- 1Department of Biological Sciences, National University of Singapore, Singapore, Singapore
- 2Division of Surgery, Yong Loo Lin School of Medicine, National University of Singapore, Singapore, Singapore
Even under normoxia, cancer cells exhibit increased glucose uptake and glycolysis, an occurrence known as the Warburg effect. This altered metabolism results in increased lactic acid production, leading to extracellular acidosis and contributing to metastasis and chemoresistance. Current pH imaging methods are invasive, costly, or require long acquisition times, and may not be suitable for high-throughput pre-clinical small animal studies. Here, we present a ratiometric pH-sensitive bioluminescence reporter called pHLuc for in vivo monitoring of tumor acidosis. pHLuc consists of a pH-sensitive GFP (superecliptic pHluorin or SEP), a pH-stable OFP (Antares), and Nanoluc luciferase. The resulting reporter produces a pH-responsive green 510nm emission (from SEP) and a pH-insensitive red-orange 580nm emission (from Antares). The ratiometric readout (R580/510) is indicative of changes in extracellular pH (pHe). In vivo proof-of-concept experiments with NSG mice model bearing human synovial sarcoma SW982 xenografts that stably express the pHLuc reporter suggest that the level of acidosis varies across the tumor. Altogether, we demonstrate the diagnostic value of pHLuc as a bioluminescent reporter for pH variations across the tumor microenvironment. The pHLuc reporter plasmids constructed in this work are available from Addgene.
Introduction
A hallmark of neoplastic diseases is the reprogramming of cellular energy metabolism to actively support cell proliferation (Hanahan and Weinberg, 2011). Unlike normal cells, cancer cells display an increased rate of glycolysis even under normal oxygen conditions. This “Warburg effect” leads to excessive production of lactic acid, and acidification of the tumor microenvironment, with the extracellular pH (pHe) dropping to as low as 6.4 (Chen et al., 2015). Tumor acidosis has been shown to promote invasion, metastasis, and drug resistance due to neutralization of weak base chemotherapeutic drugs, resulting in aggressive cancer phenotypes and ultimately, reduced patient survival (Chen et al., 2015; Corbet and Feron, 2017; Pillai et al., 2019).
Despite the significance of studying the role of pHe in tumor progression, limited methods exist to monitor the pHe of tumors in vivo. Measuring the pH of local tissues via invasive methods such as the surgical insertion of pH microelectrodes, may trigger inflammatory processes that can, by itself, alter the pH (Menkin, 1933; Chen et al., 2015). In vivo imaging approaches utilizing pH sensitive magnetic resonance imaging (MRI) dyes (Sun and Gregory Sorensen, 2008; Hashim et al., 2011; Chen and Pagel, 2015; Longo et al., 2016) or positron emission tomography (PET) dyes tagged to the pH-sensitive pHLIP peptide (Reshetnyak et al., 2007; Chen and Pagel, 2015) require costly equipment and lengthy image acquisition times. On the other hand, a genetically encoded pH-sensitive luminescence reporter would provide a simple and inexpensive means to study the pHe of tumors in vivo. However, to the best of our knowledge, no such bioluminescence pH reporter exists hitherto.
Superecliptic pHluorin (SEP) is a mutant of GFP that is widely used in vitro as a fluorescence reporter of pH, and is nearly non-fluorescent at pH 6 but brightly green fluorescent at pH 7.4 (Miesenböck et al., 1998). However, SEP is ill-suited for in vivo imaging due the high background autofluorescence, typically encountered during in vivo fluorescence imaging (Puaux et al., 2011). Due to the high background autofluorescence brought about by fluorescent probes, in vivo imaging is most commonly performed with luminescent reporters such as Firefly or Renilla luciferase reporters, and the more recent Nanoluc luciferase reporters (Schaub et al., 2015). Nanoluc reporters hold many advantages over Firefly or Renilla luciferase, being 100-fold brighter and not requiring ATP as a substrate. The ATP-free reaction allows Nanoluc to be used in the ATP-deficient extracellular space (Pfleger and Eidne, 2006; Hall et al., 2012). Thus, an ideal reporter to study the pHe of tumors in vivo would possess the excellent pH-sensitivity of SEP and the bright extracellular luminescent signal potential of Nanoluc.
Here, we describe a genetically encoded luminescence reporter, pHLuc, which combines the pH-sensitivity of SEP with the bright extracellular luminescent signal of Nanoluc to allow for the in vivo whole animal imaging of tumor pHe (Figure 1). The pHluc system consists of two bioluminescent reporters, SEPLuc and Antares. SEPLuc is an optimized fusion of SEP and Nanoluc that is anchored to the cell surface via glycosylphosphatidylinositol (GPI). Through efficient bioluminescence resonance energy transfer (BRET) of the donor Nanoluc signal to pH-sensitive SEP, SEPLuc has a pH-sensitive green emission that peaks at 510 nm and is progressively reduced from pHe 7.4 to 6. SEPLuc is bicistronically co-expressed with Antares, a cytoplasmic Nanoluc fusion that utilizes the same furimazine substrate but has pH-insensitive red-orange emission that peaks at 580 nm (Chu et al., 2016). By obtaining the bioluminescence emission ratio of SEPLuc over Antares (R580/510), the pHLuc reporter controls for pH-independent confounding variables such as changes in reporter expression, substrate availability, optical path length, movement, and growth artifacts (Thestrup et al., 2014). Through in vivo imaging, the R580/510 bioluminescence emission ratio of the resulting pHLuc reporter showed that human synovial sarcoma SW982 xenografts in NSG mice displayed acidosis that varied across the tumor with lower pH values observed at the center of the tumors. On the whole, pHLuc is a ratiometric bioluminescent reporter of tumor pH that allows for the non-invasive monitoring of tumor acidosis in mice via IVIS Spectrum whole animal imaging.
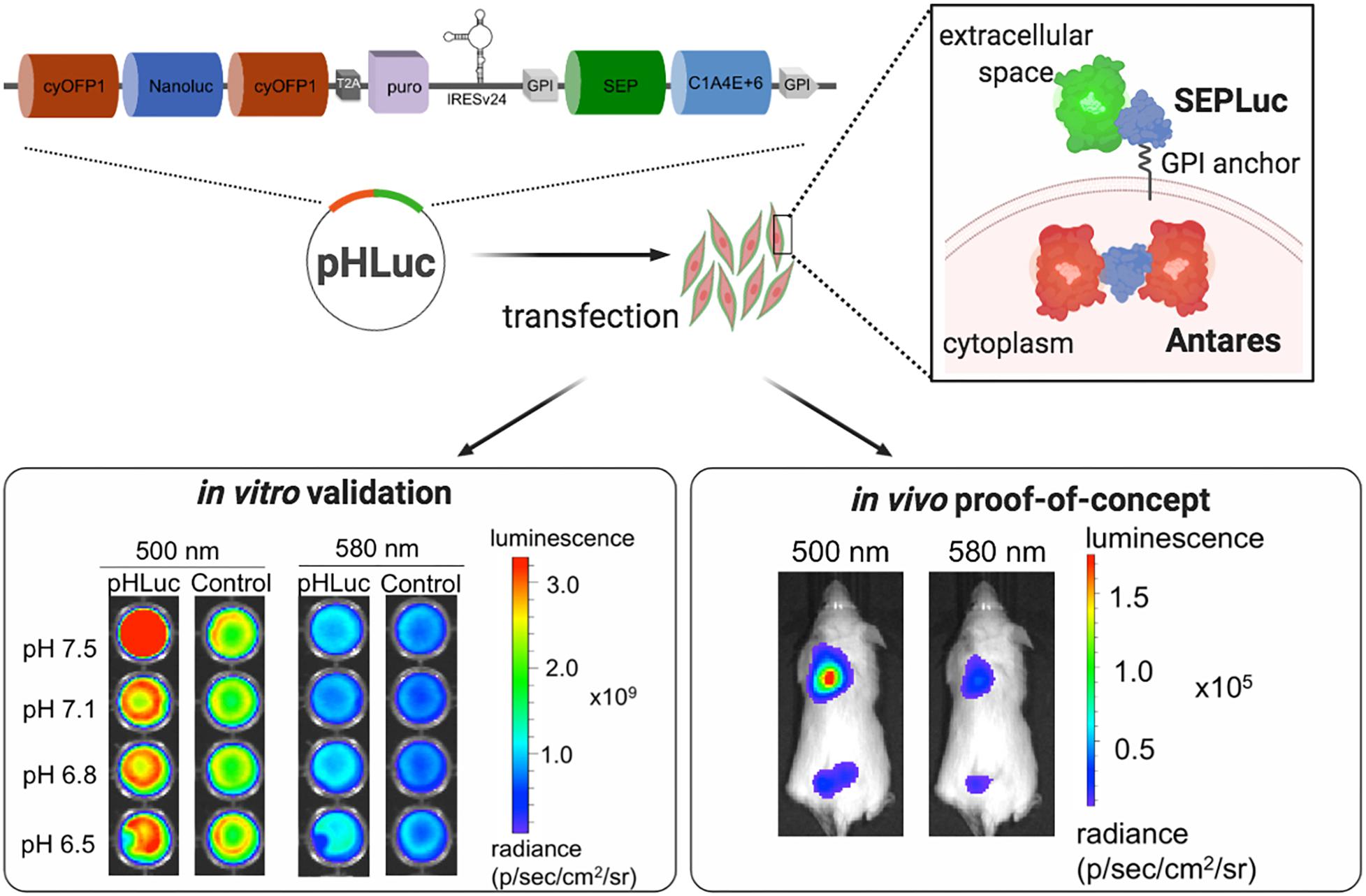
Figure 1. Overview of the pHLuc system and experimental workflow. The pHLuc reporter is a multicistronic cassette that comprises of cytoplasmic Antares, T2A-puromycin, a mutated internal ribosome entry site (IRES) v24, and a membrane-bound SEPLuc that utilizes a weaker variant of Nanoluc. Cells transfected with pHLuc have shown that the reporter is pH-responsive compared to a pH-stable control. Since the SEP signal is pH-dependent, the ratio of SEP to Antares (R580/510) is indicative of changes in extracellular pH. When cells stably expressing pHLuc were injected into mice, bioluminescence imaging also showed differences in the ratiometric readout. In vivo ratiometric images showed the capacity of pHLuc to detect extracellular pH changes. Image generated via BioRender.
