- 1Engineering Department, Hospital Universitario de Canarias, Tenerife, Spain
- 2Chronical Diseases Research Functional Unit, Instituto de Salud Carlos III, Madrid, Spain
- 3RF Laboratory, Telecommunications General Direction and Audiovisual Communication Services Ordenation, Madrid, Spain
- 4Microbiology National Center, Instituto de Salud Carlos III, Madrid, Spain
- 5Telemedicine and Digital Health Research Unit, Instituto de Salud Carlos III, Madrid, Spain
- 6Environmental Health National Center, Instituto de Salud Carlos III, Madrid, Spain
Introduction: This study is an introduction to the empirical and impact evaluation of radiofrequency electromagnetic field (RF-EMF) radiation exposure in a healthcare environment, focusing on an indoor microenvironment. It explores the expression of various genes associated with cellular responses, cell proliferation, senescence, and apoptotic cell death. The assessment analyzes current personal mobile communications (2G-5G FR1), providing a clear understanding of RF-EMF exposure and compliance with regulatory limits.
Methods: The signals from different wireless communication systems at Hospital Universitario de Canarias (HUC) in Tenerife, Canary Islands, Spain, were examined in 11 locations. Four measurement campaigns were performed with frequency-selective exposimeters (PEMs) and an EME Spy 200 MVG, and experimental electric field values were compared as a long-term exposition. The frequency with the highest contribution (2.174 V/m) observed (1840 MHz) in UMTS was selected for biological effects evaluation.
Results: The study focuses on four locations with the highest exposure to communication systems (downlinks), analyzing the results to verify compliance with regulations that ensure the safety of patients, the general public, and healthcare workers. LTE B20 (DL), GSM+UMTS 900 (DL), GSM 1800 (DL), UMTS 2100 (DL), and LTE B7 (DL) exhibited relatively higher E/m values throughout the campaigns, and these values consistently remained below the ICNIRP reference levels, signifying a consistently low level of exposure. In addition, this work presents the biological effects on neural stem cells (NSCs) using 3D brain organoids (BOs) exposed to RF signals in a validated and commercial experimental setting: the Gigahertz Transverse Electromagnetic cell (GTEM). The GTEM allows for the creation of homogeneous field electromagnetic fields in a small, enclosed setting and guarantees exposure conditions in a wide range of frequencies. BOs are an in vitro 3D cell-culture technology that reproduces the cellular composition and structure of the developing brain. Analyzing the expression of several genes associated with cellular responses, cell proliferation, senescence, and apoptotic cell death, we found that exposure of BOs at 1840 MHz did not affect mRNA expression in brain genes related to apoptosis or senescence. However, a decrease in gene expression for cell proliferation and cell activity markers was observed during the differentiation stage of BOs.
Discussion: The discussion emphasizes the coexistence and evolution of various heterogeneous networks and services throughout the four measurement campaigns. Across all measured results, the levels of the obtained E-field were consistently well below the exposure limits set by internationally accepted standards and guidelines. These obtained values have been established in order to consider their potential effects on cell proliferation and cell activity, especially in differentiating biological organisms. Consequently, the results obtained and the methodology presented could serve as a foundational framework for establishing the basis of RF-EMF assessment in future heterogeneous 5G developments, particularly in the millimeter wave (mmWave) frequency range, where the forecast is for massive high-node density networks.
1 Introduction
The extremely intensive development of mobile (cellular) communications systems in healthcare centers has produced a new medical ecosystem limited by infrastructure deployment. It plays a pivotal role in determining anthropogenic electromagnetic pollution and influencing the public perception and acceptance of new technology (Solanas et al., 2014). The majority of electromagnetic field (EMF) sources are associated with wireless public information services. In smart health environments, heterogeneous wireless networks contribute to context awareness within healthcare centers. From a propagation standpoint, several recent studies evaluate RF-EMF exposure levels (González-Rubio et al., 2016; Celaya-Echarri et al., 2020; Celaya-Echarri et al., 2021), and dynamic campaigns employing personal exposimeters (PEM) have been conducted to assess RF-EMF levels. These studies focus on integral levels of electromagnetic background generated by spatially distributed base stations of 4G/5G systems. They illustrate the evolution of electromagnetic background generation across different frequency ranges, considering variations in area traffic capacity and base station service area sizes. Given that the majority of EMF sources are associated with wireless public information services, particularly in communication and broadcasting, the extensive development of mobile (cellular) communication systems (MC) positions them as the primary contributors to the electromagnetic microenvironment.
Several experimental investigations (Burgi et al., 2008; Gotsis et al., 2008; Troisi et al., 2008; Tomitsch et al., 2010; Rufo et al., 2011; Joseph et al., 2012; Rowley and Joyner, 2012; Ozdemir et al., 2014; Gajsek et al., 2015; Ibrani et al., 2017; Karpowicz et al., 2017) into the radio frequency electromagnetic background (EMB) have been conducted globally. These have primarily focused on the influence of second- and third-generation (2G/3G) MC systems and span numerous countries. Their findings consistently suggest that the intensity of EMB, even before the active deployment of fourth- (4G) and fifth- (5G) generation MC systems, approaches or reaches the maximum permissible levels (MPL) of 2.5.10 μW/cm2. This evaluation takes into consideration the long-term consequences associated with “non-thermal” effects (Grigoriev et al., 2019). There is a difference in approach to its protection (Grigoriev et al., 2020) and orientation to “thermal” recommendations (International Commission on Non-Ionizing Radiation Protection ICNIRP, 2020) which actually determines the limit of EMB intensity connected with the physical destruction of biological tissue. However, the effectiveness of such “passive” protection of populations from the effects of EMB in conditions of intensive implementation of 4G/5G has been questioned. Drawing on insights from (ITU, 2009; GSMA, 2014; ETSI, 2015; IMT, 2015; Rodriguez, 2015; ITU, 2017) and future predictions (Calvanese Strinati et al., 2019; Zhang et al., 2019), the anticipated progression from 4G to 5G and eventually to 6G raises concerns regarding the substantial growth of key parameters in the main MC systems, particularly in those crucial for safety, connection density (devices/km2), and area traffic capacity (Mbit/s/m2). These are expected to experience significant growth, potentially by many orders of magnitude. This growth raises reasonable doubts about the feasibility of compensating for the intensity of electromagnetic background (EMB) solely through advances in MC technologies and system solutions. In such circumstances, ensuring the electromagnetic safety of the population necessitates effective control and prediction of EMB levels. This involves considering all conceivable scenarios for the implementation of new-generation MC systems.
The public debate around the possible health effects of emitted radiation usually leads to changes in the behavior of individuals and the use of technology. Taking into account EM environmental evaluation, this study presents some of these biological effects assessed by analyzing the expression of certain genes associated with cellular responses, signaling processes, cell proliferation, and neural development in “organoid” culture derived from human neural stem cells.
