- 1College of Materials Science and Engineering, Nanjing Tech University, Nanjing, China
- 2Sino-German Joint Research Lab for Space Biomaterials and Translational Technology, School of Life Sciences, Northwestern Polytechnical University, Xi’an, China
- 3Institute of Microbiology, Technische Universität Dresden, Dresden, Germany
- 4Department of Chemistry, Technische Universität Dresden, Dresden, Germany
- 5Department of Physics, Chemistry and Pharmacy University of Southern Denmark, Odense, Denmark
- 6Danish Institute for Advanced Study, University of Southern Denmark, Odense, Denmark
In situ immobilization of enzyme into metal–organic frameworks (MOFs) is performed through a one-step and facile method. Candida antarctica lipase B (CalB) is directly embedded in zeolitic imidazolate framework (ZIF)-8 by simply mixing an aqueous solution of 2-methylimidazole and zinc nitrate hexahydrate [Zn(NO3)2⋅6H2O] containing CalB at room temperature. Due to the intrinsic micropores of ZIF-8, the obtained CalB@ZIF composite is successfully applied in size-selective transesterification reaction in organic solvent. CalB@ZIF not only shows much higher catalytic activity but also exhibits higher thermal stability than free CalB. Besides, the robust ZIF-8 shell also offers the hybrid composites excellent reusability.
Introduction
Enzymatic catalysis is one of the most important catalytic processes used in modern industries to manufacture chemicals, pharmaceuticals, food, and materials for human society. Immobilized enzymes are preferred in the industry since free enzymes are usually vulnerable in non-aqueous media or at elevated temperatures (Sheldon, 2007; Zhang et al., 2015). To date, various kinds of solid materials have been employed as carriers for the immobilization of enzymes, including natural polymers, synthetic polymers, inorganic materials, etc. (Hartmann and Jung, 2010; Datta et al., 2013; Zhou and Hartmann, 2013). The immobilization can be approached by different techniques, e.g., adsorption (Wang et al., 2019), covalent binding (Engstrom et al., 2013), affinity immobilization (Keller et al., 2017), and entrapment (Majewski et al., 2017). Generally, the stability of immobilized enzymes is usually enhanced compared with free enzymes, which thus enables the long-term and repeated usage of the enzymes. The ideal enzyme immobilization method should retain maximal enzyme activity while minimizing mass transfer limitation of substrates/products. However, no existing method has entirely fulfilled these requirements (Gkaniatsou et al., 2017). Therefore, developing novel immobilization matrices and methods still remain of key interest. Moreover, the immobilization of enzymes for size-selective catalysis has been rarely reported, though size-selective catalysis is quite important when a reaction is conducted in a complex system containing substrates with the same or similar reaction groups.
Metal–organic frameworks (MOFs) are an attractive class of porous crystalline solids built from metal ions and organic linkers. Due to the easily tunable chemical and structural properties, MOFs have shown great potential in applications in various areas, including gas adsorption (Xiao et al., 2016), catalysis (Huang et al., 2017; Zhu et al., 2017), and drug delivery (Chen et al., 2017; Wu and Yang, 2017). Recently, the application of MOFs as matrices for immobilizing proteins/enzymes has also emerged (Liang et al., 2020). Enzymes could be immobilized through adsorbing (Liu et al., 2013, 2014, 2015; Zhao et al., 2015; Cao Y. et al., 2016; Lu et al., 2016; Zhang et al., 2017; Samui and Sahu, 2018) or crosslinking (Jung et al., 2011; Shih et al., 2012; Doherty et al., 2013; Patra et al., 2015; Cao S. et al., 2016; Patra et al., 2016; Wang et al., 2016; Wen et al., 2016) onto the surface of MOFs. It was also reported that some enzymes could enter the interior of MOFs and be stabilized in the pores (Lykourinou et al., 2011; Chen et al., 2012a; Deng et al., 2012; Kim et al., 2015), whereby enzymes undergo significant conformational change due to the strong interactions between enzymes and organic linkers in MOFs (Chen et al., 2012b, 2014). Prominent enhancement of stability and/or activity of enzymes was observed when they were immobilized on MOFs, indicating that MOFs were among the promising matrices for immobilizing enzymes (Li et al., 2016a, b; Nadar and Rathod, 2018a).
It should be noted that the abovementioned immobilizing enzymes on/in MOFs are usually realized through at least two steps, namely, preparation of MOFs and immobilization of enzymes. One-step fabrication of enzyme/MOF hybrids would be of great interest for its ease of preparation, which requires that the formation of MOFs should proceed in a fast and mild manner and would preserve the activity of enzymes (Gascon Perez et al., 2017). Zeolitic imidazolate frameworks (ZIFs), especially ZIF-8, which grow under mild biocompatible conditions and feature with exceptional chemical and thermal stability, have been primarily studied for the immobilization of enzymes in a one-pot reaction. Liu et al. did the pioneering work in this field, embedding protein (cytochrome c, Cyt c) in ZIF-8 in methanol. During the synthesis, polyvinylpyrrolidone (PVP) was utilized for enhancing the dispersion and stabilization of Cyt c (Lyu et al., 2014). Besides, the protective effect of ZIF-8 for enzymes/proteins, including urease and horseradish peroxidase (HRP), against polar solvents, high temperature, and trypsin was demonstrated by Falcaro’s group (Liang et al., 2015). Shieh et al. (2015) embedded catalase into ZIF-90, showing that the catalase exhibited hydrogen peroxide degradation even in the presence of protease because the catalase was sheltered and protected by ZIF-90 shell. Ge group prepared a hybrid biocatalyst by embedding glucose oxidase (GOx) into ZIF-8, which was further modified with polydopamine (PDA). This composite can be used repeatedly without obvious activity loss (Wu et al., 2015b). Later, GOx/ZIF-8 composites were reported as chemical sensors (Hou et al., 2015; Wang et al., 2017). Ge group further investigated the protective effect of ZIF-8 for the embedded enzymes in various denaturing organic solvents (Wu et al., 2017). The same group also developed multienzyme-containing ZIF-8 composites by immobilizing different enzymes in ZIF-8 in one-pot in aqueous solution at 25°C. High catalytic efficiency, selectivity, and stability of this hybrid material were displayed due to the existence of ZIF-8 shell (Wu et al., 2015a; Chen et al., 2018). Laccase was also entrapped into ZIF-8, showing increased thermostability, reusability, and storage stability (Patil and Yadav, 2018). Based on these reports, it is demonstrated that embedding enzymes in ZIF-8 can proceed in biocompatible conditions in a facile one-step method, holding considerable potential in developing enzyme/MOF hybrid catalysts.
