Towards the Anaerobic Production of Surfactin Using Bacillus subtilis
- Department of Bioprocess Engineering (150k), Institute of Food Science and Biotechnology (150), University of Hohenheim, Stuttgart, Germany
The anaerobic growth of B. subtilis to synthesize surfactin poses an alternative strategy to conventional aerobic cultivations. In general, the strong foam formation observed during aerobic processes represents a major obstacle. Anaerobic processes have, amongst others, the distinct advantage that the total bioreactor volume can be exploited as foaming does not occur. Recent studies also reported on promising product per biomass yields. However, anaerobic growth in comparison to aerobic processes has several disadvantages. For example, the overall titers are comparably low and cultivations are time-consuming due to low growth rates. B. subtilis JABs24, a derivate of strain 168 with the ability to synthesize surfactin, was used as model strain in this study. Ammonium and nitrite were hypothesized to negatively influence anaerobic growth. Ammonium with initial concentrations up to 0.2 mol/L was shown to have no significant impact on growth, but increasing concentrations resulted in decreased surfactin titers and reduced nitrate reductase expression. Anaerobic cultivations spiked with increasing nitrite concentrations resulted in prolonged lag-phases. Indeed, growth rates were in a similar range after the lag-phase indicating that nitrite has a neglectable effect on the observed decreasing growth rates. In bioreactor cultivations, the specific growth rate decreased with increasing glucose concentrations during the time course of both batch and fed-batch processes to less than 0.05 1/h. In addition, surfactin titers, overall YP/X and YP/S were 53%, ∼42%, and ∼57% lower than in serum flask with 0.190 g/L, 0.344 g/g and 0.015 g/g. The YX/S, on the contrary, was 30% lower in the serum flask with 0.044 g/g. The productivities q were similar with ∼0.005 g/(g⋅h). However, acetate strongly accumulated during cultivation and was posed as further metabolite that might negatively influence anaerobic growth. Acetate added to anaerobic cultivations in a range from 0 g/L up to 10 g/L resulted in a reduced maximum and overall growth rate μ by 44% and 30%, respectively. To conclude, acetate was identified as a promising target for future process enhancement and strain engineering. Though, the current study demonstrates that the anaerobic cultivation to synthesize surfactin represents a reasonable perspective and feasible alternative to conventional processes.
Introduction
The cyclic lipopeptide surfactin synthesized by Bacillus subtilis is often described as a promising alternative to surfactants of petrochemical and oleochemical origin (Henkel et al., 2017) with additional antimicrobial properties (Ongena and Jacques, 2008; Li et al., 2019). However, conventional aerobic processes targeting at surfactin production share one major bottleneck, namely excessive foaming. The presence of foam in biotechnological processes often results, amongst others, in lower productivity (St-Pierre Lemieux et al., 2019). Excessive foaming may hinder probes to measure correctly, blocks exhaust air filters and hence pressure increases, and leads to heterogeneity in the cultivation broth (St-Pierre Lemieux et al., 2019). Different processes targeting surfactin production were reported that either integrate or avoid foaming. Integration of foaming is mostly performed as in situ product removal (Cooper et al., 1981; Davis et al., 2001; Chen et al., 2006; Willenbacher et al., 2014). Here, the ability of surfactin to accumulate at air-liquid interfaces is used as advantage and can be regarded as a first enrichment and purification step. Nevertheless, the uncontrolled foaming is reported to hinder process control and next to surfactin, also producer cells and the medium is lost for further cultivation (Willenbacher et al., 2014; Rangarajan and Clarke, 2015; Coutte et al., 2017). The membrane-bioreactor presented by Coutte et al. (2010) is an alternative foam-free cultivation strategy. This set-up yielded concentrations of 0.242 g/L surfactin. However, productivity was reduced during the time course of cultivation due to the adsorbance of surfactin onto the membranes which further reduced oxygen transfer. Chtioui et al. (2012) designed a rotating disk bioreactor where surfactin was produced by B. subtilis ATCC 21332 both in free cells and cells immobilized as a biofilm on the rotating disks. Aeration was performed above the liquid level and was reported to not be sufficient and surfactin concentrations did not surpass 0.212 g/L. Another strategy to synthesize surfactin was illustrated by Davis et al. (1999). Different batch cultivations with e.g., nitrate-limitation, carbon-limitation or oxygen-limitation demonstrated that highest specific product yield per biomass (YP/X) was achieved in nitrate-limited oxygen-depleted cultures. The authors reported that anaerobic growth occurred in oxygen-depleted conditions. However, aeration was still maintained at 0.5 vvm indicating the presence of microaerophilic conditions. This strategy was further adapted by Willenbacher et al. (2015a) employing strain B. subtilis DSM 10T. Anaerobic conditions were obtained as aeration was completely avoided, which also resulted in a foam-free environment without the need of adding antifoam. This process reached high values for the product yield per biomass YP/X with 0.278 g/g employing 2.5 g/L glucose. The anaerobic cultivation takes advantage of the ability of B. subtilis to use nitrate as alternative electron acceptor in the absence of oxygen. During nitrate respiration, nitrate is reduced to ammonium via nitrite using the enzymes nitrate reductase NarGHI and nitrite reductase NasDE (Nakano et al., 1998a). A recent study demonstrated that anaerobic serum flask cultivations employing strain B. subtilis JABs24, which is the well-established laboratory strain 168 with the ability to synthesize surfactin due to integration of a functional sfp gene, reached excellent values for YP/X with 1.541 g/g and these values surpassed aerobic results (Geissler et al., 2019b). Next to the foam-free environment that can be achieved employing anaerobic cultivations, another advantage is the more than hundred thousand times higher solubility of nitrate compared to oxygen in the medium. This allows for more flexibility in the design of batch and fed-batch processes. Furthermore, the development of nitrate respiration processes might generally be beneficial for products sensitive against oxidation. However, recent studies of both Willenbacher et al. (2015a) and Geissler et al. (2019b) have reported a much lower cell dry weight accompanied by overall inferior surfactin titers compared to aerobic counterparts. As a consequence, this study aimed at further evaluating the relevance of nitrite and ammonium as well as the impact of glucose concentrations for an envisioned foam-free anaerobic surfactin bioproduction process.
We hypothesized that the presence of both nitrite and ammonium has a negative impact on anaerobic nitrate respiration, while different initial glucose concentrations play a minor role. To further substantiate this hypothesis, reporter strains carrying PnarG-lacZ and PnasD-lacZ fusion were included to evaluate effects on the most important enzymes during anaerobic nitrate respiration.
Materials and Methods
Chemicals and Materials
All chemicals used were of analytical grade and were purchased from Carl Roth GmbH & Co., KG (Karlsruhe, Germany). The surfactin reference standard (≥98% purity) and glucose standard were obtained from Sigma-Aldrich Laborchemikalien GmbH (Seelze, Germany).
Microorganisms, Genetic Engineering and Strain Maintenance
All strains used within this study are listed in Table 1. Strain B. subtilis JABs24, constructed as described in Geissler et al. (2019b), is derived from the laboratory strain 168 with functional sfp and was used as initial strain for the construction of the reporter strains MG1 and MG5. The oligonucleotides (Eurofins Genomics Germany GmbH, Ebersberg, Germany) and plasmids used are listed in Supplementary Table S1 and Table 2, respectively. The promoter regions of narG and nasD were amplified through PCR (peqSTAR 96X, VWR GmbH, Darmstadt, Germany) using primers s1001/s1002, and s1011/s1012, respectively. The PCR products were purified (GeneMATRIX basic DNA purification Kit, EURx Sp. Z o.o, Gdańsk, Poland) and ligated into plasmid pJOE4786.1 (Altenbuchner et al., 1992) with T4 DNA ligase (New England BioLabs GmbH, Frankfurt am Main, Germany) after digestion with Sma I (New England BioLabs GmbH, Frankfurt am Main, Germany). The obtained plasmids, pKAM0182 for PnarG and pSHX1 for PnasD, were transformed into chemical competent E. coli JM109. Strains carrying the plasmid were selected on LB plates supplemented with ampicillin (100 μg/mL). Isolated plasmids pKAM0182 and pSHX1 (QIAamp DNA Mini Kit (50), QIAGEN GmbH, Hilden, Germany) were digested with Nde I and Age I (New England BioLabs GmbH, Frankfurt am Main, Germany) and fragments of interest were isolated (MinElute Gel Extraction Kit (50), QIAGEN GmbH, Hilden, Germany). Afterwards, digestion products were ligated into pKAM312 (Morabbi Heravi and Altenbuchner, 2018) resulting in pKAM452 and pSHX2 and transformed in competent E. coli JM109. Plasmids pKAM452 (PnarG-lacZ) and pSHX2 (PnasD-lacZ) were isolated and were transformed into natural competent B. subtilis JABs24. By double cross-over into the amyE gene, reconstructed strains were selected by agar plates supplemented with either spectinomycin (100 μg/mL) or erythromycin (10 μg/mL), and by starch plates dyed with Lugol’s iodine solution. Positive colonies were further checked by PCR using primers s7406 and s7409 to confirm insertion of PnarG-lacZ and PnasD-lacZ into amyE gene.
Media Composition
The LB medium used for the first pre-culture composed of 5 g/L tryptone, 10 g/L NaCl and 10 g/L yeast extract. An adapted medium based on the medium described by Willenbacher et al. (2015b) was used for all further pre-cultures and main cultures. The glucose concentration was 20 g/L for the second mineral salt pre-culture, 10 g/L and 2.5 g/L glucose for the batch and fed-batch bioreactor process, as well as either 2.5, 5, 7.5, or 10 g/L glucose for the serum flask cultivations as indicated in the respective results. The buffer composed of 0.03 mol/L KH2PO4 and 0.04 mol/L Na2HPO4 ⋅ 2 H2O in the mineral salt pre-culture and serum flask cultivations, and 5.71 ⋅ 10–3 mol/L KH2PO4 and 4.29 ⋅ 10–3 mol/L Na2HPO4 ⋅ 2 H2O in the bioreactor cultivations. The nitrogen source used for the mineral salt pre-culture was 0.1 mol/L NaNO3 and for the bioreactor and serum flask cultivation 0.1 mol/L NaNO3 and 5.0 ⋅ 10–4 mol/L (NH4)2SO4. In case of MgSO4 and trace element solution, which were prepared separately as autoclaved, respectively, filter sterilized stock solutions, all cultivations had the same final concentrations with 8.0 ⋅ 10–6 mol/L Na3-citrate, 7.0 ⋅ 10–6 mol/L CaCl2, 4.0 ⋅ 10–6 mol/L FeSO4 ⋅ 7 H2O, 1.0 ⋅ 10–6 mol/L MnSO4 ⋅ H2O, 4.0 ⋅ 10–6 mol/L Na2MoO4 ⋅ 2 H2O and 8.0 ⋅ 10–4 mol/L MgSO4 ⋅ 7 H2O. A 25% (w/w) autoclaved (121°C, 20 min) glucose solution was used for the bioreactor feed. For the cultivations investigating the effect of ammonium, the concentration of (NH4)2SO4 was adjusted. In case of nitrite and acetate experiments, the targeted concentrations were added from an autoclaved 0.1 mol/L NaNO2 or 277.88 g/L Na-acetate stock solution, respectively.
Cultivation Conditions
Pre-cultures were run at 120 rpm and 37°C in an incubator shaker (NewbrunswickTM/Innova 44, Eppendorf AG, Hamburg, Germany). A first overnight pre-culture was performed in a 100 mL baffled shake flask by inoculating 20 mL LB medium with 100 μL of the respective glycerol stock. This pre-culture was diluted 1:100 in mineral salt medium for a second pre-culture and incubated for 36 h. The shake flask size was adjusted to the amount of inoculation material needed and flasks were filled with 10–13% of the mineral salt medium.
Anaerobic Serum Flask Cultivation
Anaerobic serum flasks were prepared as described in Geissler et al. (2019b). Briefly, the buffer and nitrogen sources were autoclaved inside the flasks and all other solutions were added afterwards through a disinfected septum using a syringe. Anaerobic conditions were set by flushing the flasks with nitrogen and degassing through a filter element.