In order to evaluate RFR effects on these organoids, we propose the use of a validated and commercial experimental setting—the Giga Hertz Transverse Electromagnetic cell (GTEM) (Ramos et al., 2021; Torres-Ruiz et al., 2024). GTEMs allow the creation of homogeneous electromagnetic fields in small, enclosed settings and guarantee exposure conditions in a wide range of frequencies. In GTEMs, uniformity volume may be determined according to the field homogeneity requirements of Annex B of IEC standard 61000-4-20:2022 (IEC Standard, 2022) for emission and immunity testing in transverse electromagnetic (TEM) waveguides and is thus a better choice for exposing biological systems (Tkalec et al., 2005; Vian et al., 2016).
Two-dimensional (2D) cultures are commonly used to study how radiation affects neural stem cells due to their ease of use and manipulation (Eghlidospour et al., 2017). However, these cultures do not adequately reflect the complexity of brain tissues and the interaction between cells in a three-dimensional (3D) environment. An organoid is an in vitro 3D cell-culture technology that reproduces the physiological and cellular composition of tissue and/or organ evaluation and presents a novel approach as a very useful tool. Specifically, brain organoids (BOs)—3D models of brain tissue generated from human pluripotent stem cells—provide a more realistic representation of brain structure and function, allowing for more precise and relevant studies on the effects of radiation on the brain (Lancaster and Knoblich, 2014; Paşca et al., 2015). Therefore, this work uses BOs to study how radiation affects some aspects related to cell proliferation, cell death, and immediate early gene expression in these cultures.
Guidelines established by the International Commission on Non-Ionizing Radiation Protection (International Commission on Non-Ionizing Radiation Protection ICNIRP, 2020) are based on recorded thermal effects, which are the most common. Exposure to non-thermal levels is considered safe. However, some studies suggest possible long-term adverse health effects from exposure to levels below those set by regulation, such as increased risk of cancer (Qin et al., 2022), headaches, sleep disorders, and effects on the immune or nervous systems (Cui et al., 2017). Although more research is needed to definitively determine the health risks of exposure to radio frequency electromagnetic fields, current regulations and safety guidelines establish exposure limits to protect public health, and health agencies continue to monitor scientific evidence in this field to update and adjust these regulations. However, it is also noted that many of these studies are limited and contradictory and that more research is needed to definitively determine the health risks of exposure to MRI-RFR, specially utilizing controlled environments that control for temperature and potency.
RFR exposure and the possible impact of RFR signals on human health have been the subject of extensive research. Possible negative effects have been observed on memory (Qin et al., 2022), cellular function (Cui et al., 2017), the immune (Benavides et al., 2023) and nervous systems (Chen et al., 2021), and sleep quality. However, positive effects have also been observed in the application of electromagnetic radiation in neuronal survival and differentiation (Urnukhsaikhan et al., 2016) and in the prevention of melanin-related diseases (Kim et al., 2022). These discrepant results are sometimes explained by different frequencies and/or exposure settings applied to biological models. Although some studies have shown no important relations between RFR exposure and human health, more research is needed to understand the effects and determine whether long-term exposure to 5G radiation has any negative effects on human health.
2 Materials and methods
In this study, the signals emitted by various wireless communication systems across 11 locations within Hospital Universitario de Canarias (HUC) were examined. The analysis was conducted in an indoor environment and involved comparison across four distinct measurement campaigns. Specifically, we focused on four selected locations (1, 4, 5, and 6) characterized by significant exposure to communication system downlinks. The evolution of results in these key locations was carefully scrutinized to assess compliance with regulations for safeguarding the safety of patients, the general public, and healthcare workers in these environments.
2.1 Electromagnetic microenvironment evaluation
The study comprised four campaigns dedicated to measuring the electric field (E-field) in HUC. The primary focus of these measurements was to evaluate wireless signals and determine their adherence to legally established exposure thresholds at mobile frequencies. The observed E-field background served as crucial data for assessing potential health effects and interactions with neighboring wireless networks, including devices emitting within the same frequency band. To ensure comprehensive coverage, 11 measurement locations were strategically chosen, taking into account base station information within a 500-m radius around HUC. These locations were identified based on data collected and documented by the Ministry of Digital Transformation (2023) (Figure 1). Measurement locations inside HUC are shown in Figure 2.
The measurements were conducted utilizing the MVG EME SPY 200 Personal E-field Exposimeter, which is a type of radiofrequency (RFR) frequency-selective exposure meter commonly called an exposimeter or PEM (personal E-field exposimeter). Measurements were taken every 5 s with a three-axial E-field probe that measures E-field V/m and calculates total exposures based on recorded samples in 20 predefined frequency bands in the 88 MHz—5850 MHz range. The measurement protocol differentiates uplink (UL) and downlink (DL) for mobile communications. The upper detection limit of measurement instrumentation is 6 V/m, while the sensitivity varies for different bands and is 0.005 V/m for most, excluding WiFi5G with a sensitivity of 0.01 V/m.
The PEMs utilized in this study were specifically designed for environmental surveillance, enabling the execution of radiofrequency (RFR) static measurement campaigns at designated locations. These devices monitor electromagnetic field (EMF) exposure over time in complex and heterogeneous environments. Numerous epidemiological studies (Gotsis et al., 2008; Troisi et al., 2008; Grigoriev et al., 2019; Grigoriev et al., 2020; International Commission on Non-Ionizing Radiation Protection ICNIRP, 2020) have utilized PEMs to assess and evaluate EMF exposure in such environments. To measure the multi-spectral distribution of radiofrequency (RF) energy and determine the signal strength of specific frequencies, an experimental protocol was employed with the PEM. Figure 3 illustrates the PEM’s capacity to measure 20 frequency bands, including both DL and UL communications. It is notable that this study excluded consideration of exposure from wireless handsets and UL communications [LTE B12 (UL), LTE B13 (UL), LTE B4 (UL), and LTE B7 (UL)] as discerning the E-field due to UL from individuals’ phones proves challenging. The analysis focuses on the DL measures of various communication systems, including LTE B20 DL, GSM+UMTS 900 DL, GSM 1800 DL, UMTS 2100 DL, LTE B7 DL, WIFI 2G, WIFI 5G, and DECT 6.0. The measurements were conducted in February 2022, June 2022, December 2022, and June 2023.
A multi-band scenario setup was chosen, employing an E-field sampling rate of 4 s in the SPY 200 PEM. The procedure encompasses five primary steps. Initially, the locations of base stations for each campaign were determined (Ramos et al., 2021). Subsequently, specific measurement locations within the HUC contour were identified. The PEM data were then collected from the 11 selected locations. Following data collection, the fourth step involved the analysis and presentation of four locations with higher E-levels registered in both campaigns. The frequency exhibiting the highest observed level was utilized as the E-field level for organoid exposure. A significant portion of the measurements fell below the lower detection limit of the PEM (0.005 V/m) in each individual frequency band, leading to censorship. The placement of the PEM hardware device aimed to mitigate underestimation by locating it away from the staff member’s body, minimizing body-shielding effect underestimation (only 1 V/m of body-shielding effect when another user/scatterer is located between the radiating antenna and the receiver) (de Miguel-Bilbao et al., 2019). After sample collection, the dosimeter generated graphics depicting the electric field vs. time (Figure 4A) and frequency (Figure 4B). Approximately 400 realistic exposure samples were generated in 30 min to ensure statistically relevant results.