Candida antarctica lipase B is a well-known and easily available lipase, which can catalyze esterification, hydrolysis, and transesterification reaction efficiently. Recently, hybrid materials prepared through embedding CalB and other lipases in ZIF-8 were reported by several research groups (Pitzalis et al., 2018; Cai et al., 2020). Rathod group encapsulated lipase (from Aspergillus niger source) in ZIF-8 by mixing an aqueous solution of Zn(NO3)2 containing lipase with an aqueous solution of 2-methylimidazole (Nadar and Rathod, 2018b). It was found that the ultrasound treatment of lipase before encapsulation increased its activity, probably due to the favorable conformational changes of lipase during sonication. Further, the same group found that the activity of the lipase could be improved in the presence of proline, which was able to maintain active conformation of enzyme and protect active sites under high-temperature conditions (Nadar and Rathod, 2019). Surfactants were also capable to enhance the activity of encapsulated lipase in ZIF-8, as the hydrogen bonding and hydrophobic–hydrophilic interactions between surfactants and lipase were believed to improve the 3D conformation of lipase (Vaidya et al., 2020). It is noticed that these works are mainly focusing on the improvement of catalytic activity of encapsulated CalB or other lipases in ZIF-8 by various methods. Due to the relatively small aperture diameter (3.4 Å) of ZIF-8, assembled ZIF-8 particles have been applied as shells of hybrid catalytic materials for size-selective catalysis (Huo et al., 2015). However, the direct immobilization of enzymes in ZIFs for size-selective catalysis in organic solvents has not been reported so far, which is very important for the controllable conversion of substrates with the same reactive groups.
In this work, we present the entrapment of enzyme CalB in ZIF-8 through biomimetic mineralization under room temperature, obtaining the hybrid material CalB@ZIF. Its application in size-selective biocatalysis for organic synthesis was investigated for the first time. In this hybrid CalB@ZIF, the ZIF-8 shell can not only enhance the activity and stability of CalB but also regulate the accessibility of substrates to the interior CalB.
Materials and Methods
Materials and Chemicals
Candida antarctica lipase B was purchased from c-LEcta GmbH (Leipzig, Germany), and the weight percentage of pure CalB in the received product is about 10% based on the Bradford test. Zn(NO3)2⋅6H2O, ethylenediaminetetraacetic disodium salt (EDTA-2Na), and Bradford test reagent Roti-Nanoquant were purchased from Carl Roth GmbH & Co. KG (Karlsruhe, Germany). Vinyl acetate, vinyl laurate, 2-methylimidazole, 1-butanol, and 3-(4-hydroxyphenyl)propan-1-ol were purchased from ABCR GmbH & Co. KG. Fluorescein isothiocyanate (FITC) was purchased from Sigma-Aldrich. Unless otherwise noted, all the chemicals were used as received without purification.
Characterizations
Bradford test was performed on TECAN infinite M200. Thermogravimetric analysis (TGA) was carried out on NETZSCH STA 449F3 (NETZSCH, Germany) by heating samples from 25 to 800°C in a dynamic Ar atmosphere with a heating rate of 10°C min–1. Fourier transform infrared spectroscopy (FTIR) spectra were recorded on an FTIR spectrometer Tensor II (Bruker) with an attenuated total reflectance (ATR) unit. Powder X-ray diffraction (PXRD) was performed on a PANalytical X’Pert Pro powder diffractometer with Bragg–Brentano geometry equipped with a Ge(111)-monochromator, a rotating sample stage, and a PIXcel detector, using Cu Kα1 radiation (λ = 154.06 pm). The data were collected in reflection mode using a divergence slit that kept the illuminated sample area constant. Optical and fluorescence microscopy images were recorded on Olympus Provis AX70. N2 sorption was measured at 77 K on BeiShiDe (3H-2000PS2). The pore diameter of ZIF-8 was calculated through the Horvath–Kawazoe (H-K) method due to its microporous feature, while the pore diameter of CalB@ZIF was calculated through the Barrett–Joyner–Halenda (BJH) method because of its mesoporous feature. The results were given by the measurement instrument directly. Gas chromatography (GC) analysis was performed on a Shimadzu GC2010 PLUS gas chromatograph equipped with a BPX5 column (25 m × 0.22 mm) using a flame ionization detector (FID).
Preparations
Preparation of CalB@ZIF
CalB@ZIF was synthesized following a reported method with modification (Liang et al., 2015). CalB (200 mg) was dissolved in 400 ml Zn(NO3)2⋅6H2O aqueous solution (80 mM). After stirring for 10 min under room temperature, 400 ml 2-methylimidazole aqueous solution (320 mM) was added. The mixture was stirred for 30 min, and the precipitated white solid was isolated through centrifugation at 4°C. After washing thoroughly with deionized water, the white solid was lyophilized and stored at −20°C before use. The amount of encapsulated CalB was determined by the Bradford test. CalB@ZIF (24.3 mg) was first digested in 1 ml saturated EDTA-2Na aqueous solution. After a transparent solution was obtained, the solution was dialyzed in deionized water for 24 h and lyophilized. The residual solid was then dissolved in 4 ml deionized water and subjected to the Bradford test.
Preparation of ZIF-8
Zeolitic imidazolate framework-8 was prepared by mixing 400 ml Zn(NO3)2⋅6H2O aqueous solution (80 mM) and 400 ml 2-methylimidazole aqueous solution (320 mM). After stirring for 30 min under room temperature, the white solid was isolated through centrifugation and washed thoroughly with deionized water before being lyophilized.
Catalysis
A Typical Procedure for Transesterification Reaction
To a glass vial containing catalyst (CalB@ZIF or ZIF-8, 20 mg) or free CalB (366 μg, the amount was determined through Bradford test), alcohol (300 mM) and vinyl ester (200 mM) in acetone (250 μl) were added. The sealed vial was shaken at 800 rpm under 25°C. At interval time, the reaction mixture (5 μl) was withdrawn and diluted to 100 μl with acetone. The solid catalyst was removed through centrifugation, and the obtained supernatant was analyzed by GC. The conversion was determined by the diminishment of the peak of vinyl ester.
Thermal Stability Measurement
CalB@ZIF (20 mg) or free CalB (366 μg, the amount was determined through Bradford test) was dispersed in 250 μl acetone in glass vials. The sealed vials were shaken at 800 rpm under 50°C. At intervals (0, 1, 2, 4, and 8 h), the glass vials were removed from heating. After cooling to room temperature, n-butanol (5.56 mg, 0.075 mmol) and vinyl acetate (4.30 mg, 0.050 mmol) were added. After being shaken at 800 rpm under 25°C for 25 min, 5 μl reaction mixture was withdrawn and diluted to 100 μl. The supernatant was subjected to GC analysis.