Anaerobic Bioreactor Cultivation
Bioreactor cultivations were performed in 42 L custom-built bioreactors (ZETA GmbH, Graz/Lieboch, Switzerland). The bioreactors are mounted on a scale and are equipped with pH (EasyFerm Bio HB Arc 120, Hamilton Bonaduz AG, Bonaduz, Switzerland) and pO2 (VisiFerm DO ARC 120 H0, Hamilton Bonaduz AG, Bonaduz, Switzerland) probes. Acid, base and feed solutions were on individual scales and added via peristaltic pumps. Bioreactors were equipped with three Rushton turbines and four baffle plates. The buffer and nitrogen source were autoclaved inside the bioreactor and the other components were added sterile through a septum. Prior to inoculation, the medium was flushed with N2 to ensure anaerobic conditions and pO2 measurement throughout cultivation confirmed absence of oxygen. Stirrer speed was kept at 120 rpm and pH 7 was maintained by adding either 1 mol/L NaOH or 1 mol/L H3PO4. Temperature was set to 37°C.
Sampling and Sample Analysis
For all cultivations performed, samples were taken in regular intervals. The OD600 was measured with a spectrophotometer (Biochrom WPA CO8000, Biochrom Ltd., Cambridge, United Kingdom). The cell dry weight was calculated by dividing the OD600 by the factor 3.762 which was determined previously (Geissler et al., 2019b). Prior to further analyses, samples were centrifuged for 10 min at 4°C and 4816 g (Heraeus X3R, Thermo Fisher Scientific GmbH, Braunschweig, Germany) and stored at −20°C until further processing.
Glucose was measured with a HPTLC system (CAMAG AG, Muttenz, Switzerland) as described in Geissler et al. (2019b). Briefly, the mobile phase used was acetonitrile/H2O (85:15, v/v) and plates were developed over a migration distance of 70 mm. After development, plates were derivatized with diphenylamine (DPA) reagent. DPA was prepared by diluting 2.4 g diphenylamine and 2.4 g aniline in 200 mL methanol and then adding 20 mL 85% phosphoric acid. After derivatization, plates were scanned at 620 nm and the glucose concentration was calculated in dependence of the standard curve.
Surfactin analysis was performed as described in Geissler et al. (2017) using a HPTLC method. Briefly, samples were extracted three times with an equal volume of chloroform:methanol 2:1 (v/v). The pooled solvent layers were evaporated and the crude surfactin was resuspended in methanol to match the initial sample volume. Plates were developed using the mobile phase chloroform:methanol:water 65:25:4 (v/v/v) over a migration distance of 60 mm. After development, the plates were scanned at 195 nm and evaluation was performed by peak area in correspondence to a standard curve.
Spectrophotometric assays (Merck KGaA, Darmstadt, Germany) were used to measure nitrate (Cat. No. 1.09713.0001), nitrite (Cat. No. 1.14776.0001) and ammonium (Cat. No. 1.14752.0001) concentrations.
Acetate concentration was determined with an enzymatic kit (Cat. No. 10148261035, r-biopharm AG, Pfungstadt, Germany).
For ß-galactosidase assay, a volume of 100 μL of cell suspension from strain MG1 or MG5 was mixed with 900 μL Z-Buffer (0.06 mol/L Na2HPO4, 0.04 mol/L NaH2PO4, 0.01 mol/L KCl, 1 mmol/L MgSO4 ⋅ 7 H2O, 0.04 mol/L mercaptoethanol). After addition of 10 μL toluol, the mixture was incubated for 30 min at 37°C and 750 rpm. 200 μL of 20 mmol/L ortho-nitrophenylgalactopyranoside was added and the reaction was stopped when the solution turned yellow by adding 500 μL of 1 mol/L Na2CO3. Samples were centrifuged for 2 min at 19283 g and 250 μL were transferred to a microtiter plate. Absorbance was measured at both 420 nm and 550 nm and the Miller Units (MU) were calculated according to the following equation:
Data Analysis
For the bioreactor cultivations performed, as well as for serum flask cultivations if required, the biomass yield per substrate YX/S [g/g], product yield per substrate YP/S [g/g], the product yield per biomass YP/X [g/g], the specific surfactin productivity q [g/(g⋅h)] and the specific growth rate μ [1/h] were determined. The respective equations are listed in the Supplementary Material (S2). Depending on the evaluation, either surfactin or acetate was considered as product P. These parameters were calculated in two distinct approaches. Maximum yields were determined by calculating the respective parameter in between sampling points and overall yields were calculated based on the data of inoculation and at CDWmax of the process. For the bioreactor cultivation employing a feed, the glucose fed at sampling was added to the respective time point.
Results and Discussion
Influence of Different Ammonium Concentrations on Anaerobic Growth and Effect on Promoter Activity of PnarG and PnasD
Under aerobic conditions, ammonium is the preferred nitrogen source and in the presence of both ammonium and nitrate, nitrate consumption is induced after depletion of ammonium (Davis et al., 1999). During anaerobic nitrate respiration, however, nitrate is used as alternative electron acceptor and is thereby reduced to nitrite which is further reduced to ammonium by the enzymes nitrate reductase NarGHI and nitrite reductase NasDE, respectively (Hoffmann et al., 1998; Nakano et al., 1998a; Härtig and Jahn, 2012). As these enzymes are crucial for anaerobic nitrate respiration, the corresponding gene expressions were monitored by the respective promoters PnarG and PnasD. As the concentration of ammonium is expected to increase during cultivation due to nitrate reduction, it was hypothesized that the increase in ammonium might have a negative impact on both enzyme activity as well as gene expression, the latter one being studied using the reporter strains. In addition, a low initial ammonium concentration was hypothesized to be sufficient as ample pool for the incorporation of ammonium into biomass until ammonium is provided by nitrate respiration. In this sense, the influence of different ammonium concentrations on the growth of B. subtilis under anaerobic conditions was examined. In a first screening, B. subtilis JABs24 was cultivated in duplicate employing 2.5 g/L glucose with five various ammonium concentrations ranging from 0.001 mol/L NH4+ up to 0.2 mol/L NH4+. The overall growth rates μ were in the range of 0.068 ± 0.006 1/h. Also with respect to the final CDW, which was in the range of 0.194 ± 0.015 g/L, and the time to reach CDWmax, an influence of the ammonium concentration was not observed indicating that a high initial ammonium concentration as well as the increase in ammonium due to nitrate respiration did not negatively influence anaerobic growth when employing 2.5 g/L glucose. However, as reported by Willenbacher et al. (2015a), an increase in the initial glucose concentration from 7.5 g/L to 10 g/L resulted in a lower CDW and YX/S employing strain B. subtilis DSM 10T. The initial NH4+ concentration in this study was 0.1 mol/L. Assuming a complete conversion from nitrate to ammonium, final concentrations were about 0.16 and 0.14 mol/L NH4+ for 7.5 and 10 g/L glucose, respectively. In this sense, further cultivations employing the reporter strains B. subtilis MG1 (PnarG-lacZ) and MG5 (PnasD-lacZ) with both 7.5 and 10 g/L glucose, as well as 0.001 mol/L, 0.1 mol/L, and 0.2 mol/L NH4+ were further used to examine the combinative effect of different ammonium and glucose concentrations on anaerobic growth by nitrate respiration.
Figure 1 displays an exemplary cultivation plot of strain MG1 employing 10 g/L glucose and 0.2 mol/L NH4+. The biomass increased up to 0.5 g/L after 70.5 h of cultivation. Glucose was almost depleted when CDWmax was reached and hence the drop in CDWmax was caused by glucose depletion. About 70 mmol/L NO3– was reduced and ammonium increased by ∼60 mmol/L. Nitrite peaked at the beginning of cultivation to 0.558 mmol/L and was further reduced to 0.007 mmol/L at CDWmax. Another increase was measured when CDW decreased. This nitrite pattern was observed in almost all cultivations tested. The activity of PnarG increased up to 119 MU after 32.5 h of cultivation and slightly decreased to 76 MU until CDWmax. For a better evaluation, the results of the different ammonium and glucose concentrations tested are summarized in Table 3.
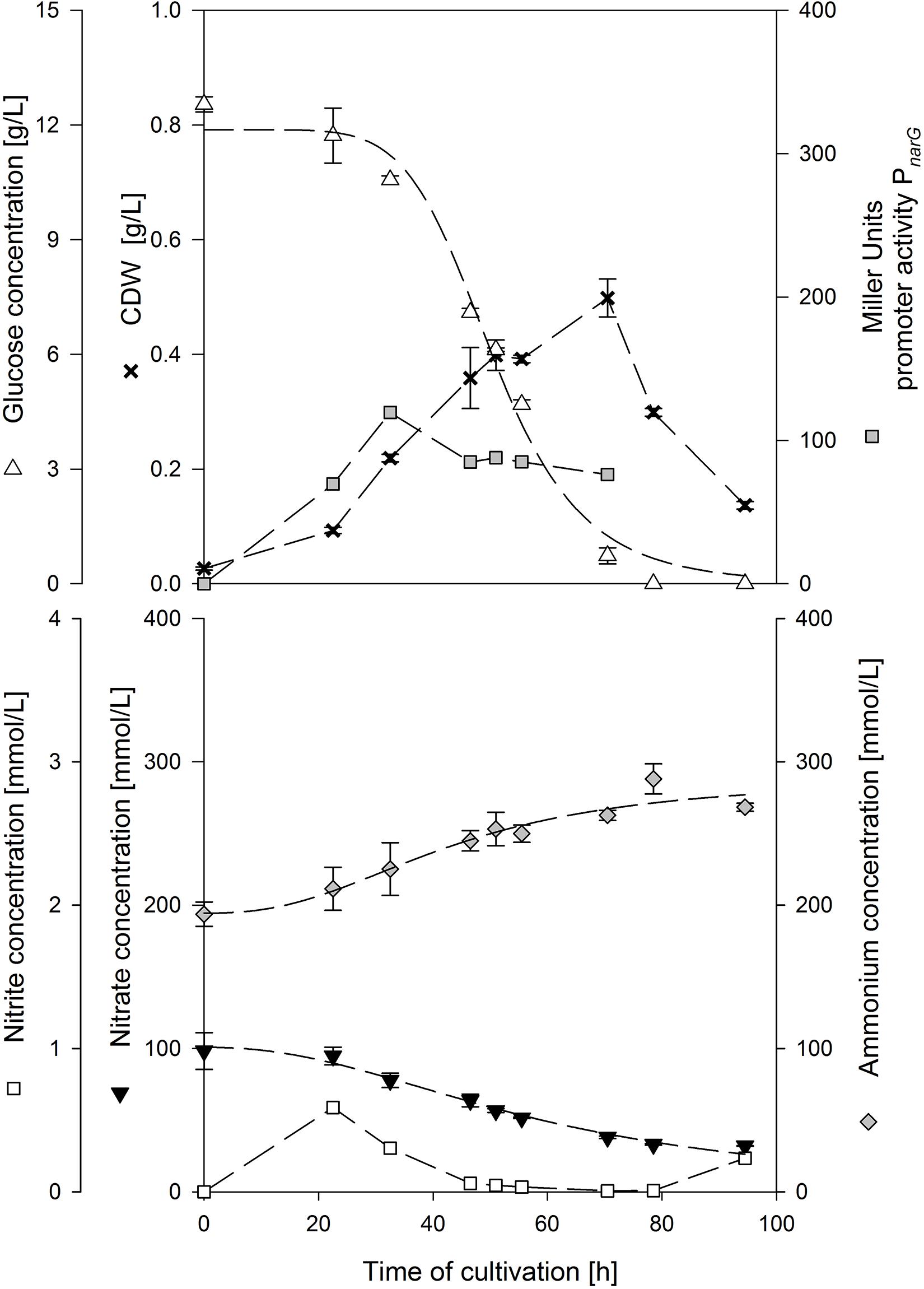
Figure 1. Exemplary anaerobic serum flask cultivation employing strain B. subtilis MG1 in a mineral salt medium containing 10 g/L glucose, 0.1 mol/L NO3– and 0.2 mol/L NH4+.
