Surface Triggered Self-Assembly of Fmoc-Tripeptide as an Antibacterial Coating
- 1Université de Strasbourg, CNRS, Institut Charles Sadron UPR 22, Strasbourg, France
- 2Institut National de la Santé et de la Recherche Médicale, UMR-S 1121, “Biomatériaux et Bioingénierie”, Strasbourg, France
- 3Faculté de Chirurgie Dentaire, Fédération de Médecine Translationnelle de Strasbourg and Fédération des Matériaux et Nanoscience d’Alsace, Université de Strasbourg, Strasbourg, France
In western countries, one patient on twenty will develop a nosocomial infection during his hospitalization at health care facilities. Classical antibiotics being less and less effective, this phenomenon is expanding year after year. Prevention of bacteria colonization of implantable medical devices constitutes a major medical and financial issue. In this study, we developed an antibacterial coating based on self-assembled Fmoc-tripeptide. Fmoc-FFpY peptides (F: phenylalanine; Y: tyrosine; p: PO42–) are dephosphorylated enzymatically into Fmoc-FFY by action of alkaline phosphatase functionalized silica nanoparticles (NPs@AP), previously deposited on a surface. Fmoc-FFY peptides then self-assemble through π–π stacking interactions, hydrogen bonds and hydrophobic interactions adopting β-sheets secondary structures. The obtained hydrogel coatings show fibrillary structures observed by cryo-scanning electron microscopy with a thickness of few micrometers. At low concentration (≤0.5 mg.mL–1), self-assembled Fmoc-FFY has a superior antibacterial activity than Fmoc-FFpY peptide in solution. After 24 h of incubation, Fmoc-FFY hydrogel coatings fully inhibit the development of Gram-positive Staphylococcus aureus (S. aureus). The antibacterial effect is maintained on an in vitro model of repetitive infection in the case of S. aureus. This coating could serve in infections were Gram positive bacteria are prevalent, e.g., intravascular catheter infections. This work gives new insights toward the design of an alternative antimicrobial coating.
Introduction
Biomedical implants, i.e., prosthetics, catheters or intraocular lenses, are indispensable in medicine and gain increasing attention over the years (Lombardi et al., 2019). However, the direct contact of their surfaces with biological fluids becomes susceptible to bacterial colonization and biofilm formation leading to a major medical and financial issue (McCloskey et al., 2014). Implant-associated infections increase the probability of implant failure and may result, if untreated, in chronic microbial infection, inflammation, tissue necrosis, and even morbidity (McCloskey et al., 2014). In western countries one patient on twenty will develop a nosocomial infection during his hospitalization at health care facilities (Cassini et al., 2016). These infections are mostly due to Staphylococcus epidermidis, in the case of intravascular catheter-associated infections (Rupp and Archer, 1994), and Staphylococcus aureus (S. aureus) in the case of metallic implants (Barth et al., 1989). Biofilm formation contributes to the resistance to antibiotic treatments, it protects the bacterial colonies from host defense systems and bactericidal agents (Kaplan, 2011). This leads to the chemotherapeutic failure which often results in an increase of the resistance mechanism adopted by many bacterial strains, particularly those involving S. aureus (Darouiche, 2004). In this scenario, the development of antimicrobial coatings to protect against such infections has become a major field of scientific and technological research (Séon et al., 2015). As alternative to antibiotics, antimicrobial peptides are promising candidates to overcome pathogen resistance and for clinical exploitation (Lombardi et al., 2019). Self-assembly of peptides, with a sequenced-defined chemical structure, can give hydrogels with a desired functionality which becomes increasingly attractive in the development of therapeutics materials (Makam and Gazit, 2018; Criado-Gonzalez et al., 2020a).
In the last decade, low molecular weight hydrogelators (LMWH) fabricated from natural biomolecules such as amino acids got great interest due to their ease and powerful bottom-up fabrication. They self-assemble into supramolecular hydrogels by non-covalent interactions, i.e., electrostatic, van der Waals forces, hydrogen bonding and π-π stacking (McCloskey et al., 2014), in response to external stimuli, such as temperature change (Collier et al., 2001), pH switch (Aggeli et al., 2003; Chen et al., 2011), solvent change (Mahler et al., 2006), electrostatic interactions (Criado-Gonzalez et al., 2020b), chemical (Bowerman and Nilsson, 2010; Zhao et al., 2011) or enzymatic reactions (Yang et al., 2004; Yang and Xu, 2006; Criado-Gonzalez et al., 2019b). Ultrashort peptides, up to 7 amino acids in length, are an emerging class of LMWH of increasing interest due to their versatility in molecular design, ease synthesis, and costs reduction. Few studies have been carried out on the development of self-assembling materials with antibacterial properties using short peptides. Based on the pioneering work of Moir and coll. (Soscia et al., 2010; Kumar et al., 2016) which demonstrated the antimicrobial activity of an amyloid-β peptide, Schnaider et al. (2017) proved the antibacterial activity of a self-assembled dipeptide, diphenylalanine (FF), against Escherichia Coli (E. coli). In this work, FF moiety was identified as the central recognition module of amyloid, as well as the fundamental self-assembly motif proving the efficiency of this unit for the development of self-assembling antimicrobial hydrogels. Fluorenylmethyloxycarbonyl-FF (Fmoc-FF) derived peptides were shown to possess excellent activity against the most antibiotic resistant biofilm phenotype of both Gram-positive and Gram-negative bacteria (McCloskey et al., 2017). Fmoc-FF self-assemblies were employed as host hydrogels to incorporate silver nanoparticles to improve the antimicrobial response (Paladini et al., 2013). The backbone of this dipeptide was modified incorporating an urea moiety to inhibit the E. coli bacterial adhesion (Basavalingappa et al., 2019).
Poorly soluble in water, Fmoc-FF peptide self-assembly is induced by dissolution in DMSO followed by a dilution step in water (Basavalingappa et al., 2019), by change of pH (Paladini et al., 2013), or by heating the solution, up to 90°C for solubilization, followed by a cooling step (Kumar et al., 2016). To overcome these tedious processes, the enzyme assisted self-assembly (EASA) of peptides was introduced by Xu and co-workers (Yang et al., 2004; Yang and Xu, 2006). Soluble at room temperature in aqueous solution, phosphorylated peptides were transformed into gelators by alkaline phosphatase (AP) catalyzing the removal of the phosphate groups. Later, we introduced the use of non-self-assembling Fmoc-FFpY peptide (Y: tyrosine; p: PO42–), which is transformed into the hydrogelator Fmoc-FFY by AP. The enzymatic assisted self-assembly of Fmoc-FFpY was localized on a planar substrate (Vigier-Carrière et al., 2017) or on silica nanoparticles (Criado-Gonzalez et al., 2019a) thanks to the functionalization of their surfaces by AP. In contrast to the pH triggered self-assembly (Jayawarna et al., 2006; Raeburn et al., 2012), the EASA allows to use a more water-soluble peptide as a precursor and to obtain, by simple contact with the peptide solution, a fast and localized self-assembly on the surface of a substrate, previously functionalized by the enzyme.