Results
Engineering a SEP-Nanoluc Fusion for Bioluminescence pH Sensing
To develop a ratiometric pH-sensitive luminescent reporter, we first fused Nanoluc downstream of SEP through a flexible, five-residue linker sequence (Asp-Ile-Ser-Gly-Gly). This linker was recently described to optimize the folding of the two proteins (Nanoluc and eGFP) while maintaining close proximity for efficient energy transfer via BRET (Schaub et al., 2015). To constitutively express this fusion construct as a membrane protein, the N- and C-termini signals of the GPI anchor sequence were fused to the 5′ and 3′ ends of the fusion construct, respectively; henceforth, this membrane-bound SEP-Nanoluc fusion is termed SEPLuc. This initial construct was transiently transfected into HEK293T cells. Fluorescence microscopy confirmed the localization of SEP to the cell membrane (Figure 2A, left). Bioluminescence assays confirmed the capacity of SEPLuc to detect pHe change in vitro. As the pH of the MOPS buffer was gradually decreased from 7.5 to 6.5, we observed a progressive reduction in the 510 nm emission (Figure 2A, right).
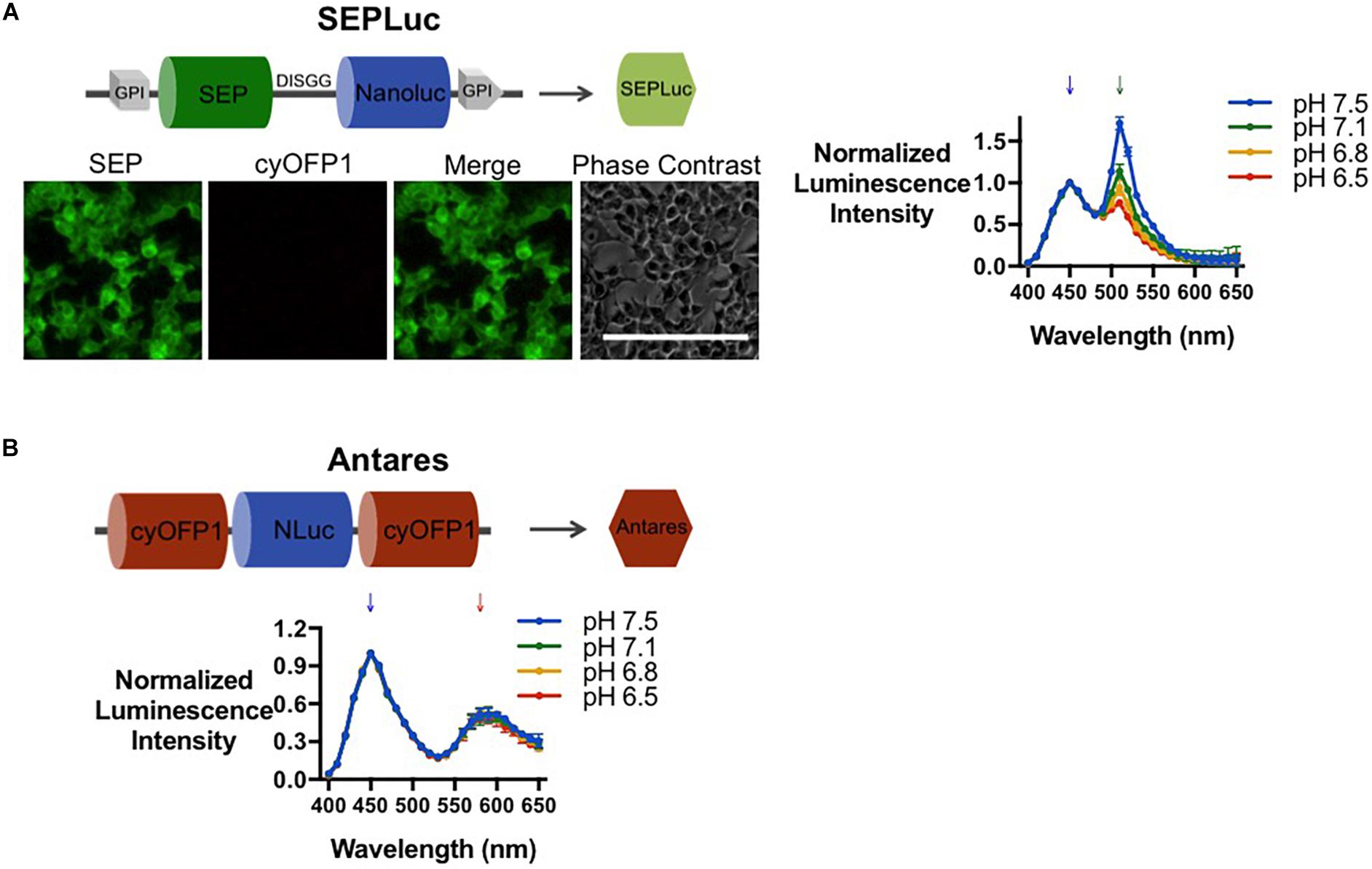
Figure 2. Characterization of the Nanoluc-fluorescent protein fusions expressed in HEK293T cells. (A) Microscopy and luciferase assay of SEPLuc (n = 2). (B) Luciferase assay of Antares (n = 2). Hereon, SEPLuc and Antares will be depicted as green and red shapes as indicated above (diagrams not drawn to scale). Downward arrows on spectral scan values indicate the wavelengths at which peaks are expected (450 nm for Nanoluc, 510 nm for SEP, and 580 nm for R/OFPs). All luminescence values were normalized to the Nanoluc emission (450 nm). Error bars are SD. Scale bars = 200 μm.