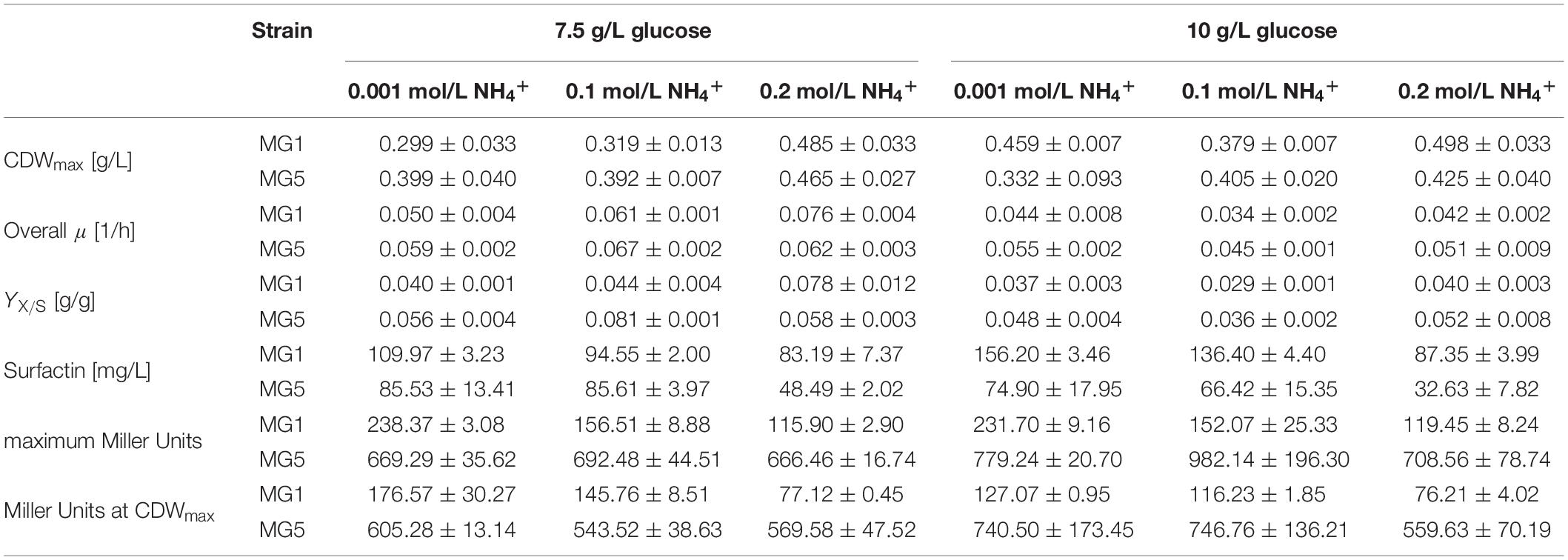
Table 3. Summary of the results and calculated yields for the serum flask cultivations of strains B. subtilis MG1 (PnarG-lacZ) and MG5 (PnasD-lacZ) employing 7.5 g/L and 10 g/L glucose and different ammonium concentrations.
A higher glucose concentration and especially the presence of 0.2 mol/L NH4+ within the same glucose level resulted in a higher CDWmax. Discrepancies, however, must be considered as the determined CDWmax in several cultivations was prior to glucose depletion or even slightly after glucose depletion due to the time intervals of sampling. A CDWmax of 0.388 g/L was reported by Geissler et al. (2019b) employing strain B. subtilis JABs24 using 10 g/L glucose, 0.1 mol/L NO3– and 0.025 mol/L NH4+. This value is in a similar range in comparison to this study. For strain MG1 with 7.5 g/L, both the YX/S and the overall growth rate μ increased when more ammonium was added to the medium. For all other cultivations, this trend for both the overall growth rate μ and the YX/S was not observed. Hence, no generally admitted influence of ammonium could be observed at these experimental conditions, which is also in agreement to the results employing 2.5 g/L glucose. Under aerobic conditions, for example, Leejeerajumnean et al. (2000) reported that 26 tested Bacillus strains, among them strain B. subtilis NCIMB 3610, grew in the presence of 931 mmol/L NH4+ at pH 7. Müller et al. (2006) described that 500 mmol/L did not cause growth inhibition using strain B. subtilis 168. A defect was observed with more than 750 mmol/L NH4+, but the authors stated that this is due to osmotic or ionic stress and not due to the presence of ammonium itself. However, no reports on the effect of ammonium under anaerobic conditions were found.
Interestingly, the surfactin concentration was lower the more ammonium was present at the beginning of cultivation, with a more drastic change in between 0.1 mol/L and 0.2 mol/L NH4+. Exemplary, employing 10 g/L glucose and 0.001 mol/L NH4+ resulted in a surfactin concentration of 156.20 mg/L, while the titer obtained with 0.2 mol/L NH4+ was only 87.35 mg/L for strain MG1. On the contrary, when the ratio of NO3–:NH4+ was shifted towards higher nitrate concentrations in studies on the surfactin synthesis under aerobic conditions, a decrease in biomass and surfactin concentration, but an increase in the YP/X was observed. For example, media containing only NH4+ or NO3– resulted in a surfactin titer of ∼1 g/L and ∼0.35 g/L at an CDWmax of 2 g/L after 21 h and of 0.5 g/L after 36 h of cultivation with strain B. subtilis JABs24, respectively. This results in YP/X values of 0.466 g/g and 0.668 g/g (data not shown). On the contrary, Davis et al. (1999) reported on an improvement in the YP/X when cultivating B. subtilis ATCC 21332 in a nitrate-limited oxygen-depleted process. Consequently, further medium optimization studies on the impact of different nitrate concentrations at constant ammonium levels on surfactin synthesis are a crucial approach also to investigate the impact on both the dissimilatory and assimilatory nitrogen metabolism.
With respect to the promoter activities, averaged maximum Miller Units for PnarG were overall lower with ∼170 MU than for PnasD with ∼750 MU. During the time course of cultivation, as illustrated in Supplementary Figure S3 and summarized in Table 3, the promoter activity decreased after reaching the maximum until CDWmax. For PnarG, both the maximum promoter activity as well as the activity at CDWmax was lower the more ammonium was present. Differences amongst the glucose concentrations tested were less distinct. Hence, the impact of glucose was less significant than the ammonium concentration and lower ammonium concentrations yielded a higher PnarG activity. The activity of PnasD was overall much higher with Miller Units up to 1000, but the pattern was similar to PnarG and activity reached a maximum and further declined until CDWmax. For the PnasD activity, values at 10 g/L glucose were overall higher in comparison to the respective data at 7.5 g/L glucose. However, highest values were obtained for 0.1 mol/L NH4+. Hence, a trend was not observed regarding the influence of increasing ammonium concentrations. This is in agreement to the results of Nakano et al. (1998a) reporting that the presence of ammonium did not alter the anaerobic expression levels of a transcriptional fusion nasD-lacZ strain.
Generally, in the current experimental set-up, the increase of ammonium showed a tendency to an overall improvement with respect to CDWmax while a distinct trend for growth rate μ, YX/S and promoter activity PnasD was not observed. However, a decrease in both PnarG activity and surfactin synthesis was noticed. Due to this ambiguous effect of ammonium on growth but the negative effect on surfactin synthesis and PnarG activity, an initial ammonium concentration of 0.001 mol/L was used for all further experiments, as surfactin is the product of interest. In addition, as a further increase in CDW during anaerobic growth beyond 10 g/L glucose will result in a further accumulation of ammonium, the reduction to a minimum from the beginning is expected to be more profitable at long-term view.
Influence of Different Nitrite Concentrations on Anaerobic Growth
As previously described and illustrated in Figure 1, nitrite concentrations peaked shortly after inoculation and decreased during the time course of further cultivation. On the one hand, nitrite is often stated to be toxic and hence cells need to detoxify accumulated nitrite, on the other hand, the reduction to ammonium by nitrite reductase is an electron sink which allows the reoxidation of NADH to NAD+ (Cruz Ramos et al., 1995; Nakano et al., 1998a; Reents et al., 2006). NAD+ itself is needed for glycolysis, oxidative decarboxylation and the citric acid cycle. Inversely, in the absence of nitrate or nitrite as electron acceptor, B. subtilis growth by fermentation and NAD+ would be regenerated through end product phosphorylation, with the main fermentative metabolites produced being lactate, acetate and 2,3-butandiol (Cruz Ramos et al., 2000).
To further elucidate the impact of nitrite on anaerobic growth, the influence of various concentrations in the range from 0 mmol/L up to 20 mmol/L on the growth behavior of strain JABs24 employing 2.5 g/L glucose was investigated. In the time frame cultivated, the overall growth rate was reduced which was basically due to an increase in lag-phase. For 8 mmol/L and 6 mmol/L NO2–, strains restored growth after a lag-phase of around 30 h. With lower nitrite concentrations of 4, 2.5, and 1 mmol/L, growth was detected after a lag-phase of 26, 24, and 12 h of cultivation, respectively. These results are further affirmed by the overall growth rate and maximum growth rate, illustrated in Figure 2. A decrease in the overall growth rate μ from 0.068 1/h to 0.027 1/h was observed for 0 mmol/L and 10 mmol/L NO2– added in the time window tested. For the maximum growth rates, mean values varied in between 0.141 1/h and 0.208 1/h. However, higher nitrite concentrations did not result in lower growth rates. This is also in agreement to literature, where anaerobic growth of different B. subtilis strains employing either 10 mmol/L nitrate or nitrite resulted in the same OD-values indicating the suitability of nitrite as alternative electron acceptor (Hoffmann et al., 1998; Cruz Ramos et al., 2000; Marino et al., 2001). Interestingly, authors did not report on an increased lag-phase as observed in this study. For 20 mmol/L NO2– and 2.5 g/L glucose, growth was not detected during the current experiment. To further investigate the effect of high nitrite concentrations and a longer time frame, a further cultivation employing 10 g/L glucose with both 10 and 20 mmol/L NO2– was performed. For 20 mmol/L, growth was not detected even after 90 h of cultivation. For 10 mmol/L NO2–, CDW values up to 0.5 g/L were measured after ∼115 h. Compared to the cultivations employing different ammonium concentrations, the time to reach CDWmax was hence almost twice as long. However, the final CDW was comparably high and the concentration of nitrite was also drastically reduced until CDWmax was reached (data not shown).
