- 1Laboratory for Multifunctional Materials, Department of Materials, ETH, Zurich, Switzerland
- 2Department of Chemistry and Bioengineering, National Institute of Technology, Oyama College, Oyama, Japan
- 3Faculty of Physical Chemistry, University of Belgrade, Belgrade, Serbia
The polymerization of aniline to polyaniline (PANI) can be achieved chemically, electrochemically or enzymatically. In all cases, the products obtained are mixtures of molecules which are constituted by aniline units. Depending on the synthesis conditions there are variations (i) in the way the aniline molecules are connected, (ii) in the average number of aniline units per molecule, (iii) in the oxidation state, and (iv) in the degree of protonation. For many possible applications, the synthesis of electroconductive PANI with para-N-C-coupled aniline units in their half-oxidized and protonated state is of interest. This is the emeraldine salt form of PANI, abbreviated as PANI-ES. The enzymatic synthesis of PANI-ES is an environmentally friendly alternative to conventional chemical or electrochemical methods. Although many studies have been devoted to the in vitro synthesis of PANI-ES by using heme peroxidases with added hydrogen peroxide (H2O2) as the oxidant, the application of laccases is of particular interest since the oxidant for these multicopper enzymes is molecular oxygen (O2) from air, which is beneficial from environmental and economic points of view. In vivo, laccases participate in the synthesis and degradation of lignin. Various attempts of synthesizing PANI-ES with laccase/O2 in slightly acidic aqueous media from aniline or the linear aniline dimer PADPA (p-aminodiphenylamine) are summarized. Advances in the understanding of the positive effects of soft dynamic templates, as chemical structure guiding additives (anionic polyelectrolytes, micelles, or vesicles), for obtaining PANI-ES-rich products are highlighted. Conceptually, some of these template effects appear to be related to the effect “dirigent proteins” exert in the biosynthesis of lignin. In both cases intermediate radicals are formed enzymatically which then must react in a controlled way in follow-up reactions for obtaining the desired products. These follow-up reactions are controlled to some extent by the templates or specific proteins.
The Participation of Laccases in the In Vivo Synthesis and Degradation of Lignin
Laccases (EC 1.10.3.2) are metalloenzymes that form a subfamily within the superfamily of multicopper oxidases found in fungi, higher plants, bacteria, and insects (Solomon et al., 1996, 2014; Sirim et al., 2011). Laccases have four copper ions that constitute two spatially separated active sites. One active site is formed by one copper ion (type 1, abbreviated as T1), the other by a trinuclear copper cluster (TNC) consisting of T2 (one copper ion) and T3 (two copper ions). Laccases oxidize a broad range of substrates at T1 in a one-electron oxidation reaction, for example the oxidation of phenol derivatives (Ar-OH) to the corresponding phenoxy radicals (Ar-O•), whereby the electron which is released from the substrate is transferred via a His-Cys-His tripeptide from T1 to the TNC where dissolved molecular oxygen (O2) is bound, activated and reduced in a four-electron reduction (Bertrand et al., 2002; Morozova et al., 2007a; Solomon et al., 2014; Jones and Solomon, 2015). In one catalytic laccase cycle, four substrate molecules (e.g., Ar-OH) are oxidized at the expenses of one molecule O2, yielding in the case of Ar-OH four phenoxy radicals (Ar-O•) and two water molecules as side products: 4 Ar-OH + O2 → 4 Ar-O• + 2 H2O (Figure 1A). Ar-O• then undergoes follow-up reactions. The copper ion at T1 is responsible for the blue color of laccases, with λmax ≈ 600 nm and a molar absorption ε≈600 ≈ 5,000 M−1 cm−1 (Solomon et al., 1996). Although these “blue laccases” (Morozova et al., 2007a) are the ones we think of if laccases are mentioned, white and yellow laccases also exist as modifications of blue laccases, lacking the absorption band at λ ≈ 600 nm (Leontievsky et al., 1997; Agrawal et al., 2018).
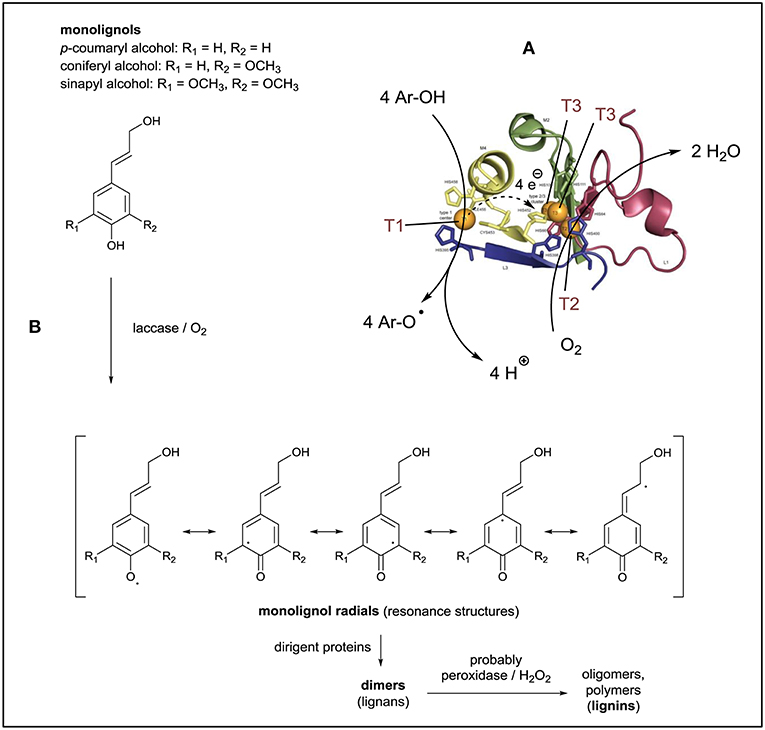
Figure 1. Laccase/O2-catalyzed reactions in connection to the synthesis of lignin. (A) The crystal structure of the two active sites of Trametes versicolor laccase illustrates the four copper ions which participate in the reaction. At the T1 site, the substrate molecules are oxidized, followed by electron transfer from T1 to the trinuclear copper cluster (TNC) where reoxidation takes place with dissolved O2. Reproduced and modified from Sirim et al. (2011). (B) Monolignols as natural substrates of plant laccases, showing the resonance structures of the intermediates obtained from the one-electron oxidation of the monolignols. The initial follow-up reactions are assisted by dirigent proteins and lead to the formation of lignans, which upon further oxidative oligomerization yield lignin.
In plants, laccases participate in the biosynthesis of lignin, which is a complex, branched, heterogeneous, and water-insoluble, amorphous polymer (Roth and Spiess, 2015). Due to the insolubility of lignin it is difficult to determine its molar mass. Reported values for number or weight averaged molar masses are in the range of a few thousands to tens of thousands grams per mol (with a rather high polydispersity), depending on the lignin source, the pre-treatment conditions, and the isolation method (Tolbert et al., 2014). Lignin is formed from basically three hydroxycinnamyl alcohols (so-called monolignols): non-methoxylated p-cumaryl alcohol, mono-methoxylated coniferyl alcohol, and di-methoxylated sinapyl alcohol (Figure 1B). These monolignols are polymerized through radical couplings of their oxidized forms, resulting in an entangled polymeric network of phenolic and non-phenolic linking units (Barros et al., 2015; Roth and Spiess, 2015; Munk et al., 2018). Plant laccases participate at least in the very first step of lignin formation (Sterjiades et al., 1992; Solomon et al., 2014), which is the laccase/O2-catalyzed oxidation of monilignols to the corresponding monolignol phenoxy radicals. Since the unpaired electron can be located on different atoms of the radicals (see the different resonance structures in Figure 1B), the next step of the reaction, the coupling of two radicals to form a dimer (called lignan), leads to products which can have very diverse constitutions and different configurations. This is due to the various radical-radical coupling possibilities. Follow-up reactions, in which peroxidases and hydrogen peroxide (H2O2) are involved as well (Solomon et al., 2014; Barros et al., 2015), lead to the formation of oligomers and the final polymeric product (lignin). There is increasing evidence that the in vivo coupling of the phenoxy radicals which are produced from monolignols by oxidative enzymes like laccases (Figure 1B) is controlled, at least to some extent, through interactions with so-called “dirigent proteins” (Davin and Lewis, 2005). Whether these directing proteins are true enzymes with catalytic activity or not, needs to be clarified (Gasper et al., 2016). In any case it is evident that “dirigent proteins” direct regio- and stereoselectivity in bimolecular phenoxy radical coupling during lignan biosynthesis, and they may play the same role during the follow-up steps leading to the formation of lignin (Pickel and Schaller, 2013; Kim et al., 2015; Guerriero et al., 2016; Paniagua et al., 2017).