Reusability of CalB@ZIF
To a glass vial containing 20 mg CalB@ZIF, n-butanol (300 mM) and vinyl acetate (200 mM) in acetone (250 μl) were added. The sealed vial was shaken at 800 rpm under 25°C for 2 h. After isolating the solid catalyst from the reaction mixture, the supernatant was analyzed with GC. The isolated CalB@ZIF was washed with acetone (3 × 1 ml) and dried in a vacuum under room temperature before being used in the next run.
Results and Discussion
CalB@ZIF was prepared by mixing an aqueous solution of Zn(NO3)2⋅6H2O containing CalB and an aqueous solution of 2-methylimidazole, following a reported method with modification (Liang et al., 2015). The schematic illustration of the synthesis of CalB@ZIF is depicted in Scheme 1. ZIF-8 was prepared under identical reaction conditions but in the absence of CalB. It should be mentioned that the formation of ZIF-8 is much slower compared with the formation of CalB@ZIF, indicating that the nucleation of ZIF-8 precursors can be triggered by CalB (Maddigan et al., 2018). The content of trapped CalB in CalB@ZIF was determined based on a standard Bradford assay after digesting CalB@ZIF in EDTA-2Na solution, which turned out to be 18.3 μg mg–1.
Powder X-ray diffraction measurement was performed to characterize the crystallinity of CalB@ZIF (Figure 1A). Unexpectedly, no characteristic peaks from ZIF-8 are observed in the PXRD pattern of CalB@ZIF, indicating the formation of amorphous ZIF-8. Amorphous MOFs (aMOFs) are an emerging family of MOF materials, which maintain the basic building blocks but without long-range crystallinity (Bennett and Cheetham, 2014; Bennett and Horike, 2018). Preparation of aMOFs usually requires harsh conditions, including high-pressure treatment (Chapman et al., 2009, 2011), high-temperature heating (Bennett et al., 2011), electron-beam treatment (Conrad et al., 2018), and high-energy ball-milling (Cao et al., 2012). While in our case, the amorphization of ZIF-8 is realized through a biomolecule-induced process in a mild condition. The amorphous feature of CalB@ZIF is probably caused by the coordination between amine groups of CalB and Zn2+, whereby CalB functions as a competitive ligand against 2-methylimidazole and disturbs the periodic structure of ZIF-8 (Ge et al., 2012; Liu et al., 2020).
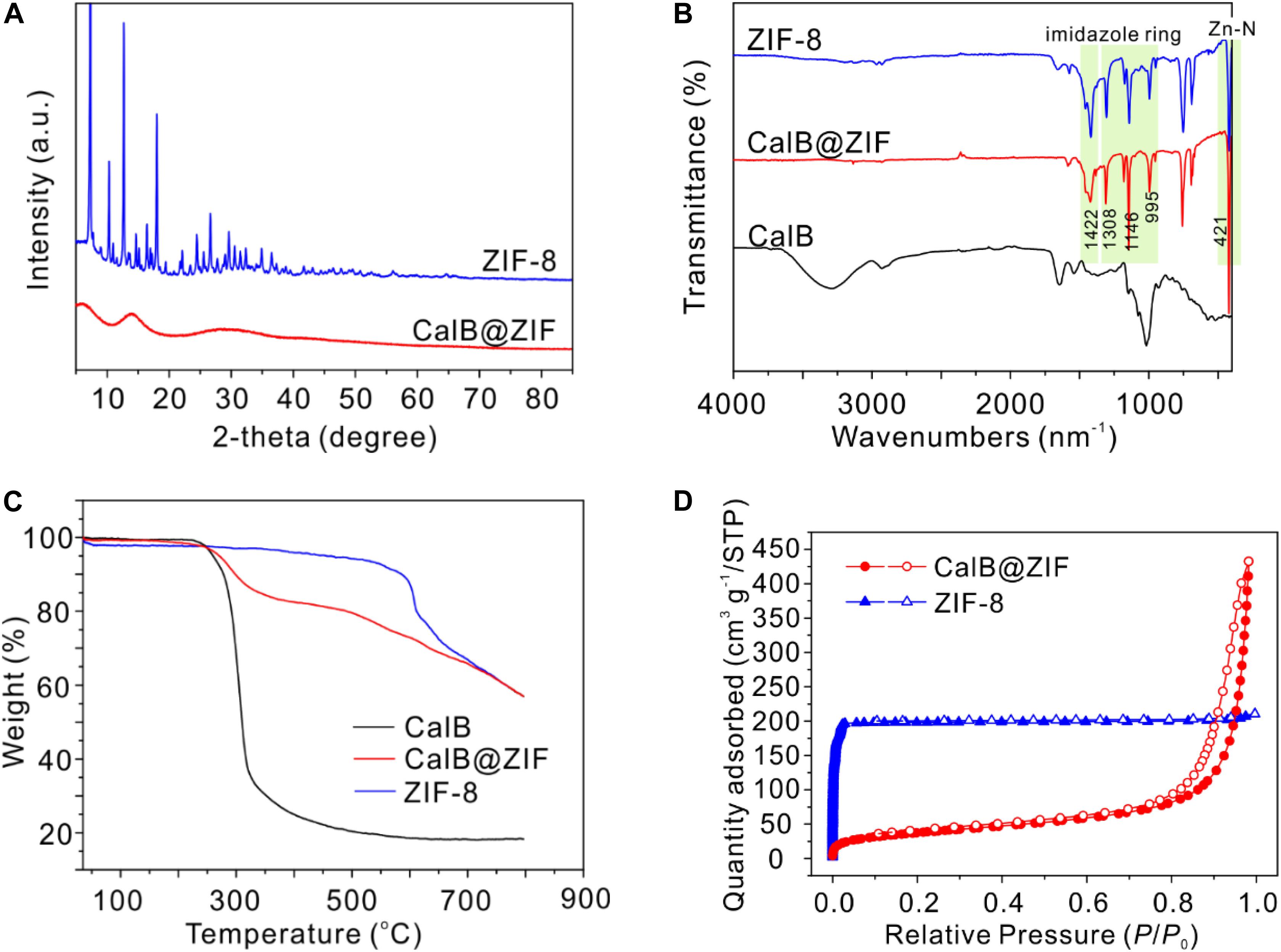
Figure 1. (A) Powder X-ray diffraction (PXRD) patterns of CalB@ZIF and ZIF-8. (B) Fourier transform infrared spectroscopy (FTIR) spectra of ZIF-8, CalB@ZIF, and CalB. (C) Thermogravimetric analysis (TGA) curves of ZIF-8, CalB@ZIF, and CalB. (D) N2 sorption isotherms of ZIF-8 and CalB@ZIF.