Co-expression of SEPLuc With Antares Generates a Ratiometric Signal
Next, we attempted to identify a suitable pH-insensitive red-orange bioluminescent Nanoluc reporter that can be co-expressed with SEPLuc for in vivo ratiometric sensing. A red-orange bioluminescent partner is necessary because the 460 nm emission of Nanoluc is too blue-shifted for detection by in vivo imaging systems such as the IVIS Spectrum, which can only resolve luminescent emissions with wavelengths between 490 to 850 nm. Given that the signal of SEPLuc started to diminish at around 550 nm onward (Figure 2A), candidate ratiometric partners including the previously described OgNluc, ReNL, and Antares Nanoluc fusion reporters, which produce bioluminescent emission peaks at 572 nm (Schaub et al., 2015), 585 nm (Suzuki et al., 2016), and 584 nm (Chu et al., 2016), respectively, were tested. Among these red-orange reporters, Antares, which consists of cyOFP1s flanking the Nanoluc, displayed the highest BRET-efficiency and the most red-shifted emission (Supplementary Figure 1A). Antares also retained the most prominent 510 and 580 nm emission peaks when co-transfected with the SEPLuc plasmid (Supplementary Figure 1B) and had a 580 mm emission that was insensitive to changes in pHe (Figure 2B). Thus, Antares was chosen as the pH-independent ratiometric partner for SEP-Nanoluc, for further optimization.
Iterative Optimization of the Signal Ratios of SEPLuc and Antares During Bicistronic Co-expression
Our initial reporter prototype was a simple C-terminal fusion of cyOFP1 to SEPLuc (termed NC3) via the same Asp-Ile-Ser-Gly-Gly linker used in Antares (Chu et al., 2016). While cyOFP1 was faintly visible under the microscope (Figure 3A, top), we were unable to detect the 580 nm emission peak corresponding to cyOFP1 in the luciferase assay (Figure 3A, bottom). We surmised that this may be due to the fusion of SEPLuc to cyOFP leading to protein misfolding. To overcome this, the Nanoluc-cyOFP1 fusion was separated from SEPLuc via an E2A cleavage peptide (Liu et al., 2017). However, neither of the fluorescent proteins was detected via microscopy (Figure 3B, top), although a peak corresponding to SEPLuc was detected in luciferase assay (Figure 3B, bottom). Since the E2A cleavage peptide failed, we co-expressed Antares downstream of SEPLuc via an encephalomyocarditis (EMCV) internal ribosome entry site (IRES) sequences. Microscopy showed the presence of both SEP and Antares (Figure 3C, top), but Antares was not detectable in luciferase assay (Figure 3C, bottom). Since IRES-dependent expression of the second gene may be significantly lower (Mizuguchi et al., 2002), we cloned SEPLuc downstream of the IRES to maximize the signal output at 580 nm. The Antares-SEPLuc construct also contains a T2A-puromycin fusion incorporated between Antares and SEPLuc to improve antibiotic selection of stably transfected cells. Microscopy detected both SEP and Antares (Figure 3D, top), but the Antares signal was again absent in the luciferase assay (Figure 3D, bottom).
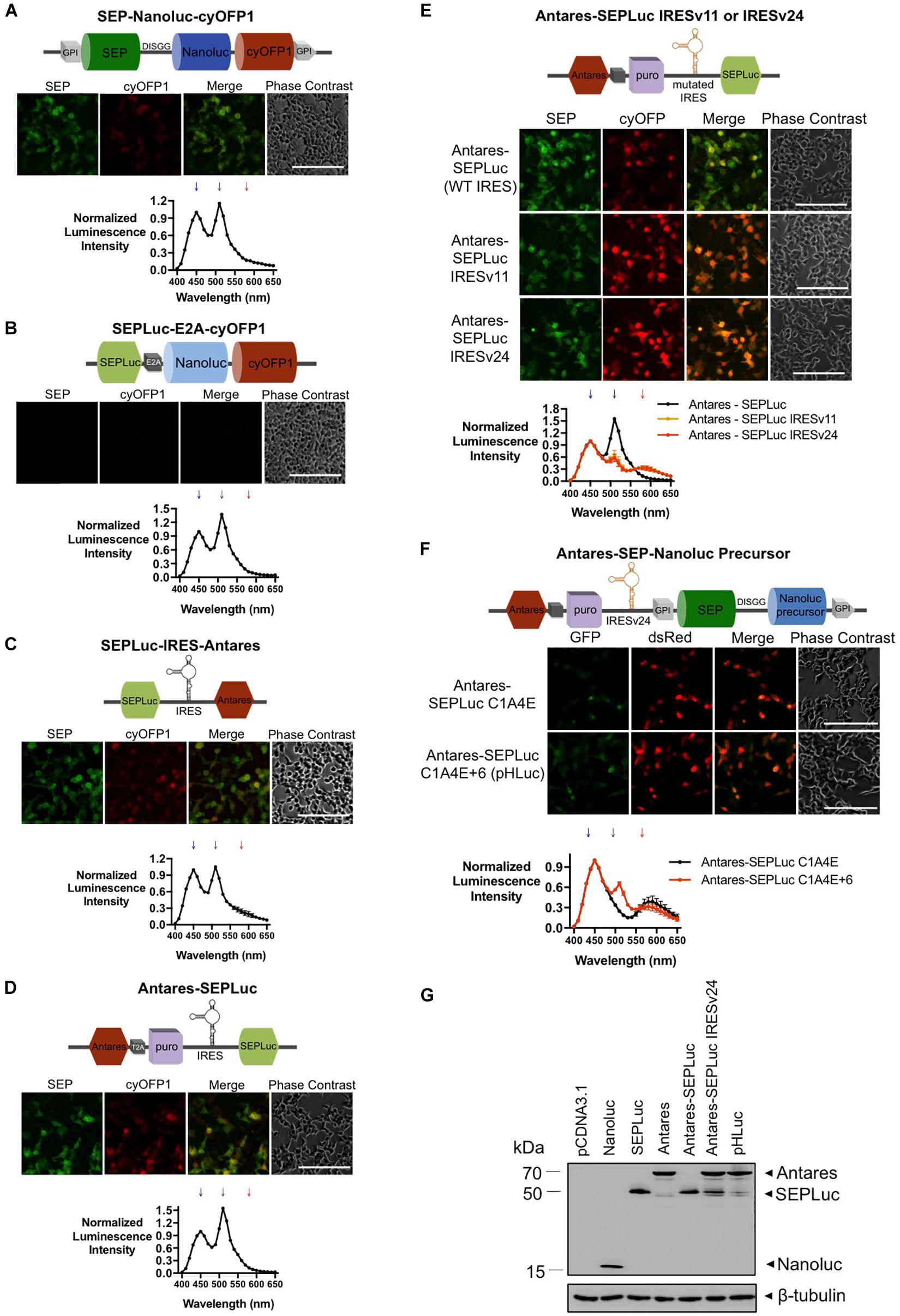
Figure 3. Iterative optimization of the SEPLuc and Antares bioluminescence signal ratios. (A) cyOFP1 was inserted into the existing membrane-anchored SEPLuc fusion via the 5-amino acid linker (n = 2). (B) cyOFP1 was fused to another Nanoluc sequence, then linked downstream of SEPLuc via E2A (n = 2). (C) Antares was cloned downstream of SEPLuc and IRES (n = 2). (D) The Antares-SEPLuc construct. Antares is followed by T2A and puromycin (colored purple), then by IRES and SEPLuc. (n = 3). (E) Comparison of the Antares-SEPLuc constructs utilizing wildtype IRES and the mutant variants IRESv11 and IRESv24 (n = 3). (F) Comparison of the Antares-SEPLuc IRESv24 constructs utilizing two weaker Nanoluc precursors, C1A4E and C1A4E+6, transiently expressed in SW982 cells (n = 2). (G) Western blot of whole cell lysates from SW982 transfected with vector control, Nanoluc, SEPLuc, Antares, Antares-SEPLuc, Antares-SEPLuc IRESv24, and pHLuc. All constructs were transfected into a model cell line, HEK293T for initial assessment of their expression, unless stated otherwise. Downward arrows on spectral scan values indicate the wavelengths at which peaks are expected (450 nm for Nanoluc, 510 nm for SEP, and 580 nm for Antares). Luciferase assay values were normalized to the Nanoluc emission at 450 nm. Error bars are SD.