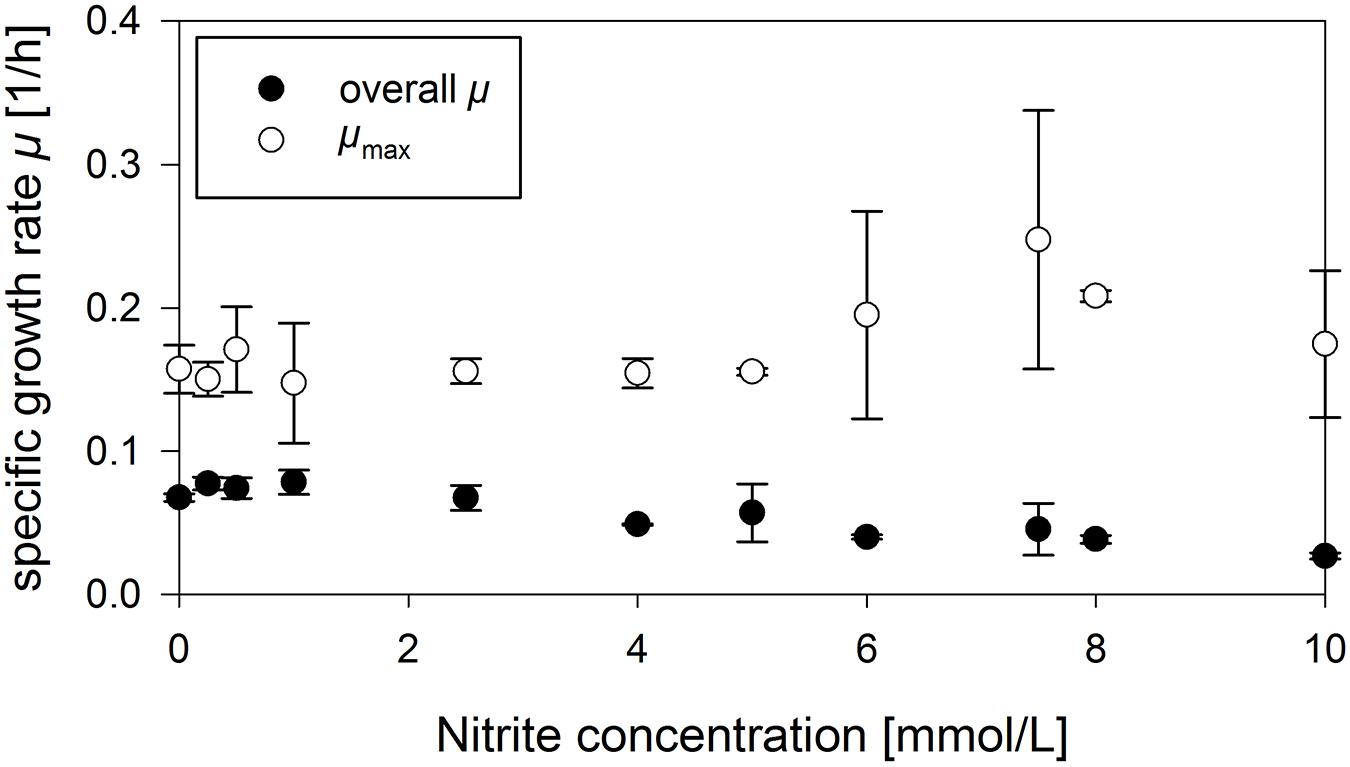
Figure 2. Influence of different nitrite concentrations on the overall and maximum specific growth rate μ in anaerobic serum flask cultivations employing strain B. subtilis JABs24.
To sum up the effect of nitrite, only at very high levels, nitrite is indeed growth limiting or even inhibiting. Concentrations up to 10 mmol/L did significantly increase the time of cultivation employing strain B. subtilis JABs24, but the maximum growth rates μ as well as CDWmax prior to glucose depletion were similar to the references without further additives and nitrite was also reduced to ammonium.
Impact of Various Glucose Concentrations on Anaerobic Growth and Effect on Promoter Activity of PnarG and PnasD
During the study of Willenbacher et al. (2015a) using strain B. subtilis DSM 10T, a benefit of employing 10 g/L glucose with respect to higher CDW and surfactin concentrations was not given. Willenbacher et al. (2015a) stated that a concentration of 10 g/L glucose leads to overflow metabolism in B. subtilis. However, no data regarding this hypothesis, such as acetate concentrations, were shown. The previous results of cultivations with strain B. subtilis JABs24 and various ammonium concentrations indicated that the effect of 7.5 g/L and 10 g/L is less severe than reported by Willenbacher et al. (2015a) considering the CDWmax, but an increase in glucose indeed decreased overall growth rates μ and resulted in lower YX/S values (Table 3). As the glucose concentration plays a major role in the design of batch and fed-batch processes, cultivations with 2.5, 5, 7.5, and 10 g/L glucose were performed in serum flasks employing the strains B. subtilis JABs24, MG1 and MG5 as one triplicate set-up.
Figure 3A illustrates the change in CDWmax, YX/S as well as μmax and overall μ of the serum flask cultivations employing different glucose concentrations. CDWmax increased by employing higher glucose concentrations from 0.239 ± 0.011 g/L up to 0.492 ± 0.011 g/L. In addition, the time to reach CDWmax increased from 30 h to 69 h the higher the glucose concentration was. The observation from Willenbacher et al. (2015a) could consequently not be confirmed and higher cell densities were actually reached the more glucose was added. However, it must be emphasized that different strains were used and in addition, even a frequent sampling does not assure to measure the CDW when glucose is about to be depleted, which was also reported by Geissler et al. (2019b). For the overall growth rate μ, a decrease from 0.072 ± 0.002 1/h to 0.041 ± 0.000 1/h was observed with increasing glucose concentration. In contrast, the differences in μmax and the YX/S were less distinct. For μmax, values ranged from 0.098 ± 0.004 1/h to 0.133 ± 0.001 1/h, and for the overall YX/S between 0.044 ± 0.002 g/g and 0.070 ± 0.005 g/g.
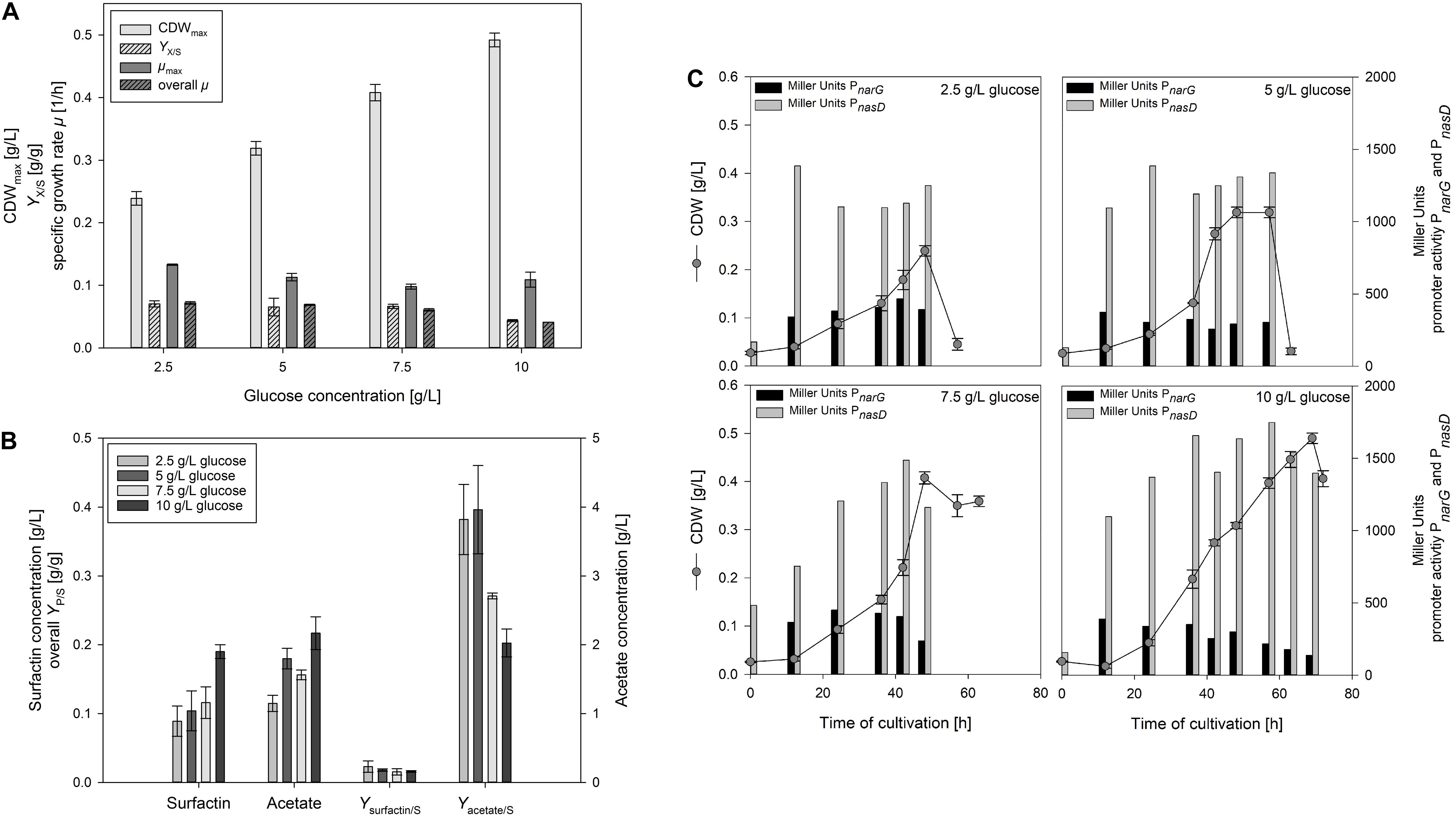
Figure 3. Influence of different glucose concentrations in anaerobic B. subtilis JABs24, MG1 and MG5 serum flask cultivations on (A) CDWmax, biomass per substrate yield YX/S, specific maximum and overall growth rate μ and (B) surfactin and acetate concentrations at CDWmax, surfactin per substrate yield Ysurfactin/S and acetate per substrate yield Yacetate/S. In addition, (C) CDW and corresponding Miller Units measured for expression of promoters PnarG and PnasD during the time course of cultivation.
Figure 3B displays the surfactin and acetate concentrations at CDWmax as well as the corresponding overall YP/S. The surfactin concentration employing 10 g/L glucose was ∼2-fold higher with 189.72 ± 10.50 mg/L than at the other concentrations tested. On the contrary, maximum surfactin titers at the other glucose levels tested were in a similar range and only a slight trend towards an increase in surfactin at higher glucose levels was monitored. The Ysurfactin/S showed a similar trend than the YX/S and decreased with increasing glucose concentrations from 0.023 ± 0.008 g/g to 0.016 ± 0.001 g/g. As reported by Willenbacher et al. (2015a), authors assumed that overflow metabolism led to their results employing 10 g/L glucose. In the current study, acetate concentrations were measured for the samples at CDWmax. Acetate is reported to be the most abundant end product during anaerobic growth of B. subtilis (Cruz Ramos et al., 2000). The acetate concentrations produced during anaerobic growth increased from 1.15 ± 0.12 g/L to 2.17 ± 0.24 g/L with increasing glucose concentrations. The Yacetate/S was more than 10-fold higher than the Ysurfactin/S and decreased as well with increasing glucose concentration from 0.382 ± 0.051 g/g to 0.202 ± 0.020 g/g. This result is, however, rather counter intuitive, as higher glucose concentrations actually do not result in more acetate per glucose. Although further fermentative end products were not expected as nitrate respiration is reported to suppress fermentative growth (Cruz Ramos et al., 2000), lactate was measured as well. As assumed, at CDWmax employing 10 g/L glucose the lactate concentration reached 0.24 ± 0.01 g/L, and 0.16 ± 0.01 g/L employing 7.5 g/L glucose. This indicates that the glucose flux, in contrast to acetate, into lactate is comparably low, but that a high amount of glucose converts into acetate and not to the target product. Nakano et al. (1998b) reported that several enzymes of the citric acid cycle show a reduced activity anaerobically and authors assumed that citrate deficiency might cause citZ repression. In this sense, carbon flux studies to investigate glucose degradation are another interesting option for future studies.
Similarly to the previous cultivations, the effect of glucose on anaerobic nitrate respiration was also investigated by the inclusion of the reporter strains MG1 and MG5. Figure 3C illustrates the growth curves as well as the corresponding measured promoter activities for both PnarG and PnasD. For PnarG, the activity at CDWmax was lower with increasing glucose concentrations and decreased from 392 MU to 137 MU. However, MU values in the early stages of cultivation also reached highest MU values in between 370 – 440 MU. Hence, the promoter activity of the nitrate reductase was lower the longer the cultivation lasted. In combination with the previous results (Table 3), both high glucose as well as high ammonium concentrations decreased the PnarG activity, but further studies have to address the question if actually the decreasing concentration of nitrate has an impact on PnarG activities. In accordance to the previous results, the activity of PnasD was much higher with MU values up to ∼1700 MU in comparison to PnarG. The promoter activity for PnasD also reached a maximum prior to CDWmax and a slight trend was observed with an increase in activity at higher glucose levels. In addition, the decrease in activity for PnarG was observed earlier during cultivation, while the maximum activity of PnasD was achieved later. This would also explain the nitrite peak observed and is in agreement to the results of Nakano et al. (1998a), who reported that the presence of nitrite along with the global regulator ResDE stimulates the expression of nasD. In this sense, nitrate first has to be reduced, and the nitrite produced stimulates nasD expression.