Integrating self-assembling peptides with antimicrobial property on the surface of a biomaterial is an innovative design for the development of antibacterial materials. Herein, we report an antibacterial coating based on Fmoc-FFY hydrogel self-assembled by enzymatic dephosphorylation. To this aim, AP functionalized silica nanoparticles (NPs@AP) were first deposited on the surface of a material using the layer-by-layer method and then put in contact with Fmoc-FFpY solution to obtain the Fmoc-FFY hydrogel coating (Scheme 1). NPs@AP allowed to immobilize a higher quantity of enzyme on the surface than AP monolayer. The antimicrobial property was tested against S. aureus, Gram-positive strain which is one of the most virulent bacteria leading to high rates of device-related systemic infections and mortality and E. coli, a Gram-negative strain mainly found on the surface of urinary catheters.
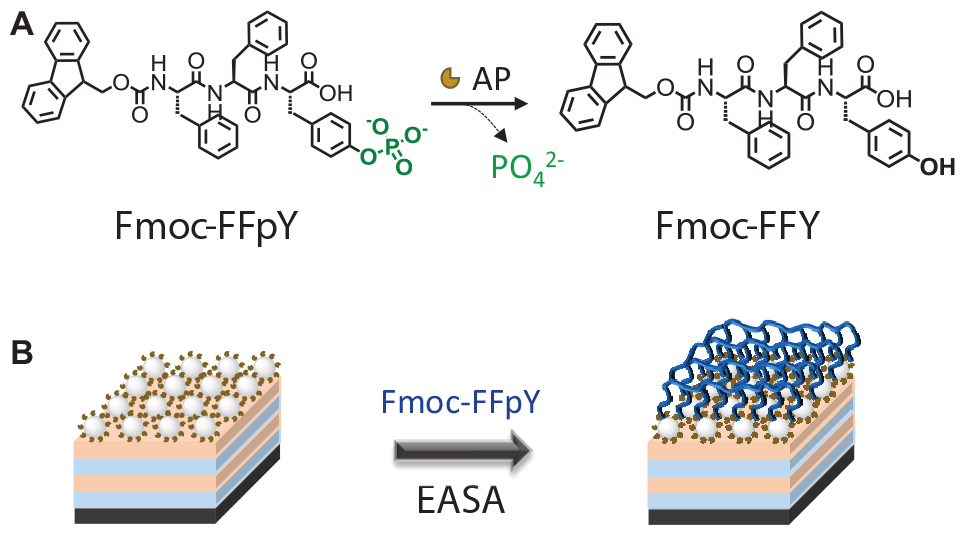
Scheme 1 Schematic representation of Fmoc-FFY hydrogel coating: (A) Enzyme-assisted self-assembly (EASA) of Fmoc-FFY by Fmoc-FFpY dephosphorylation (B) obtained on the surface of NPs@AP coating deposited on polyelectrolyte multilayer film.
Materials and Methods
Materials
Poly(ethylene imine) (PEI, Mw = 250 000 g.mol–1), Poly(allylamine hydrochloride) (PAH, Mw = 58 000 g.mol–1), Alkaline Phosphatase (AP, 10 DEA units.mg protein–1) from bovine intestinal mucosa, Poly(sodium 4-styrenesulfonate) (PSS, Mw = 70 000 g.mol–1), Tetraethyl orthosilicate (TEOS), (3-glycidyloxypropyl) trimethoxysilane (GPMS) were from Sigma Aldrich. p-Nitrophenyl phosphate (PNP) was from ThermoFisher Scientific. Sodium tetra-borate anhydrous (borax) and dry toluene were supplied by Acros Organics. Fmoc-FFpY was purchased by PepMic, ammonium hydroxide by Carlo Erba and ethanol by VWR. Mueller Hinton (MH) broth was purchased from Merck (Germany).
Synthesis and Functionalization of Silica Nanoparticles
The synthesis of silica nanoparticles (NP) and their functionalization with AP were carried out following the procedure described previously (Criado-Gonzalez et al., 2019a). After their synthesis, NPs were functionalized covalently by AP using an epoxy silane coupling agent, giving rise to NPs@AP (Supplementary Data). The catalytic activity of NPs@AP suspensions was determined employing PNP, a substrate which is transformed in para-nitrophenol (λmax = 405 nm) by action of AP.
Multilayer Film Preparation and Fmoc-FFpY Self-Assembly
All solutions were prepared in 25 mM borax buffer pH 9.5. Multilayer films were built on glass slides of 12 mm diameter (Marienfeld) or silicon slides of 10 × 10 mm (Sil’tronix Silicon Technologies). As the substrates are negatively charged, a precursor layer of branched PEI (1 mg.mL–1) was deposited to achieve a homogeneous distribution of positive charges over the surface allowing an efficient deposition of the coating. For that, substrates were immersed in a solution of PEI for 5 min followed by a rinsing step with borax buffer for 2 min. After that, the multilayer film was built up through sequential contact of the substrate with 400 μL of PSS (1 mg.mL–1) (polyanion) or PAH (1 mg.mL–1) (polycation) solutions for 5 min with a rinsing step in borax buffer of 2 min after each polyelectrolyte deposition. This cycle was repeated two times and samples were denoted as polyelectrolyte multilayer (PEM). Then the substrate was put in contact with 400 μL of NPs@AP (1.25% w/v in borax buffer) suspension for 60 min followed by three rinsing steps of 2 min in borax buffer. Samples were denoted as NPs@AP coatings. Subsequently, 400 μL Fmoc-FFpY (1 mg.mL–1) solution was brought in contact with NPs@AP coating for 16 h.
Quartz Crystal Microbalance With Dissipation Monitoring (QCM-D)
Quartz crystal microbalance with dissipation monitoring experiments were performed in a QCM-D cell on a Q-Sense E1 apparatus (Q-Sense AB, Gothenburg, Sweden) at 22°C using an open cell. The resonance frequencies of a gold coated crystal and the dissipation factors at the fundamental frequency at 5 MHz (ν = 1) were monitored during the deposition of NPs@AP and the Fmoc-FFY self-assembly. The perturbation due to the pipetting of the solutions at each deposition steps were removed from the plot.
Atomic Force Microscopy (AFM)
Atomic force microscopy Multimode Nanoscope IV (Bruker, Palaiseau, France) was used to analyze the surface topography of the coatings. Micrographs were recorded in Peak Force Tapping (ScanAsyst) mode by using silicon tips from Bruker (Model: ScanAsyst-Air) mounted on aluminum coated silicon nitride cantilevers. All samples were observed in dry state with triangular cantilevers having a spring constant of around 0.4 N.m–1 and a nominal tip radius of 2 nm. Selected AFM images were treated with the NanoScope Analysis Software (version 1.7). Samples were prepared on silicon substrates and air dried before analysis. Prior to the sample preparation, the silicon wafers were incubated in ethanol/water (50% by vol) mixture for 15 min and activated using plasma treatment for 3 min.
Circular Dichroism (CD)
Circular dichroism (CD) spectra were recorded between 190 and 320 nm using a Jasco J-1100 spectropolarimeter with a data pitch of 1 nm on the light wavelength. Samples were built up on a quartz slide of 1 mm thickness.