The formation of ZIF-8 structure in CalB@ZIF is confirmed by FTIR measurement (Figure 1B). In the FTIR spectrum of CalB@ZIF, the adsorption bands, which can be assigned to ZIF-8 structure, are clearly observed. The sharp signal at 421 cm–1 is attributed to Zn-N stretching, while the bands at 1,422 and 900–1,300 cm–1 are derived from the stretching and plane bending of the imidazole ring, respectively (Hou et al., 2015). The signal from CalB in CalB@ZIF is almost invisible probably due to the low amount of trapped CalB. TGA results confirm the presence of CalB in CalB@ZIF (Figure 1C). The first-stage weight loss of CalB@ZIF starts from around 250°C due to the decomposition of CalB within the framework, while pure ZIF-8 starts to decompose at nearly 450°C. It is noted that the thermal stability of ZIF-8 decreases slightly in CalB@ZIF, which is possible because of its amorphous structure in the hybrid material.
The porosity of CalB@ZIF and pure ZIF-8 was investigated with the N2 adsorption/desorption experiment (Figure 1D). The isotherm of ZIF-8 displays a steep increase at low relative pressure (P/P0 < 0.1), indicating the existence of permanent micropores. And the average pore diameter of ZIF-8 is 0.799 nm calculated through the H-K method. On the contrary, a sharp rise at high relative pressure in the N2 adsorption isotherm of CalB@ZIF is observed, suggesting the presence of interparticle mesopores. The average pore diameter of CalB@ZIF is 18.380 nm calculated through the BJH method. Meanwhile, the appearance of hysteresis demonstrates the deformation and swelling of CalB@ZIF (Weber et al., 2010). The discrepancy between the N2 adsorption properties of CalB@ZIF and ZIF-8 is possible because of the presence of CalB and CalB-induced amorphization of ZIF-8, which may affect its pore structures (Chapman et al., 2009).
To further confirm the presence of CalB in CalB@ZIF, CalB labeled with FITC (CalB-FITC) was embedded in ZIF-8 following the same procedure for preparing CalB@ZIF, and the obtained sample was denoted as CalB-FITC@ZIF. The black particles observed on the optical microscopic image are the sample CalB-FITC@ZIF (Figure 2A). These particles emit green fluorescence when observed under a fluorescence microscope (Figure 2B). In a control experiment, no fluorescence is emitted from ZIF-8 particles (Supplementary Figure 1). These results manifest the successful entrapment of enzymes in CalB@ZIF.
Candida antarctica lipase B is a widely used broad-substrate lipase for esterification and transesterification reactions. The catalytic performance of CalB@ZIF was assessed by carrying out transesterification reactions between a pair of small substrates (butanol and vinyl acetate) and a pair of larger ones [3-(4-hydroxyphenyl)propan-1-ol and vinyl laurate] in acetone (Figure 3A; Huo et al., 2015). The reactions were carried out under room temperature, and the conversion was monitored by GC. Initially, the reactions were performed with free CalB as the catalyst. After 120 min, moderate conversion of vinyl acetate and vinyl laurate was found, which was 51.4 and 64.7%, respectively (Supplementary Figure 2). When CalB@ZIF was used as a catalyst, it was found that vinyl acetate was almost totally converted within 120 min using the small substrates (Figure 3B). However, the conversion of vinyl laurate was only 8.2% using the large substrates. In a control experiment, it was also found that 8.5% of vinyl acetate was converted in the presence of ZIF-8 instead of CalB@ZIF. These results reveal that the smaller substrates and their products can readily diffuse through the apertures of ZIF-8, while larger substrates cannot pass through the pores. Leaching test was carried out by isolating CalB@ZIF from the reaction mixture after reacting 30 min, and almost no further conversion of vinyl acetate was observed, indicating that no CalB leached out from CalB@ZIF during the catalysis. It is noteworthy that CalB@ZIF shows a higher catalytic activity than free CalB in catalyzing the transesterification reaction. The enhanced activity of CalB in CalB@ZIF is presumably derived from the conformational change of CalB induced by coordination between CalB and Zn2+, which is called allosteric effect (Ge et al., 2012; Wang et al., 2013). Meanwhile, the microenvironments created by ZIF-8 shell may also contribute to the enhanced activity of encapsulated CalB (Lyu et al., 2014; Sun et al., 2018). Additionally, CalB@ZIF has a much better dispersibility than free CalB in acetone, which is very important to the higher catalytic activity as well. As shown in Figure 3C, when CalB-FITC@ZIF is added into acetone and observed under a UV light (245 nm), the whole mixture emits green fluorescence due to the good dispersibility of CalB-FITC@ZIF. But when CalB-FITC is added into acetone, green fluorescence is only observed at the bottom part. The aggregation of free CalB restricts its catalytic ability in organic solvents (Stepankova et al., 2013).