To sum up, the serum flask cultivations illustrated that the growth rate was reduced with increasing glucose concentration and that the promoter activity of PnarG declined as well during the time course of cultivation. However, the experiments have also demonstrated that the cell density increased the more glucose was added. In addition, results revealed that a high amount of glucose is converted into acetate, while lactate can be considered as a neglectable metabolite in this experimental set-up. Furthermore, acetate production was not lower with less glucose present in the medium. The synthesis of acetate might also be needed to generate ATP, although nitrate respiration is the most efficient alternative respiratory mechanism compared to aerobic cultivations with oxygen as electron acceptor (Strohm et al., 2007).
Batch vs. Fed-Batch Bioreactor Cultivation
To further evaluate the impact of various glucose concentrations, the aim was to perform a batch bioreactor cultivation with strain B. subtilis JABs24 employing 10 g/L glucose and a fed-batch cultivation with an initial glucose concentration of 2.5 g/L glucose. A diagrammatic representation of the processes performed as well as the advantage over aerobic batch cultivations is given in Figure 4A. For the fed-batch process, an exponential feed phase which matches the glucose added in the batch cultivation was performed to evaluate the impact of a constantly low glucose concentration on growth, surfactin and acetate production.
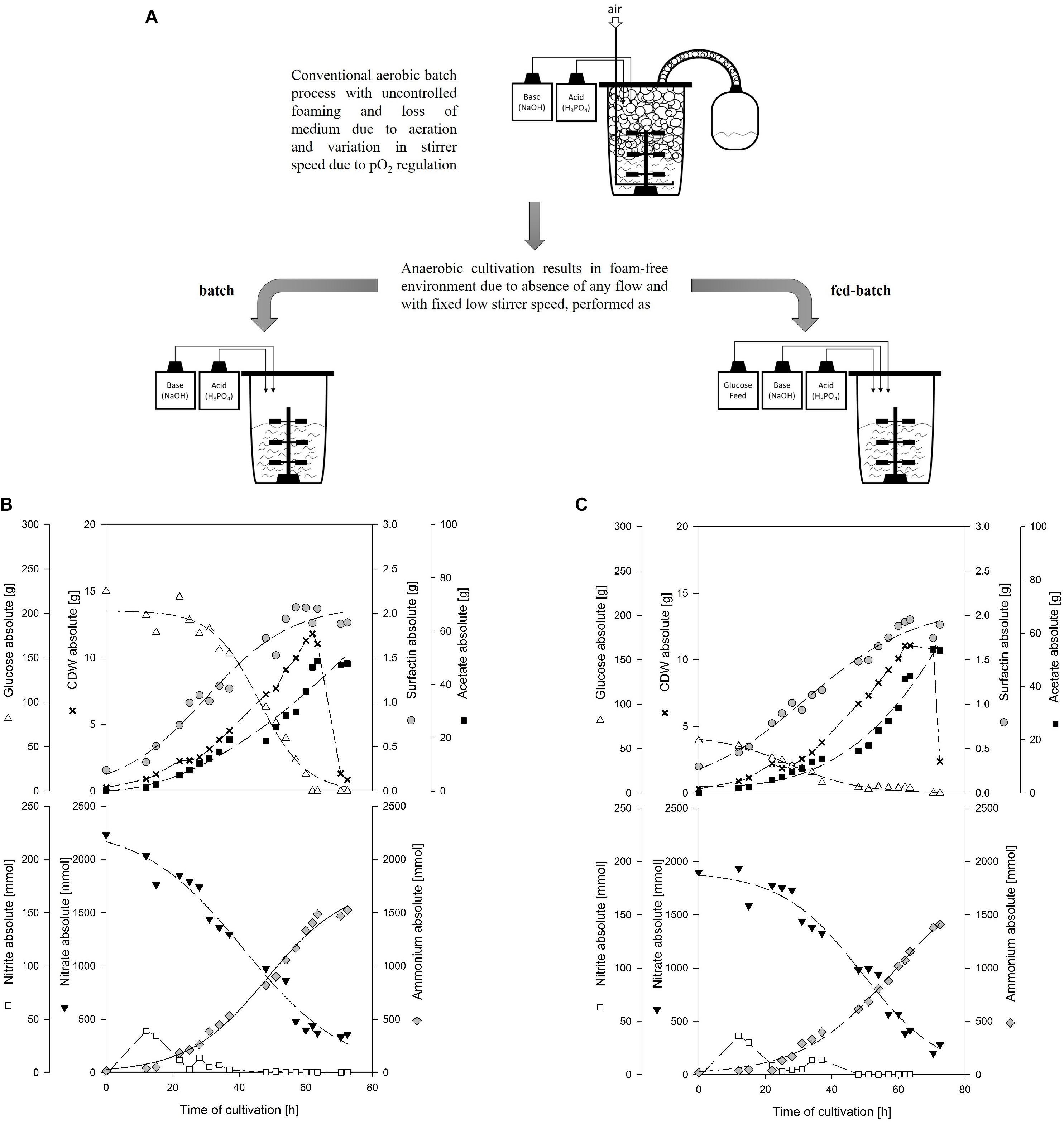
Figure 4. Diagrammatic representation of conventional aerobic batch process with foaming and anaerobic foam-free batch and fed-batch process (A). Time course of 20 kg anaerobic bioreactor cultivation with strain B. subtilis JABs24 in a medium containing 0.1 mol/L NO3– and 0.001 mol/L NH4+ performed as (B) batch with 10 g/L glucose and (C) fed-batch with 2.5 g/L glucose employing a feed with 600 g of a 25% glucose solution.
Figure 4B (batch) and Figure 4C (fed-batch) illustrate the absolute values for cell dry weight, glucose, surfactin and acetate, as well as the absolute values of the anaerobic respiration metabolites nitrate, nitrite and ammonium for the two process strategies applied. All important process results as well as calculated yields are furthermore summarized in Table 4. For comparison, results of the reference serum flask cultivation employing 10 g/L glucose and yields of further non-conventional cultivation strategies to produce surfactin reported in literature are given as well.
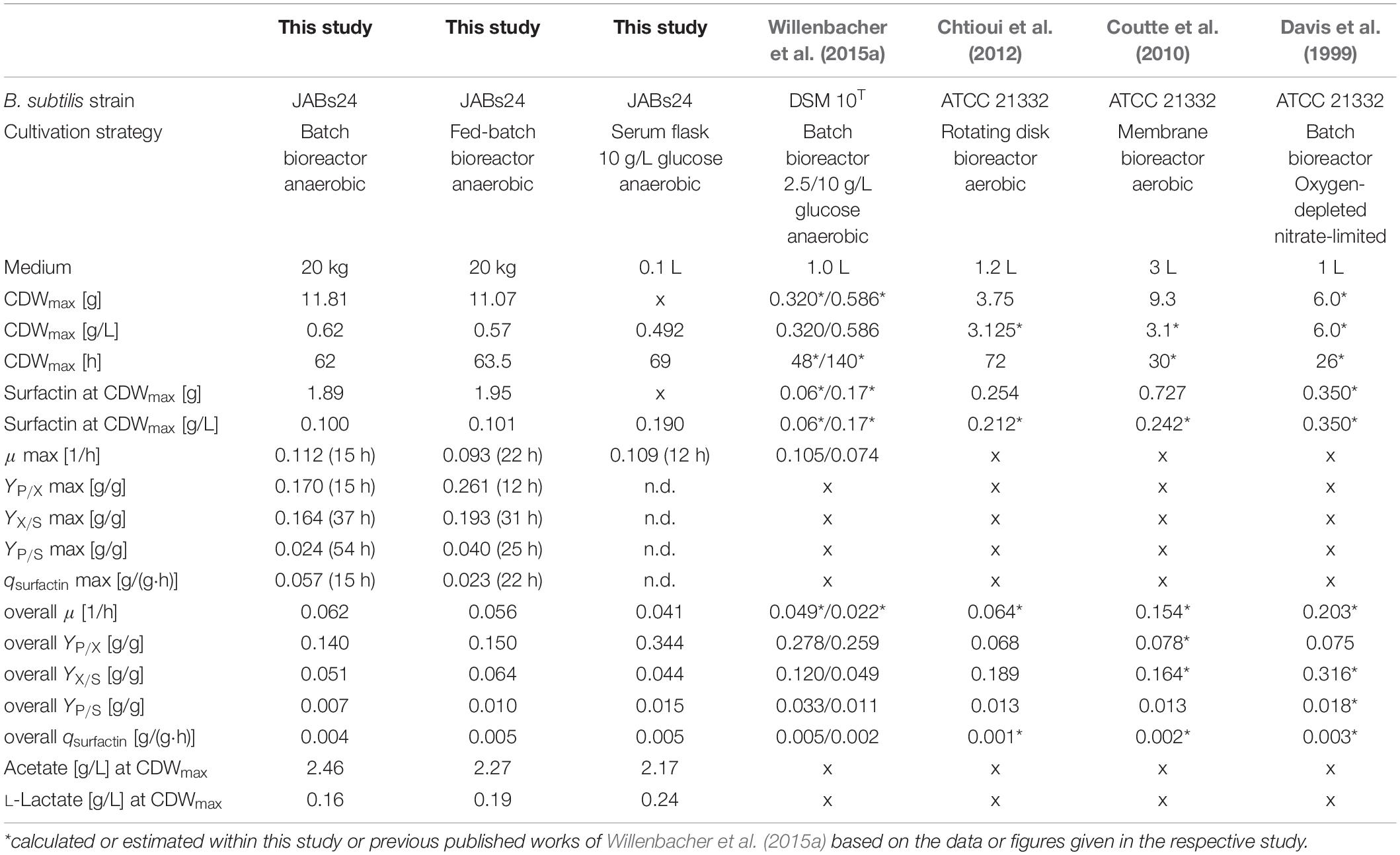
Table 4. Summary of the results and calculated yields for the batch and fed-batch bioreactor process in comparison to a reference serum flask cultivation, as well as comparison to process parameters and yields obtained in different cultivation strategies reported.
The glucose concentration in the batch process was depleted after 62 h of cultivation and the CDWmax was reached at this time with 11.81 g (OD600 of 2.35). For the fed-batch process, the feed was started after 37 h of cultivation with a set growth rate of 0.04 1/h and an initial feed rate of 0.01 kg/h. This growth rate was chosen based on the results of the serum flask cultivations and was expected to not cause glucose accumulation during the feed phase. The glucose concentration at feed start was 0.61 g/L and maintained in a range of 0.31 ± 0.04 g/L during the feed phase. The CDWmax before glucose consumption was 11.07 g (OD600 of 2.15). For both cultivations and similar to the serum flask cultivations, cell density dropped after glucose depletion. According to Espinosa-de-los-Monteros et al. (2001) cell lysis occurred after the growth phase instead of sporulation. The surfactin concentration in both cultivations increased throughout cultivation and at CDWmax, absolute values of 1.89 g and 1.95 g were reached. Consequently, both process strategies resulted in comparable surfactin titers and glucose limitation neither improved nor impaired surfactin productivity.
Analysis of nitrate revealed that there was no limitation and the reduction of nitrate as well as the increase in ammonium until the end of cultivation indicated that nitrate respiration occurred until growth stopped. At CDWmax, 439.41 mmol and 417.30 mmol NO3– were present in the medium for the batch and fed-batch process, respectively. Considering the results of both bioreactor and the serum flask cultivations, the nitrate demand for anaerobic nitrate respiration can be calculated as ∼150 mmol (NO3–)/g (CDW). Ammonium increased constantly up to 1403.49 and 1153.00 mmol until CDWmax was reached. For both cultivations, nitrite peaked at the beginning of cultivation and a second low nitrite peak was observed which actually occurred in a phase with reduced growth. After 48 h of cultivation nitrite was below 1 mmol. The highest concentration of nitrite was measured for both experiments after 12 h of cultivation with 39.13 mmol for the batch and 36.41 mmol for the fed-batch process. These observations match the data obtained from the serum flask cultivations where a nitrite peak was observed as well.