Fluorescence Spectroscopy
Fluorescence spectra were recorded between 300 and 355 nm using a Fluoromax-4 (Horiba Jobin Yvon – Edison, NJ, United States) at an excitation wavelength of 290 nm using a quartz slide of 1 mm thickness to build up the samples.
Scanning Electron Microscopy (SEM) and Cryo-SEM
The Fmoc-FFY hydrogel coating on a Silicon wafer was placed on a home-made cryo-holder (Vigier-Carrière et al., 2017) to be quickly plunged into an ethane slush. As the sample is free standing over the holder, the sample is rapidly frozen during the plunging by direct contact with the liquid ethane. Subsequently, the sample is transferred into the Quorum PT 3010 chamber attached to the microscope. There, the frozen sample is fractured with a razor blade. A slight etching at −90°C may be performed to render the fibers more visible. The sample is eventually transferred in the FEG-cryo SEM (Hitachi SU8010) and observed at 1 kV at −150°C.
Inverted Tube Tests
All solutions were prepared in 25 mM borax buffer pH = 9.5. 150 μL of Fmoc-FFpY (1 mg.mL–1) was mixed with 50 μL of NPs@AP (5% w/v) in vials. After 24 h, inverted tube tests were carried out to determine the hydrogel formation. Then, 400 μL of bacteria culture medium (MH) was added and the stability was checked up to 9 days.
Antibacterial Activity
Antibacterial tests were carried out employing one strain Gram-positive bacteria, Staphylococcus aureus (S. aureus, ATCC 25923) and another strain Gram-negative bacteria, Escherichia coli (E. coli, ATCC 25922). S. aureus and E. coli were precultured separately in aerobic conditions at 37°C in a MH broth medium (Merck, Germany), pH 7.4. One colony from previously prepared agar plates by bacteria streaking protocol was transferred to 7 mL of MH medium and incubated in an agitator overnight at 37°C. To obtain bacteria in their mid-logarithmic phase of growth, the absorbance at 620 nm (OD620) of overnight cultures was adjusted to 0.001 by diluting in MH, corresponding to a final cell density of approximately 8 × 105 CFU.mL–1. Cultures growing in the presence of antibiotics (Tetracycline and Cefotaxime) were taken as positive control. Bacteria quantification (in colony forming unit per mL, CFU.mL–1) was performed at time zero and 24 h after the incubation with the samples. This was determined by plating 100 μL of the supernatant, after serial dilution, on nutrient agar plates at 37°C overnight, then the viable cell colonies were counted and represented as log10 reduction (CFU.mL–1). To determine the Minimum Inhibitory Concentration (MIC) value of Fmoc-FFpY and Fmoc-FFY hydrogel, the peptide was dissolved in RPMI medium at 2 mg.mL–1 and at lower concentrations by serial dilution in RPMI. S. aureus was pre-cultured overnight in MH medium, and diluted in RPMI medium. MIC value of Fmoc-FFpY was determined by mixing 100 μL of the peptide solution and 100 μL of S. aureus suspension with a final optical density of OD = 0.001. To form the Fmoc-FFY hydrogel, 100 μL of the peptide solution (at different concentrations) was mixed with 100 μL of AP solution (1.4 μg.mL–1) and left 24 h at 22°C. MIC value of Fmoc-FFY hydrogel was determined by adding to the hydrogel 200 μL of S. aureus inoculation with a final optical density of OD620 = 0.001. Fmoc-FFY hydrogel coating samples were built up on glass substrates of 12 mm diameter, placed in 24-well plates and sterilized for 20 min by UV light. 400 μL of S. aureus or E. coli inoculation (OD620 = 0.001) were added to each well and incubated at 37°C for 24 h. In repetitive culture experiments, the supernatant was totally removed and replaced by 400 μL of freshly prepared S. aureus or E. coli inoculation (OD620 = 0.001). Regarding the statistical analysis, all experiments were carried out independently in triplicate and three analyses per replication at least were done. The significant differences in the experimental data were analyzed using the ANOVA procedure (SAS Institute Inc., Cary, NC, United States) at p < 0.01 with mean separation determined by Tukey’s multiple range tests.
Cell Cytotoxicity Test
Cytotoxicity assays were carried out by incubating the gels with 1 mL of DMEM at 37°C. After 24 and 48 h, the extracts were removed under sterile conditions. Separately, NIH 3T3 mouse embryonic fibroblasts cells were seeded at a density of 1 × 105 cells.mL–1 in complete medium in a sterile 24 well culture plate and incubated to confluence. After 24 and 48 h of incubation, the medium was replaced with the corresponding extracts and incubated at 37°C in humidified air with 5% CO2 for 24 h and 48 h. Subsequently, plates were incubated with 500 μL per well of a MTT solution (0.1% w/v 3-(4,5-dimethylthiozol-2-yl)-2,5-diphenyltetrazolium bromide in Phosphate Buffer Saline) and incubated for 180 min at 37°C. Medium was displaced by 500 μL of DMSO. Optical Density (OD) was measured at 570 nm. The cell viability was calculated from Eq. (1):
where ODS, ODB, and ODC are the optical density for the sample (S), blank (culture medium) (B), and control (glass) (C), respectively.
Results and Discussion
Physico-Chemical Characterization of Fmoc-FFY Hydrogel Coating
Alkaline phosphatase functionalized silica nanoparticles suspensions were obtained with a solid content of 5% (w/v) and had an average diameter of 132 ± 10 nm (Supplementary Figure S1). The suspension showed an activity equivalent to 30 units.mL–1 determined using PNP, a model substrate of AP, with a calibration curve (Supplementary Figure S2). To immobilize NPs@AP on a planar surface, a PEI-(PSS/PAH)2 polyelectrolyte multilayer film (PEM) was first deposited as precursor film. When NPs@AP suspension is put in contact with the PEM precursor film, a high increase of the normalized frequency shift is observed by QCM-D reaching about 700 Hz after the rinsing steps (Figure 1A).
