory cells and mediators in the central nervous system of rats with experimental autoimmune encephalomyelitis. J Neuroimmunol. (2017) 313:145–51. doi: 10.1016/j.jneuroim.2017.09.012
20. Shi Y, Cui M, Ochs K, Brendel M, Strubing FL, Briel N, et al. Long-term diazepam treatment enhances microglial spine engulfment and impairs cognitive performance via the mitochondrial 18 kDa translocator protein (TSPO). Nat Neurosci. (2022) 25:317–29. doi: 10.1038/s41593-022-01013-9
21. Shen X, Dong Y, Xu Z, Wang H, Miao C, Soriano SG, et al. Selective anesthesia-induced neuroinflammation in developing mouse brain and cognitive impairment. Anesthesiology. (2013) 118:502–15. doi: 10.1097/ALN.0b013e3182834d77
22. Sun Z, Lin Y, Li Y, Ren T, Du G, Wang J, et al. The effect of dexmedetomidine on inflammatory inhibition and microglial polarization in BV-2 cells. Neurol Res. (2018) 40:838–46. doi: 10.1080/01616412.2018.1493849
23. Wang N, Nie H, Zhang Y, Han H, Wang S, Liu W, et al. Dexmedetomidine exerts cerebral protective effects against cerebral ischemic injury by promoting the polarization of M2 microglia via the Nrf2/HO-1/NLRP3 pathway. Inflamm Res. (2022) 71:93–106. doi: 10.1007/s00011-021-01515-5
24. Fu S, Zhao X, Li Y, Fan X, Huang Z. Dexmedetomidine alleviates hippocampal neuronal loss and cognitive decline in rats undergoing open surgery under sevoflurane anaesthesia by suppressing CCAAT/enhancer-binding protein beta. Eur J Neurosci. (2023) 59:36–53. doi: 10.1111/ejn.16193
25. Gao J, Sun Z, Xiao Z, Du Q, Niu X, Wang G, et al. Dexmedetomidine modulates neuroinflammation and improves outcome via alpha2-adrenergic receptor signaling after rat spinal cord injury. Br J Anaesth. (2019) 123:827–38. doi: 10.1016/j.bja.2019.08.026
26. Huang C, Ng OT, Chu JM, Irwin MG, Hu X, Zhu S, et al. Differential effects of propofol and dexmedetomidine on neuroinflammation induced by systemic endotoxin lipopolysaccharides in adult mice. Neurosci Lett. (2019) 707:134309. doi: 10.1016/j.neulet.2019.134309
27. Xu Z, Yao X, Zhao Y, Yao B. C/EBPalpha involvement in microglial polarization via HDAC1/STAT3 pathway aggravated sevoflurane-induced cognitive impairment in aged rats. PeerJ. (2023) 11:e15466. doi: 10.7717/peerj.15466
28. Xu LL, Xie JQ, Shen JJ, Ying MD, Chen XZ. Neuron-derived exosomes mediate sevoflurane-induced neurotoxicity in neonatal mice via transferring lncRNA Gas5 and promoting M1 polarization of microglia. Acta Pharmacol Sin. (2023) 45:298–311. doi: 10.1038/s41401-023-01173-9
29. Xu F, Han L, Wang Y, Deng D, Ding Y, Zhao S, et al. Prolonged anesthesia induces neuroinflammation and complement-mediated microglial synaptic elimination involved in neurocognitive dysfunction and anxiety-like behaviors. BMC Med. (2023) 21:7. doi: 10.1186/s12916-022-02705-6
30. Pei Z, Wang S, Li Q. Sevoflurane suppresses microglial M2 polarization. Neurosci Lett. (2017) 655:160–5. doi: 10.1016/j.neulet.2017.07.001
31. Zhang L, Zhang J, Yang L, Dong Y, Zhang Y, Xie Z. Isoflurane and sevoflurane increase interleukin-6 levels through the nuclear factor-kappa B pathway in neuroglioma cells. Br J Anaesth. (2013) 110(Suppl 1):i82–91. doi: 10.1093/bja/aet115
32. Wang Z, Meng S, Cao L, Chen Y, Zuo Z, Peng S. Critical role of NLRP3-caspase-1 pathway in age-dependent isoflurane-induced microglial inflammatory response and cognitive impairment. J Neuroinflammation. (2018) 15:109. doi: 10.1186/s12974-018-1137-1
33. Wu X, Lu Y, Dong Y, Zhang G, Zhang Y, Xu Z, et al. The inhalation anesthetic isoflurane increases levels of proinflammatory TNF-alpha, IL-6, and IL-1beta. Neurobiol Aging. (2012) 33:1364–78. doi: 10.1016/j.neurobiolaging.2010.11.002
34. Jiang T, Xu S, Shen Y, Xu Y, Li Y. Genistein attenuates isoflurane-induced neuroinflammation by inhibiting TLR4-mediated microglial-polarization in vivo and in vitro. J Inflamm Res. (2021) 14:2587–600. doi: 10.2147/JIR.S304336
35. Li QF, Zhu YS, Jiang H. Isoflurane preconditioning activates HIF-1alpha, iNOS and Erk1/2 and protects against oxygen-glucose deprivation neuronal injury. Brain Res. (2008) 1245:26–35. doi: 10.1016/j.brainres.2008.09.069
36. Guan S, Sun L, Wang X, Huang X, Luo T. Propofol inhibits neuroinflammation and metabolic reprogramming in microglia in vitro and in vivo. Front Pharmacol. (2023) 14:1161810. doi: 10.3389/fphar.2023.1161810
37. Wu Q, Zhao Y, Chen X, Zhu M, Miao C. Propofol attenuates BV2 microglia inflammation via NMDA receptor inhibition. Can J Physiol Pharmacol. (2018) 96:241–8. doi: 10.1139/cjpp-2017-0243
38. Gui B, Su M, Chen J, Jin L, Wan R, Qian Y. Neuroprotective effects of pretreatment with propofol in LPS-induced BV-2 microglia cells: role of TLR4 and GSK-3beta. Inflammation. (2012) 35:1632–40. doi: 10.1007/s10753-012-9478-x
39. Luo T, Wu J, Kabadi SV, Sabirzhanov B, Guanciale K, Hanscom M, et al. Propofol limits microglial activation after experimental brain trauma through inhibition of nicotinamide adenine dinucleotide phosphate oxidase. Anesthesiology. (2013) 119:1370–88. doi: 10.1097/ALN.0000000000000020
40. Qin X, Sun ZQ, Zhang XW, Dai XJ, Mao SS, Zhang YM. TLR4 Signaling is involved in the protective effect of propofol in BV2 microglia against OGD/reoxygenation. J Physiol Biochem. (2013) 69:707–18. doi: 10.1007/s13105-013-0247-6
41. Wu M, Zhao L, Wang Y, Guo Q, An Q, Geng J, et al. Ketamine regulates the autophagy flux and polarization of microglia through the HMGB1-RAGE axis and exerts antidepressant effects in mice. J Neuropathol Exp Neurol. (2022) 81:931–42. doi: 10.1093/jnen/nlac035
42. Zhang N, Yao L, Wang P, Liu Z. Immunoregulation and antidepressant effect of ketamine. Transl Neurosci. (2021) 12:218–36. doi: 10.1515/tnsci-2020-0167
43. Wu GJ, Chen TL, Ueng YF, Chen RM. Ketamine inhibits tumor necrosis factor-alpha and interleukin-6 gene expressions in lipopolysaccharide-stimulated macrophages through suppression of toll-like receptor 4-mediated c-Jun N-terminal kinase phosphorylation and activator protein-1 activation. Toxicol Appl Pharmacol. (2008) 228:105–13. doi: 10.1016/j.taap.2007.11.027
44. Chen TL, Chang CC, Lin YL, Ueng YF, Chen RM. Signal-transducing mechanisms of ketamine-caused inhibition of interleukin-1 beta gene expression in lipopolysaccharide-stimulated murine macrophage-like raw 264.7 cells. Toxicol Appl Pharmacol. (2009) 240:15–25. doi: 10.1016/j.taap.2009.06.013
45. Cavalleri L, Merlo Pich E, Millan MJ, Chiamulera C, Kunath T, Spano PF, et al. Ketamine enhances structural plasticity in mouse mesencephalic and human iPSC-derived dopaminergic neurons via AMPAR-driven BDNF and mTOR signaling. Mol Psychiatry. (2018) 23:812–23. doi: 10.1038/mp.2017.241
46. Zhu SH, Ji MH, Gao DP, Li WY, Yang JJ. Association between perioperative blood transfusion and early postoperative cognitive dysfunction in aged patients following total hip replacement surgery. Ups J Med Sci. (2014) 119:262–7. doi: 10.3109/03009734.2013.873502
47. Travica N, Lotfaliany M, Marriott A, Safavynia SA, Lane MM, Gray L, et al. Peri-operative risk factors associated with post-operative cognitive dysfunction (POCD): an Umbrella review of meta-analyses of observational studies. J Clin Med. (2023) 12. doi: 10.3390/jcm12041610
48. Krenk L, Rasmussen LS, Kehlet H. New insights into the pathophysiology of postoperative cognitive dysfunction. Acta Anaesthesiol Scand. (2010) 54:951–6. doi: 10.1111/j.1399-6576.2010.02268.x
49. Newman MF, Kirchner JL, Phillips-Bute B, Gaver V, Grocott H, Jones RH, et al., for the Neurological Outcome Research Group and the Cardiothoracic Anesthesiology Research Endeavors Investigators. Longitudinal assessment of neurocognitive function after coronary-artery bypass surgery. N Engl J Med. (2001) 344:395–402. doi: 10.1056/NEJM200102083440601
50. American Psychiatric Association, and American Psychiatric Association. Dsm-5 task force. In: Diagnostic and Statistical Manual of Mental Disorders: DSM-5. Washington, DC: American Psychiatric Association (2013).