With respect to acetate, both processes showed a drastic increase in acetate with more than 40 g at CDWmax. The acetate concentration increased almost parallel to the biomass and a significant difference in between the two process strategies was not observed. The overall Yacetate/X and the Yacetate/S for both batch and fed-batch process were in a similar range at CDWmax and reached 3.920 g/g and 0.206 g/g for the batch, and 3.961 g/g and 0.260 g/g for the fed-batch cultivation, respectively. The results obtained are also in agreement with the serum flask cultivations and illustrate that a lower glucose concentration even below 0.31 g/L throughout cultivation did not result in lower acetate production.
In comparison to acetate, lactate was produced as minor by-product in both cultivations with less than 4 g at CDWmax. In a study performed by Espinosa-de-los-Monteros et al. (2001), authors reported that acetic acid and acetoin accumulated in cultivations with excess nitrate, whereas lactate and butanediol were produced when nitrate became limiting due to the presence of excess reduced cofactors. This would be in accordance to the current study, as nitrate decreased from 0.1 mol/L to ∼0.02 mol/L, but further investigations regarding these findings are necessary. Contrariwise, Cruz Ramos et al. (2000) reported a production of 23.3 mmol/L lactate and 16.4 mmol/L acetate cultivating strain B. subtilis 168 with nitrate as electron acceptor. However, they reported that the presence of nitrate reduced the formation of lactate and increased the production of acetate. In comparison to the current study, 50 mmol/L glucose and 50 mmol/L pyruvate were used as carbon source, which makes a comparison difficult, as the influence of pyruvate is not known. In addition, only 10 mmol/L NO3– were used. This, based on the results of the current study, is not sufficient to ensure nitrate respiration throughout the cultivation in the presence of these amounts of carbon source. Consequently, it might be that the cultivation switched from nitrate respiration to fermentative growth and by that lactate was produced. This would also be in agreement with another statement made by Cruz Ramos et al. (2000) namely that the presence of nitrate actually represses the transcription of lactate dehydrogenase ldh and acetolactate synthase alsS genes.
Regarding the yields and process parameters, the fed-batch process reached higher maximum and overall yields YP/X, YX/S and YP/S. This is in agreement with the results of different glucose concentrations tested, as lower glucose levels led to an improved YX/S and YP/S and this is hence also valid for a feed phase. However, the employment of a fed-batch process did result neither in a better biomass or surfactin production, nor in a significantly lower acetate or lactate formation. Interestingly, in comparison to the serum flask cultivation, the surfactin concentration in both bioreactor cultivations reached only 0.100 g/L and 0.101 g/L, while 0.190 g/L surfactin was produced in the serum flask with less biomass. This is also illustrated by the YP/X, which is more than double as high with 0.344 g/g in the serum flask. This observation is also in agreement with the YX/S, indicating a better glucose conversion into biomass in the bioreactor cultivation in comparison to the serum flask, while the YP/S is superior in the serum flask cultivations.
To conclude, the fed-batch and batch cultivations showed that cell growth was observed as long as glucose was present in the medium, illustrating the general feasibility of anaerobic cultivations with strain B. subtilis JABs24 for the production of surfactin. Obviously, the next step in bioreactor process development would be to elongate the feed phase. In addition, neither process showed a significant superiority indicating that the initial glucose does not influence the overall performance of the cultivation. However, acetate production was also not reduced at lower glucose levels which makes this metabolite another interesting candidate for further investigations regarding its impact on anaerobic growth.
Impact of Various Acetate Concentrations on Anaerobic Growth and Effect on Promoter Activity of PnarG and PnasD
During the previous cultivations, nitrite was shown to be reduced during the time course of cultivation even at an initial concentration up to 10 mmol/L, while acetate was detected in high amounts and the concentration curve was almost parallel to the growth curve with final acetate concentrations up to 2.5 g/L. Under aerobic conditions, the production of acetate is often correlated to overflow metabolism (Presecan-Siedel et al., 1999; Kabisch et al., 2013). In addition, B. subtilis is not able to grow on acetate aerobically due to the absence of genes of the glyoxylate shunt (Kabisch et al., 2013). However, under anaerobic fermentative conditions, acetate synthesis is important for generation of energy because acetate synthesis goes along with the AckA dependent formation of ATP (Cruz Ramos et al., 2000). Under anaerobic respiratory conditions, no distinct hypothesis was found that explains the acetate formation under nitrate respiratory conditions. The utilization of nitrate as alternative electron acceptor is reported to be the most favorable in view of ATP yield (Marino et al., 2001). Nevertheless, acetate is also often declared as growth inhibiting substance, but the effect varies in between different bacterial species as demonstrated by Lasko et al. (2000) testing E. coli, Acetobacter aceti, Staphylococcus capitis, Gluconobacter suboxydans, Lactobacillus acetotolerans, and L. bulgaricus in aerobic cultures. In another study, addition of 128 mmol/L acetate resulted in a reduced growth rate from 0.75 1/h to 0.4 1/h in an E. coli culture at pH 7.4 (Pinhal et al., 2019). However, little information is available on the effect of acetate on B. subtilis. Studies dealing with acetate and declaring growth inhibiting effect mostly refer to experiments with E. coli.
In contrast to nitrite, the concentration of acetate was increasing steadily. Hence, serum flask cultivations were performed with various initial acetate concentrations. Other than for nitrite, cultivations were performed directly with 10 g/L glucose to monitor effects in longer cultivations. The cultivation results with strains JABs24, MG1 and MG5 as triplicate are given in Table 5. The maximum CDWs were in a similar range and varied in between 0.465 and 0.532 g/L and no correlation was found between initial acetate concentration and CDWmax. The time to reach CDWmax was ∼69 h for the reference and ∼105 h for 10 g/L acetate added. In this sense, acetate has an influence comparable to that of nitrite with respect to the time of cultivation to reach CDWmax. However, while μmax for nitrite was in an overall similar range (Figure 2), μmax for acetate was lower the more acetate was added. This indicates that acetate did not prolong the lag-phase such as demonstrated for nitrite but has an overall negative effect on cellular growth. Furthermore, the overall growth rate μ was reduced from 0.041 ± 0.000 1/h to 0.029 ± 0.001 1/h applying 0 g/L and 10 g/L acetate, respectively. Due to the similar final CDW values obtained, the YX/S for all cultivations was as expected in the same range and the mean yield was determined as 0.042 ± 0.004 g/g. Hence, added acetate did not reduce biomass formation. With respect to the acetate produced on top of the initial concentration, the reference cultivation reached the lowest values, while for the other cultivations a slight overall increase was detected. In accordance to this slight increase, also the yield Yacetate/X showed this effect with the same significant change in between 0 g/L and 0.5 g/L acetate added. To further illustrate the trend of the activities of both PnarG and PnasD, the data after 36 h and 67 h of cultivation, as well as at CDWmax, are illustrated in Figure 5. In general, the activity decreased during the time course of cultivation within the different acetate concentrations tested. In regard to the promoter activity at CDWmax, a reduction in activity was observed for PnarG and Miller Units decreased from 137 MU for 0 g/L acetate to 35 MU in the cultivation with 10 g/L acetate added. Considering the activity of PnasD, values were as previously reported much higher, and except for 10 g/L the activity at CDWmax was also reduced from 1397 MU for the reference and 815 MU employing 5 g/L. Interestingly, considering the percentage change, the decrease in activity was overall more severe for the nitrate reductase and further studies regarding the negative effect of either acetate directly or for example the onset of nitrate limitation as mentioned before on the reduced activity must be performed.
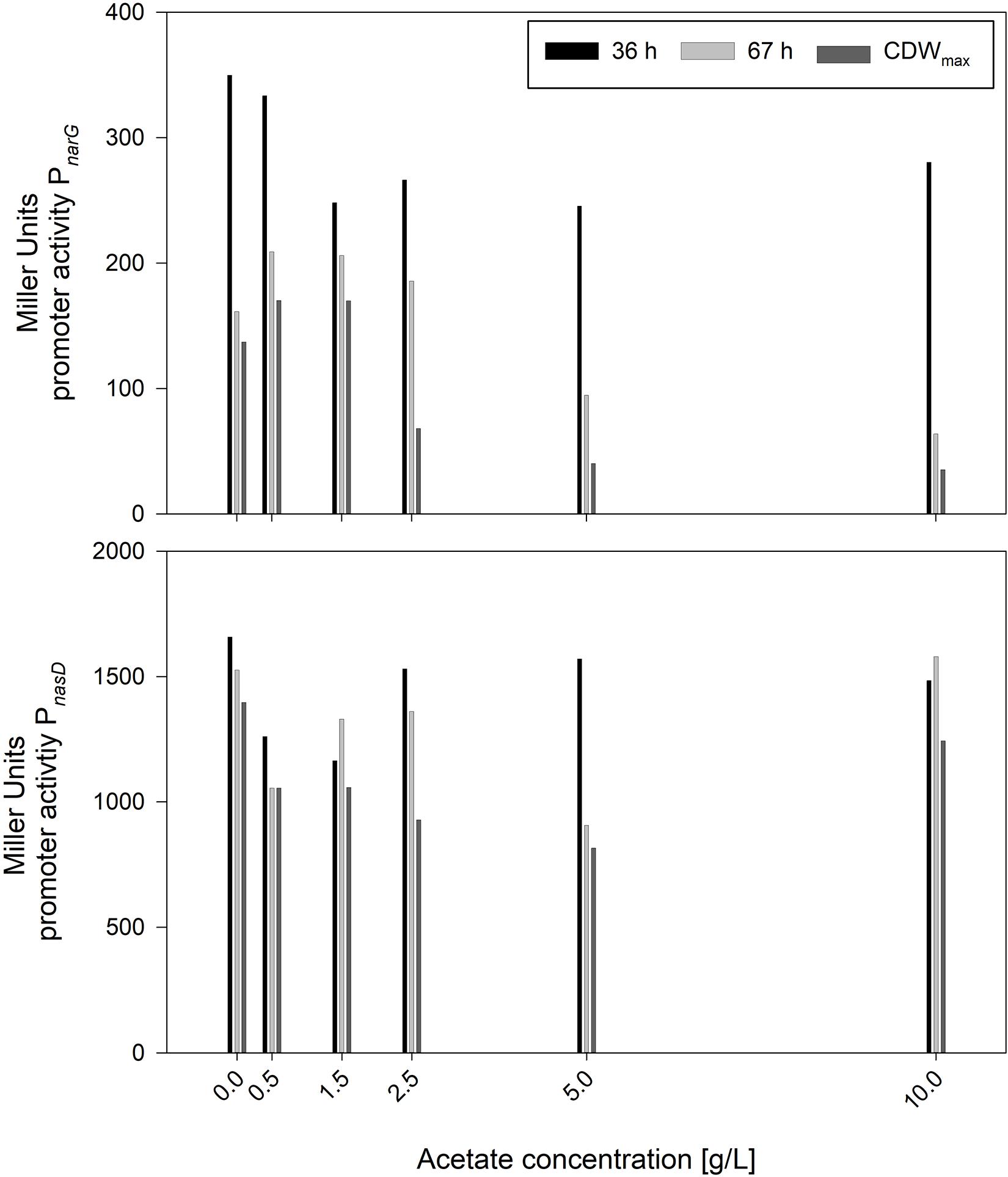
Figure 5. Influence of different acetate concentrations on the expression of PnarG and PnasD after 36 and 67 h of cultivation and at CDWmax employing strains B. subtilis MG1 and MG5.
To sum up, the inhibitory effect of acetate was more pronounced than for ammonium and especially nitrite, as acetate synthesis occurred throughout growth and concentrations did not decrease as shown for nitrite. High acetate concentrations reduced both the overall growth rate as well as the maximum growth rate and also the expression of narG and nasD were shown to be affected. Indeed, it is of upmost interest to further study this effect. These observations are counterintuitive, as acetate formation results in ATP supply, but in the same time its accumulation led to notable growth inhibition. Further studies regarding the role of acetate, e.g., by deleting the genes involved in acetate synthesis, namely acetate kinase ackA and phosphate acetyltransferase pta, displays one option. Regarding this approach, Cruz Ramos et al. (2000) already reported that a B. subtilis Δpta mutant strain produced less acetate in the presence of nitrate, but growth was also drastically reduced, indicating the importance of this metabolic pathway for anaerobic growth by nitrate respiration. Still, strains unable to produce acetate or able to utilize acetate anaerobically might be an interesting alternative for surfactin production processes. For example, a B. subtilis strain carrying the glyoxylate shunt genes from B. licheniformis DSM 13 was reported to be more robust and showed a better growth aerobically (Kabisch et al., 2013). Longing for more robust strains able to grow anaerobically and synthesize surfactin without acetate accumulation clearly presents an opportunity for further strain engineering. Even if growth rates are lower, but productivity is maintained throughout a prolonged time window, a significantly increased product titer could be achieved in a foam-free environment.