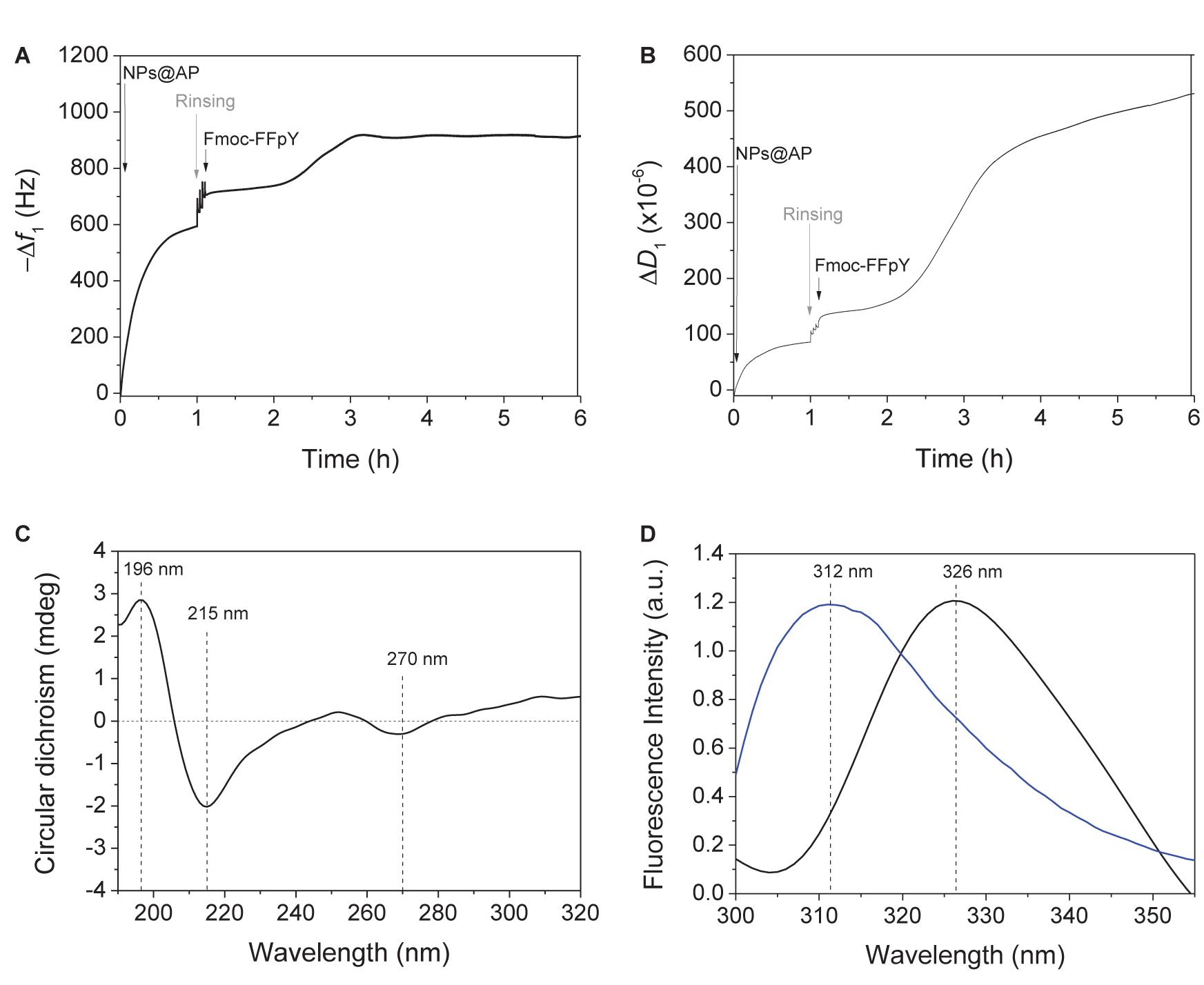
Figure 1. Evolution of the (A) fundamental frequency shift and (B) dissipation value, measured by QCM-D at 5 MHz, during the deposition of NPs@AP on PEM precursor film, leading to NPs@AP coating, followed by the contact with Fmoc-FFpY solution leading to the Fmoc-FFY hydrogel coating. (C) Circular Dichroism and (D) Fluorescence emission (λex = 290 nm) spectra of Fmoc-FFY hydrogel coating (black curve) and Fmoc-FFpY solution (blue curve).
The obtained NPs@AP coating has a catalytic activity equivalent to 0.6 μg.mL–1 of AP in solution, corresponding to 0.08 μg.cm−2 of active enzyme. AFM images of NPs@AP coating showed round shape NPs all over the surface (Supplementary Figure S3). The section profile of the AFM images provides an average diameter of the deposited NPs of 140 nm (Supplementary Figure S3C) in agreement with SEM observations (Supplementary Figure S1). The NPs@AP coating was then employed as catalytic support for the localized growth of supramolecular peptide by putting it in contact with Fmoc-FFpY solution (1 mg.mL–1) (Scheme 1). The Fmoc-FFY self-assembly induced an increase of the frequency shift of about 230 Hz after 3.5 h (Figure 1A). The dissipation value reached 400 × 10–6 which is the signature of highly hydrated films (Figure 1B). In contrast, NPs@AP coating had a dissipation value of 130 × 10–6. By decreasing Fmoc-FFpY concentration of the solution in contact with NPs@AP coating, the final frequency shift decreased reaching 100 Hz at 0.5 and 0.25 mg.mL–1 and 50 Hz at 0.1 mg.mL–1. This was corroborated by the decrease of the dissipation value (Supplementary Figures S4A,B). As a control experiment, the contact of a non-self-assembling peptide, Fmoc-G-OH (1 mg.mL–1), with the NPs@AP coating induces an increase of 43 Hz of the frequency shift (Supplementary Figures S4C,D). Fmoc-G-OH peptide is probably physisorbed on the surface such as Fmoc-FFpY when the concentration is lower than 0.1 mg.mL–1.
The structural organization of Fmoc-FFY self-assembled on NPs@AP coating, named Fmoc-FFY hydrogel coating, was investigated by CD (Figure 1C and Supplementary Figure S5). The CD spectrum shows a positive band at 196 nm and a strong negative band at 215 nm characteristics of β-sheets structures (Smith et al., 2008). The presence of a negative band at 270 nm is assigned to offset face-to-face stacking of the Fmoc moieties which is not observed in the case of non-self-assembling Fmoc-FFpY in solution (Ryan et al., 2010). The excimer formation of Fmoc moieties was checked after Fmoc-FFY self-assembly by fluorescence spectroscopy (Tang et al., 2011; Criado-Gonzalez et al., 2019b). When excited at 290 nm, Fmoc-FFpY solution have a fluorescence emission peak at 312 nm due to fluorenyl moieties (Figure 1D). The self-assembly of Fmoc-FFY on NPs@AP coating induced a shift of this peak up to 326 nm, due to fluorenyl excimers.
Morphological Characterization of Fmoc-FFY Deposited Hydrogel
The morphology of the Fmoc-FFY hydrogel coating was visualized by Cryo-SEM (Figure 2). The cross-section of the coating showed three different areas. The magnification of the bottom part allowed to distinguish monolayer and multilayers of round shape NPs@AP, with a size of ∼ 120 nm determined using ImageJ, on the surface of the glass slide (Figure 2B). It can be noticed that there is no diffusion of NPs@AP throughout the whole Fmoc-FFY hydrogel after 16 h in contact (dashed line in Figure 2B). Fmoc-FFY fibers grow from the NPs@AP giving rise to a hydrogel coating with a thickness of ∼ 5 μm. A gradient of organic matter is observed in the self-assembled Fmoc-FFY hydrogel with two different areas (Figure 2A). Higher density of fibers is close to the bottom, in direct contact NPs@AP, and a lower density on the upper part of the Fmoc-FFY hydrogel. A magnification of the upper part allows to distinguish long fibers of micrometer length oriented in the vertical direction perpendicular to the substrate (Figure 2C). Fmoc-FFY fibers have a diameter ranging from 13 to 40 nm (red arrows in Figure 2C).