51. Kotekar N, Kuruvilla CS, Murthy V. Post-operative cognitive dysfunction in the elderly: a prospective clinical study. Indian J Anaesth. (2014) 58:263–8. doi: 10.4103/0019-5049.135034
52. Monk TG. Predictors of cognitive dysfunction after major noncardiac surgery. Anesthesiology. (2008) 108(1):18–30. doi: 10.1097/01.anes.0000296071.19434.1e
53. Deiner S, Silverstein JH. Postoperative delirium and cognitive dysfunction. Br J Anaesth. (2009) 103(Suppl 1):i41–46. doi: 10.1093/bja/aep291
54. Moller JT, Cluitmans P, Rasmussen LS, Houx P, Rasmussen H, Canet J, et al. Long-term postoperative cognitive dysfunction in the elderly ISPOCD1 study. ISPOCD investigators. International study of post-operative cognitive dysfunction. Lancet. (1998) 351:857–61. doi: 10.1016/S0140-6736(97)07382-0
55. Gruber-Baldini AL, Zimmerman S, Morrison RS, Grattan LM, Hebel JR, Dolan MM, et al. Cognitive impairment in hip fracture patients: timing of detection and longitudinal follow-up. J Am Geriatr Soc. (2003) 51:1227–36. doi: 10.1046/j.1532-5415.2003.51406.x
56. Monk TG, Price CC. Postoperative cognitive disorders. Curr Opin Crit Care. (2011) 17:376–81. doi: 10.1097/MCC.0b013e328348bece
57. Silbert B, Evered L, Scott DA, Mcmahon S, Choong P, Ames D, et al. Preexisting cognitive impairment is associated with postoperative cognitive dysfunction after hip joint replacement surgery. Anesthesiology. (2015) 122:1224–34. doi: 10.1097/ALN.0000000000000671
58. Chen L, Au E, Saripella A, Kapoor P, Yan E, Wong J, et al. Postoperative outcomes in older surgical patients with preoperative cognitive impairment: a systematic review and meta-analysis. J Clin Anesth. (2022) 80:110883. doi: 10.1016/j.jclinane.2022.110883
59. Au E, Thangathurai G, Saripella A, Yan E, Englesakis M, Nagappa M, et al. Postoperative outcomes in elderly patients undergoing cardiac surgery with preoperative cognitive impairment: a systematic review and meta-analysis. Anesth Analg. (2023) 136:1016–28. doi: 10.1213/ANE.0000000000006346
60. Needham MJ, Webb CE, Bryden DC. Postoperative cognitive dysfunction and dementia: what we need to know and do. Br J Anaesth. (2017) 119:i115–25. doi: 10.1093/bja/aex354
61. Bhamidipati D, Goldhammer JE, Sperling MR, Torjman MC, Mccarey MM, Whellan DJ. Cognitive outcomes after coronary artery bypass grafting. J Cardiothorac Vasc Anesth. (2017) 31:707–18. doi: 10.1053/j.jvca.2016.09.028
62. Bhushan S, Li Y, Huang X, Cheng H, Gao K, Xiao Z. Progress of research in postoperative cognitive dysfunction in cardiac surgery patients: a review article. Int J Surg. (2021) 95:106163. doi: 10.1016/j.ijsu.2021.106163
63. Culley DJ, Baxter MG, Yukhananov R, Crosby G. Long-term impairment of acquisition of a spatial memory task following isoflurane-nitrous oxide anesthesia in rats. Anesthesiology. (2004) 100:309–14. doi: 10.1097/00000542-200402000-00020
64. Negrini D, Wu A, Oba A, Harnke B, Ciancio N, Krause M, et al. Incidence of postoperative cognitive dysfunction following inhalational vs total intravenous general anesthesia: a systematic review and meta-analysis. Neuropsychiatr Dis Treat. (2022) 18:1455–67. doi: 10.2147/NDT.S374416
65. Ding Y YJ, Cui F, Li J. Comparison of intravenous and inhalational anesthetic on postoperative cognitive outcomes in elderly patients undergoing cancer surgery: systematic review and meta-analysis. J Perianesth Nurs. (2022) 37(5):683–90. doi: 10.1016/j.jopan.2021.11.017
66. Schenning KJ, Murchison CF, Mattek NC, Kaye JA, Quinn JF. Sex and genetic differences in postoperative cognitive dysfunction: a longitudinal cohort analysis. Biol Sex Differ. (2019) 10:14. doi: 10.1186/s13293-019-0228-8
67. Abildstrom H, Christiansen M, Siersma VD, Rasmussen LS, Investigators I. Apolipoprotein E genotype and cognitive dysfunction after noncardiac surgery. Anesthesiology. (2004) 101:855–61. doi: 10.1097/00000542-200410000-00009
68. Mathew JP, Podgoreanu MV, Grocott HP, White WD, Morris RW, Stafford-Smith M, et al. Genetic variants in P-selectin and C-reactive protein influence susceptibility to cognitive decline after cardiac surgery. J Am Coll Cardiol. (2007) 49:1934–42. doi: 10.1016/j.jacc.2007.01.080
69. Brull DJ, Serrano N, Zito F, Jones L, Montgomery HE, Rumley A, et al. Human CRP gene polymorphism influences CRP levels: implications for the prediction and pathogenesis of coronary heart disease. Arterioscler Thromb Vasc Biol. (2003) 23:2063–9. doi: 10.1161/01.ATV.0000084640.21712.9C
70. Westphal S, Stoppe C, Gruenewald M, Bein B, Renner J, Cremer J, et al. Genome-wide association study of myocardial infarction, atrial fibrillation, acute stroke, acute kidney injury and delirium after cardiac surgery—a sub-analysis of the RIPHeart-study. BMC Cardiovasc Disord. (2019) 19:26. doi: 10.1186/s12872-019-1002-x
71. Wei XE, Zhang FY, Wang K, Zhang QX, Rong LQ. Assembly of the FKBP51-PHLPP2-AKT signaling complex in cerebral ischemia/reperfusion injury in rats. Brain Res. (2014) 1566:60–8. doi: 10.1016/j.brainres.2014.04.009
72. Heinrich M, Sieg M, Kruppa J, Nurnberg P, Schreier PH, Heilmann-Heimbach S, et al. Association between genetic variants of the cholinergic system and postoperative delirium and cognitive dysfunction in elderly patients. BMC Med Genomics. (2021) 14:248. doi: 10.1186/s12920-021-01071-1
73. Xie S, Yu L, Zhou M, Liu L, Lei D, Han C. Association between BDNF rs6265 polymorphisms and postoperative cognitive dysfunction in Chinese han population. Brain Behav. (2020) 10:e01800. doi: 10.1002/brb3.1800
74. Schenning KJ, Holden S, Davis BA, Mulford A, Nevonen KA, Quinn JF, et al. Gene-specific DNA methylation linked to postoperative cognitive dysfunction in apolipoprotein E3 and E4 mice. J Alzheimers Dis. (2021) 83:1251–68. doi: 10.3233/JAD-210499
75. He B, Wang J. METTL3 Regulates hippocampal gene transcription via N6-methyladenosine methylation in sevoflurane-induced postoperative cognitive dysfunction mouse. Aging (Albany NY). (2021) 13:23108–18. doi: 10.18632/aging.203604
76. Chen L, Xie W, Xie W, Zhuang W, Jiang C, Liu N. Apigenin attenuates isoflurane-induced cognitive dysfunction via epigenetic regulation and neuroinflammation in aged rats. Arch Gerontol Geriatr. (2017) 73:29–36. doi: 10.1016/j.archger.2017.07.004
77. Chen R, Kang Z, Wang Y, Zhao J, Li S. The anti-inflammatory effect of dexmedetomidine administration on patients undergoing intestinal surgery: a randomized study. Drugs R D. (2021) 21:445–53. doi: 10.1007/s40268-021-00368-x