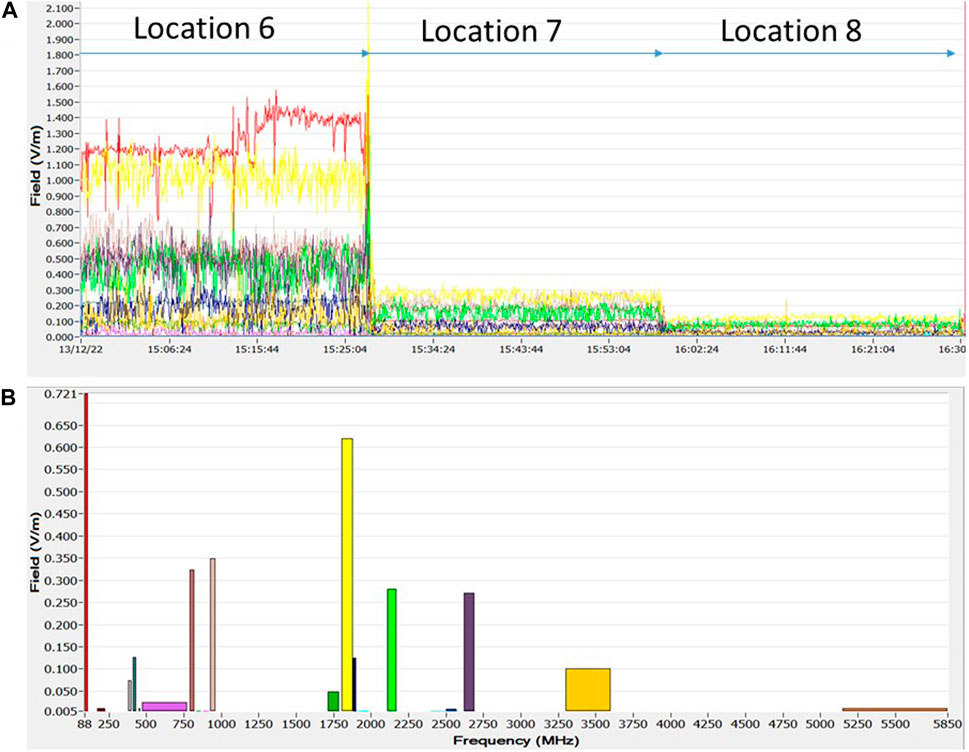
Figure 4. Graphics provided by dosimeter: (A) Electric field vs. time; (B) Electric field vs. frequency.
The analysis utilized median and maximum values extracted from specific datasets of E-field strength, denoted in volts per meter (V/m). This approach aimed to assess the impact of exposure for each measured frequency band outlined in Table 1, including LTE B20 (DL), GSM+UMTS 900 (DL), GSM 1800 (DL), UMTS 2100 (DL), and LTE B7 (DL).
2.2 Experimental setup and organoid exposure conditions
Neural stem cell organoid exposure was performed in GTEM exposure cell GTEM ETS-LINDGREN 5402 coupled with RF Signal Generator Rohde & Schwarz SMBV 100B (8 kHz–6 GHz) to obtain the GSM 1840 MHz (DL) signal with 2.1 V/m during exposure. The generator is connected to a power amplifier ZHL-42 W (10 MHz–4200 MHz). This set up is presented in Figure 5. Control organoids were located outside the chamber and were evaluated for background exposure using a MVG EME SPY 200 Dosimeter. Temperature was maintained at 25 °C both inside and outside the GTEM during the whole exposure period.
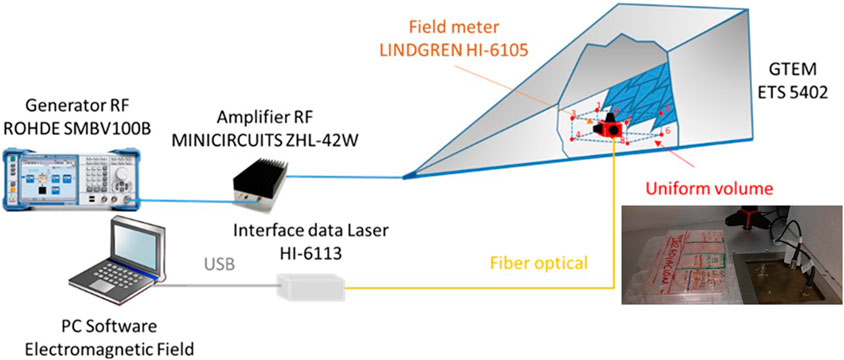
Figure 5. Experimental setup. Radiation exposure chamber GTEM ETS-LINDGREN 5402 (DC-20 GHz), generator RF Rohde & Schwarz SMBV100B (8 kHz–6 GHz), and amplifier RF (AMP) ZHL-42 W (10 MHz—4200 MHz). Plate configuration inside chamber and field meter ETS-Lindgren HI-6105 (100 kHz—6 GHz).
The purpose of this cell was to create a uniform electromagnetic field that is the most important factor in a GTEM cell. To verify its field uniformity, it was measured by Isotropic Probe (IP) ETS-Lindgren HI-6105 (100 kHz—6 GHz). The exposure chamber was designed based on a field uniformity check based on planar measurements. The field conditions of uniformity inside GTEM are defined for the different frequencies as per Annex B of IEC standard 61000-4-20:2022 (IEC Standard, 2022). This process has been presented in in-depth in previous publications by authors Ramos et al. (2021); IEC Standard, 2022.
To select the exposure level, a field value 2.15 V/m at a frequency of 1840 MHz was selected inside the uniformity volume. The level of the RF generator to obtain a field level of 2.15 V/m was −32.6 dBm.
Biological-model control samples were assessed under baseline exposure conditions outside the GTEM. This evaluation was conducted using the MVG EME SPY 200 Dosimeter covering a frequency range of 88–5850 MHz. The dosimeter allowed for the isotropic recording of the electric field across various preselected frequency sub-bands. Additionally, it captured the total electric field for the entire measurement range, exemplified by wideband recordings spanning 27 MHz–6 GHz (Figures 5, 6).