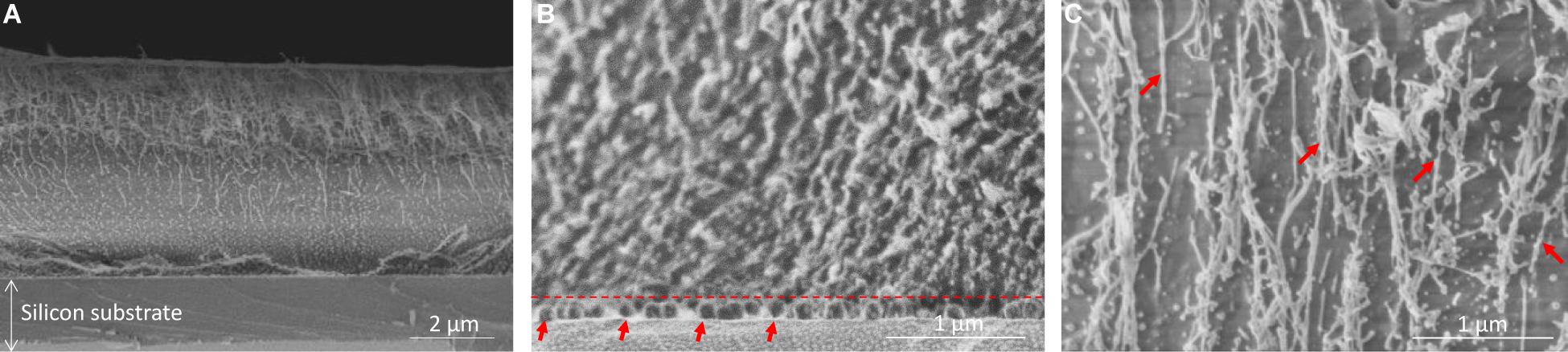
Figure 2. Cryo-SEM images of (A) Fmoc-FFY hydrogel self-assembled on NPs@AP coating, (B) zoom-in of the bottom area to show the localization of the NPs@AP delimited with a dashed line and highlighted with red arrows and (C) zoom-in of the upper part showing Fmoc-FFY fibers (red arrows).
Antimicrobial Properties of Fmoc-FFY Hydrogel Coating
Before to study the antibacterial properties of the Fmoc-FFY hydrogel coating, the stability of the hydrogel was tested in contact with bacteria culture medium. For that purpose, Fmoc-FFY hydrogel was formed in a vial by mixing Fmoc-FFpY solution and NPs@AP suspension. The inverted tube test indicated the formation of a gel after 24 h (Supplementary Figure S6). The hydrogel remains stable up to 9 days in contact with MH culture medium. To underline the necessity of the enzymatic dephosphorylation of the peptide, the antibacterial properties of Fmoc-FFpY and Fmoc-FFY hydrogel were studied against Gram-positive S. aureus in RPMI media at different concentrations in peptide (Figure 3A). Bacteria quantification (in colony forming unit per mL, CFU.mL–1) was performed at time zero and 24 h after the incubation with the samples and presented in log10 reduction. At 1 mg.mL–1, 3-log10 (99%) reduction bacterial load against S. aureus is obtained for Fmoc-FFpY peptide, whereas 4-log10 (99.99%) reduction were achieved with Fmoc-FFY hydrogel, obtained by dephosphorylation of the peptide. At lower peptide concentrations (≤0.5 mg.mL–1), the antibacterial property of Fmoc-FFpY is lost whereas Fmoc-FFY hydrogel showed 3-log10 (99%) reduction of S. aureus suspension up to 0.1 mg.mL–1 of peptide. The self-assembly of the peptide ensure the antibacterial effect at low concentration. Bare NPs@AP coatings did not prevent the proliferation of the bacteria (Supplementary Figure S7). The antibacterial effect of the Fmoc-FFY hydrogel coating, self-assembled on NPs@AP at different concentrations in Fmoc-FFpY, was tested against S. aureus (Figure 3B). After 24 h of contact, Fmoc-FFY hydrogel coatings demonstrated efficacy of more than 4-log10 (99.99%) reduction of bacterial load against S. aureus whatever the concentration of peptide used for their self-assembly up to 0.1 mg.mL–1. To mimic the worst conditions of repetitive bacterial infections, for example in the case of urinary or venous catheter-associated infections, the bacterial inoculation in contact with the hydrogel coating was completely renewed every 24 h. At least 3-log10 (99%) reduction of S. aureus was observed for the second culture in contact with Fmoc-FFY hydrogel coating self-assembled with 1 mg.mL–1 Fmoc-FFpY. At lower concentration in peptide, the second culture with S. aureus showed between 2 and 1-log10 (90%) reduction. In the following culture, a significant decrease in efficiency is detectable with 1-log reduction only with 1 mg.mL–1. Fmoc-FFY hydrogel coating, self-assembled with 1 mg.mL–1 Fmoc-FFpY, showed only 1-log reduction of bacterial load against Gram-negative E. coli after 24 h with no significant inhibition at the second culture (Figure 3C). A selective antibacterial effect of Fmoc-FFY hydrogel coating was observed against S. aureus Gram-positive bacteria in contrast to E. coli Gram-negative bacteria.