78. Luo F, Min J, Wu J, Zuo Z. Histone deacetylases may mediate surgery-induced impairment of learning, memory, and dendritic development. Mol Neurobiol. (2020) 57:3702–11. doi: 10.1007/s12035-020-01987-2
79. Yang L, Hao JR, Gao Y, Yang X, Shen XR, Wang HY, et al. HDAC3 Of dorsal hippocampus induces postoperative cognitive dysfunction in aged mice. Behav Brain Res. (2022) 433:114002. doi: 10.1016/j.bbr.2022.114002
80. Bie B, Wu J, Yang H, Xu JJ, Brown DL, Naguib M. Epigenetic suppression of neuroligin 1 underlies amyloid-induced memory deficiency. Nat Neurosci. (2014) 17:223–31. doi: 10.1038/nn.3618
81. Min J, Lai Z, Wang H, Zuo Z. Preoperative environment enrichment preserved neuroligin 1 expression possibly via epigenetic regulation to reduce postoperative cognitive dysfunction in mice. CNS Neurosci Ther. (2022) 28:619–29. doi: 10.1111/cns.13777
82. Wu C, Wang R, Li X, Chen J. Preoperative Serum MicroRNA-155 expression independently predicts postoperative cognitive dysfunction after laparoscopic surgery for colon cancer. Med Sci Monit. (2016) 22:4503–8. doi: 10.12659/MSM.898397
83. Mahesh G, Biswas R. MicroRNA-155: a master regulator of inflammation. J Interferon Cytokine Res. (2019) 39:321–30. doi: 10.1089/jir.2018.0155
84. Cao RY, Li Q, Miao Y, Zhang Y, Yuan W, Fan L, et al. The emerging role of MicroRNA-155 in cardiovascular diseases. Biomed Res Int. (2016) 2016:9869208. doi: 10.1155/2016/9869208
85. Khalife J, Radomska HS, Santhanam R, Huang X, Neviani P, Saultz J, et al. Pharmacological targeting of miR-155 via the NEDD8-activating enzyme inhibitor MLN4924 (pevonedistat) in FLT3-ITD acute myeloid leukemia. Leukemia. (2015) 29:1981–92. doi: 10.1038/leu.2015.106
86. Galea I, Bechmann I, Perry VH. What is immune privilege (not)? Trends Immunol. (2007) 28:12–8. doi: 10.1016/j.it.2006.11.004
87. Nayak D, Roth TL, Mcgavern DB. Microglia development and function. Annu Rev Immunol. (2014) 32:367–402. doi: 10.1146/annurev-immunol-032713-120240
88. Prinz M, Jung S, Priller J. Microglia biology: one century of evolving concepts. Cell. (2019) 179:292–311. doi: 10.1016/j.cell.2019.08.053
89. Lawson LJ, Perry VH, Gordon S. Turnover of resident microglia in the normal adult mouse brain. Neuroscience. (1992) 48:405–15. doi: 10.1016/0306-4522(92)90500-2
90. Savage JC, Carrier M, Tremblay ME. Morphology of microglia across contexts of health and disease. Methods Mol Biol. (2019) 2034:13–26. doi: 10.1007/978-1-4939-9658-2_2
91. Nimmerjahn A, Kirchhoff F, Helmchen F. Resting microglial cells are highly dynamic surveillants of brain parenchyma in vivo. Science. (2005) 308:1314–8. doi: 10.1126/science.1110647
92. Tay TL, Savage JC, Hui CW, Bisht K, Tremblay ME. Microglia across the lifespan: from origin to function in brain development, plasticity and cognition. J Physiol. (2017) 595:1929–45. doi: 10.1113/JP272134
93. Block ML, Zecca L, Hong JS. Microglia-mediated neurotoxicity: uncovering the molecular mechanisms. Nat Rev Neurosci. (2007) 8:57–69. doi: 10.1038/nrn2038
94. Orihuela R, Mcpherson CA, Harry GJ. Microglial M1/M2 polarization and metabolic states. Br J Pharmacol. (2016) 173:649–65. doi: 10.1111/bph.13139
95. Liu LR, Liu JC, Bao JS, Bai QQ, Wang GQ. Interaction of microglia and astrocytes in the neurovascular unit. Front Immunol. (2020) 11:1024. doi: 10.3389/fimmu.2020.01024
96. Tang Y, Le W. Differential roles of M1 and M2 microglia in neurodegenerative diseases. Mol Neurobiol. (2016) 53:1181–94. doi: 10.1007/s12035-014-9070-5
97. Borst K, Dumas AA, Prinz M. Microglia: immune and non-immune functions. Immunity. (2021) 54:2194–208. doi: 10.1016/j.immuni.2021.09.014
98. Davalos D, Grutzendler J, Yang G, Kim JV, Zuo Y, Jung S, et al. ATP Mediates rapid microglial response to local brain injury in vivo. Nat Neurosci. (2005) 8:752–8. doi: 10.1038/nn1472
99. Haynes SE, Hollopeter G, Yang G, Kurpius D, Dailey ME, Gan WB, et al. The P2Y12 receptor regulates microglial activation by extracellular nucleotides. Nat Neurosci. (2006) 9:1512–9. doi: 10.1038/nn1805
100. Dissing-Olesen L, Ledue JM, Rungta RL, Hefendehl JK, Choi HB, Macvicar BA. Activation of neuronal NMDA receptors triggers transient ATP-mediated microglial process outgrowth. J Neurosci. (2014) 34:10511–27. doi: 10.1523/JNEUROSCI.0405-14.2014
101. Eyo UB, Gu N, De S, Dong H, Richardson JR, Wu LJ. Modulation of microglial process convergence toward neuronal dendrites by extracellular calcium. J Neurosci. (2015) 35:2417–22. doi: 10.1523/JNEUROSCI.3279-14.2015
102. Jha MK, Jo M, Kim JH, Suk K. Microglia-Astrocyte crosstalk: an intimate molecular conversation. Neuroscientist. (2019) 25:227–40. doi: 10.1177/1073858418783959
103. Zlokovic BV. Neurovascular pathways to neurodegeneration in Alzheimer’s disease and other disorders. Nat Rev Neurosci. (2011) 12:723–38. doi: 10.1038/nrn3114
104. Hussain B, Fang C, Chang J. Blood–brain barrier breakdown: an emerging biomarker of cognitive impairment in normal aging and dementia. Front Neurosci. (2021) 15. doi: 10.3389/fnins.2021.688090
105. Verheggen ICM, De Jong JJA, Van Boxtel MPJ, Gronenschild E, Palm WM, Postma AA, et al. Increase in blood–brain barrier leakage in healthy, older adults. Geroscience. (2020) 42:1183–93. doi: 10.1007/s11357-020-00211-2
106. Elahy M, Jackaman C, Mamo JC, Lam V, Dhaliwal SS, Giles C, et al. Blood-brain barrier dysfunction developed during normal aging is associated with inflammation and loss of tight junctions but not with leukocyte recruitment. Immun Ageing. (2015) 12:2. doi: 10.1186/s12979-015-0029-9
107. Mattson MP, Arumugam TV. Hallmarks of brain aging: adaptive and pathological modification by metabolic states. Cell Metab. (2018) 27:1176–99. doi: 10.1016/j.cmet.2018.05.011
108. Prasad S, Sajja RK, Naik P, Cucullo L. Diabetes Mellitus and blood-brain barrier dysfunction: an overview. J Pharmacovigil. (2014) 2:125. doi: 10.4172/2329-6887.1000125
109. Skvarc DR, Berk M, Byrne LK, Dean OM, Dodd S, Lewis M, et al. Post-Operative cognitive dysfunction: an exploration of the inflammatory hypothesis and novel therapies. Neurosci Biobehav Rev. (2018) 84. doi: 10.1016/j.neubiorev.2017.11.011
110. Buvanendran A, Kroin JS, Berger RA, Hallab NJ, Saha C, Negrescu C, et al. Upregulation of prostaglandin E2 and interleukins in the central nervous system and peripheral tissue during and after surgery in humans. Anesthesiology. (2006) 104:403–10. doi: 10.1097/00000542-200603000-00005