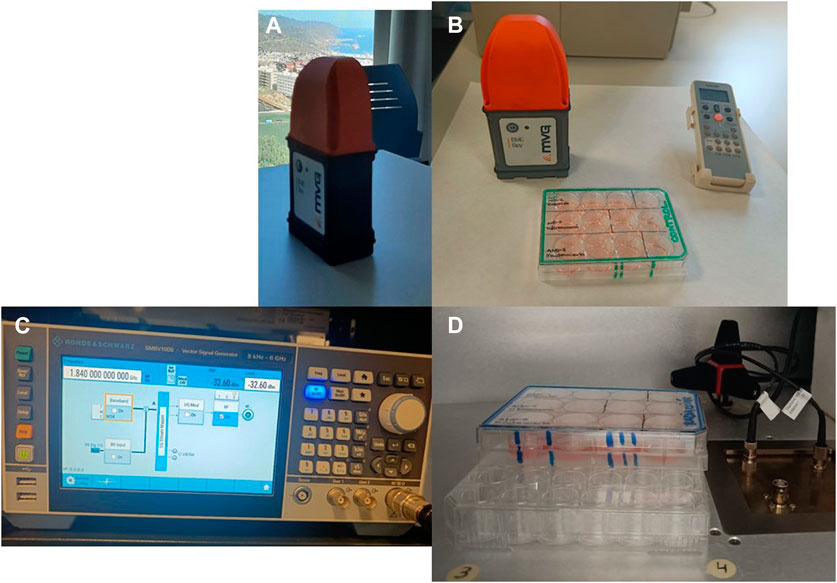
Figure 6. Experiment environment. (A). Dosimetric evaluation at HUC. (B) Radiofrequency generator. (C) Control samples evaluated under background exposition. (D) Culture exposition inside GTEM cell.
2.3 Organoid evaluation
2.3.1 Generation of human brain organoids from AND-2 and the radiation process
The hESC cell line AND-2 was obtained from the Granada Stem Cell Biobank (ISCIII, Spain). The brain organoids generated a protocol adapted from previous ones (Paşca et al., 2015; Lancaster and Knoblich, 2014) through the phases of neural induction, differentiation, and maintenance. BOs at different stages of development (neural induction, differentiation, and maintenance) were radiated at 1840 MHz for 1 h at RT for 5 days. This was a first approximation to short- and long-term exposure of the organoids, taking into account their dimensions and evolutionary time. They were subsequently processed for analysis and compared with the respective controls.
In all cases, the cultures were maintained at 37 °C in an atmosphere of 5% CO2 and 95% relative humidity in an incubator (Forma).
2.3.2 RNA extraction and RT-q-PCR
BOs were collected and carefully washed with PBS, and lysis buffer was added. They were mechanically dissociated with the pipette and stored at −20°C until RNA extraction.
Total RNA was extracted with the RNeasy Mini kit (QIAGEN) as per manufacturer’s instructions and treated with DNAses. We reverse-transcribed 1 μg of total RNA using SuperScriptIII-RT (Life Technologies), following this thermal program: 25 °C 10 min, 50 °C 60 min, and 75 °C 10 min.
Relative cDNA expression by RT-qPCR was done using PowerUpTM SYBRTM Green Master Mix (Applied Biosystems). We amplified 10 ng of total cDNA using these primers for the corresponding human genes: MKI67 (f: 5′-TGACCCTGATGAGAAAGCTCAA-3’; r: 5′-CCCTGAGCAACACTGTCTTTT-3′), BAX (f: 5′-AGCAAACTGGTGCTCAAGG-3’; r: 5′-CTTGGATCCAGCCCAACA-3′), BCL-2 (f: 5′-TTGACAGAGGATCATGCTGTACT-3’; r: 5′-ATCTTTATTTCATGAGGCACGTT-3′), C-FOS (f: 5′-CTACCACTCACCCGCAGACT; r: 5′-AGGTCCGTGCAGAAGTCCT), C-JUN (f: 5′-CCAAAGGATAGTGCGATGTTT; r: 5′-CTGTCCCTCTCCACTGCAAC), CDKN1A (f: 5′-AGGTGGACCTGGAGACTCTC-3’; r: 5′-CTTCCTGTGGGCGGATTAGG-3′), and TBP as the house-keeping gene (f: 5′-GAGCTGTGATGTGAAGTTTCC-3’; r: 5′-TCTGGGTTTGATCATTCTGTAG-3′).
2.3.3 Statistical analysis
The data obtained are presented as the mean ± SD. Statistical analyses were accomplished using GraphPad Prism 6.0. Mean values were compared, and we consider p-values <0.05 to be statistically significant (*p< 0.05).
3 Results
3.1 Electromagnetic survey
In total, our measurement campaign collected around 7500 RF-EMF samples. In this section, we present the comparative analysis based on frequency-selective measurement of the E-field in the 88 MHz–6 GHz frequency range. To better understand the impact of each technology and their contribution to the total exposure, this section presents the most significant bands that caused relatively higher V/m values: LTE B20 (DL), GSM+UMTS 900 (DL), GSM 1800 (DL), UMTS 2100 (DL), and LTE B7 (DL). All presented higher levels in the fourth campaign and did not in any case exceed the ICNIRP reference levels, remaining significantly lower.
A PEM generates numerical datasheets in Excel format (MS Office). Initial visual inspection of the measurement results (Figure 4) indicated that certain frequency bands did not significantly contribute to the evaluated exposure, with cellular phones being the most prominent contributors, along with DECT frequency bands. Wi-Fi 2G and 5G bands were excluded from consideration due to their consistently low registered levels. It is worth noting that PEMs were positioned at a distance from routers and that no individuals were in proximity during measurements. Measurement results that fell within the sensitivity range of the employed devices are omitted. The distinctive features of the experimental results are then compared to total E-field measurements for RF systems during Campaign A in February 2022, Campaign B in June 2022, Campaign C in December 2022, and Campaign D in June 2023, respectively.
Figures 7–10 depict the consideration of distances to electromagnetic radiation (EMR) sources and the evolving landscape of radiocommunication services along with their physical infrastructure.
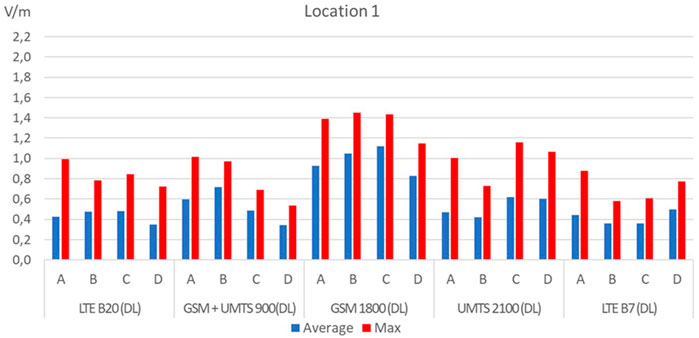
Figure 7. Location 1. Median, maximum values E-field measurements for campaigns (A), (B), (C), and (D).
Consistent with earlier research findings, similar conclusions emerge. Generally, radiofrequency electromagnetic field (RF-EMF) exposure levels across a wide frequency spectrum remain very low in typical daily life situations. These exposure levels consistently fall well below 1 V/m when compared to the existing international limits for RF-EMF exposure (Grigoriev et al., 2020).