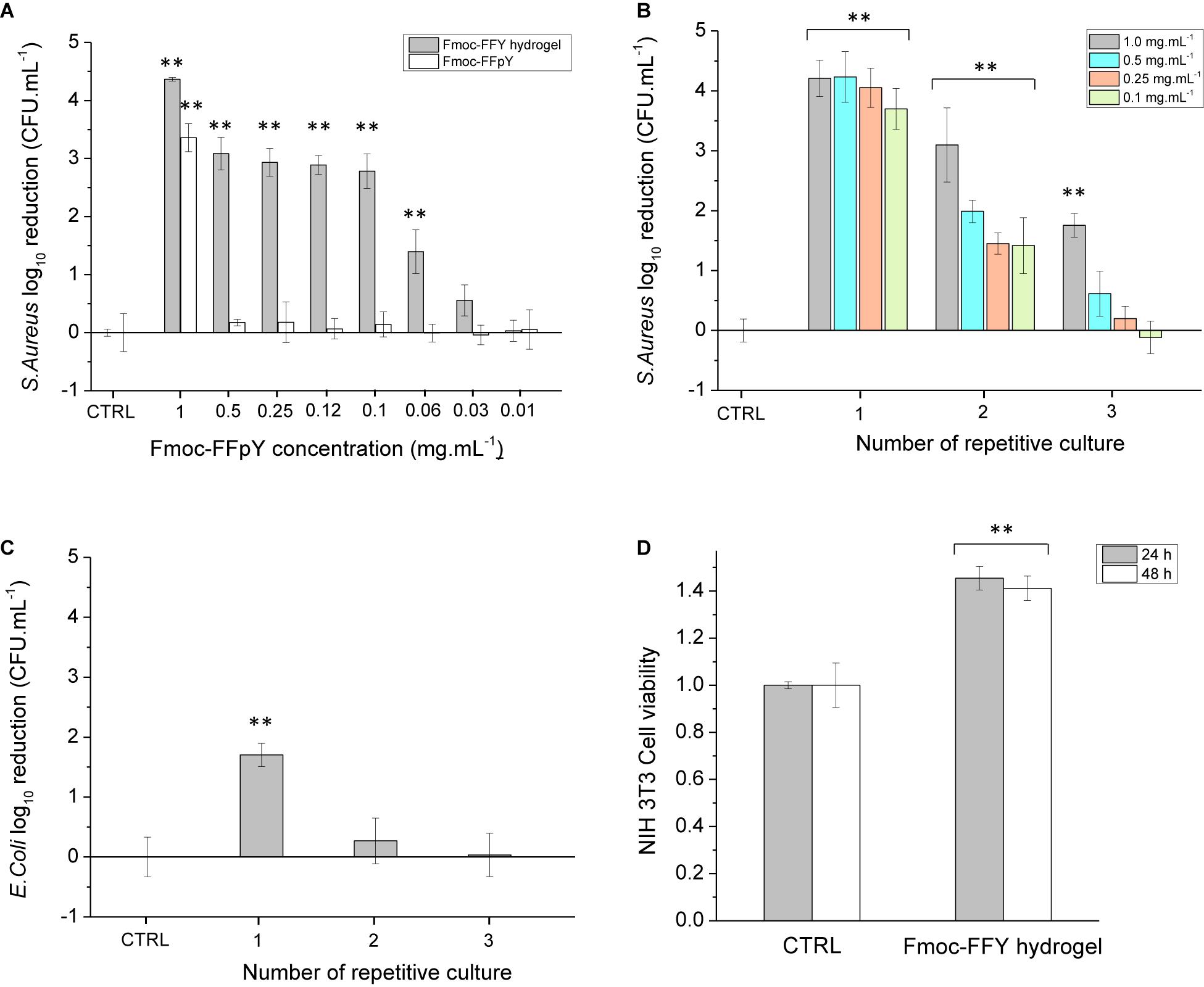
Figure 3. Colony-forming unit (CFU) log10 reduction on planktonic (A) S. aureus cells incubated in the presence of different concentrations of Fmoc-FFpY and of Fmoc-FFY hydrogel, self-assembled with different concentrations of Fmoc-FFpY, (B) S. aureus cells incubated on Fmoc-FFY hydrogel coatings, self-assembled with different concentrations of Fmoc-FFpY on NPs@AP coating and (C) E. coli cells incubated on Fmoc-FFY hydrogel coating, self-assembled with 1 mg.mL– 1 Fmoc-FFpY solution on NPs@AP coating, as a function of the number of repetitive culture. The coating was brought in contact with a fresh pathogen suspension for 24 h. In repetitive culture experiments, the supernatant was removed and replaced every 24 h by a fresh suspension. (D) Cytotoxicity assay of Fmoc-FFY gel through MTT indirect test using NIH 3T3 mouse embryonic fibroblasts cells. Uncoated glass substrates were employed as controls (CTRL). Diagrams include the results from three independent experiments in triplicate and results are shown as mean ± s.e.m. and the ANOVA results at a significance level of **p < 0.01 vs. uncoated glass (negative control, CTRL).
The mechanism of action of Fmoc-FFY hydrogels could be attributed to a combination of two factors: the fibrillary β-sheet structure of the self-assembly and the peptide hydrophobicity as it was reported for β-amyloid peptides (Kagan et al., 2012; Last and Miranker, 2013). Pioneering works of Moir and coll. demonstrated the antimicrobial activity of Alzheimer’s β-amyloid peptides in vitro and in vivo (Soscia et al., 2010; Kumar et al., 2016). Diphenylalanine moieties (FF), identified as the core recognition motif of β-amyloid peptides, were reported to self-assemble in discrete and stiff nanotubes adopting β-sheet structures (Reches and Gazit, 2003). Self-assembled FF peptides have the ability to bind to the surface of bacterial membrane, to aggregate and to orient themselves to optimize hydrophilic/hydrophobic interactions, inducing a surface tension leading to membrane depolarization, pore formation and release of cellular content (Schnaider et al., 2017). Moreover, FF peptides also induce upregulation of stress response regulons at sub-lethal concentration causing severe damage to bacterial morphology. Recently, the antibacterial activity of self-assembled Fmoc-F was attributed to the peptide release from the hydrogel inducing oxidative and osmotic stress as well as altering bacterial membrane integrity (Gahane et al., 2018). The comparison of Fmoc-FF cationic peptide, designed with a pyridinium moiety at the C-terminal, with other Fmoc-cationic peptides showed that the proximity of fluorenyl and phenyl moieties achieves an optimum hydrophobicity environment improving the antibacterial activity (Debnath et al., 2010). In summary, the antibacterial property of the Fmoc-FFY hydrogel coating could be explained by the ability of the β-sheet self-assembled nanofibers to bind and aggregate on the surface of the bacterial membrane, altering the membrane integrity and also to oxidative and osmotic stresses leading to the death of the bacteria. This mechanism is confirmed by the efficiency of peptide self-assembly at low concentration in comparison to the non-self-assembled peptide. In contrast to Gram-positive, Gram-negative bacteria have an additional outer bilayer membrane, formed by lipopolysaccharides and phospholipids, that makes difficult the diffusion of molecules across the membrane and renders them more resistant to break (Haldar et al., 2005). The cytotoxicity of the Fmoc-FFY gel was determined in vitro, through a MTT indirect test, employing NIH 3T3 mouse embryonic fibroblasts cells (Figure 3D). NIH 3T3 cell viability do not show any decrease of up to 48 h proving that Fmoc-FFY gel is not cytotoxic. This result confirmed the absence of toxicity of Fmoc-FF peptides when they are in self-assembled form (Truong et al., 2015). Besides, Fmoc-FF peptides have been used as 3-D cell culture scaffolds for chondrocytes (Jayawarna et al., 2006) or astrocytes (Liebmann et al., 2007).
Conclusion
In this work a phosphorylated peptide, Fmoc-FFpY, able to be solubilized in water at room temperature, was transformed in Fmoc-FFY hydrogelator by action of NPs@AP immobilized on a surface. The hydrogel coating, based on fibers homogeneously distributed all around the sample, was efficient to prevent S. aureus growth with 4-log10 of viable bacteria. This coating could serve in infections were Gram positive bacteria are prevalent, e.g., intravascular catheter infections. This kind of antibacterial self-assembled hydrogel represents a very simple and efficient method to coat biomaterial surfaces for protection against bacteria proliferation. In clinical practice to ensure the integrity of the hydrogel coating, one idea would be to trigger the peptide self-assembly in situ after implantation of the medical device by addition of the peptide solution near the enzyme functionalized implant surface. With the prospect of long-term use of the antibacterial coating, the surface triggered self-assembly of peptide could be done on porous surfaces or even inside the pores of foams. The high surface of coverage, obtained on rough or porous implants, could lead to sustained antibacterial property as the coating maintained its property even after being in contact several times with fresh inoculated S. aureus suspension. Moreover in a curative strategy, the peptide self-assembly could be triggered by using enzymes naturally secreted by bacteria to obtain the antibacterial gel either inside the cells or at their surface using overexpression of phosphatase by bacteria (Yang et al., 2007) or by mammalian cancer cells (Pires et al., 2015).