111. Beloosesky Y, Hendel D, Weiss A, Hershkovitz A, Grinblat J, Pirotsky A, et al. Cytokines and C-reactive protein production in hip-fracture-operated elderly patients. J Gerontol A Biol Sci Med Sci. (2007) 62:420–6. doi: 10.1093/gerona/62.4.420
112. Donato AJ, Eskurza I, Silver AE, Levy AS, Pierce GL, Gates PE, et al. Direct evidence of endothelial oxidative stress with aging in humans: relation to impaired endothelium-dependent dilation and upregulation of nuclear factor-kappaB. Circ Res. (2007) 100:1659–66. doi: 10.1161/01.RES.0000269183.13937.e8
113. Bake S, Friedman JA, Sohrabji F. Reproductive age-related changes in the blood brain barrier: expression of IgG and tight junction proteins. Microvasc Res. (2009) 78:413–24. doi: 10.1016/j.mvr.2009.06.009
114. Mooradian AD. Effect of aging on the blood-brain barrier. Neurobiol Aging. (1988) 9:31–9. doi: 10.1016/S0197-4580(88)80013-7
115. Schultz N, Brännström K, Byman E, Moussaud S, Nielsen HM, Olofsson A, et al. Amyloid-beta 1-40 is associated with alterations in NG2+ pericyte population ex vivo and in vitro. Aging Cell. (2018) 17:e12728. doi: 10.1111/acel.12728
116. Bell RD, Winkler EA, Sagare AP, Singh I, Larue B, Deane R, et al. Pericytes control key neurovascular functions and neuronal phenotype in the adult brain and during brain aging. Neuron. (2010) 68:409–27. doi: 10.1016/j.neuron.2010.09.043
117. Berthiaume AA, Schmid F, Stamenkovic S, Coelho-Santos V, Nielson CD, Weber B, et al. Pericyte remodeling is deficient in the aged brain and contributes to impaired capillary flow and structure. Nat Commun. (2022) 13:5912. doi: 10.1038/s41467-022-33464-w
118. Choe YG, Yoon JH, Joo J, Kim B, Hong SP, Koh GY, et al. Pericyte loss leads to capillary stalling through increased leukocyte-endothelial cell interaction in the brain. Front Cell Neurosci. (2022) 16:848764. doi: 10.3389/fncel.2022.848764
119. Hughes S, Gardiner T, Hu P, Baxter L, Rosinova E, Chan-Ling T. Altered pericyte-endothelial relations in the rat retina during aging: implications for vessel stability. Neurobiol Aging. (2006) 27:1838–47. doi: 10.1016/j.neurobiolaging.2005.10.021
120. He H, Lam M, Mccormick TS, Distelhorst CW. Maintenance of calcium homeostasis in the endoplasmic reticulum by Bcl-2. J Cell Biol. (1997) 138:1219–28. doi: 10.1083/jcb.138.6.1219
121. Candelario-Jalil E, Thompson J, Taheri S, Grossetete M, Adair JC, Edmonds E, et al. Matrix metalloproteinases are associated with increased blood-brain barrier opening in vascular cognitive impairment. Stroke. (2011) 42:1345–50. doi: 10.1161/STROKEAHA.110.600825
122. Cortes-Canteli M, Paul J, Norris EH, Bronstein R, Ahn HJ, Zamolodchikov D, et al. Fibrinogen and beta-amyloid association alters thrombosis and fibrinolysis: a possible contributing factor to Alzheimer’s disease. Neuron. (2010) 66:695–709. doi: 10.1016/j.neuron.2010.05.014
123. Cortes-Canteli M, Mattei L, Richards AT, Norris EH, Strickland S. Fibrin deposited in the Alzheimer’s disease brain promotes neuronal degeneration. Neurobiol Aging. (2015) 36:608–17. doi: 10.1016/j.neurobiolaging.2014.10.030
124. Garner KM, Amin R, Johnson RW, Scarlett EJ, Burton MD. Microglia priming by interleukin-6 signaling is enhanced in aged mice. J Neuroimmunol. (2018) 324:90–9. doi: 10.1016/j.jneuroim.2018.09.002
125. Yoo H-J, Kwon M-S. Aged microglia in neurodegenerative diseases: microglia lifespan and culture methods. Front Aging Neurosci. (2022) 13. doi: 10.3389/fnagi.2021.766267
126. Grabert K, Michoel T, Karavolos MH, Clohisey S, Baillie JK, Stevens MP, et al. Microglial brain region-dependent diversity and selective regional sensitivities to aging. Nat Neurosci. (2016) 19:504–16. doi: 10.1038/nn.4222
127. Deczkowska A, Amit I, Schwartz M. Microglial immune checkpoint mechanisms. Nat Neurosci. (2018) 21:779–86. doi: 10.1038/s41593-018-0145-x
128. Safaiyan S, Kannaiyan N, Snaidero N, Brioschi S, Biber K, Yona S, et al. Age-related myelin degradation burdens the clearance function of microglia during aging. Nat Neurosci. (2016) 19:995–8. doi: 10.1038/nn.4325
129. Galloway DA, Phillips AEM, Owen DRJ, Moore CS. Phagocytosis in the brain: homeostasis and disease. Front Immunol. (2019) 10:790. doi: 10.3389/fimmu.2019.00790
130. Damisah EC, Hill RA, Rai A, Chen F, Rothlin CV, Ghosh S, et al. Astrocytes and microglia play orchestrated roles and respect phagocytic territories during neuronal corpse removal in vivo. Sci Adv. (2020) 6:eaba3239. doi: 10.1126/sciadv.aba3239
131. Eckenhoff RG, Johansson JS, Wei H, Carnini A, Kang B, Wei W, et al. Inhaled anesthetic enhancement of amyloid-beta oligomerization and cytotoxicity. Anesthesiology. (2004) 101:703–9. doi: 10.1097/00000542-200409000-00019
132. Carnini A, Lear JD, Eckenhoff RG. Inhaled anesthetic modulation of amyloid beta(1–40) assembly and growth. Curr Alzheimer Res. (2007) 4:233–41. doi: 10.2174/156720507781077278
133. Mandal PK, Fodale V. Isoflurane and desflurane at clinically relevant concentrations induce amyloid beta-peptide oligomerization: an NMR study. Biochem Biophys Res Commun. (2009) 379:716–20. doi: 10.1016/j.bbrc.2008.12.092
134. Ge X, Zuo Y, Xie J, Li X, Li Y, Thirupathi A, et al. A new mechanism of POCD caused by sevoflurane in mice: cognitive impairment induced by cross-dysfunction of iron and glucose metabolism. Aging (Albany NY). (2021) 13:22375–89. doi: 10.18632/aging.203544
135. Alexander JJ. Blood-brain barrier (BBB) and the complement landscape. Mol Immunol. (2018) 102:26–31. doi: 10.1016/j.molimm.2018.06.267
136. Vasileiou I, Xanthos T, Koudouna E, Perrea D, Klonaris C, Katsargyris A, et al. Propofol: a review of its non-anaesthetic effects. Eur J Pharmacol. (2009) 605:1–8. doi: 10.1016/j.ejphar.2009.01.007
137. Inada T, Kubo K, Ueshima H, Shingu K. Intravenous anesthetic propofol suppresses prostaglandin E2 production in murine dendritic cells. J Immunotoxicol. (2011) 8:359–66. doi: 10.3109/1547691X.2011.620036
138. Inada T, Hirota K, Shingu K. Intravenous anesthetic propofol suppresses prostaglandin E2 and cysteinyl leukotriene production and reduces edema formation in arachidonic acid-induced ear inflammation. J Immunotoxicol. (2015) 12:261–5. doi: 10.3109/1547691X.2014.938874
139. Pappa M, Theodosiadis N, Tsounis A, Sarafis P. Pathogenesis and treatment of post-operative cognitive dysfunction. Electron Physician. (2017) 9(2):3768–75. doi: 10.19082/3768
140. Acharya NK, Goldwaser EL, Forsberg MM, Godsey GA, Johnson CA, Sarkar A, et al. Sevoflurane and isoflurane induce structural changes in brain vascular endothelial cells and increase blood–brain barrier permeability: possible link to postoperative delirium and cognitive decline. Brain Res. (2015) 1620:29–41. doi: 10.1016/j.brainres.2015.04.054