As can be observed in Figures 7–10, the location that registered the highest E-field level was Location 6. The frequency with the highest contribution GSM 1800 (DL) and 2.174 V/m observed (1840 MHz) was selected for biological effects evaluation.
The radiation levels to which the control samples were exposed never exceeded 0.36 V/m. In the 1840-MHz frequency band, the maximum value recorded throughout all exposures was 0.005 V/m and so did not exceed the PEM detection threshold.
3.2 Impact on organoids of 1840-MHz RF exposure
The appearance and morphology of the BOs were not altered by the applied radiation (Figure 11A). The molecular analysis by quantitative real-time PCR detected no significant variations in gene expression associated with apoptosis (BAX, BCL-2) or cellular senescence (CDKN1A). However, a significant decrease in the modulation of genes involved in proliferation and cellular cycle (MKI67 and CCND1) and cellular activity (C-FOS) was observed in radiated BOs with respect to the control group during the differentiation stage, with no changes in the other stages presented in (Figures 11B–G). This suggests that more attention should be given to the potential effects of RF exposure on early brain development.
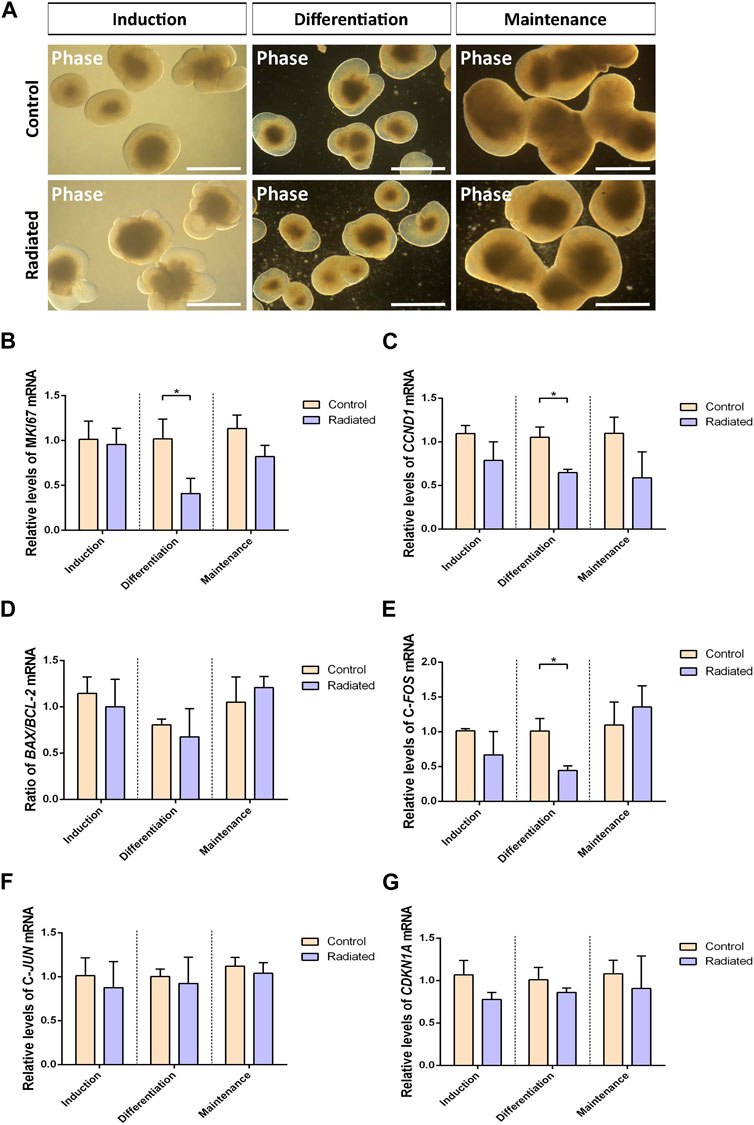
Figure 11. Effects of radiation on BOs. (A) Phase contrast images of control and radiated BOs at different developmental stages. Scale bar 500 µm. Relative mRNA expression levels of (B) MKI67, (C) CCND1, (D) BAX/BCL-2 (E) C-FOS, (F) C-JUN, and (G) CDKN1A obtained by RT-qPCR in BOs. Results are shown as mean ± SD at least of three different experiments (n = 3). Statistical analysis performed between control and radiated groups (*p<0.05).
4 Discussion
4.1 Electromagnetic environment
The most notable frequency bands contributing to relatively higher E/m values were LTE B20 (DL), GSM+UMTS 900 (DL), GSM 1800 (DL), UMTS 2100 (DL), and LTE B7 (DL). Among these, GSM 1800 (DL) exhibited the most significant radiation exposure in all measured locations, with higher levels observed during the third campaign. It is important to note that, as explained in Section 2, the measurements presented here do not account for the impact of radiation exposure from individual cell handsets. These findings align with the existing literature, including a recent study across five EU countries (Birks et al. 2018), research in five different urban areas in Belgium (Velghe et al., 2019), work presented in (Ramos et al., 2023) in outdoor healthcare environment, and a study conducted in public shopping malls (Celaya-Echarri et al., 2021).
Table 2 provides a summary of the RF-EMF exposure levels obtained from the six selected locations, presenting percentage values compared to the lowest reference level outlined by ICNIRP guidelines for the respective frequency band (ranging from 36.37 V/m to 61 V/m). Additionally, it includes percentage values for medium and maximum exposures.
For the user category, the location exhibiting the highest peaks is Location 6, registering a maximum of 2.2 V/m (equivalent to 0.029% of the ICNIRP reference level). Notably, all maximum peaks were observed within LTE (DL) frequency bands across the four campaigns.
The evolution of the infrastructures observed by the Ministery of Digital Transformation (2023) is the provision of service in 5G technology at frequencies of 700, 3500, and 3400 MHz. As 4G services decreased over time, there was an increase in 5G. At the time of this study, there were 43 3700 MHz stations, 54 700 MHz stations, and four 3500 MHz stations. The 3500-MHz band is not registered in the SPY 200 and is one of the 5G bands in increasing use. The cells were adjusted depending on usage and the distance of users. Lower levels may indicate network optimization.
The progression and densification of base stations (BS) can potentially elevate exposure levels. However, it is crucial to emphasize that, in any scenario, these levels do not surpass 0.5% of the ICNIRP reference levels compared to the threshold values established by prevailing standards and guidelines. This observation is significant in preventing electromagnetic interference to medical equipment, mitigating potential harm to workers, monitoring exposure levels within the workforce and the public at large, and ensuring that emission levels remain below recommended thresholds.
4.2 Organoid development
The effects of the level of cell proliferation, apoptotic cell death, and senescence were analyzed, which is useful for preventing potential damage to the general public (including workers) and biological effects by means of organoid evaluation. This study presents a novel approach to wireless technology effects produced at frequencies where radiation absorption by tissues is higher and the assessment of non-thermal effects or system alterations.