Data Availability Statement
All datasets presented in this study are included in the article/Supplementary Material.
Author Contributions
PS and FB conceived the project. MC-G, LJ, PS, and FB designed the experiments. MC-G carried out the preparation of all samples under study, QCM-D analyses, gelation tests, circular dichroism, and fluorescence spectroscopy characterization. MI performed the AFM characterization and bacteria tests. MC-G, MI, and FB contributed to the interpretation of the results. AC and MS carried out the SEM and cryo-SEM experiments. All authors contributed to the article and approved the submitted version.
Funding
MI thanks the Higher Education Commission (HEC) Pakistan for his Ph.D. scholarship. We gratefully acknowledge the financial support from the Agence Nationale de la Recherche (EASA, ANR-18-CE06-0025-03).
Conflict of Interest
The authors declare that the research was conducted in the absence of any commercial or financial relationships that could be construed as a potential conflict of interest.
Acknowledgments
ICS microscopy and characterization platforms are acknowledged for the use of the SEM and the fluorescence spectroscopy, respectively.
Supplementary Material
The Supplementary Material for this article can be found online at: https://www.frontiersin.org/articles/10.3389/fbioe.2020.00938/full#supplementary-material
References
Aggeli, A., Bell, M., Carrick, L. M., Fishwick, C. W. G., Harding, R., Mawer, P. J., et al. (2003). pH as a trigger of peptide β-Sheet self-assembly and reversible switching between nematic and isotropic phases. J. Am. Chem. Soc. 125, 9619–9628. doi: 10.1021/ja021047i
Barth, E., Myrvik, Q. M., Wagner, W., and Gristina, A. G. (1989). In vitro and in vivo comparative colonization of Staphylococcus aureus and Staphylococcus epidermidis on orthopaedic implant materials. Biomaterials 10, 325–328. doi: 10.1016/0142-9612(89)90073-2
Basavalingappa, V., Guterman, T., Tang, Y., Nir, S., Lei, J., Chakraborty, P., et al. (2019). Expanding the functional scope of the fmoc-diphenylalanine hydrogelator by introducing a rigidifying and chemically active urea backbone modification. Adv. Sci. 6:1900218. doi: 10.1002/advs.201900218
Bowerman, C. J., and Nilsson, B. L. (2010). A reductive trigger for peptide self-assembly and hydrogelation. J. Am. Chem. Soc. 132, 9526–9527. doi: 10.1021/ja1025535
Cassini, A., Plachouras, D., Eckmanns, T., Abu Sin, M., Blank, H.-P., Ducomble, T., et al. (2016). Burden of six healthcare-associated infections on european population health: estimating incidence-based disability-adjusted life years through a population prevalence-based modelling study. PLoS Med. 13:e1002150. doi: 10.1371/journal.pmed.1002150
Chen, L., Pont, G., Morris, K., Lotze, G., Squires, A., Serpell, L. C., et al. (2011). Salt-induced hydrogelation of functionalised-dipeptides at high pH. Chem. Commun. 47, 12071–12073.
Collier, J. H., Hu, B. H., Ruberti, J. W., Zhang, J., Shum, P., Thompson, D. H., et al. (2001). Thermally and photochemically triggered self-assembly of peptide hydrogels. J. Am. Chem. Soc. 123, 9463–9464. doi: 10.1021/ja011535a
Criado-Gonzalez, M., Fores, J. R., Carvalho, A., Blanck, C., Schmutz, M., Kocgozlu, L., et al. (2019a). Phase separation in supramolecular hydrogels based on peptide self-assembly from enzyme-coated nanoparticles. Langmuir 35, 10838–10845. doi: 10.1021/acs.langmuir.9b01420
Criado-Gonzalez, M., Rodon Fores, J., Wagner, D., Schröder, A. P., Carvalho, A., Schmutz, M., et al. (2019b). Enzyme-assisted self-assembly within a hydrogel induced by peptide diffusion. Chem. Commun. 55, 1156–1159. doi: 10.1039/c8cc09437c
Criado-Gonzalez, M., Loftin, B., Rodon Fores, J., Vautier, D., Kocgozlu, L., Jierry, L., et al. (2020a). Enzyme assisted peptide self-assemblies trigger cell adhesion in high density oxime based host gels. J. Mater. Chem. B 8, 4419–4427. doi: 10.1039/d0tb00456a
Criado-Gonzalez, M., Wagner, D., Rodon Fores, J., Blanck, C., Schmutz, M., Chaumont, A., et al. (2020b). Supramolecular hydrogel induced by electrostatic interactions between polycation and phosphorylated-fmoc-tripeptide. Chem. Mater. 32, 1946–1956. doi: 10.1021/acs.chemmater.9b04823
Darouiche, R. O. (2004). Treatment of infections associated with surgical implants. N. Engl. J. Med. 350, 1422–1429. doi: 10.1056/nejmra035415
Debnath, S., Shome, A., Das, D., and Das, P. K. (2010). Hydrogelation through self-assembly of fmoc-peptide functionalized cationic amphiphiles: potent antibacterial agent. J. Phys. Chem. B 114, 4407–4415. doi: 10.1021/jp909520w
Gahane, A. Y., Ranjan, P., Singh, V., Sharma, R. K., Sinha, N., Sharma, M., et al. (2018). Fmoc-phenylalanine displays antibacterial activity against Gram-positive bacteria in gel and solution phases. Soft Matter. 14, 2234–2244. doi: 10.1039/c7sm02317k
Haldar, J., Kondaiah, P., and Bhattacharya, S. (2005). Synthesis and antibacterial properties of novel hydrolyzable cationic amphiphiles. incorporation of multiple head groups leads to impressive antibacterial activity. J. Med. Chem. 48, 3823–3831. doi: 10.1021/jm049106l
Jayawarna, V., Ali, M., Jowitt, T. A., Miller, A. F., Saiani, A., Gough, J. E., et al. (2006). Nanostructured hydrogels for three-dimensional cell culture through self-assembly of fluorenylmethoxycarbonyl-dipeptides. Adv. Mater. 18, 611–614. doi: 10.1002/adma.200501522
Kagan, B. L., Jang, H., Capone, R., Teran Arce, F., Ramachandran, S., Lal, R., et al. (2012). Antimicrobial properties of amyloid peptides. Mol. Pharm. 9, 708–717. doi: 10.1021/mp200419b
Kaplan, J. B. (2011). Antibiotic-induced biofilm formation. Int. J. Artif. Organs 34, 737–751. doi: 10.5301/ijao.5000027
Kumar, D. K. V., Choi, S. H., Washicosky, K. J., Eimer, W. A., Tucker, S., Ghofrani, J., et al. (2016). Amyloid-β peptide protects against microbial infection in mouse and worm models of Alzheimer’s disease. Sci. Transl. Med. 8, 1–15.