Apart from the involvement of plant laccases in the complex biosynthesis of lignan and lignin, laccases which are released from wood-rotting fungi together with other ligninolytic enzymes (including lignin peroxidase and Manganese-dependent peroxidase) (Wong, 2009; Janusz et al., 2017; Martínez et al., 2018) also participate in the degradation of lignin present in the wood on which the fungi live (e.g., Baldrian, 2006; Giardina et al., 2010; Roth and Spiess, 2015). For an efficient in vitro degradation of lignin the removal of degradation products seems to be important for preventing a re-synthesis (Roth and Spiess, 2015; Munk et al., 2018). Furthermore, the efficiency of the laccase for degrading lignin can be improved by using so-called mediators (organic or inorganic compounds or metal ions), which can reach the T1 site of the laccase and which have redox potentials that are lower or comparable to the redox potential of the laccase involved, such that these mediator molecules are oxidized by the laccase (Bourbonnais and Paice, 1990; Morozova et al., 2007b; Roth and Spiess, 2015; Longe et al., 2018). In this case, fungal laccases oxidize the mediator molecules, and the oxidized mediator molecules oxidize lignin, which can occur via different mechanisms, finally resulting in lignin degradation. The presence of mediator molecules seems to be essential for the complete degradation of lignin by laccases; without mediators, only the breaking of bonds in phenolic model compounds of lignin is catalyzed, for non-phenolic subunits, the use of mediators appears a must (Munk et al., 2015).
The Use of Laccases for In Vitro Oligo-and Polymerization Reactions
Laccases, in particular the ones from Trametes fungi with their high oxidation potentials of ~0.78 V vs. NHE at the T1 site (Morozova et al., 2007a) and solvent exposed, about 5–8 Å deep, hydrophobic binding site near T1 (Solomon et al., 2014), have a very broad range of accessible substrates (Xu, 1996; Baldrian, 2006; Tadesse et al., 2008; Strong and Claus, 2011; Reiss et al., 2013). This allows for mediator-free in vitro applications which go beyond laccase-catalyzed oxidative transformations of physiological substrates. One example is the oxidation of aniline (Ph-NH2) (Reiss et al., 2013) despite its relatively low standard oxidation potential of −1.0 V ( (Ph-NH2/Ph-N) = −1.0 V vs. NHE) (Jonsson et al., 1994). If laccase-catalyzed transformations of non-physiological substrates in the presence of mediators are considered as well, it is not surprising that laccases are recognized as very valuable biocatalysts for many commercial and research applications in various areas (e.g., Riva, 2006; Rodríguez Couto and Toca Herrera, 2006; Kunamneni et al., 2008; Mikolasch and Schauer, 2009; Kudanga et al., 2011; Hollmann and Arends, 2012; Polak and Jarosz-Wilkolazka, 2012; Sousa et al., 2013; Pezzella et al., 2015; Mate and Alcalde, 2016; Upadhyay et al., 2016; Cannatelli and Ragauskas, 2017; Yang et al., 2017;Slagman et al., 2018).
Applications of laccase-catalyzed in vitro reactions include the laccase/O2-mediated syntheses of polymeric (or oligomeric) products from methyl methacrylate or styrene (with acetylacetone as mediator, see Tsujimoto et al., 2001), acrylamide (Ikeda et al., 1998), phenols (Mita et al., 2003; Marjasvaara et al., 2006; Sun et al., 2013; Su et al., 2018a, 2019a), pyrrole (Song and Palmore, 2005; Junker et al., 2015), dopamine (Tan et al., 2010; Li et al., 2018), 3,4-ethylenedioxythiophene (Shumakovich et al., 2012b; Vasil'eva et al., 2018), or various arylamines (Ćirić-Marjanović et al., 2017; Zhang T. et al., 2018; Su et al., 2019a,b). With respect to the latter type of monomers, the focus often was—and still is—on the synthesis of oligo- or polyaniline (PANI) from aniline (Karamyshev et al., 2003; Vasil'eva et al., 2007, 2009; Streltsov et al., 2008, 2009; Shumakovich et al., 2010, 2012a, 2014; Leppänen et al., 2013; Zhang et al., 2014, 2016; Zhang Y. et al., 2018; Junker et al., 2014a; de Salas et al., 2016; Su et al., 2018b) or from p-aminodiphenylamine (PADPA), the linear para N-C-coupled aniline dimer Shumakovich et al., 2011; Junker et al., 2014b; Janoševic Ležaić et al., 2016; Luginbühl et al., 2016; Kashima et al., 2018;Kashima et al., 2019).
Laccase/O2-catalyzed Synthesis of Polyaniline (PANI) and the Role of “Templates”
If a high oxidation potential laccase is added to an aqueous solution containing aniline (Ph-NH2) as monomer in the absence of any mediator, the one-electron oxidation of the neutral form of aniline occurs in analogy to the oxidation of monolignols (see above) according to the following stoichiometric equation: 4 Ph-NH2 + O2 → 4 Ph-NH• + 2 H2O (Figure 2A). This means that in each catalytic laccase cycle four aniline molecules are oxidized by the laccase at the expenses of one molecule O2, yielding four anilino radicals (Ph-NH•) and two water molecules as side products (Junker et al., 2014a). Ph-NH• then undergoes follow-up reactions (radical-radical couplings and/or further oxidations). All follow-up reactions are probably no more under direct control by the enzyme, resulting in a mixture of products the composition of which being determined by the actual reaction conditions, i.e., the pH of the reaction mixture, the reaction temperature, the aniline concentration, the type and amount of laccase used, and whether “templates” are added or not. The term “template” stands here for any type of reaction additive which has a positive influence on the intended outcome of the reaction in terms of chemical structure of the reaction product(s) (Walde and Guo, 2011). This is somewhat related to the role “dirigent proteins” have in the biosynthesis of lignan and possibly also lignin (see above). For the enzymatic synthesis of electroconductive PANI, excellent templates are sulfonated polystyrene as sodium salt (SPS, Karamyshev et al., 2003), the calcium salt of ligninosulfonate (Zhang et al., 2016), micelles from sodium dodecylbenzenesulfonate (SDBS, Streltsov et al., 2009), or vesicles from sodium bis(2-ethylhexyl)sulfosuccinate (AOT, Junker et al., 2014a; Figure 2B). All these template molecules have sulfonate groups in their structures. In the presence of the templates and under optimal conditions (usually pH ≈ 3–4), the laccase/O2-catalyzed oxidation of aniline results in the formation of products which have the characteristic properties of the green conductive form of PANI, known as PANI-ES, the emeraldine salt form of PANI (Figure 2A). PANI-ES is the half oxidized, protonated form of linear PANI, with the aniline monomers coupled by N-C bonds in para position to the amino group. The repeating unit of perfect, defect-free PANI-ES is the half-oxidized, protonated linear tetraaniline shown in Figure 2A. Some of the characteristic properties of solutions or dispersions of PANI-ES in their conductive, polaron state are (Kashima et al., 2019): (i) high absorption intensity in the near infrared (NIR) region of the absorption spectrum, often with an absorption maximum at λ ≈ 800–1,100 nm, assigned to the π → polaron transition (do Nascimento and de Souza, 2015); (ii) an absorption band at λ ≈ 420 nm, assigned to the polaron → π* transition (do Nascimento and de Souza, 2015); (iii) low absorption at λ ≈ 500–600 nm, indicative for the absence of extensive branching and phenazine unit formation (Liu et al., 1999b; Luginbühl et al., 2017); (iv) the presence of unpaired electrons, which results in an electron paramagnetic resonance (EPR) spectrum (Kulikov et al., 2002; Krinichnyi et al., 2006; Rakvin et al., 2014); and (v) characteristic Raman bands at ν ≈ 1,320–1,380 cm−1, originating from C–N•+ stretching vibrations of the polaronic form of PANI-ES (Ćirić-Marjanović et al., 2008a; Janoševic Ležaić et al., 2016).