Here, we used human BOs as a model to study some of the possible molecular effects of RF exposure during brain development. We found that an 1840-MHz RF—widely used in mobile phone communication—has no effect on senescence or the apoptosis of developing BOs, with no changes in the expression of the apoptosis- (BAX, BCL-2) or senescence (CDKN1A)-related genes. These data are from studies in different types of NSCs (Eghlidospour et al., 2017; Chen et al., 2021; Velghe et al., 2019; Ramos et al., 2023).
On the other hand, the cell cycle and proliferation of NSCs is related to the self-renewal capacity of the cells, which is of great importance in maintaining the total number of NSCs for generating various types of neurons and glia during brain development. Our data show that RF exposure decreases the expression of genes related to proliferation and cell cycle (MKI67 and CCND1) and activity (C-FOS) in differentiating BOs without changes in the other stages of induction and maturation/maintenance of these cultures. This suggests that the effects of RF on BOs depend on the developmental stage of the BOs. These data differ from those by (Eghlidospour et al., 2017; Chen et al., 2021; An et al., 2023; Cruz-Mendoza et al., 2022) in NSCs. This discrepancy may be due to the different cell models and the durations and frequencies of exposure.
5 Conclusion
This study offers a comprehensive RF-EMF exposure assessment conducted through four campaigns in a hospital in Tenerife, utilizing a personnel dosimeter for measurements. The results highlight the most notable radiation exposure in the GSM 1800 frequency bands, particularly in three locations near base stations.
The measurements were conducted across 11 distinct outdoor locations in four campaigns, considering areas accessible to both the general public and workers. The most significant radiation exposure was observed in the LTE downlink (DL) frequency bands for the four locations near base stations (Ministery of Digital transformation, 2023) within a 500-m radius.
Throughout the campaigns, the exposure levels which indicated total inter-system exposure at the same moment (worst-case scenario) consistently remained well below the reference levels established by the ICNIRP (0.029% of ICNIRP max. reference level). The evolution observed across campaigns showed a slight increase in these levels, correlated with a higher number of base stations, increased traffic, and modifications in the structure of radiocommunication services and their physical infrastructure.
Although the measurements were conducted only for current wireless communication technologies, the results and methodology can be extended to form the RF-EMF assessment basis for future 5G FR2 developments in the millimeter-wave frequency range. This is particularly relevant for upcoming massive high-node user density networks. Monitoring exposure levels amongst the workforce and the general public serves as a preventive measure against electromagnetic interference of medical equipment and safeguards against potential harm to the public, including workers, and ensures that emission levels remain below recommended thresholds (Valbonesi et al., 2018).
This selected frequency has been used as a biological testing platform to evaluate developmental changes in organoids as a result of RFR exposure during early development at the level of cell death/survival, cell proliferation, and phenotype specification. Organoids present an excellent model of potential health effects. Together, these results improve our understanding of the effects of RF exposure on the development of human BOs and the potential adverse effects and mechanisms of RF exposure on the developing brain. Given the wide use of mobile communications in everyday life, the question of whether RF exposure affects brain development is a major concern.
Data availability statement
The original contributions presented in the study are included in the article/Supplementary material; further inquiries can be directed to the corresponding author.
Author contributions
JH: conceptualization, formal analysis, methodology, resources, validation, writing–original draft, and writing–review and editing. AR: data curation, investigation, writing–original draft, and writing–review and editing. SS: data curation, software, and writing–original draft. RC: data curation, investigation, and writing–original draft. OS: conceptualization, formal analysis, investigation, methodology, resources, supervision, writing–original draft, and writing–review and editing. PP-R: data curation, software, and writing–original draft. PbM: data curation, software, and writing–original draft. LR: software and writing–original draft. PtM: data curation, formal analysis, investigation, and writing–original draft. IL: conceptualization, data curation, formal analysis, investigation, methodology, resources, supervision, validation, writing–original draft, and writing–review and editing. VL-A: conceptualization, formal analysis, investigation, methodology, resources, supervision, validation, visualization, writing–original draft, and writing–review and editing. MT-R: conceptualization, investigation, methodology, resources, validation, writing–original draft, and writing–review and editing. VF: data curation, methodology, software, and writing–original draft. VR: conceptualization, data curation, funding acquisition, investigation, methodology, project administration, resources, supervision, validation, visualization, writing–original draft, and writing–review and editing.
Funding
The author(s) declare that financial support was received for the research, authorship, and/or publication of this article. This work was supported at Instituto de Salud Carlos III project “(PI19CIII/00033) TMPY 508/19” “Metrics development for electromagnetic safety assessment in healthcare centers in the context of 5G” from the Sub-Directorate-General for Research Assessment and Promotion. This research was funded by the Grant PID2021-126715OB-I00 funded by MCIN/AEI/10.13039/501100011033 and by “ERDF A way of making Europe,” and by a grant of the Instituto de Salud Carlos III (ISCIII) PI22CIII/00055.
Acknowledgments
The authors especially acknowledge, for his guidance and all contributions, Dr. José Carlos Fernandez de Aldecoa at Consejo Consultivo de SEEIC-Sociedad Española de Electromedicina e Ingeniería Clínica, and David Rubio from the Secretary of State for Digital Progress, General Directorate of Telecommunications and Technologies of Information (Madrid, Spain) for his contribution in performing measurements in the GTEM chamber.
Conflict of interest
The authors declare that the research was conducted in the absence of any commercial or financial relationships that could be construed as a potential conflict of interest.
Publisher’s note
All claims expressed in this article are solely those of the authors and do not necessarily represent those of their affiliated organizations or those of the publisher, the editors, and the reviewers. Any product that may be evaluated in this article, or claim that may be made by its manufacturer, is not guaranteed or endorsed by the publisher.