To further improve the Antares signal relative to that of SEPLuc, we first reduced the efficiency of the IRES in the Antares-SEPLuc construct. We selected IRESv11 and IRESv24, which were previously described to reduce translation efficiency to 24 and 0.34%, respectively (Koh et al., 2013). Microscopy and luciferase assay data showed decreased expression of SEPLuc (Figure 3E). Next, we chose two weaker Nanoluc precursors that were derived from Oplophorus gracilirostris luciferase (Oluc-19): (1) C1A4E, which has eight mutations over Oluc-N166R (a more stable variant of Oluc-19), and (2) a mutant we dubbed C1A4E+6, which has six amino acid changes over C1A4E that contribute to enzyme stability (Hall et al., 2012). Both microscopy and luciferase assays of SW982 cells showed that C1A4E dimmed the SEPLuc signal excessively, while C1A4E+6 resulted in detectable signal at both 510 and 580 nm (Figure 3F). We therefore named this optimized final construct as pHLuc, (Antares-SEPLuc C1A4E+6). Western detection confirmed the presence and size of Antares (70 kDa) and SEPLuc (50 kDa), and showed a decreased expression of SEPLuc upon mutation of the IRES and the Nanoluc (Figure 3G).
pHLuc Can Detect pHe Changes in vitro
To investigate whether the pHLuc reporter would produce a ratiometric signal in response to pHe, we generated SW982 cells that stably expressed either the pHLuc reporter or a pH-stable Control reporter that utilizes eGFP instead of SEP (full construct shown at Figures 4A,B, top). The SEPLuc and Antares components of pHLuc were expected to localize within the cell membrane and cytoplasm, respectively; while the eGFP and the Antares components of the pH-stable control were both expected to localize within the cytoplasm. This was consistent with the widefield microscopy results (Figures 4A,B, left). When subject to a decrease in pHe from 7.5 to 6.5, the pHLuc-expressing cells displayed a progressive decrease in their bioluminescence and fluorescence emission intensity at 510 nm while the emission intensity at 580 nm remained constant (Figure 4A, right and bottom). On the other hand, the bioluminescence and fluorescence emission spectra of cells expressing the pH-stable control remained constant within the same pH range (Figure 4B, right and bottom). This pHe-dependent ratiometric bioluminescence signal was also detectable with the plated cells that were imaged with the IVIS spectrum (Figure 5A). Finally, the R580/510 (emission at 580 nm divided by emission at 510 nm) of the pHLuc reporter was further divided by the R580/510 of the pH-stable control to obtain the R580/510pHLuc/R580/510Control, which showed the expected trend of increasing values as the pHe was reduced from 7.5 to 6.5 (Figure 5B).
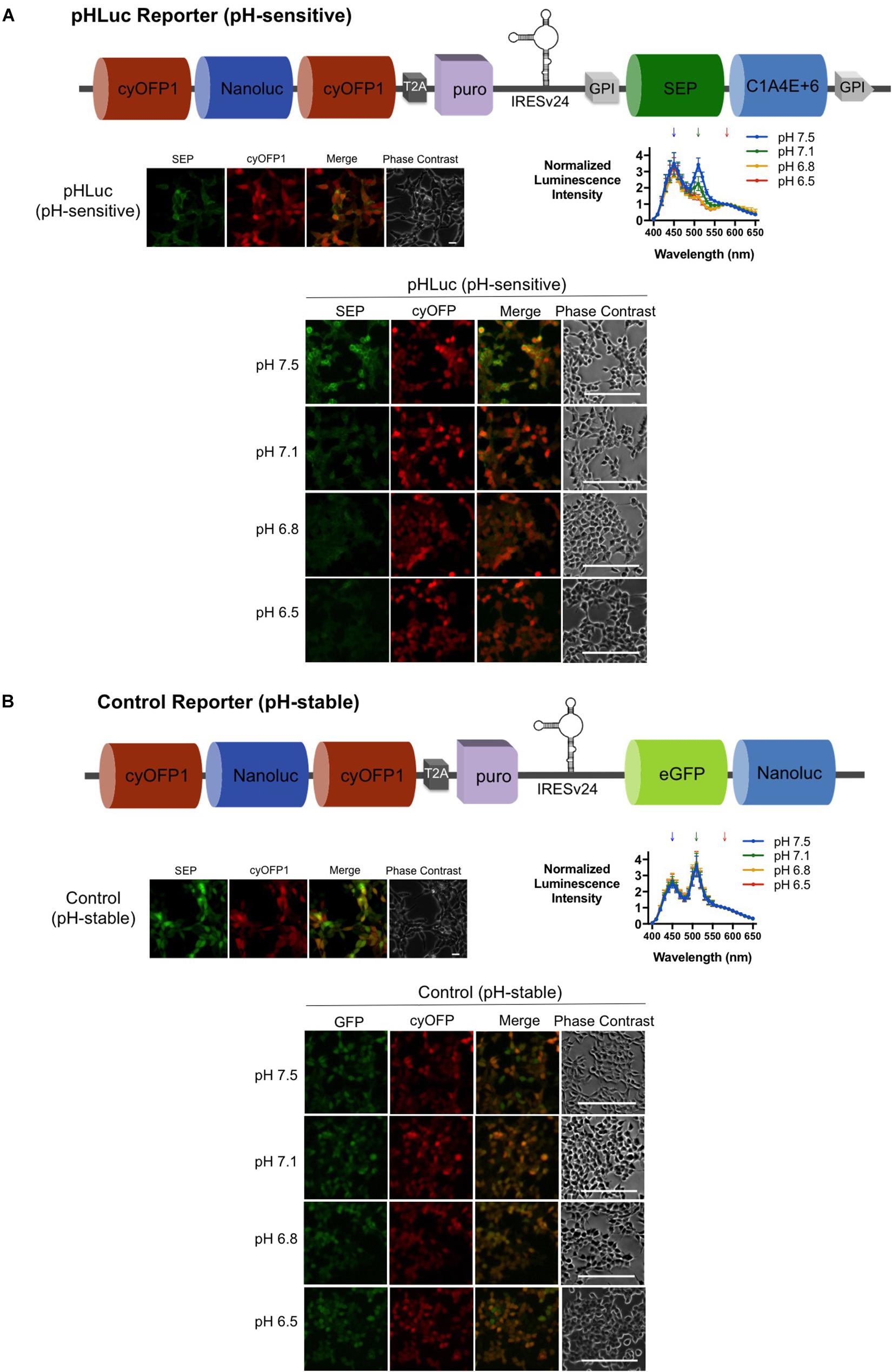
Figure 4. In vitro validation assays for SW982 cells stably expressing (A) pHLuc and (B) the pH-stable Control reporters. (A) Schematic diagram of pHLuc (top). Confocal microscopy of SW982 cells expressing pHLuc showing correct protein localization, scale bars = 20μm (left). Luciferase assay and microscopy of pHLuc-expressing SW982 cells subjected to different pH levels of MOPS buffers, scale bars = 200 μm (right and bottom). (B) Schematic diagram of the pH-stable Control (top). Confocal microscopy of SW982 cells expressing the Control reporter showing correct protein localization, scale bars = 20 μm (left). Luciferase assay and microscopy of Control reporter-expressing SW982 cells subjected to different pH levels of MOPS buffers, scale bars = 200 μm (right and bottom). Downward arrows on spectral scan values indicate the wavelengths at which peaks are expected (450 nm for Nanoluc, 510 nm for SEP, and 580 nm for Antares). Luciferase assay values were normalized to the Nanoluc emission at 450 nm. Error bars are SD.