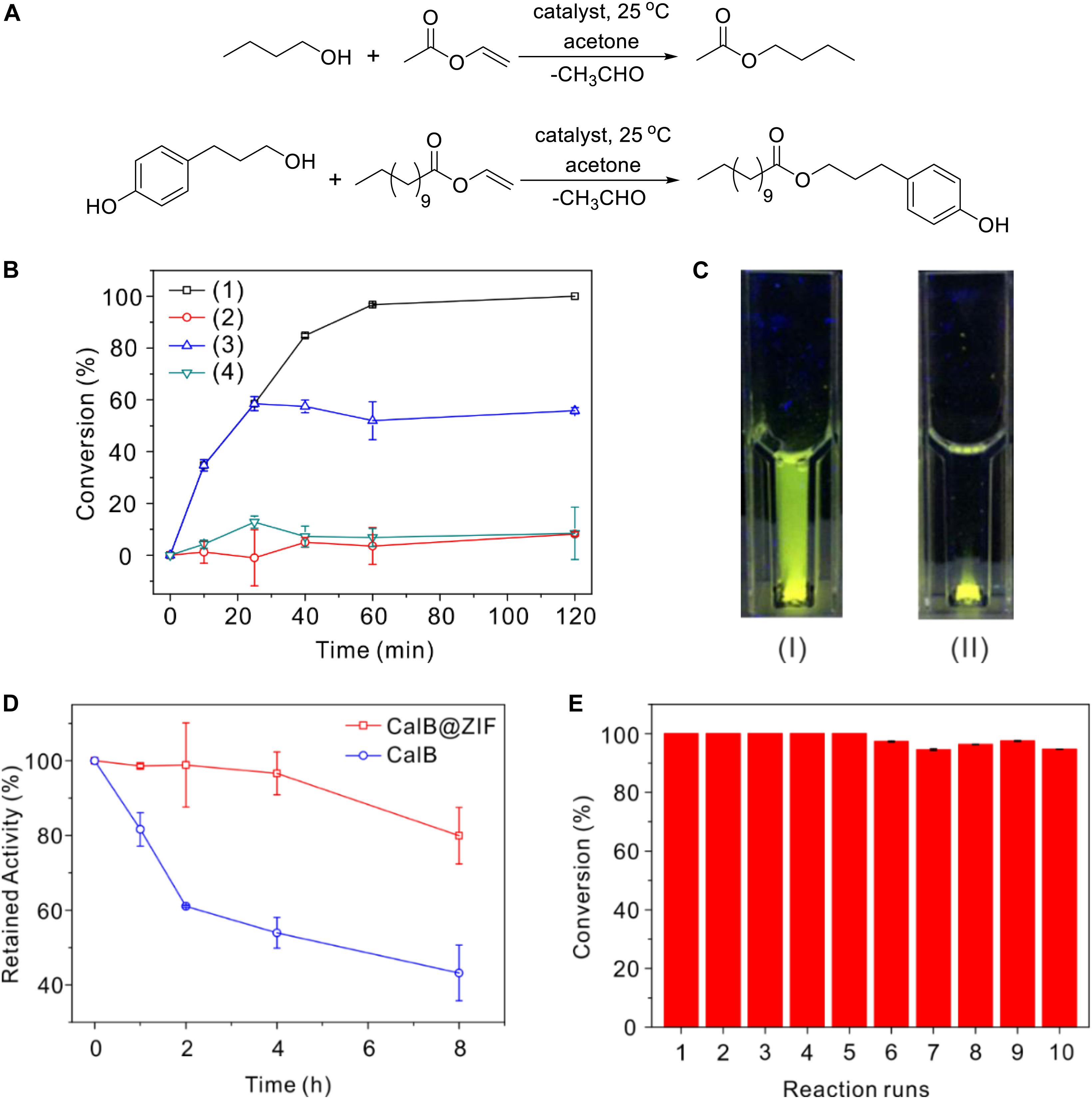
Figure 3. (A) Reaction schemes of the model reactions. (B) Time-dependent conversion of vinyl acetate or vinyl laurate: (1) conversion of vinyl acetate when CalB@ZIF is used, (2) conversion of vinyl laurate when CalB@ZIF-8 is used, (3) conversion of vinyl acetate in the leaching test (CalB@ZIF was isolated at 30 min), and (4) conversion of vinyl acetate in the presence of ZIF-8 instead of CalB@ZIF. (C) Photo picture of CalB-FITC@ZIF (I) and CalB-FITC (II) in acetone observed under a UV light (245 nm). (D) Thermal stability test of CalB@ZIF and free CalB. (E) Reusability test of CalB@ZIF.
The encapsulation of CalB with ZIF-8 not only offers the catalyst size selectivity and enhanced activity but also elevates the thermal stability of CalB. When CalB@ZIF was used to catalyze the transesterification between vinyl acetate and n-butanol after being incubated in acetone under 50°C for 8 h, it was found that the conversion of vinyl acetate was still higher than 80% of that catalyzed with untreated CalB@ZIF (Figure 3D). Yet the retained activity of free CalB decreased to only about 50%. These results illustrate the high thermal stability of CalB encapsulated in ZIF shells and their potential as a robust catalyst for industry-relevant application in organic solvents. Reusability is also a crucial parameter when catalysts are used in the industry. The reaction between vinyl acetate and n-butanol was still used as the model reaction. As shown in Figure 3E, only a slight decrease in conversion is observed even after CalB@ZIF is used for 10 times. The high reusability of CalB@ZIF is probably because the conformational change of CalB caused by acetone is inhibited by the ZIF shells.
Conclusion
In conclusion, a facile method for the direct encapsulation of the enzyme CalB in ZIF-8 is reported. Interestingly, amorphous ZIF-8 shell forms during the preparation, which is probably induced by the coordination between CalB and Zn2+. Size-selective biocatalysis properties of the hybrid CalB@ZIF is investigated for the first time through catalyzing transesterification reaction in organic solvent. The embedded CalB also shows enhanced activity and thermal stability. This work sheds light on the possibility to fabricate enzyme-MOF hybrid catalysts in a straightforward way for task-specific and long-term usage in industrial applications.
Data Availability Statement
All datasets generated for this study are included in the article/Supplementary Material.
Author Contributions
YW and CW designed this project. YW performed most of the experiments and wrote the main part of the manuscript. CW coordinated the research and corrected and revised the manuscript. NZ and DT carried out some characterization experiments. ZQ offered constructive suggestions for this research and was responsible for the final correction and English proofing. All authors contributed to the article and approved the submitted version.
Funding
We acknowledge financial support from the Deutsche Forschungsgemeinschaft (WU 814/1-1). This work was also financially supported by the Thousand Talents Program of China (1800-16GH030121), Key R&D Program of Shaanxi Province (2019KW-031 and 2019KW-038), China Postdoctoral Science Foundation (2017M623231), and Fundamental Research Funds for the Central Universities (3102018zy051).
Conflict of Interest
The authors declare that the research was conducted in the absence of any commercial or financial relationships that could be construed as a potential conflict of interest.
Acknowledgments
We thank Prof. Marion B. Ansorge-Schumacher (TU Dresden) for valuable discussions and support and Prof. Rainer Jordan (TU Dresden) for providing the lab facility.