Summarizing Remarks and Further Considerations for Future Research
The results presented illustrate the feasibility of an anaerobic nitrate respiration process which also lays the basis for the establishment of other processes where either foaming is a major issue, or the target product is sensitive towards oxygen.
The ability of B. subtilis to grow anaerobically was reported in previous studies. Table 6 summarizes different studies with the respective medium, carbon and nitrogen sources used and OD-values achieved.
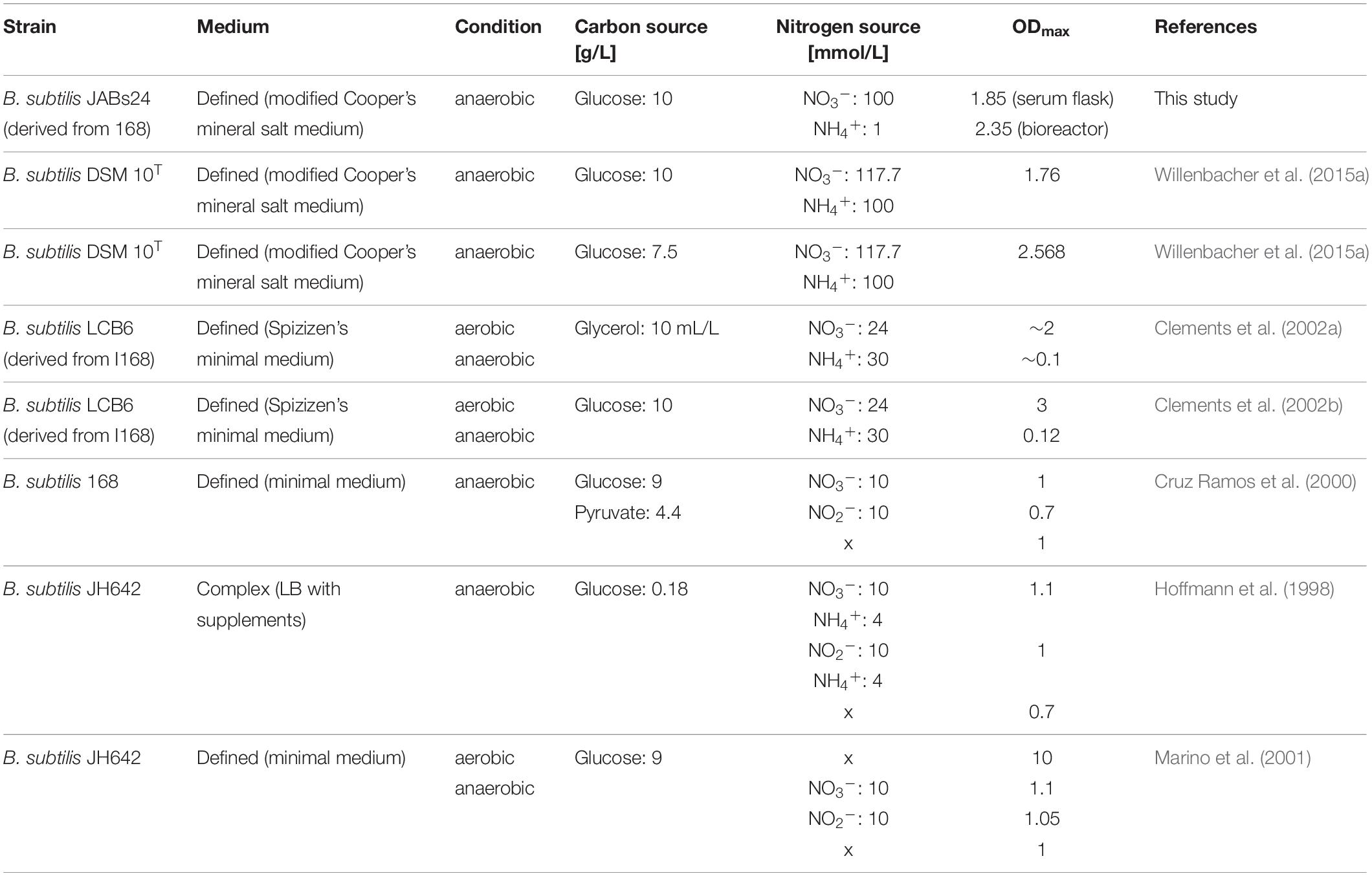
Table 6. Comparison of anaerobic cultivations with B. subtilis in literature with the medium used and OD-values reached.
Next to fundamental research on anaerobic growth, several studies used this approach to synthesize lipopeptides, and in this case surfactin (Javaheri et al., 1985; Davis et al., 1999; Willenbacher et al., 2015a; Geissler et al., 2019b). This process strategy results in promising YP/X values, meaning less biomass waste is produced per gram surfactin, and, which is an important aspect for operating and process control, foaming is completely avoided. This also allows using the full capacity of a bioreactor which leads to an improvement in the volumetric productivity, while the volume in foaming processes is often reduced so that the foam can accumulate in the headspace (St-Pierre Lemieux et al., 2019). Foam-free strategies also make the addition of antifoam agents and the implementation of foam breakers, which results in high energetical input, needless. Furthermore, both techniques to degrade foam can result in cellular stress and consequently reduced productivity (St-Pierre Lemieux et al., 2019). Another advantage of anaerobic cultivations is that the stirrer speed can be kept throughout the process, while pO2 regulation generally goes along with increasing aeration rates and stirrer speeds. Both parameters significantly influence foaming and as the process values increase with increasing biomass, also foam formation is enhanced (St-Pierre Lemieux et al., 2019).
However, several concluding remarks should be mentioned that need to be addressed in further studies, especially as the target product surfactin reached much lower concentrations in the bioreactor cultivations. One issue faced was the pH value. Ideally, also as demonstrated by Willenbacher et al. (2015a), the anaerobic cultivation leads to a basic pH shift. During that cultivation, nitrogen airflow was adjusted above the liquid level, with the aim to reduce the backflow of oxygen from the air. In the current study, the pH shifted to acidic conditions and hence base needed to be added to maintain the pH. In preliminary bioreactor cultivations, different nitrogen flows were tested. Indeed, employing a nitrogen flow through the medium resulted in a decrease in pH, but also resulted in foaming. Employing a nitrogen flow above the liquid level was also not sufficient, which illustrates the difficulties in transferring results from a 1 L bioreactor cultivation as in Willenbacher et al. (2015a) to a 20 kg cultivation as in this study. The decrease in pH was assumed to be both due to the production of acidic products such as acetate, and due to the accumulation of CO2 in the medium which converts to H2CO3 (Killam et al., 2003). Although acetate was not measured by Willenbacher et al. (2015a), it is presumably to assume that acetate was produced due to similarities in e.g. CDW, and hence the effect of the acidic pH shift in the current study is most likely due to accumulated CO2. Nevertheless, as the data for surfactin and CDW reported by Willenbacher et al. (2015a) and Geissler et al. (2019b) are well in accordance to the results obtained in this study, it can be hypothesized that there is no pivotal negative effect when CO2 is not stripped.
The anaerobic growth of B. subtilis is considered an interesting research field and many studies deal with fundamental research on sequencing, cloning or regulatory mechanisms (Cruz Ramos et al., 2000; Clements et al., 2002a, b). Consequently, many efforts are needed to improve the production process to further improve the yields. As summarized by Geissler et al. (2019a), there are three methods to improve the titers, namely medium and process parameter optimization, strain engineering and establishing process strategies. For example, addition of further carbon sources, amino acids or vitamins was reported to improve anaerobic growth (Carvalho et al., 2010; Javed and Baghaei-Yazdi, 2016). In terms of process strategies, appropriate feed profiles must be established to, for example, maintain a certain glucose/nitrate-ratio which influences the synthesis of metabolic by-products such as acetate and lactate (Espinosa-de-los-Monteros et al., 2001). The incorporation of a short aerobic phase displays an interesting option as this resulted in faster growth as reported by Cruz Ramos et al. (2000) and might be beneficial when aerobic pre-cultures are used as performed in this and many other studies. However, on the contrary, Willenbacher et al. (2015a) employed anaerobic pre-cultures and in comparison to the results of Geissler et al. (2019b) no significant differences were observed that could be attributed to the pre-cultures. The third strategy, strain engineering, poses another option to improve the anaerobic growth and the surfactin synthesis of B. subtilis. In this field, promoter exchange or gene knockout studies display a promising approach. As illustrated in the previous results, the synthesis and accumulation of acetate was pointed out as bottleneck and is hence a starting point for future studies.
Conclusion
The present study demonstrated the applicability of anaerobic foam-free processes by nitrate respiration for the synthesis of surfactin in a B. subtilis cultivation. Nevertheless, even at low substrate concentrations, significant production of acetate could be observed. As such, acetate was identified as a target metabolite for ongoing research and strain development. Furthermore, future studies should investigate the reported decrease in the promoter activity PnarG during the time course of cultivation as well as its decrease in the presence of high ammonium and acetate concentrations. Concluding, this study constitutes an important step towards the development of longer, more robust and more productive processes with B. subtilis using anaerobic nitrate respiration.
Data Availability Statement
The original contributions presented in the study are included in the manuscript/Supplementary Material, further inquiries can be directed to the corresponding author.
Author Contributions
MHo planned and executed the experiments, collected data, created the graphs, and drafted the whole manuscript. DF, LS, and KR performed part of the experiments and collected and evaluated corresponding data. SX under supervision of KH constructed the reporter strains. LL supported in interpretation of results. MHe contributed to conception and design of the study and interpretation of the experiments. RH substantially contributed to conception and design of the conducted experiments. All authors read and approved the final version of the manuscript.
Funding
This study was financially supported by the German Research Foundation (DFG), project number 365166982.
Conflict of Interest
The authors declare that the research was conducted in the absence of any commercial or financial relationships that could be construed as a potential conflict of interest.
Acknowledgments
The authors thank Stavroula Natalia Velentza from the Agricultural University of Athens for her support in performing analytics during a short-term research internship. In addition, authors thank Eike Grunwaldt (Department of Bioprocess Engineering, University of Hohenheim) for technical assistance.
Supplementary Material
The Supplementary Material for this article can be found online at: https://www.frontiersin.org/articles/10.3389/fbioe.2020.554903/full#supplementary-material
References
Altenbuchner, J., Viell, P., and Pelletier, I. (1992). Positive selection vectors based on palindromic Dna sequences Methods Enzymol. 216, 457–466. doi: 10.1016/0076-6879(92)16042-I
Carvalho, A. L. U., de Oliveira, F. H. P. C., de Mariano, R., de, L. R., Gouveia, E. R., and Souto-Maior, A. M. (2010). Growth, sporulation and production of bioactive compounds by Bacillus subtilis R14. Braz. Archiv. Biol. Technol. 53, 643–652. doi: 10.1590/S1516-89132010000300020
Chen, C.-Y., Baker, S. C., and Darton, R. C. (2006). Batch production of biosurfactant with foam fractionation. J. Chem. Technol. Biotechnol. 81, 1923–1931. doi: 10.1002/jctb.1625
Chtioui, O., Dimitrov, K., Gancel, F., Dhulster, P., and Nikov, I. (2012). Rotating discs bioreactor, a new tool for lipopeptides production. Process Biochem. 47, 2020–2024. doi: 10.1016/j.procbio.2012.07.013
Clements, L. D., Miller, B. S., and Streips, U. N. (2002a). Comparative growth analysis of the facultative anaerobes Bacillus subtilis, Bacillus licheniformis, and Escherichia coli. Syst. Appl. Microbiol. 25, 284–286. doi: 10.1078/0723-2020-00108
Clements, L. D., Streips, U. N., and Miller, B. S. (2002b). Differential proteomic analysis of Bacillus subtilis nitrate respiration and fermentation in defined medium. Proteomics 2:11.