141. Yang S, Gu C, Mandeville ET, Dong Y, Esposito E, Zhang Y, et al. Anesthesia and surgery impair blood-brain barrier and cognitive function in mice. Front Immunol. (2017) 8:902. doi: 10.3389/fimmu.2017.00902
142. Burton MD, Rytych JL, Freund GG, Johnson RW. Central inhibition of interleukin-6 trans-signaling during peripheral infection reduced neuroinflammation and sickness in aged mice. Brain Behav Immun. (2013) 30:66–72. doi: 10.1016/j.bbi.2013.01.002
143. Patterson SL. Immune dysregulation and cognitive vulnerability in the aging brain: interactions of microglia, IL-1β, BDNF and synaptic plasticity. Neuropharmacology. (2015) 96:11–8. doi: 10.1016/j.neuropharm.2014.12.020
144. Chen RM, Chen TG, Chen TL, Lin LL, Chang CC, Chang HC, et al. Anti-inflammatory and antioxidative effects of propofol on lipopolysaccharide-activated macrophages. Ann N Y Acad Sci. (2005) 1042:262–71. doi: 10.1196/annals.1338.030
145. Ramadasan-Nair R, Hui J, Itsara LS, Morgan PG, Sedensky MM. Mitochondrial function in astrocytes is essential for normal emergence from anesthesia in mice. Anesthesiology. (2019) 130:423–34. doi: 10.1097/ALN.0000000000002528
146. Liu H, Zhang J. Cerebral hypoperfusion and cognitive impairment: the pathogenic role of vascular oxidative stress. Int J Neurosci. (2012) 122:494–9. doi: 10.3109/00207454.2012.686543
147. Ronaldson PT, Davis TP. Blood-brain barrier integrity and glial support: mechanisms that can be targeted for novel therapeutic approaches in stroke. Curr Pharm Des. (2012) 18:3624–44. doi: 10.2174/138161212802002625
148. Huber JD, Campos CR, Mark KS, Davis TP. Alterations in blood-brain barrier ICAM-1 expression and brain microglial activation after lambda-carrageenan-induced inflammatory pain. Am J Physiol Heart Circ Physiol. (2006) 290:H732–740. doi: 10.1152/ajpheart.00747.2005
149. Kurtel H, Fujimoto K, Zimmerman BJ, Granger DN, Tso P. Ischemia-reperfusion-induced mucosal dysfunction: role of neutrophils. Am J Physiol. (1991) 261:G490–96. doi: 10.1152/ajpcell.1991.261.3.C490
150. Mcmillen MA, Huribal M, Sumpio B. Common pathway of endothelial-leukocyte interaction in shock, ischemia, and reperfusion. Am J Surg. (1993) 166:557–62. doi: 10.1016/S0002-9610(05)81153-5
151. Meisel SR, Shapiro H, Radnay J, Neuman Y, Khaskia AR, Gruener N, et al. Increased expression of neutrophil and monocyte adhesion molecules LFA-1 and Mac-1 and their ligand ICAM-1 and VLA-4 throughout the acute phase of myocardial infarction: possible implications for leukocyte aggregation and microvascular plugging. J Am Coll Cardiol. (1998) 31:120–5. doi: 10.1016/S0735-1097(97)00424-5
152. Gahtan E, Overmier JB. Inflammatory pathogenesis in Alzheimer’s disease: biological mechanisms and cognitive sequeli. Neurosci Biobehav Rev. (1999) 23:615–33. doi: 10.1016/S0149-7634(98)00058-X
153. González-Scarano F, Baltuch G. Microglia as mediators of inflammatory and degenerative diseases. Annu Rev Neurosci. (1999) 22:219–40. doi: 10.1146/annurev.neuro.22.1.219
154. Zhang Y, Xu Z, Wang H, Dong Y, Shi HN, Culley DJ, et al. Anesthetics isoflurane and desflurane differently affect mitochondrial function, learning, and memory. Ann Neurol. (2012) 71:687–98. doi: 10.1002/ana.23536
155. Papon MA, Whittington RA, El-Khoury NB, Planel E. Alzheimer’s disease and anesthesia. Front Neurosci. (2011) 4:272. doi: 10.3389/fnins.2010.00272
156. Bohnen N, Warner MA, Kokmen E, Kurland LT. Early and midlife exposure to anesthesia and age of onset of Alzheimer’s disease. Int J Neurosci. (1994) 77:181–5. doi: 10.3109/00207459408986029
157. Fodale V, Praticò C, Santamaria L. Anesthestic drugs act on the cerebral cholinergic system potentially mediating delirium and postoperative cognitive dysfunctions—letter. Br Med J. (2004) 328.
158. Andrews-Hanna JR, Snyder AZ, Vincent JL, Lustig C, Head D, Raichle ME, et al. Disruption of large-scale brain systems in advanced aging. Neuron. (2007) 56:924–35. doi: 10.1016/j.neuron.2007.10.038
159. Wang H, Golob EJ, Su MY. Vascular volume and blood-brain barrier permeability measured by dynamic contrast enhanced MRI in hippocampus and cerebellum of patients with MCI and normal controls. J Magn Reson Imaging. (2006) 24:695–700. doi: 10.1002/jmri.20669
160. Nation DA, Sweeney MD, Montagne A, Sagare AP, D’orazio LM, Pachicano M, et al. Blood–brain barrier breakdown is an early biomarker of human cognitive dysfunction. Nat Med. (2019) 25:270–6. doi: 10.1038/s41591-018-0297-y
161. Versele R, Sevin E, Gosselet F, Fenart L, Candela P. TNF-α and IL-1β modulate blood-brain barrier permeability and decrease amyloid-β peptide efflux in a human blood-brain barrier model. Int J Mol Sci. (2022) 23:10235. doi: 10.3390/ijms231810235
162. Wu F, Zou Q, Ding X, Shi D, Zhu X, Hu W, et al. Complement component C3a plays a critical role in endothelial activation and leukocyte recruitment into the brain. J Neuroinflammation. (2016) 13:23. doi: 10.1186/s12974-016-0485-y
163. Orsini F, De Blasio D, Zangari R, Zanier ER, De Simoni MG. Corrigendum: versatility of the complement system in neuroinflammation, neurodegeneration, and brain homeostasis. Front Cell Neurosci. (2015) 9:263. doi: 10.3389/fncel.2015.00263
164. Jacob A, Alexander JJ. Complement and blood-brain barrier integrity. Mol Immunol. (2014) 61:149–52. doi: 10.1016/j.molimm.2014.06.039
165. Kolev MV, Ruseva MM, Harris CL, Morgan BP, Donev RM. Implication of complement system and its regulators in Alzheimer’s disease. Curr Neuropharmacol. (2009) 7:1–8. doi: 10.2174/157015909787602805
166. Perucho J, Rubio I, Casarejos MJ, Gomez A, Rodriguez-Navarro JA, Solano RM, et al. Anesthesia with isoflurane increases amyloid pathology in mice models of Alzheimer’s disease. J Alzheimers Dis. (2010) 19:1245–57. doi: 10.3233/JAD-2010-1318
167. Weinstein EJ, Levene JL, Cohen MS, Andreae DA, Chao JY, Johnson M, et al. Local anaesthetics and regional anaesthesia versus conventional analgesia for preventing persistent postoperative pain in adults and children. Cochrane Database Syst Rev. (2018) 6:CD007105. doi: 10.1002/14651858.CD007105.pub3
168. Folino TB, Mahboobi SK. Regional anesthetic blocks. In: Statpearls. Treasure Island (FL): StatPearls (2023).
169. Garmon EH, Huecker MR. Topical, local, and regional anesthesia and anesthetics. In: Statpearls. Treasure Island (FL): StatPearls (2023).