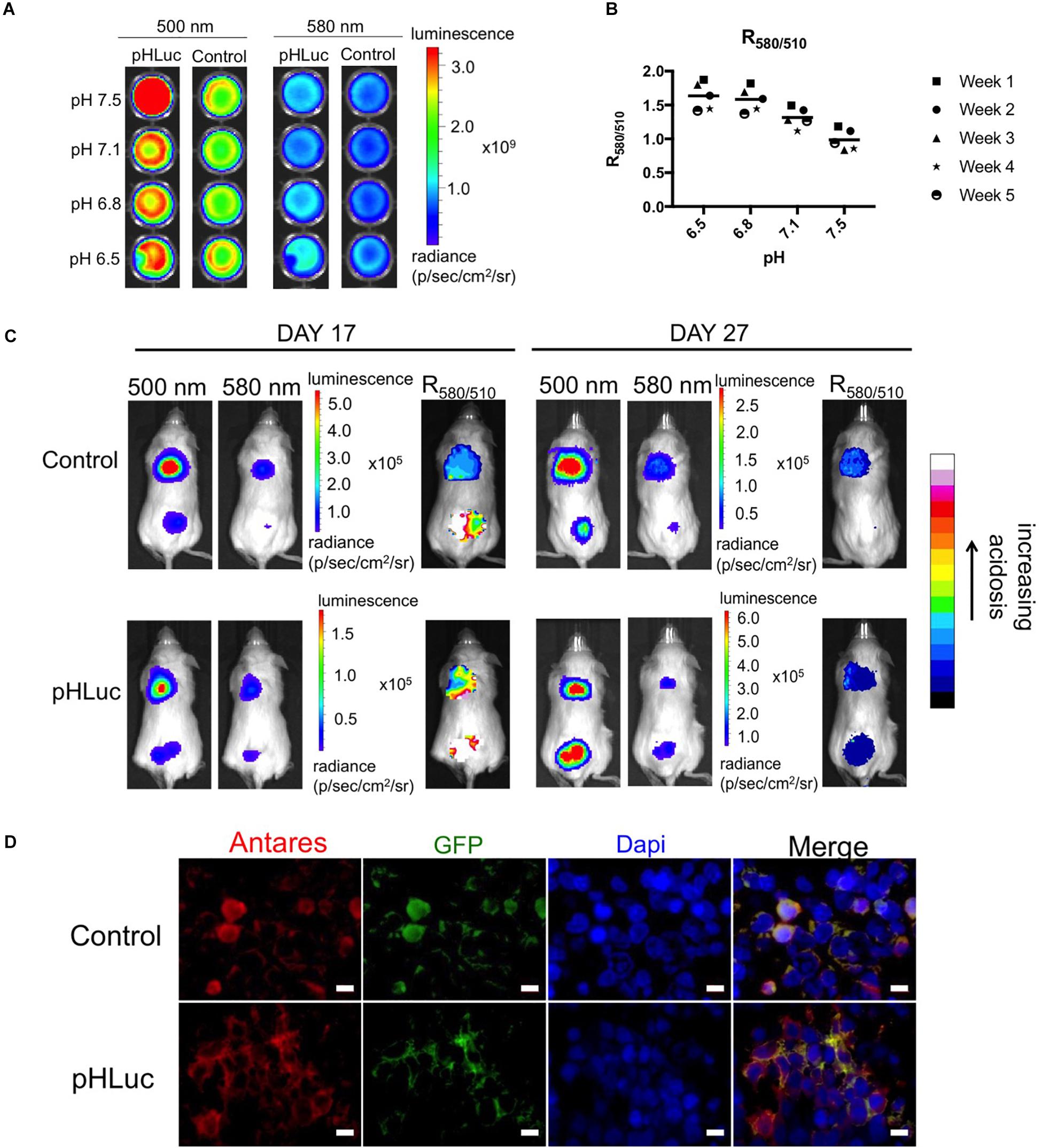
Figure 5. Representative bioluminescence images of pHLuc in vitro and in vivo. (A) Plated SW982 cells expressing the pHLuc and Control reporters, imaged under the 500 and 580 nm filters of IVIS. (B) R580/510pHLuc normalized with R580/510Control of stably expressing SW982 cells. The cells were imaged using the IVIS once a week. Images ratios were obtained using ImageJ. (C) Representative NSG mice (one for pHLuc and one for control) injected with SW982 cells expressing the respective reporters, were imaged under the 500 and 580 nm filters of IVIS on days 17 and 27. The ratiometric images (right panel of each time point) were obtained via ImageJ. (D) Fluorescence immunohistochemical analysis of representative tumor sections for SEP/eGFP (green) and Antares (red) expression. Nuclei (blue) were stained with Dapi. Analyses were done at the endpoint when animals were sacrificed. Scale bar = 10 μm.
pHLuc Detected Variations in pHe Across SW982 Tumor Xenografts in Mice
As a proof-of-concept, we tested the reporters in vivo in NSG mice. Figure 5C shows representative images of mice injected with the SW982 cells expressing the respective reporter. The 500 and 580 nm images show that the signal is brightest at the center of the tumor. The images showing R580/510 displayed a gradient of colors within a representative tumor, indicating a difference in pH distribution within the tumor, likely due to hypoxia and greater changes in cell metabolism in some areas. Ratiometric images of mice injected with pHLuc-expressing cells also displayed comparatively greater ratio variability, and hence pH, compared to the mice injected with control cells. Immunohistochemistry of tumor sections showed that cells that express SEP (for pHLuc tumors) or eGFP (for control tumors) also co-express Antares. The difference in localization of SEP and eGFP can also be seen (Figure 5D). Altogether, the data from the pHluc reporter suggests that the pHe varies across SW982 tumors in NSG mice.
Discussion
Most cancer cells have been reported to have pHi of around 7.4, and pHe of around 6.7–7.1 (Webb et al., 2011). This reversed pH gradient, often referred to as tumor acidosis, is now being considered a hallmark of invasive cancers (Chiche et al., 2010). Given the significant role of pHe in tumor progression, we present a ratiometric luminescent reporter called pHLuc that allows for live imaging of pH change with tumor progression. The pHLuc reporter detected variations in pHe across SW982 tumor xenografts in NSG mice, a finding that is consistent with other reporter systems (Anemone et al., 2019).
The initial optimizations of the pHLuc reporter utilized only cyOFP1, cloned as a fusion to SEP-Nanoluc and separately with its own Nanoluc in a bicistronic cassette. In both instances, the cyOFP1 signal was too low to be detected via microscopy and luciferase assay. In succeeding optimizations, the full Antares construct (consisting of cyOFP1s flanking the Nanoluc) was cloned upstream of SEP-Nanoluc, resulting in a detectable signal emission corresponding to Antares. However, our preliminary optimizations consistently showed that the SEP signal was higher than that of Nanoluc only, and previous studies have shown that the signal of Antares relative to Nanoluc only, is weaker (Chu et al., 2016). This may explain why the emission of Antares, although apparent, was weaker and was masked by the very strong signal of SEP. Thus, for luciferase assay data of the initial constructs (Figures 3A–E) only showed the normalized intensity because the cyOFP1 or Antares signal peak was not visible, and we were unable to obtain signal ratios. To further improve the signal of Antares in the pHLuc reporter, IRES was employed and mutated to modulate the expression of the SEP-Nanoluc, and a weaker variant of the Nanoluc was fused to SEP to reduce the green signal output. In vitro validation assays demonstrated the functionality of the reporter, and in vivo experiments showed the capacity of pHLuc to monitor pH distribution within a tumor.
To the best of our knowledge, the pHLuc reporter is the first genetically encoded pH-sensitive luminescent reporter that would provide a simple and inexpensive means to study the pHe of tumors in vivo. An advantage of utilizing BRET for pHLuc is that the reporter ultimately combines the benefits of the bright fluorophore signal and the low background of a luciferase (Dale et al., 2019; Kobayashi et al., 2019). Moreover, this reporter provides the advantage of dual-color imaging with the use of a single furimazine substrate, and allows for the monitoring of pH changes in small rodents. Previous luminescent reporters based on firefly luciferase (Gabriel and Viviani, 2014) were only applicable for measuring intracellular pH as firefly luciferase requires ATP found in the cytoplasm as a substrate. By using Nanoluc, which does not require ATP and is active in the extracellular space, the pHLuc reporter is able to report on the pHe of tumors in vivo.
Using a genetically encoded reporter such as pHLuc is preferable to measuring the pHe of tumors in vivo via the surgical insertion of pH microelectrodes, which may trigger inflammatory processes that can by itself, alter the pH (Menkin, 1933; Chen et al., 2015). Other in vivo imaging approaches such as pH-sensitive MRI dyes (Sun and Gregory Sorensen, 2008; Hashim et al., 2011; Chen and Pagel, 2015; Longo et al., 2016) or PET dyes tagged to the pH-sensitive pHLIP peptide (Reshetnyak et al., 2007; Chen and Pagel, 2015), all require lengthy image acquisition times and costly MRI or PET imaging systems that are not as widespread as the IVIS Spectrum In Vivo Imaging System (PerkinElmer). These dye based approaches also suffer from poor uptake of the dye into the tumor whereas the genetically encoded pHluc reporter is expressed throughout the tumor (Anemone et al., 2019). A current disadvantage of luminescent reporters such as the pHLuc reporter is that it can only be used to acquire 2D images while PET- or MRI-based approaches have 3D acquisition capabilities. Nevertheless, improvements in bioluminescent in vivo imaging technologies could potentially further enhance the sensitivity and resolution of the pHLuc reporter.