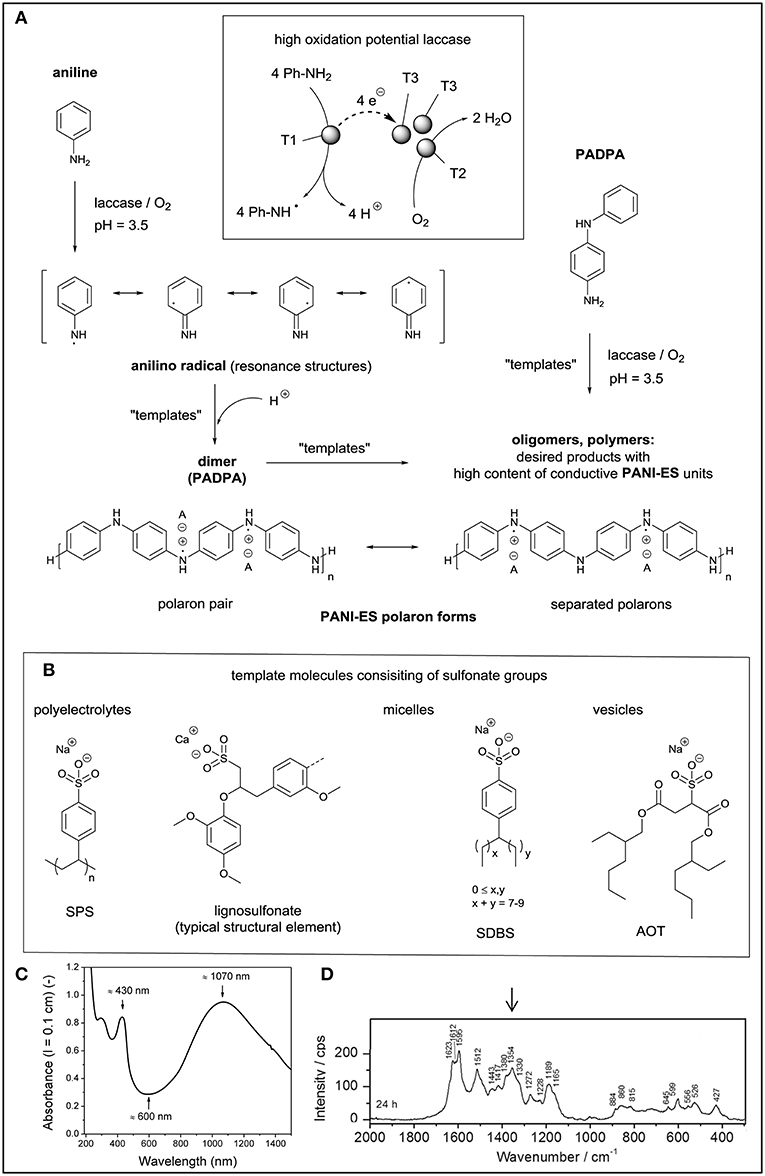
Figure 2. Template-assisted laccase/O2-catalyzed synthesis of oligomeric and polymeric products consisting of conductive PANI-ES units. (A) One-electron oxidation of aniline to the anilino radical, followed by the formation of the aniline dimer (PADPA) and polymeric products. Similarly, the use of PADPA as monomer can yield oligomeric PANI-ES-type products. (B) Some of the template molecules used, all containing sulfonate groups but differing in their state in aqueous solution: dynamic assemblies of polyelectrolytes, micelles, or vesicles. (C) Two examples of in situ recorded spectra of the obtained PANI-ES-type molecules formed with Trametes versicolor laccase/O2 from PADPA at pH = 3.5 in the presence of AOT vesicles as templates. Reproduced and modified from Kashima et al. (2019), with permission of the ACS. Further permissions related to (B) and (C) should be directed to the ACS.
The role of the templates during and at the end of the reaction has been investigated and discussed previously, mainly in reports of experiments using horseradish peroxidase isoenzyme C (HRPC) and added H2O2 as the oxidant (Liu et al., 1999a; Junker et al., 2012) instead of laccase/O2. The templates seem to direct the regioselectivity of the monomer coupling reaction due to a localization of the reaction in the vicinity of the templates, favoring para- over ortho-coupling of oxidized aniline. Furthermore, the templates act as dopants (counter ions) of the formed PANI (balancing the positive charge on the PANI polycation backbone), thereby stabilizing the PANI-ES structure which is essential for its property (electrical conductivity). This property is often the target of research on the laccase/O2- (or peroxidase/H2O2-) catalyzed polymerization of aniline, as environmentally friendly method, an alternative method to conventional chemical or electrochemical procedures (Stejskal et al., 2015). In the presence of good templates, the obtained PANI-ES products remain solubilized or dispersed in the aqueous reaction medium (no precipitation). Without template, but otherwise identical conditions, the formation of undesired, insoluble brown products is observed (Liu et al., 1999b; Guo et al., 2009).
It is very likely that in all studies that have been published on the laccase/O2-catalyzed oxidations of aniline in the presence of templates, mixtures of different products were obtained and not a single type of PANI-ES molecule with a fully defined chemical structure. This is due to the different follow-up reactions that may occur once anilino radicals (Ph-NH•) are produced by the laccase (see above). Unfortunately, due to the insolubility of at least some of the products obtained, a separation of all reaction products and an indisputable quantitative analysis of their chemical structures are impossible with current methods. Therefore, the overall product analysis mainly relies on in situ UV/vis/NIR, EPR, and cyclic voltammetry measurements and on a FTIR characterization of isolated product mixtures. The presence of the templates often assists in keeping the formed products dissolved or dispersed. This allows monitoring the formation of desired functional groups during polymerization and after reaching reaction equilibrium, for example by simple UV/vis/NIR measurements (Junker et al., 2014a). Interestingly, independent from the type of laccase and type of chosen template used, the UV/vis/NIR spectrum of the reaction mixture always appears to have an absorption maximum (λmax) between λ = 700 and 800 nm, and not at λ ≈ 800–1,100 nm, as expected for the π → polaron transition of PANI-ES (see above): λmax ≈ 750 nm (with Trametes hirsuta laccase and SPS as template at pH = 3.5 and T = 20°C; Karamyshev et al., 2003); λmax = 740–800 nm (with Trametes hirsuta laccase and SDBS micelles at pH = 3.8 and T = 20°C, Streltsov et al., 2008); λmax ≈ 700 nm (with Trametes hirsuta laccase and poly(2-acrylamido-2-methyl-1-propanesulfonic acid) at pH = 3.5 and T = 20°C, Shumakovich et al., 2010); λmax ≈ 750 nm (with Trametes versicolor laccase and κ-carrageenan at pH = 3.7 and room temperature, Leppänen et al., 2013); λmax ≈ 750 nm (with Denlite®, a laccase preparation from Aspergillus, and SDBS micelles at pH = 4.5 and T = 10°C, Zhang et al., 2014); λmax ≈ 750 nm (with Denlite® and lignosulfonate at pH = 3.5 and T = 5°C, Zhang et al., 2016); and λmax ≈ 730 nm (with Trametes versicolor laccase and AOT vesicles at pH = 3.5 and T = 25 or 8°C, Junker et al., 2014a). These observed absorption maxima contrast with what has been observed for the HRPC/H2O2-catalyzed polymerization of aniline, even if the same template was used, e.g., AOT vesicles (λmax ≈ 1,000 nm at pH = 4.3 and T ≈ 25°C, Junker et al., 2012; Pašti et al., 2017). One reason for this difference could be that the PANI-ES products which are obtained with laccase/O2 in the presence of a template are always in a “compact coil” conformation (MacDiarmid and Epstein, 1994, 1995; Yoo et al., 2007) with a shorter conjugation length and low delocalization of polarons, as compared to the PANI-ES products obtained in the presence of the same template with HRPC/H2O2; in the latter case, UV/vis/NIR spectra indicated an “extended coil” conformation and high delocalization of polarons (Xia et al., 1995). Alternatively, it may be that the PANI products obtained with laccase/O2 are overoxidized. This was the conclusion which was drawn in the case of PANI products obtained from aniline with Trametes versicolor laccase at pH = 3.5 in the presence of AOT vesicles as templates (Junker et al., 2014a).