170. Jeong HJ, Lin D, Li L, Zuo Z. Delayed treatment with lidocaine reduces mouse microglial cell injury and cytokine production after stimulation with lipopolysaccharide and interferon gamma. Anesth Analg. (2012) 114:856–61. doi: 10.1213/ANE.0b013e3182460ab5
171. Su D, Gu Y, Wang Z, Wang X. Lidocaine attenuates proinflammatory cytokine production induced by extracellular adenosine triphosphate in cultured rat microglia. Anesth Analg. (2010) 111:768–74. doi: 10.1213/ANE.0b013e3181e9e897
172. Zhang J, Deng X. Bupivacaine effectively relieves inflammation-induced pain by suppressing activation of the NF-kappaB signalling pathway and inhibiting the activation of spinal microglia and astrocytes. Exp Ther Med. (2017) 13:1074–80. doi: 10.3892/etm.2017.4058
173. Toda S, Sakai A, Ikeda Y, Sakamoto A, Suzuki H. A local anesthetic, ropivacaine, suppresses activated microglia via a nerve growth factor-dependent mechanism and astrocytes via a nerve growth factor-independent mechanism in neuropathic pain. Mol Pain. (2011) 7:2. doi: 10.1186/1744-8069-7-2
174. Wang D, Wu X, Li J, Xiao F, Liu X, Meng M. The effect of lidocaine on early postoperative cognitive dysfunction after coronary artery bypass surgery. Anesth Analg. (2002) 95:1134–41, table of contents. doi: 10.1097/00000539-200211000-00002
175. Mathew JP, Mackensen GB, Phillips-Bute B, Grocott HP, Glower DD, Laskowitz DT, et al., for the Neurologic Outcome Research Group (NORG) of the Duke Heart Center. Randomized, double-blinded, placebo controlled study of neuroprotection with lidocaine in cardiac surgery. Stroke. (2009) 40:880–7. doi: 10.1161/STROKEAHA.108.531236
176. Klinger RY, Cooter M, Bisanar T, Terrando N, Berger M, Podgoreanu MV, et al., for the Neurologic Outcomes Research Group of the Duke Heart Center. Intravenous lidocaine does not improve neurologic outcomes after cardiac surgery: a randomized controlled trial. Anesthesiology. (2019) 130:958–70. doi: 10.1097/ALN.0000000000002668
177. Wang XX, Dai J, Wang Q, Deng HW, Liu Y, He GF, et al. Intravenous lidocaine improves postoperative cognition in patients undergoing laparoscopic colorectal surgery: a randomized, double-blind, controlled study. BMC Anesthesiol. (2023) 23:243. doi: 10.1186/s12871-023-02210-0
178. Rasmussen LS, Johnson T, Kuipers HM, Kristensen D, Siersma VD, Vila P, et al. Does anaesthesia cause postoperative cognitive dysfunction? A randomised study of regional versus general anaesthesia in 438 elderly patients. Acta Anaesthesiol Scand. (2003) 47:260–6. doi: 10.1034/j.1399-6576.2003.00057.x
179. Davis N, Lee M, Lin AY, Lynch L, Monteleone M, Falzon L, et al. Postoperative cognitive function following general versus regional anesthesia: a systematic review. J Neurosurg Anesthesiol. (2014) 26:369–76. doi: 10.1097/ANA.0000000000000120
180. Bhushan S, Huang X, Duan Y, Xiao Z. The impact of regional versus general anesthesia on postoperative neurocognitive outcomes in elderly patients undergoing hip fracture surgery: a systematic review and meta-analysis. Int J Surg. (2022) 105:106854. doi: 10.1016/j.ijsu.2022.106854
181. Viderman D, Nabidollayeva F, Aubakirova M, Yessimova D, Badenes R, Abdildin Y. Postoperative delirium and cognitive dysfunction after general and regional anesthesia: a systematic review and meta-analysis. J Clin Med. (2023) 12:3549. doi: 10.3390/jcm12103549
182. Kuzminskaite V, Kontrimaviciute E, Kauzonas E, Slauzgalvyte I, Bukelyte G, Bruzyte-Narkiene G, et al. Sevoflurane and desflurane effects on early cognitive function after low-risk surgery: a randomized clinical trial. Brain Behav. (2023) 13:e3017. doi: 10.1002/brb3.3017
183. Franks JJ, Wamil AW, Janicki PK, Horn JL, Franks WT, Janson VE, et al. Anesthetic-induced alteration of Ca2+ homeostasis in neural cells: a temperature-sensitive process that is enhanced by blockade of plasma membrane Ca2+-ATPase isoforms. Anesthesiology. (1998) 89:149–64. doi: 10.1097/00000542-199807000-00022
184. Kindler CH, Eilers H, Donohoe P, Ozer S, Bickler PE. Volatile anesthetics increase intracellular calcium in cerebrocortical and hippocampal neurons. Anesthesiology. (1999) 90:1137–45. doi: 10.1097/00000542-199904000-00029
185. Wei H, Kang B, Wei W, Liang G, Meng QC, Li Y, et al. Isoflurane and sevoflurane affect cell survival and BCL-2/BAX ratio differently. Brain Res. (2005) 1037:139–47. doi: 10.1016/j.brainres.2005.01.009
186. Jiang M, Sun L, Feng DX, Yu ZQ, Gao R, Sun YZ, et al. Neuroprotection provided by isoflurane pre-conditioning and post-conditioning. Med Gas Res. (2017) 7:48–55. doi: 10.4103/2045-9912.202910
187. Warner DS, Mcfarlane C, Todd MM, Ludwig P, Mcallister AM. Sevoflurane and halothane reduce focal ischemic brain damage in the rat. Possible influence on thermoregulation. Anesthesiology. (1993) 79:985–92. doi: 10.1097/00000542-199311000-00017
188. Xu F, Wang Y, Han L, Deng D, Ding Y, Ma L, et al. PEX5R/Trip8b-HCN2 Channel regulating neuroinflammation involved in perioperative neurocognitive disorders. Cell Biosci. (2022) 12:156. doi: 10.1186/s13578-022-00892-6
189. Rajanna P, Reddy JN, Gupta PK. Ketamine for the relief of bronchospasm during anaesthesia. Anaesthesia. (1982) 37:1215. doi: 10.1111/j.1365-2044.1982.tb01793.x
190. Park GR, Manara AR, Mendel L, Bateman PE. Ketamine infusion. Its use as a sedative, inotrope and bronchodilator in a critically ill patient. Anaesthesia. (1987) 42:980–3. doi: 10.1111/j.1365-2044.1987.tb05370.x
191. Dominguini D, Steckert AV, Michels M, Spies MB, Ritter C, Barichello T, et al. The effects of anaesthetics and sedatives on brain inflammation. Neurosci Biobehav Rev. (2021) 127:504–13. doi: 10.1016/j.neubiorev.2021.05.009
192. Yao W, Cao Q, Luo S, He L, Yang C, Chen J, et al. Microglial ERK-NRBP1-CREB-BDNF signaling in sustained antidepressant actions of (R)-ketamine. Mol Psychiatry. (2022) 27:1618–29. doi: 10.1038/s41380-021-01377-7
193. Roytblat L, Talmor D, Rachinsky M, Greemberg L, Pekar A, Appelbaum A, et al. Ketamine attenuates the interleukin-6 response after cardiopulmonary bypass. Anesth Analg. (1998) 87:266–71. doi: 10.1213/00000539-199808000-00006
194. Zanos P, Moaddel R, Morris PJ, Riggs LM, Highland JN, Georgiou P, et al. Ketamine and ketamine metabolite pharmacology: insights into therapeutic mechanisms. Pharmacol Rev. (2018) 70:621–60. doi: 10.1124/pr.117.015198
195. Beilin B, Rusabrov Y, Shapira Y, Roytblat L, Greemberg L, Yardeni IZ, et al. Low-dose ketamine affects immune responses in humans during the early postoperative period. Br J Anaesth. (2007) 99:522–7. doi: 10.1093/bja/aem218
196. Hudetz JA, Iqbal Z, Gandhi SD, Patterson KM, Byrne AJ, Hudetz AG, et al. Ketamine attenuates post-operative cognitive dysfunction after cardiac surgery. Acta Anaesthesiol Scand. (2009) 53:864–72. doi: 10.1111/j.1399-6576.2009.01978.x
197. Wittwer ED, Cerhan JH, Schroeder DR, Schaff HV, Mauermann WJ. Impact of ketamine versus propofol for anesthetic induction on cognitive dysfunction, delirium, and acute kidney injury following cardiac surgery in elderly, high-risk patients. Ann Card Anaesth. (2023) 26:274–80. doi: 10.4103/aca.aca_106_22
198. Han C, Ji H, Guo Y, Fei Y, Wang C, Yuan Y, et al. Effect of subanesthetic dose of esketamine on perioperative neurocognitive disorders in elderly undergoing gastrointestinal surgery: a randomized controlled trial. Drug Des Devel Ther. (2023) 17:863–73. doi: 10.2147/DDDT.S401161
199. Verdonk F, Petit AC, Abdel-Ahad P, Vinckier F, Jouvion G, De Maricourt P, et al. Microglial production of quinolinic acid as a target and a biomarker of the antidepressant effect of ketamine. Brain Behav Immun. (2019) 81:361–73. doi: 10.1016/j.bbi.2019.06.033
200. Hollinger A, Rust CA, Riegger H, Gysi B, Tran F, Brugger J, et al. Ketamine vs. Haloperidol for prevention of cognitive dysfunction and postoperative delirium: a phase IV multicentre randomised placebo-controlled double-blind clinical trial. J Clin Anesth. (2021) 68:110099. doi: 10.1016/j.jclinane.2020.110099
201. Oriby ME, Elrashidy AA, Elsharkawy A, Ahmed SA. Effects of ketamine or dexmedetomidine on postoperative cognitive dysfunction after cataract surgery: a randomized controlled trial. Indian J Anaesth. (2023) 67:186–93. doi: 10.4103/ija.ija_429_22
202. Chen X, Liu Q, Fan L. Effects of thoracic paravertebral block combined with s-ketamine on postoperative pain and cognitive function after thoracoscopic surgery. Heliyon. (2022) 8:e12231. doi: 10.1016/j.heliyon.2022.e12231
203. Hovaguimian F, Tschopp C, Beck-Schimmer B, Puhan M. Intraoperative ketamine administration to prevent delirium or postoperative cognitive dysfunction: a systematic review and meta-analysis. Acta Anaesthesiol Scand. (2018) 62:1182–93. doi: 10.1111/aas.13168
204. Ingrande J, Pearn ML, Patel HH. General anesthetics and therapeutic gases. In: Brunton LL, Knollmann BC, editors. Goodman & Gilman’s: The Pharmacological Basis of Therapeutics, 14th ed. New York, NY: McGraw-Hill Education (2023).