In our experiments, the pHLuc reporter did not achieve a high enough signal-to-noise ratio for us to obtain quantitative measurements via comparisons with in vitro pH standards. The problem of a low signal was particularly pronounced for the red emission peak of the Antares reporter (Figure 2B). Even in our final reporter design (the IRESv24 and C1A4E+6 construct or pHLuc) the red emission peak of Antares was still significantly weaker than the green emission peak of pHLuc (Figure 3F). The red emission of Antares in the dual color construct was only achieved after multiple iterative mutations to reduce the expression level and activity of SEPLuc, which resulted in a weaker ratiometric signal overall (Figure 3). The development of a luciferase with a brighter red emission relative to Antares and its integration into the pHLuc reporter system would no doubt lead to a reporter with a better signal-to-noise ratio. This reporter would also potentially allow for pH quantification by comparing the in vivo ratiometric signal to the ratios obtained from in vitro pH standards such as those described in Figures 5A,B.
Here, we describe the generation of pHLuc, a ratiometric bioluminescent reporter of tumor pH that allows for the non-invasive monitoring of tumor acidosis in mice via IVIS whole animal imaging. Alternative approaches such as pH-sensitive MRI dyes (Sun and Gregory Sorensen, 2008; Hashim et al., 2011; Chen and Pagel, 2015; Longo et al., 2016) or PET dyes tagged to the pH-sensitive pHLIP peptide (Reshetnyak et al., 2007; Chen and Pagel, 2015) do work well and have been described in the literature. However, these approaches require costly MRI and PET instrumentation. Our aim here was to develop a bioluminescent reporter that can be acquired with the more commonly available IVIS spectrum imaging system. While this study focused on bioluminescence imaging, the pHLuc reporter system could also potentially be adapted to whole body fluorescence imaging approaches depending on the instrumentation available to the researcher (Leblond et al., 2010). The pHLuc reporter could potentially have applications in determining the relationship of chemotherapy and cancer regression by monitoring tumor microenvironment changes in pH (Mordon et al., 1994; Düwel et al., 2017; Jo et al., 2017). Specifically, our pHLuc reporter could be exploited for preclinical testing of the efficacy of cancer chemotherapeutics in vivo, in cancer-bearing mice models. For example, drugs such as doxorubicin and mitoxantrone have reduced cytotoxicity in acidic pH, while drugs such as chlorambucil and 5-fluorouracil have higher cytotoxicity at acidic pH (Jo et al., 2017). The administration of bicarbonate bases and proton pump inhibitors have also been found to reduce tumor acidosis, growth and metastasis in various mouse models (Estrella et al., 2013; Jin et al., 2014; Xie et al., 2014). This suggests the diagnostic value of pHLuc in reporting differential pH in the tumor microenvironment, further enhancing the potential for pre-clinical drug development and treatments in vivo.
Materials and Methods
Fusion Protein Construction
The DNA sequence consisting of the GPI anchor, SEP, DISGG linker and Nanoluc was generated via DNA synthesis (Integrated DNA Technologies, Singapore). A 5′ primer encoding XbaI and a 3′ primer encoding NotI were used to generate a whole DNA fragment of the said sequence, and this was ligated into the pGEM®-T Easy vector (Promega). The insert was then subcloned into pCDH-EF1-MCS-T2A-Puro (CD520A-1; Systems Biosciences) via XbaI and NotI restriction enzyme digestion. pNCS-Antares (Addgene plasmid # 74279), pcDNA3.1(+)IRES GFP (Addgene plasmid # 51406), pCDNA3.1(+)ReNL (Addgene plasmid # 85203), and pRetroX-Tight-MCS_PGK-OgNLuc (Addgene plasmid # 70186) were generous gifts from Drs. Michael Lin, Kathleen L. Collins, Takeharu Nagai, and Antonio Amelio, respectively. To generate the complete pHLuc fusion protein in the pCDNA3.1(+) vector backbone (Thermo Fisher Scientific), the homologous recombination cloning protocol based on Jacobus and Gross (2015) was used. Briefly, primers containing around 20 base pairs of overlapping sequences were designed to amplify the relevant sequences from the aforementioned plasmids (using Q5 Hi-Fidelity DNA Polymerase; NEB), such that the PCR products amplified from the separate plasmids can easily recombine into a complete pCDNA3.1(+) vector backbone (with the neomycin sequence deleted) containing the full pHLuc sequence when transformed into Escherichia coli. PCR products were digested with 10 units of DpnI (Thermo Fisher Scientific) overnight and were purified using the Wizard SV Gel and PCR Clean-Up System (Promega). At least 100 ng of each purified fragment was then transformed into 80 μL of TOP10 chemically competent E. coli. Screening was done via PCR using the GoTaq® Green Master Mix (Promega). Positive clones were propagated in LB broth containing ampicillin (Merck) diluted to a working concentration of 100 μg/mL, and plasmids were extracted using PureYieldTM Plasmid Miniprep Kit (Promega). The pHluc reporter plasmids described here are deposited with Addgene and are available upon request, from Addgene (Supplementary Table 1).
Microscopy
Human embryonic kidney 293T (HEK293T) cells were cultured in Dulbecco’s Modified Eagle Medium (DMEM; Gibco) supplemented with 10% (v/v) fetal bovine serum (FBS) (HyClone) and 1% penicillin-streptomycin (Gibco), at 37°C with 5% CO2. The cells were seeded in 24-well plates at least 4 h prior to transfection using TurboFectTM transfection reagent (Thermo Fisher Scientific), and were assayed the following day. Briefly, cells were first observed using an Axio Observer inverted microscope (Zeiss) under phase contrast as well as eGFP and dsRed filters to verify the presence of SEP and Antares. Consistent exposure times (3–10 s) were used in taking images under both eGFP and dsRed filters.
In vitro Bioluminescence Characterization Via Luciferase Assay
Transfected cells were detached with 2 mM ethylenediaminetetraacetic acid (EDTA) with 2% FBS in sterile phosphate buffer saline (PBS) (Supplementary Figure 2), and around 150,000 cells were pelleted. The pellet was resuspended in 100 μL of 25 mM 3-morpholinopropane-1-sulfonic acid (MOPS) saline buffer (adjusted to pH 7.5, 7.1, 6.8, and 6.5 using 6 M HCl) containing the Nanoluc substrate (Promega) diluted 1:1000, and dispensed into a black clear-bottom 96-well plate. Luminescence was determined using the SynergyTM MX Microplate Reader (BioTek) by obtaining the spectral scan from 400–650 nm (10-nm step increments; integration time 1 s). Readings were taken one well at a time, with the substrate added to the cells just before reading, to minimize variability. Additionally, a blank reading (substrate only) was obtained using the same plate reader settings. Raw plate reader values were subtracted with the blank values, then normalized with the value corresponding to either Nanoluc (at 450 nm) or Antares (at 580 nm).
Western Blot
SW982 human synovial sarcoma cells were cultured in Roswell Park Memorial Institute (RPMI 1640; Gibco) medium supplemented with 10% HyCloneTM FBS (GE Healthcare) and 1% penicillin-streptomycin (Gibco), at 37°C with 5% CO2. The cells were seeded in 6-well plates at least 6 h prior to transfection using the ViaFectTM transfection reagent (Promega). One day after transfection, cells were harvested and lysed. Total cell lysates were resolved on a denaturing 10% SDS-PAGE gel, and blotted onto a PVDF membrane using the Trans-Blot Turbo Transfer system (Bio-Rad). Samples were then probed with (1) Nanoluc antibody (R&D Systems; MAB100261), resuspended to 306 μg/mL and diluted to 1:200 with 5% milk in TBST, and (2) β-tubulin antibody (Cell Signaling, # 2146), which was supplied in a concentration of 100 μg/mL, and diluted to 1:1000 with the 5% bovine serum albumin (BSA) in TBST. The samples were then probed with the appropriate secondary antibody: (1) α-mouse secondary antibody diluted to 1:2500 in blocking solution for Nanoluc, and (2) α-rabbit secondary antibody diluted to 1:2500 in blocking solution for β-tubulin. The membrane was incubated in substrate ECL HRP substrate (Advansta), and chemiluminescent signals were obtained using the ImageQuant LAS 4000 mini (GE Healthcare Life Sciences).