One serious drawback of the template-assisted laccase/O2- or HRPC/H2O2-catalyzed polymerization of aniline is the relatively large amount of enzyme required for high aniline conversion. In the case of AOT vesicles as templates, for the oxidation of 1.0 g aniline, the estimated amount of pure Trametes versicolor laccase is also about 1.0 g (Junker et al., 2014a); in the case of HRPC, it is about 0.1 g enzyme (Junker et al., 2012). It is the formed polymeric product that inactivates the enzymes (Junker et al., 2012). From an economic point of view, such waste of enzyme is inacceptable, although the as obtained PANI-ES product may have excellent electrochemical properties, as shown for PANI-ES obtained with HRPC/H2O2 (Pašti et al., 2017). It is not clear at the moment how enzyme inactivation can be avoided or minimized for these reactions. Possible approaches toward an increase in operational enzyme stability could be to use mediator molecules for the reaction (Shumakovich et al., 2012a), to add polymers for stabilizing the enzyme (Junker et al., 2013), or to try to use immobilized enzymes (Vasil'eva et al., 2009).
“Template” Effect on the Laccase/O2-catalyzed Oligomerization of PADPA
The aniline dimer PADPA (p-aminodiphenylamine, Figure 2A) is the first intermediate product which forms if two anilino radicals (or their protonated forms, i.e., aniline radical cations) react with each other in the desired way (head-to-tail coupling). PADPA must then undergo further reactions with aniline to finally yield linear PANI-ES. Due to this role as important intermediate product, PADPA has also been considered as monomer instead of aniline for the laccase/O2-catalyzed synthesis of PANI-ES. However, the reactivity of PADPA and the laccase/O2-catalyzed oxidation of PADPA differ considerably from those of aniline. First of all, PADPA is much easier to oxidize than aniline. The standard oxidation potential, (PADPA) is about −0.4 to −0.5 V vs. NHE (Gospodinova and Terlemezyan, 1998), higher than in the case of aniline, (aniline) = −1.0 V (Jonsson et al., 1994). Second, like in the case of the chemical or electrochemical oxidative polymerization of PADPA (Kitani et al., 1987; Geniès et al., 1989; Ćirić-Marjanović et al., 2008b), the majority of the products obtained from the laccase/O2-catalyzed oxidation of PADPA are oligomers and not true polymers (Shumakovich et al., 2011; Junker et al., 2014b; Luginbühl et al., 2016). This may have certain disadvantages in terms of applications, but it also has an analytical advantage. The obtained product mixture can be separated by high performance liquid chromatography (HPLC) with UV/vis diode array or mass spectrometry detection. This is at least the case for the products obtained from the Trametes versicolor laccase/O2-catalyzed oxidation of PADPA at pH = 3.5 in the presence (or absence) of AOT vesicles as templates (Junker et al., 2014b; Luginbühl et al., 2016; Kashima et al., 2018). Reaction product extraction into an organic solvent (t-butylmethylether) and analysis are possible for the specific reaction conditions used. They were optimized in terms of (i) high PADPA conversion, (ii) desired formation of PANI-ES-like products by using, (iii) low amounts of template, (iv) high colloidal stability, and (v) minimal amounts of enzyme. Before extracting the products into the organic solvent, they were deprotonated to make them soluble in the solvent. Afterwards, the products were reduced before applying on the HPLC column. After the entire product separation and identification of the molecules present, information on original protonation and oxidation states is lost. Only through the combination of the HPLC analysis—which was also carried out with partially deuterated PADPA monomers and O—and complementary in situ UV/vis/NIR, EPR and Raman spectroscopy measurements of the entire reaction mixture recorded during the reaction and after reaching reaction equilibrium, clear conclusions about the effect of the AOT vesicle template on the outcome of the reaction could be drawn (Luginbühl et al., 2016; Kashima et al., 2018). Three essential findings are worth mentioning.
(i) The main product of the reaction in the presence of the vesicles is the para-N-C-coupled PADPA dimer in its half-oxidized, protonated state, i.e., the tetraaniline repeating unit of ideal PANI-ES (Figure 2A). This is the shortest possible PANI-ES type molecule and contributes most substantially among all formed products to the in situ recorded absorption spectrum of the reaction mixture, with λmax ≈ 1,070 and ≈ 430 nm (Junker et al., 2014b; Kashima et al., 2018; Figure 2C), the in situ recorded EPR spectrum (Janoševic Ležaić et al., 2016; Kashima et al., 2018), and the in situ recorded Raman spectrum with characteristic band positions at ν ≈ 1,350–1,380 cm−1 (Janoševic Ležaić et al., 2016; Kashima et al., 2018; Figure 2D). Higher PANI-ES-type oligomers are also formed but to a much lesser extent.
(ii) If the reaction is run without template, many products contain an oxygen atom which originates from bulk water. The incorporation of the oxygen atom is most probably caused by hydrolysis of an intermediate diimine (the protonated form of N-phenyl-1,4-benzoquinonediimine). If the reaction is run in the presence of vesicles as templates, undesired products containing an oxygen atom are absent (Luginbühl et al., 2016).
(iii) Oligomers built from more than two PADPA molecules, some of them containing phenazine units, are formed in the presence as well as in the absence of vesicles, with the extent of phenazine formation being considerably higher without vesicles (Luginbühl et al., 2016).
In summary, the analysis of the Trametes versicolor laccase/O2-catalyzed oxidation of PADPA in aqueous solution at pH = 3.5 in the presence of anionic AOT vesicle templates has shown that the vesicles steer the reaction toward the formation of desired PANI-ES-like products. Certain undesired reaction pathways (hydrolysis or phenazine formation) are prevented or largely avoided. Although the detailed molecular picture is still fragmentary and current mechanistic hypotheses still need to be proven or disproven, it is clear that the reproducible preparation of PANI-ES-like oligomers, which have the characteristic spectroscopic features of chemically synthesized PANI-ES, is possible under environmentally friendly conditions with laccase/O2 as efficient catalyst, PADPA as monomer, and an appropriate template. Apart from AOT vesicles, other templates with sulfonate groups can be used as well, SPS polyelectrolyte, SDBS micelles or SDBS/decanoic acid (1:1) vesicles, see Kashima et al. (2019). For each template, optimal reaction conditions have to be elaborated. Furthermore, the template type has an influence on the property of the obtained mixture of products (Kashima et al., 2019).
Conclusions
Although there is no doubt that high oxidation potential laccases can catalyze the oxidation of aniline or its dimer PADPA in aqueous media in the presence of dissolved O2, the outcome of the reaction depends on many factors. The presence of an anionic template is essential for obtaining the emeraldine salt form of oligo- or polymeric aniline products. This template effect is related to the role “dirigent proteins” have in the biosynthesis of lignin. Since the polymeric products obtained from aniline are difficult to isolate and analyze individually—often despite their promising electrochemical properties—the oligomeric products obtained from PADPA allow gaining insight into the effect the templates exert on the reaction. The templates act as soft, dispersed interface-rich additives (Serrano-Luginbühl et al., 2018) that guide the reactions toward desired products. Despite uncertainties in the composition and chemical structure of the reaction products obtained, the best choice of monomer, laccase type, and template may depend on the actual product application in mind. Nevertheless, the challenge remains in improving the knowledge about the guiding effect of the template—also for other related reactions (Junker et al., 2015)—so that the reaction conditions can be tuned in a controlled way for achieving products with desired properties in high yield under as environmentally friendly conditions as possible.