205. Huang S, Hu H, Cai YH, Hua F. Effect of parecoxib in the treatment of postoperative cognitive dysfunction: a systematic review and meta-analysis. Medicine (Baltimore). (2019) 98:e13812. doi: 10.1097/MD.0000000000013812
206. Royse CF, Andrews DT, Newman SN, Stygall J, Williams Z, Pang J, et al. The influence of propofol or desflurane on postoperative cognitive dysfunction in patients undergoing coronary artery bypass surgery. Anaesthesia. (2011) 66:455–64. doi: 10.1111/j.1365-2044.2011.06704.x
207. Schoen J, Husemann L, Tiemeyer C, Lueloh A, Sedemund-Adib B, Berger KU, et al. Cognitive function after sevoflurane- vs propofol-based anaesthesia for on-pump cardiac surgery: a randomized controlled trial. Br J Anaesth. (2011) 106:840–50. doi: 10.1093/bja/aer091
208. Geng Y-J, Wu Q-H, Zhang R-Q. Effect of propofol, sevoflurane, and isoflurane on postoperative cognitive dysfunction following laparoscopic cholecystectomy in elderly patients: a randomized controlled trial. J Clin Anesth. (2017) 38:165–71. doi: 10.1016/j.jclinane.2017.02.007
209. Li WX, Luo RY, Chen C, Li X, Ao JS, Liu Y, et al. Effects of propofol, dexmedetomidine, and midazolam on postoperative cognitive dysfunction in elderly patients: a randomized controlled preliminary trial. Chin Med J (Engl). (2019) 132:437–45. doi: 10.1097/CM9.0000000000000098
210. Guo L, Lin F, Dai H, Du X, Yu M, Zhang J, et al. Impact of sevoflurane versus propofol anesthesia on post-operative cognitive dysfunction in elderly cancer patients: a double-blinded randomized controlled trial. Med Sci Monit. (2020) 26:e919293. doi: 10.12659/MSM.919293
211. Rajaei M, Tabari M, Soltani G, Alizadeh K, Nazari A, Noroozian M, et al. Comparison between the effects of dexmedetomidine and midazolam on postoperative cognitive impairment after coronary artery bypasses graft surgery: a randomized clinical trial. J Tehran Heart Cent. (2019) 14:67–73.31723348
212. Silbert BS, Scott DA, Evered LA, Lewis MS, Kalpokas M, Maruff P, et al. A comparison of the effect of high- and low-dose fentanyl on the incidence of postoperative cognitive dysfunction after coronary artery bypass surgery in the elderly. Anesthesiology. (2006) 104:1137–45. doi: 10.1097/00000542-200606000-00007
213. Zhi Y, Li W. Effects of total intravenous anesthesia with etomidate and propofol on postoperative cognitive dysfunction. Physiol Res. (2023) 72:251–8. doi: 10.33549/physiolres.934983
214. Wang W, Ma Y, Liu Y, Wang P, Liu Y. Effects of dexmedetomidine anesthesia on early postoperative cognitive dysfunction in elderly patients. ACS Chem Neurosci. (2022) 13:2309–14. doi: 10.1021/acschemneuro.2c00173
215. Valentin LS, Pereira VF, Pietrobon RS, Schmidt AP, Oses JP, Portela LV, et al. Effects of single low dose of dexamethasone before noncardiac and nonneurologic surgery and general anesthesia on postoperative cognitive dysfunction—a phase III double blind, randomized clinical trial. PLoS One. (2016) 11:e0152308. doi: 10.1371/journal.pone.0152308
216. Hassan WF, Tawfik MH, Nabil TM, Abd Elkareem RM. Could intraoperative magnesium sulphate protect against postoperative cognitive dysfunction? Minerva Anestesiol. (2020) 86:808–15. doi: 10.23736/S0375-9393.20.14012-4
217. Kletecka J, Holeckova I, Brenkus P, Pouska J, Benes J, Chytra I. Propofol versus sevoflurane anaesthesia: effect on cognitive decline and event-related potentials. J Clin Monit Comput. (2019) 33:665–73. doi: 10.1007/s10877-018-0213-5
218. Mei B, Meng G, Xu G, Cheng X, Chen S, Zhang Y, et al. Intraoperative sedation with dexmedetomidine is superior to propofol for elderly patients undergoing hip arthroplasty: a prospective randomized controlled study. Clin J Pain. (2018) 34:811–7. doi: 10.1097/AJP.0000000000000605
219. Pang QY, Duan LP, Jiang Y, Liu HL. Effects of inhalation and propofol anaesthesia on postoperative cognitive dysfunction in elderly noncardiac surgical patients: a systematic review and meta-analysis. Medicine (Baltimore). (2021) 100:e27668. doi: 10.1097/MD.0000000000027668
220. Zeng K, Long J, Li Y, Hu J. Preventing postoperative cognitive dysfunction using anesthetic drugs in elderly patients undergoing noncardiac surgery: a systematic review and meta-analysis. Int J Surg. (2023) 109:21–31. doi: 10.1097/JS9.0000000000000001
221. Zhang J, Haddad GG, Xia Y. delta-, but not mu- and kappa-, opioid receptor activation protects neocortical neurons from glutamate-induced excitotoxic injury. Brain Res. (2000) 885:143–53. doi: 10.1016/S0006-8993(00)02906-1
222. Iwata M, Inoue S, Kawaguchi M, Nakamura M, Konishi N, Furuya H. Effects of delta-opioid receptor stimulation and inhibition on hippocampal survival in a rat model of forebrain ischaemia. Br J Anaesth. (2007) 99:538–46. doi: 10.1093/bja/aem220
223. Machelska H, Celik MO. Opioid receptors in immune and glial cells-implications for pain control. Front Immunol. (2020) 11:300. doi: 10.3389/fimmu.2020.00300
224. Ferrini F, Trang T, Mattioli TA, Laffray S, Del'guidice T, Lorenzo LE, et al. Morphine hyperalgesia gated through microglia-mediated disruption of neuronal Cl(-) homeostasis. Nat Neurosci. (2013) 16:183–92. doi: 10.1038/nn.3295
225. Tumati S, Largent-Milnes TM, Keresztes AI, Yamamoto T, Vanderah TW, Roeske WR, et al. Tachykinin NK(1) receptor antagonist co-administration attenuates opioid withdrawal-mediated spinal microglia and astrocyte activation. Eur J Pharmacol. (2012) 684:64–70. doi: 10.1016/j.ejphar.2012.03.025
226. Johnson JL, Rolan PE, Johnson ME, Bobrovskaya L, Williams DB, Johnson K, et al. Codeine-induced hyperalgesia and allodynia: investigating the role of glial activation. Transl Psychiatry. (2014) 4:e482. doi: 10.1038/tp.2014.121
227. Roeckel LA, Le Coz GM, Gaveriaux-Ruff C, Simonin F. Opioid-induced hyperalgesia: cellular and molecular mechanisms. Neuroscience. (2016) 338:160–82. doi: 10.1016/j.neuroscience.2016.06.029
228. Smith SM, Brown HO, Toman JEP, Goodman LS. The lack of cerebral effects of d-tubocurarine. Anesthesiology. (1947) 8:1–14. doi: 10.1097/00000542-194701000-00001
229. Chiodini FC, Tassonyi E, Fuchs-Buder T, Fathi M, Bertrand D, Muller D. Effects of neuromuscular blocking agents on excitatory transmission and gamma-aminobutyric acid-A-mediated inhibition in the rat hippocampal slice. Anesthesiology. (1998) 88:1003–13. doi: 10.1097/00000542-199804000-00021
230. Matteo RS, Pua EK, Khambatta HJ, Spector S. Cerebrospinal fluid levels of d-tubocurarine in man. Anesthesiology. (1977) 46:396–9. doi: 10.1097/00000542-197706000-00004
231. Eddleston JM, Harper NJ, Pollard BJ, Edwards D, Gwinnutt CL. Concentrations of atracurium and laudanosine in cerebrospinal fluid and plasma during intracranial surgery. Br J Anaesth. (1989) 63:525–30. doi: 10.1093/bja/63.5.525
232. Vernino S, Amador M, Luetje CW, Patrick J, Dani JA. Calcium modulation and high calcium permeability of neuronal nicotinic acetylcholine receptors. Neuron. (1992) 8:127–34. doi: 10.1016/0896-6273(92)90114-S
233. Cardone C, Szenohradszky J, Yost S, Bickler PE. Activation of brain acetylcholine receptors by neuromuscular blocking drugs. A possible mechanism of neurotoxicity. Anesthesiology. (1994) 80:1155–61; discussion 1129A. doi: 10.1097/00000542-199405000-00025
234. Mihic SJ, Mayfield J. Hypnotics and sedatives. In: Brunton LL, Knollmann BC, editors. Goodman & Gilman’s: The Pharmacological Basis of Therapeutics, 14th ed. New York, NY: McGraw-Hill Education (2023).