Generation of Stably Expressing SW982
SW982 human synovial sarcoma cells were cultured in Roswell Park Memorial Institute (RPMI 1640; Gibco) medium supplemented with 10% HyCloneTM FBS (GE Healthcare) and 1% penicillin-streptomycin (Gibco), at 37°C with 5% CO2. The cells were seeded in 6-well plates at least 6 h prior to transfection using the ViaFectTM transfection reagent (Promega). One day after transfection, the media was removed and replaced with RPMI 1640 containing puromycin dihydrochloride (Thermo Fisher Scientific) diluted to a final concentration of 5 μg/mL. The medium containing the selection antibiotic was changed every 3–4 days for 2 weeks, after which the puromycin concentration was lowered to 2 μg/mL for culture maintenance.
Subcutaneous Tumor Xenografts
All mice experiments were performed under guidelines and protocols (R16-1568) approved by the NUS Institutional Animal Care and Use Committee. Each 6-week old NOD.Cg-Prkdcscid Il2rgtm1Wjl/SzJ (NSG) mouse (InVivos, Singapore) was anaesthesized using 2% isoflurane (Attane), and injected subcutaneously at the dorsal side with 5.0 × 105 and 1.0 × 106 SW982 cells resuspended in 100 μL of sterile Hank’s balanced salt solution (HBSS; Gibco).
In vitro pH Calibration
For pH calibration using the IVIS, cells stably expressing both pHLuc and the control were pelleted and resuspended in MOPS buffer of varying pH levels as previously described. The bioluminescence output was taken with the following settings: excitation block, emission 500 and 580 nm (20 nm bandpass filter), aperture f/2, exposure time 1 s, binning 4, and field of view 12.5 cm. As with all luciferase assays done in this study, the substrate was added to the cells only right before the spectral scan. Samples were done in triplicates, and luciferase readings were taken around 1 week apart for at least 4 weeks to ensure consistency of the ratios even as cells are cultured over time.
In vivo Bioluminescence Imaging
In vivo imaging experiments were performed with the IVIS Spectrum (Perkin Elmer). Mice were anaesthesized using 2% isoflurane (Attane), then injected with 100 μL of the Nanoluc substrate (Promega) diluted 1:25 in sterile Hank’s balanced salt solution (HBSS; Gibco). Bioluminescence images were taken with the following settings: excitation block, emission 500 and 580 (20 nm bandpass filter), aperture f/1, exposure time 60 s, binning 8, and field of view 22.5 cm. All mice were imaged within 1 min upon injection with the substrate.
Image Analysis
Image analysis of all in vitro and in vivo data taken using the IVIS was performed using the ImageJ software. Briefly, each raw grayscale TIF file was first processed to remove the background using the “HiLo lookup table,” then by setting the “Auto” contrast. “Despeckle” and “Remove outliers” (set to radius 1.00 and threshold 1.00) were applied to remove the outlier pixels. The division function of the image calculator was then used to compute for the R580/510. “Despeckle” was again applied to the resulting image to remove the noise. For in vitro imaging of plated cells, the mean IVIS R580/510 taken over several weeks was averaged and plotted out against the buffer of known pH level. For the in vivo images, R580/510 was obtained for the control mice and pHLuc mice individually. All in vivo images were presented using the 16-color lookup table. To allow for comparison of a specific mouse across different time points, the same range of maximum and minimum R580/510 pixel values were set for the same mouse across different time points.
Immunohistochemistry
Palpable SW982 tumors of around 1.5 cm3 in volume were obtained from the mice and fixed in 4% paraformaldehyde in phosphate-buffered saline (PBS) for 24–48 h. The tumors were dehydrated, embedded in paraffin, then sectioned into 5 μm-thick samples onto glass slides. Antigen retrieval was performed at around 90°C in 10 mM citrate buffer (pH 6) for around 30 min. Three percent donkey serum in 2% BSA was used as a blocking solution, and samples were subsequently stained with anti-GFP antibody (Abcam) and anti-tRFP antibody (Evrogen), both diluted to 1:500 with blocking buffer. Samples were probed with the appropriate secondary antibodies anti-goat conjugated to AlexaFluor 488 and anti-rabbit conjugated to TRITC, respectively, diluted to 1:400. After washing the samples three times with PBS, mounting medium and a cover slip was placed over the samples. Images were then taken using the Axio Observer inverted microscope (Zeiss) under phase contrast as well as eGFP, dsRed, and Dapi filters to verify the presence of the fluorescent proteins.
Data Availability Statement
The datasets generated for this study are available on request to the corresponding author.
Ethics Statement
The animal study was reviewed and approved by the NUS Institutional Animal Care and Use Committee.
Author Contributions
TO and ZA designed and performed the experiments and drafted the manuscript. RV and RK performed some experiments and contributed to manuscript writing. JT was involved in discussions. JD provided overall supervision of the project and writing of the manuscript.
Funding
This work is supported by grants from the Ministry of Education (MOE: R-154-000-A31-114, R-154-000-A82-114, and R-176-000-198-733).
Conflict of Interest
The authors declare that the research was conducted in the absence of any commercial or financial relationships that could be construed as a potential conflict of interest.
Supplementary Material
The Supplementary Material for this article can be found online at: https://www.frontiersin.org/articles/10.3389/fbioe.2020.00412/full#supplementary-material
References
Anemone, A., Consolino, L., Arena, F., Capozza, M., and Longo, D. L. (2019). Imaging tumor acidosis: a survey of the available techniques for mapping in vivo tumor pH. Cancer Metastasis Rev. 38, 25–49. doi: 10.1007/s10555-019-09782-9
Chen, L. Q., and Pagel, M. D. (2015). Evaluating pH in the extracellular tumor microenvironment using CEST MRI and other imaging methods. Adv. Radiol. 2015, 1–25. doi: 10.1155/2015/206405
Chen, L. Q., Randtke, E. A., Jones, K. M., Moon, B. F., Howison, C. M., and Pagel, M. D. (2015). Evaluations of tumor acidosis within in vivo tumor models using parametric maps generated with acidoCEST MRI. Mol. Imaging Biol. 17, 488–496. doi: 10.1007/s11307-014-0816-2
Chiche, J., Brahimi-horn, M. C., and Pouysségur, J. (2010). Tumour hypoxia induces a metabolic shift causing acidosis: a common feature in cancer. J. Cell. Mol. Med. 14, 771–794. doi: 10.1111/j.1582-4934.2009.00994.x
Chu, J., Oh, Y., Sens, A., Ataie, N., Dana, H., Macklin, J. J., et al. (2016). A bright cyan-excitable orange fluorescent protein facilitates dual-emission microscopy and enhances bioluminescence imaging in vivo. Nat. Biotechnol. 34, 760–767. doi: 10.1038/nbt.3550
Corbet, C., and Feron, O. (2017). Tumour acidosis: from the passenger to the driver’s seat. Nat. Rev. Cancer 17, 577–593. doi: 10.1038/nrc.2017.77
Dale, N. C., Johnstone, E. K. M., White, C. W., and Pfleger, K. D. G. (2019). NanoBRET: the bright future of proximity-based assays. Front. Bioeng. Biotechnol. 7:56. doi: 10.3389/fbioe.2019.00056
Düwel, S., Hundshammer, C., Gersch, M., Feuerecker, B., Steiger, K., Buck, A., et al. (2017). Imaging of pH in vivo using hyperpolarized 13C-labelled zymonic acid. Nat. Commun. 8:15126. doi: 10.1038/ncomms15126