References
An, G., Jing, Y., Zhao, T., Zhang, W., Guo, L., Guo, J., et al. (2023). Quantitative proteomics reveals effects of environmental radiofrequency electromagnetic fields on embryonic neural stem cells. Electromagn. Biol. Med. 42 (2), 41–50. doi:10.1080/15368378.2023.2243980
Benavides, R. A. S., Leiro-Vidal, J. M., Rodriguez-Gonzalez, J. A., Ares-Pena, F. J., and López-Martín, E. (2023). The HL-60 human promyelocytic cell line constitutes an effective in vitro model for evaluating toxicity, oxidative stress and necrosis/apoptosis after exposure to black carbon particles and 2.45 GHz radio frequency. Sci. Total Environ. 867, 161475. doi:10.1016/j.scitotenv.2023.161475
Birks, L. E., Struchen, B., Eeftens, M., van Wel, L., Huss, A., Gajšek, P., et al. (2018). ‘Spatial and temporal variability of personal environmental exposure to radio frequency electromagnetic fields in children in Europe. Environ. Int. 117, 204–214. doi:10.1016/j.envint.2018.04.026
Burgi, A., Theis, G., Siegenthaler, A., and Roosli, M. (2008). Exposure modeling of high-frequency electromagnetic fields. J. Expo. Sci. Environ. Epidemiol. 18, 183–191. doi:10.1038/sj.jes.7500575
Calvanese Strinati, E., Barbarossa, S., Gonzalez-Jimenez, J. L., Ktenas, D., Cassiau, N., Maret, L., et al. (2019). 6G: the next frontier: from holographic messaging to artificial intelligence using subterahertz and visible light communication. IEEE Veh. Technol. Mag. 14 (3), 42–50. doi:10.1109/mvt.2019.2921162
Celaya-Echarri, M., Azpilicueta, L., Karpowicz, J., Ramos, V., Lopez-Iturri, P., and Falcone, F. (2020). From 2G to 5G spatial modeling of personal RF- EMF exposure within urban public trams. IEEE Access 8, 100930–100947. doi:10.1109/ACCESS.2020.2997254
Celaya-Echarri, M., Azpilicueta, L., Ramos, V., Lopez-Iturri, P., and Falcone, F. (2021). Empirical and modeling approach for environmental indoor RF-EMF assessment in complex high-node density scenarios: public shopping malls case study. IEEE Access 9, 46755–46775. doi:10.1109/ACCESS.2021.3067852
Chen, C., Ma, Q., Deng, P., Lin, M., Gao, P., He, M., et al. (2021). 1800 MHz radiofrequency electromagnetic field impairs neurite outgrowth through inhibiting EPHA5 signaling. Front. Cell Dev. Biol. 9, 657623. doi:10.3389/fcell.2021.657623
Cruz-Mendoza, F., Jauregui-Huerta, F., Aguilar-Delgadillo, A., García-Estrada, J., and Luquin, S. (2022). Immediate early gene c-fos in the brain: focus on glial cells. Brain Sci. 12, 687. doi:10.3390/brainsci12060687
Cui, M., Ge, H., Zhao, H., Zou, Y., Chen, Y., and Feng, H. (2017). Electromagnetic fields for the regulation of neural stem cells. Stem Cells Int. 2017, 1–16. doi:10.1155/2017/9898439
de Miguel-Bilbao, S., and Karpowicz, J. (2019). Victoria Ramos study of body position dependence on human exposure in the 2.4 GHz band” Proceedings of the International symposium and exhibition on electromagnetic compatibility EMC EUROPE 2019. Barcelona, Spain.
Eghlidospour, M., Ghanbari, A., Mortazavi, S. M. J., and Azari, H. (2017). Effects of radiofrequency exposure emitted from a GSM mobile phone on proliferation, differentiation, and apoptosis of neural stem cells. Anat. Cell Biol. 50 (2), 115–123. doi:10.5115/acb.2017.50.2.115
Etsi, (2015). 3GPP TS 36.141 version 12. https://www.etsi.org/deliver/etsi_ts/136100_136199/136141/12.05.00_60/ts_136141v120500p.pdf.
Gajsek, P., Ravazzani, P., Wiart, J., Grellier, J., Samaras, T., and Thuroczy, G. (2015). Electromagnetic field exposure assessment in Europe radiofrequency fields (10MHz–6GHz). J. Expo. Sci. Environ. Epidemiol. 25, 37–44. doi:10.1038/jes.2013.40
González-Rubio, J., Nájera, A., and Arribas, E. (2016). Comprehensive personal RF-EMF exposure map and its potential use in epidemiological studies. Environ. Res. 149, 105–112. doi:10.1016/j.envres.2016.05.010
Gotsis, A., Papanikolaou, N., Komnakos, D., Yalofas, A., and Constantinou, P. (2008). Non-ionizing electromagnetic radiation monitoring in Greece. Ann. Telecommun. 63, 109–123. doi:10.1007/s12243-007-0006-1
Grigoriev, O., Goshin, M., Prokofyeva, А., and Alekseeva, V. (2019). Features of national policy in approaches to electromagnetic field safety of radio frequencies radiation in different countries. Hyg. sanitation 98 (11), 1184–1190. doi:10.18821/0016-9900-2019-98-11-1184-1190
Grigoriev, O. A., Nikitina, V. N., Nosov, V. N., Pekin, A. V., Alekseeva, V. A., and Dubrovskaya, E. N. (2020). Electromagnetic safety of the population. National and international standards for electromagnetic fields of the radio frequency range. ЗДОРОВЬЕ НАСЕЛЕНИЯ И СРЕДА ОБИТАНИЯ - ЗНиСО/ PUBLIC HEALTH LIFE Environ. 10 (331), 28–33. doi:10.35627/2219-5238/2020-331-10-28-33
Gsma, (2014). Understanding 5G: perspectives on future technological advancements in mobile GSMA Intelligence. www.gsmaintelligence.com.
Ibrani, M., Hamiti, E., Ahma, L., Halili, R., and Dragusha, B. (2017). “Comparative analysis of downlink signal levels emitted by GSM 900, GSM 1800, UMTS, and LTE base stations,” in Proceedings of the 16th annual mediterranean ad hoc networking workshop (Budva, Montenegro).
IEC Standard (2022). Electromagnetic compatibility (EMC) - Part 4-20: testing and measurement techniques Emission and immunity testing in transverse electromagnetic (TEM) waveguides. https://webstore.iec.ch/preview/info_iec61000-4-20%7Bed3.0%7Db.pdf.
Imt, (2015). IMT Vision framework and overall objectives of the future development of IMT for 2020 and beyond. https://www.itu.int/dms_pubrec/itu-r/rec/m/R-REC-M.2083-0-201509-I!!PDF-E.pdf.
International Commission on Non-Ionizing Radiation Protection ICNIRP (2020). Guidelines for limiting exposure to electromagnetic Fields (100 kHz to 300 GHz). Health Phys. 118, 483–524. doi:10.1097/HP.0000000000001210
International Electrotechnical Commission (2010). Electromagnetic compatibility (EMC) Part 4-20: testing and measurement techniques Emission and immunity testing in transverse electromagnetic (TEM) waveguides. International Electrotechnical Commission, London, UK,
Itu, (2009). Guidelines for evaluation of radio interface technologies for IMT-Advanced. https://www.itu.int/pub/R-REP-M.2135.
Itu, (2017). Guidelines for evaluation of radio interface technologies for IMT-2020. https://www.itu.int/dms_pub/itu-r/opb/rep/R-REP-M.2412-2017-PDF-E.pdf.