235. Tanaka T, Kai S, Matsuyama T, Adachi T, Fukuda K, Hirota K. General anesthetics inhibit LPS-induced IL-1beta expression in glial cells. PLoS One. (2013) 8:e82930. doi: 10.1371/journal.pone.0082930
236. Mansouri N, Nasrollahi K, Shetabi H. Prevention of cognitive dysfunction after cataract surgery with intravenous administration of midazolam and dexmedetomidine in elderly patients undergoing cataract surgery. Adv Biomed Res. (2019) 8:6. doi: 10.4103/abr.abr_190_18
237. Furukawa T, Nikaido Y, Shimoyama S, Masuyama N, Notoya A, Ueno S. Impaired cognitive function and hippocampal changes following chronic diazepam treatment in middle-aged mice. Front Aging Neurosci. (2021) 13:777404. doi: 10.3389/fnagi.2021.777404
238. Rasmussen LS, Steentoft A, Rasmussen H, Kristensen PA, Moller JT. Benzodiazepines and postoperative cognitive dysfunction in the elderly. ISPOCD group. International study of postoperative cognitive dysfunction. Br J Anaesth. (1999) 83:585–9. doi: 10.1093/bja/83.4.585
239. Butterworth JF IV, Mackey DC, Wasnick JD. Adjuncts to anesthesia. In: Butterworth JF IV, Mackey DC, Wasnick JD, editors. Morgan & Mikhail’s Clinical Anesthesiology, 7th ed. New York, NY: McGraw-Hill Education (2022).
241. Wang K, Wu M, Xu J, Wu C, Zhang B, Wang G, et al. Effects of dexmedetomidine on perioperative stress, inflammation, and immune function: systematic review and meta-analysis. Br J Anaesth. (2019) 123:777–94. doi: 10.1016/j.bja.2019.07.027
242. Weerink MAS, Struys M, Hannivoort LN, Barends CRM, Absalom AR, Colin P. Clinical pharmacokinetics and pharmacodynamics of dexmedetomidine. Clin Pharmacokinet. (2017) 56:893–913. doi: 10.1007/s40262-017-0507-7
243. Fu S, Zhao X, Li Y, Fan X, Huang Z. Dexmedetomidine alleviates hippocampal neuronal loss and cognitive decline in rats undergoing open surgery under sevoflurane anaesthesia by suppressing CCAAT/enhancer-binding protein beta. Eur J Neurosci. (2024) 59:36–53. doi: 10.1111/ejn.16193
244. Xu W, Zheng Y, Suo Z, Fei K, Wang Y, Liu C, et al. Effect of dexmedetomidine on postoperative systemic inflammation and recovery in patients undergoing digest tract cancer surgery: a meta-analysis of randomized controlled trials. Front Oncol. (2022) 12:970557. doi: 10.3389/fonc.2022.970557
245. Yu H, Kang H, Fan J, Cao G, Liu B. Influence of dexmedetomidine on postoperative cognitive dysfunction in the elderly: a meta-analysis of randomized controlled trials. Brain Behav. (2022) 12:e2665. doi: 10.1002/brb3.2665
246. Peng L, Liu S, Xu J, Xie W, Fang X, Xia T, et al. Metformin alleviates prolonged isoflurane inhalation induced cognitive decline via reducing neuroinflammation in adult mice. Int Immunopharmacol. (2022) 109:108903. doi: 10.1016/j.intimp.2022.108903
247. Henry CJ, Huang Y, Wynne A, Hanke M, Himler J, Bailey MT, et al. Minocycline attenuates lipopolysaccharide (LPS)-induced neuroinflammation, sickness behavior, and anhedonia. J Neuroinflammation. (2008) 5:15. doi: 10.1186/1742-2094-5-15
248. Cibelli M, Fidalgo AR, Terrando N, Ma D, Monaco C, Feldmann M, et al. Role of interleukin-1beta in postoperative cognitive dysfunction. Ann Neurol. (2010) 68:360–8. doi: 10.1002/ana.22082
249. Zhu Y, Yao R, Li Y, Wu C, Heng L, Zhou M, et al. Protective effect of celecoxib on early postoperative cognitive dysfunction in geriatric patients. Front Neurol. (2018) 9:633. doi: 10.3389/fneur.2018.00633
250. Wang YB, Chen Z, Li J, Shi J. Parecoxib improves the cognitive function of POCD rats via attenuating COX-2. Eur Rev Med Pharmacol Sci. (2019) 23:4971–9. doi: 10.26355/eurrev_201906_18088
251. Kamer AR, Galoyan SM, Haile M, Kline R, Boutajangout A, Li YS, et al. Meloxicam improves object recognition memory and modulates glial activation after splenectomy in mice. Eur J Anaesthesiol. (2012) 29:332–7. doi: 10.1097/EJA.0b013e3283534f56
252. Qiu LL, Pan W, Luo D, Zhang GF, Zhou ZQ, Sun XY, et al. Dysregulation of BDNF/TrkB signaling mediated by NMDAR/Ca(2+)/calpain might contribute to postoperative cognitive dysfunction in aging mice. J Neuroinflammation. (2020) 17:23. doi: 10.1186/s12974-019-1695-x
253. Cui RS, Wang K, Wang ZL. Sevoflurane anesthesia alters cognitive function by activating inflammation and cell death in rats. Exp Ther Med. (2018) 15:4127–30. doi: 10.3892/etm.2018.5976
254. Wang CM, Chen WC, Zhang Y, Lin S, He HF. Update on the mechanism and treatment of sevoflurane-induced postoperative cognitive dysfunction. Front Aging Neurosci. (2021) 13:702231. doi: 10.3389/fnagi.2021.702231
256. Dong W, Chen MH, Yang YH, Zhang X, Huang MJ, Yang XJ, et al. The effect of dexmedetomidine on expressions of inflammatory factors in patients with radical resection of gastric cancer. Eur Rev Med Pharmacol Sci. (2017) 21:3510–5.28829488
257. Persson NDA, Uusalo P, Nedergaard M, Lohela TJ, Lilius TO. Could dexmedetomidine be repurposed as a glymphatic enhancer? Trends Pharmacol Sci. (2022) 43:1030–40. doi: 10.1016/j.tips.2022.09.007
258. Benveniste H, Lee H, Ding F, Sun Q, Al-Bizri E, Makaryus R, et al. Anesthesia with dexmedetomidine and low-dose isoflurane increases solute transport via the glymphatic pathway in rat brain when compared with high-dose isoflurane. Anesthesiology. (2017) 127:976–88. doi: 10.1097/ALN.0000000000001888
Keywords: POCD, microglia, neuro-inflammation, glia, post-operative, cognitive dysfunction
Citation: Smith GT, Chen TJ, Shah NM, Agrest B and Grotticelli J (2024) Anesthesia-mediated neuroinflammatory sequelae in post operative cognitive dysfunction: mechanisms and therapeutic implications. Front. Anesthesiol. 3:1281034. doi: 10.3389/fanes.2024.1281034
Received: 21 August 2023; Accepted: 8 January 2024;
Published: 27 February 2024.
Edited by:
Mingqin Zhu, First Affiliated Hospital of Jilin University, ChinaReviewed by:
Afang Zhu, Peking Union Medical College Hospital (CAMS), ChinaJacob Raber, Oregon Health and Science University, United States
Hari Prasad Osuru, University of Virginia, United States
© 2024 Smith, Chen, Shah, Agrest and Grotticelli. This is an open-access article distributed under the terms of the Creative Commons Attribution License (CC BY). The use, distribution or reproduction in other forums is permitted, provided the original author(s) and the copyright owner(s) are credited and that the original publication in this journal is cited, in accordance with accepted academic practice. No use, distribution or reproduction is permitted which does not comply with these terms.
*Correspondence: Janet Grotticelli janet.grotticelli@touro.edu