Cooper, D. G., Macdonald, C. R., Duff, S. J. B., and Kosaric, N. (1981). Enhanced production of surfactin from Bacillus subtilis by continuous product removal and metal cation additions. Appl. Environ. Microbiol. 42, 408–412. doi: 10.1128/AEM.42.3.408-412.1981
Coutte, F., Lecouturier, D., Ait Yahia, S., Leclère, V., Béchet, M., Jacques, P., et al. (2010). Production of surfactin and fengycin by Bacillus subtilis in a bubbleless membrane bioreactor. Appl. Microbiolo. Biotechnol. 87, 499–507. doi: 10.1007/s00253-010-2504-2508
Coutte, F., Lecouturier, D., Dimitrov, K., Guez, J.-S., Delvigne, F., Dhulster, P., et al. (2017). Microbial lipopeptide production and purification bioprocesses, current progress and future challenges. Biotechnol. J. 12:1600566. doi: 10.1002/biot.201600566
Cruz Ramos, H., Boursier, L., Moszer, I., Kunst, F., Danchin, A., and Glaser, P. (1995). Anaerobic transcription activation in Bacillus subtilis: identification of distinct FNR-dependent and -independent regulatory mechanisms. EMBO J. 14, 5984–5994. doi: 10.1002/j.1460-2075.1995.tb00287.x
Cruz Ramos, H., Hoffmann, T., Marino, M., Nedjari, H., Presecan-Siedel, E., Dreesen, O., et al. (2000). Fermentative metabolism of Bacillus subtilis: physiology and regulation of gene expression. J. Bacteriol. 182, 3072–3080. doi: 10.1128/JB.182.11.3072-3080.2000
Davis, D. A., Lynch, H. C., and Varley, J. (1999). The production of Surfactin in batch culture by Bacillus subtilis ATCC 21332 is strongly influenced by the conditions of nitrogen metabolism. Enzyme Microb. Technol. 25, 322–329. doi: 10.1016/S0141-0229(99)00048-4
Davis, D. A., Lynch, H. C., and Varley, J. (2001). The application of foaming for the recovery of surfactin from B. subtilis ATCC 21332 cultures. Enzyme Microb. Technol. 28, 346–354. doi: 10.1016/S0141-0229(00)00327-6
Espinosa-de-los-Monteros, J., Martinez, A., and Valle, F. (2001). Metabolic profiles and aprE expression in anaerobic cultures of Bacillus subtilis using nitrate as terminal electron acceptor. Appl. Microbiol. Biotechnol. 57, 379–384. doi: 10.1007/s002530100749
Geissler, M., Heravi, K. M., Henkel, M., and Hausmann, R. (2019a). “Lipopeptide biosurfactants from Bacillus Species,” in Biobased Surfactants, eds D. G. Hayes, D. K. Y. Solaiman, and R. D. Ashby (Amsterdam: Elsevier), 205–240. doi: 10.1016/B978-0-12-812705-6.00006-X
Geissler, M., Kühle, I., Morabbi Heravi, K., Altenbuchner, J., Henkel, M., and Hausmann, R. (2019b). Evaluation of surfactin synthesis in a genome reduced Bacillus subtilis strain. AMB Express 9:84. doi: 10.1186/s13568-019-0806-5
Geissler, M., Oellig, C., Moss, K., Schwack, W., Henkel, M., and Hausmann, R. (2017). High-performance thin-layer chromatography (HPTLC) for the simultaneous quantification of the cyclic lipopeptides Surfactin, Iturin A and Fengycin in culture samples of Bacillus species. J. Chromatogr. B 1044–1045, 214–224. doi: 10.1016/j.jchromb.2016.11.013
Härtig, E., and Jahn, D. (2012). “Regulation of the anaerobic metabolism in Bacillus subtilis. Adv. Microb. Physiol. 61, 195–216. doi: 10.1016/B978-0-12-394423-8.00005-6
Henkel, M., Geissler, M., Weggenmann, F., and Hausmann, R. (2017). Production of microbial biosurfactants: status quo of rhamnolipid and surfactin towards large-scale production. Biotechnol. J. 12:1600561. doi: 10.1002/biot.201600561
Hoffmann, T., Frankenberg, N., Marino, M., and Jahn, D. (1998). Ammonification in Bacillus subtilis utilizing dissimilatory nitrite reductase is dependent onresDE. J. Bacteriol. 180, 186–189. doi: 10.1128/JB.180.1.186-189.1998
Javaheri, M., Jenneman, G. E., McInerney, M. J., and Knapp, R. M. (1985). Anaerobic production of a biosurfactant by Bacillus licheniformis JF-2. Appl. Environ. Microbiol. 50, 698–700. doi: 10.1128/AEM.50.3.698-700.1985
Javed, M., and Baghaei-Yazdi, N. (2016). Nutritional optimization for anaerobic growth of Bacillus steaothermophilus LLD-16. J. Rad. Res. Appl. Sci. 9, 170–179. doi: 10.1016/j.jrras.2015.12.007
Kabisch, J., Pratzka, I., Meyer, H., Albrecht, D., Lalk, M., Ehrenreich, A., et al. (2013). Metabolic engineering of Bacillus subtilis for growth on overflow metabolites. Microb. Cell Factor. 12:72. doi: 10.1186/1475-2859-12-72
Killam, R. L., Barenfanger, J., and Cox, M. (2003). Influence of lack of CO2 on anaerobic bacteria: a quick method to verify the absence of CO2. J. Clin. Microbiol. 41, 2201–2202. doi: 10.1128/JCM.41.5.2201-2002.2003
Lasko, D. R., Zamboni, N., and Sauer, U. (2000). Bacterial response to acetate challenge: a comparison of tolerance among species. Appl. Microbiol. Biotechnol. 54, 243–247. doi: 10.1007/s002530000339
Leejeerajumnean, A., Ames, J. M., and Owens, J. D. (2000). Effect of ammonia on the growth of Bacillus species and some other bacteria. Lett. Appl. Microbiol. 30, 385–389. doi: 10.1046/j.1472-765x.2000.00734.x
Li, Y., Héloir, M., Zhang, X., Geissler, M., Trouvelot, S., Jacquens, L., et al. (2019). Surfactin and fengycin contribute to the protection of a Bacillus subtilis strain against grape downy mildew by both direct effect and defence stimulation. Mol. Plant Pathol. 20, 1037–1050. doi: 10.1111/mpp.12809
Marino, M., Ramos, H. C., Hoffmann, T., Glaser, P., and Jahn, D. (2001). Modulation of anaerobic energy metabolism of Bacillus subtilis by arfM (ywiD). J. Bacteriol. 183, 6815–6821. doi: 10.1128/JB.183.23.6815-6821.2001
Morabbi Heravi, K., and Altenbuchner, J. (2018). Cross talk among transporters of the phosphoenolpyruvate-dependent phosphotransferase system in Bacillus subtilis. J. Bacteriol. 200, e213–e218. doi: 10.1128/JB.00213-18
Müller, T., Walter, B., Wirtz, A., and Burkovski, A. (2006). Ammonium toxicity in bacteria. Curr. Microbiol. 52, 400–406. doi: 10.1007/s00284-005-0370-x
Nakano, M. M., Hoffmann, T., Zhu, Y., and Jahn, D. (1998a). Nitrogen and oxygen regulation of Bacillus subtilis nasDEF Encoding NADH-dependent nitrite reductase by TnrA and ResDE. J. Bacteriol. 180, 5344–5350. doi: 10.1128/JB.180.20.5344-5350.1998
Nakano, M. M., Zuber, P., and Sonenshein, A. L. (1998b). Anaerobic regulation of Bacillus subtilis krebs cycle genes. J. Bacteriol. 180, 3304–3311. doi: 10.1128/JB.180.13.3304-3311.1998
Ongena, M., and Jacques, P. (2008). Bacillus lipopeptides: versatile weapons for plant disease biocontrol. Trends Microbiol. 16, 115–125. doi: 10.1016/j.tim.2007.12.009
Pinhal, S., Ropers, D., Geiselmann, J., and de Jong, H. (2019). Acetate metabolism and the inhibition of bacterial growth by acetate. J. Bacteriol. 201:e00147-19. doi: 10.1128/JB.00147-19
Presecan-Siedel, E., Galinier, A., Longin, R., Deutscher, J., Danchin, A., Glaser, P., et al. (1999). Catabolite regulation of the pta gene as part of carbon flow pathways in Bacillus subtilis. J. Bacteriol. 181, 6889–6897. doi: 10.1128/JB.181.22.6889-6897.1999
Rangarajan, V., and Clarke, K. G. (2015). Process development and intensification for enhanced production of Bacillus lipopeptides. Biotechnol. Genet. Eng. Rev. 31, 46–68. doi: 10.1080/02648725.2016.1166335
Reents, H., Munch, R., Dammeyer, T., Jahn, D., and Hartig, E. (2006). The Fnr regulon of Bacillus subtilis. J. Bacteriol. 188, 1103–1112. doi: 10.1128/JB.188.3.1103-1112.2006
St-Pierre Lemieux, G., Groleau, D., and Proulx, P. (2019). Introduction on foam and its impact in bioreactors. Can. J. Biotechnol. 3, 143–157. doi: 10.24870/cjb.2019-000131
Strohm, T. O., Griffin, B., Zumft, W. G., and Schink, B. (2007). Growth yields in bacterial denitrification and nitrate ammonification. Appl. Environ. Microbiol. 73, 1420–1424. doi: 10.1128/AEM.02508-06
Willenbacher, J., Rau, J.-T., Rogalla, J., Syldatk, C., and Hausmann, R. (2015a). Foam-free production of surfactin via anaerobic fermentation of Bacillus subtilis DSM 10T. AMB Express 5:21. doi: 10.1186/s13568-015-0107-6
Willenbacher, J., Yeremchuk, W., Mohr, T., Syldatk, C., and Hausmann, R. (2015b). Enhancement of surfactin yield by improving the medium composition and fermentation process. AMB Express 5:145. doi: 10.1186/s13568-015-0145-0
Willenbacher, J., Zwick, M., Mohr, T., Schmid, F., Syldatk, C., and Hausmann, R. (2014). Evaluation of different Bacillus strains in respect of their ability to produce Surfactin in a model fermentation process with integrated foam fractionation. Appl. Microbiol. Biotechnol. 98, 9623–9632. doi: 10.1007/s00253-014-6010-2
Keywords: Bacillus subtilis, anaerobic cultivation, lipopeptide, surfactin, process control strategy, nitrate respiration, acetate, foam-free
Citation: Hoffmann M, Fernandez Cano Luna DS, Xiao S, Stegemüller, Rief K, Heravi KM, Lilge L, Henkel M and Hausmann R (2020) Towards the Anaerobic Production of Surfactin Using Bacillus subtilis. Front. Bioeng. Biotechnol. 8:554903. doi: 10.3389/fbioe.2020.554903
Received: 23 April 2020; Accepted: 19 October 2020;
Published: 26 November 2020.
Edited by:
Susana Rodriguez-Couto, Independent Researcher, Vigo, SpainReviewed by:
Vijay Kumar Garlapati, Jaypee University of Information Technology, IndiaFrancois Coutte, Université de Lille, France
Copyright © 2020 Hoffmann, Fernandez Cano Luna, Xiao, Stegemüller, Rief, Heravi, Lilge, Henkel and Hausmann. This is an open-access article distributed under the terms of the Creative Commons Attribution License (CC BY). The use, distribution or reproduction in other forums is permitted, provided the original author(s) and the copyright owner(s) are credited and that the original publication in this journal is cited, in accordance with accepted academic practice. No use, distribution or reproduction is permitted which does not comply with these terms.
*Correspondence: Marius Henkel, Marius.henkel@uni-hohenheim.de