Supplementary Material
The Supplementary Material for this article can be found online at: https://www.frontiersin.org/articles/10.3389/fbioe.2020.00714/full#supplementary-material
References
Bennett, T. D., and Cheetham, A. K. (2014). Amorphous metal-organic frameworks. Acc. Chem. Res. 47, 1555–1562. doi: 10.1021/ar5000314
Bennett, T. D., and Horike, S. (2018). Liquid, glass and amorphous solid states of coordination polymers and metal–organic frameworks. Nat. Rev. Mater. 3, 431–440. doi: 10.1038/s41578-018-0054-3
Bennett, T. D., Keen, D. A., Tan, J. C., Barney, E. R., Goodwin, A. L., and Cheetham, A. K. (2011). Thermal amorphization of zeolitic imidazolate frameworks. Angew. Chem. Int. Ed. 50, 3067–3071. doi: 10.1002/anie.201007303
Cai, X., Zhang, M., Wei, W., Zhang, Y., Wang, Z., and Zheng, J. (2020). The immobilization of Candida antarctica lipase B by ZIF-8 encapsulation and macroporous resin adsorption: preparation and characterizations. Biotechnol. Lett. 42, 269–276. doi: 10.1007/s10529-019-02771-6
Cao, S., Bennett, T. D., Keen, D. A., Goodwin, A. L., and Cheetham, A. K. (2012). Amorphization of the prototypical zeolitic imidazolate framework ZIF-8 by ball-milling. Chem. Commun. 48, 7805–7807. doi: 10.1039/c2cc33773h
Cao, S., Yue, D., Li, X., Smith, T. J., Li, N., Zong, M., et al. (2016). Novel nano-/micro-biocatalyst: soybean epoxide hydrolase immobilized on UiO-66-NH2 mof for efficient biosynthesis of enantiopure (r)-1, 2-octanediol in deep eutectic solvents. ACS Sustainable Chem. Eng. 4, 3586–3595. doi: 10.1021/acssuschemeng.6b00777
Cao, Y., Wu, Z., Wang, T., Xiao, Y., Huo, Q., and Liu, Y. (2016). Immobilization of Bacillus subtilis lipase on a Cu-BTC based hierarchically porous metal-organic framework material: a biocatalyst for esterification. Dalton Trans. 45, 6998–7003. doi: 10.1039/c6dt00677a
Chapman, K. W., Halder, G. J., and Chupas, P. J. (2009). Pressure-induced amorphization and porosity modification in a metal-organic framework. J. Am. Chem. Soc. 131, 17546–17547. doi: 10.1021/ja908415z
Chapman, K. W., Sava, D. F., Halder, G. J., Chupas, P. J., and Nenoff, T. M. (2011). Trapping guests within a nanoporous metal-organic framework through pressure-induced amorphization. J. Am. Chem. Soc. 133, 18583–18585. doi: 10.1021/ja2085096
Chen, W., Vázquez-González, M., Zoabi, A., Abu-Reziq, R., and Willner, I. (2018). Biocatalytic cascades driven by enzymes encapsulated in metal–organic framework nanoparticles. Nat. Catal. 1, 689–695. doi: 10.1038/s41929-018-0117-2
Chen, W., Yu, X., Liao, W., Sohn, Y. S., Cecconello, A., Kozell, A., et al. (2017). ATP-responsive aptamer-based metal-organic framework nanoparticles (NMOFs) for the controlled release of loads and drugs. Adv. Funct. Mater. 27:1702102. doi: 10.1002/adfm.201702102
Chen, Y., Han, S., Li, X., Zhang, Z., and Ma, S. (2014). Why does enzyme not leach from metal-organic frameworks (MOFs)? Unveiling the interactions between an enzyme molecule and a MOF. Inorg. Chem. 53, 10006–10008. doi: 10.1021/ic501062r
Chen, Y., Lykourinou, V., Hoang, T., Ming, L. J., and Ma, S. (2012a). Size-selective biocatalysis of myoglobin immobilized into a mesoporous metal-organic framework with hierarchical pore sizes. Inorg. Chem. 51, 9156–9158. doi: 10.1021/ic301280n
Chen, Y., Lykourinou, V., Vetromile, C., Hoang, T., Ming, L. J., Larsen, R. W., et al. (2012b). How can proteins enter the interior of a MOF? Investigation of cytochrome c translocation into a MOF consisting of mesoporous cages with microporous windows. J. Am. Chem. Soc. 134, 13188–13191. doi: 10.1021/ja305144x
Conrad, S., Kumar, P., Xue, F., Ren, L., Henning, S., Xiao, C., et al. (2018). Controlling dissolution and transformation of zeolitic imidazolate frameworks by using electron-beam-induced amorphization. Angew. Chem. Int. Ed. 57, 13592–13597. doi: 10.1002/anie.201809921
Datta, S., Christena, L. R., and Rajaram, Y. R. (2013). Enzyme immobilization: an overview on techniques and support materials. 3 Biotech 3, 1–9. doi: 10.1007/s13205-012-0071-7
Deng, H., Grunder, S., Cordova, K. E., Valente, C., Furukawa, H., Hmadeh, M., et al. (2012). Large-pore apertures in a series of metal-organic frameworks. Science 336, 1018–1023. doi: 10.1126/science.1220131
Doherty, C. M., Grenci, G., Ricco, R., Mardel, J. I., Reboul, J., Furukawa, S., et al. (2013). Combining UV lithography and an imprinting technique for patterning metal-organic frameworks. Adv. Mater. 25, 4701–4705. doi: 10.1002/adma.201301383
Engstrom, K., Johnston, E. V., Verho, O., Gustafson, K. P., Shakeri, M., Tai, C. W., et al. (2013). Co-immobilization of an enzyme and a metal into the compartments of mesoporous silica for cooperative tandem catalysis: an artificial metalloenzyme. Angew. Chem. Int. Ed. 52, 14006–14010. doi: 10.1002/anie.201306487
Gascon Perez, V., Carucci, C., Jimenez, M. B., Blanco, R. M., Sanchez Sanchez, M., and Magner, E. (2017). Rapid in-situ immobilization of enzymes in metal organic framework supports under mild conditions. ChemCatChem 9, 1182–1186. doi: 10.1002/cctc.201601342
Ge, J., Lei, J. D., and Zare, R. N. (2012). Protein-inorganic hybrid nanoflowers. Nat. Nanotechnol. 7, 428–432. doi: 10.1038/Nnano.2012.80
Gkaniatsou, E., Sicard, C., Ricoux, R., Mahy, J., Steunou, N., and Serre, C. (2017). Metal-organic frameworks: a novel host platform for enzymatic catalysis and detection. Mater. Horiz. 4, 55–63. doi: 10.1039/c6mh00312e
Hartmann, M., and Jung, D. (2010). Biocatalysis with enzymes immobilized on mesoporous hosts: the status quo and future trends. J. Mater. Chem. 20, 844–857. doi: 10.1039/b907869j
Hou, C., Wang, Y., Ding, Q., Jiang, L., Li, M., Zhu, W., et al. (2015). Facile synthesis of enzyme-embedded magnetic metal-organic frameworks as a reusable mimic multi-enzyme system: mimetic peroxidase properties and colorimetric sensor. Nanoscale 7, 18770–18779. doi: 10.1039/c5nr04994f