Author Contributions
PW outlined the article. All authors contributed to the writing.
Funding
The authors acknowledge financial support from the Swiss National Science Foundation, projects 200020_150254 and IZ73Z0_152457 (PW and GĆ-M), the National Institute of Technology, Faculty Research Abroad Program, Japan (KK), and the Ministry of Education, Science and Technology of the Republic of Serbia, project OI172043 (GĆ-M).
Conflict of Interest Statement
The authors declare that the research was conducted in the absence of any commercial or financial relationships that could be construed as a potential conflict of interest.
Acknowledgments
The authors acknowledge Dr. Reinhard Kissner, Department of Chemistry and Applied Biosciences, ETH, for his comments to the manuscript.
References
Agrawal, K., Chaturvedi, V., and Verma, P. (2018). Fungal laccase discovered but yet undiscovered. Bioresour. Bioprocess. 5:4. doi: 10.1186/s40643-018-0190-z
Baldrian, P. (2006). Fungal laccases – occurrence and properties. FEMS Microbiol. Rev. 30, 215–242. doi: 10.1111/j.1574-4976.2005.00010.x
Barros, J., Serk, H., Granlund, I., and Pesquet, E. (2015). The cell biology of lignification in higher plants. Ann. Bot. 115, 1053–1074. doi: 10.1093/aob/mcv046
Bertrand, T., Jolivalt, C., Briozzo, P., Caminade, E., Joly, N., Madzak, C., et al. (2002). Crystal structure of a four-copper laccase complexed with an arylamine: insights into substrate recognition and correlation with kinetics. Biochemistry 41, 7325–7333. doi: 10.1021/bi0201318
Bourbonnais, R., and Paice, M. G. (1990). Oxidation of non-phenolic substrates. FEBS Lett. 267, 99–102. doi: 10.1016/0014-5793(90)80298-W
Cannatelli, M. D., and Ragauskas, A. J. (2017). Two decades of laccases: advancing sustainability in the chemical industry. Chem. Rec. 17, 122–140. doi: 10.1002/tcr.201600033
Ćirić-Marjanović, G., Milojević-Rakić, M., Janoševic Ležaić, A., Luginbühl, S., and Walde, P. (2017). Enzymatic oligomerization and polymerization of arylamines: state of the art and perspectives. Chem. Pap. 71, 199–242. doi: 10.1007/s11696-016-0094-3
Ćirić-Marjanović, G., Trchová, M., Konyushenko, E. N., Holler, P., and Stejskal, J. (2008b). Chemical oxidative polymerization of aminodiphenylamines. J. Phys. Chem. B 112, 6976–6987. doi: 10.1021/jp710963e
Ćirić-Marjanović, G., Trchová, M., and Stejskal, J. (2008a). The chemical oxidative polymerization of aniline in water: Raman spectroscopy. J. Raman Spectrosc. 39, 1375–1387. doi: 10.1002/jrs.2007
Davin, L. B., and Lewis, N. G. (2005). Dirigent phenoxy radical coupling: advances and challenges. Curr. Opin. Biotechnol. 16, 398–406. doi: 10.1016/j.copbio.2005.06.010
de Salas, F., Pardo, I., Salavagione, H. J., Aza, P., Amougi, E., Vind, J., et al. (2016). Advanced synthesis of conductive polyaniline using laccase as biocatalyst. PLoS ONE 11:e0164958. doi: 10.1371/journal.pone.0164958
do Nascimento, G. M., and de Souza, M. A. (2015). “Spectroscopy of nanostructured conductive polymers,” in Nanostructured Conductive Polymers, ed A. Eftekhari (Chichester: John Wiley and Sons), 341–373.
Gasper, R., Effenberger, I., Kolesinski, P., Terlecka, B., Hofmann, E., and Schaller, A. (2016). Dirigent protein mode of action revealed by the crystal structure of AtDIR6. Plant Physiol. 172, 2165–2175. doi: 10.1104/pp.16.01281
Geniès, E., Penneau, J., Lapkowski, M., and Boyle, A. (1989). Electropolymerisation reaction mechanism of para-aminodiphenylamine. J. Electroanal. Chem. Interfacial Electrochem. 269, 63–75. doi: 10.1016/0022-0728(89)80104-4
Giardina, P., Faraco, V., Pezzella, C., Piscitelli, A., Vanhulle, S., and Sannia, G. (2010). Laccases: a never-ending story. Cell. Mol. Life Sci. 67, 369–385. doi: 10.1007/s00018-009-0169-1
Gospodinova, N., and Terlemezyan, L. (1998). Conducting polymers prepared by oxidative polymerization: polyaniline. Prog. Polym. Sci. 23, 1443–1484. doi: 10.1016/S0079-6700(98)00008-2
Guerriero, G., Hausman, J.-F., Strauss, J., Ertan, H., and Siddiqui, K. S. (2016). Lignocellulosic biomass: biosynthesis, degradation, and industrial utilization. Eng. Life Sci. 16, 1–16. doi: 10.1002/elsc.201400196
Guo, Z., Rüegger, H., Kissner, R., Ishikawa, T., Willeke, M., and Walde, P. (2009). Vesicles as soft templates for the enzymatic polymerization of aniline. Langmuir 25, 11390–11405. doi: 10.1021/la901510m
Hollmann, F., and Arends, I. W. C. E. (2012). Enzyme initiated radical polymerizations. Polymers 4, 759–793. doi: 10.3390/polym4010759
Ikeda, R., Tanaka, H., Uyama, H., and Kobayashi, S. (1998). Laccase-catalyzed polymerization of acrylamide. Macromol. Rapid Commun. 19, 423–425. doi: 10.1002/(SICI)1521-3927(19980801)19:8<423::AID-MARC423>3.0.CO;2-K
Janoševic Ležaić, A., Luginbühl, S., Bajuk-Bogdanović, D., Pašti, I., Kissner, R., Rakvin, B., et al. (2016). Insight into the template effect of vesicles on the laccase-catalyzed oligomerization of N-phenyl-1,4-phenylenediamine from Raman spectroscopy and cyclic voltammetry measurements. Sci. Rep. 6:30724. doi: 10.1038/srep33770
Janusz, G., Pawlik, A., Sulej, J., Swiderska-Burek, U., Jarosz-Wilkolazka, A., and Paszcynski, A. (2017). Lignin degradation: microorganisms, enzymes involved, genomes analysis and evolution. FEMS Microbiol. Rev. 41, 941–962. doi: 10.1093/femsre/fux049
Jones, S. M., and Solomon, E. I. (2015). Electron transfer and reaction mechanism of laccases. Cell. Mol. Life Sci. 72, 869–883. doi: 10.1007/s00018-014-1826-6
Jonsson, M., Lind, J., Eriksen, T. E., and Merényi, G. (1994). Redox and acidity properties of 4-substituted aniline radical cations in water. J. Am. Chem. Soc. 116, 1423–1427. doi: 10.1021/ja00083a030
Junker, K., Gitsov, I., Quade, N., and Walde, P. (2013). Preparation of aqueous polyaniline-vesicle suspensions with class III peroxidases. Comparison between horseradish peroxidase isoenzyme C and soybean peroxidase. Chem. Pap. 67, 1028–1047. doi: 10.2478/s11696-013-0307-y
Junker, K., Kissner, R., Rakvin, B., Guo, Z., Willeke, M., Busato, S., et al. (2014a). The use of Trametes versicolor laccase for the polymerization of aniline in the presence of vesicles as templates. Enzyme Microb. Technol. 55, 72–84. doi: 10.1016/j.enzmictec.2013.12.008