Joseph, W., Verloock, L., Goeminne, F., Vermeeren, G., and Martens, L. (2012). Assessment of RF exposures from emerging wireless communication technologies in different environments. Health Phys. 102 (2), 161–172. doi:10.1097/hp.0b013e31822f8e39
Karpowicz, J., Miguel-Bilbao, S., Ramos, V., Falcone, F., Gryz, K., Leszko, W., et al. (2017). The evaluation of stationary and mobile components of radiofrequency electromagnetic exposure in the public accessible environment. Proc. Intern. Symp. EMC Eur. 4-8, 4. doi:10.1109/EMCEurope.2017.8094751
Kim, K., Lee, Y. S., Kim, N., Choi, H., and Lim, K. M. (2022). 5G electromagnetic radiation attenuates skin melanogenesis in vitro by suppressing ROS generation. Antioxidants 11 (8), 1449. doi:10.3390/antiox11081449
Lancaster, M. A., and Knoblich, J. A. (2014). Generation of cerebral organoids from human pluripotent stem cells. Nat. Protoc. 9, 2329–2340. doi:10.1038/nprot.2014.158
Ministery of Digital transformation, (2023). Mobile stations in Spain. https://geoportal.minetur.gob.es/VCTEL/vcne.do.
Ozdemir, A. R., Alkan, M., and Gulsen, M. (2014). Time dependence of environmental electric field measurements and analysis of cellular base stations. IEEE Electromagn. Compat. Mag. 3, 43–48. doi:10.1109/memc.2014.6924327
Paşca, A. M., Sloan, S. A., Clarke, L. E., Tian, Y., Makinson, C. D., Huber, N., et al. (2015). Functional cortical neurons and astrocytes from human pluripotent stem cells in 3D culture. Nat. Methods 12, 671–678. doi:10.1038/nmeth.3415
Qin, T. Z., Wang, X., Du, J. Z., Lin, J. J., Xue, Y. Z., Guo, L., et al. (2022). Effects of radiofrequency field from 5G communications on the spatial memory and emotionality in mice. Int. J. Environ. Health Res. 34, 316–327. doi:10.1080/09603123.2022.2149708
Ramos, V., Febles, V. M., Suárez, S. D., and Rabassa, L. E. (2023). Exposure in a public health research environment” 2023 international symposium on electromagnetic compatibility – EMC Europe EMC EUROPE 2023. Poland, Europe EMC. doi:10.1109/EMCEurope57790.2023.10274153
Ramos, V., Marina, P., Torres-Ruiz, M., Suárez, O. J., López, V., Liste, I., et al. (2021). Experimental radiation system for effects assessment in biological in vitro and in vivo models. Proceedings of the 2021 photonics & electromagnetics research symposium (PIERS), Hangzhou, China, 2800–2807. doi:10.1109/PIERS53385.2021.9695112
Rowley, J. T., and Joyner, K. H. (2012). Comparative international analysis of radiofrequency exposure surveys of mobile communication radio base stations. J. Expo. Sci. Environ. Epidemiol. 22, 304–315. doi:10.1038/jes.2012.13
Rufo, M., Paniagua, J., Jimenez, A., and Antolín, A. (2011). Exposure to high-frequency electromagnetic fields (100 KHz–2 GHz) in Extremadura (Spain). www.health-physics.com. doi:10.1097/HP.0b013e31821fd1ec
Solanas, A., Patsakis, C., Conti, M., Vlachos, I., Ramos, V., Falcone, F., et al. (2014). Smart health: a context-aware health paradigm within smart cities. IEEE Commun. Mag. 52 (8), 74–81. doi:10.1109/MCOM.2014.6871673
Tkalec, M., Malarić, K., and Pevalek-Kozlina, B. (2005). Influence of 400, 900, and 1900 MHz electromagnetic fields on Lemna minor growth and peroxidase activity. Bioelectromagnetics 26, 185–193. doi:10.1002/bem.20104
Tomitsch, J., Dechant, E., and Frank, W. (2010). Survey of electromagnetic field exposure in bedrooms of residences in lower Austria. Bioelectromagnetics 31, 200–208. doi:10.1002/bem.20548
Torres-Ruiz, M., Suárez, O. J., López, V., Marina, P., Sanchis, A., Liste, I., et al. (2024). Effects of 700 and 3500 MHz 5G radiofrequency exposure on developing zebrafish embryos. Sci. Total Environ. 915, 169475. doi:10.1016/j.scitotenv.2023.169475
Troisi, F., Boumis, M., and Grazioso, P. (2008). The Italian national electromagnetic field monitoring network. Ann. Telecommun. 63, 97–108. doi:10.1007/s12243-007-0011-4
Urnukhsaikhan, E., Cho, H., Mishig-Ochir, T., Seo, Y. K., and Park, J. K. (2016). Pulsed electromagnetic fields promote survival and neuronal differentiation of human BM-MSCs. Life Sci. 151, 130–138. doi:10.1016/j.lfs.2016.02.066
Valbonesi, S., Carciofi, C., and Bisceglia, B. (2018). Precautionary principle application and 5G development. Proceedings of the IEEE 29th Annu. Int. Symposium Personal, Indoor Mob. Radio Commun. (PIMRC), Bologna, Italy, 1192–1196. doi:10.1109/PIMRC.2018.8580997
Velghe, M., Joseph, W., Debouvere, S., Aminzadeh, R., Martens, L., and Thielens, A. (2019). Characterisation of spatial and temporal variability of RF-EMF exposure levels in urban environments in Flanders, Belgium. Environ. Res. 175, 351–366. doi:10.1016/j.envres.2019.05.027
Vian, A., Davies, E., Gendraud, M., and Bonnet, P. (2016). Plant responses to high frequency electromagnetic fields. BioMed Res. Int. 2016, 1–13. doi:10.1155/2016/1830262
Keywords: RF measurement, E-field strength distribution, organoids, development, electromagnetic hazard, exposure levels
Citation: Hernández JA, Rosca A, Suárez S, Coronel R, Suarez OJ, Peran-Ramos P, Marina P, Rabassa LE, Mateos P, Liste I, López-Alonso V, Torres-Ruiz M, Febles VM and Ramos V (2024) Background levels and brain organoid impact of RF field exposure in a healthcare environment. Front. Antennas Propag. 2:1357649. doi: 10.3389/fanpr.2024.1357649
Received: 18 December 2023; Accepted: 20 February 2024;
Published: 26 March 2024.
Edited by:
Francesca Benassi, University of Bologna, ItalyReviewed by:
Jianfeng Zheng, University of Houston, United StatesBeadaa Mohammed, The University of Queensland, Australia
Copyright © 2024 Hernández, Rosca, Suárez, Coronel, Suarez, Peran-Ramos, Marina, Rabassa, Mateos, Liste, López-Alonso, Torres-Ruiz, Febles and Ramos. This is an open-access article distributed under the terms of the Creative Commons Attribution License (CC BY). The use, distribution or reproduction in other forums is permitted, provided the original author(s) and the copyright owner(s) are credited and that the original publication in this journal is cited, in accordance with accepted academic practice. No use, distribution or reproduction is permitted which does not comply with these terms.
*Correspondence: Victoria Ramos, dnJhbW9zQGlzY2lpaS5lcw==