Huang, Y. B., Liang, J., Wang, X. S., and Cao, R. (2017). Multifunctional metal-organic framework catalysts: synergistic catalysis and tandem reactions. Chem. Soc. Rev. 46, 126–157. doi: 10.1039/c6cs00250a
Huo, J., Aguilera-Sigalat, J., El-Hankari, S., and Bradshaw, D. (2015). Magnetic MOF microreactors for recyclable size-selective biocatalysis. Chem. Sci. 6, 1938–1943. doi: 10.1039/c4sc03367a
Jung, S., Kim, Y., Kim, S.-J., Kwon, T.-H., Huh, S., and Park, S. (2011). Bio-functionalization of metal–organic frameworks by covalent protein conjugation. Chem. Commun. 47, 2904–2906. doi: 10.1039/c0cc03288c
Keller, D., Beloqui, A., Martinez-Martinez, M., Ferrer, M., and Delaittre, G. (2017). Nitrilotriacetic amine-functionalized polymeric core-shell nanoparticles as enzyme immobilization supports. Biomacromolecules 18, 2777–2788. doi: 10.1021/acs.biomac.7b00677
Kim, Y., Yang, T., Yun, G., Ghasemian, M. B., Koo, J., Lee, E., et al. (2015). Hydrolytic transformation of microporous metal-organic frameworks to hierarchical micro- and mesoporous MOFs. Angew. Chem. Int. Ed. 54, 13273–13278. doi: 10.1002/anie.201506391
Li, P., Moon, S. Y., Guelta, M. A., Harvey, S. P., Hupp, J. T., and Farha, O. K. (2016a). Encapsulation of a nerve agent detoxifying enzyme by a mesoporous zirconium metal-organic framework engenders thermal and long-term stability. J. Am. Chem. Soc. 138, 8052–8055. doi: 10.1021/jacs.6b03673
Li, P., Moon, S. Y., Guelta, M. A., Lin, L., Gomez-Gualdron, D. A., Snurr, R. Q., et al. (2016b). Nanosizing a metal-organic framework enzyme carrier for accelerating nerve agent hydrolysis. ACS Nano 10, 9174–9182. doi: 10.1021/acsnano.6b04996
Liang, K., Ricco, R., Doherty, C. M., Styles, M. J., Bell, S., Kirby, N., et al. (2015). Biomimetic mineralization of metal-organic frameworks as protective coatings for biomacromolecules. Nat. Commun. 6:7240. doi: 10.1038/ncomms8240
Liang, S., Wu, X., Xiong, J., Zong, M., and Lou, W. (2020). Metal-organic frameworks as novel matrices for efficient enzyme immobilization: an update review. Coord. Chem. Rev. 406:213149. doi: 10.1016/j.ccr.2019.213149
Liu, C., Wang, J., Wan, J., Cheng, Y., Huang, R., Zhang, C., et al. (2020). Amorphous metal-organic framework-dominated nanocomposites with both compositional and structural heterogeneity for oxygen evolution. Angew. Chem. Int. Ed. 59, 3630–3637. doi: 10.1002/anie.201914587
Liu, W., Lo, S., Singco, B., Yang, C., Huang, H., and Lin, C. (2013). Novel trypsin–FITC@MOF bioreactor efficiently catalyzes protein digestion. J. Mater. Chem. B 1, 928–932. doi: 10.1039/c3tb00257h
Liu, W., Wu, C., Chen, C., Singco, B., Lin, C., and Huang, H. (2014). Fast multipoint immobilized MOF bioreactor. Chem. Eur. J. 20, 8923–8928. doi: 10.1002/chem.201400270
Liu, W., Yang, N., Chen, Y., Lirio, S., Wu, C., Lin, C., et al. (2015). Lipase-supported metal-organic framework bioreactor catalyzes warfarin synthesis. Chem. Eur. J. 21, 115–119. doi: 10.1002/chem.201405252
Lu, X., Wang, X., Wu, L., Wu, L., Dhanjai, and Fu, L. (2016). Response characteristics of bisphenols on a metal–organic framework-based tyrosinase nanosensor. ACS Appl. Mater. Interfaces 8, 16533–16539. doi: 10.1021/acsami.6b05008
Lykourinou, V., Chen, Y., Wang, X., Meng, L., Hoang, T., Ming, L., et al. (2011). Immobilization of MP-11 into a mesoporous metal-organic framework, MP-11@mesoMOF: a new platform for enzymatic catalysis. J. Am. Chem. Soc. 133, 10382–10385. doi: 10.1021/ja2038003
Lyu, F., Zhang, Y., Zare, R. N., Ge, J., and Liu, Z. (2014). One-pot synthesis of protein-embedded metal-organic frameworks with enhanced biological activities. Nano Lett. 14, 5761–5765. doi: 10.1021/nl5026419
Maddigan, N. K., Tarzia, A., Huang, D. M., Sumby, C. J., Bell, S. G., Falcaro, P., et al. (2018). Protein surface functionalisation as a general strategy for facilitating biomimetic mineralisation of ZIF-8. Chem. Sci. 9, 4217–4223. doi: 10.1039/c8sc00825f
Majewski, M. B., Howarth, A. J., Li, P., Wasielewski, M. R., Hupp, J. T., and Farha, O. K. (2017). Enzyme encapsulation in metal–organic frameworks for applications in catalysis. CrystEngComm 19, 4082–4091. doi: 10.1039/c7ce00022g
Nadar, S. S., and Rathod, V. K. (2018a). Encapsulation of lipase within metal-organic framework (MOF) with enhanced activity intensified under ultrasound. Enzyme Microb. Technol. 108, 11–20. doi: 10.1016/j.enzmictec.2017.08.008
Nadar, S. S., and Rathod, V. K. (2018b). Magnetic-metal organic framework (magnetic-MOF): a novel platform for enzyme immobilization and nanozyme applications. Int. J. Biol. Macromol. 120, 2293–2302. doi: 10.1016/j.ijbiomac.2018.08.126
Nadar, S. S., and Rathod, V. K. (2019). Immobilization of proline activated lipase within metal organic framework (MOF). Int. J. Biol. Macromol. 152, 1108–1112. doi: 10.1016/j.ijbiomac.2019.10.199
Patil, P. D., and Yadav, G. D. (2018). Rapid in situ encapsulation of laccase into metal-organic framework support (ZIF-8) under biocompatible conditions. ChemistrySelect 3, 4669–4675. doi: 10.1002/slct.201702852
Patra, S., Hidalgo Crespo, T., Permyakova, A., Sicard, C., Serre, C., Chaussé, A., et al. (2015). Design of metal organic framework–enzyme based bioelectrodes as a novel and highly sensitive biosensing platform. J. Mater. Chem. B 3, 8983–8992. doi: 10.1039/c5tb01412c
Patra, S., Sene, S., Mousty, C., Serre, C., Chausse, A., Legrand, L., et al. (2016). Design of laccase-metal organic framework-based bioelectrodes for biocatalytic oxygen reduction reaction. ACS Appl. Mater. Interfaces 8, 20012–20022. doi: 10.1021/acsami.6b05289
Pitzalis, F., Carucci, C., Naseri, M., Fotouhi, L., Magner, E., and Salis, A. (2018). Lipase encapuslation onto ZIF-8: a comparison between biocatalysts obtained at low and high zinc/2-methylimidazole molar ratio in aqueous medium. ChemCatChem 10, 1578–1585. doi: 10.1002/cctc.201701984