Junker, K., Luginbühl, S., Schüttel, M., Bertschi, L., Kissner, R., Schuler, L. D., et al. (2014b). Efficient polymerization of the aniline dimer p-aminodiphenylamine (PADPA) with Trametes versicolor laccase/O2 as catalyst and oxidant and AOT vesicles as templates. ACS Catal. 4, 3421–3434. doi: 10.1021/cs500769d
Junker, K., Zandomeneghi, G., Guo, Z., Kissner, R., Ishikawa, T., Kohlbrecher, J., et al. (2012). Mechansitic aspects of the horseradish peroxidase-catalysed polymerisation of aniline in the presence of AOT vesicles as templates. RSC Adv. 2, 6478–6495. doi: 10.1039/c2ra20566a
Junker, K., Zandomeneghi, G., Schuler, L. D., Kissner, R., and Walde, P. (2015). Enzymatic polymerization of pyrrole with Trametes versicolor laccase and dioxygen in the presence of vesicles formed from AOT (sodium bis-(2-ethylhexyl) sulfosuccinate) as templates. Synth. Met. 200, 123–134. doi: 10.1016/j.synthmet.2015.01.004
Karamyshev, A. V., Shleev, S. V., Koroleva, O. V., Yaropolov, A. I., and Sakharov, I. Y. (2003). Laccase-catalyzed synthesis of conducting polyaniline. Enzyme Microb. Technol. 33, 556–564. doi: 10.1016/S0141-0229(03)00163-7
Kashima, K., Fujisaki, T., Serrano-Luginbühl, S., Khaydarov, A., Kissner, R., Janoševic Ležaić, A., et al. (2018). How experimental details matter. the case of a laccase-catalysed oligomerisation reaction. RSC Adv. 8, 33229–33242. doi: 10.1039/C8RA05731A
Kashima, K., Fujisaki, T., Serrano-Luginbühl, S., Kissner, R., Janoševic Ležaić, A., Bajuk-Bogdanović, D., et al. (2019). Effect of template type on the Trametes versicolor laccase-catalyzed oligomerization of the aniline dimer p-aminodiphenylamine (PADPA). ACS Omega 4, 2931–2947. doi: 10.1021/acsomega.8b03441
Kim, K.-W., Smith, C. A., Daily, M. D., Cort, J. R., Davin, L. B., and Lewis, N. G. (2015). Trimeric structure of (+)-pinoresinol-forming dirigent protein at 1.95 Å resolution with three isolated active sites. J. Biol. Chem. 290, 1308–1318. doi: 10.1074/jbc.M114.611780
Kitani, A., Yano, J., Kunai, A., and Sasaki, K. (1987). A conducting polymer derived from para-aminodiphenylamine. J. Electroanal. Chem. Interfacial Electrochem. 221, 69–82. doi: 10.1016/0022-0728(87)80246-2
Krinichnyi, V. I., Roth, H.-K., Schrödner, M., and Wessling, B. (2006). EPR study of polyaniline highly doped by p-toluenesulfonic acid. Polymer 47, 7460–7468. doi: 10.1016/j.polymer.2006.08.025
Kudanga, T., Nyanhongo, G. S., Guebitz, G. M., and Burton, S. (2011). Potential applications of laccase-mediated coupling and grafting reactions. Enzyme Microb. Technol. 48, 195–208. doi: 10.1016/j.enzmictec.2010.11.007
Kulikov, A. V., Bogatyrenko, V. R., Belonogova, O. V., Fokeeva, L. S., Lebedev, A. V., Echmaeva, T. A., et al. (2002). ESR study of mechanism of polyaniline conductivity. Russ. Chem. Bull. Int. Edn. 51, 2216–2223. doi: 10.1023/A:1022127100633
Kunamneni, A., Camarero, S., García-Burgos, C., Plou, F. J., Ballesteros, A., and Alcalde, M. (2008). Engineering and applications of fungal laccases for organic synthesis. Microb. Cell Fact. 7:32. doi: 10.1186/1475-2859-7-32
Leontievsky, A., Myasoedova, N., Pozdnyakova, N., and Golovleva, L. (1997). “Yellow” laccase of Panus tigrinus oxidizes non-phenolic substrates without electron-transfer mediators. FEBS Lett. 413, 446–448. doi: 10.1016/S0014-5793(97)00953-8
Leppänen, A. S., Xu, C., Liu, J., Wang, X., Pesonen, M., and Willför, S. (2013). Anionic polysaccharides as templates for the synthesis of conducting polyaniline and as structural matrix for conducting biocomposites. Macromol. Rapid Commun. 34, 1056–1061. doi: 10.1002/marc.201300275
Li, F., Yu, Y., Wang, Q., Yuan, J., Wang, P., and Fan, X. (2018). Polymerization of dopamine catalyzed by laccase: comparison of enzymatic and conventional methods. Enzyme Microb. Technol. 119, 58–64. doi: 10.1016/j.enzmictec.2018.09.003
Liu, W., Cholli, A. L., Nagarajan, R., Kumar, J., Tripathy, S., Bruno, F. F., et al. (1999a). The role of template in the enzymatic synthesis of conducting polyaniline. J. Am. Chem. Soc. 121, 11345–11355. doi: 10.1021/ja9926156
Liu, W., Kumar, J., Tripathy, S., Senecal, K. J., and Samuelson, L. (1999b). Enzymatically synthesized conducting polyaniline. J. Am. Chem. Soc. 121, 71–78. doi: 10.1021/ja982270b
Longe, L. F., Couvreur, J., Leriche Grandchamp, M., Garnier, G., Allais, F., and Saito, K. (2018). Importance of mediators for lignin degradation by fungal laccase. ACS Sustainable Chem. Eng. 6, 10097–10107. doi: 10.1021/acssuschemeng.8b01426
Luginbühl, S., Bertschi, L., Willeke, M., Schuler, L. D., and Walde, P. (2016). How anionic vesicles steer the oligomerization of enzymatically oxidized p-aminodiphenylamine (PADPA) toward a polyaniline emeraldine salt (PANI-ES)-type product. Langmuir 32, 9765–9779. doi: 10.1021/acs.langmuir.6b02146
Luginbühl, S., Milojević-Rakić, M., Junker, K., Bajuk-Bogdanović, D., Pašti, I., Kissner, R., et al. (2017). The influence of anionic vesicles on the oligomerization of p-aminodiphenylamine catalyzed by horseradish peroxidase and hydrogen peroxide. Synth. Met. 226, 89–103. doi: 10.1016/j.synthmet.2017.01.011
MacDiarmid, A. G., and Epstein, A. J. (1994). The concept of secondary doping as applied to polyaniline. Synth. Met. 65, 103–116. doi: 10.1016/0379-6779(94)90171-6
MacDiarmid, A. G., and Epstein, A. J. (1995). Secondary doping in polyaniline. Synth. Met. 69, 85–92. doi: 10.1016/0379-6779(94)02374-8
Marjasvaara, A., Torvinen, M., Kinnunen, H., and Vainiotalo, P. (2006). Laccase-catalyzed polymerization of two phenolic compounds studied by matrix-assisted laser desorption/ionization time-of-flight and electrospray ionization fourier transform ion cyclotron resonance mass spectrometry with collision-induced dissociation experiments. Biomacromolecules 7, 1604–1609. doi: 10.1021/bm060038p
Martínez, A. T., Camarero, S., Ruiz-Dueñas, F. J., and Martínez, M. J. (2018). “Biological Lignin Degradation,” in Lignin Valorization: Emerging Approaches, ed G. T. Beckham (Cambridge: Royal Society of Chemistry), 188–225.