Last, N. B., and Miranker, A. D. (2013). Common mechanism unites membrane poration by amyloid and antimicrobial peptides. Proc. Natl. Acad. Sci. U.S.A. 110:6382. doi: 10.1073/pnas.1219059110
Liebmann, T., Rydholm, S., Akpe, V., and Brismar, H. (2007). Self-assembling Fmoc dipeptide hydrogel for in situ 3D cell culturing. BMC Biotechnol. 7:88. doi: 10.1186/1472-6750-7-88
Lombardi, L., Falanga, A., Del Genio, V., and Galdiero, S. (2019). A new hope: self-assembling peptides with antimicrobial activity. Pharmaceutics 11:166. doi: 10.3390/pharmaceutics11040166
Mahler, A., Reches, M., Rechter, M., Cohen, S., and Gazit, E. (2006). Rigid, self-assembled hydrogel composed of a modified aromatic dipeptide. Adv. Mater. 18, 1365–1370. doi: 10.1002/adma.200501765
Makam, P., and Gazit, E. (2018). Minimalistic peptide supramolecular co-assembly: expanding the conformational space for nanotechnology. Chem. Soc. Rev. 47, 3406–3420. doi: 10.1039/c7cs00827a
McCloskey, A. P., Draper, E. R., Gilmore, B. F., and Laverty, G. (2017). Ultrashort self-assembling Fmoc-peptide gelators for anti-infective biomaterial applications. J. Pept. Sci. 23, 131–140. doi: 10.1002/psc.2951
McCloskey, A. P., Gilmore, B. F., and Laverty, G. (2014). Evolution of antimicrobial peptides to self-assembled peptides for biomaterial applications. Pathogens 3, 791–821. doi: 10.3390/pathogens3040791
Paladini, F., Meikle, S. T., Cooper, I. R., Lacey, J., Perugini, V., and Santin, M. (2013). Silver-doped self-assembling di-phenylalanine hydrogels as wound dressing biomaterials. ıJ. Mater. Sci. Mater. Med. 24, 2461–2472. doi: 10.1007/s10856-013-4986-2
Pires, R. A., Abul-Haija, Y. M., Costa, D. S., Novoa-Carballal, R., Reis, R. L., Ulijn, R. V., et al. (2015). Controlling cancer cell fate using localized biocatalytic self-assembly of an aromatic carbohydrate amphiphile. J. Am. Chem. Soc. 137, 576–579. doi: 10.1021/ja5111893
Raeburn, J., Pont, G., Chen, L., Cesbron, Y., Lévy, R., and Adams, D. J. (2012). Fmoc-diphenylalanine hydrogels: understanding the variability in reported mechanical properties. Soft Matter. 8, 1168–1174. doi: 10.1039/c1sm06929b
Reches, M., and Gazit, E. (2003). Casting metal nanowires within discrete self-assembled peptide nanotubes. Science 300:625. doi: 10.1126/science.1082387
Rupp, M. E., and Archer, G. L. (1994). Coagulase-negative Staphylococci: pathogens associated with medical progress. Clin. Infect. Dis. 19, 231–245. doi: 10.1093/clinids/19.2.231
Ryan, D. M., Anderson, S. B., Senguen, F. T., Youngman, R. E., and Nilsson, B. L. (2010). Self-assembly and hydrogelation promoted by F5-phenylalanine. Soft Matter. 6, 475–479. doi: 10.1039/b916738b
Schnaider, L., Brahmachari, S., Schmidt, N. W., Mensa, B., Shaham-Niv, S., Bychenko, D., et al. (2017). Self-assembling dipeptide antibacterial nanostructures with membrane disrupting activity. Nat. Commun. 8:1365.
Séon, L., Lavalle, P., Schaaf, P., and Boulmedais, F. (2015). Polyelectrolyte multilayers: a versatile tool for preparing antimicrobial coatings. Langmuir 31, 12856–12872. doi: 10.1021/acs.langmuir.5b02768
Smith, A. M., Williams, R. J., Tang, C., Coppo, P., Collins, R. F., Turner, M. L., et al. (2008). Fmoc-Diphenylalanine Self assembles to a hydrogel via a novel architecture based on π-π interlocked β-sheets. Adv. Mater. 20, 37–41. doi: 10.1002/adma.200701221
Soscia, S. J., Kirby, J. E., Washicosky, K. J., Tucker, S. M., Ingelsson, M., Hyman, B., et al. (2010). The Alzheimer’s disease-associated amyloid β-protein is an antimicrobial peptide. PLoS One 5:e9505. doi: 10.1371/journal.pmed.1009505
Tang, C., Ulijn, R. V., and Saiani, A. (2011). Effect of glycine substitution on fmoc-diphenylalanine self-assembly and gelation properties. Langmuir 27, 14438–14449. doi: 10.1021/la202113j
Truong, W. T., Su, Y., Gloria, D., Braet, F., and Thordarson, P. (2015). Dissolution and degradation of Fmoc-diphenylalanine self-assembled gels results in necrosis at high concentrations in vitro. Biomater. Sci. 3, 298–307. doi: 10.1039/c4bm00244j
Vigier-Carrière, C., Wagner, D., Chaumont, A., Durr, B., Lupattelli, P., Lambour, C., et al. (2017). Control of surface-localized, enzyme-assisted self-assembly of peptides through catalyzed oligomerization. Langmuir 33, 8267–8276. doi: 10.1021/acs.langmuir.7b01532
Yang, Z., Gu, H., Fu, D., Gao, P., Lam, J. K., and Xu, B. (2004). Enzymatic formation of supramolecular hydrogels. Adv. Mater. 16, 1440–1444. doi: 10.1002/adma.200400340
Yang, Z., Liang, G., Guo, Z., Guo, Z., and Xu, B. (2007). Intracellular hydrogelation of small molecules inhibits bacterial growth. Angew. Chem. Int. Edn. 46, 8216–8219. doi: 10.1002/anie.200701697
Yang, Z., and Xu, B. (2006). Using enzymes to control molecular hydrogelation. Adv. Mater. 18, 3043–3046. doi: 10.1002/adma.200600400
Keywords: peptides, hydrogels, antimicrobial, enzyme-assisted self-assembly, nanoparticles
Citation: Criado-Gonzalez M, Iqbal MH, Carvalho A, Schmutz M, Jierry L, Schaaf P and Boulmedais F (2020) Surface Triggered Self-Assembly of Fmoc-Tripeptide as an Antibacterial Coating. Front. Bioeng. Biotechnol. 8:938. doi: 10.3389/fbioe.2020.00938
Received: 13 May 2020; Accepted: 20 July 2020;
Published: 07 August 2020.
Edited by:
Patricia Krawczak, IMT Lille Douai, FranceReviewed by:
Garry Laverty, Queen’s University Belfast, United KingdomBing Xu, Brandeis University, United States
Copyright © 2020 Criado-Gonzalez, Iqbal, Carvalho, Schmutz, Jierry, Schaaf and Boulmedais. This is an open-access article distributed under the terms of the Creative Commons Attribution License (CC BY). The use, distribution or reproduction in other forums is permitted, provided the original author(s) and the copyright owner(s) are credited and that the original publication in this journal is cited, in accordance with accepted academic practice. No use, distribution or reproduction is permitted which does not comply with these terms.
*Correspondence: Miryam Criado-Gonzalez, miryamcg22@gmail.com; Fouzia Boulmedais, fouzia.boulmedais@ics-cnrs.unistra.fr