Estrella, V., Chen, T., Lloyd, M., Wojtkowiak, J., Cornnell, H. H., Ibrahim-Hashim, A., et al. (2013). Acidity generated by the tumor microenvironment drives local invasion. Cancer Res. 73, 1524–1535. doi: 10.1158/0008-5472.CAN-12-2796
Gabriel, G. V. M., and Viviani, V. R. (2014). Novel application of pH-sensitive firefly luciferases as dual reporter genes for simultaneous ratiometric analysis of intracellular pH and gene expression/location. Photochem. Photobiol. Sci. 13, 1661–1670. doi: 10.1039/c4pp00278d
Hall, M. P., Unch, J., Binkowski, B. F., Valley, M. P., Butler, B. L., Wood, M. G., et al. (2012). Engineered luciferase reporter from a deep sea shrimp utilizing a novel imidazopyrazinone substrate. ACS Chem. Biol. 7, 1848–1857. doi: 10.1021/cb3002478
Hanahan, D., and Weinberg, R. A. (2011). Hallmarks of cancer: the next generation. Cell 144, 646–674. doi: 10.1016/j.cell.2011.02.013
Hashim, A. I., Zhang, X., Wojtkowiak, J. W., Martinez, G. V., and Gillies, R. J. (2011). Imaging pH and metastasis. NMR Biomed. 24, 582–591. doi: 10.1002/nbm.1644
Jacobus, A. P., and Gross, J. (2015). Optimal cloning of PCR fragments by homologous recombination in Escherichia coli. PLoS One 10, 1–17. doi: 10.1371/journal.pone.0119221
Jin, U. H., Lee, S. O., Pfent, C., and Safe, S. (2014). The aryl hydrocarbon receptor ligand omeprazole inhibits breast cancer cell invasion and metastasis. BMC Cancer 14:498. doi: 10.1186/1471-2407-14-498
Jo, J., Lee, C. H., Kopelman, R., and Wang, X. (2017). In vivo quantitative imaging of tumor pH by nanosonophore assisted multispectral photoacoustic imaging. Nat. Commun. 8:471. doi: 10.1038/s41467-017-00598-1
Kobayashi, H., Picard, L. P., Schönegge, A. M., and Bouvier, M. (2019). Bioluminescence resonance energy transfer–based imaging of protein–protein interactions in living cells. Nat. Protoc. 14, 1084–1107. doi: 10.1038/s41596-019-0129-7
Koh, E. Y. C., Ho, S. C. L., Mariati, Song, Z., Bi, X., Bardor, M., et al. (2013). An internal ribosome entry site (IRES) mutant library for tuning expression level of multiple genes in mammalian cells. PLoS One 8:e0082100. doi: 10.1371/journal.pone.0082100
Leblond, F., Davis, S., Valdes, P., and Pogue, B. (2010). Preclinical whole-body fluorescence imaging: review of instruments, methods and applications. J. Photochem. Photobiol. B 98, 77–94. doi: 10.1016/j.jphotobiol.2009.11.007
Liu, Z., Chen, O., Wall, J. B. J., Zheng, M., Zhou, Y., Wang, L., et al. (2017). Systematic comparison of 2A peptides for cloning multi-genes in a polycistronic vector. Sci. Rep. 7, 1–9. doi: 10.1038/s41598-017-02460-2
Longo, D. L., Bartoli, A., Consolino, L., Bardini, P., Arena, F., Schwaiger, M., et al. (2016). In vivo imaging of tumor metabolism and acidosis by combining PET and MRI-CEST pH imaging. Cancer Res. 76, 6463–6470. doi: 10.1158/0008-5472.CAN-16-0825
Menkin, V. (1933). The cytological picture of an inflammatory exudate in relation to its hydrogen ion concentration. Am. J. Pathol. 10, 193–210.
Miesenböck, G., De Angelis, D., and Rothman, J. (1998). Visualizing secretion and synaptic transmission with pH-sensitive green fluorescent proteins. Nature 394, 192–195. doi: 10.1038/nature02117.1
Mizuguchi, H., Ishii-Watabe, A., Xu, Z., Uchida, E., and Hayakawa, T. (2002). IRES-dependent second gene expression is significantly lower than cap-dependent first gene expression in a bicistronic vector. Mol. Ther. 1, 376–382. doi: 10.1006/mthe.2000.0050
Mordon, S., Devoisselle, J. M., and Maunoury, V. (1994). In vivo pH measurement and imaging of tumor tissue using a pH-sensitive fluorescent probe (5,6-carboxyfluorescein): instrumental and experimental studies. Photochem. Photobiol. 60, 1–6. doi: 10.1111/j.1751-1097.1994.tb05104.x
Pfleger, K. D. G., and Eidne, K. A. (2006). Illuminating insights into protein-protein interactions using bioluminescence resonance energy transfer (BRET). Nat. Methods 3, 165–174. doi: 10.1038/nmeth841
Pillai, S. R., Damaghi, M., Marunaka, Y., Spugnini, E. P., Fais, S., and Gillies, R. J. (2019). Causes, consequences, and therapy of tumors acidosis. Cancer Metastasis Rev. 38, 205–222. doi: 10.1007/s10555-019-09792-7
Puaux, A.-L., Ong, L. C., Jin, Y., Teh, I., Hong, M., Chow, P. K. H., et al. (2011). A comparison of imaging techniques to monitor tumor growth and cancer progression in living animals. Int. J. Mol. Imaging 2011, 1–12. doi: 10.1155/2011/321538
Reshetnyak, Y. K., Segala, M., Andreev, O. A., and Engelman, D. M. (2007). A monomeric membrane peptide that lives in three worlds: in solution, attached to, and inserted across lipid bilayers. Biophys. J. 93, 2363–2372. doi: 10.1529/biophysj.107.109967
Schaub, F. X., Reza, S., Flaveny, C. A., Li, W., Musicant, A. M., Hoxha, S., et al. (2015). Fluorophore-NanoLuc BRET reporters enable sensitive in vivo optical imaging and flow cytometry for monitoring tumorigenesis. Am. Assoc. Cancer Res. 75, 5023–5034. doi: 10.1158/0008-5472.CAN-14-3538
Sun, P. Z., and Gregory Sorensen, A. (2008). Imaging pH using the chemical exchange saturation transfer (CEST) MRI: correction of concomitant RF irradiation effects to quantify cest MRI for chemical exchange rate and pH. Magn. Reson. Med. 60, 390–397. doi: 10.1002/mrm.21653
Suzuki, K., Kimura, T., Shinoda, H., Bai, G., Daniels, M. J., Arai, Y., et al. (2016). Five colour variants of bright luminescent protein for real-time multicolour bioimaging. Nat. Commun. 7:13718. doi: 10.1038/ncomms13718
Thestrup, T., Litzlbauer, J., Bartholomäus, I., Mues, M., Russo, L., Dana, H., et al. (2014). Optimized ratiometric calcium sensors for functional in vivo imaging of neurons and T lymphocytes. Nat. Methods 11, 175–182. doi: 10.1038/nmeth.2773
Webb, B., Chimenti, M., Jacobson, M., and Barber, D. (2011). Dysregulated pH: a perfect storm for cancer progression. Nat. Rev. Cancer 11, 671–677. doi: 10.1038/nrc3110
Keywords: pH-sensitive ratiometric luminescent reporter, Superecliptic pHluorin (SEP), Nanoluc, tumor microenvironment, acidosis, bioluminescence resonance energy transfer
Citation: Ong TT, Ang Z, Verma R, Koean R, Tam JKC and Ding JL (2020) pHLuc, a Ratiometric Luminescent Reporter for in vivo Monitoring of Tumor Acidosis. Front. Bioeng. Biotechnol. 8:412. doi: 10.3389/fbioe.2020.00412
Received: 01 November 2019; Accepted: 14 April 2020;
Published: 08 May 2020.
Edited by:
Jose Ruben Morones-Ramirez, Autonomous University of Nuevo León, MexicoReviewed by:
Thomas Dandekar, Julius Maximilian University of Würzburg, GermanyOlivier Feron, Université Catholique de Louvain, Belgium
Copyright © 2020 Ong, Ang, Verma, Koean, Tam and Ding. This is an open-access article distributed under the terms of the Creative Commons Attribution License (CC BY). The use, distribution or reproduction in other forums is permitted, provided the original author(s) and the copyright owner(s) are credited and that the original publication in this journal is cited, in accordance with accepted academic practice. No use, distribution or reproduction is permitted which does not comply with these terms.
*Correspondence: Jeak Ling Ding, ZGJzZGpsQG51cy5lZHUuc2c=
†These authors share first authorship
‡These authors share senior authorship
§Present address: Zhiwei Ang, Department of Pathology and Laboratory Medicine, Children’s Hospital of Philadelphia, Philadelphia, PA, United States