Mate, D. M., and Alcalde, M. (2016). Laccase: a multi-purpose biocatalyst at the forefront of biotechnology. Microb. Biotechnol. 10, 1457–1467. doi: 10.1111/1751-7915.12422
Mikolasch, A., and Schauer, F. (2009). Fungal laccases as tools for the synthesis of new hybrid molecules and biometerials. Appl. Micorbiol. Biotechnol. 82, 605–624. doi: 10.1007/s00253-009-1869-z
Mita, N., Tawaki, S.-I., Uyama, H., and Kobayashi, S. (2003). Laccase-catalyzed oxidative polymerization of phenols. Macromol. Biosci. 3, 253–257. doi: 10.1002/mabi.200390032
Morozova, O. V., Shumakovich, G. P., Gorbacheva, M. A., Shleev, S. V., and Yaropolov, A. I. (2007a). “Blue” laccases. Biochemistry 72, 1136–1150. doi: 10.1134/S0006297907100112
Morozova, O. V., Shumakovich, G. P., Shleev, S. V., and Iaropolov, A. I. (2007b). Laccase-mediator systems and their applications: a review. Appl. Biochem. Microbiol. 43, 523–535. doi: 10.1134/S0003683807050055
Munk, L., Andersen, M. L., and Meyer, A. S. (2018). Influence of mediators on laccase catalyzed radical formation in lignin. Enzyme Microb. Technol. 116, 48–56. doi: 10.1016/j.enzmictec.2018.05.009
Munk, L., Sitarz, A. K., Kalyani, D. C., Mikkelsen, J. D., and Meyer, A. S. (2015). Can laccases catalyze bond cleavage in lignin? Biotechnol. Adv. 33, 13–24. doi: 10.1016/j.biotechadv.2014.12.008
Paniagua, C., Bilkova, A., Jackson, P., Dabravolski, S., Riber, W., Didi, V., et al. (2017). Dirigent proteins in plants: modulating cell wall metabolism during abiotic and biotic stress exposure. J. Exp. Bot. 68, 3287–3301. doi: 10.1093/jxb/erx141
Pašti, I., Milojević-Rakić, M., Junker, K., Bajuk-Bogdanović, D., Walde, P., and Cirić-Marjanović, G. (2017). Superior capacitive properties of polyaniline produced by a one-pot peroxidase/H2O2-triggered polymerization of aniline in the presence of AOT vesicles. Electrochim. Acta 258, 834–841. doi: 10.1016/j.electacta.2017.11.133
Pezzella, C., Guarino, L., and Piscitelli, A. (2015). How to enjoy laccases. Cell. Mol. Life Sci. 72, 923–940. doi: 10.1007/s00018-014-1823-9
Pickel, B., and Schaller, A. (2013). Dirigent proteins: molecular characteristics and potential biotechnological applications. Appl. Micobiol. Biotechnol. 97, 8427–8438. doi: 10.1007/s00253-013-5167-4
Polak, J., and Jarosz-Wilkolazka, A. (2012). Fungal laccases as green catalysts for dye synthesis. Process Biochem. 47, 1295–1307. doi: 10.1016/j.procbio.2012.05.006
Rakvin, B., Carić, D., Andreis, M., Junker, K., and Walde, P. (2014). EPR study of polyaniline synthesized enzymatically in the presence of submicrometer-sized AOT vesicles. J. Phys. Chem. B 118, 2205–2213. doi: 10.1021/jp411204b
Reiss, R., Ihssen, J., Richter, M., Eichhorn, E., Schilling, B., and Thöny-Meyer, L. (2013). Laccase versus laccase-like multi-copper oxidase: a comparative study of similar enzymes with diverse substrate spectra. PLoS ONE 8:e65633. doi: 10.1371/journal.pone.0065633
Riva, S. (2006). Laccases: blue enzymes for green chemistry. Trends Biotechnol. 24, 219–226. doi: 10.1016/j.tibtech.2006.03.006
Rodríguez Couto, S., and Toca Herrera, J. L. (2006). Industrial and biotechnological applications of laccases: a review. Biotechnol. Adv. 24, 500–513. doi: 10.1016/j.biotechadv.2006.04.003
Roth, S., and Spiess, A. C. (2015). Laccases for biorefinery applications: a critical review on challenges and perspectives. Bioprocess Biosyst. Eng. 38, 2285–2313. doi: 10.1007/s00449-015-1475-7
Serrano-Luginbühl, S., Ruiz-Mirazo, K., Ostaszewski, R., Gallou, F., and Walde, P. (2018). Soft and dispersed interface-rich aqueous systems that promote and guide chemical reactions. Nat. Rev. Chem. 2, 306–327. doi: 10.1038/s41570-018-0042-6
Shumakovich, G., Kurova, V., Vasil'eva, I., Pankratov, D., Otrokhov, G., Morozova, O., et al. (2012a). Laccase-mediated synthesis of conducting polyaniline. J. Mol. Catal. B Enzym. 77, 105–110. doi: 10.1016/j.molcatb.2012.01.023
Shumakovich, G., Otrokhov, G., Vasil'eva, I., Pankratov, D., Morozova, O., and Yaropolov, A. (2012b). Laccase-mediated polymerization of 3,4-ethylenedioxythiophene (EDOT). J. Mol. Catal. B Enzym. 81, 66–68. doi: 10.1016/j.molcatb.2012.05.008
Shumakovich, G., Streltsov, A., Gorshina, E., Rusinova, T., Kurova, V., Vasil'eva, I., et al. (2011). Laccase-catalyzed oxidative polymerization of aniline dimer (N-phenyl-1,4-phenylenediamine) in aqueous micellar solution of sodium dodecylbenzenesulfonate. J. Mol. Catal. B Enzym. 69, 83–88. doi: 10.1016/j.molcatb.2011.01.016
Shumakovich, G. P., Otrokhov, G. V., Khlupova, M. E., Vasil'eva, I. S., Zaitseva, E. A., Morozova, O. V., et al. (2014). Laccase-catalyzed synthesis of aniline oligomers and their application for the protection of copper against corrosion. RSC Adv. 4, 30193–30196. doi: 10.1039/C4RA04836A
Shumakovich, G. P., Vasil'eva, I. S., Morozova, O. V., Khomenkov, V. G., Staroverova, I. N., Budashov, I. A., et al. (2010). A comparative study of water dispersible polyaniline nanocomposites prepared by laccase-catalyzed and chemical methods. J. Appl. Polym. Sci. 117, 1544–1550. doi: 10.1002/app.32008
Sirim, D., Wagner, F., Wang, L., Schmid, R. D., and Pleiss, J. (2011). The laccase engineering database: a classification and analysis system for laccases and related multicopper oxidases. 2011:bar006. doi: 10.1093/database/bar006
Slagman, S., Zuilhof, H., and Franssen, M. C. R. (2018). Laccase-mediated grafting on biopolymers and synthetic polymers: a critical review. ChemBioChem 19, 288–311. doi: 10.1002/cbic.201700518
Solomon, E. I., Heppner, D. E., Johnston, E. M., Ginsbach, J. W., Cirera, J., Quayyum, M., et al. (2014). Copper active sites in biology. Chem. Rev. 114, 3659–3853. doi: 10.1021/cr400327t
Solomon, E. I., Sundaram, U. M., and Machonkin, T. E. (1996). Multicopper oxidases and oxygenases. Chem. Rev. 96, 2563–2605. doi: 10.1021/cr950046o
Song, H.-K., and Palmore, G. T. R. (2005). Conductive polypyrrole via enzymatic catalysis. J. Phys. Chem. B 109, 19278–19287. doi: 10.1021/jp0514978
Sousa, A. C., Martins, L. O., and Robalo, M. P. (2013). Laccase-catalysed homocoupling of primary aromatic amines towards the biosynthesis of dyes. Adv. Synth. Catal. 355, 2908–2917. doi: 10.1002/adsc.201300501
Stejskal, J., Trchová, M., Bober, P., Humpolíček, P., Kašpárková, V., Sapurina, I., et al. (2015). “Conducting polymers: polyaniline,” in Encyclopedia of Polymer Science and Technology, 4th Edn., ed H. F. Mark (Chichester: John Wiley and Sons), 1–44. doi: 10.1002/0471440264.pst640
Sterjiades, R., Dean, J. F., and Eriksson, K. E. (1992). Laccase from sycamore maple (Acer pseudoplatanus) polymerizes monolignols. Plant Physiol. 99, 1162–1168. doi: 10.1104/pp.99.3.1162