Samui, A., and Sahu, S. K. (2018). One-pot synthesis of microporous nanoscale metal organic frameworks conjugated with laccase as a promising biocatalyst. New J. Chem. 42, 4192–4200. doi: 10.1039/c7nj03619a
Sheldon, R. A. (2007). Enzyme immobilization: the quest for optimum performance. Adv. Synth. Catal. 349, 1289–1307. doi: 10.1002/adsc.200700082
Shieh, F. K., Wang, S., Yen, C., Wu, C., Dutta, S., Chou, L., et al. (2015). Imparting functionality to biocatalysts via embedding enzymes into nanoporous materials by a de novo approach: size-selective sheltering of catalase in metal-organic framework microcrystals. J. Am. Chem. Soc. 137, 4276–4279. doi: 10.1021/ja513058h
Shih, Y.-H., Lo, S.-H., Yang, N., Singco, B., Cheng, Y., Wu, C., et al. (2012). Trypsin-immobilized metal-organic framework as a biocatalyst in proteomics analysis. ChemPlusChem 77, 982–986. doi: 10.1002/cplu.201200186
Stepankova, V., Bidmanova, S., Koudelakova, T., Prokop, Z., Chaloupkova, R., and Damborsky, J. (2013). Strategies for stabilization of enzymes in organic solvents. ACS Catal. 3, 2823–2836. doi: 10.1021/cs400684x
Sun, Q., Fu, C., Aguila, B., Perman, J., Wang, S., Huang, H., et al. (2018). Pore environment control and enhanced performance of enzymes infiltrated in covalent organic frameworks. J. Am. Chem. Soc. 140, 984–992. doi: 10.1021/jacs.7b10642
Vaidya, L. B., Nadar, S. S., and Rathod, V. K. (2020). Entrapment of surfactant modified lipase within zeolitic imidazolate framework (ZIF)-8. Int. J. Biol. Macromol. 146, 678–686. doi: 10.1016/j.ijbiomac.2019.12.164
Wang, L., Wang, Y., He, R., Zhuang, A., Wang, X., Zeng, J., et al. (2013). A new nanobiocatalytic system based on allosteric effect with dramatically enhanced enzymatic performance. J. Am. Chem. Soc. 135, 1272–1275. doi: 10.1021/ja3120136
Wang, Q., Zhang, X., Huang, L., Zhang, Z., and Dong, S. (2017). GOx@ZIF-8(NiPd) nanoflower: an artificial enzyme system for tandem catalysis. Angew. Chem. Int. Ed. 56, 16082–16085. doi: 10.1002/anie.201710418
Wang, W., Wang, L., Huang, Y., Xie, Z., and Jing, X. (2016). Nanoscale metal-organic framework-hemoglobin conjugates. Chem. Asian. J. 11, 750–756. doi: 10.1002/asia.201501216
Wang, Y., Zhang, N., Zhang, E., Han, Y., Qi, Z., Ansorge-Schumacher, M. B., et al. (2019). Heterogeneous metal-organic-framework-based biohybrid catalysts for cascade reactions in organic solvent. Chem. Eur. J. 25, 1716–1721. doi: 10.1002/chem.201805680
Weber, J., Schmidt, J., Thomas, A., and Bohlmann, W. (2010). Micropore analysis of polymer networks by gas sorption and 129Xe NMR spectroscopy: toward a better understanding of intrinsic microporosity. Langmuir 26, 15650–15656. doi: 10.1021/la1028806
Wen, L., Gao, A., Cao, Y., Svec, F., Tan, T., and Lv, Y. (2016). Layer-by-layer assembly of metal-organic frameworks in macroporous polymer monolith and their use for enzyme immobilization. Macromol. Rapid Commun. 37, 551–557. doi: 10.1002/marc.201500705
Wu, M., and Yang, Y. (2017). Metal-organic framework (MOF)-based drug/cargo delivery and cancer therapy. Adv. Mater. 29:1606134. doi: 10.1002/adma.201606134
Wu, X., Ge, J., Yang, C., Hou, M., and Liu, Z. (2015a). Facile synthesis of multiple enzyme-containing metal-organic frameworks in a biomolecule-friendly environment. Chem. Commun. 51, 13408–13411. doi: 10.1039/c5cc05136c
Wu, X., Yang, C., Ge, J., and Liu, Z. (2015b). Polydopamine tethered enzyme/metal–organic framework composites with high stability and reusability. Nanoscale 7, 18883–18886. doi: 10.1039/c5nr05190h
Wu, X., Yang, C., and Ge, J. (2017). Green synthesis of enzyme/metal-organic framework composites with high stability in protein denaturing solvents. Bioresour. Bioprocess. 4:24. doi: 10.1186/s40643-017-0154-8
Xiao, D., Gonzalez, M. I., Darago, L. E., Vogiatzis, K. D., Haldoupis, E., Gagliardi, L., et al. (2016). Selective, tunable O2 binding in cobalt(II)-triazolate/pyrazolate metal-organic frameworks. J. Am. Chem. Soc. 138, 7161–7170. doi: 10.1021/jacs.6b03680
Zhang, C., Wang, X., Hou, M., Li, X., Wu, X., and Ge, J. (2017). Immobilization on metal-organic framework engenders high sensitivity for enzymatic electrochemical detection. ACS Appl. Mater. Interfaces 9, 13831–13836. doi: 10.1021/acsami.7b02803
Zhang, Y., Ge, J., and Liu, Z. (2015). Enhanced activity of immobilized or chemically modified enzymes. ACS Catal. 5, 4503–4513. doi: 10.1021/acscatal.5b00996
Zhao, M., Zhang, X., and Deng, C. (2015). Rational synthesis of novel recyclable Fe3O4@MOF nanocomposites for enzymatic digestion. Chem. Commun. 51, 8116–8119. doi: 10.1039/c5cc01908g
Zhou, Z., and Hartmann, M. (2013). Progress in enzyme immobilization in ordered mesoporous materials and related applications. Chem. Soc. Rev. 42, 3894–3912. doi: 10.1039/c3cs60059a
Keywords: metal–organic frameworks, enzymatic reactions, size-selective catalysis, heterogeneous catalysis, biocatalysis
Citation: Wang Y, Zhang N, Tan D, Qi Z and Wu C (2020) Facile Synthesis of Enzyme-Embedded Metal–Organic Frameworks for Size-Selective Biocatalysis in Organic Solvent. Front. Bioeng. Biotechnol. 8:714. doi: 10.3389/fbioe.2020.00714
Received: 15 February 2020; Accepted: 08 June 2020;
Published: 07 July 2020.
Edited by:
Ao Xia, Chongqing University, ChinaReviewed by:
Kit Wayne Chew, Xiamen University Malaysia, MalaysiaAnwar Sunna, Macquarie University, Australia
Copyright © 2020 Wang, Zhang, Tan, Qi and Wu. This is an open-access article distributed under the terms of the Creative Commons Attribution License (CC BY). The use, distribution or reproduction in other forums is permitted, provided the original author(s) and the copyright owner(s) are credited and that the original publication in this journal is cited, in accordance with accepted academic practice. No use, distribution or reproduction is permitted which does not comply with these terms.
*Correspondence: Changzhu Wu, d3VAc2R1LmRr