Streltsov, A. V., Morozova, O. V., Arkharova, N. A., Klechkovskaya, V. V., Staroverova, I. N., Shumakovich, G. P., et al. (2009). Synthesis and characterization of conducting polyaniline prepared by laccase-catalyzed method in sodium dodecylbenzenesulfonate micellar solutions. J. Appl. Polym. Sci. 114, 928–934. doi: 10.1002/app.30591
Streltsov, A. V., Shumakovich, G. P., Morozova, O. V., Gorbacheva, M. A., and Iaropolov, A. I. (2008). Micellar laccase-catalyzed synthesis of electroconductive polyaniline. Appl. Biochem. Microbiol. 44, 264–270. doi: 10.1134/S000368380803006X
Strong, P. J., and Claus, H. (2011). Laccase: a review of its past and its future in bioremediation. Crit. Rev. Environ. Sci. Technol. 41, 373–434. doi: 10.1080/10643380902945706
Su, J., Fu, J., Wang, Q., Silva, C., and Cavaco-Paulo, A. (2018a). Laccase: a green catalyst fort he bioynthesis of poly-phenols. Crit. Rev. Biotechnol. 38, 294–307. doi: 10.1080/07388551.2017.1354353
Su, J., Noro, J., Fu, J., Wang, Q., Silva, C., and Cavaco-Paulo, A. (2019b). Coloured and low conductive fabrics by in situ laccase-catalysed polymerization. Process Biochem. 77, 77–84. doi: 10.1016/j.procbio.2018.11.007
Su, J., Noro, J., Silva, S., Fu, J., Wang, Q., Ribeiro, A., et al. (2019a). Antimicrobial coating of textiles by laccase in situ polymerization of catechol and p-phenylenediamine. React. Funct. Polym. 136, 25–33. doi: 10.1016/j.reactfunctpolym.2018.11.015
Su, J., Shim, E., Noro, J., Fu, J., Wang, Q., Kim, H. R., et al. (2018b). Conductive cotton by in situ laccase-polymerization of aniline. Polymers 10:1023. doi: 10.3390/polym10091023
Sun, X., Bai, R., Zhang, Y., Wang, Q., Fan, X., Yuan, J., et al. (2013). Laccase-catalyzed oxidative polymerization of phenolic compounds. Appl. Biochem. Biotechnol. 171, 1673–1680. doi: 10.1007/s12010-013-0463-0
Tadesse, M. A., D'Annibale, A., Galli, C., Gentili, P., and Sergi, F. (2008). An assessment of the relative contributions of redox and steric issues to laccase specificity towards putative substrates. Org. Biomol. Chem. 6, 868–878. doi: 10.1039/b716002j
Tan, Y., Deng, W., Li, Y., Huang, Z., Meng, Y., Xie, Q., et al. (2010). Polymeric bionanocomposite cast thin films with in situ laccase-catalyzed polymerization of dopamine for biosensing and biofuel cell applications. J. Phys. Chem. B 114, 5016–5024. doi: 10.1021/jp100922t
Tolbert, A., Akinosho, H., Khunsupat, R., Naskar, A. K., and Ragauskas, A. J. (2014). Characterization and analysis of the molecular weight of lignin for biorefining studies. Biofuels, Bioprod. Bioref. 8, 836–856. doi: 10.1002/bbb.1500
Tsujimoto, T., Uyama, H., and Kobayashi, S. (2001). Polymerization of vinyl monomers using oxidase catalyst. Macromol. Biosci. 1, 228–232. doi: 10.1002/1616-5195(20010801)1:6<228::AID-MABI228>3.0.CO;2-S
Upadhyay, P., Shrivastava, R., and Agrawal, P. K. (2016). Bioprospecting and biotechnological applications of fungal laccase. 3 Biotech. 6:15. doi: 10.1007/s13205-015-0316-3
Vasil'eva, I. S., Morozova, O. V., Shumakovich, G. P., and Iaropolov, A. I. (2009). Synthesis of electroconductive polyaniline using immobilized laccase. Appl. Biochem. Microbiol. 45, 27–30. doi: 10.1134/S0003683809010050
Vasil'eva, I. S., Morozova, O. V., Shumakovich, G. P., Shleev, S. V., Sakharov, I. Y., and Yaropolov, A. I. (2007). Laccase-catalyzed synthesis of optically active polyaniline. Synth. Met. 157, 684–689. doi: 10.1016/j.synthmet.2007.07.018
Vasil'eva, I. S., Shumakovich, G. P., Morozova, O. V., Khlupova, M. E., Vasiliev, R. B., Zaitseva, E. A., et al. (2018). Efficiency of a fungal laccase in 3,4-ethylenedioxythiophene polymerization. Chem. Pap. 72, 1499–1505. doi: 10.1007/s11696-018-0396-8
Walde, P., and Guo, Z. (2011). Enzyme-catalyzed chemical structure-controlling template polymerization. Soft Matter 7, 316–331. doi: 10.1039/C0SM00259C
Wong, D. W. (2009). Structure and action mechanism of ligninolytic enzymes. Appl. Biochem. Biotechnol. 157, 174–209. doi: 10.1007/s12010-008-8279-z
Xia, Y., Wiesinger, J. M., and MacDiarmid, A. G. (1995). Camphorsulfonic acid fully doped polyaniline emeraldine salt: conformations in different solvents studied by an ultraviolet/visible/near-infrared spectroscopic method. Chem. Mater. 7, 443–445. doi: 10.1021/cm00051a002
Xu, F. (1996). Oxidation of phenols, anilines, and benzenethiols by fungal laccases: correlation between activity and redox potentials as well as halide inhibition. Biochemistry 35, 7608–7614. doi: 10.1021/bi952971a
Yang, J., Li, W., Ng, T. B., Deng, X., Lin, J., and Ye, X. (2017). Laccases: production, expression regulation, and applications in pharmaceutical biodegradation. Front. Microbiol. 8:832. doi: 10.3389/fmicb.2017.00832
Yoo, J. E., Cross, J. L., Bucholz, T. L., Lee, K. S., Espe, M. P., and Loo, Y.-L. (2007). Improving the electrical conductivity of polymer acid-doped polyaniline by controlling the template molecular weight. J. Mater. Chem. 17, 1268–1275. doi: 10.1039/b618521e
Zhang, T., Bai, R., Shen, J., Wang, Q., Wang, P., Yuan, J., et al. (2018). Laccase-catalyzed polymerization of diaminobenzenesulfonic acid for pH-responsive color-changing and conductive wool fabrics. Text. Res. J. 88, 2258–2266. doi: 10.1177/0040517517720497
Zhang, Y., Dong, A., Fan, X., Wang, Q., Zhang, Y., Yu, Y., et al. (2016). Laccase-catalyzed synthesis of conducting polyaniline-lignosulfonate composite. J. Appl. Polym. Sci. 133:42941. doi: 10.1002/app.42941
Zhang, Y., Dong, A., Wang, Q., Fan, X., Cavaco-Paulo, A., and Zhang, Y. (2014). Conductive cotton prepared by polyaniline in situ polymerization using laccase. Appl. Biochem. Biotechnol. 174, 820–831. doi: 10.1007/s12010-014-1094-9
Keywords: aniline, enzyme, lignin, laccase, peroxidase, polymerization, polyaniline, template
Citation: Walde P, Kashima K and Ćirić-Marjanović G (2019) Synthesizing Polyaniline With Laccase/O2 as Catalyst. Front. Bioeng. Biotechnol. 7:165. doi: 10.3389/fbioe.2019.00165
Received: 10 May 2019; Accepted: 24 June 2019;
Published: 10 July 2019.
Edited by:
Artur M. Cavaco-Paulo, University of Minho, PortugalReviewed by:
Carla Silva, University of Minho, PortugalQiang Wang, Jiangnan University, China
Gibson S. Nyanhongo, University of Natural Resources and Life Sciences Vienna, Austria
Copyright © 2019 Walde, Kashima and Ćirić-Marjanović. This is an open-access article distributed under the terms of the Creative Commons Attribution License (CC BY). The use, distribution or reproduction in other forums is permitted, provided the original author(s) and the copyright owner(s) are credited and that the original publication in this journal is cited, in accordance with accepted academic practice. No use, distribution or reproduction is permitted which does not comply with these terms.
*Correspondence: Peter Walde, cGV0ZXIud2FsZGVAbWF0LmV0aHouY2g=